- 1Department of Biochemistry and Pharmacology, Uzhhorod National University, Uzhhorod, Ukraine
- 2Department of Microbiology, Virology, and Immunology, I. Horbachevsky Ternopil National Medical University, Ternopil, Ukraine
- 3Department of Neurology, I. Horbachevsky Ternopil National Medical University, Ternopil, Ukraine
- 4Broegelmann Research Laboratory, Department of Clinical Science, University of Bergen, Bergen, Norway
- 5Department of Microbiology, Shahr-e-Qods Branch, Islamic Azad University, Tehran, Iran
This mini-review explores the role of short-chain fatty acids (SCFAs) in posttraumatic stress disorder (PTSD). Highlighting the microbiota-gut-brain axis, this study investigated the bidirectional communication between the gut microbiome and mental health. SCFAs, byproducts of gut microbial fermentation, have been examined for their potential impact on PTSD, with a focus on molecular mechanisms and therapeutic interventions. This review discusses changes in SCFA levels and bacterial profiles in individuals with PTSD, emphasizing the need for further research. Promising outcomes from clinical trials using probiotics and fermented formulations suggest potential avenues for PTSD management. Future directions involve establishing comprehensive human cohorts, integrating multiomics data, and employing advanced computational methods, with the goal of deepening our understanding of the role of SCFAs in PTSD and exploring microbiota-targeted interventions.
1 Introduction
Posttraumatic stress disorder (PTSD) manifests as a set of distinct symptoms arising from exposure to, or observation of, highly distressing events carrying a genuine risk of death, actual death, or severe injury (Gradus and Galea, 2022).
In line with the Diagnostic and Statistical Manual of Mental Disorders (DSM-5), PTSD is characterized by four symptom clusters: re-experiencing, avoidance, negative alterations in cognition and mood, and alterations in arousal and reactivity (American Psychiatric Association D, Association AP, 2013; Pai et al., 2017). This chronic mental health condition can instigate persistent feelings of fear, disarray, or panic following an encounter with or witnessing a traumatic incident (Panagioti et al., 2015; Pai et al., 2017). In addition to the main symptoms of PTSD, patients also experience PTSD-related symptoms such as depression, anxiety, insomnia, and circadian rhythm disturbances (Short et al., 2020; Kyzar et al., 2021).
Recent research has increasingly focused on the intricate interplay between the gut microbiome and PTSD, revealing the potential role of the gut-brain axis in influencing mental health (Gautam et al., 2015; Ke et al., 2023; Petakh et al., 2024a).
The gut microbiome, a diverse community of microorganisms residing in the gastrointestinal tract, plays a crucial role in maintaining physiological balance and impacting various aspects of human health (Thursby and Juge, 2017). Short-chain fatty acids (SCFAs), metabolic byproducts of gut microbial fermentation, have garnered attention for their potential involvement in mental health conditions, including PTSD (van de Wouw et al., 2018).
This mini-review delves into the molecular mechanisms and therapeutic possibilities of SCFAs in the context of PTSD. As we explored the intricate connections between the gut microbiota, SCFAs, and PTSD, we aimed to provide insights into the changes in SCFA levels and the profiles of bacteria producing them in individuals affected by PTSD. Additionally, we will discuss potential therapeutic interventions, drawing from both clinical trials and emerging research.
By examining the current state of knowledge on SCFAs and their impact on PTSD, this mini-review aims to contribute to the evolving understanding of the gut–brain axis and open avenues for novel approaches in the treatment and management of PTSD. Through a comprehensive exploration of the molecular underpinnings and therapeutic potential of SCFAs, we aspire to elucidate promising directions for future research and clinical applications in the field of mental health.
2 Short-chain fatty acids: overview
Among the myriad of metabolites generated by the microbiome, SCFAs are significant components. Acetate (C2), propionate (C3), and butyrate (C4) are the primary SCFAs, collectively representing a substantial portion. Acetate predominates, constituting approximately 60% of the SCFAs, while propionate and butyrate each contribute approximately 20%. Although SCFAs are prominent, they do not encompass the entirety of microbiota-derived metabolites. Other metabolites, including various lactate isomers, valerate, isobutyrate, isovalerate, and secondary bile acids, also exist, albeit at lower concentrations (Cait et al., 2018). Secondary bile acids are formed by enzymatic modifications of primary bile acids by bacteria present in the colon, where they serve as substrates for microbial metabolism (McMillin and DeMorrow, 2016). For example, secondary bile acids can activate bile acid receptors located in different organs, participating in signaling pathways from the gut to other organs. This hypothesis is supported by the presence of bile acid receptors in the liver, brain, and muscles, suggesting a bile acid-gut-organ axis (Mohanty et al., 2024).
SCFAs exert their effects in part through free fatty acid receptors (FFARs), with FFAR2 (also known as GPR43) and FFAR3 (known as GPR41) being key players. These G protein-coupled receptors are found on various cells, including neurons, colonocytes, pancreatic cells, adipocytes, and others (Yang et al., 2020). Acetate and propionate predominantly activate FFAR2, while butyrate influences FFAR3 (Brown et al., 2003). These receptors have implications for inflammation modulation, energy consumption in neurons, insulin secretion, and enteroendocrine function (Prentice et al., 2019; Jiao et al., 2021; Zou et al., 2021).
These receptors are located on the apical membrane of the colon epithelium, where luminal SCFAs activate them to initiate intracellular second messenger signaling cascades (Smith et al., 2013; McKenzie et al., 2017). Their activation triggers signaling pathways involving the Gαs and Gβγ subunits, leading to ERK activation, a reduction in cellular cyclic adenosine monophosphate (cAMP) levels, and an increase in intracellular Ca2+ concentrations. This, in turn, serves as a secondary messenger that initiates various biological responses and downstream signaling cascades, including protein phosphorylation and alterations in cellular behavior (Le Poul et al., 2003; Gaidarov et al., 2013; Park et al., 2021). Furthermore, the interaction of GPCRs with β-arrestin (β-ARR) also stimulates the activation of Gαs and Gβγ subunits, thereby modulating physiological processes such as chemotaxis, apoptosis, proliferation, differentiation, and gene expression in vivo (O’Hayre et al., 2017; Böttke et al., 2020). Notably, while the FFAR3 receptor has been reported to signal exclusively via the Gi protein family, FFAR2 has been found to activate G proteins from both the Gi and the Gq families, but induces signaling in a Gαi/o/q/11- and β-arrestin-independent fashion, which has been shown to be mediated via Gα12/13 proteins (Grundmann et al., 2021). Following their uptake by colonocytes, SCFAs proceed into the mitochondria’s citric acid cycle, where they participate in ATP synthesis, providing essential energy for cellular functions (Schönfeld and Wojtczak, 2016).
Their passage through the epithelium is crucial for reaching the serosal side and influencing immune cells in the lamina propria (Kaji et al., 2015; Stumpff, 2018). Effective transport mechanisms are essential for luminal entry and transcellular transport, without which SCFAs cannot exert intracellular effects on colon epithelial cells or impact mucosal immune cells (Binder, 2010). Given the physiological colonic pH, SCFAs predominantly exist in anionic forms, hindering simple diffusion. Consequently, ionized SCFAs are anticipated to require carrier-mediated absorption pathways. Numerous studies have highlighted evidence supporting the existence of such pathways for ionized SCFA absorption. Thus, the presence of SCFA transporters within colon epithelial cells significantly influences the beneficial effects of SCFAs on the host. Notably, transporters such as H + -coupled (e.g., monocarboxylate transporter MCT1/4) and Na + −coupled (e.g., sodium-coupled monocarboxylate transporter SMCT1/2) transporters facilitate the transfer of SCFAs into colon epithelial cells, playing pivotal roles in SCFA absorption and utilization within the intestine (Sivaprakasam et al., 2017; Holota et al., 2019).
As evidenced by experiments in cell cultures, these effects may be facilitated by the presence of abundant monocarboxylate transporters (MCTs) on endothelial cells (Mitchell et al., 2011). Studies in rats have shown that following injection into the carotid artery, butyrate exhibits the highest brain uptake, followed by propionate and acetate (Kekuda et al., 2013; Vijay and Morris, 2014). A study reported that the human brain has an average content of 17.0 pmol/mg of tissue for butyrate and 18.8 pmol/mg of tissue for propionate. When the data are recalculated to account for brain water, which makes up approximately 75% of the brain’s weight, the levels of both SCFAs increase. Specifically, the levels of butyrate and propionate are approximately 21 μM and 23 μM, respectively, which are similar to the levels found in plasma (Bachmann et al., 1979). PET imaging experiments in rats indicated that a small percentage of intravenously infused acetate was rapidly taken up by the brain, with slightly lower uptake observed after colonic infusion (Kim et al., 2013). However, studies in primates and humans using labeled SCFAs have shown minimal brain uptake, suggesting that despite their ability to cross the BBB, SCFAs are not substantially absorbed by the brain (Seltzer et al., 2004).
SCFAs also exert their effects by inhibiting histone deacetylases (HDACs), which are enzymes responsible for removing acetyl groups from histone and nonhistone complexes, thereby regulating gene expression. Inhibition of HDACs by SCFAs leads to increased histone acetylation, resulting in relaxation of chromatin structure and enhanced accessibility of certain genes to transcription factors, ultimately promoting their expression (Friedrich et al., 2019). This mechanism is particularly relevant in inflammatory diseases, where HDAC inhibitors have shown potent anti-inflammatory activity (Elfiky et al., 2022). SCFAs, which are produced by intestinal microorganisms, play a significant role in this process, especially during intestinal barrier repair and in the regulation of metabolic diseases (Bose et al., 2014; Li et al., 2018; Vizioli et al., 2020). In intestinal epithelial cells (IECs), a specific HDAC known as HDAC3 regulates histone acetylation and integrates signals from the gut microbiota to maintain intestinal homeostasis (Zhang et al., 2020; Zhou et al., 2021).
