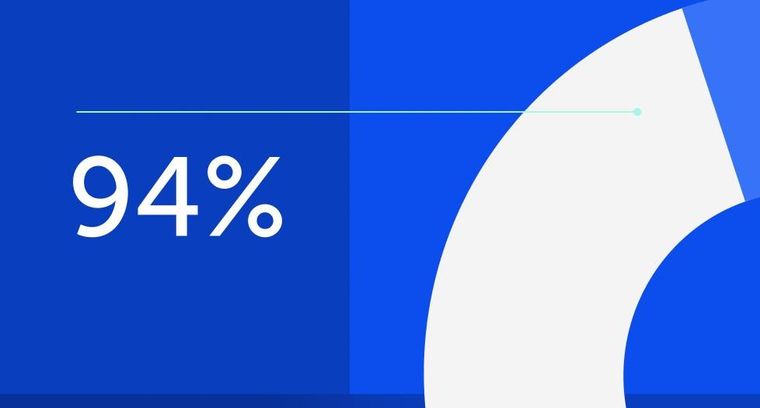
94% of researchers rate our articles as excellent or good
Learn more about the work of our research integrity team to safeguard the quality of each article we publish.
Find out more
ORIGINAL RESEARCH article
Front. Neurosci., 14 June 2024
Sec. Autonomic Neuroscience
Volume 18 - 2024 | https://doi.org/10.3389/fnins.2024.1427384
The nucleus tractus solitarii (NTS) plays a critical role in the homeostatic regulation of respiration, blood pressure, sodium consumption and metabolic processes. Despite their significance, the circuitry mechanisms facilitating these diverse physiological functions remain incompletely understood. In this study, we present a whole-brain mapping of both the afferent and efferent connections of Phox2b-expressing and GABAergic neurons within the NTS. Our findings reveal that these neuronal populations not only receive monosynaptic inputs primarily from the medulla oblongata, pons, midbrain, supra-midbrain and cortical areas, but also mutually project their axons to these same locales. Moreover, intense monosynaptic inputs are received from the central amygdala, the paraventricular nucleus of the hypothalamus, the parasubthalamic nucleus and the intermediate reticular nucleus, along with brainstem nuclei explicitly engaged in respiratory regulation. In contrast, both neuronal groups extensively innervate brainstem nuclei associated with respiratory functions, although their projections to regions above the midbrain are comparatively limited. These anatomical findings provide a foundational platform for delineating an anatomical framework essential for dissecting the specific functional mechanisms of these circuits.
The nucleus tractus solitarii (NTS) plays an important role in regulation of numerous physiological processes, such as homeostatic control of breathing (Fu et al., 2017, 2019; Yu et al., 2022; Wang et al., 2024), blood pressure (Jun et al., 2023), energy balance (Cheng et al., 2020), inflammation-induced anxiety (Brunet and Pattyn, 2002; Zhao et al., 2024), sodium appetite (Geerling et al., 2006; Resch et al., 2017) and nausea-associated behaviors (Zhang et al., 2020). These differential processes require to recruit different subpopulation of NTS neurons and the associated distinct neural circuits. For instances, cholecystokinin and dopamine β-hydroxylase (DβH) neurons in the NTS directly contribute to regulation of anorexia (Roman et al., 2016); NTS catecholaminergic neurons mediate hypoglycemic hunger via medial hypothalamic feeding pathways (Aklan et al., 2020); Calcitonin receptor-residing NTS neurons mediate food intake suppression (Cheng et al., 2020). Paired-like homeobox 2b (Phox2b), as a transcription factor, is expressed in and essential for the development of the reflex circuits that control bodily homeostasis by regulating cardiovascular, respiratory and digestive functions (Brunet and Pattyn, 2002; Dubreuil et al., 2008). Recently, we demonstrate that a subset of Phox2b-expressing NTS (NTSPhox2b) neurons serves as central respiratory chemoreceptors to maintain ventilatory homeostasis, gaining better understanding of neural control of breathing by this subpopulation. Moreover, activation of both leptin receptor b and orexin 1 receptor-expressing NTS neurons facilitates breathing (Yu et al., 2022; Wang et al., 2024), further highlighting molecular identify.
To unveil the function-specific neural circuits, it is necessary to determine the afferent and efferent projections of NTS neurons. It is well-established that glutamatergic, catecholaminergic and GABAergic neurons, accounting for the majority of subtypes in the NTS, have been observed to regulate multiple physiological functions. To date, a few studies have demonstrated neuroanatomical connections of NTS neurons with other brain regions. Neural tracing data from Loewy laboratory revealed anatomical and functional connections of the enzyme 11-beta-hydroxysteroid dehydrogenase type 2-expressing NTS neurons (Geerling and Loewy, 2006; Resch et al., 2017; Gasparini et al., 2019). Subsequently, Gasparini and colleagues utilized an axonal tracer (cholera toxin b) to provide a brain-wide map of neurons that extend their axons to the caudal NTS (Gasparini et al., 2020). Recently, we characterized whole-brain monosynaptic inputs and outputs of leptin receptor b-expressing neurons within the NTS (Sun et al., 2023), providing a neuroanatomical basis for further research on physiological and pathological functions of these neurons. NTSPhox2b neurons represent the majority of excitatory neurons, including glutamatergic and catecholaminergic, whereas Phox2b was almost absent in GABAergic NTS (NTSGABA) neurons (Kang et al., 2007). GABAergic neurons are abundant in the NTS, with a characteristic of both short- and long-range projections (Shi et al., 2021). Nevertheless, studies on connections linking both NTSPhox2b and NTSGABA neurons with other brain regions are required to provide a comprehensive understanding of function-specific neural circuits of these neurons.
In the present studies, we employed transsynaptic retrograde rabies virus and common anterograde virus-based tracing strategies to provide a detailed whole-brain mapping of afferent and efferent projections of both NTSPhox2b and NTSGABA neurons. Building on previous neural tracing evidence, we extended the investigation to concurrently visualize efferent pattern of both excitatory and inhibitory NTS neurons from Phox2b-Flop::Vgat-Cre mice. These data provide a foundational anatomical framework for future investigations into the interregional pathways that regulate NTS functions.
Adult Phox2b-Cre (IMSR Cat# JAX: 016223), Phox2b-Flpo (IMSR Cat# JAX: 022407) and Vgat-Cre (IMSR Cat# JAX: 016962) mice of either sex used in the experiments were purchased from the Jackson Laboratory. Phox2b-Cre (Scott et al., 2011) and Phox2b-Flpo (Hirsch et al., 2013) mice have Cre or Flpo recombinase expression under control of the Phox2b promoter/enhancer regions within the BAC transgenic mouse line. Phox2b-Flpo::Vgat-Cre mice were generated by crossing Vgat-Cre mice with Phox2b-Flpo mice. Mice were housed under program-controlled temperature (23 ± 1°C) and humidity (50% ± 10%) with a fixed 12 h light/12 h dark cycle and with ad libitum access to food and water. All experiments were performed in accordance with the Guide for the Care and Use of Laboratory Animals, and were approved by the Animal Care and Ethics Committee of Hebei Medical University.
AAV2/9-EF1α-DIO-EGFP-T2A-TVA (2.0 × 1012 genome copies/mL), AAV-EF1α-DIO-N2cG (2.0 × 1012 genome copies/mL) and RV-EnvA-∆G-tdTomato (1.0 × 108 genome copies/mL) were purchased from BrainCase (Wuhan, China). The two Cre-dependent AAVs were mixed at a 1:1 ratio as helper viruses for retrograde monosynaptic-tracing experiments. AAV2/9-EF1a-DIO-EGFP (5.04 × 1012 genome copies/mL) and AAV2/9-EF1a-fDIO-mCherry (6.0 × 1012 genome copies/mL) were used for anterograde tracing experiments and was packaged by BrainVTA (Wuhan, China).
