- 1Department of Neurological Surgery, University of Virginia Health System, Charlottesville, VA, United States
- 2Department of Neuroscience, University of Virginia, Charlottesville, VA, United States
- 3Department of Neurosurgery, Westchester Medical Center and New York Medical College, Valhalla, NY, United States
- 4Department of Focused Ultrasound Cancer Immunotherapy Center, University of Virginia, Charlottesville, VA, United States
- 5Biomedical Engineering, University of Virginia, Charlottesville, VA, United States
- 6School of Medicine, St. John’s Neuroscience Institute, University of Oklahoma, Tulsa, OK, United States
The Wnt pathway plays critical roles in neurogenesis. The expression of Axin2 is induced by Wnt/β-catenin signaling, making this gene a reliable indicator of canonical Wnt activity. We employed pulse-chase genetic lineage tracing with the Axin2-CreERT2 allele to follow the fate of Axin2+ lineage in the adult hippocampal formation. We found Axin2 expressed in astrocytes, neurons and endothelial cells, as well as in the choroid plexus epithelia. Simultaneously with the induction of Axin2 fate mapping by tamoxifen, we marked the dividing cells with 5-ethynyl-2′-deoxyuridine (EdU). Tamoxifen induction led to a significant increase in labeled dentate gyrus granule cells three months later. However, none of these neurons showed any EdU signal. Conversely, six months after the pulse-chase labeling with tamoxifen/EdU, we identified granule neurons that were positive for both EdU and tdTomato lineage tracer in each animal. Our data indicates that Axin2 is expressed at multiple stages of adult granule neuron differentiation. Furthermore, these findings suggest that the integration process of adult-born neurons from specific cell lineages may require more time than previously thought.
Introduction
The adult hippocampus generates new neurons from radial glia-like neural stem cells (NSCs) that are slowly dividing, or quiescent. After activation they proliferate asymmetrically to generate intermediate progenitor cells (IPCs), which differentiate further into neuroblasts. These neuroblasts develop into immature neurons and subsequently into mature granule cells that form synaptic connections and become integrated into the hippocampal circuitry (Denoth-Lippuner and Jessberger, 2021).
It is widely accepted that the dentate gyrus undergoes continuous production and integration of granule cells well into adulthood (Kempermann et al., 2018). Postnatally generated granule cells undergo a controlled maturation and differentiation process, followed by integration into existing networks (Abrous et al., 2005). Although not completely understood, it is thought that immature granule cells work with mature granule cells to integrate sensory stimuli into context, allowing for subsequent contextual discrimination of recurrent similar stimuli (Tuncdemir et al., 2019). More recent work has allowed for recording of the in vivo activity of immature, adult born granule cells. These younger adult-born granule cells in the hippocampus appear to fire more often but show less spatial tuning specificity than mature granule cells (Danielson et al., 2016). Conversely, it has been proposed that adult-born granule cells transiently support sparser hippocampal population activity structure for effective mnemonic information processing (Mchugh et al., 2022). The adult-born neurons are also morphologically distinct from neonatally-born neurons (Cole et al., 2020). Notably, it is estimated that adult neurogenesis produces half of the granule cells in the mouse dentate gyrus (Cole et al., 2020).
Several signaling pathways, such as Hedgehog, Notch and Wnt, regulate various facets of neural stem cell (NSC) behavior (Alvarez-Buylla and Lim, 2004; Kriegstein and Alvarez-Buylla, 2009). Both in the CNS and in other tissues, Wnt signals act as morphogens in a concentration-dependent manner to control progenitor proliferation, tissue domains, and cell fate specification (Grigoryan et al., 2008; Wang et al., 2012). Moreover, canonical Wnt/β-catenin has a pivotal function in neuronal circuit formation (Inestrosa and Varela-Nallar, 2015). Specifically, Axin2 is one of the first genes induced by canonical Wnt signals, and it has proved to be a valuable genetic tool in the Wnt research field. Wnt/β-catenin-responsive cells have been tracked in vivo in many tissues and disease contexts using an Axin2-CreERT2 allele model (Van Amerongen et al., 2012). This tamoxifen inducible line has been also used to detect neurogenesis and trace neuronal lineages in vivo (Bowman et al., 2013).
The time required for neural stem cells to mature and integrate fully into existing neural networks is still a subject of debate. In 2011, Encinas and coworkers reported that quiescent neural progenitors in the mouse dentate gyrus develop into fully mature cells through multiple controlled and regulated divisions (Encinas et al., 2011). They showed that this entire maturation process, from quiescent neuronal precursors to mature new cells, takes approximately 1 month. More recently, Goncalves et al. reported that these new cells form functional connections within 2–3 weeks after their last mitosis, thus integrating into already established networks more quickly than previously reported (Goncalves et al., 2016).
In order to further advance our current understanding of the role that the Wnt/β-catenin pathway plays in adult neurogenesis in the dentate gyrus, we conducted a neuronal lineage tracing experiment to measure the proliferation of Axin2+ lineage in a pulse-chase manner. We show that the first evidence of mature granule cells derived from adult neurogenesis is seen 6 months after the initial labeling with Axin2-CreERT2. We propose that different cell lineages might have a different pace of integration during adult neurogenesis.
Materials and methods
Animals and treatments
The Axin2CreERT2 mice (Bowman et al., 2013) (Strain #: 018867, RRID: IMSR_JAX:018867) were purchased from JAX Mice (The Jackson Laboratory, Bar Harbor, ME) and bred in-house. The PC::G5-tdT line (Gee et al., 2014) is maintained in our laboratory. All experiments were carried out in 10-12-week-old mice at the time of induction. All animals were fed standard rodent chow and housed under 12-h light/12-h dark cycle. Four groups of 3 mice were analyzed, for a total of 24 bilateral hippocampal samples. All mice received tamoxifen (100 mg/kg; i.p.) and 5-ethynyl-2′-deoxyuridine (EdU) (40 mg/kg body weight; i.p.) on day t = 0. The animals were sacrificed and assessed after 7, 30, 90 and 180 days, based on random assignment to the pulse-chase group.