SCFAs confer several health benefits, particularly through the upregulation of tight junction (TJ) proteins and reinforcement of the mucus layer, with butyrate notably contributing to these effects (Higashimura et al., 2015; Shimizu et al., 2019). Additionally, SCFAs contribute to the prevention of colon cancer by inducing the differentiation and apoptosis of colonic cells (Weitkunat et al., 2016). SCFAs, particularly acetate, play a role in appetite regulation and human metabolism. They may reduce appetite, body weight, and liver steatosis while modulating glucose and lipid metabolism (Zhao et al., 2017; Bartolomaeus et al., 2019; Hsu et al., 2019; Yu et al., 2021). Increased levels of butyrate and propionate are associated with reduced blood pressure and plasminogen activator inhibitor-1 (PAI-1) levels in the context of cardiometabolic health (Xu et al., 2022). SCFAs have notable immunomodulatory effects, affecting both innate and adaptive immunity. These compounds reduce neutrophil activity, inhibit inflammatory cell chemotaxis, enhance regulatory T-cell activity, and suppress gut inflammation (Li et al., 2018, 2021; Liu et al., 2021).
The primary source of bacteria responsible for SCFA production is the intestinal microbiota, which engages in the fermentation of dietary fiber and resistant starches to generate SCFAs. Intriguingly, the concentration of SCFAs exhibits dynamic changes throughout our lifespan, and these variations seem to correlate with shifts in the composition of the gut microbiome, which itself undergoes alterations across different stages of life (Li et al., 2019; Meng et al., 2019). It is important to highlight that many internal and external factors, such as diet, drug use, and exercise, play significant roles in determining the quantity of SCFAs produced within the intestines. These changes in diet, for example, in turn, affect the availability of substrate sources for bacteria engaged in SCFA production (Sanna et al., 2019).
3 The microbiota-gut-brain axis
A growing body of scientific research highlights the existence of a microbiota–gut–brain axis linking the gut microbiota to mental health (Adan et al., 2019; Bastiaanssen et al., 2019; Behzadi et al., 2024; Petakh et al., 2024b). This axis involves bidirectional communication between the brain, gut, and gut microbiome through various pathways, including nervous, endocrine, and immune signaling pathways (Cryan and Dinan, 2012).
Both chronic and acute stressors can alter the composition of gut bacteria in various areas and environments, including the interior (lumen) and the lining (mucosal) of the gut (Bailey et al., 2011; Galley et al., 2014; Madison and Kiecolt-Glaser, 2019). Chronic stress has also been linked to lasting changes in the gut microbiota in animal models. These changes can lead to variations in alpha diversity, which seem to depend on the specific characteristics of the stressors (Geng et al., 2019). For instance, research has shown that students under academic stress have a gut microbiota with a lower abundance of beneficial bacteria (Knowles et al., 2008). Additionally, recent evidence has demonstrated an imbalance in the microbiota of frontline healthcare workers who experienced psychological stress while working during the COVID-19 pandemic (Gao et al., 2022). Both acute and chronic stress can lead to structural and functional alterations in the human gut microbiota (Ma et al., 2023; Figure 1).
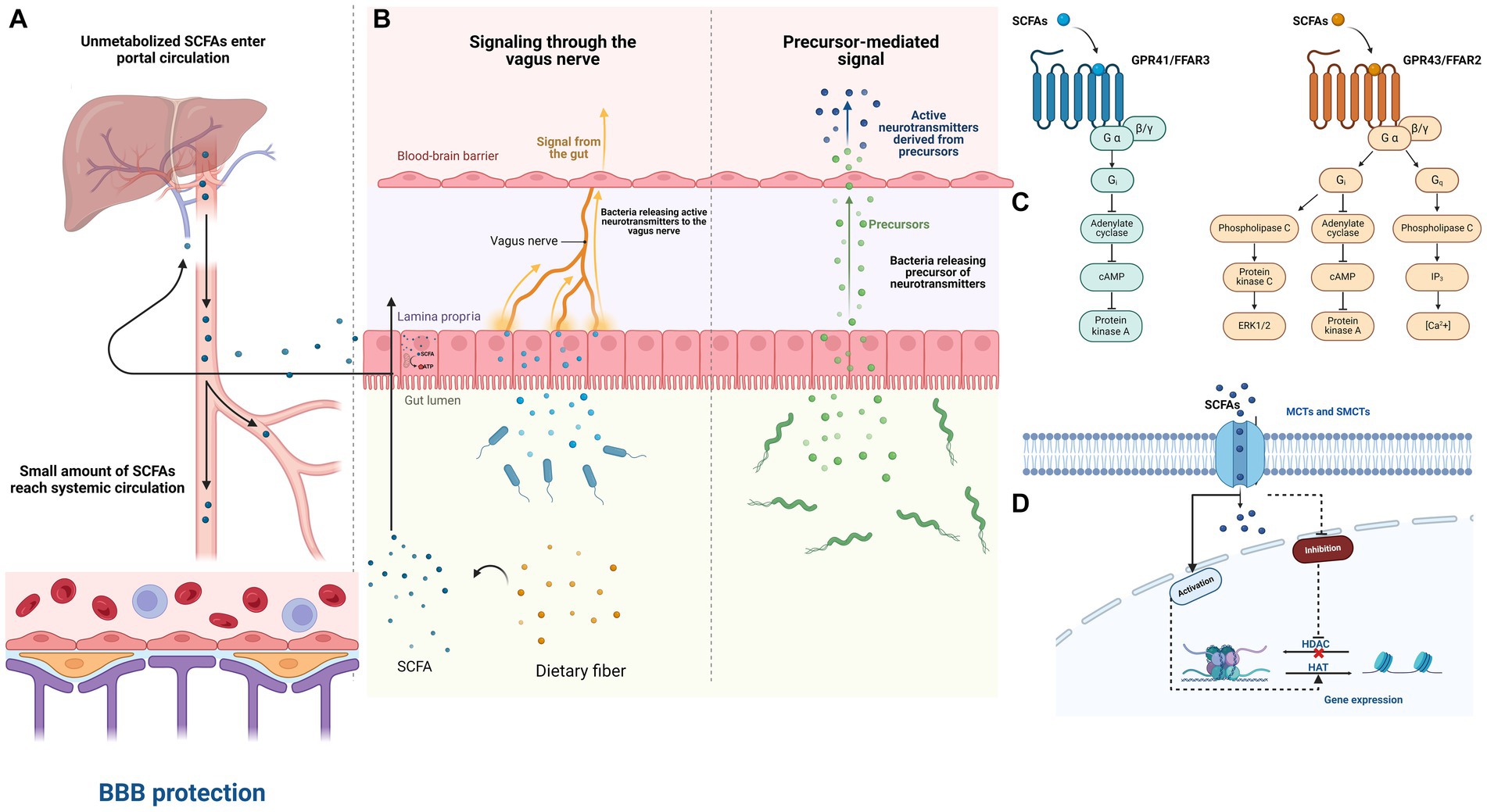
Figure 1. The impact of SCFAs on the Microbiota–Gut–Brain axis in PTSD patients. (A) The breakdown of dietary fiber by beneficial gut bacteria in the colon results in the production of short-chain fatty acids (SCFAs). These SCFAs are quickly absorbed by colon cells through various mechanisms and are partially utilized for cellular energy production. Any remaining SCFAs enter the bloodstream and are utilized as energy by liver cells or incorporated into metabolic processes. Consequently, only a limited quantity of SCFAs generated in the colon enter systemic circulation. (B) The microbiota can influence the brain through various mechanisms, such as microorganism-produced neurotransmitters affecting the brain via the vagus nerve and its afferent nerves. Additionally, neurotransmitter precursors may pass through the blood–brain barrier and be converted into active neurotransmitters. (C) SCFAs potentially impact interactions between the microbiota, gut, and brain by communicating with the host through the free fatty acid receptors (FFARs) FFAR2 and FFAR3. (D) Intracellular short-chain fatty acids (SCFAs) also play a role in histone acetylation and deacetylation, which affects gene transcription. This can occur by inhibiting histone deacetylases (HDACs), leading to more active chromatin for transcription, or by enhancing the activity of histone acetyltransferases (HATs), thus promoting acetylation. These processes may occur not only in colonocytes but also in any cell accessible to SCFAs within a tissue, either indirectly through FFARs or directly through monocarboxylate transporters (MCTs) and sodium-dependent monocarboxylate transporters (SMCTs).
Rodent models have shown that the gut microbiome affects stress-related neurocircuitry, feeding behavior, and obesity through neuroimmune-neuroendocrine pathways (Bastiaanssen et al., 2021). Concerning PTSD, both human studies and animal experiments suggest a brain-gut connection, with the gut microbiota influencing amygdala development and response, which is relevant because the amygdala plays a central role in stress and PTSD (Buffington et al., 2016; Gorecki et al., 2019; Chen et al., 2020; Ousdal et al., 2020).
PTSD-related symptoms such as anxiety, depression, and insomnia are also connected with the gut microbiota (Madison and Kiecolt-Glaser, 2019; Nikolova et al., 2021). Recently, studies have shown a connection between intestinal dysbacteriosis and stress as well as anxiety (Kelly et al., 2016; Burokas et al., 2017). Anxiety-related disorders, such as generalized anxiety disorder (GAD), are associated with gut dysbiosis (Ritchie et al., 2023). In cases of depression and anxiety, there is a notable increase in the number of gut bacteria across various taxonomic levels, including 15 genera and 18 species (Jiang et al., 2018; Ritchie et al., 2023). Patients with GAD exhibit a decrease in the abundance of bacteria that produce SCFAs and an increase in the abundances of Escherichia-Shigella, Fusobacterium, and Ruminococcus gnavus. These modifications persisted even after the alleviation of GAD (Ritchie et al., 2023). The severity of anxiety is correlated with the abundance of Escherichia-Shigella and Bacteroides in patients with active GAD (Ritchie et al., 2023). Distinct patterns have been observed in the gut microbiome related to GAD and depression. Specifically, interactions between C-reactive protein (CRP) and bacterial families indicate possible links to these conditions. For depressive symptoms (measured by the PHQ-9), notable associations included the bacterial families Ruminococcaceae, Akkermansia, and Acidaminococcaceae. For GAD (measured by GAD-7), significant relationships were found with the bacterial orders Bacteroidales, Selenomonadales, and Clostridiales. The genus Holdemanella was significantly associated with both GAD and depression. These associations suggest that inflammation and the gut microbiota may play a role in mental health (Chen et al., 2021).
