- SANA Hospital Leipzig County, Borna, Germany
The mechanisms underlying Parkinson’s disease (PD) are complex and not fully understood, and the box-and-arrow model among other current models present significant challenges. This paper explores the potential role of the allocentric brain and especially its grid cells in several PD motor symptoms, including bradykinesia, kinesia paradoxa, freezing of gait, the bottleneck phenomenon, and their dependency on cueing. It is argued that central hubs, like the locus coeruleus and the pedunculopontine nucleus, often narrowly interpreted in the context of PD, play an equally important role in governing the allocentric brain as the basal ganglia. Consequently, the motor and secondary motor (e.g., spatially related) symptoms of PD linked with dopamine depletion may be more closely tied to erroneous computation by grid cells than to the basal ganglia alone. Because grid cells and their associated central hubs introduce both spatial and temporal information to the brain influencing velocity perception they may cause bradykinesia or hyperkinesia as well. In summary, PD motor symptoms may primarily be an allocentric disturbance resulting from virtual faulty computation by grid cells revealed by dopamine depletion in PD.
Introduction
“Grid cells are such a beautiful and unique phenomenon in the nervous system that it is tempting to regard them as a crucial element of its design” (Kropff and Treves, 2008).
The existing model of the basal ganglia (BG) and dopamine depletion (DD) in Parkinson’s disease (PD) goes back to the late 1950s (Carlsson et al., 1957; Carlsson and Waldeck, 1958; Ehringer and Hornykiewicz, 1960; Birkmayer and Hornykiewicz, 1961; Hornykiewicz, 1962; Bernheimer and Hornykiewicz, 1965; Carlsson, 1971; Yeragani et al., 2010). This model was further refined in the 1980s and early 1990s with the formulation of the direct and indirect striatal output pathways and the “box-and-arrow” model (Penney and Young, 1986; Albin et al., 1989; DeLong, 1990; Nambu et al., 2002; Plotkin and Goldberg, 2018). Although this “has led to groundbreaking strategies to treat motor disorders” (Plotkin and Goldberg, 2018), it “fails to explain certain clinical findings and leaves a number of paradoxes” (Brown and Marsden, 1998, p. 1801), leaving us “far from a comprehensive mechanistic understanding of the pathophysiology of PD” (Wichmann et al., 2011). Furthermore, neurocomputational models (Gurney et al., 2001a,b; Fiore et al., 2016; Suryanarayana et al., 2019; Hjorth et al., 2020) and also trials with respect to deep brain stimulation (DBS) have demonstrated that current concepts of basal ganglia pathophysiology have reached “the point where total rejection, rather than continual attempts at modification, is necessary” (Montgomery, 2011, p. 14). Numerous critical reviews on this topic underline these limitations (Marsden and Obeso, 1994; Mink, 1996; Nambu, 2008; Wichmann et al., 2011; Plotkin and Goldberg, 2018).
The BG pathway refers to medium spiny neurons (MSN), which form clustered cell groups. These groups are activated not only by active and passive manipulation of one body part, but also by their cutaneous stimulation (Crutcher and DeLong, 1984; Alexander and DeLong, 1985; Carelli and West, 1991; Coffey et al., 2016, 2017) are referred to as single body parts (SBP). These SBP deliver a body-referenced frame that primarily “encodes action space” (Klaus et al., 2017) from the egocentric view.
However, as movement is generally goal-directed, originating from a starting point and progressing toward an endpoint, and often involving the late-stage positioning of a potential target, it relates to the external environment/space (Redgrave et al., 2010; Penner and Mizumori, 2012; Elliott et al., 2017; Grieves and Jeffery, 2017; Pernia-Andrade et al., 2021). It is therefore essential to consider not only the animal’s perspective of the goal’s position, but also the goal’s representation relative to external contextual features (the allocentric frame; Neely et al., 2008; Chersi and Burgess, 2015).
By applying the allocentric properties of grid cells to parkinsonian symptoms in connection with dopamine depletion (DD), we can gain new insights into the pivotal role of the allocentric brain not just in PD, but also in the inception of movement.
What we know
The allocentric space model
An individual’s objective position and the location of desirable goals are deduced from external landmarks and determined within the hippocampal place cells (O'Keefe and Dostrovsky, 1971). In turn, the computation of allocentric movement, necessary to navigate between landmarks, depends on grid cells (GCs) in the medial entorhinal cortex (mEC)—mainly within layer II in particular (Fyhn et al., 2004; Hafting et al., 2005; Moser et al., 2008; Boccara et al., 2010) (see Figure 1). The mEC consists of about two thirds reelin-positive stellate cells (SC), which supply the dentate gyrus and the hippocampus, also called “ocean cells,” surrounding about one third so-called pyramid cells (PC) or “island cells” projecting to mEC layer I and the contralateral EC. Both SC and PC are influenced by inhibitory microcircuits of different interneurons (Gatome et al., 2010; Witter et al., 2017; Naumann et al., 2018; Tukker et al., 2022) and can function as grid cells with an emphasis on pyramid cells (Tang et al., 2014; Rowland et al., 2018). Grid cells again receive significant dopaminergic innervation (Fallon et al., 1978; Akil and Lewis, 1993; Li et al., 2015).
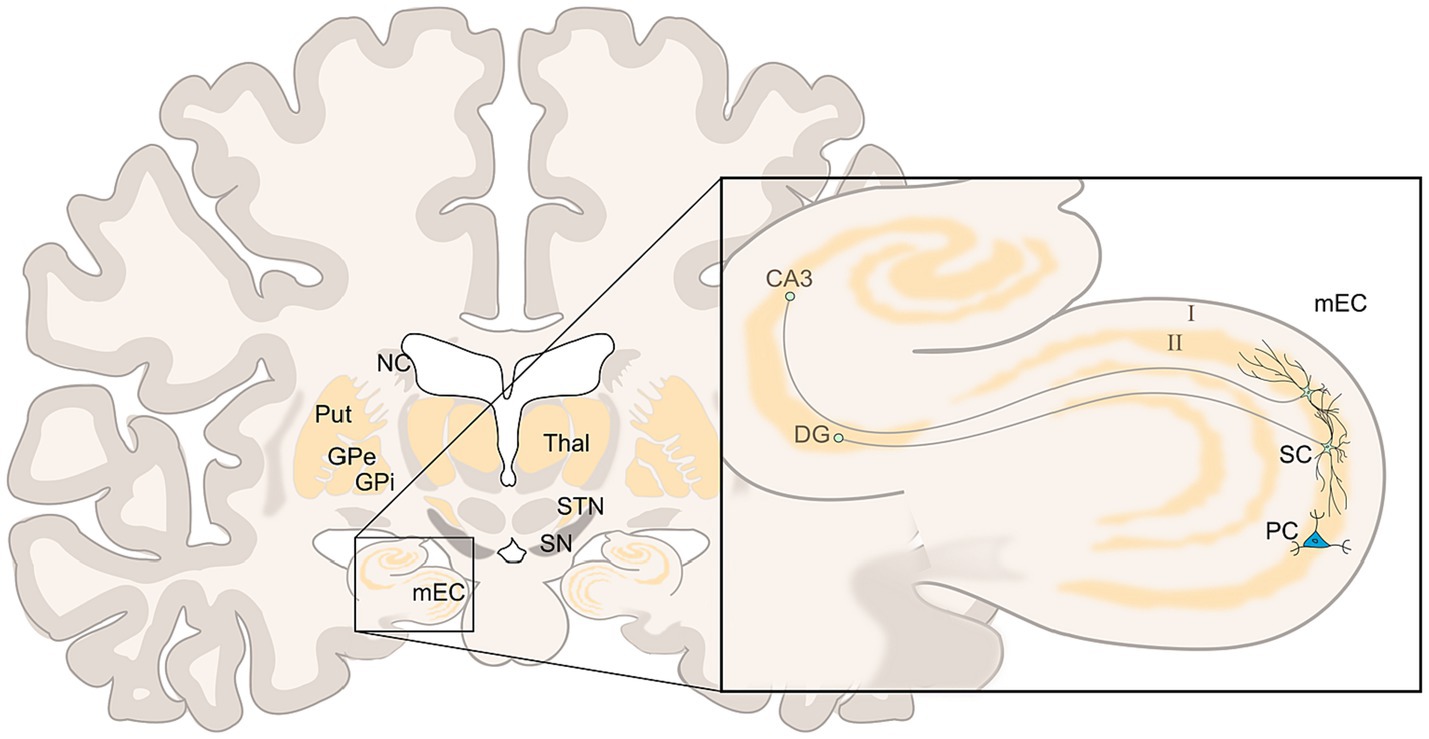
Figure 1. Coronar view of the brain at the EC/HF level (left), in detail (right) two stellate cells (SC), and one pyramid cell (PC) of layer II of mEC, the SC branching within layer II with efferences to the dentate gyrus (DG) and hippocampal cornu ammonis (CA3); left: nucleus caudatus (NC), putamen (Put), and globus pallidus (GPe and GPi) are less voluminous in this level, further shown substantia nigra (SN), subthalamic nucleus (STN), and thalamus (Thal). Adopted from Nieuwenhuys et al. (2008) and Park et al. (2019).
Grid cell generate the external spatial allocentric reference overlaying the animal’s surrounding floor with a two-dimensional hexagonal pattern/carpet (the grid fields) formed by isosceles triangles (Hafting et al., 2005; Burak, 2014; Moser et al., 2014; Knierim, 2015; Vago and Ujfalussy, 2018; Mosheiff and Burak, 2019) (see Figure 2A). The emergence and maintenance of GCs and grid fields rely on exploratory movement, which is anchored to external landmarks and borders (Hafting et al., 2005; Savelli et al., 2008; Couey et al., 2013; Pastoll et al., 2013; Stensola et al., 2015). This movement again generates a self-organizing internal spiking within GCs based on local network dynamics (LFP, described in more detail below). For this, similar to striatal SBP, GCs receive spatial information in the form of copies of multimodal sensory input from external landmarks through self-motion data. This data originate from “vestibular, proprioceptive, visual (optic flow) and motor (motor-efference copy) systems” (Jeffery, 2007; Mohedano-Moriano et al., 2008; Barry and Burgess, 2014, p R332; Perez-Escobar et al., 2016; Nadasdy et al., 2017; Campbell et al., 2018), most visual cues being particularly relevant (Maaswinkel and Whishaw, 1999; Chen et al., 2013; Kinkhabwala et al., 2020). GCs are grouped into modules that share the same scale/period (for distance computing) and orientation (relative to external references), but have different phases (relative positioning of grid fields). These modules present a dorso–ventral gradient within the mEC, with exponentially larger scaling for the ventral modules (Barry et al., 2007; Brun et al., 2008; Stensola et al., 2012; Bant et al., 2020).
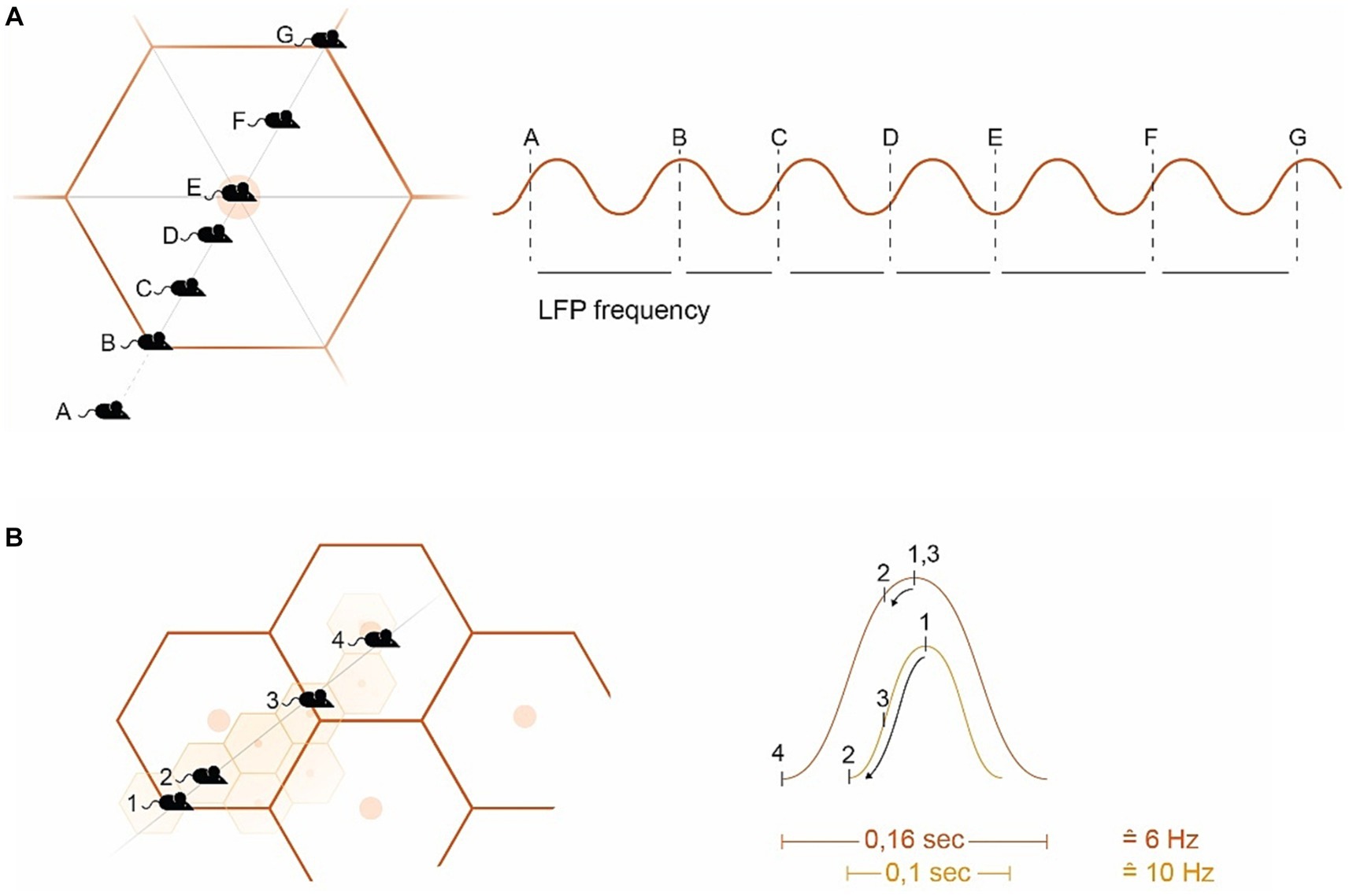
Figure 2. Introducing hexagonal grid fields (GF) (A) Example of a mouse navigating a GF. The GC spike timing within the GF aligns with the LFP’s frequency band (sine wave on the right). Starting from the edge of the GF (B), the GC spike occurs at the peak of the SW. As the mouse approaches the GF vertex (C,D), the spiking activity descends on the subsequent SW, ending in the slack portion of the sine curve when the GF vertex is reached (E). Departing from the GF vertex signals conditions climbing up (F) with (G) again starting for the next GF. There is an ambiguity in the directional interpretation of the (A) and (F) signals (toward or away from the vertex) (Mathis et al., 2013). (B) Example of overlapping GFs, where large GFs are computed in ventral GC modules at a low frequency (e.g., 6 Hz), and smaller GFs in the dorsal mEC at a higher frequency (e.g., 10 Hz) (simplified representation). Mouse position (MP)1 is at the edge of both the small GF (smGF) and the large GF (lgGF), triggering internal GC spiking at the peak of the sine wave (right). MP2 generates a partial PP from the lgGF, but a substantial PP for the smGF reaching the slack (with the descending arrows within the sine wave indication theta phase precession). MP3 is again on the edge of the lgGF but halfway to the smGF vertex, while MP4 lies on the edge of the smGF but on the vertex of the lgGF. GF, Grid field; smGF, Small GF; lg, Large GF; MP, Mouse position; PP, Phase precession; and SW, Sine wave.
Entorhinal theta phase precession and velocity integration
Within the hippocampal-entorhinal (HF/EC) formation, there is a prominent theta oscillation ranging from approximately 6–11 Hz, known as the “local field potential” (LFP) (Vanderwolf, 1969; Eggink et al., 2014). This oscillation is largely driven by the medial septum (the diagonal band of Broca, MSDB), but underlies the spatial periodicity and internal spiking properties of GCs as well (Burgess et al., 2007; Fyhn et al., 2007; Brandon et al., 2011; Barry et al., 2012; Pilly and Grossberg, 2013; Shay et al., 2016; Jacob et al., 2017; Tsanov, 2017; Joshi and Somogyi, 2020). On the other side active movement stimulates GCs’ internal spiking, which increases in frequency and aligns with the LFP frequency as the vertex of the grid field is approached (Burgess et al., 2007; Giocomo and Hasselmo, 2008; Shay et al., 2016; Gu and Yakel, 2017) such that internal spikes, originating from the peak of the sine wave, descend the wave arriving the slack of the LFP sine wave by reaching the vertex of the grid field, with the leading spike’s phase delivering mostly spatial information (Reifenstein et al., 2012) (see Figure 2A).
This precession of spikes related to the LFP (i.e., relative to the sum of nearby firing cells) is termed “theta phase precession” (TPP) initially described in place cells (O'Keefe and Recce, 1993; Skaggs et al., 1996) later in GCs as well (Hafting et al., 2008) and again later within the ventral striatum (van der Meer and Redish, 2011; Malhotra et al., 2012). As GCs’ scales compute distances, their TPP signifies distances traveled in a given time that establishes the quality of speed and therefore time, velocity, and acceleration in GC computation (Burgess et al., 2007; Zilli, 2012; Kropff et al., 2021). In this regard, the allocentric computation of GCs introduces the concept of time into the brain (Kropff et al., 2015; Heys and Dombeck, 2018; Alexander et al., 2020; Carvalho et al., 2020; Heys et al., 2020; Ridler et al., 2020) (see Figure 2B). This is complemented by the MSDB’s parvalbumin-positive cells modulating the entorhinal LFP’s speed information (Lepperod et al., 2021) and its glutamatergic circuit that controls the initiation and velocity of locomotion (Fuhrmann et al., 2015; Justus et al., 2017). I argue that the nature of GC’s theta phase precession (TPP) misgauged in dopamine depletion accounts for bradykinesia generating the slowdown of movement (see Figure 2).
The link between the striatum and the hippocampal formation
To translate goal-directed allocentric components into striatal egocentric self-motion computation and vice versa, the striatum and the HF/EC are effectively linked (Finch et al., 1995; Totterdell and Meredith, 1997; Devan and White, 1999; Hartley et al., 2003; Johnson et al., 2007; Lex and Hauber, 2010; van der Meer et al., 2010; Ghiglieri et al., 2011; Verschure et al., 2014; Stoianov et al., 2018). Constant switching takes place between them (Bohbot et al., 2004; Burgess, 2006; Harris et al., 2012; Colombo et al., 2017), a process that appears to be dopamine-dependent (Penner and Mizumori, 2012) and is driven by the locus coeruleus (LC), the structure to be impaired in PD first (Hornykiewicz and Kish, 1987; German et al., 1992; Braak et al., 2003; Zarow et al., 2003; Braak and Del Tredici, 2017; Vermeiren and De Deyn, 2017; Nahimi et al., 2018; Oertel et al., 2019; Giorgi et al., 2020; Zhou et al., 2021) (see Figure 3). Damage to connecting fibers between the striatum and the HF/EC, the striato-HF/EC loop, disrupts precise navigation in open environments (Devan et al., 1996; Gorny et al., 2002) and the deactivation of one side tends to increase the compensatory use of the other (Packard and McGaugh, 1996; Igloi et al., 2009; Sodums and Bohbot, 2020) with the LC playing a crucial role in creating spatial representations especially sensitive to environmental novelty (Harris et al., 2012; Takeuchi et al., 2016; Ruggiero et al., 2018; Zhong and Moffat, 2018).
