- 1Center for Medical Genetics and Prenatal Diagnosis, Key Laboratory of Birth Defect Prevention and Genetic Medicine of Shandong Health Commission, Key Laboratory of Birth Regulation and Control Technology of National Health Commission of China, Shandong Provincial Maternal and Child Health Care Hospital Affiliated to Qingdao University, Jinan, Shandong, China
- 2School of Life Science and Technology, Weifang Medical University, Weifang, Shandong, China
- 3Institute for Fetology, The First Affiliated Hospital of Soochow University, Suzhou, Jiangsu, China
Parkinson’s disease (PD) is one of the most common degenerative diseases. It is most typically characterized by neuronal death following the accumulation of Lewis inclusions in dopaminergic neurons in the substantia nigra region, with clinical symptoms such as motor retardation, autonomic dysfunction, and dystonia spasms. The exact molecular mechanism of its pathogenesis has not been revealed up to now. And there is a lack of effective treatments for PD, which places a burden on patients, families, and society. CRISPR Cas9 is a powerful technology to modify target genomic sequence with rapid development. More and more scientists utilized this technique to perform research associated neurodegenerative disease including PD. However, the complexity involved makes it urgent to organize and summarize the existing findings to facilitate a clearer understanding. In this review, we described the development of CRISPR Cas9 technology and the latest spin-off gene editing systems. Then we focused on the application of CRISPR Cas9 technology in PD research, summarizing the construction of the novel PD-related medical models including cellular models, small animal models, large mammal models. We also discussed new directions and target molecules related to the use of CRISPR Cas9 for PD treatment from the above models. Finally, we proposed the view about the directions for the development and optimization of the CRISPR Cas9 technology system, and its application to PD and gene therapy in the future. All these results provided a valuable reference and enhanced in understanding for studying PD.
1. Introduction
Parkinson’s disease (PD) was reported by doctor James Parkinson before 20 decades ago, the symptoms of patients include resting tremor, gait retardation, sleep problems (presence of Paralysis agitans) (Gomperts, 2016). Later, more detailed pathological features were gradually discovered clinically, manifesting as degenerative death of nigrostriatal dopaminergic neurons, a significant decrease in dopamine neuron, and accumulation of Lewy body inclusions in the substantia nigra pars compacta (Damier et al., 1999). These lesions result in the inability of dopamine neurons in the substantia nigra region to transmit dopamine to the striatum via the substantia nigra-striatal pathway. As the second largest neurodegenerative disease in the world, PD has become the fastest growing neurological disease in the world. Its incidence increases with age, with a prevalence of more than 1% in people over 60 years old and 2–3% in those over 65 years old. It is estimated that by 2040, this disease will be expected to affect more than 12 million people (Damier et al., 1999; Dorsey et al., 2018; Dorsey and Bloem, 2018). PD not only brings physical and mental pain to the patient but also imposes a heavy burden to their families and society. Although the pathological diagnosis of PD is relatively clear at the current stage, the pathogenic mechanism of PD is not definite, and exploring the causes of PD has been a focus of neuroscience research for several decades. It is reported that both environmental factors and genetic mutations contribute to the degenerative death process of dopaminergic neurons. Currently, mitochondrial dysfunction, oxidative stress, altered protein processing, and inflammatory changes are considered to be the causes of neuronal dysfunction and death through apoptosis or autophagy (Sankhla, 2017). Aging is the most noticeable risk factor for PD, and the biochemical changes caused by aging amplify these abnormalities in the PD brain (Schapira and Jenner, 2011).
PD can be divided into sporadic and familial types, with the latter accounting for 10–15% (De Plano et al., 2022). Familial PD is usually caused by mutations in PD-related genes, including SNCA (Puschmann, 2013), Parkin (Gao and Hong, 2011; Puschmann, 2013), PINK1 (Crosiers et al., 2011), DJ-1 (Blauwendraat et al., 2020), LRRK2 (van der Vegt et al., 2009), ATP13A2 (Crosiers et al., 2011), and so on. Some PD-related genes have been identified via multiple clinical cases. Nevertheless, the pathogenesis of PD is not clarified up to now. Recently, the rapid development of CRISPR Cas9 and related gene editing technology has enabled humans to explore the relationship between genes and diseases more precisely, with more and more worldwide applications in neurodegenerative diseases such as PD. In this review, a detailed introduction to the detail and development of CRISPR Cas9, the construction of PD-related animal models, and the therapeutic methods for PD via CRISPR Cas9 and related technologies will be provided.
2. CRISPR Cas9 technology
2.1. Discovery and working principle of CRISPR Cas9 gene editing technology
Clustered Regularly Interspaced Short Palindromic Repeats (CRISPR) sequences were firstly found in bacteria (Ishino et al., 1987) and definitely designation in 2002 (Jansen et al., 2002). However, scientists confirmed that CRISPR sequence functions to benefit bacteria to resist viral infections in 2007 (Barrangou and Horvath, 2017). The CRISPR family contains two main types of systems, which includes several Cas proteins. The first type of system is usually found in bacteria and archaea, such as I, III, and IV Cas proteins, which function by forming multi-subunit protein-crRNA (CRISPR RNA) effector complex. The second type of system contains II, V, and VI types, which could perform target editing relying on a single crRNA-guided protein, i.e., a single multidomain protein that exercises function (Makarova et al., 2011). Herein, the second CRISPR system is more convenient to carry out the gene editing. The detail reported Cas proteins in the second system is listed in Table 1. CRISPR Cas9 is the earliest, most widespread, and the most mature technology among second CRISPR system (Makarova et al., 2017). The CRISPR Cas9 technology includes a small guide RNA (sgRNA) used to target the desired DNA molecule and Cas9 protein, a non-specific CRISPR nuclease which could cleave double-stranded DNA molecules. The Cas9 protein has two cleavage-active domains: the HNH which cleaves the DNA strand complementary to the crRNA, and RuvC domains which cleaves the non-complementary strand (Lu et al., 2021). sgRNA is composed of a trans-activating crRNA (tracrRNA) sequence that can bind to the Cas9 protein and a crRNA containing a specific sequence with about 20 nt in length that is complementary to the target sequence, and the remaining sequence of crRNA complementary to tracrRNA. Therefore, this technology searches for the motif sequence complementary to the crRNA of gRNA in the target DNA molecule with a PAM sequence behind it. Subsequently, Cas9 protein cleaves this motif and results in the formation of double-strand breaks (DSB). Afterwards, knock-out and knock-in occur via non-homologous end joining and homologous recombination in the presence of the exogenous donor sequence in cell (Figure 1A), respectively (Kaulich et al., 2015).
