- 1Laboratório de Eletrofisiologia e Metabolismo Cerebral, Instituto de Ciências Biológicas e da Saúde, Universidade Federal de Alagoas, Maceió, Brazil
- 2Faculdade de Medicina, Universidade Federal de Alagoas, Arapiraca, Brazil
Introduction: The expression and localization of the water channel transporters, aquaporins (AQPs), in the brain are substantially modified in gliomas during tumorigenesis, cell migration, edema formation, and resolution. We hypothesized that the molecular changes associated with AQP1 and AQP4 in the brain may potentially be anticancer therapeutic targets. To test this hypothesis, a bioinformatics analysis of publicly available data from international consortia was performed.
Methods: We used RNA-seq as an experimental strategy and identified the number of differential AQP1 and AQP4 transcript expressions in glioma tissue compared to normal brain tissue.
Results: AQPs genes are overexpressed in patients with glioma. Among the glioma subtypes, AQP1 and AQP4 were overexpressed in astrocytoma (low-grade glioma) and classical (high-grade glioma). Overall survival analysis demonstrated that both AQP genes can be used as prognostic factors for patients with low-grade glioma. Additionally, we observed a correlation between the expression of genes involved in the tyrosine and thyroid hormone pathways and AQPs, namely: PNMT, ALDH1A3, AOC2, HGDATP1B1, ADCY5, PLCB4, ITPR1, ATP1A3, LRP2, HDAC1, MED24, MTOR, and ACTB1 (Spearman’s coefficient = geq 0.20 and p-value = ≤ 0.05).
Conclusion: Our findings indicate that the thyroid hormone pathways and AQPs 1 and 4 are potential targets for new anti-tumor drugs and therapeutic biomarkers for malignant gliomas.
1 Introduction
Gliomas are the most common central nervous system tumor type accounting for approximately 80% of all malignant intracranial tumors with high episodes of recurrence (Ostrom et al., 2014).
Depending on the cell type of origin, they can be classified or subdivided into astrocytomas, ependymomas, oligodendrogliomas, oligoastrocytomas, and glioblastoma multiforme (GBM) (Ostrom et al., 2014).
Glioblastoma is the most aggressive brain tumor owing to its high invasiveness. Despite advances in therapeutic modalities (tumor resection combined with chemotherapy and radiotherapy), the overall patient survival rate is 12–15 months from the initial diagnosis, with only 5% of patients reaching a 5-year survival rate (Fedele et al., 2019).
Likewise, temozolomide (TMZ), a DNA alkylating agent, is the standard first-line treatment for GBM, has a significant tumor recurrence rate and confers drug resistance (Strobel et al., 2019) due to its low specificity and limitations in crossing the blood-brain barrier (BBB).
Persistent headaches, neurological and behavioral deficits, seizures, cognitive deficits, drowsiness, dysphagia, confusion, aphasia, motor deficits, fatigue, dyspnea, and mood changes are common symptoms in patients diagnosed with glioblastoma (Maugeri et al., 2016; IJzerman-Korevaar et al., 2018). Usually, its localization, size, and grade make it inoperable and difficult to treat, drastically decreasing the quality of life of patients with glioma (Fedele et al., 2019).
Many symptoms are derived from neurological findings such as increased intracranial pressure and brain herniation. Edema around the tumor causes hypoxia (lack of oxygen supply) (Solar et al., 2022), resulting in long-term disability, psychiatric disorders, substance abuse, or self-harm (Huang et al., 2021).
A clinical study carried out by Wu et al. (2015) showed that within the identification of peritumoral edema during the preoperative period in patients with glioblastoma, factors such as necrosis and edema extent were independent factors for poor outcome and overall survival (OS).
Aquaporins (AQPs), a family of transmembrane water channel proteins, play a critical role in responding to changes in the osmotic environment. Eight members of the water channel family are expressed in the central nervous system (Saadoun et al., 2002; Maugeri et al., 2016). AQP1 and AQP4 are the major water channels in the brain and play a pivotal role in water homeostasis and the maintenance of BBB integrity (Galán-Cobo et al., 2016).
Brain AQPs are distributed primarily in astroglial membranes, especially in perivascular astrocyte foot processes and glia limitans, near the brain fluid compartments (Hubbard et al., 2018; Li et al., 2019) and ependymal cells, providing an efficient system to promote water and solute transport between the perivascular space and glial cells, and to support brain parenchymal clearance through the glymphatic system (Smith et al., 2017; Mestre et al., 2018; Lohela et al., 2022).
AQP4, an orthodox AQP type, is upregulated in gliomas and is involved in the tumorigenesis process, that is, cell migration, invasion, and functional changes in the surrounding tissue. AQP4 distribution has been hypothesized to underlie mechanisms related to the degree of malignancy (Lan et al., 2017), edema formation in the peritumoral region, increased intracranial pressure, and seizure episodes (Dubois et al., 2014; Maugeri et al., 2016).
Tumor cell migration is a complex mechanism not well understood. However, angiogenesis is a critical feature differentiating high-grade and low-grade glioma. Glioblastoma as the most aggressive brain tumor shows morphological and vascular changes characterized by a high invasiveness and migration, which are associated with the expression of AQP1 and AQP4 and mesenchymal transformation as previously described (Hardee and Zagzag, 2012; Brennan et al., 2013).
Aquaporins have been described to have a vital role in enhanced invasion and tumor cell migration as reviewed by De Ieso and Yool (2018) and Moon et al. (2022). Of special importance is the role of AQP1 and -4 in the proposed mechanism of invasiveness and migration of glioma cells. During tumor cells metastasis modifications of blood supply, cytoskeleton and extracellular matrix structure are needed to promote angiogenesis for tissue demands of oxygen and nutrient delivery. AQP1 was found to boost endothelial cell migration and, in association with overexpression of AQP4 to regulate water influx and extracellular matrix interaction leading to tumor cell membrane filopodia formation, mechanisms linked to metastasis progression.
Several in vitro and in vivo studies have reported reduced invasive capacity and migration in tumors after AQP4 depletion or downregulation (Ding et al., 2010, 2011; Cheng et al., 2017) by decreasing water permeability, which in turn upregulates transmembrane water fluxes during tumor or healthy astroglial cell movement (Huang et al., 2021).
In contrast, AQP1 (also considered a classic aquaporin) is predominantly expressed in the circumventricular brain and areas associated with cerebrospinal fluid production. Its expression is altered in the human brain under pathological conditions (Lima et al., 2012) such as cancer. In brain tumors, AQP1 and AQP4 expression is upregulated with the malignancy grade (Saadoun et al., 2002).
Inhibition of tumor growth and suppression of vasculogenic mimicry formation were observed following AQP1 silencing in vivo, which could be related to reduced tumor aggressiveness, malignancy, and metastasis (Perry and Wesseling, 2016).
More recently, the newly updated World Health Organization (WHO) Classification of Tumors of the Central Nervous System classifies brain tumors based on their histological appearance and molecular markers (genotypic classifications). Namely: isocitrate dehydrogenase (IDH) mutation, 1p/19q codeletion, and O-6-methylguanine-DNA methyltransferase (MGMT) methylation, avoiding imprecise diagnosis, thereby significantly influencing in the selection of treatment options for patients (Martinez-Lage and Sahm, 2018; Huang et al., 2020).
Owing to the role of molecular markers in the early diagnosis of gliomas, we hypothesized that AQP1 and AQP4, as homeostatic brain proteins, could act as potential anticancer therapeutic targets.
In this study, we aimed to characterize the expression patterns of genes encoding AQP1 and AQP4 proteins and to identify the genes present in the cancerous microenvironment that potentially regulate or correlate with their expression in human gliomas. Our findings, from a bioinformatic approach, allowed us to conduct a wide and diversified scale analysis and provided evidence for the role of these proteins and other related genes, thus paving the way for the development of potential biomarker candidates for the diagnosis and therapeutic targets of glioma for future research.
2 Materials and methods
2.1 Experimental design
This study was executed using publicly available data from international consortia. In this study, RNA-seq was used as the search strategy. Differential expression analysis was performed using Bioconductor statistical packages with R software (version 4.1). Genomic data on human cancer samples originated from The Cancer Genome Atlas (TCGA) and healthy tissue of the Genotype-Tissue Expression (GTEx) project.
We used web tools to assess the variables beyond differential expression. Correlation analysis was performed using Gene Expression Profiling Interactive Analysis (GEPIA2) (Tang et al., 2019),1 survival analysis, and Tumor IMmune Estimation Resource (TIMER) (Li et al., 2020).2 In addition, we used the Atlas of Human Pathology3 to visualize the distribution pattern of AQP1 and AQP4 proteins in healthy brain and cancer tissues.
The database annotation, visualization, and integrated discovery (DAVID) web server (Sherman et al., 2022)4 was used to identify a network of related genes in altered pathways in the tumor microenvironment (functional enrichment analysis). The same analysis was performed using PathfindR package (Ulgen et al., 2019).
In a short time, the genes involved in the pathways of our choice were evaluated by the degree of correlation with AQP1 and AQP4 expression in glioma tissues, both low-grade glioma (LGG) and GBM, to find similarities in expression profiles and suggest some degree of co-regulation.
Clinical data from patients regarding brain tumor location were not accessed and were not included in this study.
2.2 Transcriptional expression of AQP1 and AQP4
Genetic profiles of normal and solid primary tumor tissues for the two types of cancer were downloaded from TCGA5 using TCGAbiolinks R package version 2.12.6.
A dataset composed of 2,080 RNA-seq of both tumor stages (LGG and GBM, N = 671) and healthy patient samples (N = 1,409) from TCGA and GTEx projects was used. Data were derived from the Illumina HiSeq RNA-Seq platform. The transcript levels of orthodox AQPs (AQP1 and AQP4) in tumor and non-tumor tissues were evaluated. All data were processed and standardized using R software version 4.1.0.