Surgical procedures were conducted in accordance with methodologies outlined in prior studies (Sun et al., 2023). Adult mice underwent anesthesia induced by intraperitoneal administration of pentobarbital sodium at a dosage of 60 μg/g. Anesthetic depth was monitored by the absence of corneal and hindpaw withdrawal reflexes, with supplemental anesthetic provided as needed, amounting to 30% of the initial dosage. All procedures were performed under sterile conditions. Post-anesthesia, the mice were positioned prone in a stereotaxic frame (RWD Life Science Co., Ltd., Shenzhen, China) and body temperature was maintained at 37°C using a heating pad and a blanket. Viral vectors were delivered precisely to the NTS at the level of the calamus scriptorius (anterior–posterior (AP): + 0.2 mm, medial-lateral (ML): ± 0.3 mm; dorsal-ventral (DV): − 0.2 mm; AP: + 0.3 mm, ML: ± 0.4 mm, DV: − 0.3 mm) using a glass micropipette. For retrograde tracing, injections of helper viruses (100 nL per injection) were administered unilaterally into the NTS and were allowed to diffuse for 10 min. T Following a two-week interval, RV-EnvA-∆G-tdTomato (160 nL) was injected into the same site. Mice were perfused for immunostaining 1 week later.
In the case of anterograde tracing, we unilaterally injected AAV-EF1α-DIO-EYFP into the NTS of Phox2b-Flpo::Vgat-Cre mice (two injections, 80 nL per injection). After 2 weeks, a second series of injections involving AAV-EF1α-fDIO-mCherry (80 nL per injection) were administrated at the same locations in the same subjects. Post-injection, the mice were treated with the antibiotic ampicillin (125 mg/kg, i.p.) and the analgesic ketorolac (4 mg/kg, i.p.). A 4-week recuperation period was observed before conducting histological analyses.
Mice were subjected to deep anesthesia using urethane (1.8 g/kg, intraperitoneally) and subsequently perfused transcardially with saline, followed by fixation with 4% paraformaldehyde in phosphate-buffered saline (PBS). Post-fixation, brains were excised on ice and preserved in 4% paraformaldehyde at 4°C for 12 h, after which they were transferred into 30% sucrose solution buffered with PBS for cryoprotection. Coronal sections (25 μm) were harvested on a freezing microtome (CM1950; Leica Microsystems, Germany). For immunohistochemistry, sections were initially blocked using 5% bovine serum albumin in PBS containing 0.25% Triton X-100 for 30 min at room temperature. This step was followed by overnight incubation at 4°C with primary antibodies diluted in PBS supplemented with 2% bovine serum albumin. Sections were subsequently washed thrice in PBS for 5 min each and incubated with fluorescently-labeled secondary antibodies for 2 h at room temperature. All immersion steps and incubations were conducted on a shaker set to a gentle speed. After a final series of washes in PBS, sections were mounted on glass slides using Vectashield Antifade Mounting Medium (Vector Laboratories, Burlingame, CA, United States) to enhance fluorescence retention and prevent photobleaching during microscopic examination.
The primary antibodies used were as follows: chicken anti-EGFP (dilution 1:1000, Abcam, catalog# ab13970, RRID: AB_300798), rabbit anti-mCherry (dilution 1:1000, Novus Biologicals, catalog# NBP2-25157, RRID: AB_2753204). The fluorophore-conjugated secondary antibodies used were: Alexa Fluor® 488 Goat Anti-Chicken IgY (H + L) (dilution 1:1000, Abcam, catalog# ab150169, RRID: AB_2636803), Cy™3 AffiniPure™ Goat anti-Rabbit IgG (H + L) (dilution 1:1000, Jackson ImmunoResearch Laboratories, catalog# 111-165-003, RRID: AB_2338000).
Images of whole-brain sections were acquired using a laser scanning confocal microscope with either 10x or 20x objectives, and detailed magnification was achieved through the employment of a 20x objective on a Zeiss microscope (LSM 900; Carl Zeiss, Jena, Germany). The delimitation of subregional boundaries was referenced against the Mouse Brain Atlas (Paxinos and Franklin, 2013). Quantitative analysis involved the manual enumeration of tdTomato-expressing neurons within distinct nuclei. The relative prevalence of input neurons across 86 brain regions was determined by calculating the ratio of afferent cells in each nucleus to the total population of tdTomato-marked cells, excluding those at the injection sites. Neuronal distributions across each brain region accounted for at least 0.05% of the total monosynaptic inputs were specifically noted.
For the quantification of whole-brain efferent projections emanating from NTSPhox2b and NTSGABA neurons, analyses focused on the proportion of axonal projections, computed as the ratio between the average count of axonal varicosities and the total number of varicosities observed in whole-brain projections. Further, axonal varicosities were documented using a 20x objective on the Zeiss confocal system, and the enumeration of these structures across the brain was performed semi-automatically using a particle analyzing plugin in ImageJ software. Results and statistical analyses were restricted to data derived exclusively from mice with accurately targeted injection sites, as discerned in the accompanying figures.
Statistical analyses were performed using the Prism software version 9 (GraphPad, La Jolla, CA). All data are presented as the mean ± SEM (standard error of the mean).
To identify the whole-brain monosynaptic inputs onto NTSPhox2b and NTSGABA neurons, we utilized trans-synaptic retrograde labeling with a modified rabies virus as depicted before (Wall et al., 2010; Osakada and Callaway, 2013). This genetic approach entails two stages. Initially, the Cre-dependent helper viruses, including AAV-EF1α-DIO-EGFP-T2A-TVA and AAV-EF1α-DIO-N2cG, were unilaterally injected into the NTS from Phox2b-Cre (n = 3 mice) and Vgat-Cre mice (n = 3 mice), respectively. After 2 weeks, the modified rabies virus RV-EnvA-∆G-tdTomato was administered at the same injection site, targeting selectively infected TVA-expressing cells and transporting retrogradely into presynaptic cells. The mice were perfused 1 week after rabies virus injection, ensuring enough time for the rabies virus to retrogradely infect and express tdTomato sufficiently in the input neurons (Figures 1A,B). Since the rabies virus cannot infect cells in the absence of the TVA receptor, infection is limited to Cre+ cells expressing the helper AAV. Starter neurons was identified based on co-expression of AAV helper tag (EGFP) and RV-tdTomato around the injection site, and the input neurons were detected by the expression of tdTomato that directly input to NTSPhox2b and NTSGABA neurons. Through mapping the starter neurons in the NTS across three mouse coronal sections, we observed that they were primarily distributed in rostrocaudal position spanning from bregma −7.6 mm to −7.2 mm. In addition, we identified tdTomato-positive neurons in the NTS that do not express EGFP, indicating the presence of direct monosynaptic inputs to the NTS neurons (Figures 1C–H). However, using the same virus strategy in wild-type mice, we detected no neurons expressing EGFP or tdTomato in the NTS (n = 3, data not shown), demonstrating the reliability of this technique. Here we did not check peripherally monosynaptic inputs to both population of NTS neurons.
Figure 1. Experimental strategy to target NTSPhox2b and NTSGABA Neurons. (A) Schematic of viral vectors for RV-based trans-synaptic retrograde tracing, including helper viruses with Cre-dependent expression of TVA receptor (AAV-EF1α-DIO-EGFP-T2A-TVA) and N2cG (AAV-EF1α-DIO-N2cG). The RV was genetically modified by pseudotyping with EnvA (CVS-EnvA-∆G-tdTomato). (B) Schematic of viral injection strategy and experimental procedure performed in Phox2b-Cre and Vgat-Cre mice. (C–H) Photomicrograph showing EGFP-expressing NTSPhox2b (left) and NTSGABA (right) neurons (green), tdTomato-expressing input neurons (red) and starter neurons (yellow). In each panel, the bottom images are the enlarged view of the yellow boxed region in the top images. Bottom images (from left to right): EGFP, tdTomato, DAPI and composite merge. Scale bars: 100 μm (top images in each panel), 20 μm (bottom images in bottom panel).
To unravel the monosynaptic inputs to NTSPhox2b throughout the entire brain, we examined these input neurons in coronal sections from Phox2b-Cre mice based on the above neural tracing strategy. The neurons exclusively labeled with tdTomato were identified as the monosynaptic inputs to the NTSPhox2b neurons. Examination of representative sections demonstrated that tdTomato-positive neurons were distributed in many brain regions, encompassing the cortex, supra-midbrain, midbrain, pons, medulla oblongata and cerebellum (Figure 2). Using the same strategy in Vgat-Cre mice, tdTomato-labeled neurons were detected in almost the same regions as identified in Phox2b-Cre mice (Figure 3).