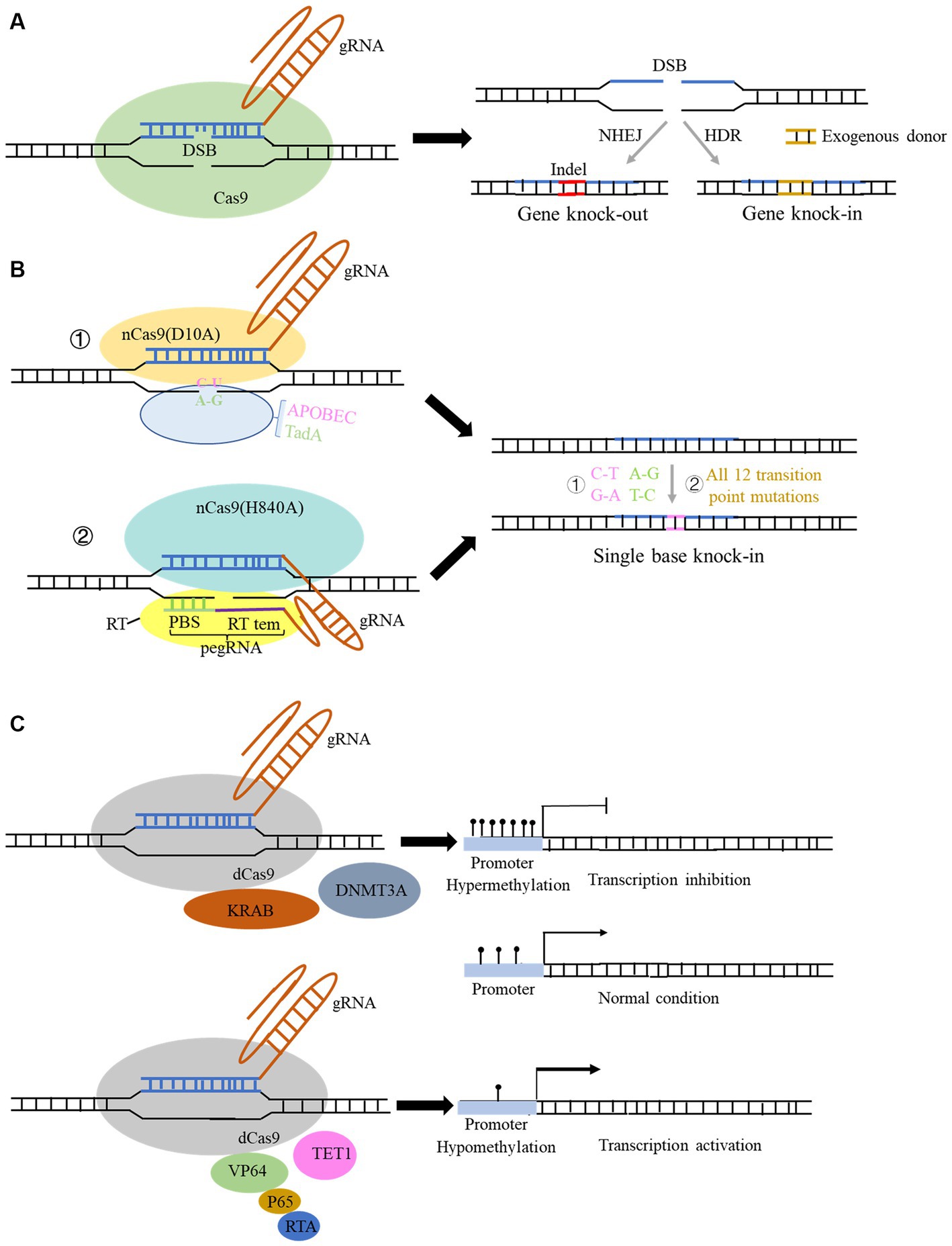
Figure 1. Structure and function of the CRISPR Cas9 and spin-off technology system. (A) Structure of the typical CRISPR Cas9 system. Genome editing using this system can be used for gene knock-out and gene knock-in with donor sequence. (B) Structure and function of derived CRISPR Cas9 system for single-base editing: ① cytidine base editor (CBE) could change base C to U, and adenine base editor (ABE) could change base A to G; ② prime editor (PE) could achieve all 12 transition point changes. (C) Structure of the derived CRISPR Cas9 technology for gene expression modulation: fusion of some transcriptional repressors or methylation transferase with dCas9 protein can repress the transcription of genes, and conversely, fusion of some transcriptional activators and demethylases with dCas9 protein can activate the gene expression.
2.2. The evolution of CRISPR Cas9 technology
CRISPR Cas9 and related CRISPR Cas systems have been continuously optimized since their emergence, with three main areas: (1) achieving more precise single base editing, (2) reducing off-target effects, and (3) improving editing efficiency. As for the optimization of its delivery approach, the main focus is to find smaller and more portable Cas proteins, which can be effectively packaged using related lentiviral or AAV vectors and more conducive to entering into cells. To reduce off-target efficiency, scientists found that converting one of the two key amino residues in RuvC I of RuvC to alanine (D10A or H840A) could produce Cas9 nickase (Cas9n). This nickase can only cleave the DNA chain that is complementary to the crRNA and cannot cleave the non-complementary DNA chain, thus reducing off-target effects and maintaining high-efficiency gene editing. Furthermore, if the RuvC catalytic domain is mutated by point mutation (D10A) and the HNH catalytic domain is mutated by point mutation (H840A), the Cas9 protein will completely lose its nuclease activity and form dead Cas9 (dCas9), which can only bind to the target gene under the guidance of sgRNA (Qi et al., 2013).
Based on the above findings, Liu et al. fused the cytidine deaminase with nCas9 or dCas9 protein, successfully converting cytidine C to uridine U, and achieved C-to-A and corresponding G-to-T conversion in DNA replication, which was termed as cytidine base editor (CBE). Later, CBE was continuously optimized to enable efficient and permanent C:G to T:A base pair conversion in bacteria, yeast, plants, zebrafish, mammalian cells, mice, and even human embryos (Komor et al., 2016, 2017; Kim et al., 2017). Likewise, by fusing the modified adenine deaminase with nCas9 protein, the system is capable of converting adenine (A) to inosine (I) on ssDNA, and inosine (I) is recognized and read as guanine (G) during DNA replication, ultimately achieving direct substitution of A: T to G:C base pairs. This system is termed as adenine base editor (ABE) (Gaudelli et al., 2017; Komor et al., 2018; Figure 1B). The application of combination of CBE and ABE can effectively perform transition of four types of bases, whereas it is still unable to achieve transversion of the other eight types of bases as well as bases insertion and deletion. Liu team then developed the prime editor (PE) which can achieve change of 12 types situation and precise insertion (up to 44 bp) and deletion (up to 80 bp) of bases without relying on DSB and donor sequence (Anzalone et al., 2019; Figure 1B). In this system, a pegRNA was added to the 3′ end of the sgRNA, which can complement the broken target DNA 3′ end to initiate the reverse transcription. It also contains target point mutations or insertion–deletion mutations to achieve precise base editing (Figure 1B). Later, many studies innovated and optimized single-base editing, and three teams successfully achieved simultaneous CBE and ABE in a single gene editing, which develop a new double-base gene editor (Grünewald et al., 2020; Sakata et al., 2020; Zhang et al., 2020). Yang et al. recently developed a new adenine base editor (AYBE), which can firstly achieve the transversion of adenine (Tong et al., 2023b).
The spin-off technologies of the CRISPR system are not only focused on the optimization of editing DNA bases, but in the regulation of gene expression without changing the genome sequence. DNA editing directly modifies genome sequence permanently and has potential off-target probability, which poses serious clinical application risks. While the regulation of gene expression is usually mild and reversible, and its application in disease treatment can also make up for the shortcomings of DNA editing. The existing CRISPR systems for regulating gene expression include RNA editing systems of Cas13-related proteins (Table 1) and DNA modification regulation systems in which dCas9 protein is coupled with various regulatory factors (Figure 1C). Binding dCas9 to the transcription start site (TSS) of a gene can block the start of transcription, thereby inhibiting gene expression (Figure 1C). Binding dCas9 coupled with transcriptional suppressors or activators can inhibit or activate downstream target gene transcription, respectively (Sen and Thummer, 2022). Fusing some methyltransferases and demethylases to dCas9 protein can selectively regulate the DNA methylation of gene promoter, regulating gene expression (Figure 1C). Cas 13 family is an RNA-dependent RNA endonuclease (Table 1). It can specifically cleave the target RNA to inhibit gene expression in the presence of a PFS sequence in target RNA. Cas13 proteins mainly includes four subtypes, namely CRISPR-Cas13a, b, c, d (Abudayyeh et al., 2017). Among them, CasRx (RfxCas13d) has received widespread attention for its higher efficiency, lower off-target rate. More importantly, Cas13d has a smaller size compared to other family members, which can be more easily packaged into viral vectors, thus having better delivery advantages and application prospects (Kushawah et al., 2020). Cas13d has been reported to achieve effective gene silencing in mouse liver (He et al., 2020). However, research has also found that the CRISPR-Cas13 editing system has significant collateral degradation effects while cleaving RNA sequences, that is, after Cas13 binds to the target RNA and is activated, it can degrade bystander RNAs to some extent. To address this problem, Yang et al. designed a dual fluorescence reporting system to detect collateral effects in mammalian cells and seek for Cas13 variants (Tong et al., 2023a). The team found that the mutated Cas13 variants Cas13d-N2V8 and Cas13X-M17YY had virtually eliminated collateral effects when editing RNA. In addition, researchers have also discovered two new proteins in the Cas13 protein family, termed as Cas13x and Cas13y, and developed a new RNA editing technology based on them. CRISPR Cas13x and CRISPR Cas13y have stronger knockdown activity than previous Cas13a and Cas13b system, and some derived truncated types can achieve RNA single-base editing after fusion with RNA single-base enzymes (Xu et al., 2021; Table 1). Yang et al. also developed a new CRISPR-Cas12f system (enOsCas12f1 and enRhCas12f1) in mammalian cells with high activity, broad targeting range, and high fidelity. After removing the cleavage activity, denOsCas12f1 is an epigenetic editor and gene expression activator (Kong et al., 2023), which shows strong regulatory activity in mammalian cells.
3. Pathogenic insights from PD-related models
Although the pathogenesis of PD is not yet understanding, the phenotype is clear. It is not possible to study the pathogenesis of PD and observe the disease process in humans due to the limitations of medical ethics and experimental risks. It is urgent to simulate the pathogenic biological PD model from the perspective of the pathogenesis and development process of the disease. In the next part, we will introduce the pathogenic insights from PD-related models including cells, small model animals, and large mammal animals (Table 2).