The GEPIA2 web-based tool was used to build graphs to better visualize the results. One-way analysis of variance (ANOVA) was used for the assessment of the disease state: tumor, N = 518 (LGG) and N = 163 (GBM), or normal brain cortex, N = 207, as the variable for calculating the differential expression. The expression data were first transformed using the formula log2 (TPM + 1).
The fold change (log2FC) was defined as the difference in the median value between the normal and tumor samples. Genes with P ≤ 0.05 and | log2fold change (FC)| ≥ 1.0 were considered differentially expressed genes (DEGs). The same analysis was performed for glioma subtypes. All the results are presented in table and box plot forms.
The values were calculated using the corresponding formula:
Where B and A are representative of the median values of the genes expressed in the tumor and median values of the expressed genes in normal tissue, respectively.
2.3 Survival analysis
We sought to assess whether our search goal genes in this study may have survival advantages and, thus, establish possible prognostic markers, confirming their clinical significance.
Patients with glioma were divided into high- or low-level groups based on the median value of genes, and OS rates were evaluated using Kaplan–Meier analysis. The TIMER tool used the log-rank test (Mantel-Cox test) for the hypothesis evaluation. The Cox proportional hazard ratio (HR) and 95% confidence interval (CI) were used as parameters in the Kaplan–Meier survival analysis. Statistical significance was set at P < 0.05.
2.4 Functional enrichment analysis
Kyoto Encyclopedia of Genes and Genomes (KEGG) is an integrated database resource for biological interpretation of genome sequences and other high-throughput data. KEGG analyses are available in the DAVID database (see text footnote 4), a data resource composed of an integrated biology knowledge base and analysis tools to extract meaningful biological information from large quantities of gene and protein collections. Dysregulated pathway identification analysis was performed on differentially expressed genes using the DAVID web tool. The DEG analysis showed 4,820 genes in glioma samples (see Supplementary material) that were divided into two groups of lists: upregulated and downregulated genes (logFC > 1 and logFC < 0). Subsequently, these lists were submitted separately to DAVID and the results were generated.
2.5 Correlation analysis using the GEPIA2 tool
A pairwise gene Spearman correlation analysis between AQP1 or AQP4 and the expression of other preselected genes was performed using GEPIA2.
GEPIA data are presented as a log-scale axis and a non-log scale was used for calculation; the strength of the correlation was determined using the following guide for the absolute value: ≤0.2 (weak), 0.21–0.50 (moderate), 0.51–0.80 (strong), and 0.81–1.0 (very strong). Spearman’s coefficients >0 indicate a positive correlation, and coefficients less than zero indicates a negative correlation. Spearman’s coefficient ≥0.20 and p-values ≤0.05 were considered significant in the current study.
3 Results
3.1 AQP1 and AQP4 genes are overexpressed in gliomas compared to normal brain samples
To characterize gene expression, differential expression analysis from TCGA and GTEx datasets was performed using the R software. This analysis revealed a list of 4,819 genes. Among these, five AQPs family members were identified: AQP1, AQP4, AQP5, AQP7, and AQP8.
As expected, both AQP1 and AQP4 mRNAs were overexpressed in glioma samples (logFC = 1.42 x 10+14 and 1.02 x 10+14; P-value = 1.66E-40 and 8.82E-26, respectively) compared with the healthy brain samples (Table 1 and Figures 1A, B, 2).
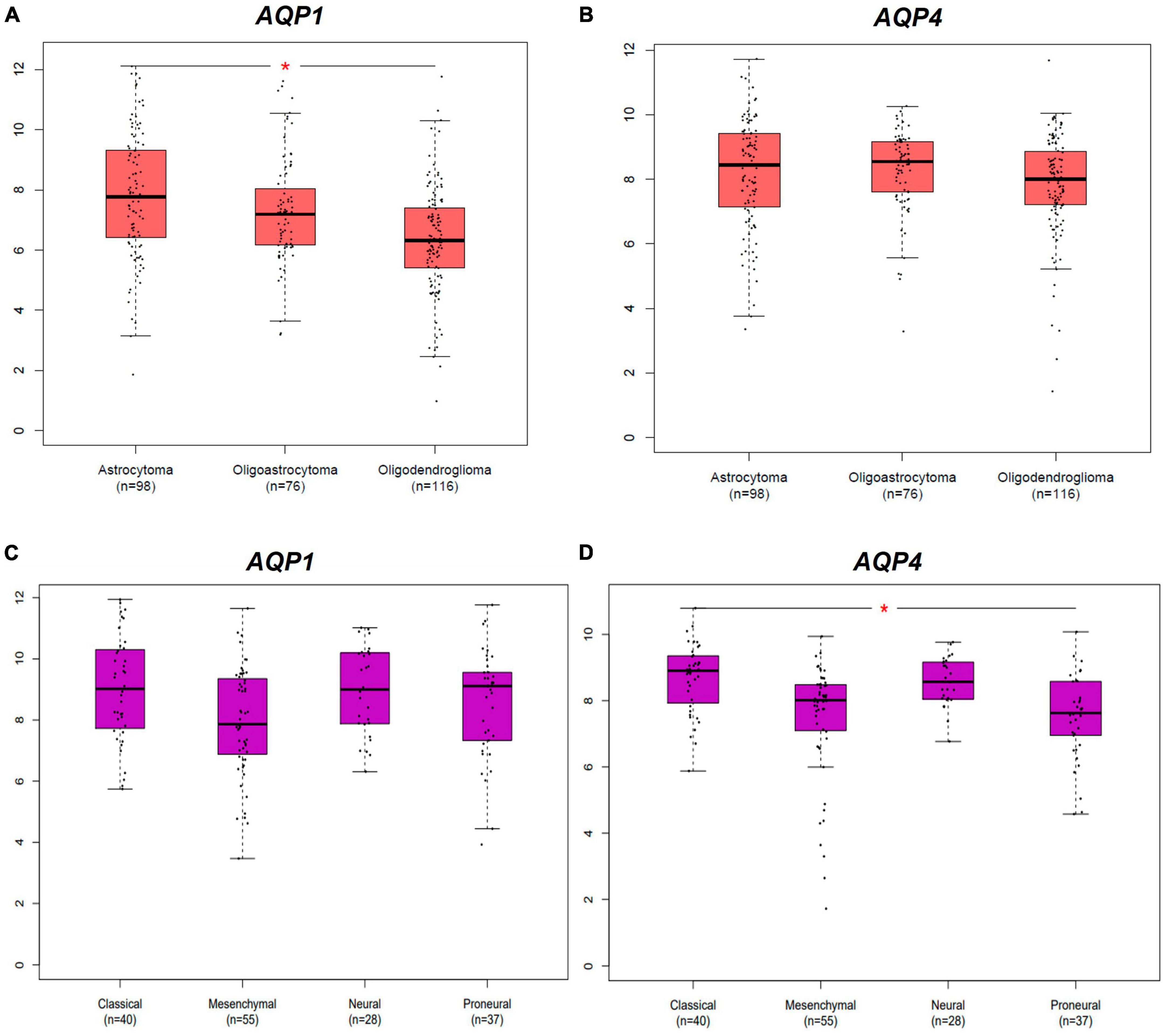
Figure 1. Differential expression AQPs mRNAs from gliomas samples from TCGA and GTEx dataset. AQP1 and AQP4 genes. Tumor samples are divided into LGG and GBM subtypes groups. (A,B) Represents both AQP1 and AQP4 profiles of expression in LGG subtypes. (C,D) Represents both AQP1 and AQP4 profiles of expression in GBM subtypes. LGG, low-grade glioma; GBM: glioblastoma multiforme. *p < 0.05. All graphs were derived by the GEPIA2 webserver in January 2021.
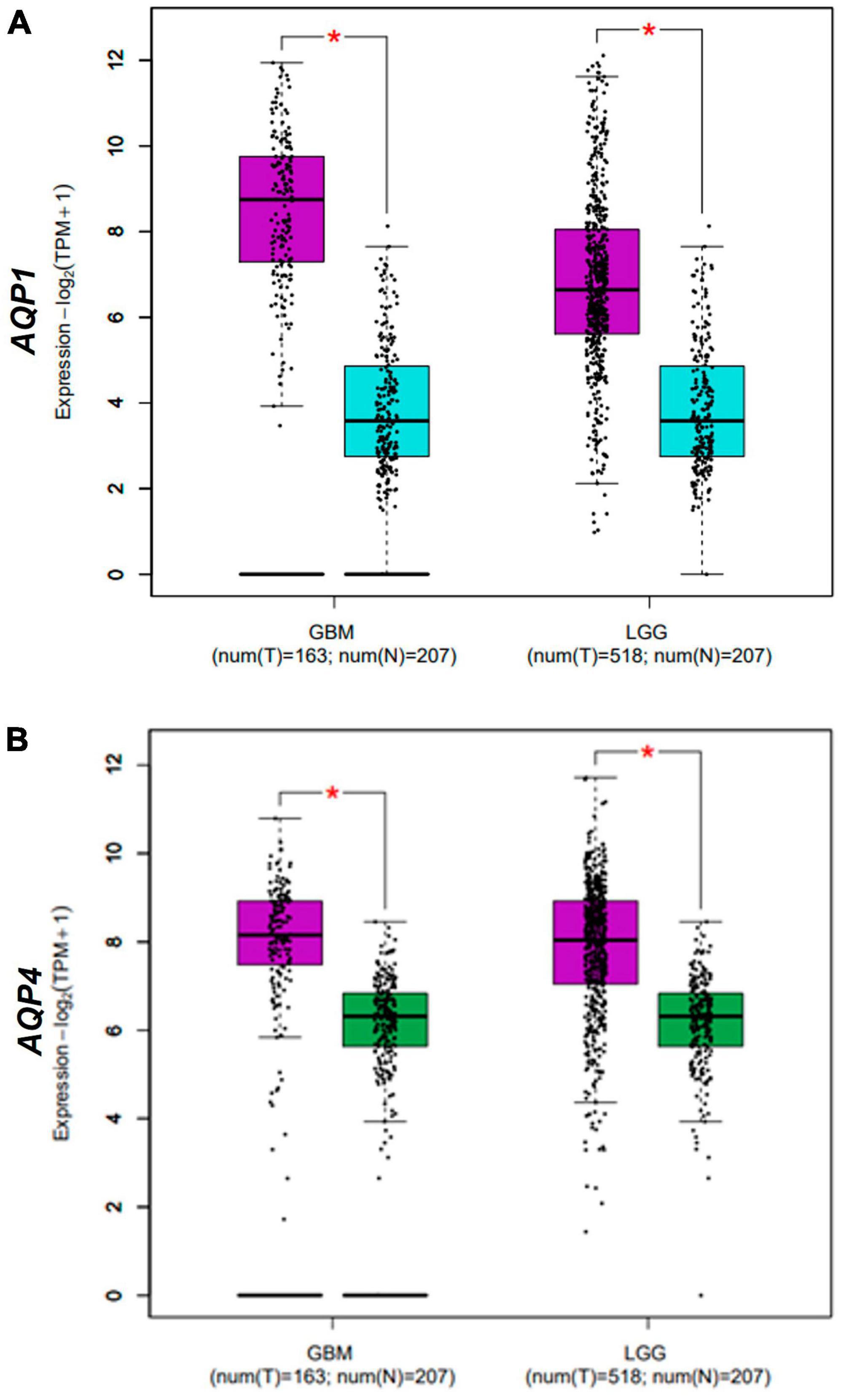
Figure 2. Differential expression AQPs mRNAs from gliomas samples from TCGA and GTEx dataset. AQP1 (A) and AQP4 (B) genes in normal (N = 163; blue box for AQP1 data and green box for AQP4 data) and tumor (N = 207; purple box) samples. Tumor samples are divided into LGG and GBM groups (X-axis of both graphs). TPM, transcripts per kilobase million; LGG, low-grade glioma; GBM, glioblastoma multiforme. *p < 0.05. Graphs (A,B) were derived by the GEPIA2 webserver in January 2021.