Figure 2. Representative images of monosynaptic inputs to NTSPhox2b neurons. Input neurons of NTSPhox2b neurons were identified by expression of the tdTomato. Each brain region containing these input neurons were annotated in accordance with the Mouse Brain Atlas for precise localization and identification. Scale bars, 200 μm. Cu, cuneate nucleus; MdD, medullary reticular nucleus, dorsal part; IRt, intermediate reticular nucleus; LRt, lateral reticular nucleus; AP, area postrema; DMNV, dorsal nucleus of vagus nerve; Amb, ambiguus nucleus; RVRG, rostral ventral respiratory group; CVLM, caudal ventrolateral medulla; PCRt, parvicellular reticular nucleus; preBötC, pre-Botzinger complex; Gi, gigantocellular reticular nucleus; LPGi, lateral paragigantocellular nucleus; RTN, retrotrapezoid nucleus; BötC, Bötzinger complex; RVLM, rostral ventrolateral medulla; Med, medial cerebellar nucleus; RMg, raphe magnus nucleus; GiA, gigantocellular reticular nucleus, alpha; RPa, raphe pallidus nucleus; 7 N, facial nucleus; Pr, prepositus nucleus; MVeMC, medial vestibular nucleus, magnocellular; DMSp5, dorsomedial spinal trigeminal nucleus; LC, locus coeruleus; Bar, Barrington’s nucleus; PCRtA, parvicellular reticular nucleus, alpha part; VLPAG, ventrolateral periaqueductal gray; LPBN, lateral parabrachial nucleus; Pr5DM, principal sensory trigeminal nucleus, dorsomedial part; LDTg, laterodorsal tegmental nucleus; MPBN, medial parabrachial nucleus; Su5, supratrigeminal nucleus; SubCV, subcoeruleus nucleus, ventral part; KF, Kolliker-Fuse nucleus; LDTgV, laterodorsal tegmental nucleus, ventral part; mRt, mesencephalic reticular formation; PSTh, parasubthalamic nucleus; CeA, central amygdaloid nucleus; LH, lateral hypothalamic area; PVN, paraventricular hypothalamic nucleus; S2, secondary somatosensory cortex; DI, dysgranular insular cortex; BNST, bed nucleus of the stria terminalis.
Figure 3. Typical images of monosynaptic inputs to NTSGABA neurons. Input neurons of NTSGABA neurons were characterized based on the expression of tdTomato. The brain regions housing these identified input neurons were denoted in accordance with the Mouse Brain Atlas for precise localization and identification. Scale bars, 200 μm. Sp5C, spinal trigeminal nucleus, caudal part; Cu, cuneate nucleus; MdD, medullary reticular nucleus, dorsal part; MdV, medullary reticular nucleus, ventral part; IRt, intermediate reticular nucleus; LRt, lateral reticular nucleus; AP, area postrema; DMNV, dorsal nucleus of vagus nerve; Amb, ambiguus nucleus; RVRG, rostral ventral respiratory group; CVLM, caudal ventrolateral medulla; PCRt, parvicellular reticular nucleus; Mx, matrix region of the medulla; Sp5I, spinal trigeminal nucleus, interpolar part; preBötC, pre-Bötzinger complex; SpVe, spinal vestibular nucleus; Gi, gigantocellular reticular nucleus; LPGi, lateral paragigantocellular nucleus; Pr, prepositus nucleus; GiV, gigantocellular reticular nucleus, ventral part; RTN, retrotrapezoid nucleus; BötC, Bötzinger complex; RVLM, rostral ventrolateral medulla; RPa, raphe pallidus nucleus; Med, medial cerebellar nucleus; MVePC, medial vestibular nucleus, parvicellular; DMSp5, dorsomedial spinal trigeminal nucleus; RMg, raphe magnus nucleus; GiA, gigantocellular reticular nucleus, alpha; RPa, raphe pallidus nucleus; 7 N, facial nucleus; Pr, prepositus nucleus; MVeMC, medial vestibular nucleus, magnocellular; DMSp5, dorsomedial spinal trigeminal nucleus; LC, locus coeruleus; Bar, Barrington’s nucleus; PCRtA, parvicellular reticular nucleus, alpha part; VLPAG, ventrolateral periaqueductal gray; LPBN, lateral parabrachial nucleus; Pr5DM, principal sensory trigeminal nucleus, dorsomedial part; LDTg, laterodorsal tegmental nucleus; MPBN, medial parabrachial nucleus; Su5, supratrigeminal nucleus; PnV, pontine reticular nucleus, ventral part; SubCV, subcoeruleus nucleus, ventral part; KF, Kolliker-Fuse nucleus; LDTgV, laterodorsal tegmental nucleus, ventral part; mRt, mesencephalic reticular formation; CnF, cuneiform nucleus; PrEW, pre-Edinger-Westphal nucleus; RPC, red nucleus, parvicellular part; RMC, red nucleus, magnocellular part; PSTh, parasubthalamic nucleus; S1, primary somatosensory cortex; M1, primary motor cortex; M2, secondary motor cortex; CeA, central amygdaloid nucleus; LH, lateral hypothalamic area; PVN, paraventricular hypothalamic nucleus; S2, secondary somatosensory cortex; DI, dysgranular insular cortex; GI, granular insular cortex; BNST, bed nucleus of the stria terminalis.
To provide a comprehensive visualization of the whole-brain distribution pattern of tdTomato-labeled input neurons to NTSPhox2b (Figure 4) and NTSGABA neurons (Figure 5), respectively, we selectively enlarged representative coronal images from the following crucial afferent nuclei, including the intermediate reticular nucleus (IRt), caudal ventrolateral medulla (CVLM), preBötzinger complex (preBötC), Bötzinger complex (BötC), lateral paragigantocellular nucleus (LPGi), rostral ventrolateral medulla (RVLM), ambiguus nucleus (Amb), locus coeruleus (LC), lateral parabrachial nucleus (LPBN), gigantocellular reticular nucleus (Gi), raphe magnus nucleus (RMg), parasubthalamic nucleus (PSTh), central amygdala (CeA), paraventricular hypothalamic nucleus (PVN) and lateral hypothalamic area (LH).
Figure 4. Photomicrographs of brain regions displaying dense monosynaptic inputs to NTSPhox2b neurons. A larger number of input neurons targeting NTSPhox2b neurons was observed in many brain regions. Scale bars, 100 μm.
Figure 5. Photomicrographs of brain regions exhibiting a high density of monosynaptic inputs targeting NTSGABA neurons. A larger number of input neurons innervating NTSGABA neurons was found in many brain regions. Scale bars, 100 μm.
Additionally, these representative observations indicated that tdTomato-positive neurons were predominantly localized in the ipsilateral brain area, with a minority dispersed in the contralateral regions. This parallel input pattern suggests that the same upstream brain regions may target both excitatory NTSPhox2b and inhibitory NTSGABA neurons, presumably providing homeostatic regulation of distinct physiological functions.
To quantify the proportion of monosynaptic input neurons that target both NTSPhox2b and NTSGABA neurons, we made a statistical analysis by calculating the ratio of the number of tdTomato-labeled afferent neurons originating from each nucleus and the total number of labeled neurons in the whole brain (Figure 6, n = 3). The brains were divided into six structures (n = 86 nuclei): cortex, supra-midbrain, midbrain, pons, medulla oblongata and cerebellum. The tdTomato-labeled neurons were counted in each brain region, with each comprising >0.05% of the total labeled neurons.
Figure 6. Quantitative analysis of input neurons innervating the NTSPhox2b neurons and NTSGABA neurons. The relative abundance of input neurons pertinent to NTSPhox2b (A) and NTSGABA (B) neuron populations across distinct brain nuclei was quantified by determining the ratio of tdTomato-tagged afferent neurons within each nucleus relative to the overall neuronal count.