Histology and immunohistochemistry
Mice were transcardially perfused with PBS and 4% phosphate-buffered formaldehyde. Brains were then dissected, post-fixed in 4% PFA and equilibrated in 10 and 30% sucrose, followed by embedding in OCT. Frozen brains were sectioned coronally at 25-μm thickness and stored at −20°C. For staining, the sections were rehydrated with PBS and permeabilized with 0.1% Triton X-100 (Sigma, United States) for 1 h at room temperature. Next, the slides were incubated for 30 min at room temperature and processed with Click-iT™ EdU Alexa Fluor™ 488 Imaging kit (Fisher Scientific), followed by after washes with Triton X-100 in PBS. Next, immunostaining was performed with rabbit anti-Red Fluorescent Protein (RFP, 1:500, Rockland, United States), guinea pig anti-doublecortin (DCX, 1:500, Millipore Sigma, USA); mouse anti-glial fibrillary acidic protein (GFAP, 1:1000, Millipore Sigma, United States), anti-NeuN (1:500, Millipore Sigma, United States), anti-Iba1 (Wako) and anti-Transthyretin (abcam). Secondary antibodies included donkey anti-rabbit Alexa 568 (1:500, Molecular Probes, Invitrogen); goat anti-guinea pig Alexa 647 (1:500, Abcam, ab150187); donkey anti-mouse Alexa 647 (1:500, Molecular Probes, Invitrogen). Slides were mounted with ProLong™ Gold antifade (Invitrogen, United States).
Confocal microscopy and image analysis
Fluorescence signals were imaged with Zeiss LSM-880 with Airyscan confocal microscope (Zeiss, Germany) using sequential scanning mode for Alexa 405, 488, 568 and 647. Stacks of images (1,024 × 1,024 pixels) were tiled across the dentate gyrus area. Dentate gyri were segmented in the tiled 3D datasets with Imaris 9 (Bitplane, Oxford), and the cells labeled with specific antibodies were detected and counted within the segmented dentate gyrus using the Spots model. Total cell counts in the segmented regions were then normalized per 1,000 DAPI-positive nuclei in the dentate gyrus.
Statistical analysis
The results were expressed as Mean ± standard deviation (SD). In all experiments, the statistical significance was set at p < 0.05. Calculations were performed with one-way ANOVA with post-hoc Tukey HSD test, or post-test for liner trend, using the statistical package software Prism 6 (GraphPad, San Diego, CA).
Results
Axin2CreERT2 genetically labels neuronal, glial and endothelial cells in the dentate gyrus
To identify Axin2-expressing cells in the hippocampus, we crossed Axin2CreERT2 mice (Van Amerongen et al., 2012) to the reporter line expressing tdTomato following Cre recombination (PC::G5-tdT) (Gee et al., 2014), and analyzed the progeny with immunohistochemistry. Previous reports induced the Axin2CreERT2 allele in embryonic and juvenile mice (Bowman et al., 2013). Consistent, adult-induced lineage labeled similar cell types in the hippocampus, one week after a single injection of tamoxifen (Figure 1). In the granule layer of dentate gyrus, a subset of Axin2-lineage-positive granule neurons was observed with mature appearing dendrites in the molecular layer. These cells co-labeled with the neuronal marker NeuN (Figure 1A). In the sub granular zone, GFAP-positive, ramified cells were observed with a characteristic appearance of astrocytes (Figure 1B). Axin2+ lineage cells lacking dendritic morphology were frequently detected in the subgranular zone. Some of these cells co-stained with doublecortin (DCX), a marker of immature neurons (La Rosa et al., 2020) (Figure 1C). We also show that Axin2 is expressed in endothelial cells on a subset of blood vessels co-staining with PECAM1/CD31 (Figure 1D). Of note, in the third ventricle choroid plexus adjacent to hippocampus, we have also detected Axin2+ lineage in the choroid epithelia, but not in myeloid cells (Supplementary Figure S1). Together, Axin2 is expressed in several cell types in the hippocampal neurovascular unit, showing increased density in the subgranular zone.
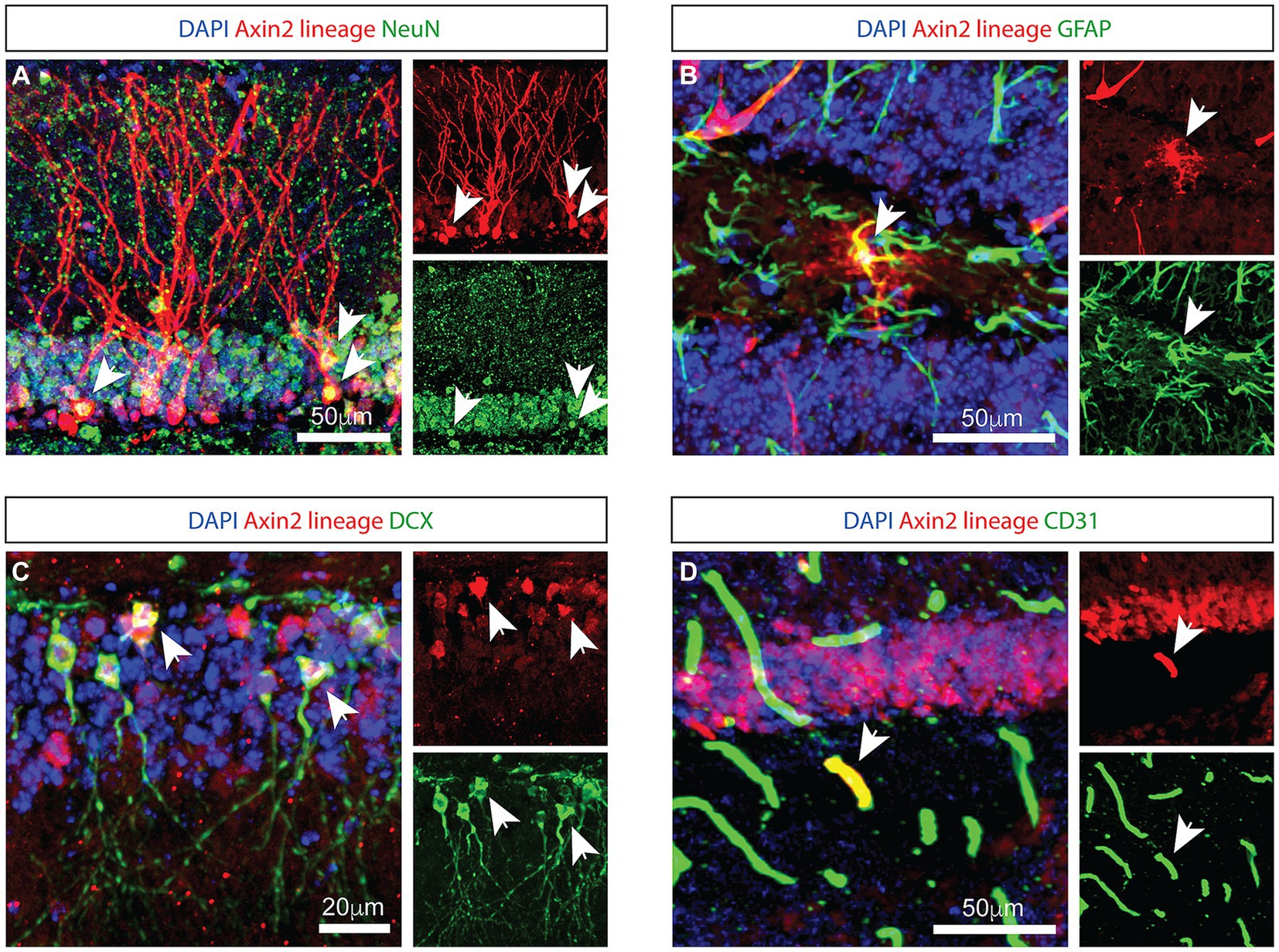
Figure 1. Genetic labeling with Axin2 CreERT2 reveals neural, astrocytic, and endothelial cells. (A) Axin2-labeled tdTomato-positive neurons in the granule layer of the dentate gyrus are NeuN-positive. Arrowheads identify corresponding neuronal cell bodies in the 3-channel overlay (left), as well as in the specific channels showing tdTomato (upper right) and NeuN (lower right). (B) Axin2-labeled astrocytes in the dentate gyrus region co-stain with GFAP antibodies. (C) Co-staining with doublecortin (DCX) identifies neuroblasts positive for Axin2 lineage markers. (D) Axin2CreERT2 also labels a subset of endothelial cells in the dentate gyrus region which react with antibodies to CD31. In all panels, white arrows identify double-positive cells. Scale bars, 50 μm, unless indicated otherwise.
