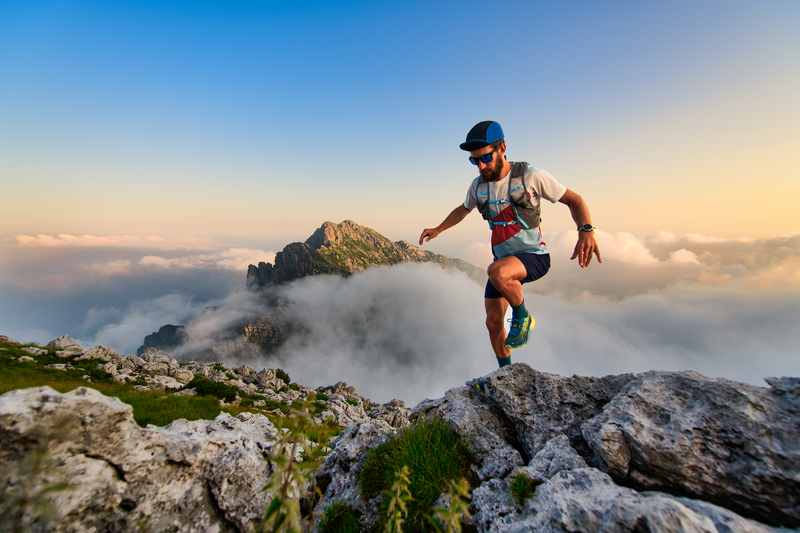
95% of researchers rate our articles as excellent or good
Learn more about the work of our research integrity team to safeguard the quality of each article we publish.
Find out more
ORIGINAL RESEARCH article
Front. Neurosci. , 16 July 2024
Sec. Neurodegeneration
Volume 18 - 2024 | https://doi.org/10.3389/fnins.2024.1381889
As a dietary strategy, methionine restriction has been reported to promote longevity and regulate metabolic disorders. However, the role and possible regulatory mechanisms underlying methionine in neurodegenerative diseases such as Alzheimer's disease (AD), remain unexplored. This study utilized the data from BXD recombinant inbred (RI) mice to establish a correlation between the AD phenotype in mice and methionine level. Gene enrichment analysis indicated that the genes associated with the concentration of methionine in the midbrain are involved in the dopaminergic synaptic signaling pathway. Protein interaction network analysis revealed that glycogen synthase kinase 3 beta (GSK-3β) was a key regulator of the dopaminergic synaptic pathway and its expression level was significantly correlated with the AD phenotype. Finally, in vitro experiments demonstrated that methionine deprivation could reduce the expression of Aβ and phosphorylated Tau, suggesting that lowering methionine levels in humans may be a preventive or therapeutic strategy for AD. In conclusion, our findings support that methionine is a high risk factor for AD. These findings predict potential regulatory network, theoretically supporting methionine restriction to prevent AD.
Alzheimer's disease (AD), the main cause of dementia (Jorfi et al., 2023), is characterized by progressive neurodegeneration (De Strooper and Karran, 2016). According to the Alzheimer's Disease International Organization, there are ~9.5 million patients with dementia in China, and it is projected to exceed 16 million by 2030 (Jia et al., 2020). Patients with AD experience memory loss, cognitive decline (Qu et al., 2022), disorientation (Atri, 2019), impaired judgment, and sometimes hallucinations or delusions (Knopman and Petersen, 2014), significantly affecting their independence and quality of life. Although there is currently no approved cure for AD (Gong et al., 2019), pharmacological and non-pharmacological therapies can help improve patients' quality of life and slow down disease progression (Zhang et al., 2013; Kong et al., 2020; Thota et al., 2020; Xu et al., 2023). Several pathophysiological factors have been implicated in the atrophy of the cerebral cortex and hippocampus (Li Y. D. et al., 2023), including abnormalities in neurotransmitters, beta-amyloid-induced plaque deposition, neurofibrillary tangle formation, inflammation (Li et al., 2019), and oxidative stress (Chen et al., 2021; Pan et al., 2021; Yang et al., 2023). Accumulation of hyperphosphorylated Tau protein disrupts synaptic function and leads to brain dysfunction by impairing glutamate receptor trafficking or synaptic anchoring (Miller et al., 2014; Tanaka et al., 2022). Furthermore, there is a correlation between Tau hyperphosphorylation and the tangles of AD (Guillozet-Bongaarts et al., 2006; Giovinazzo et al., 2021; John and Reddy, 2021). Although the triggers, underlying mechanisms, and effective treatments of AD are still unclear, recent studies have shown that dietary interventions may be potential treatment strategies (Valls-Pedret et al., 2015). Dietary restriction during adulthood (DR; reducing calorie intake while maintaining micronutrient levels) reduces the risk of AD (Nuru et al., 2018; Kovalska et al., 2023). Age-related impairments in learning, memory, and motor function were observed to improve in rodents undergoing dietary restriction, however the mechanics remain unclear (Xu et al., 2022).
Recently researchers have found that amino acid homeostasis is disrupted in the serum and brain of patients with AD (Trushina et al., 2013; Lin et al., 2018; Wang et al., 2021; Li et al., 2022; Zinellu et al., 2023). Moreover, alterations in the levels of different amino acids in the physiological range have been linked to various pathological conditions, including neurological disorders (Kepka et al., 2020). Longitudinal studies using mouse models of AD have also demonstrated abnormal essential amino acid levels (Puris et al., 2023). These findings suggest that dietary intervention may affect the progression of AD by regulating amino acids metabolism.
Methionine is a widely-used sulfur-containing amino acid that serves as a precursor for substances such as spermine, spermidine, and ethylene (Parkhitko et al., 2019). It plays a pivotal role in various aspects of growth and development (Lu et al., 2001), including cell division (Yang et al., 2022), differentiation, apoptosis, homeostasis, and gene expression (Martínez-Chantar et al., 2008; Li M. L. et al., 2023). Studies have shown that the methionine cycle is involved in the pathogenesis of AD (Zhao et al., 2022). Methionine serves as a crucial methyl donor in certain methyltransferase reactions, providing methyl groups to various compounds. High methionine diet has been proven to induce AD-like symptoms (Pi et al., 2021). As a dietary intervention, methionine restriction has been reported to alleviate AD, but the molecular mechanisms remain unclear (Alachkar et al., 2022; Kovalska et al., 2023; Xi et al., 2023). Therefore, it is of great significance to explore the specific mechanisms, by which methionine is involved in the pathogenesis of AD.
The systems genetics approach serves as a robust instrument relying on systems biology and mouse genetic reference panel (Civelek and Lusis, 2014). BXD recombinant-inbred (RI) mice derived from the crosses between C57BL/6J (B6) and DBA/2J (D2) inbred strains, is a large and well-characterized genetic reference population, and has been widely used for studying the genetic basis of various diseases (Ashbrook et al., 2021). Thousands of transcriptomic/phenomic datasets have been published using these BXD strains over the past decades, consisting of ~250 phenotypes associated with cognitive function (Philip et al., 2010). In addition, since each BXD strain is a stable inbred, it can be replicated in large numbers, to facilitate the precise localization of complex traits with low to moderate heritability (Xu et al., 2024). The BXD panel showed a strong variation in methionine concentrations in the midbrain across different strains, suggesting that it is a suitable population for exploring potential mechanisms underlying the association between methionine and AD.