3.1. Construction of PD models from cell-level
Some studies have constructed models by induced pluripotent stem cells (iPSCs) from somatic cells of PD patients with clear genetic mutations that cause the disease. The iPSCs were differentiated into neural stem cells which can simulate the relevant phenotypes of neuronal cells in the brain of PD patients. Mutations in some genes have been found in both familial and sporadic PD cases, such as the LRRK2, SNCA, and PARKIN genes (Satake et al., 2009; Simón-Sánchez et al., 2009; Nalls et al., 2011). Therefore, using cell models with these genetic mutations is more likely to reveal the pathogenesis of PD. Researchers found that neural stem cells differentiated from iPSC cells of patient with LRRK2 p.G2019S mutation showed increased susceptibility to proteasome stress, and had transgenerational defects in nuclear envelope organization, clonal expansion, and neuronal differentiation (Liu et al., 2012). Subsequently, using knock-in technology to change the point mutation to wild-type, the above phenotype can be reversed, which proposed that nuclear morphology alteration as a clinical diagnostic feature of PD. Further research found that LRRK2 forms a complex with a mitochondrial outer membrane protein Miro in iPSC cells, which then removes Miro. Once the removal of Miro is affected in LRRK2 p.G2019S mutation cells, it will delay mitochondrial autophagy, impairing cell respiration and metabolism (Hsieh et al., 2016). Mitochondrial dysfunction is a key aspect of Parkinson’s disease, and mutations in the PRKN/PARK2 gene have been reported to be associated with early-onset familial PD. This gene can regulate mitochondrial function and autophagy processes (Narendra et al., 2008). Researchers edited iPSCs cells from two PD patients with PARK2 gene mutations and found that neurons differentiated from iPSCs showed increased oxidative stress and enhanced Nrf2 pathway activity. In addition, neural cells showed abnormal mitochondrial morphology and impaired mitochondrial homeostasis (Imaizumi et al., 2012). In another study, scientists identified multiple pathogenic mutations in UQCRC1 in both familial and sporadic PD patients. After knock-in of these mutations in human dopaminergic SH-SY5Y cell lines, axonal degeneration and mitochondrial respiratory chain dysfunction were found (Lin et al., 2020).
3.2. Construction of PD models with small model animals
PINK1 and Parkin form a central signaling axis that plays an important role in controlling the mitochondrial autophagy process in dopaminergic neurons. In pink1 null Drosophila, knocking down UCHL1 gene using RNAi can rescue the PD-related pathogenesis. Specifically, the loss of UCH deubiquitination promotes mitochondrial autophagy by activating the expression of AMPK and ULK1 (Ham et al., 2021). In Drosophila, researchers used CRISPR-Cas9 to knock out tango14, the homologous gene of NUS1, and found that the lifespan of Drosophila was shortened, and cholesterol accumulation appeared in dopaminergic neurons, which confirms that this gene is associated with the occurrence of PD due to lipid metabolism abnormalities (Xue et al., 2022).
In zebrafish, researchers constructed a dj-1 null strain targeting exon 1 using CRISPR Cas9. It was found that dj-1−/− zebrafish developed normally in the early stages, but showed lower levels of tyrosine hydroxylase, skeletal muscle respiratory failure, and lower body weight as development progressed (Edson et al., 2019). Proteomic analysis of the brains from dj-1−/− zebrafish revealed downregulation of proteins related to mitochondrial metabolism, autophagy, stress response, redox regulation, and inflammatory response. Then, researchers developed a new, unbiased computational method to classify the movement disorders of adult dj-1−/− zebrafish (Hughes et al., 2020). Mitochondrial calcium uniporter (MCU) participates in mitochondrial dysfunction and cell death caused by excitotoxicity, inflammation, and oxidative stress by regulating mitochondrial calcium uptake, and plays an important role in PD (Liao et al., 2017). Soman et al. generated a zebrafish model with double knockout of mcu and pink1 using the CRISPR-Cas9 system. Compared with pink1−/− zebrafish, (pink1; mcu)−/− zebrafish showed a higher number of dopaminergic neurons and a higher mitochondrial membrane potential. In addition, mitochondrial sphericity was restored, and animals were protected from PD MPTP neurotoxin. Therefore, mcu may be an effective target for the treatment of PD (Soman et al., 2019).
Mutations in GTP cyclohydrolase 1 (GCH1) may lead to the development of PD. In zebrafish, after knocking out gch1 using CRISPR Cas9 technology, zebrafish showed monoamine neurotransmitter defects, movement defects at 8 days post fertilization (dpf), and death at 12 dpf. Tyrosine hydroxylase (Th) protein was up-regulated, but there was no loss of dopaminergic (DA) neurons (Larbalestier et al., 2022). ATP12A2 is an autosomal recessive pathogenic gene for juvenile PD, also known as Kufor-Rakeb syndrome. Atp13a2−/− zebrafish were also established by CRISPR Cas9 gene editing. The number of TH+ neurons in the posterior lobe and the locus coeruleus of atp13a2−/−zebrafish was significantly reduced, indicating dopaminergic neuron degeneration, lysosomal dysfunction, and intracellular transport disorders (Nyuzuki et al., 2020). PARL, which encodes presenilin-associated rhomboid-like protein (PARL), has been found to contribute to mitochondrial morphology, function and is associated with familial PD. PARL is mitochondrial inner membrane protease that acts on many mitochondrial proteins involved in mitochondrial morphology, apoptosis, and mitophagy. After knocking out this gene in zebrafish using CRISPR Cas9, dopamine neurons were lost in the brain, and tyrosine hydroxylase transcription levels decreased, leading to impaired olfaction and decreased motor parameters in zebrafish (Merhi et al., 2021).
Mouse has been widely used to construct models related to PD. Researchers have generated a model called MCI-Park model in mice by CRISPR Cas9 to produce dopamine neurons that lack NDUSF2, which encodes mitochondrial complex I. Mice lacking NDUSF2 showed neurodegenerative changes (González-Rodríguez et al., 2021). Point mutations in the vacuolar protein sorting 35 gene (VPS35) are associated with an autosomal dominant late-onset PD (PARK17). Homozygous deletion of Vps35 by CRISPR Cas9 resulted in survival disadvantage and significantly reduced DA release in the caudate putamen of adult homozygous Vps35 mutant mice (Ishizu et al., 2016). Cyclin-dependent kinase 5 (CDK5) negatively regulates dopamine signaling in the striatum, playing a critical role in circadian rhythm disruption and sleep disorders. After knocking out Cdk5 using CRISPR Cas9, mice showed defects in motor activity and interference with activity/rest behavior, and the number of dendritic length and functional synapses in the mouse brain were down-regulated (Zhou et al., 2022). Peroxidized phospholipids caused by ferroptosis have been reported to be associated with the onset of some PD cases. PNPLA9, as a hydrolytic enzyme, can preferentially hydrolyze peroxidized phospholipids. In mice, knocking out Pnpla9 resulted in progressive PD motor disorders and accumulation of peroxidized phospholipids (Sun et al., 2021). Although gene editing of several genes can simulate some symptoms of PD, editing classic genes related to PD has not been successful in mice. Even if simultaneously knocking out three hot genes related to PD, including Prkn/Pink1/Dj-1, no neurodegenerative phenotype was observed in mice, even in older mice (Kitada et al., 2009). This suggests that these hot genes related to PD may have functional differences in the nervous systems between humans and mice.