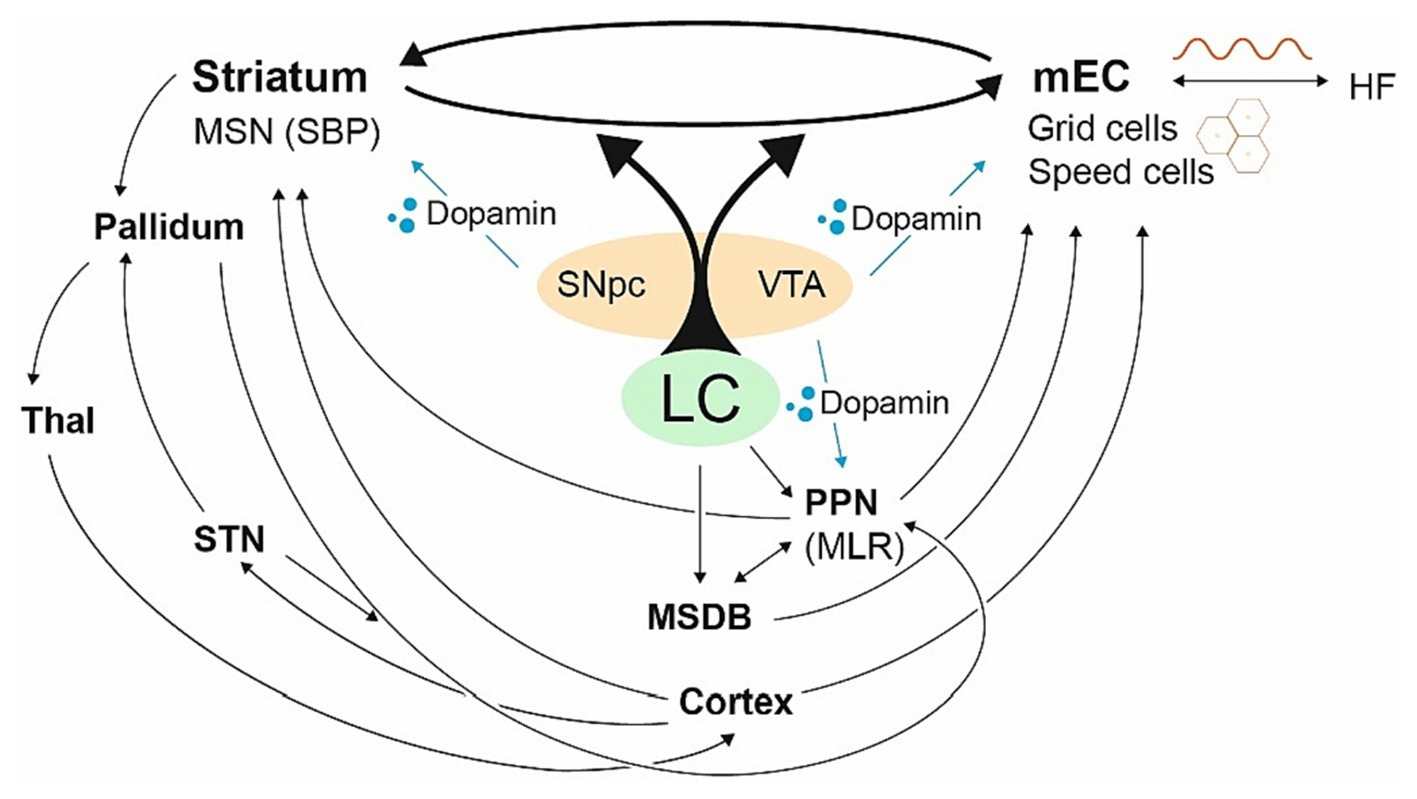
Figure 3. The striato-HF/EC loop with its central hubs. Fragmentary visualization of central hubs dedicated to the basal ganglia and the allocentric brain. The LC balances the striato-HF/EC loop, the HF/EC primarily receives dopaminergic signals from the VTA, the striatum from SNpc. The LC drives the MSDB and the PPN as well driving the HF/EC with grid cells and its LFP (sine wave). The PPN has strong connections with the striatum, modulating the SNpc and VTA (Floresco et al., 2003; Mena-Segovia et al., 2004; Valencia et al., 2014; Roseberry et al., 2016; Mena-Segovia and Bolam, 2017), yet also powerful projections via the MSDB coding mEC speed cells controlling the initiation of locomotion (Fuhrmann et al., 2015). The PPN can be powered by the pallidum and the STN as well. In general, hubs, which are closely related to PD, are heavily involved in the allocentric brain.
For both the striatum and the HF/EC, dopamine is supplied from mesencephalic structures—which undergo loss of dopaminergic neurons in PD (Bogerts et al., 1983; Akil and Lewis, 1993; Jin et al., 2019). Whereas the dorsal striatum is primarily supplied by the substantia nigra pars compacta (SNpc), HF/EC mainly receives dopamine from the ventral tegmental area (VTA) (Scatton et al., 1980; Oades and Halliday, 1987; Gasbarri et al., 1997; Rosen et al., 2015) (see Figure 3). Although dopamine depletion is comparable in early PD, the VTA dopamine supply exhibits higher inter-subject variability (Caminiti et al., 2017; Bortz and Grace, 2018).
Altered somatotopy disrupts body representation
The striatal side of the striato-HF/EC loop with its ontogenetic optimized sensorimotor SBPs undergoes an up to 16-fold decrease in PD (Cho et al., 2002). This results in SBPs becoming responsible for not one but as many as three or five body parts, becoming fragmented, existing in clusters or isolated cells outside their diminished former clusters (“satellite potentials”) (Cho et al., 2002; Obeso et al., 2008a; Bronfeld and Bar-Gad, 2011; Coffey et al., 2016). There was the argument that these (striatal) “distorted internal body representations… may contribute to bradykinesia, impaired movement scaling, and the strong reliance on visual feedback” (Contreras-Vidal and Gold, 2004, p 505) that seems to be much more attributable to the other side of the striato-HF/EC loop: the GCs with (1) their (distance) scaling properties, (2) their reference to time and velocity (Kropff et al., 2015; Heys and Dombeck, 2018; Carvalho et al., 2020; Heys et al., 2020), and (3) their ligation to external landmarks/cues, with visual cues being the most influential (Maaswinkel and Whishaw, 1999; Hardcastle et al., 2015; Stensola et al., 2015; Chen et al., 2016; Perez-Escobar et al., 2016; Campbell et al., 2018; Keinath et al., 2018; Mosheiff and Burak, 2019; Kinkhabwala et al., 2020).
Compared to the striatal system, the ontogenetically young GC system is highly malleable in its physiological state already (Barry et al., 2007; Langston et al., 2010; Wills et al., 2010; Stensola et al., 2012; Krupic et al., 2015; Latuske et al., 2015; Dunn et al., 2017; Ismakov et al., 2017). Thus, disruptive shifts occur in GCs, with half of grid cells changing to multiple distance and time computations (Kraus et al., 2015), compressing (Raudies and Hasselmo, 2015), skipping (Deshmukh et al., 2010), or even getting lost their grid fields. Even partial inactivation may be enough to disturb allocentric computing, necessitating complete spatial allocentric remapping (Miao et al., 2015; Rueckemann et al., 2016; Savelli et al., 2017). It is only their robust redundancy and the pooling of all information that allow the complete spatial function of GCs (Reifenstein et al., 2012). The recent discovery of an aperiodic 3D GC pattern in flying bats (Ginosar et al., 2021) implies that there are far more inconsistencies in the real 3D world than in the 2D lattice mazes that shape our current understanding of GCs.
Hypotheses: dopamine-depleted grid cells evoke Parkinson’s symptoms
We are all too familiar with the debilitating symptoms of PD, yet how they manifest within the framework of the BG-focused box-and-arrow model remains a mystery (Mink, 1996; Brown and Marsden, 1998; Montgomery, 2011). In contrast, when considering the allocentric brain with its grid cells in particular, PD symptoms seem to be self-explanatory, as will be shown below.
“Conceptual hypometria” as a fundamental symptom in PD
Hypometria may not be the first symptom we bear in mind when discussing PD, but it serves to introduce some key ideas. Just think of clinical signs of hypometria in PD patients, including perceiving distances as shorter (Demirci et al., 1997; Kabasakalian et al., 2013), failing to reach far enough when trying to grasp objects (Klockgether and Dichgans, 1994; Kulkarni et al., 2013; Ryckewaert et al., 2015), underestimating the sizes of objects and openings (Harris et al., 2003; Young et al., 2010; Smith et al., 2011; Laudate et al., 2013), and an impaired ability to perceive large spatial configurations (Barrett et al., 2001; Bernardinis et al., 2018). Therefore, it has been argued that in PD “the sensorimotor apparatus is ‘set smaller’” (Demirci et al., 1997) in line with a constriction of the “perception of extrapersonal space” or a virtual compressed space (Lee et al., 1998, 2001b; Davidsdottir et al., 2008). This has led to the postulation of not only a virtual egocentric but allocentric hypometria in PD, termed “conceptual hypometria” (Skidmore et al., 2009; Kabasakalian et al., 2013).
Given that GCs represent external space virtually, their dwindling in the context of dopamine depletion (DD) (see above for mEC dopamine receptors; Fallon et al., 1978; Akil and Lewis, 1993; Li et al., 2015) would first and foremost weaken the large spatial allocentric representation because ventral GCs, which represent large spatial scaling, are sparse (Brun et al., 2008; Burgess, 2008; Jeewajee et al., 2008), have a lower signal-to-noise ratio (Bant et al., 2020), and occasionally get lost or switched off (Campbell et al., 2018) in their physiological status already limiting large grid field computations, but favoring smaller ones.
Furthermore, if the mechanisms of TPP (see above), which normally record the time spent crossing a grid field, are detached from other consistent information or even become inverted in DD, they may signal premature or mistaken spiking. In particular, as the leading GC’s spike yields a clear signature of TPP (Reifenstein et al., 2012), the system might become more volatile with its disinhibition, generating an “already reached” or “closer than” signal and thus restricting the virtual space and impacting the forward planning of velocity (see Figure 4).
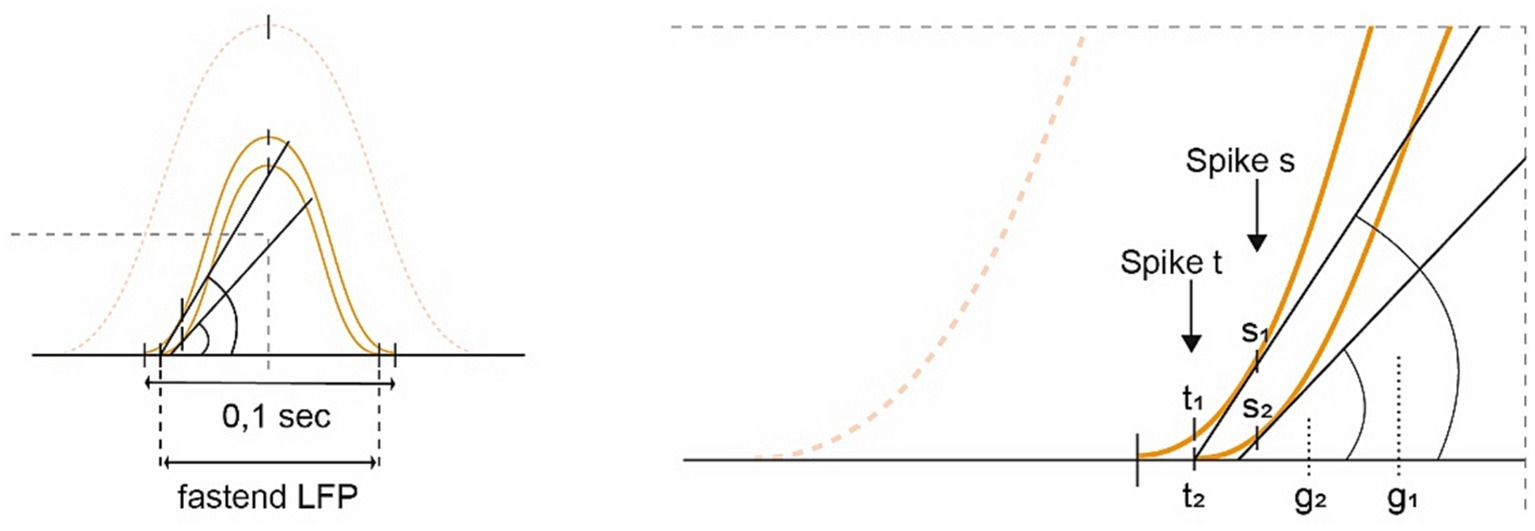
Figure 4. Diagram depicting the idea of “conceptual hypometria.” Building upon Figure 2B, this image demonstrates the effects of losing slower GC modules (dotted sine wave; SW), which increasingly accelerates the remaining ones [shifting from the outer toward the inner small SWs (SW 1 2)]. If the internal firing frequency were to remain constant, the spikes would arrive earlier on the left-hand ascending slope of the fastened and downscaled SW (SW 2), potentially exacerbated by a loss of LFP stability. For spike s, the rise (g2) is smaller in the accelerated SW compared to the original slope (g1). Whereas signal t1 from the stable SW indicates that there is still some distance to cover, t2 of the weakened SW 2 signals “already reached,” substantiating the hypothesis of “conceptual hypometria.”
All in all, this supports the idea of a downsized allocentric virtual space, in the sense of “conceptual hypometria.” Note that GC function also deteriorates under social conditions due to an increased GC firing rate (Xu et al., 2022), which is not further elaborated on in this paper.
Bradykinesia
Bradykinesia, one of the primary and most debilitating motor symptoms of PD, is characterized by a marked slowness of movement (Marsden, 1989). This symptom has been postulated to arise from the inhibition of the primary motor cortex, a consequence of the overstimulation of the globus pallidus internus (GPi) (Berardelli et al., 2001; Spiegel et al., 2007; Moccia et al., 2014). However, the validity of this model is challenged by the fact that GPi inactivation improves not just bradykinesia, but also its antithesis, L-dopa-induced dyskinesia (LID, see below) (Laitinen et al., 1992; Brown and Marsden, 1998). Consequently, doubt persists regarding this explanation and the model in general (Obeso et al., 2008b; Bologna et al., 2020).
As described above, the concept of time is conveyed to the brain via GCs’ TPP (Harris et al., 2002; Sargolini et al., 2006; Burgess, 2008; Hafting et al., 2008; Climer et al., 2013; Eichenbaum, 2014; Kraus et al., 2015; Kropff et al., 2015; Schlesiger et al., 2015; Hardcastle et al., 2017; Heys and Dombeck, 2018; Tsao et al., 2018; Ye et al., 2018; Jacob et al., 2019; Carvalho et al., 2020; Heys et al., 2020). As an animal moves faster or even accelerates its movement, the internal firing/spiking of grid cells occurs earlier in their physiological state. However, when faced with an errant virtual hypometria (see above), the GCs signal as if the animal has moved a greater distance than it actually has what would compute a demand note to slow down or even to stop movement altogether. Bradykinesia could therefore be a secondary effect of “conceptual hypometria” (see above and Figure 4), arguing for limb movements as well as for whole body motion (see below for limitations).
Furthermore, to introduce time into the brain, there are discrete “speed cells” found in GC formation—mainly fast-spiking interneurons, which constitute about 15% of layer II mEC cells (Couey et al., 2013; Buetfering et al., 2014; Kropff et al., 2015). These speed cells are driven externally by neurons from the pedunculopontine nucleus (PPN) and are highly correlated with future speed (Rolland et al., 2009; Ryczko et al., 2013; Ryczko and Dubuc, 2017), the PPN again modulating neurons in the MSDB (Justus et al., 2017; Carvalho et al., 2020). The PPN and MSDB are, in turn, both influenced by the LC and dopamine, with the MSDB containing cells that fluctuate as a function of running speed (Zhou et al., 1999; see Figure 3).
In dopamine-deficient conditions, grid and speed cells may lose their anticipatory internal spiking functions. This suggests that grid cells (GCs) have a harder time calculating the upcoming grid field, which is essential for transitioning from the current position to the next, or as Tukker and coworkers write, that changing properties of the grids in altered or novel environments might limit their representational capacity (Tukker et al., 2022), not to mention turbulences caused by dopamine deficiency (DD) of the weakened grid cells themselves, or their decoupling from the striatal egocentric counterpart (see for GC’s malleability above), negatively affecting their accelerating and computational properties promoting bradykinesia.
At the same time, speed cells may become disconnected from future movement (Kropff et al., 2015; Ye et al., 2018). Coupled with the potentially inaccurate activity of the fusimotor system, which acts as a “forward sensory model” too (Dimitriou and Edin, 2010), this can lead to a decrease in movement speed, resulting in bradykinesia.
Kinesia paradoxa
Kinesia paradoxa (KP) refers to the abrupt shift from bradykinetic to normal velocity, typically brought about by an external cue (Glickstein and Stein, 1991; Distler et al., 2016; Duysens and Nonnekes, 2021). As GCs are thought to generate multiple speed computations (Jeewajee et al., 2008; Kraus et al., 2015; Hinman et al., 2016) for example by skipping theta cycles (Deshmukh et al., 2010; Kraus et al., 2015), see above, the phenomenon of KP can also be comprehended from the GC’s perspective. Skipping from slow to “normal velocity” is probably facilitated by the noradrenergic LC, which allows for “rapid behavioral adaptation to changing environmental and (unpredicted) imperatives” (Aston-Jones and Bloom, 1981; Bouret and Sara, 2005), additionally supported by the PPN (Carvalho et al., 2020), which plays a role in escape responses (Caggiano et al., 2018) and drives both grid and speed cells.
Sequence effect
The sequence effect (SE) is a clinical term denoting the progressive shortening of step length observed during repetitive movements influencing handwriting, gait, and speech (Benecke et al., 1987; Agostino et al., 1992; Iansek et al., 2006; Wu et al., 2016). The SE does not improve with dopamine supply (Kang et al., 2010; Lee et al., 2015; Bologna et al., 2020), but tends to occur less frequently in advanced PD (Bologna et al., 2016) and responds positively to visual cues (Iansek et al., 2006; Tinaz et al., 2016). From an allocentric standpoint, this phenomenon could be seen as a form of self-perpetuating hypometria, possibly resulting from the continuous depletion of larger and more susceptible GCs or from a self-reinforcing mechanism focusing on smaller dimensions (this will be further discussed below).
Festination
Festination, another fascinating parkinsonian paradox, refers to “a progressive shortening of step length, in that case accompanied by a compensatory increase in cadence” (Iansek et al., 2006; Nonnekes et al., 2019a), affecting handwriting and speech (Martin et al., 1994; Giladi et al., 2001; Moreau et al., 2007; Nutt et al., 2011) as well. Festination essentially embodies GCs’ characteristics by reducing amplitudes and generating faster speed computations (Jeewajee et al., 2008; Kraus et al., 2015; Hinman et al., 2016). In simpler terms, it equates to pacing in smaller grid fields with compensatory acceleration for the increased cadence (Jeewajee et al., 2008; Kraus et al., 2015; Hinman et al., 2016; Kropff et al., 2021) – the last contradicting being brady-kinetic observed such as in freezing episodes and trembling (see below).
Micrographia
Micrographia is defined as an “obvious reduction in the size of letters” in handwriting. This symptom is observed in up to three quarters of PD patients (Jarzebska, 2006; Wagle Shukla et al., 2012), with about two thirds exhibiting a waning amplitude (Zham et al., 2019) known as progressive micrographia. This pattern is strikingly similar to the SE, as opposed to continuous micrographia (Kinnier Wilson, 1925; Inzelberg et al., 2016; Wu et al., 2016), which appears more hypometric. Importantly, micrographia can be improved with visual cues such as markers or lines (McLennan et al., 1972; Oliveira et al., 1997; Bryant et al., 2010).
An early study on micrographia argued that it “seems to be a compression of words into insufficient space” (McLennan et al., 1972), thus drawing an early connection to the concept of external (virtual hypometric) space. Hypothetically, if the first letters written are related to the external space, the following ones could lose their external spacing for two reasons. Firstly, writing is predominantly an egocentric activity that can overlook its allocentric calibration. Secondly, due to the enlarged SBPs, continuous calibration to a virtual oversized writing gesture occurs, in comparison to an egocentrically represented previous letter (this effect is more pronounced in progressive micrographia).
Cueing in PD
The utilization of external cues has long been recognized as a powerful tool to improve PD motor symptoms (Martin, 1967; Thaut et al., 1996; Burleigh-Jacobs et al., 1997; Lim et al., 2005; Nieuwboer, 2008; Delval et al., 2014; McCandless et al., 2016; Ginis et al., 2018; Nonnekes et al., 2019b). Flowers argued that “it seems as if the Parkinsonian subject does not seem to ‘know’ where his hand is in space nor in relation to other objects, and so must continuously monitor visually both his own movement and the external world to maintain control” (Flowers, 1976; p. 305). This observation anticipates the underlying egocentric and allocentric structures. When considering cueing and visual guiding in PD, classical PD models offer little insight, but there is evidence pointing to the GC’s significant dependency on external cues (Hardcastle et al., 2015; Chen et al., 2016; Perez-Escobar et al., 2016; Campbell et al., 2018; Mosheiff and Burak, 2019; Dannenberg et al., 2020). This is especially true for visually driven cues (Maaswinkel and Whishaw, 1999; Chen et al., 2013; Kinkhabwala et al., 2020), such as transverse bars, a laser beam, or a companion’s foot, which can enhance movement speed and accuracy, and thereby alleviate freezing of gait (FOG, see below) (Georgiou et al., 1994; Nonnekes et al., 2015; Ginis et al., 2018).