In the present study, we first investigated the associations between methionine level and AD. We then verified the correlation in SH-SY5Y cell lines. Further analysis based on the BXD RI mice database unveiled that the dopaminergic synaptic signaling pathway may play a key role in the regulation of AD through methionine. The key regulator was predicted to be glycogen synthase kinase 3 beta (GSK-3β). Our study revealed a positive association between methionine level and increased risk of AD. However, it is necessary to validate this predictive regulatory mechanism in vivo and in vitro.
The mRNA expression data set, VU BXD Midbrain Agilent SurePrint G3 Mouse GE (May12) was obtained from the GeneNetwork website (https://www.genenetwork.org/) (Xu et al., 2011). The expression data was generated from the adult male mice of 34 different BXD strains (Xu et al., 2020) between 4 and 12 months of age. They were housed in groups in a vivarium that controlled temperature and humidity levels. Additionally, the mice were subjected to a 12-h light-dark cycle. The mice had unrestricted access to both food and water. The array data in GeneNetwork have been log transformed and then the z-score normalized, where instead of leaving the mean at 0 and the standard deviation of 1 unit, the data is rescaled to a mean of 8 units with a standard deviation of 2 units (what we call 2Z + 8 normalized data). The standardized midbrain methionine level dataset is available on GeneNetwork, belonging to the group “BXD Family,” categorized as “Phenotypes,” and named “18038.”
The learning-related traits of BXD mice were retrieved from GeneNetwork. The detailed descriptions of the traits can be found in GeneNetwork and in a previous publication (Neuner et al., 2019a). The summary statistics and individual values are available under the “BXD” group, “trait and cofactors” type, and “BXD phenotypes” data set with record IDs of 20494, 20499, 20575, and 20817.
Gene-phenotype correlation analysis has been widely used to investigate key genes in a gene set. The gene-phenotype correlations were performed on the GeneNetwork online platform using Pearson's correlation method. We used this method to investigate the correlated genes in BXD mouse midbrain transcriptomic dataset with the midbrain methionine level phenotype (ID: 18038). Genes with p-values < 0.05 were considered to be significantly associated with the 18038 phenotype.
The pathway database is a collection of manually created Kyoto Encyclopedia of Genes and Genomes (KEGG) pathway maps that represent the molecular wiring diagrams of biological systems (Deng et al., 2021). Genes with statistically significant genetic correlation with 18038 phenotype (p < 0.05) were selected and uploaded to WebGestalt (http://www.webgestalt.org/) for gene enrichment analysis. This analysis employs a hypergeometric statistical test to generate adjusted p-values and enrichment ratios. The p-values generated from the hypergeometric test were corrected by the Benjamini-Hochberg method. The pathways with adjusted p < 0.05 were considered significant.
PPI networks provide useful information about cellular functions and biological processes. The gene set was submitted to the online tool STRING (Szklarczyk et al., 2019), a database of known and predicted PPIs (https://string-db.org/). We explored PPI networks for genes in the dopaminergic pathway in the STRING database.
The gene-phenotype network analysis was performed on the GeneNetwork online platform using Pearson's correlation. Using the BXD midbrain transcriptomic dataset, we deployed a gene-phenotype expression network to identify the key genes from the gene set as previously described (Deng et al., 2021). Briefly, a network was constructed using Pearson's correlation coefficient matrix. In the network, each node stood for a gene, and the correlation coefficient was set as the edge. Binomial correlations higher than 0.3 or lower than −0.3 were defined as connected. The connection weight was calculated for each node as, the sum of the binominal correlation coefficient connected to each node. The correlation coefficients with p-values < 0.05 were deemed statistically significant.
The SH-SY5Y cell line, derived from human neuroblastoma, was obtained from ATCC and was authenticated by short-tandem repeat profiling. These neuronal precursor cells were cultured in MEM-F12 medium supplemented with 10% fetal bovine serum and 1% penicillin-streptomycin solution. The cells were maintained at a temperature of 37°C and a carbon dioxide (CO2) concentration of 5%. The cells were treated with the GSK-3β inhibitor (CHIR-99021, APE × BIO) with a final concentration of 8 μM to further study.
To prepare the samples for analysis, a loading buffer was added to cells. They were then subjected to boiling for 10 min. Following boiling, centrifugation was performed at 12,000 rpm/min for 5 min to separate the samples. The resulting samples were loaded onto a 10%-12% SDS-PAGE gel and subsequently transferred onto a polyvinylidene fluoride (PVDF) membrane using a wet transfer apparatus. To block non-specific binding, the membrane was incubated with 5% skimmed milk or BSA for 1 h. After blocking, the membrane was washed three times with TBST (Tris-buffered saline with Tween 20), for 10 min each. The membrane was then incubated with the designated primary antibody at 4°C overnight. Thereafter, the secondary antibody was added and incubated for 1 h. Finally, luminescence was performed to visualize the protein bands. An imaging system and ImageJ software were used to quantify the protein bands. These tools allowed for the accurate measurement and analysis of the protein bands. The following primary antibodies were used for Western blotting: mouse anti-actin (1:2,000 dilution, GenScript, A700702), rabbit anti-Tau (1:2,000 dilution, proteintech, 10274-1-AP), mouse anti-p-Tau (1:2,000 dilution, Santa Cruz, sc-32275), rabbit anti-Aβ (1:1,000 dilution, CST, 8243T), mouse anti-GSK3 beta (1:1,000 dilution, Santa Cruz, sc-81462), and mouse anti-p-GSK3 alpha/beta (1:1,000 dilution, Santa Cruz, sc-81496).
Data were analyzed using GraphPad Prism software. The results are presented as mean ± SD. Comparisons between the two groups were evaluated using two-tailed Student's t-test to determine significant p-values. *p < 0.05, **p < 0.01, and ***p < 0.001 were considered statistically significant.
To determine whether methionine regulates AD, systems genetics analysis was conducted to uncover the expression regulation of methionine levels in the midbrain. Although AD has been mainly studied in the context of hippocampus, a relationship has also been reported between the midbrain and AD. Methionine levels were examined in the midbrain of 34 BXD mice strains in GeneNetwork. The midbrain transcriptome data of BXD RI mice used are available through our GeneNetwork website. The standardized dataset “midbrain methionine level” is available on GeneNetwork (group “BXD Family,” category “Phenotypes,” named “18038”). There were a significant differences in methionine levels among the BXD strains with thresholds ranging from 0.4 (BXD15) to 0.09 (BXD85). The average expression threshold was 0.25, while the median expression threshold was 0.25. Further, we observed a 4.4-fold change in methionine expression levels across the 34 BXD strains (Figure 1). These data were utilized for conducting association analyses with AD phenotypes.
Figure 1. Expression of methionine in the BXD family. Bar-plot of methionine expression level in the midbrain across BXD strains. BXD15 shows the highest expression and BXD85 shows the lowest expression. The x-axis shows BXD strains. The y-axis shows the normalized log2 expression levels of methionine.
The Y-maze method is a valuable tool for evaluating both conditioned reflexes and spatial memory in animals. The Y-maze is not only used for assessing spatial working and reference memory in the hippocampus but also commonly used to detect the function of learning and memory in the midbrain (Ishola et al., 2018, 2019; Bashirzade et al., 2022). To investigate the effect of methionine on memory and cognitive performance, we conducted Pearson's correlations between methionine level and Y-maze performance phenotypes. The methionine level was found to be significantly positively correlated with the percentage of unsuccessful alternations in the Y-maze test [n = 16, r = 0.498, p = 0.050 (Figure 2A), n = 9, r = 0.790, p = 0.011 (Figure 2B), n = 16, r = 0.817, p =0.0001101 (Figure 2C), and n = 17, r = 0.503, p = 0.039 (Figure 2D)], suggesting that methionine concentration positively affects the course of AD.