In their study, Zheng et al. discovered that individuals suffering from depression exhibited a greater proportion of Actinobacteria and Firmicutes, while the abundance of Bacteroidetes decreased (Zheng et al., 2016). Jiang et al. reported that patients with depression exhibited significantly greater Shannon index scores for intestinal microbiota than did patients in the control group (Jiang et al., 2015). The levels of Bacteroidetes, Proteobacteria, and Actinobacteria were significantly greater, while the level of Firmicutes was significantly lower in the active-MDD (Major Depressive Disorder) and responsive-MDD groups than in the healthy controls. Furthermore, there was a negative correlation between the abundance of Faecalibacterium and the severity of depression in the patients. These findings suggest that depression may be associated with a predominance of potentially harmful bacterial groups or a reduction in beneficial bacterial genera. Furthermore, a separate study revealed a reduction in the prevalence of intestinal Bifidobacteria and Lactobacilli among individuals suffering from depression (Aizawa et al., 2016). Moreover, Voigt et al. reported that circadian disorganization disrupts the composition of the intestinal microbiota (Voigt et al., 2014). Emerging evidence strongly indicates notable links between circadian rhythm disturbances and mental health conditions, such as PTSD (Agorastos et al., 2014; Olff et al., 2019). Furthermore, researchers have initiated investigations into the interplay between the circadian system and mental health (Walker 2nd et al., 2020). For instance, individuals who develop PTSD often experience more disrupted sleep patterns following a traumatic event (Tsanas et al., 2020). Conversely, sleep has been found to play a protective role in preventing the formation of intrusive memories (Kleim et al., 2016). The variability in the findings of these studies may be attributed to factors such as the size of the sample, ethnic origin, diet habits, and use of antidepressant medication among the participants.
Changes in the microbiota may impact both the nervous and immune systems, diminishing an individual’s ability to manage psychological and physical stress and rendering them more susceptible to stressful situations. Nevertheless, certain probiotics, such as Lactobacillus, Bifidobacterium, and Enterococcus, can modulate emotional and cognitive parameters by influencing the enteric nervous system and the immune system (Plaza-Díaz et al., 2017). This modulation can enhance stress resilience and produce an anti-anxiety effect.
Depression and insomnia often coexist, with many patients reporting poor sleep quality as a primary concern (Seow et al., 2016). Recent research has suggested a connection between the gut-brain axis (GBA) and the concurrent occurrence of depression and insomnia. Microbial activity in the gut leads to the production of various substances, including neurotransmitters like serotonin (5-HT), dopamine (DA), and GABA, metabolites such as SCFAs and melatonin, and also influences cytokine levels (Petra et al., 2015). These compounds influence both the enteric nervous system (ENS) and the central nervous system (CNS) either directly or indirectly by interacting with enteroendocrine cells (Ridaura and Belkaid, 2015).
Certain bacteria, such as Lactobacillus and Bifidobacterium, can produce GABA, which is linked to mood regulation. Studies on animals have shown that administering Lactobacillus rhamnosus (JB-1) leads to reduced anxiety and depression-like behaviors, along with changes in GABA receptor expression in the brain, akin to the effects of benzodiazepines (Bravo et al., 2011; Cryan et al., 2019). However, these effects were not observed in animals with severed vagus nerves, indicating the importance of this nerve pathway in gut–brain communication (Schulze et al., 2014). Lactobacilli can produce SCFAs by fermenting carbohydrates. This involves the use of pyruvate from glycolysis or, under heterofermentative conditions, the phosphoketolase pathway (Pessione, 2012).
In summary, there is a two-way relationship linking the gut microbiome with both sleep patterns and depression. Inflammation and hormonal changes are pivotal in this dynamic. Initially, disruptions in the body’s circadian rhythms, sleep disturbances, and depressive symptoms impact the metabolism of resident gut bacteria, leading to alterations in their composition (Thaiss et al., 2014). Gut bacteria can influence the integrity of epithelial cell junctions, thereby regulating intestinal permeability and safeguarding the intestinal barrier (Yarandi et al., 2016).
A study revealed that 4 mmol/L butyrate increased the relative expression of occludin and ZO-1 mRNA in IPEC-J2 cells and claudin-1 mRNA in rat cdx2-IEC cells (Ma et al., 2012; Wang et al., 2012). This led to reduced intestinal permeability and greater villus height in the mice. Tong et al. (2016) reported that propionic acid elevated the levels of intestinal tight junction proteins such as ZO-1, occludin, and cadherin, which supported improved intestinal function (Tong et al., 2016). SCFAs were also shown to activate the AMPK pathway, thereby boosting the expression of ZO-1 in intestinal epithelial cells (Voltolini et al., 2012; Tong et al., 2016). This action enhances transepithelial electrical resistance (TEER) and contributes to the protection of intestinal barrier integrity. Huang et al. (2015) reported that sodium butyrate considerably increased the occludin protein in the jejunum and colon of weaned piglets, decreasing diarrhea through decreased intestinal permeability (Huang et al., 2015). Studies have also shown that butyrate can control the growth, apoptosis, and differentiation of the gastrointestinal epithelium (Miao et al., 2016). Butyrate improved barrier function by positively regulating the expression of claudin-1, ZO-1, and occludin in Cdx2-IECs and Caco-2 cells (Wang et al., 2012). This led to an increase in TEER. The effect of butyrate on the epithelial barrier may be due to the upregulation of TJ proteins through the activation of AMP-activated protein kinase (Peng et al., 2009).
When this barrier breaks down, harmful bacteria and their byproducts may infiltrate mesenteric lymph tissue, inciting inflammatory immune responses and activating the vagus and spinal afferent nerves (Hsiao et al., 2013) and further impacting the central nervous system, potentially contributing to or exacerbating insomnia and depression (Yi and Li, 2012).
4 Differences in SCFA and bacterial profiles in PTSD patients
Emerging research has focused on the gut microbiota, recognizing its potential role in modulating stress responses and mental health outcomes. Here, we delve into the key findings from recent studies investigating the relationship between gut microbiota composition and PTSD severity.
Hemmings et al. (2017) and Malan-Muller et al. (2022) both conducted studies within South African cohorts, shedding light on distinct microbial signatures associated with PTSD. Hemmings et al. identified Actinobacteria, Lentisphaerae, and Verrucomicrobia as potential discriminators between PTSD-diagnosed individuals and trauma-exposed controls (Hemmings et al., 2017). Notably, Malan-Muller et al. reported that increased levels of certain genera, such as Mitsuokella, Odoribacter, Catenibacterium, and Olsenella, were linked to worsening PTSD (Malan-Muller et al., 2022).
A study by Bajaj et al. (2019) examined male cirrhotic veterans who had been in combat. They found that the microbiota was less diverse, had more pathobionts (Enterococcus and Escherichia/Shigella), and had different amounts of autochthonous taxa (Lachnospiraceae and Ruminococcaceae) in people who had PTSD related to combat compared with non-PTSD combat-exposed patients. Importantly, despite adjusting for confounding factors, functional alterations persisted, suggesting a direct link between gut dysbiosis and PTSD pathogenesis (Bajaj et al., 2019).
Ella Levert-Levitt and colleagues discovered that a distinct microbiota pattern characterized by reduced levels of certain bacteria, such as sp_HMT_914, sp_HMT_332, and sp_871, along with Noxia, was associated with the severity of PTSD symptoms, including intrusive thoughts, heightened arousal, and reactivity, as well as other psychological issues, such as anxiety, hostility, memory problems, and unexplained pain (Levert-Levitt et al., 2022).
Feldman et al. (2022) examined mother–child pairs and found that microbial trauma profiles, especially those from the genera Dialister and Veillonella, were linked to trauma-related phenotypes (Feldman et al., 2022). Finally, Yoo et al. (2023) investigated the gut microbiota of firefighters, revealing associations between specific bacterial taxa (Lachnospiraceae blautia, Lachnospiraceae coprococcus, and Alistipes onderdonkii) and PTSD symptom severity. Interestingly, they identified interactions between bacterial abundance and PTSD severity scores, offering insights into potential microbial biomarkers for PTSD risk assessment and personalized interventions (Table 1; Yoo et al., 2023).
Lachnospiraceae are SCFA-producing microorganisms (Socała et al., 2021). They have anti-inflammatory and modulatory effects on the intestinal mucosa, contributing to gut health. However, two studies have reported a significant decrease in this bacterial taxon, which might impact the levels of SCFAs.
5 Mechanisms of action: how SCFAs influence the CNS
SCFAs play a crucial role in promoting gut health by exerting various local effects. Specifically, they help maintain the integrity of the intestinal barrier and offer protection against inflammation within the intestine (Lewis et al., 2010).
Bacterial strains in the gut can modulate neurotransmitter levels, influencing microglial activation and cerebral functions. Gut microbiota-CNS communication involves the sympathetic branch of the autonomic nervous system, which encompasses sensory fibers capable of conveying signals from the gut to the CNS (Rhee et al., 2009; Kelly et al., 2017). SCFAs, which are bacterial metabolic byproducts, are considered key mediators of gut–brain communication, and altered SCFA production is observed in various neuropathologies (Tan et al., 2014).