For visual cueing, the mEC not only receives robust input from visuospatial regions (Burwell and Amaral, 1998) but also direct visual input from “intrinsic mEC visual cue cells” (Kinkhabwala et al., 2020), object-vector (OV) cells responding to visual contrasts (Killian et al., 2012; Casali et al., 2018; Andersson et al., 2021), and cells responsible for gaze position (Meister and Buffalo, 2018). The latter could be the allocentric counterpart of or be reinforced by visual streaming via the (egocentric) oculomotor loop (Alexander et al., 1986; Fooken and Spering, 2020).
Freezing of gait
One of the most debilitating symptoms of PD is freezing of gait (FOG), which is characterized by a sudden, transient inability to initiate effective steps, whether when beginning to move (“start hesitation”), turning, or continuing to move (Rahman et al., 2008; Lebold and Almeida, 2010; Vercruysse et al., 2014; Matar et al., 2019). It often occurs when adapting to new forms of locomotion, encountering specific obstacles, or managing a spatial constriction through visual or proprioceptive input (Giladi and Nieuwboer, 2008; Almeida and Lebold, 2010; Nutt et al., 2011; Matar et al., 2019). FOG is notably associated with spatial references, particularly the grid field’s faced floor as occurs when “stepping from one type of surface to another” (Freezing; Parkinson’s Foundation, n.d.).
It has previously been suggested that a “disruption of the representation of external space” contributes to FOG (Lee et al., 2001a; Almeida and Lebold, 2010), pointing again to the role of the allocentric brain. Without sufficient computing the current and the subsequent grid field to facilitate the transition from one to the next—a mechanism seen in place cells (Souza and Tort, 2017)—one might feel “lost in space” or as if “stepping into the void.” FOG is often paradoxically paired with an increased cadence and uncoordinated trembling of the knees (Hausdorff et al., 2003; Schaafsma et al., 2003; Iansek et al., 2006; Jacobs et al., 2009; Nutt et al., 2011), which contradicts the notion of being purely hypo-or bradykinetic. These expressions align with the clinical manifestations of FOG (Nakamura et al., 1978; Hausdorff et al., 2003; Plotnik et al., 2005; Okuma, 2006; Plotnik and Hausdorff, 2008; Jacobs et al., 2009; Almeida and Lebold, 2010; Rehman et al., 2019).
Turning—a movement that often triggers freezing (Schaafsma et al., 2003; Spildooren et al., 2010; Mancini et al., 2017; Park et al., 2020)—relies on GCs maintaining consistent interaction with the floor (see GC phases) and on conjunctive cells, a fusion of grid and head direction cells, computing turning properties (Sargolini et al., 2006; Keinath, 2016). Internal disturbances of grid and conjunctive cells may disrupt their rotational properties, being “lost in space” or tethering them more closely to environmental borders (see below for the bottleneck phenomenon) (Krupic et al., 2015; Stensola et al., 2015) and thereby precluding turning. Overall, freezing may result from an overload of movement computation in a disturbed allocentric virtual computation (Tukker et al., 2022), particularly during turning when the linearity of grid fields is abandoned.
Freezing of gait typically lasts a matter of seconds or even minutes, aligning with the observation that dramatic GC disruption can even persist for weeks in healthy rats (Savelli et al., 2017). FOG is likely to persist until compensation strategies, such as cueing, are initiated (see above) (Lee et al., 2001a; Schaafsma et al., 2003; Lim et al., 2005; Nieuwboer, 2008; Ginis et al., 2018; Nonnekes et al., 2019b), helping to reconcile and surpass the GC’s ambiguity level (Carpenter and Barry, 2016; Savelli et al., 2017).
Remarkably, there have been reports of patients who were able to ride a bicycle directly out of a FOG episode (while standing on the floor) (Snijders et al., 2011; Kikuchi et al., 2014). This lends support to the idea that FOG is not simply a manifestation of bradykinesia, but that moving away from the disconcerting tessellating floor could resolve the computational deadlock (Freezing; Parkinson’s Foundation, n.d.). Further highlighting the allocentric brain’s responsiveness to cueing (see above), festination can be improved with spatial cues, especially visual ones (Martin et al., 1994; Nonnekes et al., 2019a).
The anatomic structure most commonly associated with FOG is the pedunculopontine nucleus (PPN) (Lewis and Barker, 2009; Virmani et al., 2019; Craig et al., 2020) that—from the allocentric view—drives mEC speed cells (see above and Figure 4), projects strongly via the MSDB to control the initiation of locomotion (Fuhrmann et al., 2015), and determines locomotor speed and gait selection (Caggiano et al., 2018; Carvalho et al., 2020).
The bottleneck phenomenon
Here I discuss a special form of FOG, the bottleneck phenomenon (BNP), which is characterized by a halt or freeze before entering narrow spaces or passageways, or even when navigating close to the edge of a table (Cowie et al., 2012; Gomez-Jordana et al., 2018; Matar et al., 2019). BNP has previously been framed as a perceptual or visuomotor disturbance (Almeida and Lebold, 2010; Cowie et al., 2012; Sidaway et al., 2018).
For an allocentric explanation of BNP, boundary vector cells (BVC)—the ontogenetically oldest allocentric cells (Bicanski and Burgess, 2020)—have to be introduced. BVCs not only provide the intrinsic allocentric framework for native GC metric formation, but also support the continuous stabilization and error correction of GCs (Lever et al., 2009; Bjerknes et al., 2014; Hartley and Lever, 2014; Hardcastle et al., 2015; Stensola et al., 2015; Giocomo, 2016; Stensola and Moser, 2016; Savelli et al., 2017). This is important because when an animal enters a non-familiar environment, it must instantly self-organize a new grid pattern (Hafting et al., 2005; Fuhs and Touretzky, 2006; Barry et al., 2012; Giocomo, 2016; Nadasdy et al., 2017; Stangl et al., 2018) dependent on external landmarks and borders, with the GC system exhibiting the greatest flexibility (Barry et al., 2007; Langston et al., 2010; Wills et al., 2010; Stensola et al., 2012; Krupic et al., 2015; Latuske et al., 2015; Dunn et al., 2017; Ismakov et al., 2017; Keinath et al., 2018). BVC cells respond at specific distances and angles from between one and four boundaries, albeit with gaps between them (Barry et al., 2006; Savelli et al., 2008; Solstad et al., 2008; Lever et al., 2009; Stewart et al., 2014; Bicanski and Burgess, 2020). Especially in the mEC, there are border cells (BC) (Solstad et al., 2008) that respond to proximate boundaries (within “whisker’s range”) that immediately block an animal’s path (Hoydal et al., 2019). There are also retrosplenial BCs linked to the mEC that fire “prospective to the animal’s next motion” (van Wijngaarden et al., 2020).
If these cells are disinhibited by deteriorating GCs (Krupic et al., 2015), they virtually generate a stop/freeze signal when standing close to a border. This happens not only in response to borders, but also to doorways enclosed by edges because grid fields are inherently distorted at the edges of the environment (Stensola et al., 2015; Hagglund et al., 2019) bringing BVC and BC to the fore (see Figure 5).
Another potential trigger for the BNP could be the requirement to encode the geometric layout of the subsequent room when leaving the room through a doorway (see Figure 5C). This task may be skipped due to the instability of grid field computations across connected enclosures’ borders (Derdikman et al., 2009; Carpenter et al., 2015; Krupic et al., 2016; He and Brown, 2019). This instability is further compounded by the novelty beyond the bottleneck, which again enlarges and dysregulates grid fields, leading to a brief reduction in spatial stability (Hafting et al., 2005) even in healthy subjects (Figures 5A,B).
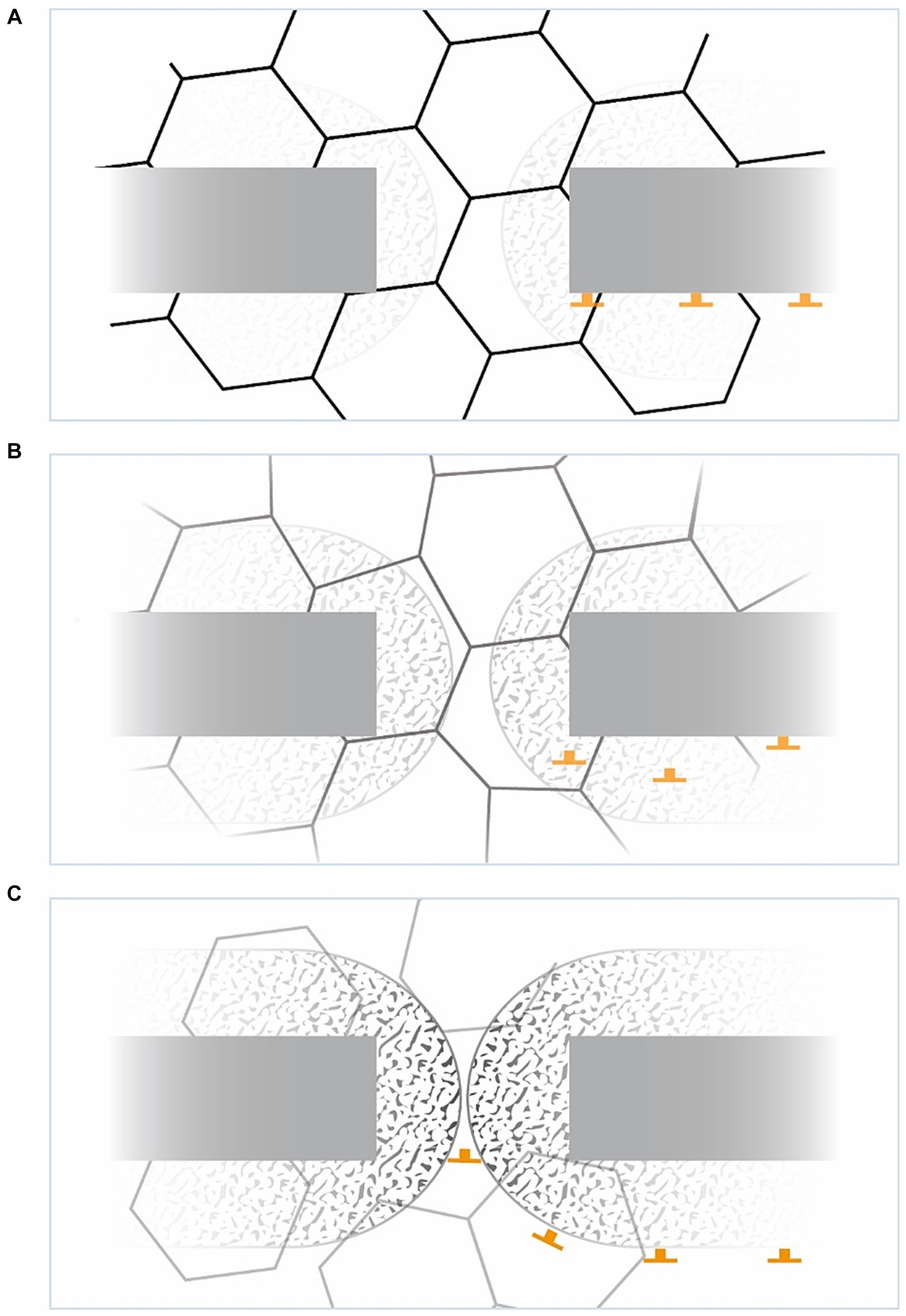
Figure 5. The bottleneck phenomenon from an allocentric view. (A) Crossing a doorway, in familiar spaces the area is tessellated with potent grid fields. Grid cells share a border at about 7.5° (Stensola et al., 2015). The area of BVCs (speckled pattern) and border cells (BCs) is shown, the latter with their immediate stop signals (inverted T). (B) In weakened GCs, their virtual fields become less pronounced, less structured, but deformed, the BVCs becoming detached from the background and the BCs disinhibited. (C) With strengthened BVCs and pushing BCs, movement can be immediately halted virtually, freezing the individuum in space. Note the incremental loss of grid field strength beyond the bottleneck (see text above).
Resting tremor (tremor-at-rest)
Resting tremor (RT), which affects about three-quarters of PD patients, is characterized by an agonist–antagonist motor action in an alert resting position with a frequency of about 4–6 Hz (Milanov, 2001; Hallett, 2012; Zach et al., 2015; Bhatia et al., 2018). Often manifesting a pill-roll component in the distal part of the limb, it is a highly specific sign of idiopathic PD. However, its origin, particularly in relation to the BG, “remains a mystery” (Obeso et al., 2014, p. 524); (Deuschl et al., 2000; Hallett, 2012). Above all, no central pacemakers have been found for PD tremor; instead, there are only cerebral “followers” (Zirh et al., 1998; Hallett, 2014). The independent oscillations of tremulous limbs suggest that individual body parts or even single muscles may each have separate tremor generators (O'Suilleabhain and Matsumoto, 1998; Hurtado et al., 2000; Raethjen et al., 2000; Hallett, 2012; Pedrosa et al., 2012).
To explore potential routes, a resting animal or limb needs reliable information about its actual position (Bush et al., 2015; Olafsdottir et al., 2018). If this information is disrupted, such as by the (egocentric) fusimotor disruption, discussed below, or any other kind of computational spatial feedback and if the allocentric brain is unable to store this information during rest due to DD, the GCs’ continuous dynamic error correction system, which again depends on ongoing movement, could lead the spatially related distal limb to seek spatial information through a (searching) movement. This could be exacerbated by unstable egocentric information arising from enlarged striatal SBP, and more so by detached feedback from the hippocampal place cells for GCs’ forward planning during immobility (Olafsdottir et al., 2016, 2017; Zhang and Liu, 2023) or even self-reliant replay (O'Neill et al., 2017).
The antagonistic rhythmicity of tremor could result from striking the virtual edge of the enlarged SBPs, as per the fusimotor “resonance hypothesis” associated with desynchronized long-loop reflexes in PD (Eklund et al., 1982; Zirh et al., 1998). Conversely, it could arise from attaining sufficient positional information and then being deflected from the edges of the associated grid field, possibly in a looser relationship with the LFP. Both peripheral perspectives support the concept of separate body part tremor generation and brain tremor hubs merely acting as “followers.” With the loss of GCs’ stable circular hexagonality, limbs—particularly distal ones responsible for interacting with the proximate, allocentric computed world—may be deflected in their positional scanning, achieving the rotating “pill roll” component.
Rigidity
Rigidity, a cardinal feature of PD, is present in up to 90% of cases and serves as a primary component of the assessment of dopamine and surgical PD treatment (Andrews and Burke, 1973; Mutch et al., 1986; Powell et al., 2012; Postuma et al., 2015; Powell et al., 2016). Parkinsonian rigidity is characterized by an intrinsically increased muscle tone with clinically uniform resistance to externally imposed joint movement in antagonist muscles throughout the range of motion. Abnormal responses to muscle stretch and long-loop latencies/reflexes have been discussed (Berardelli et al., 1983; Delwaide and Schoenen, 1985; Xia et al., 2011; Pasquereau et al., 2016; Powell et al., 2016).
Alternatively, one could revisit the inconsistent computation of SBP, the “conceptual hypometria” signaling a position beyond the real one, decelerating further movement. That would provide an allocentric explanation for the central proprioceptive disturbances, which are often posited as the actual pathogenesis of PD (Abbruzzese and Berardelli, 2003; Contreras-Vidal and Gold, 2004; Jacobs and Horak, 2006; Fiorio et al., 2007; Rand et al., 2010; Lee et al., 2013). In addition there is also peripheral fusimotor activity of the muscle spindle (Radovanovic et al., 2015), the afferent spinal dorsal horn (Skoog and Noga, 1995; Garraway and Hochman, 2001; Milla-Cruz et al., 2020), and its efferent paths along the ventral horn (Yoshida and Tanaka, 1988; Weil-Fugazza and Godefroy, 1993; Barriere et al., 2004; Han et al., 2007; Zhu et al., 2007; Schwarz and Peever, 2011; Clemens et al., 2012; Sharples, 2017; Rivera-Oliver et al., 2019), all of which are disturbed in DD. This apparatus is controlled by the CNS (Ellaway et al., 2015; Macefield and Knellwolf, 2018), with fusimotor activity operating as a “forward sensory model” (Dimitriou and Edin, 2010). Without this—or a determined virtual position to cipher forward motion (Bush et al., 2015; Olafsdottir et al., 2018)—its continuous recalculation could drive the system toward computational overcompensation, resulting in rigidity.
Often, passive turning of an extremity yields cogwheel-like jerks commonly referred to as “cogwheel” rigidity (Ghiglione et al., 2005). This rhythmic unclenching from rigidity may be due to the enlarged and consequently virtually unsubsumable SBPs or their disturbed allocentric link, causing the rigid calibration of tested body parts to break apart and slip into the unknown. The rhythmicity of the “cogwheel” could emerge based on the extent of the virtual egocentric or even ego-allocentric intangible dimensions, or the time delay for allocentric adjustment via the striato-HF/EC loop with a delayed position signal in GCs would prematurely hit the LFP, eliciting a spatial rebound signal and again forcing rigidity. This aligns with the notion of a spatial threshold (ST), the point at which the stretch reflexes and other proprioceptive reflexes activate, modulated by the “corticospinal set” which has been observed to be either hypo-sensitive or even inversely sensitive in PD (Mullick et al., 2013).
L-dopa induced dyskinesia
“Dyskinesia are involuntary hyperkinetic movements presenting mostly as chorea or choreoathetoid form, but rare ballistic, dystonic or stereotypical variants have been described as well” (McFarthing et al., 2019, p. 449). While these movements develop as a function of disease duration, dopaminergic treatment significantly escalates the probability of their occurrence (Katzenschlager et al., 2008; Nadjar et al., 2009; Nutt et al., 2010; Cilia et al., 2014; Espay et al., 2018; Ryan et al., 2018), largely contingent on plasma L-dopa concentrations. However, LID presents a key paradox in the box-and-arrow model: although therapeutic inactivation of the GPi is thought to trigger dyskinesia, it also alleviates it (Inase et al., 1996; Brown and Marsden, 1998; Desmurget and Turner, 2008; Nambu et al., 2015). LID-associated abnormal neuronal activity has been detected not only in the striatum, but also in the primary somatosensory (Alam et al., 2017) and the primary motor cortex (Halje et al., 2012; Swann et al., 2016).
Reflecting the up to 16-fold enlarged, clustered, fragmented, and at least partially overlapping striatal single body parts (SBP) in PD, along with satellite potentials within remote somatotopic clusters (Cho et al., 2002) and with “neurons that were previously deemed ‘unrelated’ [to movement and that] might now demonstrate movement-related activity” (Bronfeld and Bar-Gad, 2011), LID could signify a disruption of established sensorimotor pathways. Such disruptions disturb their spatial and, therefore, choreographic chronology, promoting chaotic, possibly bizarre movements, deviating from the well-trodden path amplified by a dopamine surplus. In this “unleashed SBP theory,” hyperkinesia in LID is no longer interpreted as an acceleration of movement per se, but rather as a secondary effect of chaotic or unmanaged somatotopic displacement activity. This theory underscores that “dyskinesia” and “hyperkinesia” in PD are not merely the antithesis of being “hypokinetic,” but rather a by-product or the other side of the parkinsonian spatial coin. Therefore, the therapeutic effect of GPi-DBS on LID would not be surprising.
Broadening the perspective to the allocentric brain, the SBP could be unleashed from the otherwise stabilizing or balancing mEC due to a dopamine surplus suppressing the mEC (Mayne et al., 2013; Jin et al., 2019) or the LC’s dyskinesia-limiting possibilities (Cedarbaum and Aghajanian, 1977; Miguelez et al., 2011) (see Figure 6). Apart from peak dose dyskinesia, there is lower body predominant diphasic dyskinesia (Manson et al., 2012; Verhagen Metman and Espay, 2017; Espay et al., 2018) occurring just below the therapeutic L-Dopa level. This could meet the criteria for being below the LC’s dyskinesia-limiting possibilities (Miguelez et al., 2011) as well (see Figure 6).