Figure 2. Scatter plot showed the correlation between midbrain methionine level and the AD-related behavioral phenotypes. (A–D) The Pearson's correlation coefficient was used to determine the relationship. (A–D) The correlations between methionine levels (log2 transformed) in the midbrain and the percentage of unsuccessful alternations in the Y-maze test in BXD mice [(A): Record ID 20494; (B): Record ID 20499; (C): Record ID 20575; (D): Record ID 20817]. Pearson correlation coefficients and p-values are indicated.
To unravel the role of methionine in AD, we performed gene-set enrichment analysis using the top 2,000 genes correlated with methionine level in the midbrain (p < 0.05, Pearson's correlation; Supplementary Table S1). We performed KEGG pathway enrichment analysis and selected the top 15 pathways for further analysis (Supplementary Table S2). The analysis indicated the enrichment of neurodegenerative disease-associated pathways, such as synaptic vesicle cycle, axon guidance, cholinergic synapse, circadian entrainment, and dopaminergic synapse (Figure 3A). Among these, the dopaminergic synapse pathway exhibited a strong association with the AD phenotypes. Dysfunction or abnormalities in dopaminergic synapses have been implicated in various neurological and psychiatric disorders, including Parkinson's disease, schizophrenia, and addiction (Song et al., 2023). To identify the specific mechanisms, we subjected the dopamine pathway genes to protein interaction analysis based on the STRING database (https://cn.string-db.org/). The PPI analysis revealed GSK-3β to be at the center of the network (Figure 3B). GSK-3β has been known to play a fundamental role in various processes, including cell division, proliferation, differentiation, and adhesion (Forde and Dale, 2007). Moreover, it has been implicated in various disorders, including AD and hyperdopamine dependent behaviors (Beaulieu et al., 2007; Lauretti et al., 2020). Using the percentage of unsuccessful alternations in the Y-maze test, we conducted a Pearson's correlation correlation analysis of the dopaminergic pathway genes in GeneNetwork to investigate the relevance of the dopaminergic pathway in AD. The results showed a strong positive correlation between GSK-3β and Y-maze phenotype (Figures 3C, D), demonstrating a positive association of GSK-3β expression with the progression of AD. These results further suggest that methionine may promote AD progression by positively regulating GSK-3β.
Figure 3. GSK-3β was associated with methionine-induced cognitive impairment. (A) The bubble plot showed the KEGG pathways significantly enriched by the methionine-correlated genes in the midbrain of BXD mice. The analysis was performed using WebGestalt (http://www.webgestalt.org/), and significant genes were identified (p < 0.05). The x-axis represents the enrichment ratio, while the y-axis represents the enriched pathways. The size of the bubbles corresponds to the number of genes, and the color represents the p-value. (B) Protein-protein interaction network of dopaminergic pathway genes. In total, 12 dopaminergic pathway genes were submitted to the STRING database (https://cn.string-db.org/). (C) The correlations between all genes of the dopaminergic pathway in the midbrain and the percentage of unsuccessful alternations in the Y-maze test in BXD mice (Record ID 20565). Red dots represent significant positive associations (p < 0.05). (D) The correlations between the expression of GSK-3β in the midbrain and the percentage of unsuccessful alternations in the Y-maze test in BXD mice (Record ID 20565).
In the central nervous system, two secretory enzymes convert amyloid precursor protein (APP) generates amyloid β (Aβ) (Soria Lopez et al., 2019). The Tau protein is primarily expressed in neurons and plays a crucial role in microtubule protein polymerization and microtubule stabilization (Ossenkoppele et al., 2022). Aβ induces Tau hyperphosphorylation, oxidative stress, and inflammatory response, leading to cell death and impairing neurotransmission. AD is diagnosed based on the presence of Aβ and phosphorylated Tau. Our results demonstrated a dose-dependent upregulation of Aβ expression with increasing methionine concentrations (Figure 4A). Phosphorylation levels of Tau protein were also found to be elevated after treatment with high concentrations of methionine (Figure 4B). GSK-3β is a multifunctional protein and plays a crucial role in the pathogenesis of AD as a small molecule kinase (Hurtado et al., 2012). Inhibiting GSK-3β activity has been shown to improve cognitive impairments and attenuate oxidative stress. It has been reported that AD brains possess high levels of GSK-3β (Farr et al., 2014). Relevant studies have shown that phosphorylation of the Tyr216 site of GSK-3β can promote its activation, hyperphosphorylate Tau, activate the Thr668 site of APP protein, and induce pathological changes in AD. Phosphorylation by activated Akt at the Ser9 site, inactivates GSK-3β (Steen et al., 2005; Martin et al., 2011; Yang et al., 2020). Our findings indicated that phosphorylation of the Tyr216 site of GSK-3β is methionine-dependent, and high concentrations of methionine promote its expression (Figure 4C). To further demonstrate whether methionine regulates AD progression by modulating GSK-3β, the results of our experiments revealed that methionine deficiency or excess no longer altered A-β and p-Tau levels when we treated SH-SY5Y cells with the GSK-3β inhibitor, the above results proved our speculation (Figure 4D). However, the mechanism of how methionine regulates GSK-3β in AD still needs further studies.
Figure 4. Methionine deficiency inhibited the progression of AD. SH-SY5Y cells were treated with methionine at different concentrations. (A, B) Phosphorylation of Tau and A-β, as typical pathological features of AD, was detected using Western blotting after treatment with different concentrations of methionine. (C) After treatment with different concentrations of methionine, GSK-3β phosphorylation was detected using Western blotting. (D). Changes in A-β and p-Tau protein levels using co-treatment with GSK-3β inhibitor and different concentrations of methionine.
In this study, we found that high levels of methionine were positively associated with the percentage of unsuccessful alternations in the Y-maze test which represents more severe learning and memory dysfunction. We then treated SH-SY5Y cells with different concentrations of methionine and found that Aβ and p-Tau levels were positively correlated with methionine levels. High methionine levels in the medium significantly upregulated Aβ and p-Tau. Removal of methionine from the medium significantly decreased Aβ and p-Tau levels. These results support methionine as a high risk factor for AD. These findings are also consistent with previous reports that dietary restriction of methionine can improve the symptoms of AD (Xi et al., 2023).
Non-pharmacological treatments are valuable for preventing AD or complementing other treatments. Dietary changes in many metabolites have shown clear preclinical benefits, some of which have also shown promise in clinical trials. Although the effect of dietary interventions on the progression of AD is well-known, the previous studies are still scarce and controversial. There are still no clear guidelines or recommended dietary change programs for patients with AD. Some studies have found that the Mediterranean diet, the Dietary Approaches to Stop Hypertension (DASH), and the Mediterranean-DASH Intervention for Neurodegenerative Delay diet (MIND), may protect against AD (van den Brink et al., 2019). Many potential dietary factors have been implicated in the development of AD, including dietary deficiencies of folic acid and vitamins E, C, B6, and B12 (Luchsinger and Mayeux, 2004; An et al., 2019; Mielech et al., 2020). Antioxidant vitamins affect lipid peroxidation and oxidative stress. Folic acid and vitamins B6 and B12 regulate DNA methylation, which can increase homocysteine levels. It has also been reported that excess saturated fatty acids may be a potential risk factor for AD (Luchsinger and Mayeux, 2004). However, there are few reports about the role of a single amino acid on AD. Previous studies have suggested that impaired BCAA metabolism can impact neuronal health and synaptic function, potentially affecting the development and progression of neurodegenerative disorders (Wang et al., 2023). More studies are needed to support the regulatory role of a single amino acid in AD.