Histological samples of tumor and normal brain tissues from the Human Protein Atlas database show the distribution of AQP1 and AQP4 proteins. The staining intensity of both proteins increased with the degree of tumor malignancy. Figures 3A–C show AQP1 staining, while Figures 3D–F show AQP4 staining.
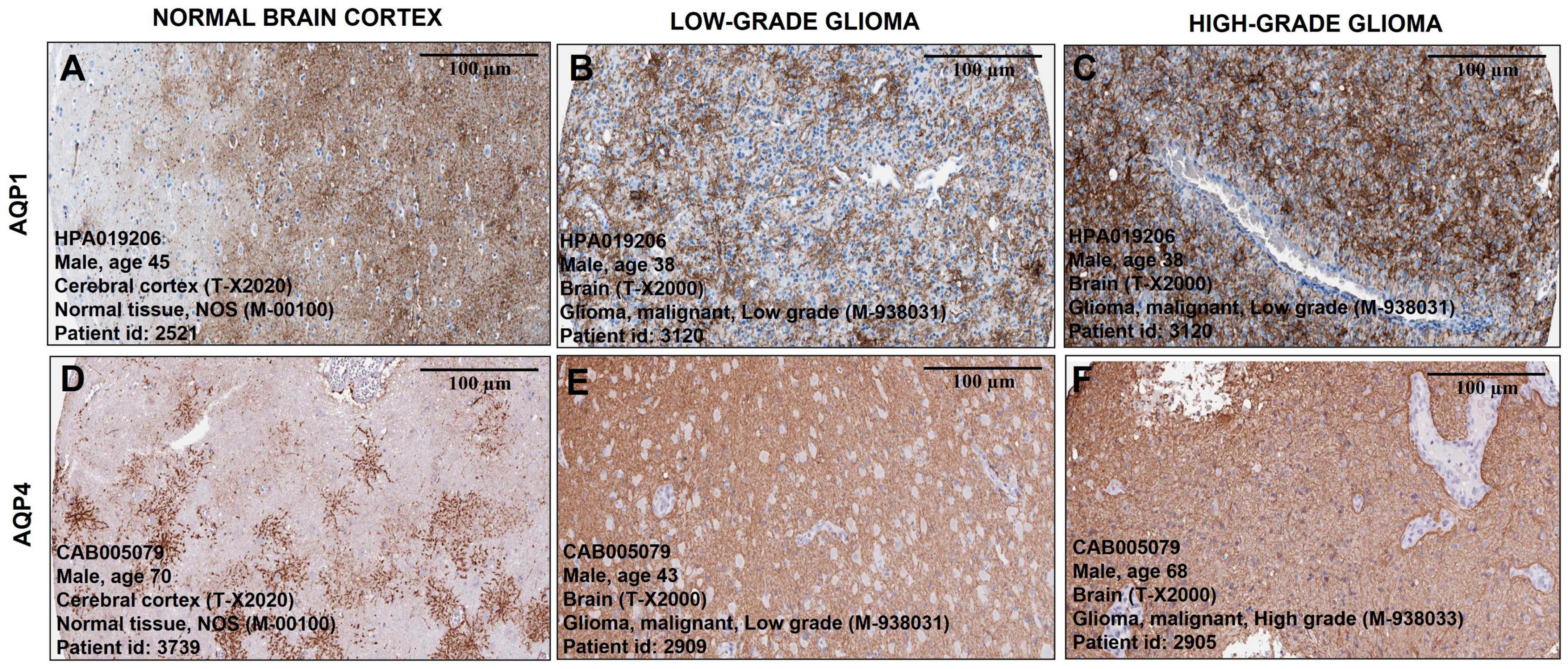
Figure 3. Representative immunohistochemical staining distribution of AQP1 and AQP4 expressions in normal and glioma patient samples. Plates (A–F) are captured in 100 μm. It is important to highlight that the staining (brownish) of the antibody for both proteins becomes more pronounced and denser as the degree of tumor malignancy increases compared to representative images of healthy brain tissue (A,D). All data were extracted from the Human Protein Atlas (https://www.proteinatlas.org/).
In addition, both AQP1 and AQP4 showed a significant difference in expression pattern upon comparison with tumor markers/antigens in gliomas that are consensually used in clinical practice to establish degrees of malignancy and prognosis (Soldatelli et al., 2022). For instance: TP53, ATRX, TERT, IDH1, EGFR, and GFAP (Figure 4).
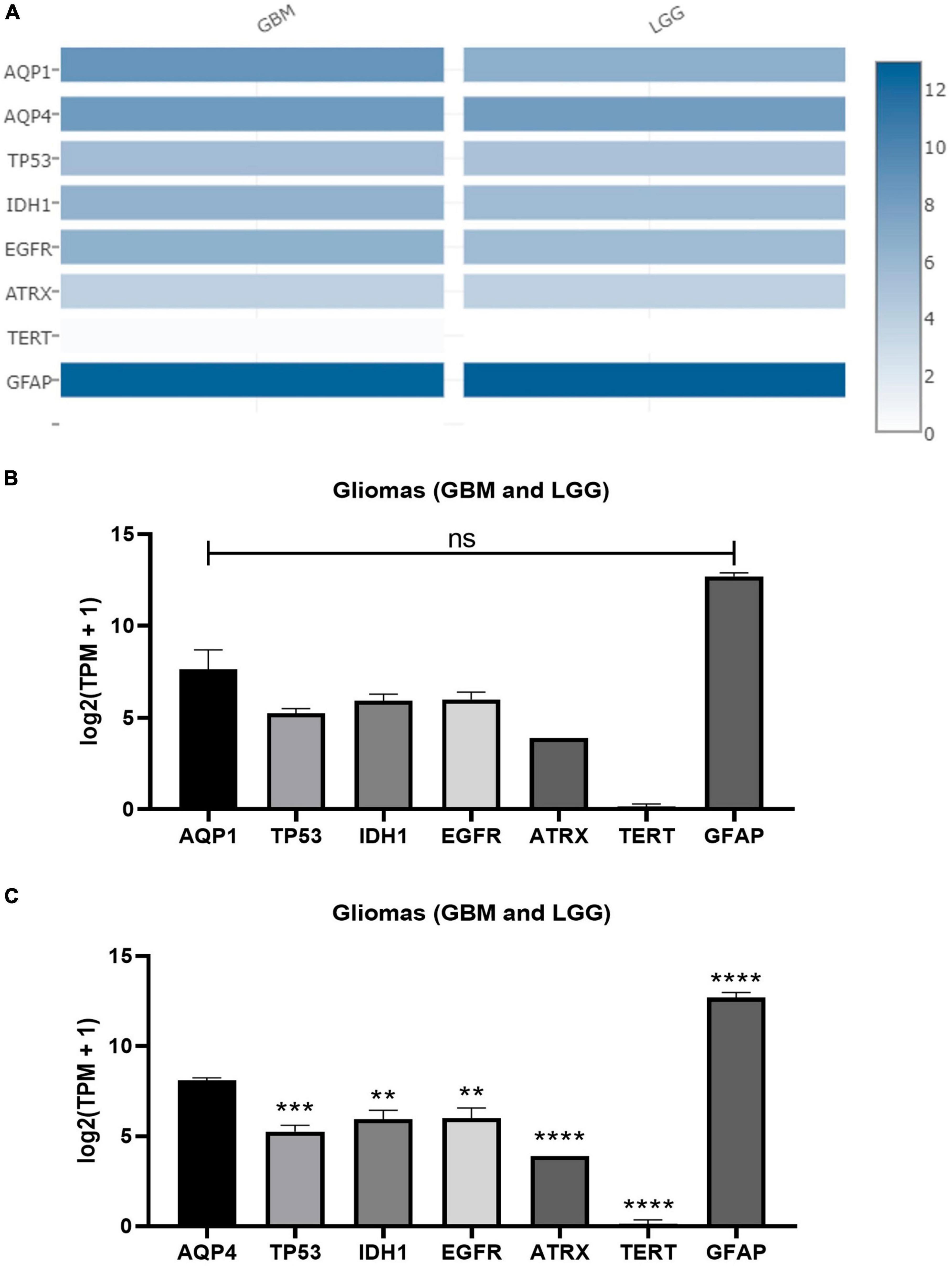
Figure 4. Expression matrix graphs based on a list of genes considered biomarkers of glioma and AQP1 and AQP4. (A) Heat Map derived from GEPIA2. The color density in each block represents the median value of gene expression in tumor tissue (GBM and LGG, separately) normalized by the maximum median value of the expression in all blocks. These data were transformed for plotting [linear to log2(TPM + 1)]. Graphs (B,C) were created from the values provided by the heap map for each gene with a subsequent comparison with both AQP1 and AQP4, respectively. For this, one-way ANOVA was performed followed by Tukey’s multiple comparisons tests using GraphPad Prism version 8.0. **p < 0.01, ***p < 0.001, ****p < 0.0001. TP53, tumor protein P53; IDH1, isocitrate dehydrogenase [NADP(+)] 1; EGFR, epidermal growth factor receptor; ATRX, ATRX chromatin remodeler; TERT, telomerase reverse transcriptase; TPM, transcripts per kilobase million; GFAP, glial fibrillary acidic protein.