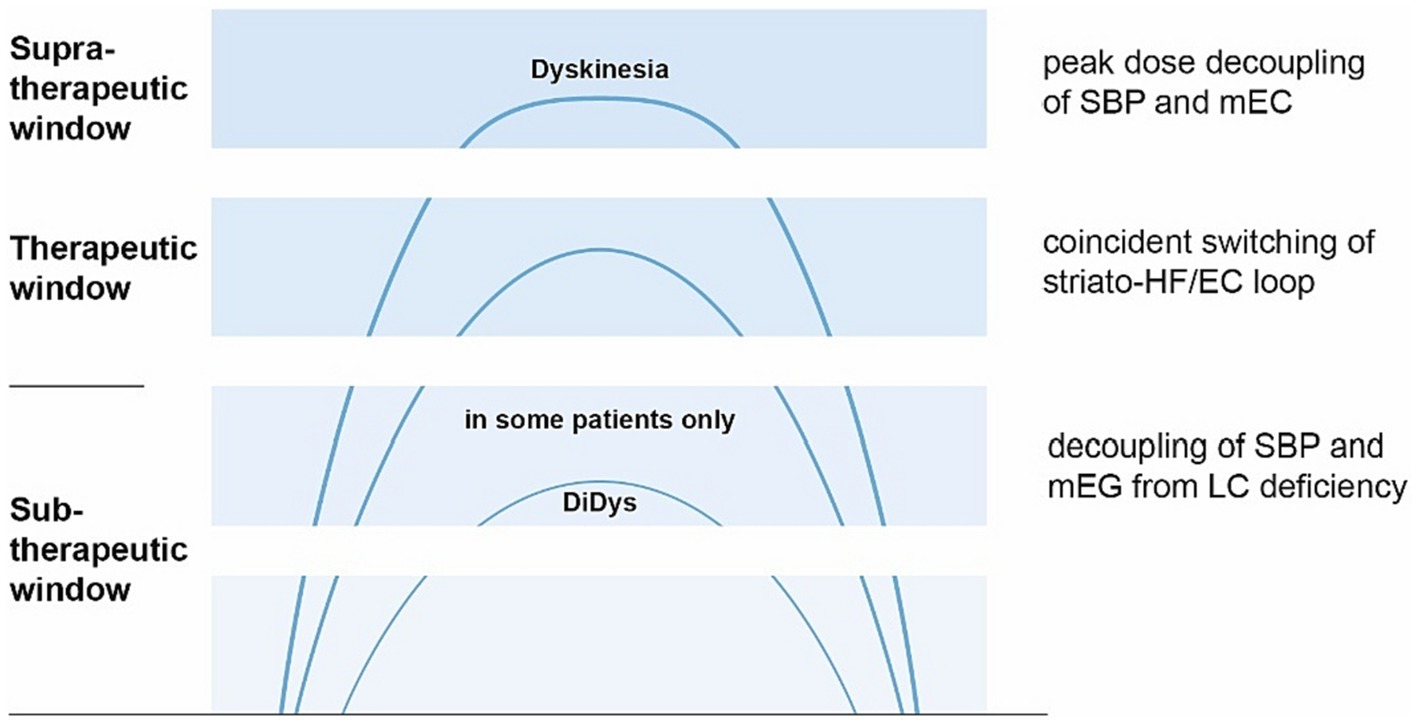
Figure 6. Concept of dyskinesia driven from allocentric central hubs. The x-axis represents the time after L-dopa intake, the y-axis L-dopa concentration (image based on Espay; Espay et al., 2018). Explanation is shown on the right (top down): Peak-dose dyskinesia arises from the unleashed SBP, decoupled from the ordinarily suppressed mEC (Mayne et al., 2013) from a dopamine surplus. In the therapeutic window, mEC and striatum are balanced by the locus coeruleus (LC) switching the striato-HF/EC loop. In some patients, diphasic dyskinesia (DiDys) occur if LC’s dyskinesia-limiting possibilities (Cedarbaum and Aghajanian, 1977; Miguelez et al., 2011) are inactive, probably due to a lack of dopamine.
Limitations
Aside from the hypotheses mentioned, there is, to the best of my knowledge, a dearth of literature discussing the potential role of mEC’s GCs in Parkinson’s disease. Recently, two papers stressed the early involvement of the allocentric brain in PD (Fernandez-Baizan et al., 2020), particularly the mEC (Pieperhoff et al., 2022). Furthermore, evidence suggesting the involvement of EC layer II in parkinsonian symptoms in postencephalitic patients is scant (Hof et al., 1992).
Allocentric “studies have mainly been conducted in simple laboratory settings in which animals explore small, two-dimensional (i.e., flat) arenas” so that “data on the issue of grid cell encoding in 3D are scarce” (Jeffery et al., 2015; Casali et al., 2019; Grieves et al., 2021; Xu et al., 2022). The existence of 3D GCs has been demonstrated in flying bats (Yartsev and Ulanovsky, 2013; Ginosar et al., 2021), and others have identified preliminary 3D grid codes at least in the left human entorhinal cortex (Kim and Maguire, 2019). This study presumes not only the three-dimensionality of GCs and their continuous interactions with striatal SBP, but also its influence on limbs acting in the proximal space liaising the egocentric and allocentric world. However, in the typical allocentric laboratory experiment, foraging—which often concludes with grabbing using the paw or picking up with the snout—demonstrates how the most distant organs complete the link between allocentric guidance and the egocentric world (Heath et al., 2007; Neely et al., 2008). Although allocentric research has already shown hippocampal theta activity accompanying isolated limb movements (Vanderwolf, 1969), there is a gap in the further exploration of this topic.
The hypothesis of translating allocentric whole-body computation to that of the distal body parts involved in goal-directed movements remains largely untested. The extent to which allocentric cells, responsible for completing tasks egocentrically, are present among the many unclassified cells in the mEC remains unknown (Diehl et al., 2017; Miao et al., 2017).
Conclusion
This paper hypothesizes and illustrates the intriguing association between allocentric properties and PD motor and secondary, spatially related, symptoms in dopamine depletion, with several examples cited throughout. The common thread among these hypotheses is the ambition to surpass the constraints of the box-and-arrow model and the narrow scope of basal ganglia-centric perspectives in PD. Much like other prevailing PD models, these have reached “the point where (their) total rejection, rather than continual attempts at (their) modification, is necessary” (Montgomery, 2011, p. 14). The compelling notion that the allocentric brain influences PD motor symptoms has the potential to substantially shape not only research into movement and movement disorders, but also the broader field of neuroscience.
Data availability statement
The original contributions presented in the study are included in the article/supplementary material; further inquiries can be directed to the corresponding author.
Author contributions
AR: Writing – original draft.
Funding
The author declares that no financial support was received for the research, authorship, and/or publication of this article.
Conflict of interest
The author declares that the research was conducted in the absence of any commercial or financial relationships that could be construed as a potential conflict of interest.
Publisher’s note
All claims expressed in this article are solely those of the authors and do not necessarily represent those of their affiliated organizations, or those of the publisher, the editors and the reviewers. Any product that may be evaluated in this article, or claim that may be made by its manufacturer, is not guaranteed or endorsed by the publisher.
References
Abbruzzese, G., and Berardelli, A. (2003). Sensorimotor integration in movement disorders. Mov. Disord. 18, 231–240. doi: 10.1002/mds.10327
Agostino, R., Berardelli, A., Formica, A., Accornero, N., and Manfredi, M. (1992). Sequential arm movements in patients with Parkinson's disease, Huntington's disease and dystonia. Brain 115, 1481–1495. doi: 10.1093/brain/115.5.1481
Akil, M., and Lewis, D. A. (1993). The dopaminergic innervation of monkey entorhinal cortex. Cereb. Cortex 3, 533–550. doi: 10.1093/cercor/3.6.533
Alam, M., Rumpel, R., Jin, X., von Wrangel, C., Tschirner, S. K., Krauss, J. K., et al. (2017). Altered somatosensory cortex neuronal activity in a rat model of Parkinson's disease and levodopa-induced dyskinesias. Exp. Neurol. 294, 19–31. doi: 10.1016/j.expneurol.2017.04.011
Albin, R. L., Young, A. B., and Penney, J. B. (1989). The functional anatomy of basal ganglia disorders. Trends Neurosci. 12, 366–375. doi: 10.1016/0166-2236(89)90074-X
Alexander, G. E., and DeLong, M. R. (1985). Microstimulation of the primate neostriatum. II. Somatotopic organization of striatal microexcitable zones and their relation to neuronal response properties. J. Neurophysiol. 53, 1417–1430. doi: 10.1152/jn.1985.53.6.1417
Alexander, G. E., DeLong, M. R., and Strick, P. L. (1986). Parallel organization of functionally segregated circuits linking basal ganglia and cortex. Annu. Rev. Neurosci. 9, 357–381. doi: 10.1146/annurev.ne.09.030186.002041
Alexander, A. S., Robinson, J. C., Dannenberg, H., Kinsky, N. R., Levy, S. J., Mau, W., et al. (2020). Neurophysiological coding of space and time in the hippocampus, entorhinal cortex, and retrosplenial cortex. Brain Neurosci. Adv. 4:2398212820972871. doi: 10.1177/2398212820972871
Almeida, Q. J., and Lebold, C. A. (2010). Freezing of gait in Parkinson's disease: a perceptual cause for a motor impairment? J. Neurol. Neurosurg. Psychiatry 81, 513–518. doi: 10.1136/jnnp.2008.160580
Andersson, S. O., Moser, E. I., and Moser, M. B. (2021). Visual stimulus features that elicit activity in object-vector cells. Commun. Biol. 4:1219. doi: 10.1038/s42003-021-02727-5
Andrews, C. J., and Burke, D. (1973). Quantitative study of the effect of L-dopa and phenoxybenzamine on the rigidity of Parkinson's disease. J. Neurol. Neurosurg. Psychiatry 36, 321–328. doi: 10.1136/jnnp.36.3.321
Aston-Jones, G., and Bloom, F. E. (1981). Activity of norepinephrine-containing locus coeruleus neurons in behaving rats anticipates fluctuations in the sleep-waking cycle. J. Neurosci. 1, 876–886. doi: 10.1523/JNEUROSCI.01-08-00876.1981
Bant, J. S., Hardcastle, K., Ocko, S. A., and Giocomo, L. M. (2020). Topography in the bursting dynamics of entorhinal neurons. Cell Rep. 30, 2349–2359. doi: 10.1016/j.celrep.2020.01.057
Barrett, A. M., Crucian, G. P., Schwartz, R., Nallamshetty, H., and Heilman, K. M. (2001). Seeing trees but not the forest: limited perception of large configurations in PD. Neurology 56, 724–729. doi: 10.1212/wnl.56.6.724
Barriere, G., Mellen, N., and Cazalets, J. R. (2004). Neuromodulation of the locomotor network by dopamine in the isolated spinal cord of newborn rat. Eur. J. Neurosci. 19, 1325–1335. doi: 10.1111/j.1460-9568.2004.03210.x
Barry, C., and Burgess, N. (2014). Neural mechanisms of self-location. Curr. Biol. 24, R330–R339. doi: 10.1016/j.cub.2014.02.049
Barry, C., Ginzberg, L. L., O'Keefe, J., and Burgess, N. (2012). Grid cell firing patterns signal environmental novelty by expansion. Proc. Natl. Acad. Sci. U.S.A. 109, 17687–17692. doi: 10.1073/pnas.1209918109
Barry, C., Hayman, R., Burgess, N., and Jeffery, K. J. (2007). Experience-dependent rescaling of entorhinal grids. Nat. Neurosci. 10, 682–684. doi: 10.1038/nn1905
Barry, C., Lever, C., Hayman, R., Hartley, T., Burton, S., O'Keefe, J., et al. (2006). The boundary vector cell model of place cell firing and spatial memory. Rev. Neurosci. 17, 71–97. doi: 10.1515/REVNEURO.2006.17.1-2.71
Benecke, R., Rothwell, J. C., Dick, J. P., Day, B. L., and Marsden, C. D. (1987). Disturbance of sequential movements in patients with Parkinson's disease. Brain 110, 361–379. doi: 10.1093/brain/110.2.361
Berardelli, A., Rothwell, J. C., Thompson, P. D., and Hallett, M. (2001). Pathophysiology of bradykinesia in Parkinson's disease. Brain 124, 2131–2146. doi: 10.1093/brain/124.11.2131
Berardelli, A., Sabra, A. F., and Hallett, M. (1983). Physiological mechanisms of rigidity in Parkinson's disease. J. Neurol. Neurosurg. Psychiatry 46, 45–53. doi: 10.1136/jnnp.46.1.45
Bernardinis, M., Atashzar, S. F., Jog, M., and Patel, R. V. (2018). Visual displacement perception in Parkinson's disease analyzed using a computer-generated graphical tool. Conf. Proc. IEEE Eng. Med. Biol. Soc. 2018, 2748–2751. doi: 10.1109/EMBC.2018.8512754
Bernheimer, H., and Hornykiewicz, O. (1965). Decreased homovanillic acid concentration in the brain in parkinsonian subjects as an expression of a disorder of central dopamine metabolism. Klin. Wochenschr. 43, 711–715. doi: 10.1007/BF01707066
Bhatia, K. P., Bain, P., Bajaj, N., Elble, R. J., Hallett, M., Louis, E. D., et al. (2018). Consensus statement on the classification of tremors. From the task force on tremor of the International Parkinson and Movement Disorder Society. Mov. Disord. 33, 75–87. doi: 10.1002/mds.27121
Bicanski, A., and Burgess, N. (2020). Neuronal vector coding in spatial cognition. Nat. Rev. Neurosci. 21, 453–470. doi: 10.1038/s41583-020-0336-9
Birkmayer, W., and Hornykiewicz, O. (1961). The L-3, 4-dioxyphenylalanine (DOPA)-effect in Parkinson-akinesia. Wien. Klin. Wochenschr. 73, 787–788.
Bjerknes, T. L., Moser, E. I., and Moser, M. B. (2014). Representation of geometric borders in the developing rat. Neuron 82, 71–78. doi: 10.1016/j.neuron.2014.02.014
Boccara, C. N., Sargolini, F., Thoresen, V. H., Solstad, T., Witter, M. P., Moser, E. I., et al. (2010). Grid cells in pre- and parasubiculum. Nat. Neurosci. 13, 987–994. doi: 10.1038/nn.2602
Bogerts, B., Hantsch, J., and Herzer, M. (1983). A morphometric study of the dopamine-containing cell groups in the mesencephalon of normals, Parkinson patients, and schizophrenics. Biol. Psychiatry 18, 951–969.
Bohbot, V. D., Iaria, G., and Petrides, M. (2004). Hippocampal function and spatial memory: evidence from functional neuroimaging in healthy participants and performance of patients with medial temporal lobe resections. Neuropsychology 18, 418–425. doi: 10.1037/0894-4105.18.3.418
Bologna, M., Leodori, G., Stirpe, P., Paparella, G., Colella, D., Belvisi, D., et al. (2016). Bradykinesia in early and advanced Parkinson's disease. J. Neurol. Sci. 369, 286–291. doi: 10.1016/j.jns.2016.08.028
Bologna, M., Paparella, G., Fasano, A., Hallett, M., and Berardelli, A. (2020). Evolving concepts on bradykinesia. Brain 143, 727–750. doi: 10.1093/brain/awz344
Bortz, D. M., and Grace, A. A. (2018). Medial septum differentially regulates dopamine neuron activity in the rat ventral tegmental area and substantia nigra via distinct pathways. Neuropsychopharmacology 43, 2093–2100. doi: 10.1038/s41386-018-0048-2
Bouret, S., and Sara, S. J. (2005). Network reset: a simplified overarching theory of locus coeruleus noradrenaline function. Trends Neurosci. 28, 574–582. doi: 10.1016/j.tins.2005.09.002
Braak, H., and Del Tredici, K. (2017). Neuropathological staging of brain pathology in sporadic Parkinson's disease: separating the wheat from the chaff. J. Parkinsons Dis. 7, S71–S85. doi: 10.3233/JPD-179001
Braak, H., Del Tredici, K., Rub, U., de Vos, R. A., Jansen Steur, E. N., and Braak, E. (2003). Staging of brain pathology related to sporadic Parkinson's disease. Neurobiol. Aging 24, 197–211. doi: 10.1016/s0197-4580(02)00065-9
Brandon, M. P., Bogaard, A. R., Libby, C. P., Connerney, M. A., Gupta, K., and Hasselmo, M. E. (2011). Reduction of theta rhythm dissociates grid cell spatial periodicity from directional tuning. Science 332, 595–599. doi: 10.1126/science.1201652
Bronfeld, M., and Bar-Gad, I. (2011). Loss of specificity in basal ganglia related movement disorders. Front. Syst. Neurosci. 5:38. doi: 10.3389/fnsys.2011.00038
Brown, P., and Marsden, C. D. (1998). What do the basal ganglia do? Lancet 351, 1801–1804. doi: 10.1016/S0140-6736(97)11225-9
Brun, V. H., Solstad, T., Kjelstrup, K. B., Fyhn, M., Witter, M. P., Moser, E. I., et al. (2008). Progressive increase in grid scale from dorsal to ventral medial entorhinal cortex. Hippocampus 18, 1200–1212. doi: 10.1002/hipo.20504
Bryant, M. S., Rintala, D. H., Lai, E. C., and Protas, E. J. (2010). An investigation of two interventions for micrographia in individuals with Parkinson's disease. Clin. Rehabil. 24, 1021–1026. doi: 10.1177/0269215510371420
Buetfering, C., Allen, K., and Monyer, H. (2014). Parvalbumin interneurons provide grid cell-driven recurrent inhibition in the medial entorhinal cortex. Nat. Neurosci. 17, 710–718. doi: 10.1038/nn.3696
Burak, Y. (2014). Spatial coding and attractor dynamics of grid cells in the entorhinal cortex. Curr. Opin. Neurobiol. 25, 169–175. doi: 10.1016/j.conb.2014.01.013
Burgess, N. (2006). Spatial memory: how egocentric and allocentric combine. Trends Cogn. Sci. 10, 551–557. doi: 10.1016/j.tics.2006.10.005
Burgess, N. (2008). Grid cells and theta as oscillatory interference: theory and predictions. Hippocampus 18, 1157–1174. doi: 10.1002/hipo.20518
Burgess, N., Barry, C., and O'Keefe, J. (2007). An oscillatory interference model of grid cell firing. Hippocampus 17, 801–812. doi: 10.1002/hipo.20327
Burleigh-Jacobs, A., Horak, F. B., Nutt, J. G., and Obeso, J. A. (1997). Step initiation in Parkinson's disease: influence of levodopa and external sensory triggers. Mov. Disord. 12, 206–215. doi: 10.1002/mds.870120211
Burwell, R. D., and Amaral, D. G. (1998). Cortical afferents of the perirhinal, postrhinal, and entorhinal cortices of the rat. J. Comp. Neurol. 398, 179–205. doi: 10.1002/(sici)1096-9861(19980824)398:2<179::aid-cne3>3.0.co;2-y
Bush, D., Barry, C., Manson, D., and Burgess, N. (2015). Using grid cells for navigation. Neuron 87, 507–520. doi: 10.1016/j.neuron.2015.07.006
Caggiano, V., Leiras, R., Goni-Erro, H., Masini, D., Bellardita, C., Bouvier, J., et al. (2018). Midbrain circuits that set locomotor speed and gait selection. Nature 553, 455–460. doi: 10.1038/nature25448
Caminiti, S. P., Presotto, L., Baroncini, D., Garibotto, V., Moresco, R. M., Gianolli, L., et al. (2017). Axonal damage and loss of connectivity in nigrostriatal and mesolimbic dopamine pathways in early Parkinson's disease. Neuroimage Clin 14, 734–740. doi: 10.1016/j.nicl.2017.03.011
Campbell, M. G., Ocko, S. A., Mallory, C. S., Low, I. I. C., Ganguli, S., and Giocomo, L. M. (2018). Principles governing the integration of landmark and self-motion cues in entorhinal cortical codes for navigation. Nat. Neurosci. 21, 1096–1106. doi: 10.1038/s41593-018-0189-y
Carelli, R. M., and West, M. O. (1991). Representation of the body by single neurons in the dorsolateral striatum of the awake, unrestrained rat. J. Comp. Neurol. 309, 231–249. doi: 10.1002/cne.903090205
Carlsson, A. (1971). Basic concepts underlying recent developments in the field of Parkinson's disease. Contemp. Neurol. Ser. 8, 1–31.