In this study, we integrated phenotypic resources from the BXD panel, including more than 300 neural phenotypes from over 150 mouse strains. The recombinant inbred population of the BXD family, as one of the largest and well-characterized genetic reference populations, is the basis for studying various diseases, such as cancer, cognitive disorders, and heart diseases (Zhu et al., 2020; Bajpai et al., 2023; Zhou et al., 2023; Xu et al., 2024). The correlation of the BXD RI family has been extended to genetic analysis of behavioral phenotypes, including drug addiction, neurodegenerative processes, etc. (Wang et al., 2016; Ashbrook et al., 2021). It provides a strong heredity resource that can be used to interpret pathways and regulatory networks using phylogenetic approaches. Some studies have suggested that it is possible to study AD through BXD panel analysis. Previous studies have reported the exploiting inbred lineage properties of AD-BXD groups to explore the molecular mechanisms underlying the early onset of the disease. In addition, several studies have utilized previously generated transcriptomic and phenotypic information from genetically diverse populations of mice to identify the molecular networks directly leading to differences in the cognitive outcomes of different groups (Neuner et al., 2019b; Heuer et al., 2020). We used this powerful resource to explore a strong relationship between amino acids and AD.
In our study, we found that various amino acids are involved in the regulation of AD (data are not shown), among which methionine levels in the midbrain level were interesting. Previous studies mostly focused on the hippocampus (Ren et al., 2021; Xi et al., 2023). For the first time, we reported the relationship between methionine levels in the midbrain and AD. Methionine may promote the progression of AD by regulating the dopaminergic synaptic pathway. The latest evidence of a selective and precocious VTA dopaminergic cell death in a mouse model of AD has implicated the midbrain dopaminergic system in the pathogenesis of AD (Nobili et al., 2017). Furthermore, subcortical dysfunctions, such as reduced dopamine (DA) levels, have also been reported in patients with AD. They lead to psychiatric symptoms, such as apathy and depression, and may be involved in cognitive decline (D'Amelio et al., 2018). Many studies have also shown that the midbrain is involved in learning and memory ability (Schott et al., 2004, 2006; Murty et al., 2011; D'Ardenne et al., 2012; Kafkas and Montaldi, 2015); thus, we speculated that similar to the hippocampus, the midbrain is involved in the development of AD.
A thorough midbrain gene profiling has shed light on the potential molecular mechanism involved in the association of methionine and AD. Through related gene search and enrichment analysis indicated the enrichment of multiple important neural signaling pathways, such as synaptic vesicle cycle, axon guidance, cholinergic synapse and dopaminergic synapse. The dopaminergic synaptic signaling pathway is one of the most important signal transduction pathways in AD and has a significant role in coordinating neurotransmitters, consolidating memory, and the functioning of synapses (Bamford et al., 2018). Protein-protein interaction network analysis showed that GSK-3β is mostly connected to other nodes in the dopaminergic synaptic pathway. GSK-3β is a downstream mediator of several signaling pathways in the brain, including DA signaling (Li and Gao, 2011; Mahmoodkhani et al., 2022). Thus, methionine may regulate the course of AD by modulating GSK-3β expression. GSK-3β, as an important serine-threonine kinase, is involved in memory consolidation, neurogenesis, synaptic plasticity, long-duration enhancement and inflammation. GSK-3β is abundant in the central nervous system (Lee et al., 2006; Leroy et al., 2007), and regulating GSK-3β activity is considered to be one of the important preventive strategies for neurodegenerative diseases. Through in vitro experiments, we found that the phosphorylation level of GSK-3β increased in the presence of high concentrations of methionine (Figure 4C), further validating our hypothesis. When we treated SH-SY5Y cells with an inhibitor of GSK-3β, methionine deficiency or excess no longer altered Aβ and p-Tau levels, demonstrating that GSK-3β is a key downstream protein in methionine-regulated AD (Figure 4D). It has been reported that activation of dopamine D1 receptors can alter synaptic strength and plasticity through GSK-3β activity, which in turn regulates Tau phosphorylation (Lebel et al., 2009). Hence, as shown in the mechanism diagram, we speculated that methionine may affect phosphorylation of Tau and AD progression through dopamine D1 receptor/GSK-3β signaling (Figure 5), but the exact mechanism needs studies.
Figure 5. The schematic drawing illustrating the mechanisms underlying the effects of methionine on AD. Accumulation of methionine in the midbrain may increase dopamine secretion, activate dopamine receptors and increase the phosphorylation of GSK-3β, thereby activating GSK-3β, upregulating A-β and p-Tau, and accelerating the progression of AD.
Still, there are significant limitations to our study. First of all, we only analyzed the correlation between methionine and AD phenotypes in the midbrain, and did not comprehensively analyze relevant data from other brain regions, such as the hippocampus. Secondly, we only preliminarily validated the relevant proteins predicted based on the database, and the more complex regulatory pathways need to be further analyzed. Our study provided a theoretical basis for the role of methionine-restricted diets in improving AD, but we did not conduct animal experiments to further validate the correlation and related pathways. For instance, manipulation of GSK-3β using targeted therapies (e.g., antisense oligonucleotides that inhibit GSK-3β in high-expressing strains or drugs that target the pathway) is needed to study changes in methionine expression and AD progression. Future studies should pay more attention to dietary recommendations for patients with AD.
In summary, using the BXD mouse family as a genetic reference group, we identified a correlation between methionine level in the midbrain and AD. Then we preliminarily explored the regulatory role of methionine in AD mechanism and verified it through in vitro experiments. Our study showed that there is a strong correlation between methionine and AD, providing a theoretical basis for the pathogenesis of AD and the prevention and treatment of AD through dietary intervention. Meanwhile, we established a research method to explore the association between metabolites and AD using systems biology. With this method, more metabolites molecules can link to the pathogenesis of AD in the future.
The original contributions presented in the study are included in the article/Supplementary material, further inquiries can be directed to the corresponding authors.
Ethical approval was not required for the studies on humans and animals in accordance with the local legislation and institutional requirements because only commercially available established cell lines were used.
CW: Writing – original draft, Investigation, Validation, Visualization. YH: Investigation, Validation, Visualization, Writing – original draft. YLiu: Validation, Writing – original draft. AB: Formal analysis, Writing – review & editing. YLi: Validation, Writing – review & editing. YG: Investigation, Writing – review & editing. FX: Conceptualization, Supervision, Writing – review & editing. CY: Conceptualization, Funding acquisition, Methodology, Resources, Supervision, Writing – review & editing.
The author(s) declare financial support was received for the research, authorship, and/or publication of this article. This research was funded by the National Natural Science Foundation of China (Grant No. 32101019 to CY); Key R&D Program of Shandong Province (2023CXPT012 to CY); grants (BY2019KYQD32 to CY) from Binzhou Medical University.
Thanks to the support of Shandong Molecular Targeting Intelligent Diagnosis and Treatment Technology Innovation Center. And we would like to express our gratitude to EditSprings (https://www.editsprings.cn) for the expert linguistic services provided.
The authors declare that the research was conducted in the absence of any commercial or financial relationships that could be construed as a potential conflict of interest.
All claims expressed in this article are solely those of the authors and do not necessarily represent those of their affiliated organizations, or those of the publisher, the editors and the reviewers. Any product that may be evaluated in this article, or claim that may be made by its manufacturer, is not guaranteed or endorsed by the publisher.