In a cohort study from the Flemish Gut Flora Project, butyrate-producing bacteria, including Faecalibacterium and Coprococcus, were positively associated with higher quality of life indicators (Valles-Colomer et al., 2019). Conversely, those with major depressive disorder (MDD) tend to have increased levels of Prevotella and Bifidobacterium, which are associated with this condition and can be easily assessed for diagnostic and therapeutic purposes (Lin et al., 2017; Rong et al., 2019). The abundances of certain bacterial families, such as Lachnospiraceae and Ruminococcaceae, particularly genera such as Roseburia and Blautia, which are known for SCFA production from carbohydrate breakdown, are reduced in individuals with MDD relative to healthy individuals (Jiang et al., 2015). Recent research has also suggested the potential involvement of SCFAs in depression, with depressed individuals in Poland showing lower fecal propionate content and higher isocaproate levels (Skonieczna-Żydecka et al., 2018). These findings were associated with specific depressive symptoms, although the small sample size and the presence of other medical conditions among the participants need to be considered.
Persistent stress significantly increases the likelihood of developing neuropsychiatric disorders, with emerging research highlighting the crucial role of the microbiome-gut-brain axis in mediating this relationship (Ramirez et al., 2017; Cruz-Pereira et al., 2020). Recent clinical studies indicate that administering SCFAs directly to the colon may regulate the fundamental responsiveness of the hypothalamic–pituitary–adrenal (HPA) axis to psychosocial stress (Dalile et al., 2020). Animal studies have robustly demonstrated that prolonged stress disrupts the composition of the gut microbiome, while interventions targeting the microbiota can mitigate the physiological and neurological impacts of stress (Burokas et al., 2017). Several preclinical investigations revealed that supplementing mice subjected to extended psychosocial stress with acetate, butyrate, and propionate had positive effects on both behavior and stress-induced gut permeability (van de Wouw et al., 2018).
6 Psychobiotic-based interventions for PTSD
In 2013, the term “psychobiotics” was coined to describe “live microorganisms that, when consumed in sufficient quantities, confer health benefits to individuals experiencing psychiatric disorders” (Dinan et al., 2013).
A pilot randomized controlled trial investigated the feasibility, acceptability, and safety of supplementing veterans with clinically significant persistent postconcussive (PPC) and posttraumatic stress disorder (PTSD) symptoms using Lactobacillus reuteri DSM 17938. Thirty-one participants were randomized to either the probiotic or placebo group for 8 weeks. Probiotic supplementation demonstrated promising results, meeting feasibility and safety thresholds. While the decrease in plasma C-reactive protein (CRP) levels approached statistical significance, the placebo group exhibited a significantly greater increase in heart rate during the Trier Social Stress Test (TSST) math task. These findings suggest that L. reuteri DSM 17938 may have anti-inflammatory effects and impact stress responsiveness (Brenner et al., 2020).
A study involving ten combat veterans diagnosed with PTSD revealed that consistent intake of a fermented soy formulation (FSWW08) over a six-month period resulted in decreased symptoms such as anxiety, derealization/detachment, general infection, headache, loss of appetite, panic, upper gastrointestinal burning, and upper respiratory infection (Gocan et al., 2012). It is important to note that the study’s ability to establish causal relationships is limited due to the absence of a control group, the small sample size, potential selection biases, and reporting biases.
A study conducted on mice revealed that the administration of L. rhamnosus JB-1 as an early intervention (within 48 h of the traumatic event) for PTSD could have an adverse impact by enhancing fear. This suggests that the timing of stress and exposure to the bacterium may play a crucial role in determining its therapeutic effectiveness. Interestingly, the same study revealed comparable unfavorable outcomes when the mouse model received early treatment with sertraline, a frequently prescribed SSRI for PTSD (Liu et al., 2020). Another notable finding from C. Lowry’s group involving Mycobacterium vaccae is its potential to enhance fear extinction in PTSD-related paradigms. Specifically, immunization with Mycobacterium vaccae postfear conditioning led to enhanced fear extinction in rats (Hassell et al., 2019).
Another promising intervention for PTSD could be postbiotics, a concept formally proposed by Tsilingiri and Rescigno (2013). Postbiotics encompass any factors derived from the metabolic activity of probiotics or any released molecules that can directly or indirectly aid the host. These may include intentionally inactivated microbial cells with or without metabolites or cell components that contribute to health benefits, excluding pure microbial metabolites and vaccinations. In recent years, researchers have identified numerous distinct chemical types of postbiotics originating from bacterial cells, both within and outside the host organism. Postbiotics hold significant potential for future therapeutic applications (Lou et al., 2023).
7 Future directions and challenges
In addressing the identified limitations and increasing the scientific robustness of research in this domain, several key directions for future studies are proposed. First, large human cohorts encompassing comprehensive data on both the gut microbiome and PTSD, along with an array of potential host factors such as demographic, socioeconomic, and health variables, are recommended. Second, to detect dynamic changes in the microbiota of patients with PTSD, longitudinal assessments rather than relying solely on single stool samples are recommended. Furthermore, initiating these longitudinal studies prior to trauma exposure is crucial for obtaining a comprehensive understanding. For instance, in a war scenario, evaluating soldiers’ predeployment allows monitoring of their microbiota and other biological markers over time, enabling the examination of PTSD development in conjunction with these factors. Similarly, in the general population, studying birth cohorts followed up for many years permits the evaluation of PTSD progression alongside the microbiome and other biological signatures. This approach is essential for disentangling causes and consequences in human studies of PTSD. Third, we recommend the use of whole-metagenome shotgun (WMS) sequencing data to obtain comprehensive taxonomic and functional profiles of the gut microbiome. Metagenomic sequencing offers a more detailed understanding of microbial communities, enabling clinicians to identify specific microbial functions and pathways associated with PTSD. This knowledge could inform targeted interventions aimed at modulating the gut microbiome to improve PTSD outcomes. Fourth, the integration of multiomics data, including metagenomic, metatranscriptomic, and metabolomic data, is proposed to systematically elucidate the mechanisms linking the intestine and PTSD, considering previous findings associating abnormal intestinal conditions with PTSD.
Furthermore, there is an emphasis on enhancing causal inference through novel computational/statistical methods to identify potential PTSD-associated species and pathways. This approach facilitates the rational design of synbiotics (combinations of specific probiotics and prebiotics) for the potential amelioration of PTSD symptoms. Finally, a combination of standardized and varied animal models could be used to explore the relationships between stress-related behavioral changes and the gut microbiome. These proposed directions have three significant implications for future research and practice. First, these findings signify a significant progression by offering insights into whether there are differences in the gut microbiome between individuals with PTSD and those who have experienced trauma alone. This analysis must be conducted with meticulous consideration of various potential host factors. Furthermore, recent findings indicate that PTSD is linked to impaired immune and inflammatory function, various chronic illnesses, and cognitive impairments, all of which have been connected to an altered microbiome. This connection underscores the importance of further research into the role of the gut microbiome in PTSD. Understanding this relationship could lead to new therapeutic strategies, such as microbiome-targeted treatments, to improve immune and inflammatory responses and potentially alleviate some of the chronic conditions and cognitive symptoms associated with PTSD.
Author contributions
PP: Visualization, Writing – original draft. KD: Writing – review & editing. VO: Writing – review & editing. PB: Writing – review & editing. OK: Supervision, Writing – review & editing.
Funding
The author(s) declare that no financial support was received for the research, authorship, and/or publication of this article.
Conflict of interest
The authors declare that the research was conducted in the absence of any commercial or financial relationships that could be construed as potential conflicts of interest.
The author(s) declared that they were an editorial board member of Frontiers, at the time of submission. This had no impact on the peer review process and the final decision.
Publisher’s note
All claims expressed in this article are solely those of the authors and do not necessarily represent those of their affiliated organizations, or those of the publisher, the editors and the reviewers. Any product that may be evaluated in this article, or claim that may be made by its manufacturer, is not guaranteed or endorsed by the publisher.
References
Adan, R. A. H., van der Beek, E. M., Buitelaar, J. K., Cryan, J. F., Hebebrand, J., Higgs, S., et al. (2019). Nutritional psychiatry: towards improving mental health by what you eat. Eur. Neuropsychopharmacol. 29, 1321–1332. doi: 10.1016/j.euroneuro.2019.10.011
Agorastos, A., Kellner, M., Baker, D. G., and Otte, C. (2014). When time stands still: an integrative review on the role of chronodisruption in posttraumatic stress disorder. Curr. Opin. Psychiatry 27, 385–392. doi: 10.1097/YCO.0000000000000079
Aizawa, E., Tsuji, H., Asahara, T., Takahashi, T., Teraishi, T., Yoshida, S., et al. (2016). Possible association of Bifidobacterium and Lactobacillus in the gut microbiota of patients with major depressive disorder. J. Affect. Disord. 202, 254–257. doi: 10.1016/j.jad.2016.05.038
American Psychiatric Association D, Association AP (2013). Diagnostic and statistical manual of mental disorders: DSM-5. Washington, DC: American Psychiatric Association.
Bachmann, C., Colombo, J. P., and Berüter, J. (1979). Short chain fatty acids in plasma and brain: quantitative determination by gas chromatography; Clinica chimica acta. Int. J. Clin. Chem. 92, 153–159. doi: 10.1016/0009-8981(79)90109-8
Bailey, M. T., Dowd, S. E., Galley, J. D., Hufnagle, A. R., Allen, R. G., and Lyte, M. (2011). Exposure to a social stressor alters the structure of the intestinal microbiota: implications for stressor-induced immunomodulation. Brain Behav. Immun. 25, 397–407. doi: 10.1016/j.bbi.2010.10.023
Bajaj, J. S., Sikaroodi, M., Fagan, A., Heuman, D., Gilles, H., Gavis, E. A., et al. (2019). Posttraumatic stress disorder is associated with altered gut microbiota that modulates cognitive performance in veterans with cirrhosis. Am. J. Physiol. Gastrointest. Liver Physiol. 317, G661–G669. doi: 10.1152/ajpgi.00194.2019
Bartolomaeus, H., Balogh, A., Yakoub, M., Homann, S., Markó, L., Höges, S., et al. (2019). Short-chain fatty acid propionate protects from hypertensive cardiovascular damage. Circulation 139, 1407–1421. doi: 10.1161/CIRCULATIONAHA.118.036652
Bastiaanssen, T. F. S., Cowan, C. S. M., Claesson, M. J., Dinan, T. G., and Cryan, J. F. (2019). Making sense of … the microbiome in psychiatry. Int. J. Neuropsychopharmacol. 22, 37–52. doi: 10.1093/ijnp/pyy067
Bastiaanssen, T. F. S., Gururajan, A., van de Wouw, M., Moloney, G. M., Ritz, N. L., Long-Smith, C. M., et al. (2021). Volatility as a concept to understand the impact of stress on the microbiome. Psychoneuroendocrinology 124:105047. doi: 10.1016/j.psyneuen.2020.105047
Behzadi, P., Dodero, V. I., and Golubnitschaja, O. (2024). “Systemic inflammation as the health-related communication tool between the human host and gut microbiota in the framework of predictive, preventive, and personalized medicine” in All around suboptimal health: advanced approaches by predictive, preventive and personalised medicine for healthy populations. ed. W. Wang (Cham: Springer Nature Switzerland).