As shown in Figure 6, our findings revealed that the medulla oblongata accounted for the highest quantity of inputs to the NTS, with the greatest number of inputs originating from the IRt (10.52 ± 3.07% for NTSPhox2b, 7.77 ± 0.88% for NTSGABA). Additionally, the CeA (6.79 ± 0.95% for NTSPhox2b, 4.66 ± 0.62% for NTSGABA), PVN (3.96 ± 1.64% for NTSPhox2b, 4.47 ± 0.90% for NTSGABA) and PSTh (3.13 ± 0.96% for NTSPhox2, 4.59 ± 1.21% for NTSGABA) in the supra-midbrain regions displayed heavy projections to the NTS neurons. The ventrolateral periaqueductal gray (VLPAG, 0.67 ± 0.11% for NTSPhox2b, 0.56 ± 0.12% for NTSGABA) in the midbrain, was another moderate nucleus providing inputs to NTS neurons. Conversely, the LC (0.88 ± 0.32% for NTSPhox2b, 0.51 ± 0.04% for NTSGABA), LPBN (0.85 ± 0.24% for NTSPhox2b, 0.40 ± 0.08% for NTSGABA) and Kölliker-Fuse nucleus (K-F, 0.37 ± 0.24% for NTSPhox2b, 0.65 ± 0.18% for NTSGABA) appeared to have minimal labeled neurons in the pontine nuclei.
Collectively, the findings offer a detailed whole-brain mapping of monosynaptic input neurons for both NTSPhox2b and NTSGABA neurons, facilitating the identification of distinct regions implicated in the modulation of various physiological processes for future investigation.
Next, we characterized the outputs of NTS neurons by mapping their axonal terminals, employing a widely-used anterograde viral tracing strategy as reported in previous studies (Sun et al., 2023). To concurrently visualize the axonal projections of NTSPhox2b and NTSGABA neurons, both AAV-EF1α-DIO-EYFP and AAV-EF1α-fDIO-mCherry were injected into the NTS from Phox2b-Flpo::Vgat-Cre mouse mice (Figure 7A). Four weeks post-injection, immunohistochemical staining revealed that both EYFP- and mCherry-expressing somata were predominantly localized within the NTS. A very small number of fluorescently labeled cells were observed in adjacent regions such as the dorsal nucleus of the vagus nerve (DMNV) and area postrema (AP) (Figure 7B). Here we presented the consistent observations of clear projection fields of both types of NTS neurons across all experiments (n = 3 mice).
Figure 7. Photomicrographs of brain regions with a significant density of axon projections of NTSPhox2b and NTSGABA neurons in brainstem. (A) Schematic of viral injection strategy in PHox2b-Flpo::Vgat-Cre mice. (B) Images showing fluorescent NTSPhox2b (mCherry) and NTSGABA (EYFP) neurons. (C–H) Photomicrographs of parallel efferent projections of NTSPhox2b and NTSGABA neurons to brainstem nuclei. Scale bars: 100 μm (B), 50 μm (C-H).
Both NTSPhox2b and NTSGABA neurons were observed primarily projecting to the medulla oblongata, where they targeted well-established brain regions responsible for control of breathing, including the preBötC, BötC, retrotrapezoid nucleus (RTN) and facial nucleus (7 N). Additionally, projections from these neurons were also directed towards the IRt, Amb, DMNV, and hypoglossal nucleus (Figures 7C,D). The pattern of medullary projections was notably similar between NTSPhox2b and NTSGABA neurons. In the pons, EYFP-labeled NTSGABA neurons were found projecting to the principal sensory trigeminal nucleus (Pr5DM) and the motor trigeminal nucleus (5 Ma/5Te) (Figure 7F), a distribution undetected in NTSPhox2b neurons. In the midbrain, mCherry-labeled NTSPhox2b neurons, rather than NTSGABA neurons, specifically targeted the dorsal raphe nucleus (Figure 7H). No significant differences in axonal projections between NTSPhox2b and NTSGABA neurons were detected in the LPBN, K-F nucleus, LC, VLPAG, and lateral periaqueductal gray (LPAG) (Figures 7E,G,H). Region-specific projections were also observed in supra-midbrain regions. As shown in Figure 8, NTSPhox2b neurons but not NTSGABA neurons, extended their axons to the PSTh (Figure 8A), LH (Figure 8B), dorsomedial hypothalamic nucleus (DMH) (Figure 8C), arcuate hypothalamic nucleus (Arc) (Figure 8D), CeA (Figure 8E), and bed nucleus of the stria terminalis (BNST) (Figure 8H). Additionally, axonal terminals from both neuron types were identified in the paraventricular thalamic nucleus (PV) (Figure 8F) and PVN (Figure 8G) projections, highlighting an overlap in their projection patterns.
Figure 8. Mapping of axonal projections of NTSPhox2b and NTSGABA neurons in supra-midbrain areas Images showing supra-midbrain axonal projections of NTSPhox2b neurons (mCherry) and NTSGABA (EYFP) neurons. Scale bars, 50 μm.
To ascertain the projection pattern for both NTSPhox2b and NTSGABA neurons, we quantified pixel of axonal varicosities. Both neuron types exhibited pronounced projections within the medulla oblongata and the pons, while projections were sparser towards the supra-midbrain and midbrain, with minimal to no projections detected reaching the cortex or cerebellum. As illustrated (Figure 9A for NTSPhox2b, Figure 9B for NTSGABA, respectively), statistical analysis revealed that the dense projections from NTS neurons predominantly targeted the IRt (20.28 ± 3.40% for NTSPhox2b, 7.99 ± 0.79% for NTSGABA). Additionally, the LPBN in the pons (6.42 ± 1.68% for NTSPhox2b, 10.42 ± 0.26% for NTSGABA) and VLPAG in the midbrain (6.25 ± 2.13% for NTSPhox2b, 6.44 ± 0.89% for NTSGABA) also received some projections from both types of NTS neurons. In contrast, within the supra-midbrain regions, the BNST (6.41 ± 1.87%) and PSTh (1.66 ± 0.60%) received projections predominantly from NTSPhox2b neurons rather than from NTSGABA neurons, suggesting a differential projection pattern between the two neuron types.
Figure 9. Quantitative analysis of efferent projection from NTSPhox2b and NTSGABA neurons. To quantify the whole-brain efferent projections of NTSPhox2b (A) and NTSGABA (B) neurons, the proportion of axonal projections was calculated as the ratio of the average number of varicosities to the total number of whole-brain projection varicosities.
In this investigation, we present a comprehensive cerebral atlas that vividly delineates both the afferent and efferent projections of NTSPhox2b and NTSGABA neurons, representing excitatory and inhibitory neuronal types, respectively. Our findings reveal that both distinct neuronal populations not only receive monosynaptic inputs from an expansive range of cerebral territories including the medulla oblongata, pons, midbrain, supra-midbrain, and cortical areas, but also reciprocate by sending axonal outputs to the majority of these identical regions. This detailed mapping accentuates the bidirectional connectivity between the NTS and these significant brain regions. Moreover, the differential projection patterns to specific brain regions by both neuronal types implicate their pivotal roles in distinct function-specific neural circuits. This comprehensive neuroanatomical characterization enhances our understanding of the integral contributions of NTSPhox2b and NTSGABA neurons in modulating complex physiological functions.
Previous studies have utilized non-specific axon tracers to explore neural pathways associated with inputs or outputs of the NTS throughout the brain (Ricardo and Koh, 1978; Shioya and Tanaka, 1989; Herbert et al., 1990; Yu and Gordon, 1996; Tanaka et al., 1997; Rinaman, 2010; Ganchrow et al., 2014; Gasparini et al., 2020). Recently, Holt summarized a comprehensive overview of the inputs and outputs of the caudal NTS, revealing widespread inputs from six brain regions (i.e., cortex, subcortical nucleus, diencephalon, mesencephalon, pons, and medulla), along with simultaneous distribution of axonal projections and bidirectional connections among multiple nuclear regions (Holt, 2022). Built upon established neural tracing evidence, our findings provide further insight into inputs and outputs of both excitatory and inhibitory neuronal populations within the NTS, facilitating subsequent investigations aimed at examining function-specific circuits. Specifically, we have demonstrated parallel efferent projections of both NTSPhox2b and NTSGABA neurons within the same animals, thereby revealing the spatial distribution patterns of axonal terminals originating from these distinct neuronal populations in their respective target regions. These findings are instrumental in elucidating the circuit mechanisms underlying the differential regulation of homeostasis mediated by both neuronal populations.