The Supplementary Material for this article can be found online at: https://www.frontiersin.org/articles/10.3389/fnins.2024.1381889/full#supplementary-material
Alachkar, A., Agrawal, S., Baboldashtian, M., Nuseir, K., Salazar, J., and Agrawal, A. (2022). L-methionine enhances neuroinflammation and impairs neurogenesis: implication for Alzheimer's disease. J. Neuroimmunol. 366:577843. doi: 10.1016/j.jneuroim.2022.577843
An, Y., Feng, L., Zhang, X., Wang, Y., Wang, Y., Tao, L., et al. (2019). Dietary intakes and biomarker patterns of folate, vitamin B(6), and vitamin B(12) can be associated with cognitive impairment by hypermethylation of redox-related genes NUDT15 and TXNRD1. Clin. Epigenetics 11:139. doi: 10.1186/s13148-019-0741-y
Ashbrook, D. G., Arends, D., Prins, P., Mulligan, M. K., Roy, S., Williams, E. G., et al. (2021). A platform for experimental precision medicine: the extended BXD mouse family. Cell Syst. 12, 235-247.e239. doi: 10.1016/j.cels.2020.12.002
Atri, A. (2019). The Alzheimer's disease clinical spectrum: diagnosis and management. Med. Clin. North Am. 103, 263–293. doi: 10.1016/j.mcna.2018.10.009
Bajpai, A. K., Gu, Q., Orgil, B. O., Xu, F., Torres-Rojas, C., Zhao, W., et al. (2023). Cardiac copper content and its relationship with heart physiology: insights based on quantitative genetic and functional analyses using BXD family mice. Front. Cardiovasc. Med. 10:1089963. doi: 10.3389/fcvm.2023.1089963
Bamford, N. S., Wightman, R. M., and Sulzer, D. (2018). Dopamine's effects on corticostriatal synapses during reward-based behaviors. Neuron 97, 494–510. doi: 10.1016/j.neuron.2018.01.006
Bashirzade, A. A. O., Cheresiz, S. V., Belova, A. S., Drobkov, A. V., Korotaeva, A. D., Azizi-Arani, S., et al. (2022). MPTP-treated zebrafish recapitulate 'late-stage' Parkinson's-like cognitive decline. Toxics 10:69. doi: 10.3390/toxics10020069
Beaulieu, J. M., Gainetdinov, R. R., and Caron, M. G. (2007). The Akt-GSK-3 signaling cascade in the actions of dopamine. Trends Pharmacol. Sci. 28, 166–172. doi: 10.1016/j.tips.2007.02.006
Chen, Y., Zhao, S., Fan, Z., Li, Z., Zhu, Y., Shen, T., et al. (2021). Metformin attenuates plaque-associated tau pathology and reduces amyloid-β burden in APP/PS1 mice. Alzheimers Res. Ther. 13:40. doi: 10.1186/s13195-020-00761-9
Civelek, M., and Lusis, A. J. (2014). Systems genetics approaches to understand complex traits. Nat. Rev. Genet. 15, 34–48. doi: 10.1038/nrg3575
D'Amelio, M., Puglisi-Allegra, S., and Mercuri, N. (2018). The role of dopaminergic midbrain in Alzheimer's disease: translating basic science into clinical practice. Pharmacol. Res. 130, 414–419. doi: 10.1016/j.phrs.2018.01.016
D'Ardenne, K., Eshel, N., Luka, J., Lenartowicz, A., Nystrom, L. E., and Cohen, J. D. (2012). Role of prefrontal cortex and the midbrain dopamine system in working memory updating. Proc. Natl. Acad. Sci. U.S.A. 109, 19900–19909. doi: 10.1073/pnas.1116727109
De Strooper, B., and Karran, E. (2016). The cellular phase of Alzheimer's disease. Cell 164, 603–615. doi: 10.1016/j.cell.2015.12.056
Deng, T., Li, J., Liu, J., Xu, F., Liu, X., Mi, J., et al. (2021). Hippocampal transcriptome-wide association study reveals correlations between impaired glutamatergic synapse pathway and age-related hearing loss in BXD-recombinant inbred mice. Front. Neurosci. 15:745668. doi: 10.3389/fnins.2021.745668
Farr, S. A., Ripley, J. L., Sultana, R., Zhang, Z., Niehoff, M. L., Platt, T. L., et al. (2014). Antisense oligonucleotide against GSK-3β in brain of SAMP8 mice improves learning and memory and decreases oxidative stress: involvement of transcription factor Nrf2 and implications for Alzheimer disease. Free Radic. Biol. Med. 67, 387–395. doi: 10.1016/j.freeradbiomed.2013.11.014
Forde, J. E., and Dale, T. C. (2007). Glycogen synthase kinase 3: a key regulator of cellular fate. Cell. Mol. Life Sci. 64, 1930–1944. doi: 10.1007/s00018-007-7045-7
Giovinazzo, D., Bursac, B., Sbodio, J. I., Nalluru, S., Vignane, T., Snowman, A. M., et al. (2021). Hydrogen sulfide is neuroprotective in Alzheimer's disease by sulfhydrating GSK3β and inhibiting Tau hyperphosphorylation. Proc. Natl. Acad. Sci. U.S.A. 118:e2017225118. doi: 10.1073/pnas.2017225118
Gong, Z., Huang, J., Xu, B., Ou, Z., Zhang, L., Lin, X., et al. (2019). Urolithin A attenuates memory impairment and neuroinflammation in APP/PS1 mice. J. Neuroinflammation 16:62. doi: 10.1186/s12974-019-1450-3
Guillozet-Bongaarts, A. L., Cahill, M. E., Cryns, V. L., Reynolds, M. R., Berry, R. W., and Binder, L. I. (2006). Pseudophosphorylation of tau at serine 422 inhibits caspase cleavage: in vitro evidence and implications for tangle formation in vivo. J. Neurochem. 97, 1005–1014. doi: 10.1111/j.1471-4159.2006.03784.x
Heuer, S. E., Neuner, S. M., Hadad, N., O'Connell, K. M. S., Williams, R. W., Philip, V. M., et al. (2020). Identifying the molecular systems that influence cognitive resilience to Alzheimer's disease in genetically diverse mice. Learn. Mem. 27, 355–371. doi: 10.1101/lm.051839.120
Hurtado, D. E., Molina-Porcel, L., Carroll, J. C., Macdonald, C., Aboagye, A. K., Trojanowski, J. Q., et al. (2012). Selectively silencing GSK-3 isoforms reduces plaques and tangles in mouse models of Alzheimer's disease. J. Neurosci. 32, 7392–7402. doi: 10.1523/JNEUROSCI.0889-12.2012
Ishola, I. O., Akataobi, O. E., Alade, A. A., and Adeyemi, O. O. (2019). Glimepiride prevents paraquat-induced Parkinsonism in mice: involvement of oxidative stress and neuroinflammation. Fundam. Clin. Pharmacol. 33, 277–285. doi: 10.1111/fcp.12434
Ishola, I. O., Akinyede, A. A., Adeluwa, T. P., and Micah, C. (2018). Novel action of vinpocetine in the prevention of paraquat-induced parkinsonism in mice: involvement of oxidative stress and neuroinflammation. Metab. Brain Dis. 33, 1493–1500. doi: 10.1007/s11011-018-0256-9
Jia, L., Quan, M., Fu, Y., Zhao, T., Li, Y., Wei, C., et al. (2020). Dementia in China: epidemiology, clinical management, and research advances. Lancet Neurol. 19, 81–92. doi: 10.1016/S1474-4422(19)30290-X