Dynamics of the labeled Axin2+ cell population in the granular layer
To follow the fate of Axin2+ lineage in dentate gyrus neurogenesis over time, we performed a time course analysis of granule cells labeled with the tracer tdTomato. Adult mice were induced with tamoxifen (100 mg/kg) and followed over time from 1 week to 6 months (Figure 2A). Differentiated neurons with mature dendrites in the dentate gyrus were counted and normalized per 1,000 DAPI-positive nuclei detected with image analysis (Figures 3A,E,I; Methods). Our analysis revealed a statistically significant 6-fold increase (from 2.0 ± 1.1 after 1 week, to 12.0 ± 3.7 after 3 months) in the density of Axin2 granular cells in the animals sacrificed 90 days after tamoxifen injection when compared to the 1-week time point (Figure 2K). There was also a statistically significant positive linear trend for the first 3 months (p = 0.0432, ANOVA, post-test for trend). The Axin2-lineage-positive granule cell population peaked around 3 months, but subsequently decreased by 6 months (Figure 2). It is also noteworthy that the Axin2CreERT2 line demonstrates baseline leakiness of recombination. As a result, a small number of cells, including granule neurons, become labeled with the tracer even without tamoxifen induction (Supplementary Figure S2). To compensate for this background leakiness, we have measured the number of false-positive granule neurons in three animals and subtracted the normalized and averaged counts from the nominal values determined at all chase time points in this study, as described in the Methods and Supplementary Figure S2.
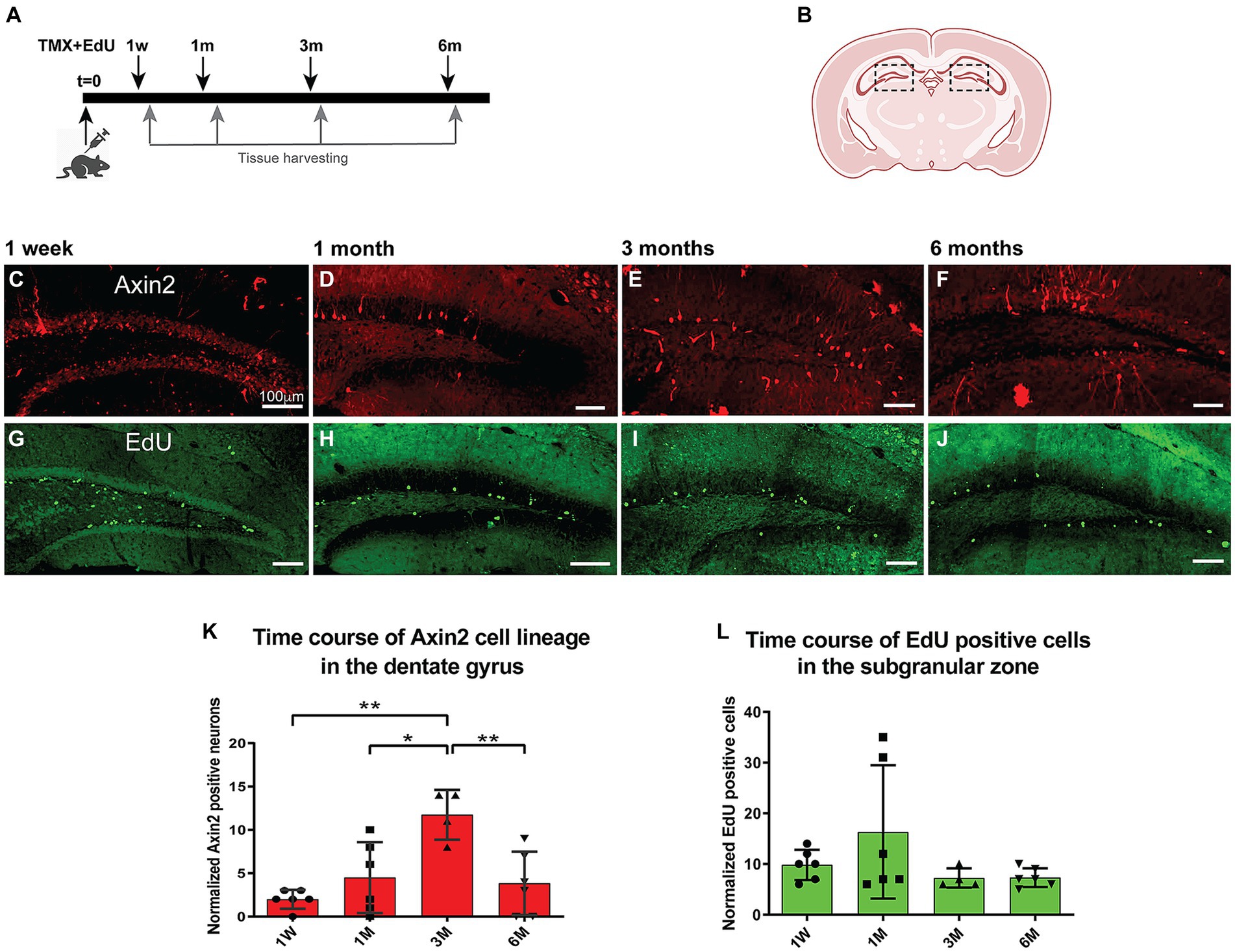
Figure 2. Time course of adult induced Axin2 cell population in the dentate gyrus. (A) Schematic diagram showing the pulse-chase strategy used in this study. Adult 12-wk-old animals were induced with tamoxifen and concurrently injected with a single dose of EdU. The brains were analyzed at 1 week (1 W), 1 month (1 M), 3 months (3 M) and 6 months (6 M) post induction. (B) The central coronal plane used as a landmark for sectioning. The dashed boxes highlight the dentate gyrus (DG) area analyzed in this study. (C–F) Representative confocal images showing tdTomato signal reporting Axin2 cell lineage. (G–J) Typical EdU staining in DG at different chase time points. The bulk of staining moved from subgranular layer to the deeper granular layer of the DG. (K) Graph showing temporal dynamics of the Axin2 cell lineage. (L) Time course of EdU-positive cell density during the chase period (1w – 6 m). Counts in both plots were normalized per 1,000 DAPI positive nuclei. N = 2–3 mice and 12–18 sections for each time point. For each brain, three sections were analyzed. The ipsilateral and contralateral sides from all section were combined and averaged, resulting in two mean values for each animal. *p < 0.05, **p < 0.01. Scale bars, 100 μm.