3.3. Construction of PD models with large mammal animals
In spite of the fact that gene editing in small rodents is convenient, it is difficult to reproduce significant neurodegeneration including loss of neurons as PD patients (Deng and Siddique, 2000; Deng et al., 2018). Therefore, it is not conductive to using mice to find therapeutic methods for PD. Recently, some large animal models of PD have been successfully constructed (Yang W. et al., 2021). These animal models are able to better simulate the phenotype of human PD, providing new insights into the pathogenesis of PD. Although the emotional and cognitive abilities of pigs are not as similar to those between monkeys and humans, their brain structure is roughly similar to that of humans. In addition, pigs reach sexual maturity (around 6 months) earlier than monkeys, have a shorter gestation period (about 4 months), and give birth to over 10 offspring in one litter. Endogenous genes in pigs can be edited through CRISPR Cas9 combined with somatic cell nuclear transfer (SCNT) to produce knock-out or knock-in models. All of these advantages make pigs a promising alternative large animal model for investigating human diseases (Lunney et al., 2021). Researchers used CRISPR Cas9 and SCNT technology to establish a Bama miniature pig models with Snca missense mutations (p.E46K, p.H50Q, p.G51D). However, PD-specific phenotype was not present such as immunopositivity for SNCA and loss of dopamine neurons in the substantia nigra (Zhu et al., 2018). TALEN combined with SNCA was utilized to construct Park7 knock-out pigs, these pigs were all dead after birth and DJ-1 protein was found significantly inhibited in various tissues (Yao et al., 2014). Another study used CRISPR Cas9 combined with SCNT to prepare Park2 and Pink1 double knock-out pigs, however, like the mouse model, the double knock-out pigs also showed no clinical signs of PD at 7 months old (Zhou et al., 2015). Wang et al. (2016) generated triple knock-out (Parkin, Dj-1, and Pink1) Bama miniature pigs, but the piglets did not exhibit the PD clinical phenotypes at 10 months of age. Considering the higher similarity of brain structure between monkey and human, gradual studies have been conducted using monkey as PD models in recent years. Li et al. performed the first knock-out of pink1 in monkey and found that knocking out pink1 gene resulted in part of monkey died and remaining monkeys displayed severe degeneration and death of neural cells in the brain (Yang et al., 2019a,b). Subsequently, researchers found Pink1 genes was expressed at a very low level in mice, whereas at a high level in primate brain tissues, suggesting it is unique to human or primates. This may explain the reason behind the inconsistent results of gene editing of Pink1 in mouse and monkey. Moreover, Pink1 was found not aggregating in mitochondria, but rather in the cytoplasm. Under non-stressful conditions, Pink1 functions to phosphorylate relevant neuronal proteins in the brain, protecting neurons from damage (Yang et al., 2022). It challenged the previous notion that Pink1 mutation causes PD due to mitochondrial dysfunction and autophagy blockade, suggesting the importance of developing drugs targeting protein phosphorylation in treating PD due to Pink1 mutation. Chen et al. developed a modified and optimized CRISPR Cas9n to knock out Pink1 gene in embryonic cells of Cynomolgus monkey. But the monkeys did not show a phenotype of neurodegenerative disease (Chen et al., 2021). Nevertheless, this study did not detect the expression of Pink1 in the brain, so it is not clear whether the lack of effect is due to editing heterozygosity. In another research, stereotactic injection of AAV to deliver CRISPR Cas9 system targeting Pink1 and Dj-1 to the monkey brain, severe loss of dopaminergic neurons in the substantia nigra and accumulation of a-synuclein pathology were observed (Li et al., 2021). Hence, once the expression of Pink1 in the brain, especially in the substantia nigra, is knocked out in monkeys, the development of loss of dopamine neurons in the substantia nigra should be a clear consequence.
4. Attempts and effects of CRISPR Cas9 in treating PD
According to the reported literature, the use of gene editing technology for the treatment of PD can be mainly divided into two categories: one is intervention and treatment during the formation process of PD, and the other is conversion of other types of neural cells into dopaminergic neurons (Kantor et al., 2018; Zhou et al., 2020). Intervention in the formation of PD is mainly carried out through editing of genes related to mitochondrial damage and autophagy, SNCA accumulation, and stabilization of oxidative phosphorylation (Figure 2).
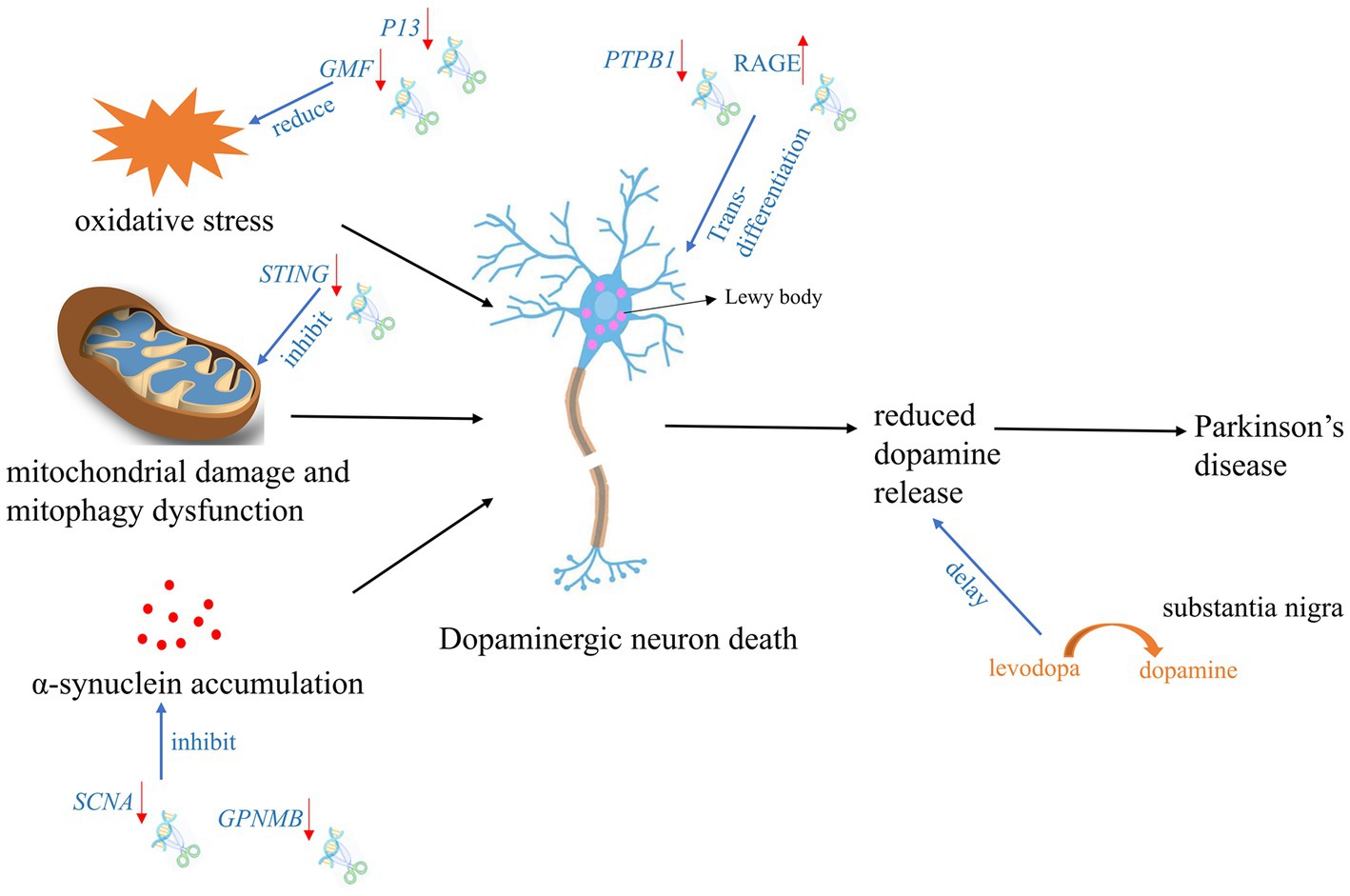
Figure 2. Targeted gene therapy using CRISPR Cas9 and spin-off technologies during PD development process. The red arrow indicates the expression tread of the target gene after gene editing.
4.1. Results in vitro cell lines
Scientists have designed a one-step lentiviral vector targeting Snca intron 1, which fused the catalytic domain of DNA methyltransferase 3A (DNMT3A) with dCas9 to carry out gene regulation (Wüllner et al., 2016; Kantor et al., 2018). Application of this system to hiPSC-derived dopaminergic neurons from PD patients with SNCA triploidy resulted in a fine down-regulation of SNCA mRNA and protein. Furthermore, reduced SNCA protein level by CRISPR-dCas9-DNMT3A system rescued disease-related cellular phenotypic features of SNCA triplicated dopaminergic neurons, such as mitochondrial ROS production and low cell viability (Wüllner et al., 2016). This suggested that combining the regulatory region sequences of genes with CRISPR-dCas9 technology may become a new epigenetic-based treatment method for PD. Apart from iPSC cells, human embryonic stem cells (hESCs) have also been used for research. Chen et al. have used CRISPR-Cas9n editing to remove SNCA gene from hESCs. These stem cells were then able to be converted into dopamine-producing neuronal cells in vitro, and were sensitive to the pS129-αSyn positive SNCA protein aggregates without forming toxic clumps. This study may benefit young patients with PD and patients with aggressive PD (Chen et al., 2019).
In BV2-G cells, knocking out of GMF significantly reduced oxidative stress by reducing ROS production and calcium flux. Furthermore, GMF deficiency significantly reduced nuclear translocation of NRF2, which regulates HO-1 and ferritin activation, the expression of cyclooxygenase 2 (COX2) and nitric oxide synthase 2 (NOS2) in BV2 microglia. GMF may regulate iron metabolism by regulating NRF2-HO1 and ferritin expression, thereby mediating protein aggregation in microglial cell homeostasis associated with progression of PD (Selvakumar et al., 2019).