John, A., and Reddy, P. H. (2021). Synaptic basis of Alzheimer's disease: focus on synaptic amyloid beta, P-tau and mitochondria. Ageing Res. Rev. 65:101208. doi: 10.1016/j.arr.2020.101208
Jorfi, M., Maaser-Hecker, A., and Tanzi, R. E. (2023). The neuroimmune axis of Alzheimer's disease. Genome Med. 15:6. doi: 10.1186/s13073-023-01155-w
Kafkas, A., and Montaldi, D. (2015). Striatal and midbrain connectivity with the hippocampus selectively boosts memory for contextual novelty. Hippocampus 25, 1262–1273. doi: 10.1002/hipo.22434
Kepka, A., Ochocinska, A., Borzym-Kluczyk, M., Skorupa, E., Stasiewicz-Jarocka, B., Chojnowska, S., et al. (2020). Preventive role of L-carnitine and balanced diet in Alzheimer's disease. Nutrients 12:1987. doi: 10.3390/nu12071987
Knopman, D. S., and Petersen, R. C. (2014). Mild cognitive impairment and mild dementia: a clinical perspective. Mayo Clin. Proc. 89, 1452–1459. doi: 10.1016/j.mayocp.2014.06.019
Kong, F., Jiang, X., Wang, R., Zhai, S., Zhang, Y., and Wang, D. (2020). Forsythoside B attenuates memory impairment and neuroinflammation via inhibition on NF-κB signaling in Alzheimer's disease. J. Neuroinflammation 17:305. doi: 10.1186/s12974-020-01967-2
Kovalska, M., Hnilicova, P., Kalenska, D., Adamkov, M., Kovalska, L., and Lehotsky, J. (2023). Alzheimer's disease-like pathological features in the dorsal hippocampus of wild-type rats subjected to methionine-diet-evoked mild hyperhomocysteinaemia. Cells 12:2087. doi: 10.3390/cells12162087
Lauretti, E., Dincer, O., and Praticò, D. (2020). Glycogen synthase kinase-3 signaling in Alzheimer's disease. Biochim. Biophys. Acta Mol. Cell Res. 1867:118664. doi: 10.1016/j.bbamcr.2020.118664
Lebel, M., Patenaude, C., Allyson, J., Massicotte, G., and Cyr, M. (2009). Dopamine D1 receptor activation induces tau phosphorylation via cdk5 and GSK3 signaling pathways. Neuropharmacology 57, 392–402. doi: 10.1016/j.neuropharm.2009.06.041
Lee, S. J., Chung, Y. H., Joo, K. M., Lim, H. C., Jeon, G. S., Kim, D., et al. (2006). Age-related changes in glycogen synthase kinase 3beta (GSK3beta) immunoreactivity in the central nervous system of rats. Neurosci. Lett. 409, 134–139. doi: 10.1016/j.neulet.2006.09.026
Leroy, K., Yilmaz, Z., and Brion, J. P. (2007). Increased level of active GSK-3beta in Alzheimer's disease and accumulation in argyrophilic grains and in neurones at different stages of neurofibrillary degeneration. Neuropathol. Appl. Neurobiol. 33, 43–55. doi: 10.1111/j.1365-2990.2006.00795.x
Li, D., Yu, S., Long, Y., Shi, A., Deng, J., Ma, Y., et al. (2022). Tryptophan metabolism: mechanism-oriented therapy for neurological and psychiatric disorders. Front. Immunol. 13:985378. doi: 10.3389/fimmu.2022.985378
Li, H. Q., Ip, S. P., Yuan, Q. J., Zheng, G. Q., Tsim, K. K. W., Dong, T. T. X., et al. (2019). Isorhynchophylline ameliorates cognitive impairment via modulating amyloid pathology, tau hyperphosphorylation and neuroinflammation: studies in a transgenic mouse model of Alzheimer's disease. Brain Behav. Immun. 82, 264–278. doi: 10.1016/j.bbi.2019.08.194
Li, M. L., Cao, S. Y., Qu, J., Zhang, L., Gao, Q., Wang, X., et al. (2023). S-adenosyl-L-methionine supplementation alleviates damaged intestinal epithelium and inflammatory infiltration caused by Mat2a deficiency. Development 150:dev201135. doi: 10.1242/dev.201135
Li, Y. C., and Gao, W. J. (2011). GSK-3β activity and hyperdopamine-dependent behaviors. Neurosci. Biobehav. Rev. 35, 645–654. doi: 10.1016/j.neubiorev.2010.08.001
Li, Y. D., Luo, Y. J., Xie, L., Tart, D. S., Sheehy, R. N., Zhang, L., et al. (2023). Activation of hypothalamic-enhanced adult-born neurons restores cognitive and affective function in Alzheimer's disease. Cell Stem Cell 30, 415-432.e416. doi: 10.1016/j.stem.2023.02.006
Lin, Y. T., Seo, J., Gao, F., Feldman, H. M., Wen, H. L., Penney, J., et al. (2018). APOE4 causes widespread molecular and cellular alterations associated with Alzheimer's disease phenotypes in human iPSC-derived brain cell types. Neuron 98, 1141-1154.e1147. doi: 10.1016/j.neuron.2018.05.008
Lu, S. C., Alvarez, L., Huang, Z. Z., Chen, L., An, W., Corrales, F. J., et al. (2001). Methionine adenosyltransferase 1A knockout mice are predisposed to liver injury and exhibit increased expression of genes involved in proliferation. Proc. Natl. Acad. Sci. U.S.A. 98, 5560–5565. doi: 10.1073/pnas.091016398
Luchsinger, J. A., and Mayeux, R. (2004). Dietary factors and Alzheimer's disease. Lancet Neurol. 3, 579–587. doi: 10.1016/S1474-4422(04)00878-6
Mahmoodkhani, M., Ghasemi, M., Derafshpour, L., Amini, M., and Mehranfard, N. (2022). Developmental effects of early-life stress on dopamine D2 receptor and proteins involved in noncanonical D2 dopamine receptor signaling pathway in the prefrontal cortex of male rats. J. Complement. Integr. Med. 19, 697–703. doi: 10.1515/jcim-2020-0539
Martin, L., Page, G., and Terro, F. (2011). Tau phosphorylation and neuronal apoptosis induced by the blockade of PP2A preferentially involve GSK3?. Neurochem. Int. 59, 235–250. doi: 10.1016/j.neuint.2011.05.010
Martínez-Chantar, M. L., Vázquez-Chantada, M., Ariz, U., Martínez, N., Varela, M., Luka, Z., et al. (2008). Loss of the glycine N-methyltransferase gene leads to steatosis and hepatocellular carcinoma in mice. Hepatology 47, 1191–1199. doi: 10.1002/hep.22159
Mielech, A., Puścion-Jakubik, A., Markiewicz-Żukowska, R., and Socha, K. (2020). Vitamins in Alzheimer's disease-review of the latest reports. Nutrients 12:3458. doi: 10.3390/nu12113458
Miller, E. C., Teravskis, P. J., Dummer, B. W., Zhao, X., Huganir, R. L., and Liao, D. (2014). Tau phosphorylation and tau mislocalization mediate soluble Aβ oligomer-induced AMPA glutamate receptor signaling deficits. Eur. J. Neurosci. 39, 1214–1224. doi: 10.1111/ejn.12507
Murty, V. P., Sambataro, F., Radulescu, E., Altamura, M., Iudicello, J., Zoltick, B., et al. (2011). Selective updating of working memory content modulates meso-cortico-striatal activity. Neuroimage 57, 1264–1272. doi: 10.1016/j.neuroimage.2011.05.006