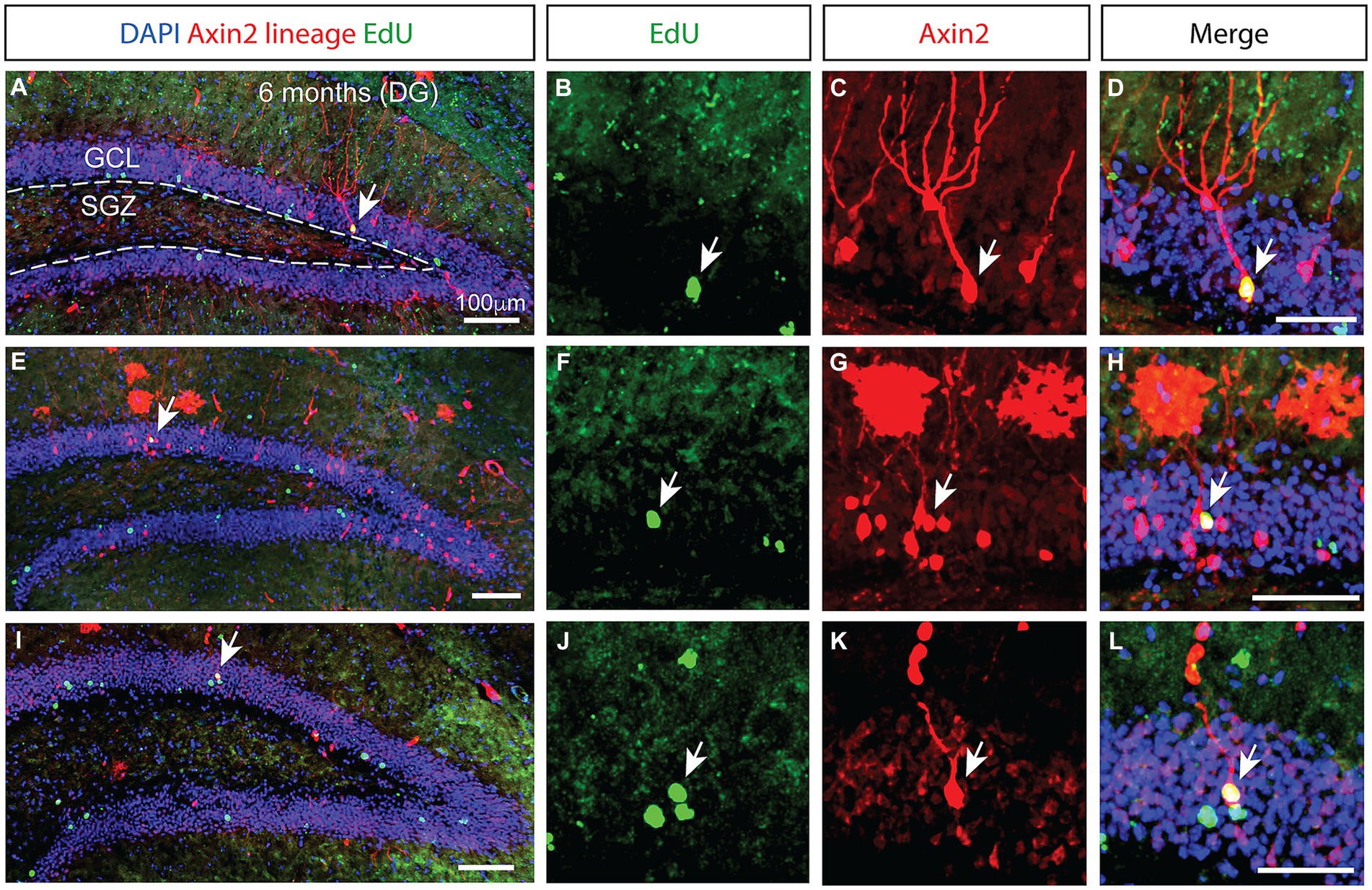
Figure 3. Prolonged integration of mitotically labeled Axin2-positive cell lineage in the granular layer of the dentate gyrus. (A,E,I) Low magnification confocal images of dentate gyrus sections from three different animals 6 months after tamoxifen induction. The staining shows Axin2 cell lineage (red), EdU (green) and DAPI (blue). (A) The granular cell layer (GCL) is outlined with white dashed lines, and the location of the subgranular zone (SGZ) is indicated. Arrows pinpoint the Axin2- and EdU-positive cells. (B,F,J) Higher magnification panels showing the EdU signal in the granule cell layer. (C,G,K) Matching panels showing the tdTomato signal indicating Axin2 cell lineage in the same region as (B,F,J). (D,H,L) Overlay of EdU and Axin2 panels with DAPI signal, highlighting the mitotically labeled granule neurons originating from the Axin2 lineage. Low magnification scale bars, 100 μm. High magnification scale bars, 50 μM. DG, dentate gyrus; GCL, granular cell layer; SGZ, subgranular zone.
The time course of aggregate EdU labeling in the dentate gyrus
Next, we investigated cell proliferation in the Axin2 cell lineage. We performed EdU pulse-chase experiments concurrent with the induction of Axin2CreERT2 labeling. The animals were injected with a single dose of EdU (40 mg/kg, i.p.) concurrently with tamoxifen, and analyzed at the same four time points from 1 week to 6 months (Figures 2G–J). Edu-positive cells were detected and measured in the subgranular zone and granular zone, and the values were normalized per DAPI-stained 1,000 nuclei (Figure 2L). After 1 month, the number of EdU-labeled cells increased 1.5-fold, but this change was not significant. The numbers of EdU-positive cells remained stable at later time points at approximately 7.3 ± 1.9 EdU-positive nuclei per 1,000 DAPI-positive nuclei.