4.2. Results in animal models
In a PD rat model with overexpression of SNCA (p. A53T), the significant rescue of α-synuclein overexpression, reactive astrogliosis, dopamine neuron degeneration, and Parkinsonian motor deficits was observed after knocking out SNCA gene using CRISPR Cas9 (Yoon et al., 2022). GWAS analysis of the chromosome 7p locus associated with SNCA identified an interaction between non-metastatic melanoma protein B (GPNMB) and SNCA. It furtherly showed that absence of GPNMB resulted in the loss of the ability to internalize α-synuclein fibrils and develop α-synuclein pathology in iPSC-derived neurons (Diaz-Ortiz et al., 2022). In addition, compared to 59 healthy individuals, GPNMB was elevated in the plasma of 731 Parkinson’s patients. And these Parkinson’s patients had more severe symptoms, indicating that GPNMB is a novel PD risk and biomarker, and may serve as a potential target for PD treatment.
P13 is a novel protein involved in mitochondrial oxidative phosphorylation and its overexpression can induce mitochondrial dysfunction and cell apoptosis. Knocking out P13 can alleviate toxin-induced mitochondrial dysfunction and apoptosis in dopaminergic SH-SY5Y cells. Furthermore, researchers constructed a mouse strain with heterozygous deletion of P13 and found that heterozygous knock-out of P13 prevented toxin-induced motor deficits and loss of dopamine neurons in the substantia nigra (Inoue et al., 2018). These results indicated that the regulation of P13 may be a new target for therapeutic interventions in PD. In Prkn−/− mice, exhaustive exercise leaded to inhibition of mitochondrial autophagy and a significant increase in the levels of inflammatory factors in the serum. Notably, knocking out STRING gene via CRISPR Cas9, the phenotype can be rescued (Sliter et al., 2018), which suggested that STRING is a target gene that regulates mitochondrial stability in PD’s patients with PRKN mutation. After knocking out NDUSF2 which is responsible for formation of mitochondrial complex I, mitochondria were damaged but neurons remained intact for a relatively long time in mice. Further studies revealed that these neurons could release dopamine through the cytosol and dendrites in the nigrostriatal fraction to maintain specific motor functions, despite the absence of dopamine in the striatum (González-Rodríguez et al., 2021). Researchers subsequently designed gene therapies targeting the substantia nigra, which enables neurons in the substantia nigra to convert levodopa to dopamine, these facilitates improved treatment with levodopa in the advanced stages of PD.
4.3. Results of cell trans-differentiation
Recently, some teams have proposed an ideal strategy for endogenous neuronal regeneration via in situ trans-differentiation of glial cells into neurons. Using CRISPR Cas9, cord blood-derived mesenchymal stem cells (UCB-MSC) could secret soluble RAGE. After transplantation of these UCB-MSC into striatum of PD mice, the mice showed a significant reduction in neuronal cell death and increased motility in striatum and substantia nigra (Lee et al., 2019). Qian et al. (2020) and Zhou et al. (2020) treated PD model mice by editing PTBP1 using shRNA and CRISPR CasRx system, respectively. After suppressing the expression of PTBP1 in astrocytes, these cells can efficiently trans-differentiate in situ into functional neurons within weeks to months, which improves the motor function of PD mouse models. While a recent repeat experiment of knocking down PTBP1 with shRNA packaged by AAV did not obtain the similar results. Neither signs of trans-differentiation of astrocytes to neurons nor increasing the number of neurons or decreasing the number of astrocytes was observed over half a month to 3 months. Furthermore, down-regulation of PTBP1 in astrocytes did not improve cognitive function, reduce synaptic damage and Aβ/tau pathology in AD model mice (Guo et al., 2022). This suggested the demand for a more systematic, rigorous and stable strategy for future research on trans-differentiation of central nervous system (CNS) cells.
5. Limitations and improvement of CRISPR Cas9 technology
Although CRISPR Cas9 has been studied in some model animals and provided new insights into the mechanisms of diseases, there are still limitations and areas for improvement. Constructing non-human primate models with CRISPR Cas9 and spin-off gene editing technologies is a trend in studying the neural system PD in the brain, but the high cost of materials, long breeding cycles, and difficulty of gene editing operations make it difficult to form large-scale studies like mice. In addition, CRISPR Cas9 technology is prone to mosaic individuals, which can be eliminated in hybrid offspring but it can be time-consuming. Meanwhile, the current CRISPR Cas9 system still suffers from low gene editing efficiency and the presence of off-target effects (Zhang et al., 2015; Liu et al., 2018). Several studies have explored to improve the gene editing efficiency of CRISPR Cas9 on various parameters, such as controlling the GC content to 40–60% (Ren et al., 2019) and using multiple sgRNAs targeting the same gene (Lin et al., 2014). In addition, researchers have designed several variants of Cas9 protein to down-regulate off-target effects, such as HypaCas9, nSpCas9, snipper-Cas9, xCas9, etc. (Chen et al., 2017; Naeem et al., 2020). At the same time, the optimization of CRISPR delivery system is also an issue that must be improved to bring it to clinical application. The current delivery system of CRISPR cas9 is divided into physical delivery, chemical delivery, and viral delivery methods. Physical delivery includes electrotransfection, microinjection, and stereotaxic injection, which have the advantage of not integrating exogenous genomic material into the host cell and low immunogenicity (Han et al., 2020), but the technique is unable to edit all the histiocyte (Li et al., 2020). Calcium phosphate and liposome mediated transfection have the same advantages as physical methods. Nevertheless, chemical delivery methods are also not applicable to whole organisms and delivery efficiency is low (Han et al., 2020). Viral delivery systems, combining adenoviruses, lentiviruses, and adeno-associated viruses, are highly efficient, persistent and can infect dividing and nondividing cells (Yang Y. et al., 2021). However, their common drawbacks are limited packaging capacity and existing immunogenicity (Han et al., 2020). Furthermore, lentiviruses are not suitable for in vivo gene therapy due to integration of host and exogenous nucleic acid. Recently, novel nanoparticles have emerged as delivery tools for in vivo gene therapy (Borbolla-Jiménez et al., 2021). To date, nanoparticles in CRISPR delivery possess some advantages including easy synthesis, high efficiency, low cost, adjustable size, non-mutagenicity, and non-immunogenicity (Yan et al., 2021).
6. Conclusion and prospectives
The manipulation CRISPR Cas9 and derivative technologies to study PD diseases has now become a common strategy, which can be applied to construct gene editing cellular and animal models. These models can be used not only to verify the pathogenicity of PD-related disease causative genes, but also to find appropriate interventions and therapeutic approaches. In this review, we first introduced the development process of CRISPR Cas9 and derivative technologies, among which, we believe that CRISPR dCas9 and CRISPR Cas13 series may be more promising for human gene editing therapy in the future. Because the former does not cut the DNA strand, and the latter only cuts RNA strand, both are relatively safe. Subsequently, we introduced PD-related models including in vitro cell and various animal models constructed using CRISPR Cas9 and spin-off technologies. Among these models, large animal models especially non-human primate models of PD pathogenicity show more advantages in terms of efficiency and success in mimicking human PD manifestations and seeking for the therapeutic methods. More non-human primate models of PD will be constructed, and the improvement of CRISPR Cas9 technology, including the reduction of vector size and off-target rate, the improvement of cutting efficiency and the optimization of delivery system, are the aspects that need to be improved in the future. Our view is that in the future, it would be more meaningful to inject optimized CRISPR Cas9 systems wrapped in nanomaterials or adenoviruses into specific brain regions in non-human primates using stereotactic injection techniques. On the one hand, this method reduces the time from editing the fertilized egg to obtaining the phenotype, and on the other hand, direct injection attempts into brain regions have greater clinical relevance, considering that some clinical experiments have successfully injected dopamine or nutrient factors into specific brain regions of PD patients with good results. Therefore, improving the delivery efficiency of the CRISPR Cas9 system and reducing the negative effects on the brain in optimized stereotactic injection experiments will be important aspects to its application in gene-editing for PD treatment.
Author contributions
JQ wrote the manuscript with support from NL, LG, JH, MS, and DY. All authors contributed to the article and approved the submitted version.
Funding
This work was funded by projects of medical and health technology development program in Shandong province (202201030960).
Conflict of interest
The authors declare that the research was conducted in the absence of any commercial or financial relationships that could be construed as a potential conflict of interest.
Publisher’s note
All claims expressed in this article are solely those of the authors and do not necessarily represent those of their affiliated organizations, or those of the publisher, the editors and the reviewers. Any product that may be evaluated in this article, or claim that may be made by its manufacturer, is not guaranteed or endorsed by the publisher.