Neuner, S. M., Heuer, S. E., Huentelman, M. J., O'Connell, K. M. S., and Kaczorowski, C. C. (2019a). Harnessing genetic complexity to enhance translatability of Alzheimer's disease mouse models: a path toward precision medicine. Neuron 101, 399-411.e395. doi: 10.1016/j.neuron.2018.11.040
Neuner, S. M., Heuer, S. E., Zhang, J. G., Philip, V. M., and Kaczorowski, C. C. (2019b). Identification of pre-symptomatic gene signatures that predict resilience to cognitive decline in the genetically diverse AD-BXD model. Front. Genet. 10:35. doi: 10.3389/fgene.2019.00035
Nobili, A., Latagliata, E. C., Viscomi, M. T., Cavallucci, V., Cutuli, D., Giacovazzo, G., et al. (2017). Dopamine neuronal loss contributes to memory and reward dysfunction in a model of Alzheimer's disease. Nat. Commun. 8:14727. doi: 10.1038/ncomms14727
Nuru, M., Muradashvili, N., Kalani, A., Lominadze, D., and Tyagi, N. (2018). High methionine, low folate and low vitamin B6/B12 (HM-LF-LV) diet causes neurodegeneration and subsequent short-term memory loss. Metab. Brain Dis. 33, 1923–1934. doi: 10.1007/s11011-018-0298-z
Ossenkoppele, R., van der Kant, R., and Hansson, O. (2022). Tau biomarkers in Alzheimer's disease: towards implementation in clinical practice and trials. Lancet Neurol. 21, 726–734. doi: 10.1016/S1474-4422(22)00168-5
Pan, K., Chen, S., Wang, Y., Yao, W., and Gao, X. (2021). MicroRNA-23b attenuates tau pathology and inhibits oxidative stress by targeting GnT-III in Alzheimer's disease. Neuropharmacology 196:108671. doi: 10.1016/j.neuropharm.2021.108671
Parkhitko, A. A., Jouandin, P., Mohr, S. E., and Perrimon, N. (2019). Methionine metabolism and methyltransferases in the regulation of aging and lifespan extension across species. Aging Cell 18:e13034. doi: 10.1111/acel.13034
Philip, V. M., Duvvuru, S., Gomero, B., Ansah, T. A., Blaha, C. D., Cook, M. N., et al. (2010). High-throughput behavioral phenotyping in the expanded panel of BXD recombinant inbred strains. Genes Brain Behav. 9, 129–159. doi: 10.1111/j.1601-183X.2009.00540.x
Pi, T., Wei, S., Jiang, Y., and Shi, J. S. (2021). High methionine diet-induced Alzheimer's disease like symptoms are accompanied by 5-methylcytosine elevated levels in the brain. Behav. Neurol. 2021:6683318. doi: 10.1155/2021/6683318
Puris, E., Saveleva, L., de Sousa Maciel, I., Kanninen, K. M., Auriola, S., and Fricker, G. (2023). Protein expression of amino acid transporters is altered in isolated cerebral microvessels of 5xFAD mouse model of Alzheimer's disease. Mol. Neurobiol. 60, 732–748. doi: 10.1007/s12035-022-03111-y
Qu, C., Li, Q. P., Su, Z. R., Ip, S. P., Yuan, Q. J., Xie, Y. L., et al. (2022). Nano-Honokiol ameliorates the cognitive deficits in TgCRND8 mice of Alzheimer's disease via inhibiting neuropathology and modulating gut microbiota. J. Adv. Res. 35, 231–243. doi: 10.1016/j.jare.2021.03.012
Ren, B., Wang, L., Shi, L., Jin, X., Liu, Y., Liu, R. H., et al. (2021). Methionine restriction alleviates age-associated cognitive decline via fibroblast growth factor 21. Redox Biol. 41:101940. doi: 10.1016/j.redox.2021.101940
Schott, B. H., Seidenbecher, C. I., Fenker, D. B., Lauer, C. J., Bunzeck, N., Bernstein, H. G., et al. (2006). The dopaminergic midbrain participates in human episodic memory formation: evidence from genetic imaging. J. Neurosci. 26, 1407–1417. doi: 10.1523/JNEUROSCI.3463-05.2006
Schott, B. H., Sellner, D. B., Lauer, C. J., Habib, R., Frey, J. U., Guderian, S., et al. (2004). Activation of midbrain structures by associative novelty and the formation of explicit memory in humans. Learn. Mem. 11, 383–387. doi: 10.1101/lm.75004
Song, P., Peng, W., Sauve, V., Fakih, R., Xie, Z., Ysselstein, D., et al. (2023). Parkinson's disease-linked parkin mutation disrupts recycling of synaptic vesicles in human dopaminergic neurons. Neuron 111, 3775-3788.e3777. doi: 10.1016/j.neuron.2023.08.018
Soria Lopez, J. A., González, H. M., and Léger, G. C. (2019). Alzheimer's disease. Handb. Clin. Neurol. 167, 231–255. doi: 10.1016/B978-0-12-804766-8.00013-3
Steen, E., Terry, B. M., Rivera, E. J., Cannon, J. L., Neely, T. R., Tavares, R., et al. (2005). Impaired insulin and insulin-like growth factor expression and signaling mechanisms in Alzheimer's disease–is this type 3 diabetes? J. Alzheimers Dis. 7, 63–80. doi: 10.3233/jad-2005-7107
Szklarczyk, D., Gable, A. L., Lyon, D., Junge, A., Wyder, S., Huerta-Cepas, J., et al. (2019). STRING v11: protein-protein association networks with increased coverage, supporting functional discovery in genome-wide experimental datasets. Nucleic Acids Res. 47, D607–D613. doi: 10.1093/nar/gky1131
Tanaka, T., Ohashi, S., Takashima, A., and Kobayashi, S. (2022). Dendritic distribution of CDK5 mRNA and p35 mRNA, and a glutamate-responsive increase of CDK5/p25 complex contribute to tau hyperphosphorylation. Biochim. Biophys. Acta 1866:130135. doi: 10.1016/j.bbagen.2022.130135
Thota, R. N., Rosato, J. I., Dias, C. B., Burrows, T. L., Martins, R. N., and Garg, M. L. (2020). Dietary supplementation with curcumin reduce circulating levels of glycogen synthase kinase-3β and islet amyloid polypeptide in adults with high risk of Type 2 diabetes and Alzheimer's disease. Nutrients 12:1032. doi: 10.3390/nu12041032
Trushina, E., Dutta, T., Persson, X. M., Mielke, M. M., and Petersen, R. C. (2013). Identification of altered metabolic pathways in plasma and CSF in mild cognitive impairment and Alzheimer's disease using metabolomics. PLoS ONE8:e63644. doi: 10.1371/journal.pone.0063644
Valls-Pedret, C., Sala-Vila, A., Serra-Mir, M., Corella, D., de la Torre, R., Martínez-González, M., et al. (2015). Mediterranean diet and age-related cognitive decline: a randomized clinical trial. JAMA Intern. Med. 175, 1094–1103. doi: 10.1001/jamainternmed.2015.1668
van den Brink, A. C., Brouwer-Brolsma, E. M., Berendsen, A. A. M., and van de Rest, O. (2019). The Mediterranean, dietary approaches to stop hypertension (DASH), and Mediterranean-DASH intervention for neurodegenerative delay (MIND) diets are associated with less cognitive decline and a lower risk of Alzheimer's disease-a review. Adv. Nutr. 10, 1040–1065. doi: 10.1093/advances/nmz054