Binder, H. J. (2010). Role of colonic short-chain fatty acid transport in diarrhea. Annu. Rev. Physiol. 72, 297–313. doi: 10.1146/annurev-physiol-021909-135817
Bose, P., Dai, Y., and Grant, S. (2014). Histone deacetylase inhibitor (HDACI) mechanisms of action: emerging insights. Pharmacol. Ther. 143, 323–336. doi: 10.1016/j.pharmthera.2014.04.004
Böttke, T., Ernicke, S., Serfling, R., Ihling, C., Burda, E., Gurevich, V. V., et al. (2020). Exploring GPCR-arrestin interfaces with genetically encoded crosslinkers. EMBO Rep. 21:e50437. doi: 10.15252/embr.202050437
Bravo, J. A., Forsythe, P., Chew, M. V., Escaravage, E., Savignac, H. M., Dinan, T. G., et al. (2011). Ingestion of Lactobacillus strain regulates emotional behavior and central GABA receptor expression in a mouse via the vagus nerve. Proc. Natl. Acad. Sci. USA 108, 16050–16055. doi: 10.1073/pnas.1102999108
Brenner, L. A., Forster, J. E., Stearns-Yoder, K. A., Stamper, C. E., Hoisington, A. J., Brostow, D. P., et al. (2020). Evaluation of an immunomodulatory probiotic intervention for veterans with co-occurring mild traumatic brain injury and posttraumatic stress disorder: a pilot study. Front. Neurol. 11:1015. doi: 10.3389/fneur.2020.01015
Brown, A. J., Goldsworthy, S. M., Barnes, A. A., Eilert, M. M., Tcheang, L., Daniels, D., et al. (2003). The orphan G protein-coupled receptors GPR41 and GPR43 are activated by propionate and other short chain carboxylic acids. J. Biol. Chem. 278, 11312–11319. doi: 10.1074/jbc.M211609200
Buffington, S. A., Di Prisco, G. V., Auchtung, T. A., Ajami, N. J., Petrosino, J. F., and Costa-Mattioli, M. (2016). Microbial reconstitution reverses maternal diet-induced social and synaptic deficits in offspring. Cell 165, 1762–1775. doi: 10.1016/j.cell.2016.06.001
Burokas, A., Arboleya, S., Moloney, R. D., Peterson, V. L., Murphy, K., Clarke, G., et al. (2017). Targeting the microbiota-gut-brain Axis: prebiotics have anxiolytic and antidepressant-like effects and reverse the impact of chronic stress in mice. Biol. Psychiatry 82, 472–487. doi: 10.1016/j.biopsych.2016.12.031
Cait, A., Hughes, M. R., Antignano, F., Cait, J., Dimitriu, P. A., Maas, K. R., et al. (2018). Microbiome-driven allergic lung inflammation is ameliorated by short-chain fatty acids. Mucosal Immunol. 11, 785–795. doi: 10.1038/mi.2017.75
Chen, Y., Fang, L., Chen, S., Zhou, H., Fan, Y., Lin, L., et al. (2020). Gut microbiome alterations precede cerebral amyloidosis and microglial pathology in a mouse model of Alzheimer’s disease. J. BioMed. Res. Int. 2020, 1–15. doi: 10.1155/2020/8456596
Chen, Y., Meng, P., Cheng, S., Jia, Y., Wen, Y., Yang, X., et al. (2021). Assessing the effect of interaction between C-reactive protein and gut microbiome on the risks of anxiety and depression. Mol. Brain 14:133. doi: 10.1186/s13041-021-00843-1
Cruz-Pereira, J. S., Rea, K., Nolan, Y. M., O’Leary, O. F., Dinan, T. G., and Cryan, J. F. (2020). Depression’s unholy trinity: dysregulated stress, immunity, and the microbiome. Annu. Rev. Psychol. 71, 49–78. doi: 10.1146/annurev-psych-122216-011613
Cryan, J. F., and Dinan, T. G. (2012). Mind-altering microorganisms: the impact of the gut microbiota on brain and behaviour. Nat. Rev. Neurosci. 13, 701–712. doi: 10.1038/nrn3346
Cryan, J. F., O’Riordan, K. J., Cowan, C. S. M., Sandhu, K. V., Bastiaanssen, T. F. S., Boehme, M., et al. (2019). The microbiota-gut-brain Axis. Physiol. Rev. 99, 1877–2013. doi: 10.1152/physrev.00018.2018
Dalile, B., Vervliet, B., Bergonzelli, G., Verbeke, K., and Van Oudenhove, L. (2020). Colon-delivered short-chain fatty acids attenuate the cortisol response to psychosocial stress in healthy men: a randomized, placebo-controlled trial. Neuropsychopharmacology 45, 2257–2266. doi: 10.1038/s41386-020-0732-x
Dinan, T. G., Stanton, C., and Cryan, J. F. (2013). Psychobiotics: a novel class of psychotropic. Biol. Psychiatry 74, 720–726. doi: 10.1016/j.biopsych.2013.05.001
Elfiky, A. M. I., Ghiboub, M., Li Yim, A. Y. F., Hageman, I. L., Verhoeff, J., de Krijger, M., et al. (2022). Carboxylesterase-1 assisted targeting of HDAC inhibitors to mononuclear myeloid cells in inflammatory bowel disease. J. Crohns Colitis 16, 668–681. doi: 10.1093/ecco-jcc/jjab176
Feldman, R., Yirmiya, K., Turjeman, S., Shtossel, O., Zagoory-Sharon, O., Moadi, L., et al. (2022). Microbiome Mediates Development of PTSD and Resilience. doi: 10.21203/rs.3.rs-1940296/v1
Friedrich, M., Gerbeth, L., Gerling, M., Rosenthal, R., Steiger, K., Weidinger, C., et al. (2019). HDAC inhibitors promote intestinal epithelial regeneration via autocrine TGFβ1 signalling in inflammation. Mucosal Immunol. 12, 656–667. doi: 10.1038/s41385-019-0135-7
Gaidarov, I., Chen, X., Anthony, T., Maciejewski-Lenoir, D., Liaw, C., and Unett, D. J. (2013). Differential tissue and ligand-dependent signaling of GPR109A receptor: implications for anti-atherosclerotic therapeutic potential. Cell. Signal. 25, 2003–2016. doi: 10.1016/j.cellsig.2013.06.008
Galley, J. D., Yu, Z., Kumar, P., Dowd, S. E., Lyte, M., and Bailey, M. T. (2014). The structures of the colonic mucosa-associated and luminal microbial communities are distinct and differentially affected by a prolonged murine stressor. Gut Microbes 5, 748–760. doi: 10.4161/19490976.2014.972241
Gao, F., Guo, R., Ma, Q., Li, Y., Wang, W., Fan, Y., et al. (2022). Stressful events induce long-term gut microbiota dysbiosis and associated post-traumatic stress symptoms in healthcare workers fighting against COVID-19. J. Affect. Disord. 303, 187–195. doi: 10.1016/j.jad.2022.02.024
Gautam, A., D’Arpa, P., Donohue, D. E., Muhie, S., Chakraborty, N., Luke, B. T., et al. (2015). Acute and chronic plasma metabolomic and liver transcriptomic stress effects in a mouse model with features of post-traumatic stress disorder. PLoS One 10:e0117092. doi: 10.1371/journal.pone.0117092
Geng, S., Yang, L., Cheng, F., Zhang, Z., Li, J., Liu, W., et al. (2019). Gut microbiota are associated with psychological stress-induced defections in intestinal and blood-brain barriers. Front. Microbiol. 10:3067. doi: 10.3389/fmicb.2019.03067
Gocan, A. G., Bachg, D., Schindler, A. E., and Rohr, U. D. (2012). Balancing steroidal hormone cascade in treatment-resistant veteran soldiers with PTSD using a fermented soy product (FSWW08): a pilot study. Horm. Mol. Biol. Clin. Invest. 10, 301–314. doi: 10.1515/hmbci-2011-0135
Gorecki, A. M., Preskey, L., Bakeberg, M. C., Kenna, J. E., Gildenhuys, C., MacDougall, G., et al. (2019). Altered gut microbiome in Parkinson’s disease and the influence of lipopolysaccharide in a human α-Synuclein over-expressing mouse model. Front. Neurosci. 13:839. doi: 10.3389/fnins.2019.00839
Gradus, J. L., and Galea, S. (2022). Reconsidering the definition of trauma. Lancet Psychiatry 9, 608–609. doi: 10.1016/S2215-0366(22)00196-1
Grundmann, M., Bender, E., Schamberger, J., and Eitner, F. (2021). Pharmacology of free fatty acid receptors and their allosteric modulators. Int. J. Mol. Sci. 22, 1763–1801. doi: 10.3390/ijms22041763
Hassell, J. E. Jr., Fox, J. H., Arnold, M. R., Siebler, P. H., Lieb, M. W., Schmidt, D., et al. (2019). Treatment with a heat-killed preparation of Mycobacterium vaccae after fear conditioning enhances fear extinction in the fear-potentiated startle paradigm. Brain Behav. Immun. 81, 151–160. doi: 10.1016/j.bbi.2019.06.008
Hemmings, S. M. J., Malan-Müller, S., van den Heuvel, L. L., Demmitt, B. A., Stanislawski, M. A., Smith, D. G., et al. (2017). The microbiome in posttraumatic stress disorder and trauma-exposed controls: an exploratory study. Psychosom. Med. 79, 936–946. doi: 10.1097/PSY.0000000000000512
Higashimura, Y., Naito, Y., Takagi, T., Uchiyama, K., Mizushima, K., and Yoshikawa, T. (2015). Propionate promotes fatty acid oxidation through the up-regulation of peroxisome proliferator-activated receptor α in intestinal epithelial cells. J. Nutr. Sci. Vitaminol. 61, 511–515. doi: 10.3177/jnsv.61.511