References
Abudayyeh, O. O., Gootenberg, J. S., Essletzbichler, P., Han, S., Joung, J., Belanto, J. J., et al. (2017). RNA targeting with CRISPR-Cas13. Nature 550, 280–284. doi: 10.1038/nature24049
Anzalone, A. V., Randolph, P. B., Davis, J. R., Sousa, A. A., Koblan, L. W., Levy, J. M., et al. (2019). Search-and-replace genome editing without double-strand breaks or donor DNA. Nature 576, 149–157. doi: 10.1038/s41586-019-1711-4
Barrangou, R., and Horvath, P. (2017). A decade of discovery: CRISPR functions and applications. Nat. Microbiol. 2:17092. doi: 10.1038/nmicrobiol.2017.92
Blauwendraat, C., Nalls, M. A., and Singleton, A. B. (2020). The genetic architecture of Parkinson's disease. Lancet Neurol. 19, 170–178. doi: 10.1016/s1474-4422(19)30287-x
Borbolla-Jiménez, F. V., Del Prado-Audelo, M. L., Cisneros, B., Caballero-Florán, I. H., Leyva-Gómez, G., and Magaña, J. J. (2021). New perspectives of gene therapy on polyglutamine spinocerebellar ataxias: from molecular targets to novel nanovectors. Pharmaceutics 13:1018. doi: 10.3390/pharmaceutics13071018
Chen, J. S., Dagdas, Y. S., Kleinstiver, B. P., Welch, M. M., Sousa, A. A., Harrington, L. B., et al. (2017). Enhanced proofreading governs CRISPR-Cas9 targeting accuracy. Nature 550, 407–410. doi: 10.1038/nature24268
Chen, Y., Dolt, K. S., Kriek, M., Baker, T., Downey, P., Drummond, N. J., et al. (2019). Engineering synucleinopathy-resistant human dopaminergic neurons by CRISPR-mediated deletion of the SNCA gene. Eur. J. Neurosci. 49, 510–524. doi: 10.1111/ejn.14286
Chen, Z. Z., Wang, J. Y., Kang, Y., Yang, Q. Y., Gu, X. Y., Zhi, D. L., et al. (2021). PINK1 gene mutation by pair truncated sgRNA/Cas9-D10A in cynomolgus monkeys. Zool. Res. 42, 469–477. doi: 10.24272/j.issn.2095-8137.2021.023
Crosiers, D., Theuns, J., Cras, P., and Van Broeckhoven, C. (2011). Parkinson disease: insights in clinical, genetic and pathological features of monogenic disease subtypes. J. Chem. Neuroanat. 42, 131–141. doi: 10.1016/j.jchemneu.2011.07.003
Damier, P., Hirsch, E. C., Agid, Y., and Graybiel, A. M. (1999). The substantia nigra of the human brain. II. Patterns of loss of dopamine-containing neurons in Parkinson's disease. Brain 122, 1437–1448. doi: 10.1093/brain/122.8.1437
De Plano, L. M., Calabrese, G., Conoci, S., Guglielmino, S. P. P., Oddo, S., and Caccamo, A. (2022). Applications of CRISPR-Cas9 in Alzheimer's disease and related disorders. Int. J. Mol. Sci. 23:8714. doi: 10.3390/ijms23158714
Deng, H. X., and Siddique, T. (2000). Transgenic mouse models and human neurodegenerative disorders. Arch. Neurol. 57, 1695–1702. doi: 10.1001/archneur.57.12.1695
Deng, H., Wang, P., and Jankovic, J. (2018). The genetics of Parkinson disease. Ageing Res. Rev. 42, 72–85. doi: 10.1016/j.arr.2017.12.007
Diaz-Ortiz, M. E., Seo, Y., Posavi, M., Carceles Cordon, M., Clark, E., Jain, N., et al. (2022). GPNMB confers risk for Parkinson’s disease through interaction with α-synuclein. Science 377:eabk0637. doi: 10.1126/science.abk0637
Dorsey, E. R., and Bloem, B. R. (2018). The Parkinson pandemic-a call to action. JAMA Neurol. 75, 9–10. doi: 10.1001/jamaneurol.2017.3299
Dorsey, E. R., Sherer, T., Okun, M. S., and Bloem, B. R. (2018). The emerging evidence of the Parkinson pandemic. J. Parkinsons Dis. 8, S3–S8. doi: 10.3233/jpd-181474
Edson, A. J., Hushagen, H. A., Frøyset, A. K., Elda, I., Khan, E. A., Di Stefano, A., et al. (2019). Dysregulation in the brain protein profile of zebrafish lacking the Parkinson’s disease-related protein DJ-1. Mol. Neurobiol. 56, 8306–8322. doi: 10.1007/s12035-019-01667-w
Gao, H. M., and Hong, J. S. (2011). Gene-environment interactions: key to unraveling the mystery of Parkinson's disease. Prog. Neurobiol. 94, 1–19. doi: 10.1016/j.pneurobio.2011.03.005
Gaudelli, N. M., Komor, A. C., Rees, H. A., Packer, M. S., Badran, A. H., Bryson, D. I., et al. (2017). Programmable base editing of a•T to G•C in genomic DNA without DNA cleavage. Nature 551, 464–471. doi: 10.1038/nature24644
Gomperts, S. N. (2016). Lewy body dementias: dementia with Lewy bodies and Parkinson disease dementia. Continuum 22, 435–463. doi: 10.1212/con.0000000000000309
González-Rodríguez, P., Zampese, E., Stout, K. A., Guzman, J. N., Ilijic, E., Yang, B., et al. (2021). Disruption of mitochondrial complex I induces progressive parkinsonism. Nature 599, 650–656. doi: 10.1038/s41586-021-04059-0
Grünewald, J., Zhou, R., Lareau, C. A., Garcia, S. P., Iyer, S., Miller, B. R., et al. (2020). A dual-deaminase CRISPR base editor enables concurrent adenine and cytosine editing. Nat. Biotechnol. 38, 861–864. doi: 10.1038/s41587-020-0535-y
Guo, T., Pan, X., Jiang, G., Zhang, D., Qi, J., Shao, L., et al. (2022). Downregulating PTBP1 fails to convert astrocytes into hippocampal neurons and to alleviate symptoms in Alzheimer's mouse models. J. Neurosci. 42, 7309–7317. doi: 10.1523/jneurosci.1060-22.2022
Ham, S. J., Lee, D., Xu, W. J., Cho, E., Choi, S., Min, S., et al. (2021). Loss of UCHL1 rescues the defects related to Parkinson’s disease by suppressing glycolysis. Sci. Adv. 7:eabg4574. doi: 10.1126/sciadv.abg4574
Han, H. A., Pang, J. K. S., and Soh, B. S. (2020). Mitigating off-target effects in CRISPR/Cas9-mediated in vivo gene editing. J. Mol. Med. 98, 615–632. doi: 10.1007/s00109-020-01893-z
He, B., Peng, W., Huang, J., Zhang, H., Zhou, Y., Yang, X., et al. (2020). Modulation of metabolic functions through Cas13d-mediated gene knockdown in liver. Protein Cell 11, 518–524. doi: 10.1007/s13238-020-00700-2
Hsieh, C. H., Shaltouki, A., Gonzalez, A. E., Bettencourt da Cruz, A., Burbulla, L. F., St Lawrence, E., et al. (2016). Functional impairment in Miro degradation and mitophagy is a shared feature in familial and sporadic Parkinson’s disease. Cell Stem Cell 19, 709–724. doi: 10.1016/j.stem.2016.08.002
Huang, C. J., Adler, B. A., and Doudna, J. A. (2022). A naturally DNase-free CRISPR-Cas12c enzyme silences gene expression. Mol. Cell 82, 2148–2160.e4. doi: 10.1016/j.molcel.2022.04.020
Hughes, G. L., Lones, M. A., Bedder, M., Currie, P. D., Smith, S. L., and Pownall, M. E. (2020). Machine learning discriminates a movement disorder in a zebrafish model of Parkinson’s disease. Dis. Model. Mech. 13:dmm045815. doi: 10.1242/dmm.045815
Imaizumi, Y., Okada, Y., Akamatsu, W., Koike, M., Kuzumaki, N., Hayakawa, H., et al. (2012). Mitochondrial dysfunction associated with increased oxidative stress and α-synuclein accumulation in PARK2 iPSC-derived neurons and postmortem brain tissue. Mol. Brain 5:35. doi: 10.1186/1756-6606-5-35
Inoue, N., Ogura, S., Kasai, A., Nakazawa, T., Ikeda, K., Higashi, S., et al. (2018). Knockdown of the mitochondria-localized protein p13 protects against experimental parkinsonism. EMBO Rep. 19:e44860. doi: 10.15252/embr.201744860