We present that NTSPhox2b neurons are recipients of monosynaptic inputs originating from a broad spectrum of brain regions including the medulla oblongata, pons, midbrain, supra-midbrain areas, cerebellum and cortex, with a significant density of input neurons located in the IRt, AP, CeA and PVN. Moreover, these NTSPhox2b neurons project their axons towards the medulla, pons, midbrain, and supra-midbrain regions, exhibiting dense outputs particularly in the IRt, Amb, RTN, LPBN, VLPAG and BNST, while conspicuously lacking detectable projections to the cerebellum and cortex. Intriguingly, there exists an array of reciprocal connections between NTSPhox2b neurons and several brain regions, predominantly including the IRt, Amb, RTN, DMNV, BNST and PSTh. Likewise, NTSGABA neurons receive and dispatch monosynaptic inputs and axonal projections to the same array of brain regions as NTSPhox2b neurons, with an increased prevalence of input neurons in the IRt, Gi, AP, CeA, PSTh and PVN, and more pronounced outputs in the IRt, 12 N, LPBN and VLPAG. Additionally, NTSGABA neurons also establish reciprocal connections with the IRt, DMNV, AP and LPBN, illustrating complex patterns of intra- and inter-regional neural circuitries.
The quantitative assessment of synaptic inputs reveals that both NTSPhox2b and NTSGABA neurons receive robust monosynaptic inputs predominantly from regions located in the supra-midbrain areas such as the CeA, PVN, PSTh, and an array of medullary regions including the IRt, AP, parvicellular reticular nucleus (PCRt) and LPGi. Notably, a greater density of midbrain neurons appears to monosynaptically innervate NTSGABA neurons as compared to their NTSPhox2b counterparts. Furthermore, a rigorous exploration of the axonal outputs of these neuronal populations indicates that NTSPhox2b neurons preferentially extend axons towards supra-midbrain nuclei like the BNST and PSTh. In contrast, the projections of NTSGABA neurons are more extensively directed towards pontine regions including the Pr5DM and Pr5VL. This divergent connectivity pattern underscores the roles of NTSPhox2b and NTSGABA neurons in orchestrating function-specific neural circuits, which may influence various physiological processes or behaviors through distinct neural pathways.
Although we have elucidated the connectivity between the brain and NTS neurons, the present data did not encompass NTS projections to or from the spinal cord. Specifically, the descending projection to the spinal cord is derived exclusively from a small population of neurons located in the ventrolateral NTS (Saper and Stornetta, 2014). The spinal targets of this pathway include respiratory motor neurons as well as the sympathetic preganglionic column (Loewy and Burton, 1978; Dobbins and Feldman, 1994). Additionally, the NTS also receives afferents from the superficial layers of the spinal and trigeminal dorsal horns (Menetrey and Basbaum, 1987). Many of the dorsal horn neurons contributing to this pathway have neurokinin-1 receptors (Al-Khater and Todd, 2009), are activated by visceral stimuli and contain glutamate (Gamboa-Esteves et al., 2001).
In conjunction with similarities and distinctions in long-range connectivity, our observations also underline reciprocal innervation between NTSPhox2b and NTSGABA neurons, thereby establishing local circuits of interaction among these neuronal populations. It is noteworthy that while neurons within the NTS receive substantial afferent inputs of visceral origin, the focus of the current study is primarily centered on elucidating the central connective architecture, eschewing considerations related to peripheral inputs. This delineation allows for a more targeted analysis of intracerebral neural pathways, which contributes to a deeper understanding of the regulatory mechanisms underpinning these complex neuronal interactions.
The NTS plays an essential role in homeostatic control of breathing. In this study, we elucidate that some brainstem regions establish direct projections to both NTSPhox2b and NTSGABA neurons, forming recurrent circuits that modulate respiratory homeostasis. NTS neurons are integral in processing afferent signals originating from carotid body chemoreceptors, thus playing a crucial role in the modulation of peripheral respiratory chemoreflex pathways. Additionally, NTSPhox2b neurons function as central respiratory chemoreceptors to provide excitatory drive to the respiratory central pattern generator (rCPG) (Fu et al., 2017, 2019). Our findings indicate that NTSPhox2b neurons exhibit reciprocal projections with the RTN and LC, both acknowledged as central respiratory chemoreceptors (Wang et al., 2013a,b; Liu et al., 2021). This arrangement suggests the formation of a regulatory network of central respiratory chemoreceptors, instrumental in maintaining respiratory homeostasis. Moreover, NTSPhox2b neurons establish reciprocal projection with the preBötC, BötC, LPBN and K-F nucleus, all of which contribute to rhymogenesis and pattern generation (Del Negro et al., 2018; Krohn et al., 2023). Likewise, NTSGABA neurons also have bidirectional connections with putative central respiratory chemoreceptors and the rCPG as depicted above. To date, experimental data are lacked regarding control of breathing by NTSGABA neurons. Takakura et al. have made initial observations indicating that a subset of NTSGABA neurons interfaces with RTN chemoreceptors, mediating their inhibition in response to lung inflation, thus hinting at the complex regulatory roles these neurons may play within the respiratory control network (Takakura et al., 2007). In addition to the RTN region, pump cells of the NTS, which were first described in 2004 (Ezure and Tanaka, 2004), also projected their axons to the ipsilateral ventrolateral medulla. And axonal arborizations were found in respiration-related areas and their vicinity between the level of the retrofacial nucleus and the level a few millimeters caudal to the obex (Ezure and Tanaka, 1996).
Based on neuroanatomical evidence, activation of NTSPhox2b neurons through its own sensing mechanism or synaptic inputs may provide excitatory drive to breathing, whereas stimulation of NTSGABA neurons is prone to exert a brake-like effect on breathing likely through acting on central chemoreceptors or the rCPG. Of note, both types of population receive monosynaptic input arising from the preBötC, a kernel structure responsible for inspiratory rhythmogenesis. Recent findings demonstrate that in addition to inspiratory motor output, Cdh9/Dbx1-residing preBötC neurons contribute to regulating the balance between calm and arousal behaviors (Yackle et al., 2017). According to the present and previous data (Yang and Feldman, 2018; Yang et al., 2020), whether SST-expressing preBötC neurons regulate breathing pattern through a feedback circuit mechanism, for example, acting on NTSPhox2b and NTSGABA neurons remains unclear.
The PAG contributes to integrating motor, limbic, and sensory information to modulate behavior-related breathing, such as gasping and vocalization (Subramanian and Holstege, 2010). The stimulation of dorsal PAG and LPAG induces tachypnea and they are mostly involved in active coping strategies, such as fighting or fleeing, while the stimulation of dorsomedial PAG and VLPAG elicited bradypnea, profound respiration, dyspnea, and inspiratory apneas that it is associated with passive coping strategies, such as freezing (Gonzalez-Garcia et al., 2024). The reciprocal projections between NTSPhox2b and NTSGABA neurons and the PAG may reveal circuit mechanisms underlying the above respiratory effects.
Descending inputs from the forebrain provide information regarding emotional, cognitive, and physiological state to the NTS. Thereby, the NTS neurons may orchestrate these behaviors and trigger different autonomic output, including breathing. Our findings demonstrate that the cortex project directly to both NTSPhox2b and NTSGABA neurons, with the putative purpose of promoting behaviors that need to be timed with certain autonomic function such as the breathing cycle, e.g., breath-hold, chewing, swallowing and vocalization.