These results revealed a pivotal role of the above-mentioned genes in the gliomagenesis process.
3.2 AQP1 and AQP4 seem to play substantial roles in the molecular glioma subtype
In a complementary manner, Figure 2 shows the differential expression of AQP1 and AQP4 in glioma subtypes. A and B represent the expression patterns in the LGG subtypes (astrocytoma, oligoastrocytoma, and oligodendroglioma). In this case, AQP1 showed an increased number of transcripts in astrocytomas compared than in oligodendroglioma tumors (*p ≤ 0.05) (Figure 2A). AQP4 mRNAs levels were not significant in these LGG samples (Figure 2B).
Regarding the GBM subtypes (classical, mesenchymal, neural, and proneural), only AQP4 presented considerable variation in its expression between classical and proneural subtypes, being more expressed in the classical case (*p ≤ 0.05) (Figure 2D). AQP1 mRNAs levels were not significant in these GBM samples (Figure 2C).
These results indicate that the aquaporins in question most likely have an oncogenic role only in cells of astrocytic origin.
3.3 AQP1 and AQP4 could be potential risk factors and prognostic indicators for patients with low-grade glioma
One of the factors in assigning clinical importance to a therapeutic target is the probability of increasing the chances of patient survival. Kaplan–Meier analysis revealed that OS was lower in patients with LGG who had high AQP1 (HR = 1.458; log-rank = 0) and AQP4 (HR:1.191; log-rank = 0.003) expression (Figures 5B, D). In the GBM patient groups, there was no difference between the high and low expression groups of these selected genes (Figures 5A, C).
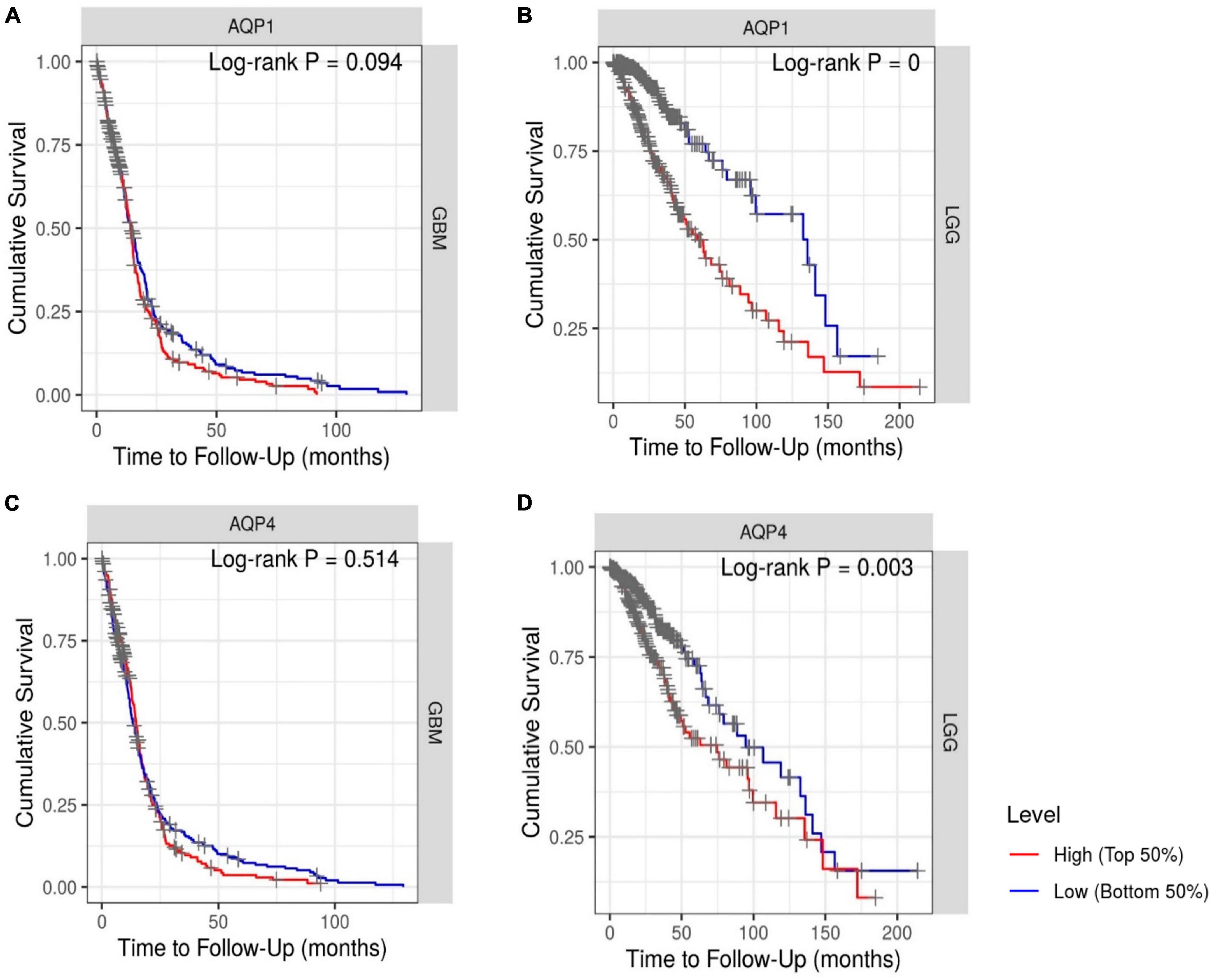
Figure 5. Kaplan–Meier survival analysis. (A,C) Patients with GBM and (B,D) patients with LGG. The Cox proportional hazard ratio (HR) and the 95% confidence interval were used in the Kaplan–Meier survival analysis. Red and blue curves represent high and low expression profiles, respectively. GBM, N = 523 patients with 448 dying. LGG, N = 514 patients with 125 dying. Log-rank P-values ≤0.05 were considered statistically significant. All graphs were derived by the TIMER database in September 2021.
Our findings suggest that high expression of AQP1 and AQP4 may be a risk factor for poor prognosis in patients with LGG because they are significantly associated with reduced survival.
3.4 Functional enrichment analysis
Understanding the disease functioning from different levels of information is a crucial step in this line of work, as we look for potential regulators of AQPs only in gliomas.
The DAVID tool analysis generated two lists of dysregulated pathways from upregulated and downregulated genes in the glioma microenvironment. A list of genes with LogFC ≥ 1.0 revealed 64 altered pathways (Supplementary Table 1). Among them, 31 presented statistical significance (p < 0.05). Sixty-six pathways were identified in genes with negative LogFC values (Table 2). Among them, 30 were statistically significant.
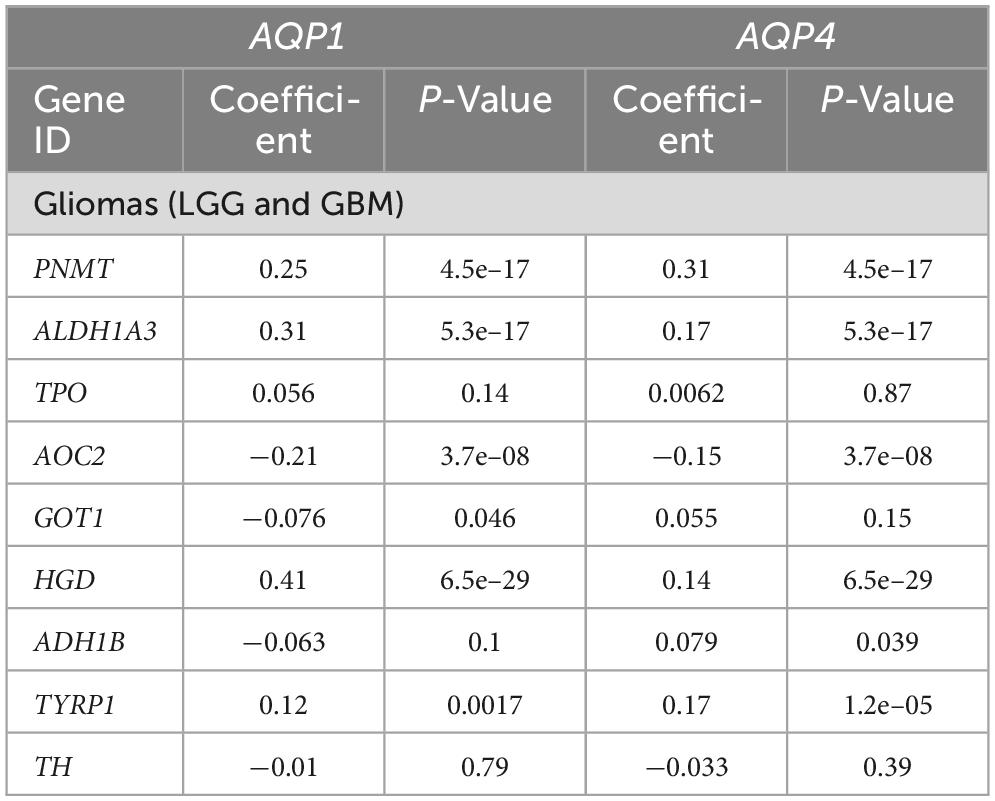
Table 2. Correlation analysis between genes involved in the tyrosine metabolism pathway and AQP’s genes.