Wang, C., Xiong, M., Gratuze, M., Bao, X., Shi, Y., Andhey, P. S., et al. (2021). Selective removal of astrocytic APOE4 strongly protects against tau-mediated neurodegeneration and decreases synaptic phagocytosis by microglia. Neuron 109, 1657-1674.e1657. doi: 10.1016/j.neuron.2021.03.024
Wang, X., Pandey, A. K., Mulligan, M. K., Williams, E. G., Mozhui, K., Li, Z., et al. (2016). Joint mouse-human phenome-wide association to test gene function and disease risk. Nat. Commun. 7:10464. doi: 10.1038/ncomms10464
Wang, Y., Rong, X., Guan, H., Ouyang, F., Zhou, X., Li, F., et al. (2023). The potential effects of isoleucine restricted diet on cognitive impairment in high-fat-induced obese mice via gut microbiota-brain axis. Mol. Nutr. Food Res. 67:e2200767. doi: 10.1002/mnfr.202200767
Xi, Y., Zhang, Y., Zhou, Y., Liu, Q., Chen, X., Liu, X., et al. (2023). Effects of methionine intake on cognitive function in mild cognitive impairment patients and APP/PS1 Alzheimer's disease model mice: role of the cystathionine-β-synthase/H(2)S pathway. Redox Biol. 59:102595. doi: 10.1016/j.redox.2022.102595
Xu, F., Chen, A., Pan, S., Wu, Y., He, H., Han, Z., et al. (2024). Systems genetics analysis reveals the common genetic basis for pain sensitivity and cognitive function. CNS Neurosci. Ther. 30:e14557. doi: 10.1111/cns.14557
Xu, F., Gao, J., Bergmann, S., Sims, A. C., Ashbrook, D. G., Baric, R. S., et al. (2020). Genetic dissection of the regulatory mechanisms of Ace2 in the infected mouse lung. Front. Immunol. 11:607314. doi: 10.3389/fimmu.2020.607314
Xu, L., Furlotte, N., Lin, Y., Heinrich, K., Berry, M. W., George, E. O., et al. (2011). Functional cohesion of gene sets determined by latent semantic indexing of PubMed abstracts. PLoS ONE6:e18851. doi: 10.1371/journal.pone.0018851
Xu, Q. Q., Su, Z. R., Yang, W., Zhong, M., Xian, Y. F., and Lin, Z. X. (2023). Patchouli alcohol attenuates the cognitive deficits in a transgenic mouse model of Alzheimer's disease via modulating neuropathology and gut microbiota through suppressing C/EBPβ/AEP pathway. J. Neuroinflammation 20:19. doi: 10.1186/s12974-023-02704-1
Xu, Y., Yang, Y., Li, B., Xie, Y., Shi, Y., and Le, G. (2022). Dietary methionine restriction improves gut microbiota composition and prevents cognitive impairment in D-galactose-induced aging mice. Food Funct. 13, 12896–12914. doi: 10.1039/D2FO03366F
Yang, P. W., Jiao, J. Y., Chen, Z., Zhu, X. Y., and Cheng, C. S. (2022). Keep a watchful eye on methionine adenosyltransferases, novel therapeutic opportunities for hepatobiliary and pancreatic tumours. Biochim. Biophys. Acta Rev. Cancer 1877:188793. doi: 10.1016/j.bbcan.2022.188793
Yang, W., Liu, Y., Xu, Q. Q., Xian, Y. F., and Lin, Z. X. (2020). Sulforaphene ameliorates neuroinflammation and hyperphosphorylated tau protein via regulating the PI3K/Akt/GSK-3? pathway in experimental models of Alzheimer's disease. Oxid. Med. Cell. Longev. 2020:4754195. doi: 10.1155/2020/4754195
Yang, W., Xu, Q. Q., Yuan, Q., Xian, Y. F., and Lin, Z. X. (2023). Sulforaphene, a CDK5 Inhibitor, attenuates cognitive deficits in a transgenic mouse model of Alzheimer's disease via reducing Aβ Deposition, tau hyperphosphorylation and synaptic dysfunction. Int. Immunopharmacol. 114:109504. doi: 10.1016/j.intimp.2022.109504
Zhang, J., Cao, Q., Li, S., Lu, X., Zhao, Y., Guan, J. S., et al. (2013). 3-Hydroxybutyrate methyl ester as a potential drug against Alzheimer's disease via mitochondria protection mechanism. Biomaterials 34, 7552–7562. doi: 10.1016/j.biomaterials.2013.06.043
Zhao, Y., Dong, X., Chen, B., Zhang, Y., Meng, S., Guo, F., et al. (2022). Blood levels of circulating methionine components in Alzheimer's disease and mild cognitive impairment: a systematic review and meta-analysis. Front. Aging Neurosci. 14:934070. doi: 10.3389/fnagi.2022.934070
Zhou, Y., Li, H., Liu, X., Chi, X., Gu, Z., Cui, B., et al. (2023). The combination of quantitative proteomics and systems genetics analysis reveals that PTN is associated with sleep-loss-induced cognitive impairment. J. Proteome Res. 22, 2936–2949. doi: 10.1021/acs.jproteome.3c00269
Zhu, Y., Zhang, C., Xu, F., Zhao, M., Bergquist, J., Yang, C., et al. (2020). System biology analysis reveals the role of voltage-dependent anion channel in mitochondrial dysfunction during non-alcoholic fatty liver disease progression into hepatocellular carcinoma. Cancer Sci. 111, 4288–4302. doi: 10.1111/cas.14651
Keywords: Alzheimer's disease, methionine, GSK-3β, dopaminergic synapse, GeneNetwork
Citation: Wang C, Hei Y, Liu Y, Bajpai AK, Li Y, Guan Y, Xu F and Yao C (2024) Systems genetics identifies methionine as a high risk factor for Alzheimer's disease. Front. Neurosci. 18:1381889. doi: 10.3389/fnins.2024.1381889
Received: 04 February 2024; Accepted: 25 June 2024;
Published: 16 July 2024.
Edited by:
Willayat Yousuf Wani, Northwestern University, United StatesReviewed by:
Janakiraman Udaiyappan, Southern Methodist University, United StatesCopyright © 2024 Wang, Hei, Liu, Bajpai, Li, Guan, Xu and Yao. This is an open-access article distributed under the terms of the Creative Commons Attribution License (CC BY). The use, distribution or reproduction in other forums is permitted, provided the original author(s) and the copyright owner(s) are credited and that the original publication in this journal is cited, in accordance with accepted academic practice. No use, distribution or reproduction is permitted which does not comply with these terms.
*Correspondence: Cuifang Yao, eWFvY3VpZmFuZ2Jpb0AxMjYuY29t; Fuyi Xu, eHVmdXlpcGhkQGdhbWlsLmNvbQ==
†These authors have contributed equally to this work
Disclaimer: All claims expressed in this article are solely those of the authors and do not necessarily represent those of their affiliated organizations, or those of the publisher, the editors and the reviewers. Any product that may be evaluated in this article or claim that may be made by its manufacturer is not guaranteed or endorsed by the publisher.
Research integrity at Frontiers
Learn more about the work of our research integrity team to safeguard the quality of each article we publish.