In the current investigation, the CeA, PVN and PSTh project heavily to the NTS, in line with previous studies obtained using neural tracing dye (Gasparini et al., 2020; Kim et al., 2022; Huo et al., 2024). Previous studies applied the conventional anterograde tracing method to demonstrate the axonal connections of the NTS with the PVN (Shi et al., 2021; Liu et al., 2023), BNST (Shi et al., 2021), CeA (He et al., 2022) and Arc (Martinez de Morentin et al., 2024), a framework that was further extended using specific cell types of the NTS in the present study. The CeA is the critical centers for processing emotional behaviors, learning and fear response. The amygdala, receiving respiratory inputs, exhibits rhythmic activity that correlates with inspiratory activity signaled by the phrenic nerve root (Onimaru and Homma, 2007), suggesting that the amygdala’s spontaneous oscillatory behavior is associated with respiratory functions. In humans, electrical stimulation of the amygdala leads to an apnea (Dlouhy et al., 2015). Therefore, it appears that the CeA-NTS circuits mediate behavioral/emotional regulation of autonomic output.
The PVN plays imperative roles in the regulation of energy balance and various endocrinological activities, as well as ventilatory homeostasis (Fukushi et al., 2019). It has been shown that electrical stimulation of the PVN in anesthetized rabbits produced an increase in respiratory frequency (Duan et al., 1997); injection of glutamate into the PVN increased electromyographic activity of the diaphragm in anesthetized rats (Yeh et al., 1997). Moreover, disinhibition of the PVN with GABAA receptor antagonist increased both respiratory frequency and tidal volume in conscious rats (Schlenker et al., 2001). The most prominent role of the PVN is its involvement in the mediation of the respiratory response to hypoxia (Ruyle et al., 2019). Furthermore, the role of the PVN in mediating chemoreflex may be specific to hypoxic but not to hypercapnic stimulation (Reddy et al., 2005). However, the neural circuit mechanism through which the PVN regulates ventilation remain to be fully elucidated. The present neural tracing data may provide putative circuit mechanism underlying these effects.
In the present investigation, we have elucidated the whole-brain mapping of projections of both NTSPhox2b and NTSGABA neurons. However, the study has certain limitations due to its aim and technical challenges. First, it should be noted that this study did not examine projections to or from the spinal cord, nor did it address potential peripheral inputs to these neuronal populations. Such inputs may originate from critical regions like the carotid body and carotid sinus, as well as relay cardiopulmonary and gastrointestinal information. It stands to reason that NTSPhox2b and NTSGABA neurons are poised to concurrently receive both central and peripheral inputs, thereafter integrating these signals and projecting outputs to specific target neurons. Second, based on injection sites and reporter expression, NTSPhox2b and NTSGABA neurons were primarily distributed in the intermediate and caudal parts of the NTS (bregma −7.0 to −8.0 mm). These neurons did not extensively cover the rostral gustatory portions or the caudal commissural portion of the NTS. Third, the current study did not employ cell type-specific markers to identify target brain regions, such as the use of tyrosine hydroxylase as a marker for locus coeruleus (LC) neurons. This absence of cell type-specific markers could potentially limit the scope of quantitative analyses, particularly in the accurate enumeration of cells and axonal varicosities.
Collectively, we established precise anatomical connections between the specific NTS subpopulations and many brain nuclei. The whole brain mapping of inputs and outputs of both excitatory and inhibitory NTS neurons provides a foundational anatomical framework for forthcoming investigations into the inter-regional pathways involved in the respiratory functions of the NTS.
The raw data supporting the conclusions of this article will be made available by the authors, without undue reservation.
The animal study was approved by the Animal Care and Ethics Committee of Hebei Medical University. The study was conducted in accordance with the local legislation and institutional requirements.
LS: Data curation, Investigation, Writing – original draft. FK: Investigation, Writing – review & editing. XT: Writing – review & editing. TD: Writing – review & editing. YW: Writing – review & editing. YJ: Writing – review & editing. XW: Writing – review & editing. HY: Writing – review & editing. FY: Writing – review & editing. CF: Methodology, Writing – original draft, Writing – review & editing. SW: Conceptualization, Supervision, Writing – original draft, Writing – review & editing.
The author(s) declare financial support was received for the research, authorship, and/or publication of this article. This work was supported by the National Natural Science Foundation of China grant (U23A20431 and 31971058 to SW; 82000001 to CF), the Natural Science Foundation of Hebei Province for Innovative Research Group Project (H2021206203 to SW), and the Natural Science Foundation of Hebei Province for Precision Medicine Joint Funds (C2020206028 to CF).
We are grateful to the Core Facilities and Centers, Hebei Medical University Institute of Medicine and Health for experimental and technical support.
The authors declare that the research was conducted in the absence of any commercial or financial relationships that could be construed as a potential conflict of interest.
All claims expressed in this article are solely those of the authors and do not necessarily represent those of their affiliated organizations, or those of the publisher, the editors and the reviewers. Any product that may be evaluated in this article, or claim that may be made by its manufacturer, is not guaranteed or endorsed by the publisher.
Aklan, I., Sayar Atasoy, N., Yavuz, Y., Ates, T., Coban, I., Koksalar, F., et al. (2020). NTS catecholamine neurons mediate hypoglycemic hunger via medial hypothalamic feeding pathways. Cell Metab. 31, 313–326.e5. doi: 10.1016/j.cmet.2019.11.016
Al-Khater, K. M., and Todd, A. J. (2009). Collateral projections of neurons in laminae I, III, and IV of rat spinal cord to thalamus, periaqueductal gray matter, and lateral parabrachial area. J. Comp. Neurol. 515, 629–646. doi: 10.1002/cne.22081
Brunet, J. F., and Pattyn, A. (2002). Phox2 genes – from patterning to connectivity. Curr. Opin. Genet. Dev. 12, 435–440. doi: 10.1016/s0959-437x(02)00322-2
Cheng, W., Gonzalez, I., Pan, W., Tsang, A. H., Adams, J., Ndoka, E., et al. (2020). Calcitonin receptor neurons in the mouse nucleus Tractus Solitarius control energy balance via the non-aversive suppression of feeding. Cell Metab. 31, 301–312.e5. doi: 10.1016/j.cmet.2019.12.012
Del Negro, C. A., Funk, G. D., and Feldman, J. L. (2018). Breathing matters. Nat. Rev. Neurosci. 19, 351–367. doi: 10.1038/s41583-018-0003-6
Dlouhy, B. J., Gehlbach, B. K., Kreple, C. J., Kawasaki, H., Oya, H., Buzza, C., et al. (2015). Breathing inhibited when seizures spread to the amygdala and upon amygdala stimulation. J. Neurosci. 35, 10281–10289. doi: 10.1523/JNEUROSCI.0888-15.2015
Dobbins, E. G., and Feldman, J. L. (1994). Brainstem network controlling descending drive to phrenic motoneurons in rat. J. Comp. Neurol. 347, 64–86. doi: 10.1002/cne.903470106
Duan, Y. F., Winters, R., McCabe, P. M., Green, E. J., Huang, Y., and Schneiderman, N. (1997). Cardiorespiratory components of defense reaction elicited from paraventricular nucleus. Physiol. Behav. 61, 325–330. doi: 10.1016/s0031-9384(96)00410-6
Dubreuil, V., Ramanantsoa, N., Trochet, D., Vaubourg, V., Amiel, J., Gallego, J., et al. (2008). A human mutation in Phox2b causes lack of CO2 chemosensitivity, fatal central apnea, and specific loss of parafacial neurons. Proc. Natl. Acad. Sci. U. S. A. 105, 1067–1072. doi: 10.1073/pnas.0709115105