Holota, Y., Dovbynchuk, T., Kaji, I., Vareniuk, I., Dzyubenko, N., Chervinska, T., et al. (2019). The long-term consequences of antibiotic therapy: role of colonic short-chain fatty acids (SCFA) system and intestinal barrier integrity. PLoS One 14:e0220642. doi: 10.1371/journal.pone.0220642
Hsiao, E. Y., McBride, S. W., Hsien, S., Sharon, G., Hyde, E. R., McCue, T., et al. (2013). Microbiota modulate behavioral and physiological abnormalities associated with neurodevelopmental disorders. Cell 155, 1451–1463. doi: 10.1016/j.cell.2013.11.024
Hsu, C. N., Chang-Chien, G. P., Lin, S., Hou, C. Y., and Tain, Y. L. (2019). Targeting on gut microbial metabolite trimethylamine-N-oxide and Short-chain fatty acid to prevent maternal high-fructose-diet-induced developmental programming of hypertension in adult male offspring. Mol. Nutr. Food Res. 63:e1900073. doi: 10.1002/mnfr.201900073
Huang, C., Song, P., Fan, P., Hou, C., Thacker, P., and Ma, X. (2015). Dietary sodium butyrate decreases Postweaning diarrhea by modulating intestinal permeability and changing the bacterial communities in weaned piglets. J. Nutr. 145, 2774–2780. doi: 10.3945/jn.115.217406
Jiang, H., Ling, Z., Zhang, Y., Mao, H., Ma, Z., Yin, Y., et al. (2015). Altered fecal microbiota composition in patients with major depressive disorder. Brain Behav. Immun. 48, 186–194. doi: 10.1016/j.bbi.2015.03.016
Jiang, H.-y., Zhang, X., Yu, Z.-h., Zhang, Z., Deng, M., Zhao, J.-h., et al. (2018). Altered gut microbiota profile in patients with generalized anxiety disorder. J. Psychiatr. Res. 104, 130–136. doi: 10.1016/j.jpsychires.2018.07.007
Jiao, A., Yu, B., He, J., Yu, J., Zheng, P., Luo, Y., et al. (2021). Sodium acetate, propionate, and butyrate reduce fat accumulation in mice via modulating appetite and relevant genes. Nutrition 87-88:111198. doi: 10.1016/j.nut.2021.111198
Kaji, I., Iwanaga, T., Watanabe, M., Guth, P. H., Engel, E., Kaunitz, J. D., et al. (2015). SCFA transport in rat duodenum. Am. J. Physiol. Gastrointest. Liver Physiol. 308, G188–G197. doi: 10.1152/ajpgi.00298.2014
Ke, S., Hartmann, J., Ressler, K. J., Liu, Y. Y., and Koenen, K. C. (2023). The emerging role of the gut microbiome in posttraumatic stress disorder. Brain Behav. Immun. 114, 360–370. doi: 10.1016/j.bbi.2023.09.005
Kekuda, R., Manoharan, P., Baseler, W., and Sundaram, U. (2013). Monocarboxylate 4 mediated butyrate transport in a rat intestinal epithelial cell line. Dig. Dis. Sci. 58, 660–667. doi: 10.1007/s10620-012-2407-x
Kelly, J. R., Clarke, G., Cryan, J. F., and Dinan, T. G. (2016). Brain-gut-microbiota axis: challenges for translation in psychiatry. Ann. Epidemiol. 26, 366–372. doi: 10.1016/j.annepidem.2016.02.008
Kelly, J. R., Minuto, C., Cryan, J. F., Clarke, G., and Dinan, T. G. (2017). Cross talk: the microbiota and neurodevelopmental disorders. Front. Neurosci. 11:490. doi: 10.3389/fnins.2017.00490
Kim, S. W., Hooker, J. M., Otto, N., Win, K., Muench, L., Shea, C., et al. (2013). Whole-body pharmacokinetics of HDAC inhibitor drugs, butyric acid, valproic acid and 4-phenylbutyric acid measured with carbon-11 labeled analogs by PET. Nucl. Med. Biol. 40, 912–918. doi: 10.1016/j.nucmedbio.2013.06.007
Kleim, B., Wysokowsky, J., Schmid, N., Seifritz, E., and Rasch, B. (2016). Effects of sleep after experimental trauma on intrusive emotional memories. Sleep 39, 2125–2132. doi: 10.5665/sleep.6310
Knowles, S. R., Nelson, E. A., and Palombo, E. A. (2008). Investigating the role of perceived stress on bacterial flora activity and salivary cortisol secretion: a possible mechanism underlying susceptibility to illness. Biol. Psychol. 77, 132–137. doi: 10.1016/j.biopsycho.2007.09.010
Kyzar, E. J., Purpura, L. J., Shah, J., Cantos, A., Nordvig, A. S., and Yin, M. T. (2021). Anxiety, depression, insomnia, and trauma-related symptoms following COVID-19 infection at long-term follow-up. Brain Behav. Immun. Health 16:100315. doi: 10.1016/j.bbih.2021.100315
Le Poul, E., Loison, C., Struyf, S., Springael, J. Y., Lannoy, V., Decobecq, M. E., et al. (2003). Functional characterization of human receptors for short chain fatty acids and their role in polymorphonuclear cell activation. J. Biol. Chem. 278, 25481–25489. doi: 10.1074/jbc.M301403200
Levert-Levitt, E., Shapira, G., Sragovich, S., Shomron, N., Lam, J. C. K., Li, V. O. K., et al. (2022). Oral microbiota signatures in post-traumatic stress disorder (PTSD) veterans. Mol. Psychiatry 27, 4590–4598. doi: 10.1038/s41380-022-01704-6
Lewis, K., Lutgendorff, F., Phan, V., Söderholm, J. D., Sherman, P. M., and McKay, D. M. (2010). Enhanced translocation of bacteria across metabolically stressed epithelia is reduced by butyrate. Inflamm. Bowel Dis. 16, 1138–1148. doi: 10.1002/ibd.21177
Li, M., van Esch, B., Henricks, P. A. J., Folkerts, G., and Garssen, J. (2018). The anti-inflammatory effects of Short chain fatty acids on lipopolysaccharide- or tumor necrosis factor α-stimulated endothelial cells via activation of GPR41/43 and inhibition of HDACs. Front. Pharmacol. 9:533. doi: 10.3389/fphar.2018.00533
Li, M., Wang, J., Wang, F., Strappe, P., Liu, W., Zheng, J., et al. (2021). Microbiota fermentation characteristics of acylated starches and the regulation mechanism of short-chain fatty acids on hepatic steatosis. Food Funct. 12, 8659–8668. doi: 10.1039/D1FO01226F
Li, B. Y., Xu, X. Y., Gan, R. Y., Sun, Q. C., Meng, J. M., Shang, A., et al. (2019). Targeting gut microbiota for the prevention and Management of Diabetes Mellitus by dietary natural products. Foods (Basel, Switzerland) 8, 440–458. doi: 10.3390/foods8100440
Li, W., Zhang, K., and Yang, H. (2018). Pectin alleviates high fat (lard) diet-induced nonalcoholic fatty liver disease in mice: possible role of Short-chain fatty acids and gut microbiota regulated by pectin. J. Agric. Food Chem. 66, 8015–8025. doi: 10.1021/acs.jafc.8b02979
Lin, P., Ding, B., Feng, C., Yin, S., Zhang, T., Qi, X., et al. (2017). Prevotella and Klebsiella proportions in fecal microbial communities are potential characteristic parameters for patients with major depressive disorder. J. Affect. Disord. 207, 300–304. doi: 10.1016/j.jad.2016.09.051
Liu, W., Luo, X., Tang, J., Mo, Q., Zhong, H., Zhang, H., et al. (2021). A bridge for short-chain fatty acids to affect inflammatory bowel disease, type 1 diabetes, and non-alcoholic fatty liver disease positively: by changing gut barrier. Eur. J. Nutr. 60, 2317–2330. doi: 10.1007/s00394-020-02431-w
Liu, Y., Steinhausen, K., Bharwani, A., Mian, M. F., McVey Neufeld, K. A., and Forsythe, P. (2020). Increased persistence of avoidance behaviour and social deficits with L.rhamnosus JB-1 or selective serotonin reuptake inhibitor treatment following social defeat. Sci. Rep. 10, 13485–13498. doi: 10.1038/s41598-020-69968-y
Lou, X., Xue, J., Shao, R., Mo, C., Wang, F., and Chen, G. (2023). Postbiotics as potential new therapeutic agents for sepsis. Burns Trauma 11:11. doi: 10.1093/burnst/tkad022
Ma, X., Fan, P., Li, L. S., Qiao, S. Y., Zhang, G. L., and Li, D. F. (2012). Butyrate promotes the recovering of intestinal wound healing through its positive effect on the tight junctions1. J. Anim. Sci. 90, 266–268. doi: 10.2527/jas.50965
Ma, L., Yan, Y., Webb, R., Li, Y., Mehrabani, S., Xin, B., et al. (2023). Psychological stress and gut microbiota composition: a systematic review of human studies. Neuropsychobiology 82, 247–262. doi: 10.1159/000533131
Madison, A., and Kiecolt-Glaser, J. K. (2019). Stress, depression, diet, and the gut microbiota: human–bacteria interactions at the core of psychoneuroimmunology and nutrition. Curr. Opin. Behav. Sci. 28, 105–110. doi: 10.1016/j.cobeha.2019.01.011
Malan-Muller, S., Valles-Colomer, M., Foxx, C. L., Vieira-Silva, S., van den Heuvel, L. L., Raes, J., et al. (2022). Exploring the relationship between the gut microbiome and mental health outcomes in a posttraumatic stress disorder cohort relative to trauma-exposed controls. Eur. Neuropsychopharmacol. 56, 24–38. doi: 10.1016/j.euroneuro.2021.11.009