Delayed emergence of Edu-positive Axin2+ granule neurons in the dentate gyrus
While the number of Axin2-lineage-positive granule neurons peaked 3 months following the induction, we found no ramified, differentiated neurons containing EdU marker at this stage. However, in the animals analyzed 6 months after the initial pulse, double positive neurons containing the Axin2 lineage markers as well as the EdU label were detected (Figure 3), indicating their derivation from the Wnt-dependent progenitor pool. The neuronal bodies of these double-positive cells were located in the central part of the granular layer (Figures 3H,L). Additional Axin2-negative nuclei labeled with EdU were seen in the granular layer (Figure 3L), consistent with neuronal identity. Together, our findings confirm that Wnt-dependent Axin2 cell lineage in the adult brain gives rise to a subset of dentate gyrus granule neurons. Surprisingly, the timeline of maturation and integration of these neurons is considerably longer than what is generally accepted as the time interval needed for neuron differentiation during the adult neurogenesis (Figure 4).
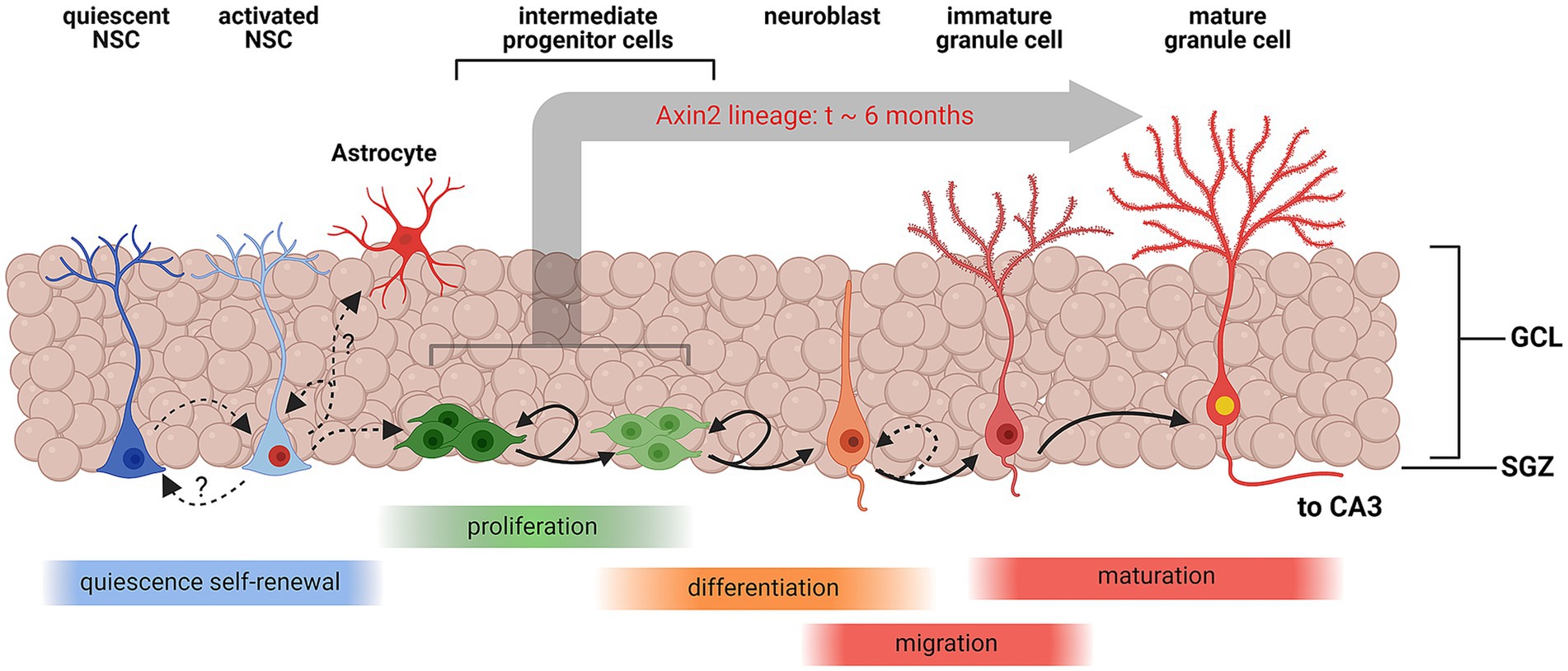
Figure 4. Schematic representation of Wnt-associated subgranular neurogenesis. A summary diagram of the stages in subgranular neurogenesis from quiescent neuronal stem cells (NSCs) to mature granule cells. Quiescent NSCs are multipotent undifferentiated cells. Once induced, quiescent NSCs differentiate into activated NSCs, which may replenish the pool of quiescent NSCs, or commit to the astrocyte maturation pathway. Activated NSCs also develop into multipolar intermediate progenitor cells and neuroblasts which continue to proliferate and eventually migrate inside the granular layer and enter the granule cell maturation pathway. Axin2-positive NSCs and progenitors represent a sub-lineage of the cell lines, contributing to subgranular neurogenesis. In this study, we have unequivocally detected Axin2 lineage markers in the intermediate progenitors and neuroblasts, which are amenable to mitotic labeling, and post-mitotic mature neurons. Our data suggest that the process of integration of progenitors into mature granule cells can take as long as 6 months in this cell lineage. Created with BioRender.com.
Discussion
We have characterized the cell fate of the adult induced Axin2 cell lineage. While others have previously shown that Axin2 expression is active in hippocampus (Bowman et al., 2013), the genetic labeling in their study was induced at the embryonic and juvenile stages within first two postnatal weeks of development. Our experiments confirm that Axin2 plays role in the neurogenesis of granule cells of the adult dentate gyrus. Consistent, Axin2 labeling was observed in DCX-positive immature neurons. Conversely, we have not identified Axin2 in NSC or IPC cells, and more precise characterization of the Axin2 progenitor population is warranted. Axin2 expression has been previously reported in astrocytes (Kalani et al., 2008). It is also possible that these tdTomato-labeled astrocytes are born from the Axin2-positive NSCs (Figure 4), and thus derived from the Axin2 domain in NSC. We have noticed larger counts of tdTomato-labeled astrocytes at 3- and 6-month pulse-chase time points, but these increases were not formally analyzed. Furthermore, we have also shown that Axin2 expression is present in microvascular endothelia, in line with the role of Wnt/β-catenin signaling in angiogenesis and vascular integrity (Daneman et al., 2009; Dejana, 2010; Foulquier et al., 2018; Liebner et al., 2018).