PathfindR analysis showed 113 pathways over-represented according to glioma differential expression (Supplementary Table 3).
Based on our previous study (Costa et al., 2019), where AQP4 was overexpressed both during central nervous system development and in human glioma cells and triiodothyronine (T3) played a negative role in this expression pattern, we chose tree-specific pathways in the downregulated list of the functional enrichment analysis: hsa00350 (Tyrosine metabolism [9 genes]); hsa04918 (Thyroid hormone synthesis [15 genes]); and hsa04919 (Thyroid hormone signaling pathway [7 genes]) (Supplementary Table 3). It is worth noting that choosing the genes from downregulated pathways was timely to antagonize the overexpression of AQPs as well as in our experimental previous study (in vivo and in vitro). In total, we identified 31 genes from the cellular pathways identified in the enrichment analysis.
3.5 Spearman’s correlation
We hypothesized that the expression of both AQP1 and AQP4 could be modulated by genes involved in thyroid function. Although still controversial, accumulated evidence suggests that thyroid function plays an important role in several pathological and non-pathological processes of the central nervous system, including gliomagenesis, cell migration and cerebral edema resolution (Schiera et al., 2021) having AQP4 as one of its action targets, as shown in two experimental circumstances, mentioned above, evidenced by our research group (Costa et al., 2019). Tyrosine, in turn, is the precursor amino acid of thyroid hormones by the iodination of tyrosine residues in thyroglobulin and has their levels altered in some disease status (Khaliq et al., 2015). Here, we attempted to identify potential regulators that could interfere with some degree of regulation of the disposition and function of AQP1 and AQP4 in tumor cells (Tables 2–4).
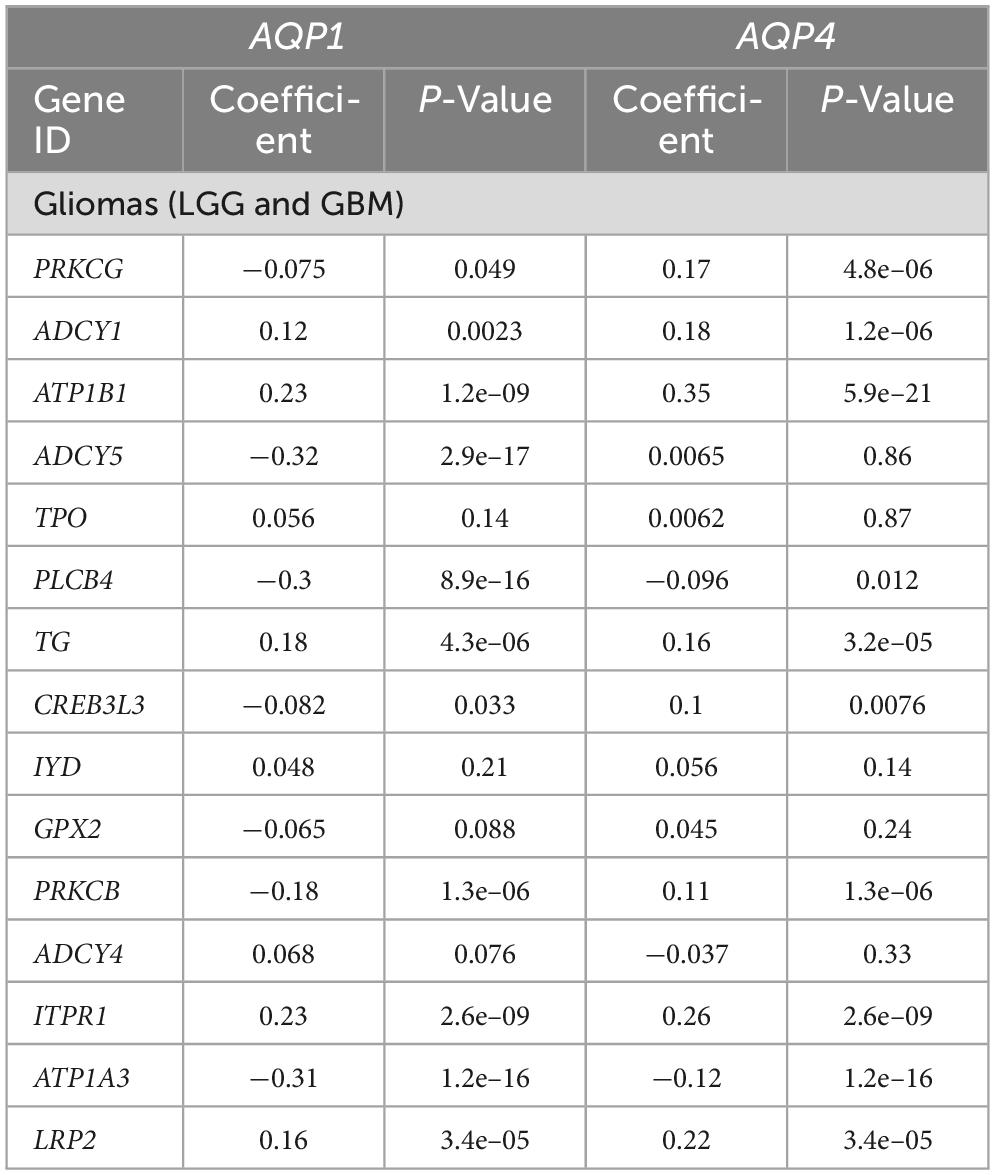
Table 3. Correlation analysis between genes involved in the thyroid hormones synthesis pathway and AQP’s genes.
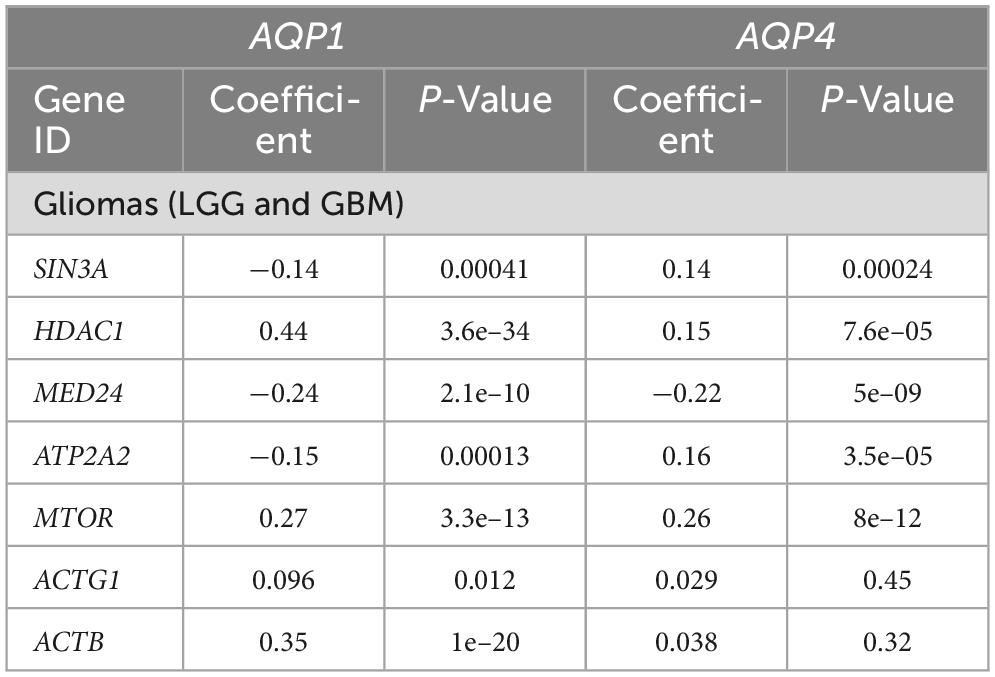
Table 4. Correlation analysis between genes involved in the thyroid hormones signaling pathway and AQP’s genes.
Within the tyrosine metabolism pathway, five genes were correlated with AQP1 and AQP4, although only four met the statistical requirements already established: PNMT, ALDH1A3, AOC2, and HGD (Table 2).
In the thyroid hormone synthesis pathway, six genes were statistically significant (Table 3): ATP1B1, ADCY5, PLCB4, ITPR1, ATP1A3, and LRP2. Only four genes involved in the thyroid hormone signaling pathway (Table 4) were within the statistical inclusion criteria described in Subsection 2.5: HDAC1, MED24, MTOR, and ACTB1. All these genes are also listed and have potential actions on AQPs, as summarized in Table 5. Our data refer to the number of transcripts using Spearman’s correlation analysis (AQP1 and AQP4 vs. genes selected from the functional enrichment analysis). Thus, we can consider them regulators at the translational level.
4 Discussion
In this study, we aimed to identify the expression pattern of AQP1 and AQP4 genes in human gliomas, as well as to highlight their regulatory potential within the cancer microenvironment.
We used RNA-seq as a search strategy to identify the differential expression of AQP1 and AQP4 transcripts in glioma tissues data compared to normal brain tissues data. Indeed, AQPs genes are overexpressed in patients with glioma. Among the glioma subtypes, AQP1 and AQP4 are overexpressed in astrocytoma (LGG) and classical glioma (GBM). OS analysis showed that both AQP genes can be used as prognostic factors for patients with LGG, confirming the results of previous studies and reinforcing their clinical value. We also observed a correlation between the expression of genes involved in the tyrosine and thyroid hormone pathways and AQPs.