Ishino, Y., Shinagawa, H., Makino, K., Amemura, M., and Nakata, A. (1987). Nucleotide sequence of the iap gene, responsible for alkaline phosphatase isozyme conversion in Escherichia coli, and identification of the gene product. J. Bacteriol. 169, 5429–5433. doi: 10.1128/jb.169.12.5429-5433.1987
Ishizu, N., Yui, D., Hebisawa, A., Aizawa, H., Cui, W., Fujita, Y., et al. (2016). Impaired striatal dopamine release in homozygous Vps35 D620N knock-in mice. Hum. Mol. Genet. 25, 4507–4517. doi: 10.1093/hmg/ddw279
Jansen, R., Embden, J. D., Gaastra, W., and Schouls, L. M. (2002). Identification of genes that are associated with DNA repeats in prokaryotes. Mol. Microbiol. 43, 1565–1575. doi: 10.1046/j.1365-2958.2002.02839.x
Kantor, B., Tagliafierro, L., Gu, J., Zamora, M. E., Ilich, E., Grenier, C., et al. (2018). Downregulation of SNCA expression by targeted editing of DNA methylation: a potential strategy for precision therapy in PD. Mol. Ther. 26, 2638–2649. doi: 10.1016/j.ymthe.2018.08.019
Kaulich, M., Lee, Y. J., Lönn, P., Springer, A. D., Meade, B. R., and Dowdy, S. F. (2015). Efficient CRISPR-rAAV engineering of endogenous genes to study protein function by allele-specific RNAi. Nucleic Acids Res. 43:e45. doi: 10.1093/nar/gku1403
Kim, Y. B., Komor, A. C., Levy, J. M., Packer, M. S., Zhao, K. T., and Liu, D. R. (2017). Increasing the genome-targeting scope and precision of base editing with engineered Cas9-cytidine deaminase fusions. Nat. Biotechnol. 35, 371–376. doi: 10.1038/nbt.3803
Kitada, T., Tong, Y., Gautier, C. A., and Shen, J. (2009). Absence of nigral degeneration in aged parkin/DJ-1/PINK1 triple knockout mice. J. Neurochem. 111, 696–702. doi: 10.1111/j.1471-4159.2009.06350.x
Komor, A. C., Badran, A. H., and Liu, D. R. (2017). CRISPR-based Technologies for the manipulation of eukaryotic genomes. Cells 168, 20–36. doi: 10.1016/j.cell.2016.10.044
Komor, A. C., Badran, A. H., and Liu, D. R. (2018). Editing the genome without double-stranded DNA breaks. ACS Chem. Biol. 13, 383–388. doi: 10.1021/acschembio.7b00710
Komor, A. C., Kim, Y. B., Packer, M. S., Zuris, J. A., and Liu, D. R. (2016). Programmable editing of a target base in genomic DNA without double-stranded DNA cleavage. Nature 533, 420–424. doi: 10.1038/nature17946
Kong, X., Zhang, H., Li, G., Wang, Z., Kong, X., Wang, L., et al. (2023). Engineered CRISPR-OsCas12f1 and RhCas12f1 with robust activities and expanded target range for genome editing. Nat. Commun. 14:2046. doi: 10.1038/s41467-023-37829-7
Kurihara, N., Nakagawa, R., Hirano, H., Okazaki, S., Tomita, A., Kobayashi, K., et al. (2022). Structure of the type V-C CRISPR-Cas effector enzyme. Mol. Cell 82, 1865–1877.e4. doi: 10.1016/j.molcel.2022.03.006
Kushawah, G., Hernandez-Huertas, L., Abugattas-Nuñez Del Prado, J., Martinez-Morales, J. R., DeVore, M. L., Hassan, H., et al. (2020). CRISPR-Cas13d induces efficient mRNA knockdown in animal embryos. Dev. Cell 54, 805–817.e7. doi: 10.1016/j.devcel.2020.07.013
Larbalestier, H., Keatinge, M., Watson, L., White, E., Gowda, S., Wei, W., et al. (2022). GCH1 deficiency activates brain innate immune response and impairs tyrosine hydroxylase homeostasis. J. Neurosci. 42, 702–716. doi: 10.1523/jneurosci.0653-21.2021
Lee, J., Bayarsaikhan, D., Arivazhagan, R., Park, H., Lim, B., Gwak, P., et al. (2019). CRISPR/Cas9 edited sRAGE-MSCs protect neuronal death in Parkinson’s disease model. Int J Stem Cells 12, 114–124. doi: 10.15283/ijsc18110
Li, Y., Glass, Z., Huang, M., Chen, Z. Y., and Xu, Q. (2020). Ex vivo cell-based CRISPR/Cas9 genome editing for therapeutic applications. Biomaterials 234:119711. doi: 10.1016/j.biomaterials.2019.119711
Li, H., Wu, S., Ma, X., Li, X., Cheng, T., Chen, Z., et al. (2021). Co-editing PINK1 and DJ-1 genes via adeno-associated virus-delivered CRISPR/Cas9 system in adult monkey brain elicits classical parkinsonian phenotype. Neurosci. Bull. 37, 1271–1288. doi: 10.1007/s12264-021-00732-6
Liao, Y., Dong, Y., and Cheng, J. (2017). The function of the mitochondrial calcium uniporter in neurodegenerative disorders. Int. J. Mol. Sci. 18:248. doi: 10.3390/ijms18020248
Lin, Y., Cradick, T. J., Brown, M. T., Deshmukh, H., Ranjan, P., Sarode, N., et al. (2014). CRISPR/Cas9 systems have off-target activity with insertions or deletions between target DNA and guide RNA sequences. Nucleic Acids Res. 42, 7473–7485. doi: 10.1093/nar/gku402
Lin, C. H., Tsai, P. I., Lin, H. Y., Hattori, N., Funayama, M., Jeon, B., et al. (2020). Mitochondrial UQCRC1 mutations cause autosomal dominant parkinsonism with polyneuropathy. Brain 143, 3352–3373. doi: 10.1093/brain/awaa279
Liu, Z., Cai, Y., Wang, Y., Nie, Y., Zhang, C., Xu, Y., et al. (2018). Cloning of macaque monkeys by somatic cell nuclear transfer. Cells 172, 881–887.e7. doi: 10.1016/j.cell.2018.01.020
Liu, L., Li, X., Ma, J., Li, Z., You, L., Wang, J., et al. (2017). The molecular architecture for RNA-guided RNA cleavage by Cas13a. Cells 170, 714–726.e10. doi: 10.1016/j.cell.2017.06.050
Liu, G. H., Qu, J., Suzuki, K., Nivet, E., Li, M., Montserrat, N., et al. (2012). Progressive degeneration of human neural stem cells caused by pathogenic LRRK2. Nature 491, 603–607. doi: 10.1038/nature11557
Lu, L., Yu, X., Cai, Y., Sun, M., and Yang, H. (2021). Application of CRISPR/Cas9 in Alzheimer’s disease. Front. Neurosci. 15:803894. doi: 10.3389/fnins.2021.803894
Lunney, J. K., Van Goor, A., Walker, K. E., Hailstock, T., Franklin, J., and Dai, C. (2021). Importance of the pig as a human biomedical model. Sci. Transl. Med. 13:eabd5758. doi: 10.1126/scitranslmed.abd5758
Makarova, K. S., Haft, D. H., Barrangou, R., Brouns, S. J., Charpentier, E., Horvath, P., et al. (2011). Evolution and classification of the CRISPR-Cas systems. Nat. Rev. Microbiol. 9, 467–477. doi: 10.1038/nrmicro2577
Makarova, K. S., Zhang, F., and Koonin, E. V. (2017). SnapShot: class 2 CRISPR-Cas systems. Cells 168, 328–328.e1. doi: 10.1016/j.cell.2016.12.038
Mali, P., Yang, L., Esvelt, K. M., Aach, J., Guell, M., DiCarlo, J. E., et al. (2013). RNA-guided human genome engineering via Cas9. Science 339, 823–826. doi: 10.1126/science.1232033
Merhi, R., Kalyn, M., Zhu-Pawlowsky, A., and Ekker, M. (2021). Loss of parla function results in inactivity, olfactory impairment, and dopamine neuron loss in zebrafish. Biomedicine 9:205. doi: 10.3390/biomedicines9020205
Naeem, M., Majeed, S., Hoque, M. Z., and Ahmad, I. (2020). Latest developed strategies to minimize the off-target effects in CRISPR-Cas-mediated genome editing. Cells 9:1608. doi: 10.3390/cells9071608
Nalls, M. A., Plagnol, V., Hernandez, D. G., Sharma, M., Sheerin, U. M., Saad, M., et al. (2011). Imputation of sequence variants for identification of genetic risks for Parkinson's disease: a meta-analysis of genome-wide association studies. Lancet 377, 641–649. doi: 10.1016/s0140-6736(10)62345-8
Narendra, D., Tanaka, A., Suen, D. F., and Youle, R. J. (2008). Parkin is recruited selectively to impaired mitochondria and promotes their autophagy. J. Cell Biol. 183, 795–803. doi: 10.1083/jcb.200809125