In addition, Axin2 is expressed in the choroid plexus. We found that the Axin2 cell lineage did not label any myeloid cells, neither microglia nor macrophages. Instead, these cells co-stained with Transthyretin, a marker of choroid plexus epithelial cells (Supplementary Figures S1E–H; Vancamp et al., 2019). The choroid plexus generates cerebrospinal fluid, and the epithelia distributes thyroid hormone and secretes numerous factors, including Wnt proteins. Thus, the Axin2-lineage of choroid plexus can potentially affect cerebral proliferative processes in a long-range manner (Kaiser et al., 2019; Vancamp et al., 2019).
The Axin2+ granule neurons presented a dynamic cell population in our experiments. Our measurements imply that the Axin2-lineage-positive granule cell population is replenished from the Axin2-labeled progenitors and increases six-fold after 3 months. Interestingly, our work also showed that the Axin2-lineage-positive granule cells subsequently decreased between 3 months and 6 months. This suggests that these neurons may have a limited lifespan, but additional research is required to explore this possibility.
Encinas et al. have demonstrated that both NSCs and amplifying IPCs decrease with age in mouse lines (Encinas et al., 2011). Yet here we show that the Axin2 arm of the canonical Wnt pathway remains active in the adult dentate gyrus neurogenic niche. Along with other neurogenic factors, like sonic hedgehog (Shh), bone morphogenetic proteins (BMPs), and Notch, Wnt ligands are known regulators of adult hippocampal neurogenesis (Inestrosa and Varela-Nallar, 2015; Urbach and Witte, 2019). Wnts are expressed by local astrocytes and NSCs themselves, acting as paracrine and autocrine factors in the NSC niche (Lie et al., 2005; Wexler et al., 2009). Specific ligands such as Wnt3 have been shown to promote neuroblast proliferation and neuronal differentiation through the canonical Wnt pathway (Lie et al., 2005). In addition, Wnt signaling also emerged as important pathway promoting multipotency and self-renewal of NSCs (Mao et al., 2009). However, the specific roles of Axin2 in these processes remain to be characterized.
One important question to address is whether different subsets of NSCs exist with various degrees of quiescence and distinct abilities to generate neurons and glial cells. Genetic lineage tracing with inducible version of the Cre recombinase under different promoters has provided evidence for the functional heterogeneity of NSCs in the dentate gyrus (Decarolis et al., 2013; Urban et al., 2019). Some NSCs have been shown to be neurogenic and short lived, while others are multipotent and self-renew for longer periods (Ibrayeva et al., 2021). Using genetic labeling with nestin-CreER and performing a double pulse-chase experiment with tamoxifen and BrdU, Encinas detected BrdU- and NeuN-positive granule neurons in the nestin cell lineage 30 days after pulse-chase induction (Encinas et al., 2011). This is in stark contrast with our results suggesting that Axin2 cell lineage requires much longer for neuronal differentiation and integration into granule neurons. Since Axin2CreERT2 labels fewer neuronal progenitors than nestin-CreER, some co-labeled cells could have been missed. It is surprising, however, that in comparison to the 6-month time point, twice as many Axin2+ lineage cells were seen after the 3-month pulse-chase, with a similar general level of EdU staining, yet no tdTomato+ EdU+ neurons were detected (Figures 3K,L). The underrepresentation of EdU-positive neurons at earlier time points may be partially due to dilution of the EdU label in progenitor cells. Conversely, it is unlikely but not entirely impossible that the observed tdTomato labeling in the adult EdU-positive granule cells results from nonspecific activation by the leaky Axin2CreERT2 driver.
We also acknowledge that the genetic labeling approach used in this study was rather sparse, consisting of a single simultaneous injection of tamoxifen and EdU. Designed to facilitate effective lineage tracing, the resulting labeling density did not provide sufficient labeling of stem cells and neuronal progenitors, as stated above. More saturating labeling strategies will have to be employed in future studies to reveal the presence of Axin2 in quiescent adult neural stem cells. During the completion of this study, Luo et al. (2023) conducted a comprehensive study comparing Axin2CreERT2 and Gli1CreERT2 expression in neural stem cells of the adult brain (Luo et al., 2023). The authors observed Axin2CreERT2 activity in both quiescent and activated stem cells 1 month after a single tamoxifen injection at 3, 6, and 12 months. Furthermore, they found a higher number of activated Axin2+ clones compared to Gli1+ clones 1 month after tamoxifen injection, suggesting that Axin2+ adult dentate NSCs are more likely to sustain neurogenesis over time, while Gli1+ adult dentate NSCs tend to become quiescent with age and contribute to the decline in age-induced neurogenesis. However, it should be noted that the leakiness of the Axin2CreERT2 genetic system, demonstrated in our study, may have influenced their measurements.
A potential direction for future research is to explore whether neurogenesis in the aging brain can be safely and non-invasively stimulated. One emerging opportunity is to use focused ultrasound. Focused ultrasound (FUS) is a non-invasive technique that can be used to precisely target regions in the brain for various therapeutic purposes including thermal ablation and drug delivery (Meng et al., 2021). When combined with systemically delivered, gas-filled microbubbles, FUS can safely and transiently increase the permeability of the blood–brain barrier (BBB) to facilitate the delivery of therapeutic agents. Recent reports have demonstrated that FUS mediated BBB opening can induce hippocampal neurogenesis in adult mice, independent of delivery of therapeutic agents (Scarcelli et al., 2014). While the mechanism remains to be elucidated, the neurogenic effect is likely due to localized increases in BDNF, VEGF, and bFGF expression following FUS delivery (Shin et al., 2019) and only in the presence of transient BBB opening (Mooney et al., 2016). Future experiments investigating the relationship between opening BBB, stimulating the Wnt/β-catenin pathway, and utilizing the Axin2CreERT2 cell lineage as a readout could provide valuable insights into the potential for FUS-mediated neurogenesis in various neurodegenerative disorders.
Data availability statement
The original contributions presented in the study are included in the article/Supplementary material, further inquiries can be directed to the corresponding authors.
Ethics statement
The animal study was approved by the Institutional Animal Care and Use Committee (IACUC) at the University of Virginia. The study was conducted in accordance with the local legislation and institutional requirements.