Carlsson, A., Lindqvist, M., and Magnusson, T. (1957). 3, 4-Dihydroxyphenylalanine and 5-hydroxytryptophan as reserpine antagonists. Nature 180:1200. doi: 10.1038/1801200a0
Carlsson, A., and Waldeck, B. (1958). A fluorimetric method for the determination of dopamine (3-hydroxytyramine). Acta Physiol. Scand. 44, 293–298. doi: 10.1111/j.1748-1716.1958.tb01628.x
Carpenter, F., and Barry, C. (2016). Distorted grids as a spatial label and metric. Trends Cogn. Sci. 20, 164–167. doi: 10.1016/j.tics.2015.12.004
Carpenter, F., Manson, D., Jeffery, K., Burgess, N., and Barry, C. (2015). Grid cells form a global representation of connected environments. Curr. Biol. 25, 1176–1182. doi: 10.1016/j.cub.2015.02.037
Carvalho, M. M., Tanke, N., Kropff, E., Witter, M. P., Moser, M. B., and Moser, E. I. (2020). A brainstem locomotor circuit drives the activity of speed cells in the medial entorhinal cortex. Cell Rep. 32:108123. doi: 10.1016/j.celrep.2020.108123
Casali, G., Bush, D., and Jeffery, K. (2019). Altered neural odometry in the vertical dimension. Proc. Natl. Acad. Sci. USA 116, 4631–4636. doi: 10.1073/pnas.1811867116
Casali, G., Shipley, S., Dowell, C., Hayman, R., and Barry, C. (2018). Entorhinal neurons exhibit Cue locking in rodent VR. Front. Cell. Neurosci. 12:512. doi: 10.3389/fncel.2018.00512
Cedarbaum, J. M., and Aghajanian, G. K. (1977). Catecholamine receptors on locus coeruleus neurons: pharmacological characterization. Eur. J. Pharmacol. 44, 375–385. doi: 10.1016/0014-2999(77)90312-0
Chen, G., King, J. A., Burgess, N., and O'Keefe, J. (2013). How vision and movement combine in the hippocampal place code. Proc. Natl. Acad. Sci. USA 110, 378–383. doi: 10.1073/pnas.1215834110
Chen, G., Manson, D., Cacucci, F., and Wills, T. J. (2016). Absence of visual input results in the disruption of grid cell firing in the mouse. Curr. Biol. 26, 2335–2342. doi: 10.1016/j.cub.2016.06.043
Chersi, F., and Burgess, N. (2015). The cognitive architecture of spatial navigation: hippocampal and striatal contributions. Neuron 88, 64–77. doi: 10.1016/j.neuron.2015.09.021
Cho, J., Duke, D., Manzino, L., Sonsalla, P. K., and West, M. O. (2002). Dopamine depletion causes fragmented clustering of neurons in the sensorimotor striatum: evidence of lasting reorganization of corticostriatal input. J. Comp. Neurol. 452, 24–37. doi: 10.1002/cne.10349
Cilia, R., Akpalu, A., Sarfo, F. S., Cham, M., Amboni, M., Cereda, E., et al. (2014). The modern pre-levodopa era of Parkinson's disease: insights into motor complications from sub-Saharan Africa. Brain 137, 2731–2742. doi: 10.1093/brain/awu195
Clemens, S., Belin-Rauscent, A., Simmers, J., and Combes, D. (2012). Opposing modulatory effects of D1- and D2-like receptor activation on a spinal central pattern generator. J. Neurophysiol. 107, 2250–2259. doi: 10.1152/jn.00366.2011
Climer, J. R., Newman, E. L., and Hasselmo, M. E. (2013). Phase coding by grid cells in unconstrained environments: two-dimensional phase precession. Eur. J. Neurosci. 38, 2526–2541. doi: 10.1111/ejn.12256
Coffey, K. R., Nader, M., Bawa, J., and West, M. O. (2017). Homogeneous processing in the striatal direct and indirect pathways: single body part sensitive type IIb neurons may express either dopamine receptor D1 or D2. Eur. J. Neurosci. 46, 2380–2391. doi: 10.1111/ejn.13690
Coffey, K. R., Nader, M., and West, M. O. (2016). Single body parts are processed by individual neurons in the mouse dorsolateral striatum. Brain Res. 1636, 200–207. doi: 10.1016/j.brainres.2016.01.031
Colombo, D., Serino, S., Tuena, C., Pedroli, E., Dakanalis, A., Cipresso, P., et al. (2017). Egocentric and allocentric spatial reference frames in aging: a systematic review. Neurosci. Biobehav. Rev. 80, 605–621. doi: 10.1016/j.neubiorev.2017.07.012
Contreras-Vidal, J. L., and Gold, D. R. (2004). Dynamic estimation of hand position is abnormal in Parkinson's disease. Parkinsonism Relat. Disord. 10, 501–506. doi: 10.1016/j.parkreldis.2004.06.002
Couey, J. J., Witoelar, A., Zhang, S. J., Zheng, K., Ye, J., Dunn, B., et al. (2013). Recurrent inhibitory circuitry as a mechanism for grid formation. Nat. Neurosci. 16, 318–324. doi: 10.1038/nn.3310
Cowie, D., Limousin, P., Peters, A., Hariz, M., and Day, B. L. (2012). Doorway-provoked freezing of gait in Parkinson's disease. Mov. Disord. 27, 492–499. doi: 10.1002/mds.23990
Craig, C. E., Jenkinson, N. J., Brittain, J. S., Grothe, M. J., Rochester, L., Silverdale, M., et al. (2020). Pedunculopontine nucleus microstructure predicts postural and gait symptoms in Parkinson's disease. Mov. Disord. 35, 1199–1207. doi: 10.1002/mds.28051
Crutcher, M. D., and DeLong, M. R. (1984). Single cell studies of the primate putamen. II. Relations to direction of movement and pattern of muscular activity. Exp. Brain Res. 53, 244–258. doi: 10.1007/BF00238154
Dannenberg, H., Lazaro, H., Nambiar, P., Hoyland, A., and Hasselmo, M. E. (2020). Effects of visual inputs on neural dynamics for coding of location and running speed in medial entorhinal cortex. elife 9, 1–34. doi: 10.7554/eLife.62500
Davidsdottir, S., Wagenaar, R., Young, D., and Cronin-Golomb, A. (2008). Impact of optic flow perception and egocentric coordinates on veering in Parkinson's disease. Brain 131, 2882–2893. doi: 10.1093/brain/awn237
DeLong, M. R. (1990). Primate models of movement disorders of basal ganglia origin. Trends Neurosci. 13, 281–285. doi: 10.1016/0166-2236(90)90110-V
Delval, A., Moreau, C., Bleuse, S., Tard, C., Ryckewaert, G., Devos, D., et al. (2014). Auditory cueing of gait initiation in Parkinson's disease patients with freezing of gait. Clin. Neurophysiol. 125, 1675–1681. doi: 10.1016/j.clinph.2013.12.101
Delwaide, P. J., and Schoenen, J. (1985). Clinical neurophysiology in the evaluation and physiopathology of Parkinson's disease. Rev. Neurol. (Paris) 141, 759–773.
Demirci, M., Grill, S., McShane, L., and Hallett, M. (1997). A mismatch between kinesthetic and visual perception in Parkinson's disease. Ann. Neurol. 41, 781–788. doi: 10.1002/ana.410410614
Derdikman, D., Whitlock, J. R., Tsao, A., Fyhn, M., Hafting, T., Moser, M. B., et al. (2009). Fragmentation of grid cell maps in a multicompartment environment. Nat. Neurosci. 12, 1325–1332. doi: 10.1038/nn.2396
Deshmukh, S. S., Yoganarasimha, D., Voicu, H., and Knierim, J. J. (2010). Theta modulation in the medial and the lateral entorhinal cortices. J. Neurophysiol. 104, 994–1006. doi: 10.1152/jn.01141.2009
Desmurget, M., and Turner, R. S. (2008). Testing basal ganglia motor functions through reversible inactivations in the posterior internal globus pallidus. J. Neurophysiol. 99, 1057–1076. doi: 10.1152/jn.01010.2007
Deuschl, G., Raethjen, J., Baron, R., Lindemann, M., Wilms, H., and Krack, P. (2000). The pathophysiology of parkinsonian tremor: a review. J. Neurol. 247, V33–V48. doi: 10.1007/pl00007781
Devan, B. D., Goad, E. H., and Petri, H. L. (1996). Dissociation of hippocampal and striatal contributions to spatial navigation in the water maze. Neurobiol. Learn. Mem. 66, 305–323. doi: 10.1006/nlme.1996.0072
Devan, B. D., and White, N. M. (1999). Parallel information processing in the dorsal striatum: relation to hippocampal function. J. Neurosci. 19, 2789–2798. doi: 10.1523/JNEUROSCI.19-07-02789.1999
Diehl, G. W., Hon, O. J., Leutgeb, S., and Leutgeb, J. K. (2017). Grid and nongrid cells in medial entorhinal cortex represent spatial location and environmental features with complementary coding schemes. Neuron 94, 83–92.e6. doi: 10.1016/j.neuron.2017.03.004
Dimitriou, M., and Edin, B. B. (2010). Human muscle spindles act as forward sensory models. Curr. Biol. 20, 1763–1767. doi: 10.1016/j.cub.2010.08.049
Distler, M., Schlachetzki, J. C., Kohl, Z., Winkler, J., and Schenk, T. (2016). Paradoxical kinesia in Parkinson's disease revisited: anticipation of temporal constraints is critical. Neuropsychologia 86, 38–44. doi: 10.1016/j.neuropsychologia.2016.04.012
Dunn, B., Wennberg, D., Huang, Z., and Roudi, Y. (2017). Grid cells show field-to-field variability and this explains the aperiodic response of inhibitory interneurons. arXiv [Preprint].
Duysens, J., and Nonnekes, J. (2021). Parkinson's Kinesia Paradoxa is not a paradox. Mov. Disord. 36, 1115–1118. doi: 10.1002/mds.28550
Eggink, H., Mertens, P., Storm, E., and Giocomo, L. M. (2014). Hyperpolarization-activated cyclic nucleotide-gated 1 independent grid cell-phase precession in mice. Hippocampus 24, 249–256. doi: 10.1002/hipo.22231
Ehringer, H., and Hornykiewicz, O. (1960). Distribution of noradrenaline and dopamine (3-hydroxytyramine) in the human brain and their behavior in diseases of the extrapyramidal system. Klin. Wochenschr. 38, 1236–1239. doi: 10.1007/BF01485901
Eichenbaum, H. (2014). Time cells in the hippocampus: a new dimension for mapping memories. Nat. Rev. Neurosci. 15, 732–744. doi: 10.1038/nrn3827
Eklund, G., Hagbarth, K. E., Hagglund, J. V., and Wallin, E. U. (1982). The 'late' reflex responses to muscle stretch: the 'resonance hypothesis' versus the 'long-loop hypothesis'. J. Physiol. 326, 79–90. doi: 10.1113/jphysiol.1982.sp014178
Ellaway, P. H., Taylor, A., and Durbaba, R. (2015). Muscle spindle and fusimotor activity in locomotion. J. Anat. 227, 157–166. doi: 10.1111/joa.12299
Elliott, D., Lyons, J., Hayes, S. J., Burkitt, J. J., Roberts, J. W., Grierson, L. E., et al. (2017). The multiple process model of goal-directed reaching revisited. Neurosci. Biobehav. Rev. 72, 95–110. doi: 10.1016/j.neubiorev.2016.11.016
Espay, A. J., Morgante, F., Merola, A., Fasano, A., Marsili, L., Fox, S. H., et al. (2018). Levodopa-induced dyskinesia in Parkinson disease: current and evolving concepts. Ann. Neurol. 84, 797–811. doi: 10.1002/ana.25364
Fallon, J. H., Koziell, D. A., and Moore, R. Y. (1978). Catecholamine innervation of the basal forebrain. II. Amygdala, suprarhinal cortex and entorhinal cortex. J. Comp. Neurol. 180, 509–531. doi: 10.1002/cne.901800308
Fernandez-Baizan, C., Paula Fernandez, G. M., Diaz-Caceres, E., Menendez-Gonzalez, M., Arias, J. L., and Mendez, M. (2020). Patients with Parkinson's disease show alteration in their visuospatial abilities and in their egocentric and Allocentric spatial orientation measured by card placing tests. J. Parkinsons Dis. 10, 1807–1816. doi: 10.3233/JPD-202122
Finch, D. M., Gigg, J., Tan, A. M., and Kosoyan, O. P. (1995). Neurophysiology and neuropharmacology of projections from entorhinal cortex to striatum in the rat. Brain Res. 670, 233–247. doi: 10.1016/0006-8993(94)01279-q
Fiore, V. G., Rigoli, F., Stenner, M. P., Zaehle, T., Hirth, F., Heinze, H. J., et al. (2016). Changing pattern in the basal ganglia: motor switching under reduced dopaminergic drive. Sci. Rep. 6:23327. doi: 10.1038/srep23327
Fiorio, M., Stanzani, C., Rothwell, J. C., Bhatia, K. P., Moretto, G., Fiaschi, A., et al. (2007). Defective temporal discrimination of passive movements in Parkinson's disease. Neurosci. Lett. 417, 312–315. doi: 10.1016/j.neulet.2007.02.050
Floresco, S. B., West, A. R., Ash, B., Moore, H., and Grace, A. A. (2003). Afferent modulation of dopamine neuron firing differentially regulates tonic and phasic dopamine transmission. Nat. Neurosci. 6, 968–973. doi: 10.1038/nn1103
Flowers, K. A. (1976). Visual "closed-loop" and "open-loop" characteristics of voluntary movement in patients with parkinsonism and intention tremor. Brain 99, 269–310. doi: 10.1093/brain/99.2.269
Fooken, J., and Spering, M. (2020). Eye movements as a readout of sensorimotor decision processes. J. Neurophysiol. 123, 1439–1447. doi: 10.1152/jn.00622.2019
Fuhrmann, F., Justus, D., Sosulina, L., Kaneko, H., Beutel, T., Friedrichs, D., et al. (2015). Locomotion, Theta oscillations, and the speed-correlated firing of hippocampal neurons are controlled by a medial septal glutamatergic circuit. Neuron 86, 1253–1264. doi: 10.1016/j.neuron.2015.05.001
Fuhs, M. C., and Touretzky, D. S. (2006). A spin glass model of path integration in rat medial entorhinal cortex. J. Neurosci. 26, 4266–4276. doi: 10.1523/JNEUROSCI.4353-05.2006
Fyhn, M., Hafting, T., Treves, A., Moser, M. B., and Moser, E. I. (2007). Hippocampal remapping and grid realignment in entorhinal cortex. Nature 446, 190–194. doi: 10.1038/nature05601
Fyhn, M., Molden, S., Witter, M. P., Moser, E. I., and Moser, M. B. (2004). Spatial representation in the entorhinal cortex. Science 305, 1258–1264. doi: 10.1126/science.1099901
Garraway, S. M., and Hochman, S. (2001). Modulatory actions of serotonin, norepinephrine, dopamine, and acetylcholine in spinal cord deep dorsal horn neurons. J. Neurophysiol. 86, 2183–2194. doi: 10.1152/jn.2001.86.5.2183
Gasbarri, A., Sulli, A., and Packard, M. G. (1997). The dopaminergic mesencephalic projections to the hippocampal formation in the rat. Prog. Neuropsychopharmacol. Biol.Psychiatry 21, 1–22. doi: 10.1016/S0278-5846(96)00157-1
Gatome, C. W., Slomianka, L., Lipp, H. P., and Amrein, I. (2010). Number estimates of neuronal phenotypes in layer II of the medial entorhinal cortex of rat and mouse. Neuroscience 170, 156–165. doi: 10.1016/j.neuroscience.2010.06.048
Georgiou, N., Bradshaw, J. L., Iansek, R., Phillips, J. G., Mattingley, J. B., and Bradshaw, J. A. (1994). Reduction in external cues and movement sequencing in Parkinson's disease. J. Neurol. Neurosurg. Psychiatry 57, 368–370. doi: 10.1136/jnnp.57.3.368
German, D. C., Manaye, K. F., White, C. L. 3rd, Woodward, D. J., McIntire, D. D., Smith, W. K., et al. (1992). Disease-specific patterns of locus coeruleus cell loss. Ann. Neurol. 32, 667–676. doi: 10.1002/ana.410320510
Ghiglieri, V., Sgobio, C., Costa, C., Picconi, B., and Calabresi, P. (2011). Striatum-hippocampus balance: from physiological behavior to interneuronal pathology. Prog. Neurobiol. 94, 102–114. doi: 10.1016/j.pneurobio.2011.04.005
Ghiglione, P., Mutani, R., and Chio, A. (2005). Cogwheel rigidity. Arch. Neurol. 62, 828–830. doi: 10.1001/archneur.62.5.828
Giladi, N., and Nieuwboer, A. (2008). Understanding and treating freezing of gait in parkinsonism, proposed working definition, and setting the stage. Mov. Disord. 23, S423–S425. doi: 10.1002/mds.21927
Giladi, N., Shabtai, H., Rozenberg, E., and Shabtai, E. (2001). Gait festination in Parkinson's disease. Parkinsonism Relat. Disord. 7, 135–138. doi: 10.1016/s1353-8020(00)00030-4
Ginis, P., Nackaerts, E., Nieuwboer, A., and Heremans, E. (2018). Cueing for people with Parkinson's disease with freezing of gait: a narrative review of the state-of-the-art and novel perspectives. Ann. Phys. Rehabil. Med. 61, 407–413. doi: 10.1016/j.rehab.2017.08.002
Ginosar, G., Aljadeff, J., Burak, Y., Sompolinsky, H., Las, L., and Ulanovsky, N. (2021). Locally ordered representation of 3D space in the entorhinal cortex. Nature 596, 404–409. doi: 10.1038/s41586-021-03783-x
Giocomo, L. M. (2016). Environmental boundaries as a mechanism for correcting and anchoring spatial maps. J. Physiol. 594, 6501–6511. doi: 10.1113/JP270624
Giocomo, L. M., and Hasselmo, M. E. (2008). Time constants of h current in layer ii stellate cells differ along the dorsal to ventral axis of medial entorhinal cortex. J. Neurosci. 28, 9414–9425. doi: 10.1523/JNEUROSCI.3196-08.2008
Giorgi, F. S., Biagioni, F., Galgani, A., Pavese, N., Lazzeri, G., and Fornai, F. (2020). Locus coeruleus modulates neuroinflammation in parkinsonism and dementia. Int. J. Mol. Sci. 21, 1–21. doi: 10.3390/ijms21228630
Glickstein, M., and Stein, J. (1991). Paradoxical movement in Parkinson's disease. Trends Neurosci. 14, 480–482. doi: 10.1016/0166-2236(91)90055-y
Gomez-Jordana, L. I., Stafford, J., Peper, C. L. E., and Craig, C. M. (2018). Crossing virtual doors: a new method to study gait impairments and freezing of gait in Parkinson's disease. Parkinsons Dis. 2018:2957427. doi: 10.1155/2018/2957427
Gorny, J. H., Gorny, B., Wallace, D. G., and Whishaw, I. Q. (2002). Fimbria-fornix lesions disrupt the dead reckoning (homing) component of exploratory behavior in mice. Learn. Mem. 9, 387–394. doi: 10.1101/lm.53002
Grieves, R. M., Jedidi-Ayoub, S., Mishchanchuk, K., Liu, A., Renaudineau, S., Duvelle, E., et al. (2021). Irregular distribution of grid cell firing fields in rats exploring a 3D volumetric space. Nat. Neurosci. 24, 1567–1573. doi: 10.1038/s41593-021-00907-4
Grieves, R. M., and Jeffery, K. J. (2017). The representation of space in the brain. Behav. Process. 135, 113–131. doi: 10.1016/j.beproc.2016.12.012
Gu, Z., and Yakel, J. L. (2017). Inducing theta oscillations in the entorhinal hippocampal network in vitro. Brain Struct. Funct. 222, 943–955. doi: 10.1007/s00429-016-1256-3
Gurney, K., Prescott, T. J., and Redgrave, P. (2001a). A computational model of action selection in the basal ganglia. I. A new functional anatomy. Biol. Cybern. 84, 401–410. doi: 10.1007/PL00007984
Gurney, K., Prescott, T. J., and Redgrave, P. (2001b). A computational model of action selection in the basal ganglia. II. Analysis and simulation of behaviour. Biol. Cybern. 84, 411–423. doi: 10.1007/PL00007985
Hafting, T., Fyhn, M., Bonnevie, T., Moser, M. B., and Moser, E. I. (2008). Hippocampus-independent phase precession in entorhinal grid cells. Nature 453, 1248–1252. doi: 10.1038/nature06957
Hafting, T., Fyhn, M., Molden, S., Moser, M. B., and Moser, E. I. (2005). Microstructure of a spatial map in the entorhinal cortex. Nature 436, 801–806. doi: 10.1038/nature03721
Hagglund, M., Morreaunet, M., Moser, M. B., and Moser, E. I. (2019). Grid-cell distortion along geometric Borders. Curr. Biol. 29, 1047–1054. doi: 10.1016/j.cub.2019.01.074
Halje, P., Tamte, M., Richter, U., Mohammed, M., Cenci, M. A., and Petersson, P. (2012). Levodopa-induced dyskinesia is strongly associated with resonant cortical oscillations. J. Neurosci. 32, 16541–16551. doi: 10.1523/JNEUROSCI.3047-12.2012
Hallett, M. (2012). Parkinson's disease tremor: pathophysiology. Parkinsonism Relat. Disord. 18, S85–S86. doi: 10.1016/S1353-8020(11)70027-X
Hallett, M. (2014). Tremor: pathophysiology. Parkinsonism Relat. Disord. 20, S118–S122. doi: 10.1016/S1353-8020(13)70029-4
Han, P., Nakanishi, S. T., Tran, M. A., and Whelan, P. J. (2007). Dopaminergic modulation of spinal neuronal excitability. J. Neurosci. 27, 13192–13204. doi: 10.1523/JNEUROSCI.1279-07.2007
Hardcastle, K., Ganguli, S., and Giocomo, L. M. (2015). Environmental boundaries as an error correction mechanism for grid cells. Neuron 86, 827–839. doi: 10.1016/j.neuron.2015.03.039
Hardcastle, K., Maheswaranathan, N., Ganguli, S., and Giocomo, L. M. (2017). A multiplexed, heterogeneous, and adaptive code for navigation in medial entorhinal cortex. Neuron 94, 375–387.e7. doi: 10.1016/j.neuron.2017.03.025
Harris, J. P., Atkinson, E. A., Lee, A. C., Nithi, K., and Fowler, M. S. (2003). Hemispace differences in the visual perception of size in left hemi Parkinson's disease. Neuropsychologia 41, 795–807. doi: 10.1016/S0028-3932(02)00285-3
Harris, K. D., Henze, D. A., Hirase, H., Leinekugel, X., Dragoi, G., Czurko, A., et al. (2002). Spike train dynamics predicts theta-related phase precession in hippocampal pyramidal cells. Nature 417, 738–741. doi: 10.1038/nature00808
Harris, M. A., Wiener, J. M., and Wolbers, T. (2012). Aging specifically impairs switching to an allocentric navigational strategy. Front. Aging Neurosci. 4:29. doi: 10.3389/fnagi.2012.00029
Hartley, T., and Lever, C. (2014). Know your limits: the role of boundaries in the development of spatial representation. Neuron 82, 1–3. doi: 10.1016/j.neuron.2014.03.017
Hartley, T., Maguire, E. A., Spiers, H. J., and Burgess, N. (2003). The well-worn route and the path less traveled: distinct neural bases of route following and wayfinding in humans. Neuron 37, 877–888. doi: 10.1016/s0896-6273(03)00095-3
Hausdorff, J. M., Balash, J., and Giladi, N. (2003). Time series analysis of leg movements during freezing of gait in Parkinson’s disease: akinesia, rhyme or reason? Phys. A 321, 565–570. doi: 10.1016/S0378-4371(02)01744-2
He, Q., and Brown, T. I. (2019). Environmental barriers disrupt grid-like representations in humans during navigation. Curr. Biol. 29, 2718–2722. doi: 10.1016/j.cub.2019.06.072
Heath, M., Neely, K., and Binsted, G. (2007). Allocentric visual cues influence online limb adjustments. Mot. Control. 11, 54–70.