Emerging evidence suggests a positive relationship between AQP1 and AQP4 expression and histological tumor grade and brain edema volume. Poor neurological prognosis has also been reported (Suzuki et al., 2018; Chow et al., 2020). Our results are supported by these findings, as aquaporin expression patterns increased when compared between molecular subtypes of gliomas: AQP1 is increased in other subtypes of LGG classification (astrocytoma); in contrast, AQP4 is increased in only the classical subtype GBM.
Some current studies have highlighted the regulatory function of AQPs on brain volume in many other neurological diseases besides tumors (Sun et al., 2022; Ohmura et al., 2023). In gliomas, both AQP1 and AQP4, when suppressed, offer a better prognosis for patients affected by these types of tumors by edema reduction (Faraj et al., 2022; Ohmura et al., 2023), facilitating the management of neurological deficits in the pre-and postoperative periods, with peritumoral edema being more relevant, in terms of concerns the aggressiveness of this cancer, rather than its extent, per se (Faraj et al., 2022). AQP1, for example, is upregulated in the peritumoral area, especially in the vessels surrounding reactive astrocytes in high-grade gliomas. In LGG, it showed an intense pattern of intratumoral expression (Zoccarato et al., 2021). The discovery of the glymphatic system was also important for the consolidation of AQPs as targets therapeutic in brain edema regulation (Ding et al., 2023).
From these findings it is possible to suggest that AQPs are perhaps more relevant in the tumor progression process, that is, the transformation of LGG into GBM through the formation of expansive edema, facilitating the invasion of tumor cells into healthy tissue. The determining factors in the survival rate of patients with GBM, in turn, are other and more diversified, in addition to the expression pattern of AQPs (Deb et al., 2012; Saito et al., 2022), which would explain our results from the survival analysis, where AQP genes only functioned as a risk factor for survival in low-grade gliomas.
AQP4 isoforms, known as M1 and M23, have been shown to change the aggregation/disaggregation state into orthogonal arrangements of particles (OAPs) and glioma cell survival. One study revealed that the isoform M23 reduced the invasion and proliferation of glioma cells to promote their apoptosis (Simone et al., 2019).
Some endogenous factors such as hormonal and metabolic changes also modulate AQP4 expression in human gliomas (Lan et al., 2017). Likewise, an increase in AQP1 expression was observed during central necrosis and hypoxia, which are characteristics of GBM tumors (Hayashi et al., 2007; Honasoge and Sontheimer, 2013).
Gene expression is orchestrated by several endogenous and exogenous factors, such as DNA-binding transcription factors, regulatory proteins, and profile hormones (Bhat et al., 2021).
A study conducted by Nauman et al. (2004) evaluated the cellular concentrations of thyroxine (T4) and triiodothyronine (T3) in non-thyroidal illness syndrome (NTIS) patients with gliomas, where the thyroid stimulating hormone (TSH) serum concentration was within the normal range and T4/T3 levels were lower than normal (Nauman et al., 2004). They demonstrated that thyroid hormone levels were significantly lower in glioma tissues than in healthy tissues. In addition, both iodothyronine deiodinases (types 2 and 3) were higher in tumor tissues than in non-glioma tissues.
Some studies have shown that hypothyroidism is an important factor in the prognosis and progression of metastatic cancer in patients with brain cancer. For example, a cohort study (Berghoff et al., 2020) identified a beneficial role of this clinical condition in overall survival in patients with brain metastases, almost doubling life expectancy from the diagnosis of primary and metastatic cancer. Furthermore, the results of a clinical survey showed that almost 40% of patients with brain tumors evaluated had overt or subclinical hypothyroidism and 31% required hormone replacement (Faghih-Jouybari et al., 2018).
In pediatric patients, an important probability of developing thyroid dysfunction was found post-treatment with surgery, chemotherapy, and radiotherapy (Jin et al., 2018; Cosnarovici et al., 2020); moreover, abnormalities in thyroid function were found in patients with AQP4 antibody–seropositive optic neuritis (Zhao et al., 2017).
We previously identified a negative modulator of AQP4 protein and demonstrated that triiodothyronine (T3) treatment of GBM-95-line cells resulted in a slight reduction in cell migration (Costa et al., 2019); other studies have also evaluated the modulatory action of this hormone on the expression of AQP4 in stroke animal models (Sadana et al., 2015).
In recent years, a specific AQP4 inhibitor has been tested in rodents and human brain glioma to directly measure water efflux rate during tumorigeneses (Harrison et al., 2020; Rosu et al., 2020). A promising study conducted by Jia et al. (2023) found a correlation with water efflux in glioma cell cultures, rat and human glioma and cell proliferation as measured by magnetic resonance imaging. This discovery is in agreement with our results showing overexpression of AQP4 genes in gliomas, placing aquaporin 4 as an important biomarker to be used both in bioinformatics analyzes and in brain imaging as a potential therapeutic treatment of gliomas. A summary of what is hypothesized/proven about the modulation of the expression of AQPs 1 and 4 in gliomas is briefly outlined in Figure 6.
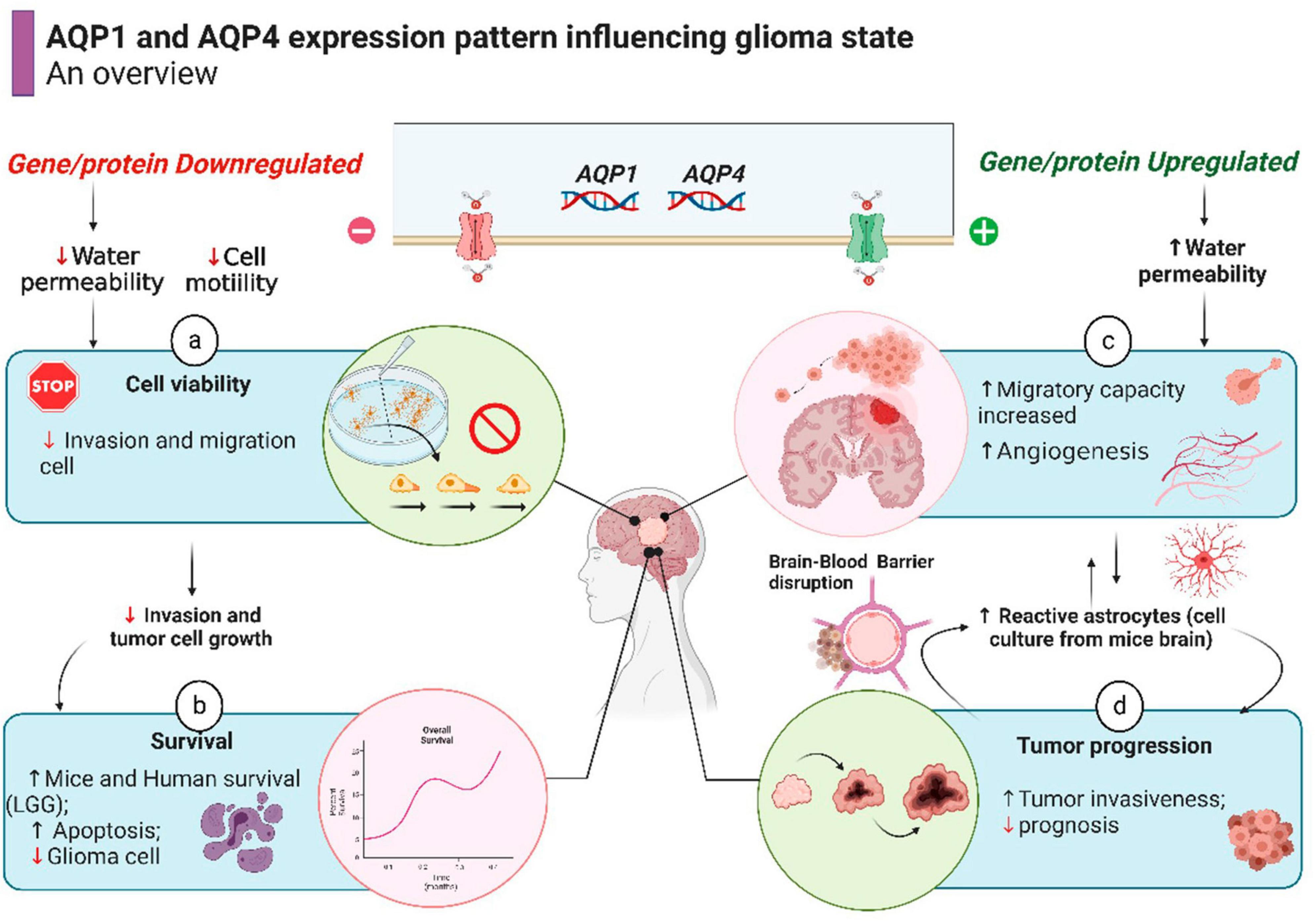
Figure 6. Schematic representation of cell processes involving AQPs activity in glioma state (already demonstrated by prior studies), resulting in better or worse outcome for a patient. The overall survival in human patient with LGG is shown in Figure 5. Source: Subscription: Individual, Agreement number: XZ26G6XU1D, Journal name: Frontiers in Neuroscience.
Therefore, according to our results, we suggest two new hypotheses: (1) As all patient data are post-mortem (including data other than this one), it is possible that standard cancer treatment led to hypothyroidism and subsequently downregulated the thyroid hormone pathways. This could result in the overexpression of AQP1 and AQP4 in the more aggressive glioma subtypes [astrocytoma (LGG) and classical, (GBM)], as we present in the differential expression results, (2) hypothyroidism would already be a clinical risk condition for the development of glioma; patients diagnosed early with the tumor would present a sub-clinical pattern of thyroid gland dysfunction. This condition would be accentuated by the anticancer therapeutic approach, and these patients would have the same outcome and genetic, and molecular profiles as mentioned in hypothesis 1.