Ezure, K., and Tanaka, I. (1996). Pump neurons of the nucleus of the solitary tract project widely to the medulla. Neurosci. Lett. 215, 123–126. doi: 10.1016/0304-3940(96)12968-2
Ezure, K., and Tanaka, I. (2004). GABA, in some cases together with glycine, is used as the inhibitory transmitter by pump cells in the Hering-Breuer reflex pathway of the rat. Neuroscience 127, 409–417. doi: 10.1016/j.neuroscience.2004.05.032
Fu, C., Shi, L., Wei, Z., Yu, H., Hao, Y., Tian, Y., et al. (2019). Activation of Phox2b-expressing neurons in the nucleus Tractus Solitarii drives breathing in mice. J. Neurosci. 39, 2837–2846. doi: 10.1523/JNEUROSCI.2048-18.2018
Fu, C., Xue, J., Wang, R., Chen, J., Ma, L., Liu, Y., et al. (2017). Chemosensitive Phox2b-expressing neurons are crucial for hypercapnic ventilatory response in the nucleus tractus solitarius. J. Physiol. 595, 4973–4989. doi: 10.1113/JP274437
Fukushi, I., Yokota, S., and Okada, Y. (2019). The role of the hypothalamus in modulation of respiration. Respir. Physiol. Neurobiol. 265, 172–179. doi: 10.1016/j.resp.2018.07.003
Gamboa-Esteves, F. O., Kaye, J. C., McWilliam, P. N., Lima, D., and Batten, T. F. (2001). Immunohistochemical profiles of spinal lamina I neurones retrogradely labelled from the nucleus tractus solitarii in rat suggest excitatory projections. Neuroscience 104, 523–538. doi: 10.1016/s0306-4522(01)00071-9
Ganchrow, D., Ganchrow, J. R., Cicchini, V., Bartel, D. L., Kaufman, D., Girard, D., et al. (2014). Nucleus of the solitary tract in the C57BL/6J mouse: subnuclear parcellation, chorda tympani nerve projections, and brainstem connections. J. Comp. Neurol. 522, 1565–1596. doi: 10.1002/cne.23484
Gasparini, S., Howland, J. M., Thatcher, A. J., and Geerling, J. C. (2020). Central afferents to the nucleus of the solitary tract in rats and mice. J. Comp. Neurol. 528, 2708–2728. doi: 10.1002/cne.24927
Gasparini, S., Resch, J. M., Narayan, S. V., Peltekian, L., Iverson, G. N., Karthik, S., et al. (2019). Aldosterone-sensitive HSD2 neurons in mice. Brain Struct. Funct. 224, 387–417. doi: 10.1007/s00429-018-1778-y
Geerling, J. C., Engeland, W. C., Kawata, M., and Loewy, A. D. (2006). Aldosterone target neurons in the nucleus tractus solitarius drive sodium appetite. J. Neurosci. 26, 411–417. doi: 10.1523/JNEUROSCI.3115-05.2006
Geerling, J. C., and Loewy, A. D. (2006). Aldosterone-sensitive neurons in the nucleus of the solitary tract: efferent projections. J. Comp. Neurol. 497, 223–250. doi: 10.1002/cne.20993
Gonzalez-Garcia, M., Carrillo-Franco, L., Morales-Luque, C., Dawid-Milner, M. S., and Lopez-Gonzalez, M. V. (2024). Central autonomic mechanisms involved in the control of laryngeal activity and vocalization. Biology (Basel) 13:118. doi: 10.3390/biology13020118
He, S., Huang, X., Zheng, J., Zhang, Y., and Ruan, X. (2022). An NTS-CeA projection modulates depression-like behaviors in a mouse model of chronic pain. Neurobiol. Dis. 174:105893. doi: 10.1016/j.nbd.2022.105893
Herbert, H., Moga, M. M., and Saper, C. B. (1990). Connections of the parabrachial nucleus with the nucleus of the solitary tract and the medullary reticular formation in the rat. J. Comp. Neurol. 293, 540–580. doi: 10.1002/cne.902930404
Hirsch, M. R., d'Autreaux, F., Dymecki, S. M., Brunet, J. F., and Goridis, C. (2013). A Phox2b::FLPo transgenic mouse line suitable for intersectional genetics. Genesis 51, 506–514. doi: 10.1002/dvg.22393
Holt, M. K. (2022). The ins and outs of the caudal nucleus of the solitary tract: an overview of cellular populations and anatomical connections. J. Neuroendocrinol. 34:e13132. doi: 10.1111/jne.13132
Huo, L., Ye, Z., Liu, M., He, Z., Huang, M., Li, D., et al. (2024). Brain circuits for retching-like behavior. Natl. Sci. Rev. 11:nwad256. doi: 10.1093/nsr/nwad256
Jun, S., Ou, X., Shi, L., Yu, H., Deng, T., Chen, J., et al. (2023). Circuit-specific control of blood pressure by PNMT-expressing nucleus Tractus Solitarii neurons. Neurosci. Bull. 39, 1193–1209. doi: 10.1007/s12264-022-01008-3
Kang, B. J., Chang, D. A., Mackay, D. D., West, G. H., Moreira, T. S., Takakura, A. C., et al. (2007). Central nervous system distribution of the transcription factor Phox2b in the adult rat. J. Comp. Neurol. 503, 627–641. doi: 10.1002/cne.21409
Kim, J. H., Kromm, G. H., Barnhill, O. K., Sperber, J., Heuer, L. B., Loomis, S., et al. (2022). A discrete parasubthalamic nucleus subpopulation plays a critical role in appetite suppression. eLife 11:e75470. doi: 10.7554/eLife.75470
Krohn, F., Novello, M., van der Giessen, R. S., De Zeeuw, C. I., Pel, J. J. M., and Bosman, L. W. J. (2023). The integrated brain network that controls respiration. eLife 12:e83654. doi: 10.7554/eLife.83654
Liu, N., Fu, C., Yu, H., Wang, Y., Shi, L., Hao, Y., et al. (2021). Respiratory control by Phox2b-expressing neurons in a locus Coeruleus-preBotzinger complex circuit. Neurosci. Bull. 37, 31–44. doi: 10.1007/s12264-020-00519-1
Liu, Y., Wei, J. A., Luo, Z., Cui, J., Luo, Y., Mak, S. O. K., et al. (2023). A gut-brain axis mediates sodium appetite via gastrointestinal peptide regulation on a medulla-hypothalamic circuit. Sci. Adv. 9:eadd5330. doi: 10.1126/sciadv.add5330
Loewy, A. D., and Burton, H. (1978). Nuclei of the solitary tract: efferent projections to the lower brain stem and spinal cord of the cat. J. Comp. Neurol. 181, 421–449. doi: 10.1002/cne.901810211
Martinez de Morentin, P. B., Gonzalez, J. A., Dowsett, G. K. C., Martynova, Y., Yeo, G. S. H., Sylantyev, S., et al. (2024). A brainstem to hypothalamic arcuate nucleus GABAergic circuit drives feeding. Curr. Biol. 34, 1646–1656.e4. doi: 10.1016/j.cub.2024.02.074
Menetrey, D., and Basbaum, A. I. (1987). Spinal and trigeminal projections to the nucleus of the solitary tract: a possible substrate for somatovisceral and viscerovisceral reflex activation. J. Comp. Neurol. 255, 439–450. doi: 10.1002/cne.902550310
Onimaru, H., and Homma, I. (2007). Spontaneous oscillatory burst activity in the piriform-amygdala region and its relation to in vitro respiratory activity in newborn rats. Neuroscience 144, 387–394. doi: 10.1016/j.neuroscience.2006.09.033
Osakada, F., and Callaway, E. M. (2013). Design and generation of recombinant rabies virus vectors. Nat. Protoc. 8, 1583–1601. doi: 10.1038/nprot.2013.094
Paxinos, G., and Franklin, K. B. J. (2013). The mouse brain in stereotaxic coordinates. San Diego: Academic Press.
Reddy, M. K., Patel, K. P., and Schultz, H. D. (2005). Differential role of the paraventricular nucleus of the hypothalamus in modulating the sympathoexcitatory component of peripheral and central chemoreflexes. Am. J. Physiol. Regul. Integr. Comp. Physiol. 289, R789–R797. doi: 10.1152/ajpregu.00222.2005
Resch, J. M., Fenselau, H., Madara, J. C., Wu, C., Campbell, J. N., Lyubetskaya, A., et al. (2017). Aldosterone-sensing neurons in the NTS exhibit state-dependent pacemaker activity and drive sodium appetite via synergy with angiotensin II signaling. Neuron 96, 190–206.e7. doi: 10.1016/j.neuron.2017.09.014
Ricardo, J. A., and Koh, E. T. (1978). Anatomical evidence of direct projections from the nucleus of the solitary tract to the hypothalamus, amygdala, and other forebrain structures in the rat. Brain Res. 153, 1–26. doi: 10.1016/0006-8993(78)91125-3
Rinaman, L. (2010). Ascending projections from the caudal visceral nucleus of the solitary tract to brain regions involved in food intake and energy expenditure. Brain Res. 1350, 18–34. doi: 10.1016/j.brainres.2010.03.059
Roman, C. W., Derkach, V. A., and Palmiter, R. D. (2016). Genetically and functionally defined NTS to PBN brain circuits mediating anorexia. Nat. Commun. 7:11905. doi: 10.1038/ncomms11905
Ruyle, B. C., Martinez, D., Heesch, C. M., Kline, D. D., and Hasser, E. M. (2019). The PVN enhances cardiorespiratory responses to acute hypoxia via input to the nTS. Am. J. Physiol. Regul. Integr. Comp. Physiol. 317, R818–R833. doi: 10.1152/ajpregu.00135.2019
Saper, C. B., and Stornetta, R. L. (2014). “Central autonomic system,” in The rat nervous system. (Fourth Edition). ed. G. Paxinos (Academic Press), 629–673.