Heys, J. G., and Dombeck, D. A. (2018). Evidence for a subcircuit in medial entorhinal cortex representing elapsed time during immobility. Nat. Neurosci. 21, 1574–1582. doi: 10.1038/s41593-018-0252-8
Heys, J. G., Wu, Z., Allegra Mascaro, A. L., and Dombeck, D. A. (2020). Inactivation of the medial entorhinal cortex selectively disrupts learning of interval timing. Cell Rep. 32:108163. doi: 10.1016/j.celrep.2020.108163
Hinman, J. R., Brandon, M. P., Climer, J. R., Chapman, G. W., and Hasselmo, M. E. (2016). Multiple running speed signals in medial entorhinal cortex. Neuron 91, 666–679. doi: 10.1016/j.neuron.2016.06.027
Hjorth, J. J. J., Kozlov, A., Carannante, I., Frost Nylen, J., Lindroos, R., Johansson, Y., et al. (2020). The microcircuits of striatum in silico. Proc. Natl. Acad. Sci. USA 117, 9554–9565. doi: 10.1073/pnas.2000671117
Hof, P. R., Charpiot, A., Delacourte, A., Buee, L., Purohit, D., Perl, D. P., et al. (1992). Distribution of neurofibrillary tangles and senile plaques in the cerebral cortex in postencephalitic parkinsonism. Neurosci. Lett. 139, 10–14. doi: 10.1016/0304-3940(92)90846-y
Hornykiewicz, O. (1962). Dopamine (3-hydroxytyramine) in the central nervous system and its relation to the Parkinson syndrome in man. Dtsch. Med. Wochenschr. 87, 1807–1810. doi: 10.1055/s-0028-1114024
Hornykiewicz, O., and Kish, S. J. (1987). Biochemical pathophysiology of Parkinson's disease. Adv. Neurol. 45, 19–34.
Hoydal, O. A., Skytoen, E. R., Andersson, S. O., Moser, M. B., and Moser, E. I. (2019). Object-vector coding in the medial entorhinal cortex. Nature 568, 400–404. doi: 10.1038/s41586-019-1077-7
Hurtado, J. M., Lachaux, J. P., Beckley, D. J., Gray, C. M., and Sigvardt, K. A. (2000). Inter- and intralimb oscillator coupling in parkinsonian tremor. Mov. Disord. 15, 683–691. doi: 10.1002/1531-8257(200007)15:4<683::aid-mds1013>3.0.co;2-#
Iansek, R., Huxham, F., and McGinley, J. (2006). The sequence effect and gait festination in Parkinson disease: contributors to freezing of gait? Mov. Disord. 21, 1419–1424. doi: 10.1002/mds.20998
Igloi, K., Zaoui, M., Berthoz, A., and Rondi-Reig, L. (2009). Sequential egocentric strategy is acquired as early as allocentric strategy: parallel acquisition of these two navigation strategies. Hippocampus 19, 1199–1211. doi: 10.1002/hipo.20595
Inase, M., Buford, J. A., and Anderson, M. E. (1996). Changes in the control of arm position, movement, and thalamic discharge during local inactivation in the globus pallidus of the monkey. J. Neurophysiol. 75, 1087–1104. doi: 10.1152/jn.1996.75.3.1087
Inzelberg, R., Plotnik, M., Harpaz, N. K., and Flash, T. (2016). Micrographia, much beyond the writer's hand. Parkinsonism Relat. Disord. 26, 1–9. doi: 10.1016/j.parkreldis.2016.03.003
Ismakov, R., Barak, O., Jeffery, K., and Derdikman, D. (2017). Grid cells encode local positional information. Curr. Biol. 27, 2337–2343. doi: 10.1016/j.cub.2017.06.034
Jacob, P. Y., Capitano, F., Poucet, B., Save, E., and Sargolini, F. (2019). Path integration maintains spatial periodicity of grid cell firing in a 1D circular track. Nat. Commun. 10:840. doi: 10.1038/s41467-019-08795-w
Jacob, P. Y., Gordillo-Salas, M., Facchini, J., Poucet, B., Save, E., and Sargolini, F. (2017). Medial entorhinal cortex and medial septum contribute to self-motion-based linear distance estimation. Brain Struct. Funct. 222, 2727–2742. doi: 10.1007/s00429-017-1368-4
Jacobs, J. V., and Horak, F. B. (2006). Abnormal proprioceptive-motor integration contributes to hypometric postural responses of subjects with Parkinson's disease. Neuroscience 141, 999–1009. doi: 10.1016/j.neuroscience.2006.04.014
Jacobs, J. V., Nutt, J. G., Carlson-Kuhta, P., Stephens, M., and Horak, F. B. (2009). Knee trembling during freezing of gait represents multiple anticipatory postural adjustments. Exp. Neurol. 215, 334–341. doi: 10.1016/j.expneurol.2008.10.019
Jarzebska, E. (2006). Evaluation of effectiveness of the micrographia's therapy in Parkinson's disease patients. Pol. Merkur. Lekarski 20, 688–690.
Jeewajee, A., Barry, C., O'Keefe, J., and Burgess, N. (2008). Grid cells and theta as oscillatory interference: electrophysiological data from freely moving rats. Hippocampus 18, 1175–1185. doi: 10.1002/hipo.20510
Jeffery, K. J. (2007). Integration of the sensory inputs to place cells: what, where, why, and how? Hippocampus 17, 775–785. doi: 10.1002/hipo.20322
Jeffery, K. J., Wilson, J. J., Casali, G., and Hayman, R. M. (2015). Neural encoding of large-scale three-dimensional space-properties and constraints. Front. Psychol. 6:927. doi: 10.3389/fpsyg.2015.00927
Jin, X., Chen, Q., Song, Y., Zheng, J., Xiao, K., Shao, S., et al. (2019). Dopamine D2 receptors regulate the action potential threshold by modulating T-type calcium channels in stellate cells of the medial entorhinal cortex. J. Physiol. 597, 3363–3387. doi: 10.1113/JP277976
Johnson, A., van der Meer, M. A., and Redish, A. D. (2007). Integrating hippocampus and striatum in decision-making. Curr. Opin. Neurobiol. 17, 692–697. doi: 10.1016/j.conb.2008.01.003
Joshi, A., and Somogyi, P. (2020). Changing phase relationship of the stepping rhythm to neuronal oscillatory theta activity in the septo-hippocampal network of mice. Brain Struct. Funct. 225, 871–879. doi: 10.1007/s00429-020-02031-8
Justus, D., Dalugge, D., Bothe, S., Fuhrmann, F., Hannes, C., Kaneko, H., et al. (2017). Glutamatergic synaptic integration of locomotion speed via septoentorhinal projections. Nat. Neurosci. 20, 16–19. doi: 10.1038/nn.4447
Kabasakalian, A., Kesayan, T., Williamson, J. B., Skidmore, F. M., Falchook, A. D., Harciarek, M., et al. (2013). Hypometric allocentric and egocentric distance estimates in Parkinson disease. Cogn. Behav. Neurol. 26, 133–139. doi: 10.1097/WNN.0000000000000007
Kang, S. Y., Wasaka, T., Shamim, E. A., Auh, S., Ueki, Y., Lopez, G. J., et al. (2010). Characteristics of the sequence effect in Parkinson's disease. Mov. Disord. 25, 2148–2155. doi: 10.1002/mds.23251
Katzenschlager, R., Head, J., Schrag, A., Ben-Shlomo, Y., Evans, A., Lees, A. J., et al. (2008). Fourteen-year final report of the randomized PDRG-UK trial comparing three initial treatments in PD. Neurology 71, 474–480. doi: 10.1212/01.wnl.0000310812.43352.66
Keinath, A. T. (2016). The preferred directions of conjunctive grid X Head direction cells in the medial entorhinal cortex are periodically organized. PLoS One 11:e0152041. doi: 10.1371/journal.pone.0152041
Keinath, A. T., Epstein, R. A., and Balasubramanian, V. (2018). Environmental deformations dynamically shift the grid cell spatial metric. elife 7, 1–22. doi: 10.7554/eLife.38169
Kikuchi, A., Baba, T., Hasegawa, T., Sugeno, N., Konno, M., Miura, E., et al. (2014). Improvement of freezing of gait in patients with Parkinson's disease by imagining bicycling. Case Rep. Neurol. 6, 92–95. doi: 10.1159/000362119
Killian, N. J., Jutras, M. J., and Buffalo, E. A. (2012). A map of visual space in the primate entorhinal cortex. Nature 491, 761–764. doi: 10.1038/nature11587
Kim, M., and Maguire, E. A. (2019). Can we study 3D grid codes non-invasively in the human brain? Methodological considerations and fMRI findings. NeuroImage 186, 667–678. doi: 10.1016/j.neuroimage.2018.11.041
Kinkhabwala, A. A., Gu, Y., Aronov, D., and Tank, D. W. (2020). Visual cue-related activity of cells in the medial entorhinal cortex during navigation in virtual reality. elife 9, 1–24. doi: 10.7554/eLife.43140
Kinnier Wilson, S. A. (1925). The Croonian lectures on some disorders of motility and of muscle tone, with special reference to the corpus striatum. Lancet 206, 1–10. doi: 10.1016/S0140-6736(01)20638-2
Klaus, A., Martins, G. J., Paixao, V. B., Zhou, P., Paninski, L., and Costa, R. M. (2017). The spatiotemporal Organization of the Striatum Encodes Action Space. Neuron 95, 1171–1180.e.1177. doi: 10.1016/j.neuron.2017.08.015
Klockgether, T., and Dichgans, J. (1994). Visual control of arm movement in Parkinson's disease. Mov. Disord. 9, 48–56. doi: 10.1002/mds.870090108
Knierim, J. J. (2015). From the GPS to HM: place cells, grid cells, and memory. Hippocampus 25, 719–725. doi: 10.1002/hipo.22453
Kraus, B. J., Brandon, M. P., Robinson, R. J., Connerney, M. A., Hasselmo, M. E., and Eichenbaum, H. (2015). During running in place, grid cells integrate elapsed time and distance run. Neuron 88, 578–589. doi: 10.1016/j.neuron.2015.09.031
Kropff, E., Carmichael, J. E., Moser, M. B., and Moser, E. I. (2015). Speed cells in the medial entorhinal cortex. Nature 523, 419–424. doi: 10.1038/nature14622
Kropff, E., Carmichael, J. E., Moser, E. I., and Moser, M. B. (2021). Frequency of theta rhythm is controlled by acceleration, but not speed, in running rats. Neuron 109, 1029–1039.e.1028. doi: 10.1016/j.neuron.2021.01.017
Kropff, E., and Treves, A. (2008). The emergence of grid cells: intelligent design or just adaptation? Hippocampus 18, 1256–1269. doi: 10.1002/hipo.20520
Krupic, J., Bauza, M., Burton, S., Barry, C., and O'Keefe, J. (2015). Grid cell symmetry is shaped by environmental geometry. Nature 518, 232–235. doi: 10.1038/nature14153
Krupic, J., Bauza, M., Burton, S., and O'Keefe, J. (2016). Framing the grid: effect of boundaries on grid cells and navigation. J. Physiol. 594, 6489–6499. doi: 10.1113/JP270607
Kulkarni, O., Lafaver, K., and Tarsy, D. (2013). The "floating door sign" in Parkinson's disease. Parkinsonism Relat. Disord. 19, 825–826. doi: 10.1016/j.parkreldis.2013.04.013
Laitinen, L. V., Bergenheim, A. T., and Hariz, M. I. (1992). Leksell's posteroventral pallidotomy in the treatment of Parkinson's disease. J. Neurosurg. 76, 53–61. doi: 10.3171/jns.1992.76.1.0053
Langston, R. F., Ainge, J. A., Couey, J. J., Canto, C. B., Bjerknes, T. L., Witter, M. P., et al. (2010). Development of the spatial representation system in the rat. Science 328, 1576–1580. doi: 10.1126/science.1188210
Latuske, P., Toader, O., and Allen, K. (2015). Interspike intervals reveal functionally distinct cell populations in the medial entorhinal cortex. J. Neurosci. 35, 10963–10976. doi: 10.1523/JNEUROSCI.0276-15.2015
Laudate, T. M., Neargarder, S., and Cronin-Golomb, A. (2013). Line bisection in Parkinson's disease: investigation of contributions of visual field, retinal vision, and scanning patterns to visuospatial function. Behav. Neurosci. 127, 151–163. doi: 10.1037/a0031618
Lebold, C. A., and Almeida, Q. J. (2010). Evaluating the contributions of dynamic flow to freezing of gait in Parkinson's disease. Parkinsons Dis. 2010:732508. doi: 10.4061/2010/732508
Lee, A. C., Harris, J. P., Atkinson, E. A., and Fowler, M. S. (2001a). Disruption of estimation of body-scaled aperture width in Hemiparkinson's disease. Neuropsychologia 39, 1097–1104. doi: 10.1016/S0028-3932(01)00032-X
Lee, A. C., Harris, J. P., Atkinson, E. A., and Fowler, M. S. (2001b). Evidence from a line bisection task for visuospatial neglect in left hemiparkinson's disease. Vis. Res. 41, 2677–2686. doi: 10.1016/S0042-6989(01)00129-8
Lee, A. C., Harris, J. P., and Calvert, J. E. (1998). Impairments of mental rotation in Parkinson's disease. Neuropsychologia 36, 109–114. doi: 10.1016/S0028-3932(97)00017-1
Lee, D., Henriques, D. Y., Snider, J., Song, D., and Poizner, H. (2013). Reaching to proprioceptively defined targets in Parkinson's disease: effects of deep brain stimulation therapy. Neuroscience 244, 99–112. doi: 10.1016/j.neuroscience.2013.04.009
Lee, M. J., Kim, S. L., Lyoo, C. H., Rinne, J. O., and Lee, M. S. (2015). Impact of regional striatal dopaminergic function on kinematic parameters of Parkinson's disease. J. Neural Transm. (Vienna) 122, 669–677. doi: 10.1007/s00702-014-1296-x
Lepperod, M. E., Christensen, A. C., Lensjo, K. K., Buccino, A. P., Yu, J., Fyhn, M., et al. (2021). Optogenetic pacing of medial septum parvalbumin-positive cells disrupts temporal but not spatial firing in grid cells. Sci. Adv. 7, 1–18. doi: 10.1126/sciadv.abd5684
Lever, C., Burton, S., Jeewajee, A., O'Keefe, J., and Burgess, N. (2009). Boundary vector cells in the subiculum of the hippocampal formation. J. Neurosci. 29, 9771–9777. doi: 10.1523/JNEUROSCI.1319-09.2009
Lewis, S. J., and Barker, R. A. (2009). A pathophysiological model of freezing of gait in Parkinson's disease. Parkinsonism Relat. Disord. 15, 333–338. doi: 10.1016/j.parkreldis.2008.08.006
Lex, B., and Hauber, W. (2010). Disconnection of the entorhinal cortex and dorsomedial striatum impairs the sensitivity to instrumental contingency degradation. Neuropsychopharmacology 35, 1788–1796. doi: 10.1038/npp.2010.46
Li, H. B., Lin, L., Yang, L. Y., and Xie, C. (2015). Dopaminergic facilitation of GABAergic transmission in layer III of rat medial entorhinal cortex. Chin. J. Phys. 58, 46–54. doi: 10.4077/CJP.2015.BAC241
Lim, I., van Wegen, E., de Goede, C., Deutekom, M., Nieuwboer, A., Willems, A., et al. (2005). Effects of external rhythmical cueing on gait in patients with Parkinson's disease: a systematic review. Clin. Rehabil. 19, 695–713. doi: 10.1191/0269215505cr906oa
Maaswinkel, H., and Whishaw, I. Q. (1999). Homing with locale, taxon, and dead reckoning strategies by foraging rats: sensory hierarchy in spatial navigation. Behav. Brain Res. 99, 143–152. doi: 10.1016/S0166-4328(98)00100-4
Macefield, V. G., and Knellwolf, T. P. (2018). Functional properties of human muscle spindles. J. Neurophysiol. 120, 452–467. doi: 10.1152/jn.00071.2018
Malhotra, S., Cross, R. W., and van der Meer, M. A. (2012). Theta phase precession beyond the hippocampus. Rev. Neurosci. 23, 39–65. doi: 10.1515/revneuro-2011-0064
Mancini, M., Smulders, K., Cohen, R. G., Horak, F. B., Giladi, N., and Nutt, J. G. (2017). The clinical significance of freezing while turning in Parkinson's disease. Neuroscience 343, 222–228. doi: 10.1016/j.neuroscience.2016.11.045
Manson, A., Stirpe, P., and Schrag, A. (2012). Levodopa-induced-dyskinesias clinical features, incidence, risk factors, management and impact on quality of life. J. Parkinsons Dis. 2, 189–198. doi: 10.3233/JPD-2012-120103
Marsden, C. D. (1989). Slowness of movement in Parkinson's disease. Mov. Disord. 4, S26–S37. doi: 10.1002/mds.870040505
Marsden, C. D., and Obeso, J. A. (1994). The functions of the basal ganglia and the paradox of stereotaxic surgery in Parkinson's disease. Brain 117, 877–897. doi: 10.1093/brain/117.4.877
Martin, K. E., Phillips, J. G., Iansek, R., and Bradshaw, J. L. (1994). Inaccuracy and instability of sequential movements in Parkinson's disease. Exp. Brain Res. 102, 131–140. doi: 10.1007/BF00232445
Matar, E., Shine, J. M., Gilat, M., Ehgoetz Martens, K. A., Ward, P. B., Frank, M. J., et al. (2019). Identifying the neural correlates of doorway freezing in Parkinson's disease. Hum. Brain Mapp. 40, 2055–2064. doi: 10.1002/hbm.24506