McKenzie, C., Tan, J., Macia, L., and Mackay, C. R. (2017). The nutrition-gut microbiome-physiology axis and allergic diseases. Immunol. Rev. 278, 277–295. doi: 10.1111/imr.12556
McMillin, M., and DeMorrow, S. (2016). Effects of bile acids on neurological function and disease. FASEB J. 30, 3658–3668. doi: 10.1096/fj.201600275R
Meng, J. M., Cao, S. Y., Wei, X. L., Gan, R. Y., Wang, Y. F., Cai, S. X., et al. (2019). Effects and mechanisms of tea for the prevention and Management of Diabetes Mellitus and Diabetic Complications: an updated review. Antioxidants (Basel, Switzerland) 8, 170–195. doi: 10.3390/antiox8060170
Miao, W., Wu, X., Wang, K., Wang, W., Wang, Y., Li, Z., et al. (2016). Sodium butyrate promotes reassembly of tight junctions in Caco-2 monolayers involving inhibition of MLCK/MLC2 pathway and phosphorylation of PKCβ2. Int. J. Mol. Sci. 17, 1696–1708. doi: 10.3390/ijms17101696
Mitchell, R. W., On, N. H., Del Bigio, M. R., Miller, D. W., and Hatch, G. M. (2011). Fatty acid transport protein expression in human brain and potential role in fatty acid transport across human brain microvessel endothelial cells. J. Neurochem. 117, 735–746. doi: 10.1111/j.1471-4159.2011.07245.x
Mohanty, I., Mannochio-Russo, H., Schweer, J. V., El Abiead, Y., Bittremieux, W., Xing, S., et al. (2024). The underappreciated diversity of bile acid modifications. Cell 187, 1801–18.e20. doi: 10.1016/j.cell.2024.02.019
Nikolova, V. L., Smith, M. R. B., Hall, L. J., Cleare, A. J., Stone, J. M., and Young, A. H. (2021). Perturbations in gut microbiota composition in psychiatric disorders: a review and Meta-analysis. JAMA Psychiatry 78, 1343–1354. doi: 10.1001/jamapsychiatry.2021.2573
O’Hayre, M., Eichel, K., Avino, S., Zhao, X., Steffen, D. J., Feng, X., et al. (2017). Genetic evidence that β-arrestins are dispensable for the initiation of β(2)-adrenergic receptor signaling to ERK. Sci. Signal. 10. doi: 10.1126/scisignal.aal3395
Olff, M., Amstadter, A., Armour, C., Birkeland, M. S., Bui, E., Cloitre, M., et al. (2019). A decennial review of psychotraumatology: what did we learn and where are we going? Eur. J. Psychotraumatol. 10:1672948. doi: 10.1080/20008198.2019.1672948
Ousdal, O. T., Milde, A. M., Hafstad, G. S., Hodneland, E., Dyb, G., Craven, A. R., et al. (2020). The association of PTSD symptom severity with amygdala nuclei volumes in traumatized youths. Transl. Psychiatry 10:288. doi: 10.1038/s41398-020-00974-4
Pai, A., Suris, A. M., and North, C. S. (2017). Posttraumatic stress disorder in the DSM-5: controversy, change, and conceptual considerations. Behav. Sci. 7, 7–14. doi: 10.3390/bs7010007
Panagioti, M., Gooding, P. A., Triantafyllou, K., and Tarrier, N. (2015). Suicidality and posttraumatic stress disorder (PTSD) in adolescents: a systematic review and meta-analysis. Soc. Psychiatry Psychiatr. Epidemiol. 50, 525–537. doi: 10.1007/s00127-014-0978-x
Park, B. O., Kim, S. H., Kim, J. H., Kim, S. Y., Park, B. C., Han, S. B., et al. (2021). The Short-chain fatty acid receptor GPR43 modulates YAP/TAZ via RhoA. Mol. Cells 44, 458–467. doi: 10.14348/molcells.2021.0021
Peng, L., Li, Z. R., Green, R. S., Holzman, I. R., and Lin, J. (2009). Butyrate enhances the intestinal barrier by facilitating tight junction assembly via activation of AMP-activated protein kinase in Caco-2 cell monolayers. J. Nutr. 139, 1619–1625. doi: 10.3945/jn.109.104638
Pessione, E. (2012). Lactic acid bacteria contribution to gut microbiota complexity: lights and shadows. Front. Cell. Infect. Microbiol. 2:86. doi: 10.3389/fcimb.2012.00086
Petakh, P., Oksenych, V., Kamyshna, I., Boisak, I., Lyubomirskaya, K., and Kamyshnyi, O. (2024a). Exploring the interplay between posttraumatic stress disorder, gut microbiota, and inflammatory biomarkers: a comprehensive meta-analysis. Front. Immunol. 15:883. doi: 10.3389/fimmu.2024.1349883
Petakh, P., Oksenych, V., Kamyshna, I., Boisak, I., Lyubomirskaya, K., and Kamyshnyi, O. (2024b). Exploring the complex interplay: gut microbiome, stress, and leptospirosis. Front. Microbiol. 15:684. doi: 10.3389/fmicb.2024.1345684
Petra, A. I., Panagiotidou, S., Hatziagelaki, E., Stewart, J. M., Conti, P., and Theoharides, T. C. (2015). Gut-microbiota-brain Axis and its effect on neuropsychiatric disorders with suspected immune dysregulation. Clin. Ther. 37, 984–995. doi: 10.1016/j.clinthera.2015.04.002
Plaza-Díaz, J., Ruiz-Ojeda, F. J., Vilchez-Padial, L. M., and Gil, A. (2017). Evidence of the anti-inflammatory effects of probiotics and Synbiotics in intestinal chronic diseases. Nutrients 9, 555–574. doi: 10.3390/nu9060555
Prentice, P. M., Schoemaker, M. H., Vervoort, J., Hettinga, K., Lambers, T. T., van Tol, E. A. F., et al. (2019). Human Milk Short-chain fatty acid composition is associated with adiposity outcomes in infants. J. Nutr. 149, 716–722. doi: 10.1093/jn/nxy320
Ramirez, K., Fornaguera-Trías, J., and Sheridan, J. F. (2017). Stress-induced microglia activation and monocyte trafficking to the brain underlie the development of anxiety and depression. Curr. Top. Behav. Neurosci. 31, 155–172. doi: 10.1007/7854_2016_25
Rhee, S. H., Pothoulakis, C., and Mayer, E. A. (2009). Principles and clinical implications of the brain-gut-enteric microbiota axis. Nat. Rev. Gastroenterol. Hepatol. 6, 306–314. doi: 10.1038/nrgastro.2009.35
Ridaura, V., and Belkaid, Y. (2015). Gut microbiota: the link to your second brain. Cell 161, 193–194. doi: 10.1016/j.cell.2015.03.033
Ritchie, G., Strodl, E., Parham, S., Bambling, M., Cramb, S., and Vitetta, L. (2023). An exploratory study of the gut microbiota in major depression with anxious distress. J. Affect. Disord. 320, 595–604. doi: 10.1016/j.jad.2022.10.001
Rong, H., Xie, X. H., Zhao, J., Lai, W. T., Wang, M. B., Xu, D., et al. (2019). Similarly in depression, nuances of gut microbiota: evidences from a shotgun metagenomics sequencing study on major depressive disorder versus bipolar disorder with current major depressive episode patients. J. Psychiatr. Res. 113, 90–99. doi: 10.1016/j.jpsychires.2019.03.017
Sanna, S., van Zuydam, N. R., Mahajan, A., Kurilshikov, A., Vich Vila, A., Võsa, U., et al. (2019). Causal relationships among the gut microbiome, short-chain fatty acids and metabolic diseases. Nat. Genet. 51, 600–605. doi: 10.1038/s41588-019-0350-x
Schönfeld, P., and Wojtczak, L. (2016). Short- and medium-chain fatty acids in energy metabolism: the cellular perspective. J. Lipid Res. 57, 943–954. doi: 10.1194/jlr.R067629
Schulze, T. G., Akula, N., Breuer, R., Steele, J., Nalls, M. A., Singleton, A. B., et al. (2014). Molecular genetic overlap in bipolar disorder, schizophrenia, and major depressive disorder. World J. Biol. Psych. Off. J. World Feder. Societ. Biol. Psych. 15, 200–208. doi: 10.3109/15622975.2012.662282
Seltzer, M. A., Jahan, S. A., Sparks, R., Stout, D. B., Satyamurthy, N., Dahlbom, M., et al. (2004). Radiation dose estimates in humans for (11)C-acetate whole-body PET. J. Nucl. Med. Off. Publ. Soc. Nucl. Med. 45, 1233–1236
Seow, L. S., Subramaniam, M., Abdin, E., Vaingankar, J. A., and Chong, S. A. (2016). Sleep disturbance among people with major depressive disorders (MDD) in Singapore. J. Ment. Health 25, 492–499. doi: 10.3109/09638237.2015.1124390
Shimizu, H., Masujima, Y., Ushiroda, C., Mizushima, R., Taira, S., Ohue-Kitano, R., et al. (2019). Dietary short-chain fatty acid intake improves the hepatic metabolic condition via FFAR3. Sci. Rep. 9:16574. doi: 10.1038/s41598-019-53242-x
Short, N. A., Boffa, J. W., Wissemann, K., and Schmidt, N. B. (2020). Insomnia symptoms predict the development of post-traumatic stress symptoms following an experimental trauma. J. Sleep Res. 29:e12909. doi: 10.1111/jsr.12909
Sivaprakasam, S., Bhutia, Y. D., Yang, S., and Ganapathy, V. (2017). Short-chain fatty acid transporters: role in colonic homeostasis. Compr. Physiol. 8, 299–314. doi: 10.1002/cphy.c170014