Additional studies to clarify the specific inhibitors or promoters of these pathways, as well as transcription factors and post-transcription/translation modification methods, will help to identify the most appropriate therapeutic targets for AQP1 and AQP4 modulation, relationships between genes, and signaling pathways in gliomas.
To the best of our knowledge, this is the first study to suggest a modulatory interaction between the gene encoding AQP1 and pathways related to thyroid hormones.
5 Limitations
The brain tissue is substantially plastic and heterogeneous. Factors such as age and sex can influence their genetic constitution. Glial tumor characteristics may also change depending on the topographic region of the brain where the tumor occurs. The non-separation of categorical patient groups based on the aforementioned factors is a limitation of this study. Single-cell analysis from different glioma patient groups would help us to confirm the oncogenic role of the AQPs studied here, as well as their modulation by thyroid hormones.
6 Conclusion
To the best of our knowledge this study is the first to show a strong potential functional relationship between AQP1 and thyroid hormone pathways in brain tumors. The expression of AQP1 and AQP4 is significantly associated with a worse prognosis in patients with LGG. The molecular pathways and AQP1 and AQP4 genes identified here may be useful for the molecular diagnosis of gliomas and for screening new anti-tumor drugs for these malignant tumors.
Data availability statement
The datasets presented in this study can be found in online repositories. The names of the repository/repositories and accession number(s) can be found in the article/Supplementary material.
Author contributions
CM: Conceptualization, Formal Analysis, Investigation, Writing – original draft, Writing – review and editing. LR: Writing – review and editing. CC: Data curation, Writing – original draft. AX: Conceptualization, Data curation, Project administration, Supervision, Writing – original draft, Writing – review and editing.
Funding
The author(s) declare financial support was received for the research, authorship, and/or publication of this article. CM was recipient of a Master fellowship from Brazilian Federal Agency for Support and Evaluation of Graduate Education (Capes).
Acknowledgments
To the Coordination for the Improvement of Higher Education Personnel (CAPES) for granting a master’s scholarship (CBM). The results shown here are in part based on data generated by TCGA Research Network: https://www.cancer.gov/tcga. The Genotype-Tissue Expression (GTEx) Project was supported by the Common Fund of the Office of the Director of the National Institutes of Health and by NCI, NHGRI, NHLBI, NIDA, NIMH, and NINDS. The data used for the analyses described in this manuscript were obtained from the GTEx Portal on 08/12/2022.
Conflict of interest
The authors declare that the research was conducted in the absence of any commercial or financial relationships that could be construed as a potential conflict of interest.
Publisher’s note
All claims expressed in this article are solely those of the authors and do not necessarily represent those of their affiliated organizations, or those of the publisher, the editors and the reviewers. Any product that may be evaluated in this article, or claim that may be made by its manufacturer, is not guaranteed or endorsed by the publisher.
Supplementary material
The Supplementary Material for this article can be found online at: https://www.frontiersin.org/articles/10.3389/fnins.2024.1349421/full#supplementary-material
Abbreviations
ANOVA, analysis of variance; AQP, aquaporin; BBB, blood-brain barrier; CI, confidence interval; CNS, central nervous system; CSF, cerebrospinal fluid; DEG, differentially expressed gene; GBM, glioblastoma; GTEx, the genotype-tissue expression; GEPIA, gene expression profiling interactive analysis; HR, hazard ratio; IDH, isocitrate dehydrogenase; KEGG, Kyoto Encyclopedia of Genes and Genomes; LGG, low-grade glioma; MGMT, O-6-methylguanine-DNA methyltransferase; NCBI, national center for biotechnology information; NTIS, Non-thyroidal illness syndrome; OS, overall survival; RNA-seq, Ribonucleic Acid sequencing; TCGA, the cancer genome atlas; TMZ, temozolomide; TIMER, tumor IMmune estimation resource; TPM, transcript per million; TSH, thyroid-stimulating hormone; WHO, World Health Organization.
Footnotes
- ^ http://gepia2.cancer-pku.cn
- ^ http://timer.cistrome.org/
- ^ https://www.proteinatlas.org/
- ^ https://david.ncifcrf.gov/
- ^ https://portal.gdc.cancer.gov/
References
Berghoff, A., Wippel, C., Starzer, A., Ballarini, N., Wolpert, F., and Bergen, E. (2020). Hypothyroidism correlates with favourable survival prognosis in patients with brain metastatic cancer. Eur. J. Cancer 135, 150–158. doi: 10.1016/j.ejca.2020.05.011
Bhat, P., Honson, D., and Guttman, M. (2021). Nuclear compartmentalization as a mechanism of quantitative control of gene expression. Nat. Rev. Mol. Cell Biol. 22, 653–670.
Brennan, C., Verhaak, R., McKenna, A., Campos, B., Noushmehr, H., Salama, S., et al. (2013). The somatic genomic landscape of glioblastoma. Cell 155, 462–477.
Cheng, Y., Gao, F., Jiang, R., Lui, H., Hou, J., Yi, Y., et al. (2017). Down-regulation of AQP4 expression via p38 MAPK signaling in temozolomide-induced glioma cells growth inhibition and invasion impairment. J. Cell Biochem. 118, 4905–4913.
Chow, P., Bowen, J., and Yool, A. (2020). Combined systematic review and transcriptomic analyses of mammalian aquaporin classes 1 to 10 as biomarkers and prognostic indicators in diverse cancers. Cancers 12:1911.
Cosnarovici, M., Piciu, A., Bonci, E., Bãdan, M., Bãdulescu, C., and Stefan, A. (2020). Post-treatment thyroid diseases in children with brain tumors: a single-center experience at “Prof. Dr. Ion Chiricuţă” institute of oncology, Cluj-Napoca. Diagnostics 10:142.
Costa, L., Clementino-Neto, J., Mendes, C., Franzon, N., Costa, E., Moura-Neto, V., et al. (2019). Evidence of aquaporin 4 regulation by thyroid hormone during mouse brain development and in cultured human glioblastoma multiforme cells. Front. Neurosci. 13:137. doi: 10.3389/fnins.2019.00317
De Ieso, M., and Yool, A. (2018). Mechanisms of aquaporin-facilitated cancer invasion and metastasis. Front. Chem. 6:135. doi: 10.3389/fchem.2018.00135
Deb, P., Pal, S., Dutta, V., Boruah, D., Chandran, V. M., and Bhatoe, H. S. (2012). Correlation of expression pattern of aquaporin-1 in primary central nervous system tumors with tumor type, grade, proliferation, microvessel density, contrast-enhancement and perilesional edema. J. Cancer Res. Therapeutics 8, 571–577.
Ding, T., Gu, F., Fu, L., and Ma, Y. (2010). Aquaporin-4 in glioma invasion and an analysis of molecular mechanisms. J. Clin. Neurosci. 17, 1359–1361.
Ding, T., Ma, Y., Li, W., Liu, X., Ying, G., Fu, L., et al. (2011). Role of aquaporin-4 in the regulation of migration and invasion of human glioma cells. Int. J. Oncol. 38, 1521–1531.
Ding, Z., Fan, X., Zhang, Y., Yao, M., Wang, G., Dong, Y., et al. (2023). The glymphatic system: a new perspective on brain diseases. Front. Aging Neurosci. 15:1179988. doi: 10.3389/fnagi.2023.1179988
Dubois, L., Campanati, L., Righy, C., D’Andrea-Meira, I., Spohr, T., Porto-Carreiro, I., et al. (2014). Gliomas and the vascular fragility of the blood brain barrier. Front. Cell Neurosci. 8:418. doi: 10.3389/fncel.2014.00418
Faghih-Jouybari, M., Naderi, S., Mashayekhi, S., Padeganeh, T., and Abdollahzade, S. (2018). Hypothyroidism among patients with glioblastoma multiforme. Iran J. Neurol. 17, 149–151.
Faraj, C. A., Snyder, R. I., and McCutcheon, I. E. (2022). Intracranial emergencies in neurosurgical oncology: pathophysiology and clinical management. Emerg. Cancer Care 1:13.
Fedele, M., Cerchia, L., Pegoraro, S., Sgarra, R., and Manfioletti, G. (2019). Proneural-mesenchymal transition: phenotypic plasticity to acquire multitherapy resistance in glioblastoma. Int. J. Mol. Sci. 20:2746.
Galán-Cobo, A., Ramírez-Lorca, R., and Echevarría, M. (2016). Role of aquaporins in cell proliferation: what else beyond water permeability? Channels 10, 185–201.
Hardee, M., and Zagzag, D. (2012). Mechanisms of glioma-associated neovascularization. Am. J. Pathol. 181, 1126–1141.
Harrison, I., Ismail, O., Machhada, A., Colgan, N., Ohene, Y., Nahavandi, P., et al. (2020). Impaired glymphatic function and clearance of tau in an Alzheimer’s disease model. Brain 143, 2576–2593.
Hayashi, Y., Edwards, N., Proescholdt, M., Oldfield, E., and Merrill, M. (2007). Regulation and function of aquaporin-1 in glioma cells. Neoplasia 9, 777–787.
Honasoge, A., and Sontheimer, H. (2013). Involvement of tumor acidification in brain cancer pathophysiology. Front Physiol. 4:316. doi: 10.3389/fphys.2013.00316
Huang, S., Jiang, H., Hu, H., and Lv, D. (2021). Targeting AQP4 localization as a novel therapeutic target in CNS edema. Acta Biochim. Biophys. Sin. 53, 269–272.
Huang, Z., Wong, L., Su, Y., Huang, X., Wang, N., Chen, H., et al. (2020). Blood-brain barrier integrity in the pathogenesis of Alzheimer’s disease. Front. Neuroendocrinol. 59:100857. doi: 10.1016/j.yfrne.2020.100857
Hubbard, J., Szu, J., and Binder, D. (2018). The role of aquaporin-4 in synaptic plasticity, memory and disease. Brain Res. Bull. 136, 118–129.