Mathis, A., Herz, A. V., and Stemmler, M. B. (2013). Multiscale codes in the nervous system: the problem of noise correlations and the ambiguity of periodic scales. Phys. Rev. E Stat. Nonlinear Soft Matter Phys. 88:022713. doi: 10.1103/PhysRevE.88.022713
Mayne, E. W., Craig, M. T., McBain, C. J., and Paulsen, O. (2013). Dopamine suppresses persistent network activity via D (1) -like dopamine receptors in rat medial entorhinal cortex. Eur. J. Neurosci. 37, 1242–1247. doi: 10.1111/ejn.12125
McCandless, P. J., Evans, B. J., Janssen, J., Selfe, J., Churchill, A., and Richards, J. (2016). Effect of three cueing devices for people with Parkinson's disease with gait initiation difficulties. Gait Posture 44, 7–11. doi: 10.1016/j.gaitpost.2015.11.006
McFarthing, K., Prakash, N., and Simuni, T. (2019). Clinical trial highlights-dyskinesia. J. Parkinsons Dis. 9, 449–465. doi: 10.3233/JPD-199002
McLennan, J. E., Nakano, K., Tyler, H. R., and Schwab, R. S. (1972). Micrographia in Parkinson's disease. J. Neurol. Sci. 15, 141–152. doi: 10.1016/0022-510x(72)90002-0
Meister, M. L. R., and Buffalo, E. A. (2018). Neurons in primate entorhinal cortex represent gaze position in multiple spatial reference frames. J. Neurosci. 38, 2430–2441. doi: 10.1523/JNEUROSCI.2432-17.2018
Mena-Segovia, J., and Bolam, J. P. (2017). Rethinking the Pedunculopontine nucleus: from cellular organization to function. Neuron 94, 7–18. doi: 10.1016/j.neuron.2017.02.027
Mena-Segovia, J., Bolam, J. P., and Magill, P. J. (2004). Pedunculopontine nucleus and basal ganglia: distant relatives or part of the same family? Trends Neurosci. 27, 585–588. doi: 10.1016/j.tins.2004.07.009
Miao, C., Cao, Q., Ito, H. T., Yamahachi, H., Witter, M. P., Moser, M. B., et al. (2015). Hippocampal remapping after partial inactivation of the medial entorhinal cortex. Neuron 88, 590–603. doi: 10.1016/j.neuron.2015.09.051
Miao, C., Cao, Q., Moser, M. B., and Moser, E. I. (2017). Parvalbumin and somatostatin interneurons control different space-coding networks in the medial entorhinal cortex. Cell 171, 507–521.e17. doi: 10.1016/j.cell.2017.08.050
Miguelez, C., Aristieta, A., Cenci, M. A., and Ugedo, L. (2011). The locus coeruleus is directly implicated in L-DOPA-induced dyskinesia in parkinsonian rats: an electrophysiological and behavioural study. PLoS One 6:e24679. doi: 10.1371/journal.pone.0024679
Milanov, I. (2001). Electromyographic differentiation of tremors. Clin. Neurophysiol. 112, 1626–1632. doi: 10.1016/S1388-2457(01)00629-0
Milla-Cruz, J. J., Mena-Avila, E., Calvo, J. R., Hochman, S., Villalon, C. M., and Quevedo, J. N. (2020). The activation of D2 and D3 receptor subtypes inhibits pathways mediating primary afferent depolarization (PAD) in the mouse spinal cord. Neurosci. Lett. 736:135257. doi: 10.1016/j.neulet.2020.135257
Mink, J. W. (1996). The basal ganglia: focused selection and inhibition of competing motor programs. Prog. Neurobiol. 50, 381–425. doi: 10.1016/S0301-0082(96)00042-1
Moccia, M., Pappata, S., Picillo, M., Erro, R., Coda, A. R., Longo, K., et al. (2014). Dopamine transporter availability in motor subtypes of de novo drug-naive Parkinson's disease. J. Neurol. 261, 2112–2118. doi: 10.1007/s00415-014-7459-8
Mohedano-Moriano, A., Martinez-Marcos, A., Pro-Sistiaga, P., Blaizot, X., Arroyo-Jimenez, M. M., Marcos, P., et al. (2008). Convergence of unimodal and polymodal sensory input to the entorhinal cortex in the fascicularis monkey. Neuroscience 151, 255–271. doi: 10.1016/j.neuroscience.2007.09.074
Montgomery, E. B. Jr. (2011). One view of the current state of understanding in basal ganglia pathophysiology and what is needed for the future. J. Mov Disord. 4, 13–20. doi: 10.14802/jmd.11003
Moreau, C., Ozsancak, C., Blatt, J. L., Derambure, P., Destee, A., and Defebvre, L. (2007). Oral festination in Parkinson's disease: biomechanical analysis and correlation with festination and freezing of gait. Mov. Disord. 22, 1503–1506. doi: 10.1002/mds.21549
Moser, E. I., Kropff, E., and Moser, M. B. (2008). Place cells, grid cells, and the brain's spatial representation system. Annu. Rev. Neurosci. 31, 69–89. doi: 10.1146/annurev.neuro.31.061307.090723
Moser, E. I., Moser, M. B., and Roudi, Y. (2014). Network mechanisms of grid cells. Philos. Trans. R. Soc. Lond. Ser. B Biol. Sci. 369:20120511. doi: 10.1098/rstb.2012.0511
Mosheiff, N., and Burak, Y. (2019). Velocity coupling of grid cell modules enables stable embedding of a low dimensional variable in a high dimensional n-eural attractor. elife 8, 1–32. doi: 10.7554/eLife.48494
Mullick, A. A., Musampa, N. K., Feldman, A. G., and Levin, M. F. (2013). Stretch reflex spatial threshold measure discriminates between spasticity and rigidity. Clin. Neurophysiol. 124, 740–751. doi: 10.1016/j.clinph.2012.10.008
Mutch, W. J., Strudwick, A., Roy, S. K., and Downie, A. W. (1986). Parkinson's disease: disability, review, and management. Br. Med. J. (Clin. Res. Ed.) 293, 675–677. doi: 10.1136/bmj.293.6548.675
Nadasdy, Z., Nguyen, T. P., Torok, A., Shen, J. Y., Briggs, D. E., Modur, P. N., et al. (2017). Context-dependent spatially periodic activity in the human entorhinal cortex. Proc. Natl. Acad. Sci. USA 114, E3516–E3525. doi: 10.1073/pnas.1701352114
Nadjar, A., Gerfen, C. R., and Bezard, E. (2009). Priming for l-dopa-induced dyskinesia in Parkinson's disease: a feature inherent to the treatment or the disease? Prog. Neurobiol. 87, 1–9. doi: 10.1016/j.pneurobio.2008.09.013
Nahimi, A., Kinnerup, M. B., Sommerauer, M., Gjedde, A., and Borghammer, P. (2018). Molecular imaging of the noradrenergic system in idiopathic Parkinson's disease. Int. Rev. Neurobiol. 141, 251–274. doi: 10.1016/bs.irn.2018.07.028
Nakamura, R., Nagasaki, H., and Narabayashi, H. (1978). Disturbances of rhythm formation in patients with Parkinson's disease: part I. Characteristics of tapping response to the periodic signals. Percept. Mot. Skills 46, 63–75. doi: 10.2466/pms.1978.46.1.63
Nambu, A. (2008). Seven problems on the basal ganglia. Curr. Opin. Neurobiol. 18, 595–604. doi: 10.1016/j.conb.2008.11.001
Nambu, A., Tachibana, Y., and Chiken, S. (2015). Cause of parkinsonian symptoms: firing rate, firing pattern or dynamic activity changes? Basal Ganglia 5, 1–6. doi: 10.1016/j.baga.2014.11.001
Nambu, A., Tokuno, H., and Takada, M. (2002). Functional significance of the cortico-subthalamo-pallidal 'hyperdirect' pathway. Neurosci. Res. 43, 111–117. doi: 10.1016/S0168-0102(02)00027-5
Naumann, R. K., Preston-Ferrer, P., Brecht, M., and Burgalossi, A. (2018). Structural modularity and grid activity in the medial entorhinal cortex. J. Neurophysiol. 119, 2129–2144. doi: 10.1152/jn.00574.2017
Neely, K. A., Tessmer, A., Binsted, G., and Heath, M. (2008). Goal-directed reaching: movement strategies influence the weighting of allocentric and egocentric visual cues. Exp. Brain Res. 186, 375–384. doi: 10.1007/s00221-007-1238-z
Nieuwboer, A. (2008). Cueing for freezing of gait in patients with Parkinson's disease: a rehabilitation perspective. Mov. Disord. 23, S475–S481. doi: 10.1002/mds.21978
Nieuwenhuys, R, Voogd, J, and Huijzen, C (eds.) (2008). The Human Central Nervous System. Berlin, Heidelberg, New York: Springer
Nonnekes, J., Giladi, N., Guha, A., Fietzek, U. M., Bloem, B. R., and Ruzicka, E. (2019a). Gait festination in parkinsonism: introduction of two phenotypes. J. Neurol. 266, 426–430. doi: 10.1007/s00415-018-9146-7
Nonnekes, J., Ruzicka, E., Nieuwboer, A., Hallett, M., Fasano, A., and Bloem, B. R. (2019b). Compensation strategies for gait impairments in Parkinson disease: a review. JAMA Neurol. 76, 718–725. doi: 10.1001/jamaneurol.2019.0033
Nonnekes, J., Snijders, A. H., Nutt, J. G., Deuschl, G., Giladi, N., and Bloem, B. R. (2015). Freezing of gait: a practical approach to management. Lancet Neurol. 14, 768–778. doi: 10.1016/S1474-4422(15)00041-1
Nutt, J. G., Bloem, B. R., Giladi, N., Hallett, M., Horak, F. B., and Nieuwboer, A. (2011). Freezing of gait: moving forward on a mysterious clinical phenomenon. Lancet Neurol. 10, 734–744. doi: 10.1016/S1474-4422(11)70143-0
Nutt, J. G., Chung, K. A., and Holford, N. H. (2010). Dyskinesia and the antiparkinsonian response always temporally coincide: a retrospective study. Neurology 74, 1191–1197. doi: 10.1212/WNL.0b013e3181d90050
Oades, R. D., and Halliday, G. M. (1987). Ventral tegmental (A10) system: neurobiology. 1. Anatomy and connectivity. Brain Res. 12, 117–165. doi: 10.1016/0165-0173(87)90011-7
Obeso, J. A., Marin, C., Rodriguez-Oroz, C., Blesa, J., Itez-Temino, B., Mena-Segovia, J., et al. (2008a). The basal ganglia in Parkinson's disease: current concepts and unexplained observations. Ann. Neurol. 64, S30–S46. doi: 10.1002/ana.21481
Obeso, J. A., Rodriguez-Oroz, M. C., Benitez-Temino, B., Blesa, F. J., Guridi, J., Marin, C., et al. (2008b). Functional organization of the basal ganglia: therapeutic implications for Parkinson's disease. Mov. Disord. 23, S548–S559. doi: 10.1002/mds.22062
Obeso, J. A., Rodriguez-Oroz, M. C., Stamelou, M., Bhatia, K. P., and Burn, D. J. (2014). The expanding universe of disorders of the basal ganglia. Lancet 384, 523–531. doi: 10.1016/S0140-6736(13)62418-6
Oertel, W. H., Henrich, M. T., Janzen, A., and Geibl, F. F. (2019). The locus coeruleus: another vulnerability target in Parkinson's disease. Mov. Disord. 34, 1423–1429. doi: 10.1002/mds.27785
O'Keefe, J., and Dostrovsky, J. (1971). The hippocampus as a spatial map. Preliminary evidence from unit activity in the freely-moving rat. Brain Res. 34, 171–175. doi: 10.1016/0006-8993(71)90358-1
O'Keefe, J., and Recce, M. L. (1993). Phase relationship between hippocampal place units and the EEG theta rhythm. Hippocampus 3, 317–330. doi: 10.1002/hipo.450030307
Okuma, Y. (2006). Freezing of gait in Parkinson's disease. J. Neurol. 253:VII27-32. doi: 10.1007/s00415-006-7007-2
Olafsdottir, H. F., Bush, D., and Barry, C. (2018). The role of hippocampal replay in memory and planning. Curr. Biol. 28, R37–R50. doi: 10.1016/j.cub.2017.10.073
Olafsdottir, H. F., Carpenter, F., and Barry, C. (2016). Coordinated grid and place cell replay during rest. Nat. Neurosci. 19, 792–794. doi: 10.1038/nn.4291
Olafsdottir, H. F., Carpenter, F., and Barry, C. (2017). Task demands predict a dynamic switch in the content of awake hippocampal replay. Neuron 96, 925–935.e6. doi: 10.1016/j.neuron.2017.09.035
Oliveira, R. M., Gurd, J. M., Nixon, P., Marshall, J. C., and Passingham, R. E. (1997). Micrographia in Parkinson's disease: the effect of providing external cues. J. Neurol. Neurosurg. Psychiatry 63, 429–433. doi: 10.1136/jnnp.63.4.429
O'Neill, J., Boccara, C. N., Stella, F., Schoenenberger, P., and Csicsvari, J. (2017). Superficial layers of the medial entorhinal cortex replay independently of the hippocampus. Science 355, 184–188. doi: 10.1126/science.aag2787
O'Suilleabhain, P. E., and Matsumoto, J. Y. (1998). Time-frequency analysis of tremors. Brain 121, 2127–2134. doi: 10.1093/brain/121.11.2127
Packard, M. G., and McGaugh, J. L. (1996). Inactivation of hippocampus or caudate nucleus with lidocaine differentially affects expression of place and response learning. Neurobiol. Learn. Mem. 65, 65–72. doi: 10.1006/nlme.1996.0007
Park, S. W., Jang, H. J., Kim, M., and Kwag, J. (2019). Spatiotemporally random and diverse grid cell spike patterns contribute to the transformation of grid cell to place cell in a neural network model. PLoS One 14:e0225100. doi: 10.1371/journal.pone.0225100
Park, H., Youm, C., Lee, M., Noh, B., and Cheon, S. M. (2020). Turning characteristics of the more-affected side in Parkinson's disease patients with freezing of gait. Sensors 20, 1–15. doi: 10.3390/s20113098
Parkinson’s_Foundation (n.d.). Freezing. FL: 200 SE 1st Street, Ste 800, Miami, FL 33131, United States. Available at: https://www.parkinson.org/living-with-parkinsons/management/activities-daily-living/freezing (Accessed July 17, 2023).
Pasquereau, B., DeLong, M. R., and Turner, R. S. (2016). Primary motor cortex of the parkinsonian monkey: altered encoding of active movement. Brain 139, 127–143. doi: 10.1093/brain/awv312
Pastoll, H., Solanka, L., van Rossum, M. C., and Nolan, M. F. (2013). Feedback inhibition enables theta-nested gamma oscillations and grid firing fields. Neuron 77, 141–154. doi: 10.1016/j.neuron.2012.11.032
Pedrosa, D. J., Reck, C., Florin, E., Pauls, K. A., Maarouf, M., Wojtecki, L., et al. (2012). Essential tremor and tremor in Parkinson's disease are associated with distinct 'tremor clusters' in the ventral thalamus. Exp. Neurol. 237, 435–443. doi: 10.1016/j.expneurol.2012.07.002
Penner, M. R., and Mizumori, S. J. (2012). Neural systems analysis of decision making during goal-directed navigation. Prog. Neurobiol. 96, 96–135. doi: 10.1016/j.pneurobio.2011.08.010
Penney, J. B. Jr., and Young, A. B. (1986). Striatal inhomogeneities and basal ganglia function. Mov. Disord. 1, 3–15. doi: 10.1002/mds.870010102
Perez-Escobar, J. A., Kornienko, O., Latuske, P., Kohler, L., and Allen, K. (2016). Visual landmarks sharpen grid cell metric and confer context specificity to neurons of the medial entorhinal cortex. elife 5, 1–21. doi: 10.7554/eLife.16937
Pernia-Andrade, A. J., Wenger, N., Esposito, M. S., and Tovote, P. (2021). Circuits for state-dependent modulation of locomotion. Front. Hum. Neurosci. 15:745689. doi: 10.3389/fnhum.2021.745689
Pieperhoff, P., Sudmeyer, M., Dinkelbach, L., Hartmann, C. J., Ferrea, S., Moldovan, A. S., et al. (2022). Regional changes of brain structure during progression of idiopathic Parkinson's disease—a longitudinal study using deformation based morphometry. Cortex 151, 188–210. doi: 10.1016/j.cortex.2022.03.009
Pilly, P. K., and Grossberg, S. (2013). How reduction of theta rhythm by medial septum inactivation may covary with disruption of entorhinal grid cell responses due to reduced cholinergic transmission. Front. Neural Circuits 7:173. doi: 10.3389/fncir.2013.00173
Plotkin, J. L., and Goldberg, J. A. (2018). Thinking outside the box (and arrow): current themes in striatal dysfunction in movement disorders. Neuroscientist 25, 359–379. doi: 10.1177/1073858418807887
Plotnik, M., Giladi, N., Balash, Y., Peretz, C., and Hausdorff, J. M. (2005). Is freezing of gait in Parkinson's disease related to asymmetric motor function? Ann. Neurol. 57, 656–663. doi: 10.1002/ana.20452
Plotnik, M., and Hausdorff, J. M. (2008). The role of gait rhythmicity and bilateral coordination of stepping in the pathophysiology of freezing of gait in Parkinson's disease. Mov. Disord. 23, S444–S450. doi: 10.1002/mds.21984
Postuma, R. B., Berg, D., Stern, M., Poewe, W., Olanow, C. W., Oertel, W., et al. (2015). MDS clinical diagnostic criteria for Parkinson's disease. Mov. Disord. 30, 1591–1601. doi: 10.1002/mds.26424
Powell, D., Muthumani, A., and Xia, R. (2016). A comparison of the effects of continuous versus discontinuous movement patterns on parkinsonian rigidity and reflex responses to passive stretch and shortening. J. Nat. Sci. 2, 1–19.