Author contributions
KS: Conceptualization, Data curation, Formal analysis, Investigation, Methodology, Software, Supervision, Visualization, Writing – original draft. FF: Data curation, Formal analysis, Investigation, Methodology, Software, Visualization, Writing – original draft. SS: Conceptualization, Investigation, Methodology, Writing – original draft. MD: Investigation, Methodology, Writing - review & editing. RP: Conceptualization, Funding acquisition, Resources, Writing – review & editing. JS: Resources, Investigation, Writing - review & editing. MK: Conceptualization, Funding acquisition, Project administration, Resources, Supervision, Writing – review & editing. PT: Conceptualization, Writing – original draft, Writing – review & editing, Funding acquisition, Methodology, Project administration, Resources, Software, Supervision.
Funding
The author(s) declare financial support was received for the research, authorship, and/or publication of this article. This work was supported with UVA School of Medicine Dean’s fund to YK, Focused Ultrasound Foundation (FUSF) and, NIH R21NS116431 funding to PT, and NIH R01CA279134 to PT and RP.
Acknowledgments
We thank Drs. Kevin Lee and Elise Cope, Department of Neuroscience, University of Virginia, for critical reading of the manuscript and valuable comments. We also thank Dr. Hyung-song Nam, Sloan Kettering Institute, for helpful comments on the manuscript.
Conflict of interest
The authors declare that the research was conducted in the absence of any commercial or financial relationships that could be construed as a potential conflict of interest.
The author(s) declared that they were an editorial board member of Frontiers, at the time of submission. This had no impact on the peer review process and the final decision.
Publisher’s note
All claims expressed in this article are solely those of the authors and do not necessarily represent those of their affiliated organizations, or those of the publisher, the editors and the reviewers. Any product that may be evaluated in this article, or claim that may be made by its manufacturer, is not guaranteed or endorsed by the publisher.
Supplementary material
The Supplementary material for this article can be found online at: https://www.frontiersin.org/articles/10.3389/fnins.2024.1353142/full#supplementary-material
References
Abrous, D. N., Koehl, M., and Le Moal, M. (2005). Adult neurogenesis: from precursors to network and physiology. Physiol. Rev. 85, 523–569. doi: 10.1152/physrev.00055.2003
Alvarez-Buylla, A., and Lim, D. A. (2004). For the long run: maintaining germinal niches in the adult brain. Neuron 41, 683–686. doi: 10.1016/S0896-6273(04)00111-4
Bowman, A. N., Van Amerongen, R., Palmer, T. D., and Nusse, R. (2013). Lineage tracing with Axin2 reveals distinct developmental and adult populations of Wnt/beta-catenin-responsive neural stem cells. Proc. Natl. Acad. Sci. USA 110, 7324–7329. doi: 10.1073/pnas.1305411110
Cole, J. D., Espinueva, D. F., Seib, D. R., Ash, A. M., Cooke, M. B., Cahill, S. P., et al. (2020). Adult-born hippocampal neurons undergo extended development and are morphologically distinct from Neonatally-born neurons. J. Neurosci. 40, 5740–5756. doi: 10.1523/JNEUROSCI.1665-19.2020
Daneman, R., Agalliu, D., Zhou, L., Kuhnert, F., Kuo, C. J., and Barres, B. A. (2009). Wnt/beta-catenin signaling is required for CNS, but not non-CNS, angiogenesis. Proc. Natl. Acad. Sci. USA 106, 641–646. doi: 10.1073/pnas.0805165106
Danielson, N. B., Kaifosh, P., Zaremba, J. D., Lovett-Barron, M., Tsai, J., Denny, C. A., et al. (2016). Distinct contribution of adult-born hippocampal granule cells to context encoding. Neuron 90, 101–112. doi: 10.1016/j.neuron.2016.02.019
Decarolis, N. A., Mechanic, M., Petrik, D., Carlton, A., Ables, J. L., Malhotra, S., et al. (2013). In vivo contribution of nestin- and GLAST-lineage cells to adult hippocampal neurogenesis. Hippocampus 23, 708–719. doi: 10.1002/hipo.22130
Dejana, E. (2010). The role of wnt signaling in physiological and pathological angiogenesis. Circ. Res. 107, 943–952. doi: 10.1161/CIRCRESAHA.110.223750
Denoth-Lippuner, A., and Jessberger, S. (2021). Formation and integration of new neurons in the adult hippocampus. Nat. Rev. Neurosci. 22, 223–236. doi: 10.1038/s41583-021-00433-z
Encinas, J. M., Michurina, T. V., Peunova, N., Park, J. H., Tordo, J., Peterson, D. A., et al. (2011). Division-coupled astrocytic differentiation and age-related depletion of neural stem cells in the adult hippocampus. Cell Stem Cell 8, 566–579. doi: 10.1016/j.stem.2011.03.010
Foulquier, S., Daskalopoulos, E. P., Lluri, G., Hermans, K. C. M., Deb, A., and Blankesteijn, W. M. (2018). WNT signaling in cardiac and vascular disease. Pharmacol. Rev. 70, 68–141. doi: 10.1124/pr.117.013896
Gee, J. M., Smith, N. A., Fernandez, F. R., Economo, M. N., Brunert, D., Rothermel, M., et al. (2014). Imaging activity in neurons and glia with a Polr2a-based and cre-dependent GCaMP5G-IRES-tdTomato reporter mouse. Neuron 83, 1058–1072. doi: 10.1016/j.neuron.2014.07.024
Goncalves, J. T., Schafer, S. T., and Gage, F. H. (2016). Adult neurogenesis in the Hippocampus: from stem cells to behavior. Cell 167, 897–914. doi: 10.1016/j.cell.2016.10.021
Grigoryan, T., Wend, P., Klaus, A., and Birchmeier, W. (2008). Deciphering the function of canonical Wnt signals in development and disease: conditional loss- and gain-of-function mutations of beta-catenin in mice. Genes Dev. 22, 2308–2341. doi: 10.1101/gad.1686208
Ibrayeva, A., Bay, M., Pu, E., Jorg, D. J., Peng, L., Jun, H., et al. (2021). Early stem cell aging in the mature brain. Cell Stem Cell 28:955:966. doi: 10.1016/j.stem.2021.03.018
Inestrosa, N. C., and Varela-Nallar, L. (2015). Wnt signalling in neuronal differentiation and development. Cell Tissue Res. 359, 215–223. doi: 10.1007/s00441-014-1996-4