Skonieczna-Żydecka, K., Grochans, E., Maciejewska, D., Szkup, M., Schneider-Matyka, D., Jurczak, A., et al. (2018). Faecal Short chain fatty acids profile is changed in polish depressive women. Nutrients 10, 1939–1953. doi: 10.3390/nu10121939
Smith, P. M., Howitt, M. R., Panikov, N., Michaud, M., Gallini, C. A., Bohlooly-y, M., et al. (2013). The microbial metabolites, short-chain fatty acids, regulate colonic Treg cell homeostasis. Science 341, 569–573. doi: 10.1126/science.1241165
Socała, K., Doboszewska, U., Szopa, A., Serefko, A., Włodarczyk, M., Zielińska, A., et al. (2021). The role of microbiota-gut-brain axis in neuropsychiatric and neurological disorders. Pharmacol. Res. 172:105840. doi: 10.1016/j.phrs.2021.105840
Stumpff, F. (2018). A look at the smelly side of physiology: transport of short chain fatty acids. Pflugers Archiv. Euro. J. Physiol. 470, 571–598. doi: 10.1007/s00424-017-2105-9
Tan, J., McKenzie, C., Potamitis, M., Thorburn, A. N., Mackay, C. R., and Macia, L. (2014). The role of short-chain fatty acids in health and disease. Adv. Immunol. 121, 91–119. doi: 10.1016/B978-0-12-800100-4.00003-9
Thaiss, C. A., Zeevi, D., Levy, M., Zilberman-Schapira, G., Suez, J., Tengeler, A. C., et al. (2014). Transkingdom control of microbiota diurnal oscillations promotes metabolic homeostasis. Cell 159, 514–529. doi: 10.1016/j.cell.2014.09.048
Thursby, E., and Juge, N. (2017). Introduction to the human gut microbiota. Biochem. J. 474, 1823–1836. doi: 10.1042/BCJ20160510
Tong, L. C., Wang, Y., Wang, Z. B., Liu, W. Y., Sun, S., Li, L., et al. (2016). Propionate ameliorates dextran sodium sulfate-induced colitis by improving intestinal barrier function and reducing inflammation and oxidative stress. Front. Pharmacol. 7:253. doi: 10.3389/fphar.2016.00253
Tsanas, A., Woodward, E., and Ehlers, A. (2020). Objective characterization of activity, sleep, and circadian rhythm patterns using a wrist-worn Actigraphy sensor: insights into posttraumatic stress disorder. JMIR Mhealth Uhealth 8:e14306. doi: 10.2196/14306
Tsilingiri, K., and Rescigno, M. (2013). Postbiotics: what else? Benefic. Microbes 4, 101–107. doi: 10.3920/BM2012.0046
Valles-Colomer, M., Falony, G., Darzi, Y., Tigchelaar, E. F., Wang, J., Tito, R. Y., et al. (2019). The neuroactive potential of the human gut microbiota in quality of life and depression. Nat. Microbiol. 4, 623–632. doi: 10.1038/s41564-018-0337-x
van de Wouw, M., Boehme, M., Lyte, J. M., Wiley, N., Strain, C., O’Sullivan, O., et al. (2018). Short-chain fatty acids: microbial metabolites that alleviate stress-induced brain-gut axis alterations. J. Physiol. 596, 4923–4944. doi: 10.1113/JP276431
Vijay, N., and Morris, M. E. (2014). Role of monocarboxylate transporters in drug delivery to the brain. Curr. Pharm. Des. 20, 1487–1498. doi: 10.2174/13816128113199990462
Vizioli, M. G., Liu, T., Miller, K. N., Robertson, N. A., Gilroy, K., Lagnado, A. B., et al. (2020). Mitochondria-to-nucleus retrograde signaling drives formation of cytoplasmic chromatin and inflammation in senescence. Genes Dev. 34, 428–445. doi: 10.1101/gad.331272.119
Voigt, R. M., Forsyth, C. B., Green, S. J., Mutlu, E., Engen, P., Vitaterna, M. H., et al. (2014). Circadian disorganization alters intestinal microbiota. PLoS One 9:e97500. doi: 10.1371/journal.pone.0097500
Voltolini, C., Battersby, S., Etherington, S. L., Petraglia, F., Norman, J. E., and Jabbour, H. N. (2012). A novel Antiinflammatory role for the Short-chain fatty acids in human labor. Endocrinology 153, 395–403. doi: 10.1210/en.2011-1457
Walker, W. H. 2nd, Walton, J. C., DeVries, A. C., and Nelson, R. J. (2020). Circadian rhythm disruption and mental health. Transl. Psychiatry 10:28. doi: 10.1038/s41398-020-0694-0
Wang, H.-B., Wang, P.-Y., Wang, X., Wan, Y.-L., and Liu, Y.-C. (2012). Butyrate enhances intestinal epithelial barrier function via up-regulation of tight junction protein Claudin-1 transcription. J. Digest. Dis. Sci. 57, 3126–3135. doi: 10.1007/s10620-012-2259-4
Weitkunat, K., Schumann, S., Nickel, D., Kappo, K. A., Petzke, K. J., Kipp, A. P., et al. (2016). Importance of propionate for the repression of hepatic lipogenesis and improvement of insulin sensitivity in high-fat diet-induced obesity. Mol. Nutr. Food Res. 60, 2611–2621. doi: 10.1002/mnfr.201600305
Xu, Q., Zhang, R., Mu, Y., Song, Y., Hao, N., Wei, Y., et al. (2022). Propionate ameliorates alcohol-induced liver injury in mice via the gut-liver Axis: focus on the improvement of intestinal permeability. J. Agric. Food Chem. 70, 6084–6096. doi: 10.1021/acs.jafc.2c00633
Yang, W., Yu, T., Huang, X., Bilotta, A. J., Xu, L., Lu, Y., et al. (2020). Intestinal microbiota-derived short-chain fatty acids regulation of immune cell IL-22 production and gut immunity. Nat. Commun. 11:4457. doi: 10.1038/s41467-020-18262-6
Yarandi, S. S., Peterson, D. A., Treisman, G. J., Moran, T. H., and Pasricha, P. J. (2016). Modulatory effects of gut microbiota on the central nervous system: how gut could play a role in neuropsychiatric health and diseases. J. Neurogastroenterol. Motil. 22, 201–212. doi: 10.5056/jnm15146
Yi, P., and Li, L. (2012). The germfree murine animal: an important animal model for research on the relationship between gut microbiota and the host. Vet. Microbiol. 157, 1–7. doi: 10.1016/j.vetmic.2011.10.024
Yirmiya, K, Turjeman, S, Shtossel, O, Zagoory-Sharon, O, Moadi, L, Louzoun, Y, et al. Microbiome mediates development of PTSD and resilience (2022).
Yu, Z., Han, J., Chen, H., Wang, Y., Zhou, L., Wang, M., et al. (2021). Oral supplementation with butyrate improves myocardial ischemia/reperfusion injury via a gut-brain neural circuit. Front. Cardiovasc. Med. 8:718674. doi: 10.3389/fcvm.2021.718674
Zhang, J., Peng, J., Huang, Y., Meng, L., Li, Q., Xiong, F., et al. (2020). Identification of histone deacetylase (HDAC)-associated proteins with DNA-programmed affinity labeling. Angew. Chem. Int. Ed. Engl. 59, 17525–17532. doi: 10.1002/anie.202001205
Zhao, Y., Liu, J., Hao, W., Zhu, H., Liang, N., He, Z., et al. (2017). Structure-specific effects of Short-chain fatty acids on plasma cholesterol concentration in male Syrian hamsters. J. Agric. Food Chem. 65, 10984–10992. doi: 10.1021/acs.jafc.7b04666
Zheng, P., Zeng, B., Zhou, C., Liu, M., Fang, Z., Xu, X., et al. (2016). Gut microbiome remodeling induces depressive-like behaviors through a pathway mediated by the host’s metabolism. Mol. Psychiatry 21, 786–796. doi: 10.1038/mp.2016.44
Zhou, Y., Xu, H., Xu, J., Guo, X., Zhao, H., Chen, Y., et al. (2021). F. Prausnitzii and its supernatant increase SCFAs-producing bacteria to restore gut dysbiosis in TNBS-induced colitis. AMB Express 11, 33–43. doi: 10.1186/s13568-021-01197-6
Keywords: gut microbiome, stress, posttraumatic stress disorder, probiotic, SCFA, acetate, propionate, butyrate
Citation: Petakh P, Duve K, Oksenych V, Behzadi P and Kamyshnyi O (2024) Molecular mechanisms and therapeutic possibilities of short-chain fatty acids in posttraumatic stress disorder patients: a mini-review. Front. Neurosci. 18:1394953. doi: 10.3389/fnins.2024.1394953
Edited by:
Christopher A. Lowry, University of Colorado Boulder, United StatesReviewed by:
Robin Michelle Voigt, Rush University, United StatesCopyright © 2024 Petakh, Duve, Oksenych, Behzadi and Kamyshnyi. This is an open-access article distributed under the terms of the Creative Commons Attribution License (CC BY). The use, distribution or reproduction in other forums is permitted, provided the original author(s) and the copyright owner(s) are credited and that the original publication in this journal is cited, in accordance with accepted academic practice. No use, distribution or reproduction is permitted which does not comply with these terms.
*Correspondence: Pavlo Petakh, cGF2bG8ucGV0YWtoQHV6aG51LmVkdS51YQ==; Valentyn Oksenych, dmFsZW50eW4ub2tzZW55Y2hAdWliLm5v