Powell, D., Threlkeld, A. J., Fang, X., Muthumani, A., and Xia, R. (2012). Amplitude- and velocity-dependency of rigidity measured at the wrist in Parkinson's disease. Clin. Neurophysiol. 123, 764–773. doi: 10.1016/j.clinph.2011.08.004
Radovanovic, D., Peikert, K., Lindstrom, M., and Domellof, F. P. (2015). Sympathetic innervation of human muscle spindles. J. Anat. 226, 542–548. doi: 10.1111/joa.12309
Raethjen, J., Lindemann, M., Schmaljohann, H., Wenzelburger, R., Pfister, G., and Deuschl, G. (2000). Multiple oscillators are causing parkinsonian and essential tremor. Mov. Disord. 15, 84–94. doi: 10.1002/1531-8257(200001)15:1<84::AID-MDS1014>3.0.CO;2-K
Rahman, S., Griffin, H. J., Quinn, N. P., and Jahanshahi, M. (2008). The factors that induce or overcome freezing of gait in Parkinson's disease. Behav. Neurol. 19, 127–136. doi: 10.1155/2008/456298
Rand, M. K., Lemay, M., Squire, L. M., Shimansky, Y. P., and Stelmach, G. E. (2010). Control of aperture closure initiation during reach-to-grasp movements under manipulations of visual feedback and trunk involvement in Parkinson's disease. Exp. Brain Res. 201, 509–525. doi: 10.1007/s00221-009-2064-2
Raudies, F., and Hasselmo, M. E. (2015). Differences in visual-spatial input may underlie different compression properties of firing fields for grid cell modules in medial entorhinal cortex. PLoS.Comput. Biol. 11, 1–27. doi: 10.1371/journal.pcbi.1004596
Redgrave, P., Rodriguez, M., Smith, Y., Rodriguez-Oroz, M. C., Lehericy, S., Bergman, H., et al. (2010). Goal-directed and habitual control in the basal ganglia: implications for Parkinson's disease. Nat. Rev. Neurosci. 11, 760–772. doi: 10.1038/nrn2915
Rehman, R. Z. U., Del Din, S., Guan, Y., Yarnall, A. J., Shi, J. Q., and Rochester, L. (2019). Selecting clinically relevant gait characteristics for classification of early Parkinson's disease: a comprehensive machine learning approach. Sci. Rep. 9:17269. doi: 10.1038/s41598-019-53656-7
Reifenstein, E. T., Kempter, R., Schreiber, S., Stemmler, M. B., and Herz, A. V. (2012). Grid cells in rat entorhinal cortex encode physical space with independent firing fields and phase precession at the single-trial level. Proc. Natl. Acad. Sci. USA 109, 6301–6306. doi: 10.1073/pnas.1109599109
Ridler, T., Witton, J., Phillips, K. G., Randall, A. D., and Brown, J. T. (2020). Impaired speed encoding and grid cell periodicity in a mouse model of tauopathy. elife 9, 1–22. doi: 10.7554/eLife.59045
Rivera-Oliver, M., Moreno, E., Alvarez-Bagnarol, Y., Ayala-Santiago, C., Cruz-Reyes, N., Molina-Castro, G. C., et al. (2019). Adenosine A1-dopamine D1 receptor Heteromers control the excitability of the spinal Motoneuron. Mol. Neurobiol. 56, 797–811. doi: 10.1007/s12035-018-1120-y
Rolland, A. S., Tande, D., Herrero, M. T., Luquin, M. R., Vazquez-Claverie, M., Karachi, C., et al. (2009). Evidence for a dopaminergic innervation of the pedunculopontine nucleus in monkeys, and its drastic reduction after MPTP intoxication. J. Neurochem. 110, 1321–1329. doi: 10.1111/j.1471-4159.2009.06220.x
Roseberry, T. K., Lee, A. M., Lalive, A. L., Wilbrecht, L., Bonci, A., and Kreitzer, A. C. (2016). Cell-type-specific control of brainstem locomotor circuits by basal ganglia. Cell 164, 526–537. doi: 10.1016/j.cell.2015.12.037
Rosen, Z. B., Cheung, S., and Siegelbaum, S. A. (2015). Midbrain dopamine neurons bidirectionally regulate CA3-CA1 synaptic drive. Nat. Neurosci. 18, 1763–1771. doi: 10.1038/nn.4152
Rowland, D. C., Obenhaus, H. A., Skytoen, E. R., Zhang, Q., Kentros, C. G., Moser, E. I., et al. (2018). Functional properties of stellate cells in medial entorhinal cortex layer II. elife 7, 1–17. doi: 10.7554/eLife.36664
Rueckemann, J. W., DiMauro, A. J., Rangel, L. M., Han, X., Boyden, E. S., and Eichenbaum, H. (2016). Transient optogenetic inactivation of the medial entorhinal cortex biases the active population of hippocampal neurons. Hippocampus 26, 246–260. doi: 10.1002/hipo.22519
Ruggiero, G., Iavarone, A., and Iachini, T. (2018). Allocentric to egocentric spatial switching: impairment in aMCI and Alzheimer's disease patients? Curr. Alzheimer Res. 15, 229–236. doi: 10.2174/1567205014666171030114821
Ryan, M. B., Bair-Marshall, C., and Nelson, A. B. (2018). Aberrant striatal activity in parkinsonism and levodopa-induced dyskinesia. Cell Rep. 23, 3438–3446. doi: 10.1016/j.celrep.2018.05.059
Ryckewaert, G., Luyat, M., Rambour, M., Tard, C., Noel, M., Defebvre, L., et al. (2015). Self-perceived and actual ability in the functional reach test in patients with Parkinson's disease. Neurosci. Lett. 589, 181–184. doi: 10.1016/j.neulet.2015.01.039
Ryczko, D., and Dubuc, R. (2017). Dopamine and the brainstem locomotor networks: from lamprey to human. Front. Neurosci. 11:295. doi: 10.3389/fnins.2017.00295
Ryczko, D., Gratsch, S., Auclair, F., Dube, C., Bergeron, S., Alpert, M. H., et al. (2013). Forebrain dopamine neurons project down to a brainstem region controlling locomotion. Proc. Natl. Acad. Sci. USA 110, E3235–E3242. doi: 10.1073/pnas.1301125110
Sargolini, F., Fyhn, M., Hafting, T., McNaughton, B. L., Witter, M. P., Moser, M. B., et al. (2006). Conjunctive representation of position, direction, and velocity in entorhinal cortex. Science 312, 758–762. doi: 10.1126/science.1125572
Savelli, F., Luck, J. D., and Knierim, J. J. (2017). Framing of grid cells within and beyond navigation boundaries. elife 6, 1–29. doi: 10.7554/eLife.21354
Savelli, F., Yoganarasimha, D., and Knierim, J. J. (2008). Influence of boundary removal on the spatial representations of the medial entorhinal cortex. Hippocampus 18, 1270–1282. doi: 10.1002/hipo.20511
Scatton, B., Simon, H., Le Moal, M., and Bischoff, S. (1980). Origin of dopaminergic innervation of the rat hippocampal formation. Neurosci. Lett. 18, 125–131. doi: 10.1016/0304-3940(80)90314-6
Schaafsma, J. D., Balash, Y., Gurevich, T., Bartels, A. L., Hausdorff, J. M., and Giladi, N. (2003). Characterization of freezing of gait subtypes and the response of each to levodopa in Parkinson's disease. Eur. J. Neurol. 10, 391–398. doi: 10.1046/j.1468-1331.2003.00611.x
Schlesiger, M. I., Cannova, C. C., Boublil, B. L., Hales, J. B., Mankin, E. A., Brandon, M. P., et al. (2015). The medial entorhinal cortex is necessary for temporal organization of hippocampal neuronal activity. Nat. Neurosci. 18, 1123–1132. doi: 10.1038/nn.4056
Schwarz, P. B., and Peever, J. H. (2011). Dopamine triggers skeletal muscle tone by activating D1-like receptors on somatic motoneurons. J. Neurophysiol. 106, 1299–1309. doi: 10.1152/jn.00230.2011
Sharples, S. A. (2017). Dopamine pumping up spinal locomotor network function. J. Neurosci. 37, 3103–3105. doi: 10.1523/JNEUROSCI.0019-17.2017
Shay, C. F., Ferrante, M., Chapman, G. W., and Hasselmo, M. E. (2016). Rebound spiking in layer II medial entorhinal cortex stellate cells: possible mechanism of grid cell function. Neurobiol. Learn. Mem. 129, 83–98. doi: 10.1016/j.nlm.2015.09.004
Sidaway, B., Aaroe, A., Albert, M., LePage, K., Desrosiers, G., Keith, M., et al. (2018). Visual detection of affordances for aperture negotiation in people with Parkinson disease. Neuropsychologia 120, 59–64. doi: 10.1016/j.neuropsychologia.2018.10.010
Skaggs, W. E., McNaughton, B. L., Wilson, M. A., and Barnes, C. A. (1996). Theta phase precession in hippocampal neuronal populations and the compression of temporal sequences. Hippocampus 6, 149–172. doi: 10.1002/(SICI)1098-1063(1996)6:2<149::AID-HIPO6>3.0.CO;2-K
Skidmore, F. M., Drago, V., Pav, B., Foster, P. S., Mackman, C., and Heilman, K. M. (2009). Conceptual hypometria? An evaluation of conceptual mapping of space in Parkinson's disease. Neurocase 15, 119–125. doi: 10.1080/13554790802637743
Skoog, B., and Noga, B. R. (1995). Dopaminergic control of transmission from group II muscle afferents to spinal neurones in the cat and guinea-pig. Exp. Brain Res. 105, 39–47. doi: 10.1007/BF00242180
Smith, J. G., Harris, J. P., Khan, S., Atkinson, E. A., Fowler, M. S., Ewins, D., et al. (2011). Motor asymmetry and estimation of body-scaled aperture width in Parkinson's disease. Neuropsychologia 49, 3002–3010. doi: 10.1016/j.neuropsychologia.2011.06.025
Snijders, A. H., Toni, I., Ruzicka, E., and Bloem, B. R. (2011). Bicycling breaks the ice for freezers of gait. Mov. Disord. 26, 367–371. doi: 10.1002/mds.23530
Sodums, D. J., and Bohbot, V. D. (2020). Negative correlation between grey matter in the hippocampus and caudate nucleus in healthy aging. Hippocampus 30, 892–908. doi: 10.1002/hipo.23210
Solstad, T., Boccara, C. N., Kropff, E., Moser, M. B., and Moser, E. I. (2008). Representation of geometric borders in the entorhinal cortex. Science 322, 1865–1868. doi: 10.1126/science.1166466
Souza, B. C., and Tort, A. B. L. (2017). Asymmetry of the temporal code for space by hippocampal place cells. Sci. Rep. 7:8507. doi: 10.1038/s41598-017-08609-3
Spiegel, J., Hellwig, D., Samnick, S., Jost, W., Mollers, M. O., Fassbender, K., et al. (2007). Striatal FP-CIT uptake differs in the subtypes of early Parkinson's disease. J. Neural Transm. (Vienna) 114, 331–335. doi: 10.1007/s00702-006-0518-2
Spildooren, J., Vercruysse, S., Desloovere, K., Vandenberghe, W., Kerckhofs, E., and Nieuwboer, A. (2010). Freezing of gait in Parkinson's disease: the impact of dual-tasking and turning. Mov. Disord. 25, 2563–2570. doi: 10.1002/mds.23327
Stangl, M., Achtzehn, J., Huber, K., Dietrich, C., Tempelmann, C., and Wolbers, T. (2018). Compromised grid-cell-like representations in old age as a key mechanism to explain age-related navigational deficits. Curr. Biol. 28, 1108–1115. doi: 10.1016/j.cub.2018.02.038
Stensola, T., and Moser, E. I. (2016). “Grid cells and spatial maps in entorhinal cortex and hippocampus” in Micro-, Meso- and Macro-Dynamics of the Brain. eds. G. Buzsaki and Y. Christen (Cham (CH): Springer Cham), 59–80.
Stensola, T., Stensola, H., Moser, M. B., and Moser, E. I. (2015). Shearing-induced asymmetry in entorhinal grid cells. Nature 518, 207–212. doi: 10.1038/nature14151
Stensola, H., Stensola, T., Solstad, T., Froland, K., Moser, M. B., and Moser, E. I. (2012). The entorhinal grid map is discretized. Nature 492, 72–78. doi: 10.1038/nature11649
Stewart, S., Jeewajee, A., Wills, T. J., Burgess, N., and Lever, C. (2014). Boundary coding in the rat subiculum. Philos. Trans. R. Soc. Lond. Ser. B Biol. Sci. 369:20120514. doi: 10.1098/rstb.2012.0514
Stoianov, I. P., Pennartz, C. M. A., Lansink, C. S., and Pezzulo, G. (2018). Model-based spatial navigation in the hippocampus-ventral striatum circuit: a computational analysis. PLoS Comput. Biol. 14:e1006316. doi: 10.1371/journal.pcbi.1006316
Suryanarayana, S. M., Hellgren Kotaleski, J., Grillner, S., and Gurney, K. N. (2019). Roles for globus pallidus externa revealed in a computational model of action selection in the basal ganglia. Neural Netw. 109, 113–136. doi: 10.1016/j.neunet.2018.10.003
Swann, N. C., de Hemptinne, C., Miocinovic, S., Qasim, S., Wang, S. S., Ziman, N., et al. (2016). Gamma oscillations in the hyperkinetic state detected with chronic human brain recordings in Parkinson's disease. J. Neurosci. 36, 6445–6458. doi: 10.1523/JNEUROSCI.1128-16.2016
Takeuchi, T., Duszkiewicz, A. J., Sonneborn, A., Spooner, P. A., Yamasaki, M., Watanabe, M., et al. (2016). Locus coeruleus and dopaminergic consolidation of everyday memory. Nature 537, 357–362. doi: 10.1038/nature19325
Tang, Q., Burgalossi, A., Ebbesen, C. L., Ray, S., Naumann, R., Schmidt, H., et al. (2014). Pyramidal and stellate cell specificity of grid and border representations in layer 2 of medial entorhinal cortex. Neuron 84, 1191–1197. doi: 10.1016/j.neuron.2014.11.009
Thaut, M. H., McIntosh, G. C., Rice, R. R., Miller, R. A., Rathbun, J., and Brault, J. M. (1996). Rhythmic auditory stimulation in gait training for Parkinson's disease patients. Mov. Disord. 11, 193–200. doi: 10.1002/mds.870110213
Tinaz, S., Pillai, A. S., and Hallett, M. (2016). Sequence effect in Parkinson's disease is related to motor energetic cost. Front. Neurol. 7:83. doi: 10.3389/fneur.2016.00083
Totterdell, S., and Meredith, G. E. (1997). Topographical organization of projections from the entorhinal cortex to the striatum of the rat. Neuroscience 78, 715–729. doi: 10.1016/s0306-4522(96)00592-1
Tsanov, M. (2017). Speed and oscillations: medial septum integration of attention and navigation. Front. Syst. Neurosci. 11:67. doi: 10.3389/fnsys.2017.00067
Tsao, A., Sugar, J., Lu, L., Wang, C., Knierim, J. J., Moser, M. B., et al. (2018). Integrating time from experience in the lateral entorhinal cortex. Nature 561, 57–62. doi: 10.1038/s41586-018-0459-6
Tukker, J. J., Beed, P., Brecht, M., Kempter, R., Moser, E. I., and Schmitz, D. (2022). Microcircuits for spatial coding in the medial entorhinal cortex. Physiol. Rev. 102, 653–688. doi: 10.1152/physrev.00042.2020
Vago, L., and Ujfalussy, B. B. (2018). Robust and efficient coding with grid cells. PLoS Comput. Biol. 14:e1005922. doi: 10.1371/journal.pcbi.1005922
Valencia, M., Chavez, M., Artieda, J., Bolam, J. P., and Mena-Segovia, J. (2014). Abnormal functional connectivity between motor cortex and pedunculopontine nucleus following chronic dopamine depletion. J. Neurophysiol. 111, 434–440. doi: 10.1152/jn.00555.2013
van der Meer, M. A., Johnson, A., Schmitzer-Torbert, N. C., and Redish, A. D. (2010). Triple dissociation of information processing in dorsal striatum, ventral striatum, and hippocampus on a learned spatial decision task. Neuron 67, 25–32. doi: 10.1016/j.neuron.2010.06.023
van der Meer, M. A., and Redish, A. D. (2011). Theta phase precession in rat ventral striatum links place and reward information. J. Neurosci. 31, 2843–2854. doi: 10.1523/JNEUROSCI.4869-10.2011
van Wijngaarden, J. B., Babl, S. S., and Ito, H. T. (2020). Entorhinal-retrosplenial circuits for allocentric-egocentric transformation of boundary coding. elife 9, 1–25. doi: 10.7554/eLife.59816
Vanderwolf, C. H. (1969). Hippocampal electrical activity and voluntary movement in the rat. Electroencephalogr. Clin. Neurophysiol. 26, 407–418. doi: 10.1016/0013-4694(69)90092-3
Vercruysse, S., Gilat, M., Shine, J. M., Heremans, E., Lewis, S., and Nieuwboer, A. (2014). Freezing beyond gait in Parkinson's disease: a review of current neurobehavioral evidence. Neurosci. Biobehav. Rev. 43, 213–227. doi: 10.1016/j.neubiorev.2014.04.010
Verhagen Metman, L., and Espay, A. J. (2017). Teaching video neuro images: the underrecognized diphasic dyskinesia of Parkinson disease. Neurology 89, e83–e84. doi: 10.1212/WNL.0000000000004238
Vermeiren, Y., and De Deyn, P. P. (2017). Targeting the norepinephrinergic system in Parkinson's disease and related disorders: the locus coeruleus story. Neurochem. Int. 102, 22–32. doi: 10.1016/j.neuint.2016.11.009
Verschure, P. F., Pennartz, C. M., and Pezzulo, G. (2014). The why, what, where, when and how of goal-directed choice: neuronal and computational principles. Philos. Trans. R. Soc. Lond. Ser. B Biol. Sci. 369:20130483. doi: 10.1098/rstb.2013.0483
Virmani, T., Urbano, F. J., Bisagno, V., and Garcia-Rill, E. (2019). The pedunculopontine nucleus: from posture and locomotion to neuroepigenetics. AIMS Neurosci. 6, 219–230. doi: 10.3934/Neuroscience.2019.4.219
Wagle Shukla, A., Ounpraseuth, S., Okun, M. S., Gray, V., Schwankhaus, J., and Metzer, W. S. (2012). Micrographia and related deficits in Parkinson's disease: a cross-sectional study. BMJ Open 2:e000628. doi: 10.1136/bmjopen-2011-000628
Weil-Fugazza, J., and Godefroy, F. (1993). Dorsal and ventral dopaminergic innervation of the spinal cord: functional implications. Brain Res. Bull. 30, 319–324. doi: 10.1016/0361-9230(93)90259-e
Wichmann, T., DeLong, M. R., Guridi, J., and Obeso, J. A. (2011). Milestones in research on the pathophysiology of Parkinson's disease. Mov. Disord. 26, 1032–1041. doi: 10.1002/mds.23695
Wills, T. J., Cacucci, F., Burgess, N., and O'Keefe, J. (2010). Development of the hippocampal cognitive map in preweanling rats. Science 328, 1573–1576. doi: 10.1126/science.1188224
Witter, M. P., Doan, T. P., Jacobsen, B., Nilssen, E. S., and Ohara, S. (2017). Architecture of the entorhinal cortex a review of entorhinal anatomy in rodents with some comparative notes. Front. Syst. Neurosci. 11:46. doi: 10.3389/fnsys.2017.00046
Wu, T., Zhang, J., Hallett, M., Feng, T., Hou, Y., and Chan, P. (2016). Neural correlates underlying micrographia in Parkinson's disease. Brain 139, 144–160. doi: 10.1093/brain/awv319
Xia, R., Powell, D., Rymer, W. Z., Hanson, N., Fang, X., and Threlkeld, A. J. (2011). Differentiation between the contributions of shortening reaction and stretch-induced inhibition to rigidity in Parkinson's disease. Exp. Brain Res. 209, 609–618. doi: 10.1007/s00221-011-2594-2
Xu, Z., Mo, F., Yang, G., Fan, P., Wang, Y., Lu, B., et al. (2022). Grid cell remapping under three-dimensional object and social landmarks detected by implantable microelectrode arrays for the medial entorhinal cortex. Microsyst. Nanoeng. 8:104. doi: 10.1038/s41378-022-00436-5
Yartsev, M. M., and Ulanovsky, N. (2013). Representation of three-dimensional space in the hippocampus of flying bats. Science 340, 367–372. doi: 10.1126/science.1235338
Ye, J., Witter, M. P., Moser, M. B., and Moser, E. I. (2018). Entorhinal fast-spiking speed cells project to the hippocampus. Proc. Natl. Acad. Sci. U.S.A. 115, E1627–E1636. doi: 10.1073/pnas.1720855115
Yeragani, V. K., Tancer, M., Chokka, P., and Baker, G. B. (2010). Arvid Carlsson, and the story of dopamine. Indian J. Psychiatry 52, 87–88. doi: 10.4103/0019-5545.58907
Yoshida, M., and Tanaka, M. (1988). Existence of new dopaminergic terminal plexus in the rat spinal cord: assessment by immunohistochemistry using anti-dopamine serum. Neurosci. Lett. 94, 5–9. doi: 10.1016/0304-3940(88)90261-3
Young, D. E., Wagenaar, R. C., Lin, C. C., Chou, Y. H., Davidsdottir, S., Saltzman, E., et al. (2010). Visuospatial perception and navigation in Parkinson's disease. Vis. Res. 50, 2495–2504. doi: 10.1016/j.visres.2010.08.029
Zach, H., Dirkx, M., Bloem, B. R., and Helmich, R. C. (2015). The clinical evaluation of Parkinson's tremor. J. Parkinsons Dis. 5, 471–474. doi: 10.3233/JPD-150650
Zarow, C., Lyness, S. A., Mortimer, J. A., and Chui, H. C. (2003). Neuronal loss is greater in the locus coeruleus than nucleus basalis and substantia nigra in Alzheimer and Parkinson diseases. Arch. Neurol. 60, 337–341. doi: 10.1001/archneur.60.3.337
Zham, P., Raghav, S., Kempster, P., Poosapadi Arjunan, S., Wong, K., Nagao, K. J., et al. (2019). A kinematic study of progressive Micrographia in Parkinson's disease. Front. Neurol. 10:403. doi: 10.3389/fneur.2019.00403
Zhang, B., and Liu, J. (2023). Hippocampal replay facilitates the formation of entorhinal grid cells. bioRxiv [Preprint]. doi: 10.1101/2023.02.19.529130
Zhong, J. Y., and Moffat, S. D. (2018). Extrahippocampal contributions to age-related changes in spatial navigation ability. Front. Hum. Neurosci. 12:272. doi: 10.3389/fnhum.2018.00272
Zhou, C., Guo, T., Wu, J., Wang, L., Bai, X., Gao, T., et al. (2021). Locus Coeruleus degeneration correlated with levodopa resistance in Parkinson's disease: a retrospective analysis. J. Parkinsons Dis. 11, 1631–1640. doi: 10.3233/JPD-212720
Zhou, T. L., Tamura, R., Kuriwaki, J., and Ono, T. (1999). Comparison of medial and lateral septal neuron activity during performance of spatial tasks in rats. Hippocampus 9, 220–234. doi: 10.1002/(SICI)1098-1063(1999)9:3<220::AID-HIPO3>3.0.CO;2-E
Zhu, H., Clemens, S., Sawchuk, M., and Hochman, S. (2007). Expression and distribution of all dopamine receptor subtypes (D (1)-D (5)) in the mouse lumbar spinal cord: a real-time polymerase chain reaction and non-autoradiographic in situ hybridization study. Neuroscience 149, 885–897. doi: 10.1016/j.neuroscience.2007.07.052
Zilli, E. A. (2012). Models of grid cell spatial firing published 2005-2011. Front. Neural Circuits 6:16. doi: 10.3389/fncir.2012.00016
Keywords: grid cell, Parkinson ‘s disease, allocentric, dopamine, medial entorhinal cortex, striatum, striato-HF/EC loop
Citation: Reinshagen A (2024) Grid cells: the missing link in understanding Parkinson’s disease? Front. Neurosci. 18:1276714. doi: 10.3389/fnins.2024.1276714
Edited by:
Frank Hirth, King's College London, United KingdomReviewed by:
Patrick Santens, Ghent University, BelgiumGiorgio Vivacqua, Campus Bio-Medico University, Italy
Copyright © 2024 Reinshagen. This is an open-access article distributed under the terms of the Creative Commons Attribution License (CC BY). The use, distribution or reproduction in other forums is permitted, provided the original author(s) and the copyright owner(s) are credited and that the original publication in this journal is cited, in accordance with accepted academic practice. No use, distribution or reproduction is permitted which does not comply with these terms.
*Correspondence: Alexander Reinshagen, cmVpbnNoYWdlbmFAb25saW5lLmRl