Kaiser, K., Gyllborg, D., Prochazka, J., Salasova, A., Kompanikova, P., Molina, F. L., et al. (2019). WNT5A is transported via lipoprotein particles in the cerebrospinal fluid to regulate hindbrain morphogenesis. Nat. Commun. 10:1498. doi: 10.1038/s41467-019-09298-4
Kalani, M. Y., Cheshier, S. H., Cord, B. J., Bababeygy, S. R., Vogel, H., Weissman, I. L., et al. (2008). Wnt-mediated self-renewal of neural stem/progenitor cells. Proc. Natl. Acad. Sci. USA 105, 16970–16975. doi: 10.1073/pnas.0808616105
Kempermann, G., Gage, F. H., Aigner, L., Song, H., Curtis, M. A., Thuret, S., et al. (2018). Human adult neurogenesis: evidence and remaining questions. Cell Stem Cell 23, 25–30. doi: 10.1016/j.stem.2018.04.004
Kriegstein, A., and Alvarez-Buylla, A. (2009). The glial nature of embryonic and adult neural stem cells. Annu. Rev. Neurosci. 32, 149–184. doi: 10.1146/annurev.neuro.051508.135600
La Rosa, C., Parolisi, R., and Bonfanti, L. (2020). Brain structural plasticity: from adult neurogenesis to immature neurons. Front. Neurosci. 14:75. doi: 10.3389/fnins.2020.00075
Lie, D. C., Colamarino, S. A., Song, H. J., Desire, L., Mira, H., Consiglio, A., et al. (2005). Wnt signalling regulates adult hippocampal neurogenesis. Nature 437, 1370–1375. doi: 10.1038/nature04108
Liebner, S., Dijkhuizen, R. M., Reiss, Y., Plate, K. H., Agalliu, D., and Constantin, G. (2018). Functional morphology of the blood-brain barrier in health and disease. Acta Neuropathol. 135, 311–336. doi: 10.1007/s00401-018-1815-1
Luo, X., Dai, M., Wang, M., Wang, X., and Guo, W. (2023). Functional heterogeneity of Wnt-responsive and hedgehog-responsive neural stem cells in the murine adult hippocampus. Dev. Cell 58, 2545–2562. doi: 10.1016/j.devcel.2023.07.021
Mao, Y., Ge, X., Frank, C. L., Madison, J. M., Koehler, A. N., Doud, M. K., et al. (2009). Disrupted in schizophrenia 1 regulates neuronal progenitor proliferation via modulation of GSK3beta/beta-catenin signaling. Cell 136, 1017–1031. doi: 10.1016/j.cell.2008.12.044
Mchugh, S. B., Lopes-Dos-Santos, V., Gava, G. P., Hartwich, K., Tam, S. K. E., Bannerman, D. M., et al. (2022). Adult-born dentate granule cells promote hippocampal population sparsity. Nat. Neurosci. 25, 1481–1491. doi: 10.1038/s41593-022-01176-5
Meng, Y., Hynynen, K., and Lipsman, N. (2021). Applications of focused ultrasound in the brain: from thermoablation to drug delivery. Nat. Rev. Neurol. 17, 7–22. doi: 10.1038/s41582-020-00418-z
Mooney, S. J., Shah, K., Yeung, S., Burgess, A., Aubert, I., and Hynynen, K. (2016). Focused ultrasound-induced neurogenesis requires an increase in blood-brain barrier permeability. PLoS One 11:e0159892. doi: 10.1371/journal.pone.0159892
Scarcelli, T., Jordao, J. F., O'reilly, M. A., Ellens, N., Hynynen, K., and Aubert, I. (2014). Stimulation of hippocampal neurogenesis by transcranial focused ultrasound and microbubbles in adult mice. Brain Stimul. 7, 304–307. doi: 10.1016/j.brs.2013.12.012
Shin, J., Kong, C., Lee, J., Choi, B. Y., Sim, J., Koh, C. S., et al. (2019). Focused ultrasound-induced blood-brain barrier opening improves adult hippocampal neurogenesis and cognitive function in a cholinergic degeneration dementia rat model. Alzheimers Res. Ther. 11:110. doi: 10.1186/s13195-019-0569-x
Tuncdemir, S. N., Lacefield, C. O., and Hen, R. (2019). Contributions of adult neurogenesis to dentate gyrus network activity and computations. Behav. Brain Res. 374:112112. doi: 10.1016/j.bbr.2019.112112
Urbach, A., and Witte, O. W. (2019). Divide or commit - revisiting the role of cell cycle regulators in adult hippocampal neurogenesis. Front. Cell Dev. Biol. 7:55. doi: 10.3389/fcell.2019.00055
Urban, N., Blomfield, I. M., and Guillemot, F. (2019). Quiescence of adult mammalian neural stem cells: a highly regulated rest. Neuron 104, 834–848. doi: 10.1016/j.neuron.2019.09.026
Van Amerongen, R., Bowman, A. N., and Nusse, R. (2012). Developmental stage and time dictate the fate of Wnt/beta-catenin-responsive stem cells in the mammary gland. Cell Stem Cell 11, 387–400. doi: 10.1016/j.stem.2012.05.023
Vancamp, P., Gothie, J. D., Luongo, C., Sebillot, A., Le Blay, K., Butruille, L., et al. (2019). Gender-specific effects of transthyretin on neural stem cell fate in the subventricular zone of the adult mouse. Sci. Rep. 9:19689. doi: 10.1038/s41598-019-56156-w
Wang, X., Kopinke, D., Lin, J., Mcpherson, A. D., Duncan, R. N., Otsuna, H., et al. (2012). Wnt signaling regulates postembryonic hypothalamic progenitor differentiation. Dev. Cell 23, 624–636. doi: 10.1016/j.devcel.2012.07.012
Keywords: adult neurogenesis, dentate gyrus, Wnt signaling, Axin2 cell lineage, focused ultrasound
Citation: Sharifi KA, Farzad F, Soldozy S, DeWitt MR, Price RJ, Sheehan J, Kalani MYS and Tvrdik P (2024) Exploring the dynamics of adult Axin2 cell lineage integration into dentate gyrus granule neurons. Front. Neurosci. 18:1353142. doi: 10.3389/fnins.2024.1353142
Edited by:
Gerd Kempermann, Helmholtz Association of German Research Centers (HZ), GermanyReviewed by:
Alwyn Dady, Université Paris-Est Créteil Val de Marne, FranceRichard Dorsky, The University of Utah, United States
Copyright © 2024 Sharifi, Farzad, Soldozy, DeWitt, Price, Sheehan, Kalani and Tvrdik. This is an open-access article distributed under the terms of the Creative Commons Attribution License (CC BY). The use, distribution or reproduction in other forums is permitted, provided the original author(s) and the copyright owner(s) are credited and that the original publication in this journal is cited, in accordance with accepted academic practice. No use, distribution or reproduction is permitted which does not comply with these terms.
*Correspondence: M. Yashar S. Kalani, eWFzaGFyLmthbGFuaUBhc2NlbnNpb24ub3Jn; Petr Tvrdik, dHZyZGlrQHZpcmdpbmlhLmVkdQ==