IJzerman-Korevaar, M., Snijders, T., de Graeff, A., Teunissen, S., and de Vos, F. (2018). Prevalence of symptoms in glioma patients throughout the disease trajectory: a systematic review. J. Neurooncol. 140, 485–496.
Jia, Y., Xu, S., Han, G., Wang, B., Wang, Z., and Lan, C. (2023). Transmembrane water-efflux rate measured by magnetic resonance imaging as a biomarker of the expression of aquaporin-4 in gliomas. Nat. Biomed. Eng. 7, 236–252.
Jin, S., Choi, J., Park, K., Kang, H., Shin, H., and Phi, J. (2018). Thyroid dysfunction in patients with childhood-onset medulloblastoma or primitive neuroectodermal tumor. Ann. Pediatr. Endocrinol. Metab. 23, 88–93.
Khaliq, W., Andreis, D., Kleyman, A., Gräler, M., and Singer, M. (2015). Reductions in tyrosine levels are associated with thyroid hormone and catecholamine disturbances in sepsis. Intensive Care Med Exp. 3, (Suppl. 1), A686.
Lan, Y., Wang, X., Lou, J., Ma, X., and Zhang, B. (2017). The potential roles of aquaporin 4 in malignant gliomas. Oncotarget 8, 32345–32355.
Li, B., Li, T., Liu, J., and Liu, X. (2020). Computational deconvolution of tumor-infiltrating immune components with bulk tumor gene expression data. Methods Mol. Biol. 2120, 249–262.
Li, J., Jia, M., Chen, G., Nie, S., Zheng, C., and Zeng, W. (2019). Involvement of p38 mitogen-activated protein kinase in altered expressions of AQP1 and AQP4 after carbon monoxide poisoning in rat astrocytes. Basic Clin. Pharmacol. Toxicol. 125, 394–404.
Lima, F., Kahn, S., Soletti, R., Biasoli, D., Alves, T., da Fonseca, A., et al. (2012). Glioblastoma: therapeutic challenges, what lies ahead. Biochim. Biophys. Acta Rev. Cancer 2, 338–349.
Lohela, T., Lilius, T., and Nedergaard, M. (2022). The glymphatic system: implications for drugs for central nervous system diseases. Nat. Rev. Drug Discov. Epub ahead of print. doi: 10.1038/s41573-022-00500-9
Martinez-Lage, M., and Sahm, F. (2018). Practical implications of the updated WHO classification of brain tumors. Semin. Neurol. 38, 11–18.
Maugeri, R., Schiera, G., Di Liegro, C., Fricano, A., Iacopino, D., and Di Liegro, I. (2016). Aquaporins and brain tumors. Int. J. Mol. Sci. 17:1029.
Mestre, H., Tithof, J., Du, T., Song, W., Peng, W., Sweeney, A., et al. (2018). Flow of cerebrospinal fluid is driven by arterial pulsations and is reduced in hypertension. Nat. Commun. 9:4878.
Moon, C., Moon, D., and Kang, S. (2022). Aquaporins in cancer biology. Front. Oncol. 12:782829. doi: 10.3389/fonc.2022.782829
Nauman, P., Bonicki, W., Michalik, R., Warzecha, A., and Czernicki, Z. (2004). The concentration of thyroid hormones and activities of iodothyronine deiodinases are altered in human brain gliomas. Folia Neuropathol. 42, 67–73.
Ohmura, K., Tomita, H., and Hara, A. (2023). Peritumoral edema in gliomas: a review of mechanisms and management. Biomedicines 11: 2731.
Ostrom, Q., Bauchet, L., Davis, F., Deltour, I., Fisher, J., Langer, C., et al. (2014). The epidemiology of glioma in adults: a state of the science review. Neuro Oncol. 16, 896–913.
Perry, A., and Wesseling, P. (2016). “Histologic classification of gliomas,” in Handbook of Clinical Neurology, ed. Elsevier (Cambridge, MA: Academic Press).
Rosu, G., Catalin, B., Balseanu, T., Laurentiu, M., Claudiu, M., Kumar-Singh, S., et al. (2020). Inhibition of Aquaporin 4 Decreases Amyloid Abeta40 Drainage Around Cerebral Vessels. Mol. Neurobiol. 57, 4720–4734.
Saadoun, S., Papadopoulos, M., Davies, D., Krishna, S., and Bell, B. (2002). Aquaporin-4 expression is increased in oedematous human brain tumours. J. Neurol. Neurosurg. Psychiatry 72, 262–265.
Sadana, P., Coughlin, L., Burke, J., Woods, R., and Mdzinarishvili, A. (2015). Anti-edema action of thyroid hormone in MCAO model of ischemic brain stroke: possible association with AQP4 modulation. J. Neurol. Sci. 354, 37–45.
Saito, T., Mizumoto, M., Liang, H., Nakai, K., Sumiya, T., and Iizumi, T. (2022). Factors involved in preoperative edema in high-grade gliomas. Cureus 14: e31379.
Schiera, G., Di Liegro, C., and Di Liegro, I. (2021). Involvement of thyroid hormones in brain development and cancer. Cancers 13:2693.
Sherman, B., Hao, M., Qiu, J., Jiao, X., Baseler, M., Lane, H., et al. (2022). DAVID: a web server for functional enrichment analysis and functional annotation of gene lists (2021 update). Nucleic Acids Res. 50, W216–W221.
Simone, L., Pisani, F., Mola, M., De Bellis, M., Merla, G., Micale, L., et al. (2019). AQP4 aggregation state is a determinant for glioma cell fate. Cancer Res. 79, 2182–2194.
Smith, A., Yao, X., Dix, J., Jin, B., and Verkman, A. (2017). Test of the ‘glymphatic’ hypothesis demonstrates diffusive and aquaporin-4-independent solute transport in rodent brain parenchyma. eLife 6:e27679.
Solar, P., Hendrych, M., Barak, M., Valekova, H., Hermanova, M., and Jancalek, R. (2022). Blood-brain barrier alterations and edema formation in different brain mass lesions. Front. Cell Neurosci. 16:922181. doi: 10.3389/fncel.2022.922181
Soldatelli, J., Oliveira, I., Kneubil, M., and Henriques, J. (2022). Gliomas molecular markers: importance in treatment, prognosis and applicability in brazilian health system. Acad. Bras. Cienc. 94:e20211075.
Soveral, G., Prista, C., and Moura, T. F. Loureiro-Dias, M. C. (2010). Yeast water channels: One overview of orthodox aquaporins. Cell Biol. 103, 35–54. doi: 10.1042/BC20100102
Strobel, H., Baisch, T., Fitzel, R., Schilberg, K., Siegelin, M., Karpel-Massler, G., et al. (2019). Temozolomide and other alkylating agents in glioblastoma therapy. Biomedicines 7:69.
Sun, C., Lin, L., Yin, L., Hao, X., Tian, J., Zhang, X., et al. (2022). Acutely inhibiting AQP4 With TGN-020 improves functional outcome by attenuating edema and peri-infarct astrogliosis after cerebral ischemia. Front. Immunol. 13:870029. doi: 10.3389/fimmu.2022.870029
Suzuki, Y., Nakamura, Y., Yamada, K., Kurabe, S., Okamoto, K., and Aoki, H. (2018). Aquaporin positron emission tomography differentiates between grade III and IV human astrocytoma. Neurosurgery 82, 842–846.
Tang, Z., Kang, B., Li, C., Chen, T., and Zhang, Z. (2019). GEPIA2: an enhanced web server for large-scale expression profiling and interactive analysis. Nucleic Acids Res. 47, W556–W560.
Ulgen, E., Ozisik, O., and Sezerman, O. (2019). pathfindR: an r package for comprehensive identification of enriched pathways in omics data through active subnetworks. Front. Genet. 10:858. doi: 10.3389/fgene.2019.00858
Wu, C., Lin, G., Lin, Z., Zhang, J., Liu, S., and Zhou, C. (2015). Peritumoral edema shown by MRI predicts poor clinical outcome in glioblastoma. World J. Surg. Oncol. 13:97.
Yasui, M., Hazama, A., Kwon, T. H., Nielsen, S., Guggino, W. B., and Agre, P. (1999). Rapid gating and anion permeability of an intracellular aquaporin. Nature 402, 184–187. doi: 10.1038/46045
Zhao, S., Zhou, H., Peng, X., Tan, S., Liu, Z., Chen, T., et al. (2017). Detection of thyroid abnormalities in Aquaporin-4 antibody-seropositive optic neuritis patients. J. Neuroophthalmol. 37, 24–29.
Keywords: gliomas, AQPs, water movement, biomarkers, gene expression, thyroid hormones, correlation analysis
Citation: Mendes CB, da Rocha LS, de Carvalho Frag CA and Ximenes-da-Silva A (2024) Homeostatic status of thyroid hormones and brain water movement as determinant factors in biology of cerebral gliomas: a pilot study using a bioinformatics approach. Front. Neurosci. 18:1349421. doi: 10.3389/fnins.2024.1349421
Received: 04 December 2023; Accepted: 07 February 2024;
Published: 27 February 2024.
Edited by:
Fahmeed Hyder, Yale University, United StatesReviewed by:
Ana-Maria Oros-Peusquens, Helmholtz Association of German Research Centres (HZ), GermanyCharles Springer, Oregon Health and Science University, United States
Copyright © 2024 Mendes, da Rocha, de Carvalho Fraga and Ximenes-da-Silva. This is an open-access article distributed under the terms of the Creative Commons Attribution License (CC BY). The use, distribution or reproduction in other forums is permitted, provided the original author(s) and the copyright owner(s) are credited and that the original publication in this journal is cited, in accordance with accepted academic practice. No use, distribution or reproduction is permitted which does not comply with these terms.
*Correspondence: Adriana Ximenes-da-Silva, YXhpbWVuZXNAY2NiaS51ZmFsLmJy