Schlenker, E., Barnes, L., Hansen, S., and Martin, D. (2001). Cardiorespiratory and metabolic responses to injection of bicuculline into the hypothalamic paraventricular nucleus (PVN) of conscious rats. Brain Res. 895, 33–40. doi: 10.1016/s0006-8993(01)02011-x
Scott, M. M., Williams, K. W., Rossi, J., Lee, C. E., and Elmquist, J. K. (2011). Leptin receptor expression in hindbrain Glp-1 neurons regulates food intake and energy balance in mice. J. Clin. Invest. 121, 2413–2421. doi: 10.1172/JCI43703
Shi, M. Y., Ding, L. F., Guo, Y. H., Cheng, Y. X., Bi, G. Q., and Lau, P. M. (2021). Long-range GABAergic projections from the nucleus of the solitary tract. Mol. Brain 14:38. doi: 10.1186/s13041-021-00751-4
Shioya, M., and Tanaka, J. (1989). Inputs from the nucleus of the solitary tract to subfornical organ neurons projecting to the paraventricular nucleus in the rat. Brain Res. 483, 192–195. doi: 10.1016/0006-8993(89)90054-1
Subramanian, H. H., and Holstege, G. (2010). Periaqueductal gray control of breathing. Adv. Exp. Med. Biol. 669, 353–358. doi: 10.1007/978-1-4419-5692-7_72
Sun, L., Zhu, M., Wang, M., Hao, Y., Hao, Y., Jing, X., et al. (2023). Whole-brain monosynaptic inputs and outputs of leptin receptor b neurons of the nucleus tractus solitarii in mice. Brain Res. Bull. 201:110693. doi: 10.1016/j.brainresbull.2023.110693
Takakura, A. C., Moreira, T. S., West, G. H., Gwilt, J. M., Colombari, E., Stornetta, R. L., et al. (2007). GABAergic pump cells of solitary tract nucleus innervate retrotrapezoid nucleus chemoreceptors. J. Neurophysiol. 98, 374–381. doi: 10.1152/jn.00322.2007
Tanaka, J., Hayashi, Y., Shimamune, S., and Nomura, M. (1997). Ascending pathways from the nucleus of the solitary tract to the subfornical organ in the rat. Brain Res. 777, 237–241. doi: 10.1016/s0006-8993(97)01211-0
Wall, N. R., Wickersham, I. R., Cetin, A., De La Parra, M., and Callaway, E. M. (2010). Monosynaptic circuit tracing in vivo through Cre-dependent targeting and complementation of modified rabies virus. Proc. Natl. Acad. Sci. USA 107, 21848–21853. doi: 10.1073/pnas.1011756107
Wang, S., Benamer, N., Zanella, S., Kumar, N. N., Shi, Y., Bevengut, M., et al. (2013a). TASK-2 channels contribute to pH sensitivity of retrotrapezoid nucleus chemoreceptor neurons. J. Neurosci. 33, 16033–16044. doi: 10.1523/JNEUROSCI.2451-13.2013
Wang, Y. K., Deng, T. J., Zhao, X., Shao, L. Q., Chen, J. T., Fu, C. R., et al. (2024). Control of breathing by orexinergic signaling in the nucleus tractus solitarii. Sci. Rep. 14:7473. doi: 10.1038/s41598-024-58075-x
Wang, S., Shi, Y., Shu, S., Guyenet, P. G., and Bayliss, D. A. (2013b). Phox2b-expressing retrotrapezoid neurons are intrinsically responsive to H+ and CO2. J. Neurosci. 33, 7756–7761. doi: 10.1523/JNEUROSCI.5550-12.2013
Yackle, K., Schwarz, L. A., Kam, K., Sorokin, J. M., Huguenard, J. R., Feldman, J. L., et al. (2017). Breathing control center neurons that promote arousal in mice. Science 355, 1411–1415. doi: 10.1126/science.aai7984
Yang, C. F., and Feldman, J. L. (2018). Efferent projections of excitatory and inhibitory preBotzinger complex neurons. J. Comp. Neurol. 526, 1389–1402. doi: 10.1002/cne.24415
Yang, C. F., Kim, E. J., Callaway, E. M., and Feldman, J. L. (2020). Monosynaptic projections to excitatory and inhibitory preBotzinger complex neurons. Front. Neuroanat. 14:58. doi: 10.3389/fnana.2020.00058
Yeh, E. R., Erokwu, B., LaManna, J. C., and Haxhiu, M. A. (1997). The paraventricular nucleus of the hypothalamus influences respiratory timing and activity in the rat. Neurosci. Lett. 232, 63–66. doi: 10.1016/s0304-3940(97)00579-x
Yu, D., and Gordon, F. J. (1996). Anatomical evidence for a bi-neuronal pathway connecting the nucleus tractus solitarius to caudal ventrolateral medulla to rostral ventrolateral medulla in the rat. Neurosci. Lett. 205, 21–24. doi: 10.1016/0304-3940(96)12383-1
Yu, H., Shi, L., Chen, J., Jun, S., Hao, Y., Wang, S., et al. (2022). A neural circuit mechanism controlling breathing by leptin in the nucleus Tractus Solitarii. Neurosci. Bull. 38, 149–165. doi: 10.1007/s12264-021-00742-4
Zhang, C., Kaye, J. A., Cai, Z., Wang, Y., Prescott, S. L., and Liberles, S. D. (2020). Area Postrema cell types that mediate nausea-associated behaviors. Neuron 109, 461–472.e5. doi: 10.1016/j.neuron.2020.11.010
Keywords: nucleus tractus solitarii, breathing, neural circuit, neural tracing, modified rabies
Citation: Shao L, Kong F, Tian X, Deng T, Wang Y, Ji Y, Wang X, Yu H, Yuan F, Fu C and Wang S (2024) Whole-brain inputs and outputs of Phox2b and GABAergic neurons in the nucleus tractus solitarii. Front. Neurosci. 18:1427384. doi: 10.3389/fnins.2024.1427384
Received: 03 May 2024; Accepted: 04 June 2024;
Published: 14 June 2024.
Edited by:
David D. Kline, University of Missouri, United StatesReviewed by:
Baojian Xue, The University of Iowa, United StatesCopyright © 2024 Shao, Kong, Tian, Deng, Wang, Ji, Wang, Yu, Yuan, Fu and Wang. This is an open-access article distributed under the terms of the Creative Commons Attribution License (CC BY). The use, distribution or reproduction in other forums is permitted, provided the original author(s) and the copyright owner(s) are credited and that the original publication in this journal is cited, in accordance with accepted academic practice. No use, distribution or reproduction is permitted which does not comply with these terms.
*Correspondence: Congrui Fu, MTg4MDE0NDdAaGVibXUuZWR1LmNu; Sheng Wang, d2FuZ3NoZW5nQGhlYm11LmVkdS5jbg==
†These authors have contributed equally to this work
Disclaimer: All claims expressed in this article are solely those of the authors and do not necessarily represent those of their affiliated organizations, or those of the publisher, the editors and the reviewers. Any product that may be evaluated in this article or claim that may be made by its manufacturer is not guaranteed or endorsed by the publisher.
Research integrity at Frontiers
Learn more about the work of our research integrity team to safeguard the quality of each article we publish.