Nyuzuki, H., Ito, S., Nagasaki, K., Nitta, Y., Matsui, N., Saitoh, A., et al. (2020). Degeneration of dopaminergic neurons and impaired intracellular trafficking in Atp13a2 deficient zebrafish. IBRO Rep 9, 1–8. doi: 10.1016/j.ibror.2020.05.002
Paul, B., and Montoya, G. (2020). CRISPR-Cas12a: functional overview and applications. Biom. J. 43, 8–17. doi: 10.1016/j.bj.2019.10.005
Pausch, P., Al-Shayeb, B., Bisom-Rapp, E., Tsuchida, C. A., Li, Z., Cress, B. F., et al. (2020). CRISPR-CasΦ from huge phages is a hypercompact genome editor. Science 369, 333–337. doi: 10.1126/science.abb1400
Puschmann, A. (2013). Monogenic Parkinson’s disease and parkinsonism: clinical phenotypes and frequencies of known mutations. Parkinsonism Relat. Disord. 19, 407–415. doi: 10.1016/j.parkreldis.2013.01.020
Qi, L. S., Larson, M. H., Gilbert, L. A., Doudna, J. A., Weissman, J. S., Arkin, A. P., et al. (2013). Repurposing CRISPR as an RNA-guided platform for sequence-specific control of gene expression. Cells 152, 1173–1183. doi: 10.1016/j.cell.2013.02.022
Qian, H., Kang, X., Hu, J., Zhang, D., Liang, Z., Meng, F., et al. (2020). Reversing a model of Parkinson’s disease with in situ converted nigral neurons. Nature 582, 550–556. doi: 10.1038/s41586-020-2388-4
Ren, F., Ren, C., Zhang, Z., Duan, W., Lecourieux, D., Li, S., et al. (2019). Efficiency optimization of CRISPR/Cas9-mediated targeted mutagenesis in grape. Front. Plant Sci. 10:612. doi: 10.3389/fpls.2019.00612
Sakata, R. C., Ishiguro, S., Mori, H., Tanaka, M., Tatsuno, K., Ueda, H., et al. (2020). Base editors for simultaneous introduction of C-to-T and A-to-G mutations. Nat. Biotechnol. 38, 865–869. doi: 10.1038/s41587-020-0509-0
Sankhla, C. S. (2017). Oxidative stress and Parkinson’s disease. Neurol. India 65, 269–270. doi: 10.4103/0028-3886.201842
Satake, W., Nakabayashi, Y., Mizuta, I., Hirota, Y., Ito, C., Kubo, M., et al. (2009). Genome-wide association study identifies common variants at four loci as genetic risk factors for Parkinson’s disease. Nat. Genet. 41, 1303–1307. doi: 10.1038/ng.485
Schapira, A. H., and Jenner, P. (2011). Etiology and pathogenesis of Parkinson’s disease. Mov. Disord. 26, 1049–1055. doi: 10.1002/mds.23732
Selvakumar, G. P., Ahmed, M. E., Raikwar, S. P., Thangavel, R., Kempuraj, D., Dubova, I., et al. (2019). CRISPR/Cas9 editing of glia maturation factor regulates mitochondrial dynamics by attenuation of the NRF2/HO-1 dependent ferritin activation in glial cells. J. Neuroimmune Pharmacol. 14, 537–550. doi: 10.1007/s11481-019-09833-6
Sen, T., and Thummer, R. P. (2022). CRISPR and iPSCs: recent developments and future perspectives in neurodegenerative disease modelling, research, and therapeutics. Neurotox. Res. 40, 1597–1623. doi: 10.1007/s12640-022-00564-w
Shmakov, S., Smargon, A., Scott, D., Cox, D., Pyzocha, N., Yan, W., et al. (2017). Diversity and evolution of class 2 CRISPR-Cas systems. Nat. Rev. Microbiol. 15, 169–182. doi: 10.1038/nrmicro.2016.184
Simón-Sánchez, J., Schulte, C., Bras, J. M., Sharma, M., Gibbs, J. R., Berg, D., et al. (2009). Genome-wide association study reveals genetic risk underlying Parkinson’s disease. Nat. Genet. 41, 1308–1312. doi: 10.1038/ng.487
Sliter, D. A., Martinez, J., Hao, L., Chen, X., Sun, N., Fischer, T. D., et al. (2018). Parkin and PINK1 mitigate STING-induced inflammation. Nature 561, 258–262. doi: 10.1038/s41586-018-0448-9
Soman, S. K., Bazała, M., Keatinge, M., Bandmann, O., and Kuznicki, J. (2019). Restriction of mitochondrial calcium overload by mcu inactivation renders a neuroprotective effect in zebrafish models of Parkinson’s disease. Biol Open 8:bio044347. doi: 10.1242/bio.044347
Sun, W. Y., Tyurin, V. A., Mikulska-Ruminska, K., Shrivastava, I. H., Anthonymuthu, T. S., Zhai, Y. J., et al. (2021). Phospholipase iPLA(2)β averts ferroptosis by eliminating a redox lipid death signal. Nat. Chem. Biol. 17, 465–476. doi: 10.1038/s41589-020-00734-x
Tong, H., Huang, J., Xiao, Q., He, B., Dong, X., Liu, Y., et al. (2023a). High-fidelity Cas13 variants for targeted RNA degradation with minimal collateral effects. Nat. Biotechnol. 41, 108–119. doi: 10.1038/s41587-022-01419-7
Tong, H., Wang, X., Liu, Y., Liu, N., Li, Y., Luo, J., et al. (2023b). Programmable A-to-Y base editing by fusing an adenine base editor with an N-methylpurine DNA glycosylase. Nat. Biotechnol. doi: 10.1038/s41587-022-01595-6 [Epub ahead of print]
Van der Vegt, J. P., van Nuenen, B. F., Bloem, B. R., Klein, C., and Siebner, H. R. (2009). Imaging the impact of genes on Parkinson’s disease. Neuroscience 164, 191–204. doi: 10.1016/j.neuroscience.2009.01.055
Wang, X., Cao, C., Huang, J., Yao, J., Hai, T., Zheng, Q., et al. (2016). One-step generation of triple gene-targeted pigs using CRISPR/Cas9 system. Sci. Rep. 6:20620. doi: 10.1038/srep20620
Wu, Z., Zhang, Y., Yu, H., Pan, D., Wang, Y., Wang, Y., et al. (2021). Programmed genome editing by a miniature CRISPR-Cas12f nuclease. Nat. Chem. Biol. 17, 1132–1138. doi: 10.1038/s41589-021-00868-6
Wüllner, U., Kaut, O., deBoni, L., Piston, D., and Schmitt, I. (2016). DNA methylation in Parkinson’s disease. J. Neurochem. 139, 108–120. doi: 10.1111/jnc.13646
Xu, C., Zhou, Y., Xiao, Q., He, B., Geng, G., Wang, Z., et al. (2021). Programmable RNA editing with compact CRISPR-Cas13 systems from uncultivated microbes. Nat. Methods 18, 499–506. doi: 10.1038/s41592-021-01124-4
Xue, J., Zhu, Y., Wei, L., Huang, H., Li, G., Huang, W., et al. (2022). Loss of Drosophila NUS1 results in cholesterol accumulation and Parkinson’s disease-related neurodegeneration. FASEB J. 36:e22411. doi: 10.1096/fj.202200212R
Yan, W. X., Chong, S., Zhang, H., Makarova, K. S., Koonin, E. V., Cheng, D. R., et al. (2018). Cas13d is a compact RNA-targeting type VI CRISPR effector positively modulated by a WYL-domain-containing accessory protein. Mol. Cell 70, 327–339.e5. doi: 10.1016/j.molcel.2018.02.028
Yan, J., Kang, D. D., and Dong, Y. (2021). Harnessing lipid nanoparticles for efficient CRISPR delivery. Biomater. Sci. 9, 6001–6011. doi: 10.1039/d1bm00537e
Yang, W., Chen, X., Li, S., and Li, X. J. (2021). Genetically modified large animal models for investigating neurodegenerative diseases. Cell Biosci. 11:218. doi: 10.1186/s13578-021-00729-8
Yang, H., Gao, P., Rajashankar, K. R., and Patel, D. J. (2016). PAM-dependent target DNA recognition and cleavage by C2c1 CRISPR-Cas endonuclease. Cells 167, 1814–1828.e12. doi: 10.1016/j.cell.2016.11.053
Yang, W., Guo, X., Tu, Z., Chen, X., Han, R., Liu, Y., et al. (2022). PINK1 kinase dysfunction triggers neurodegeneration in the primate brain without impacting mitochondrial homeostasis. Protein Cell 13, 26–46. doi: 10.1007/s13238-021-00888-x
Yang, W., Li, S., and Li, X. J. (2019a). A CRISPR monkey model unravels a unique function of PINK1 in primate brains. Mol. Neurodegener. 14:17. doi: 10.1186/s13024-019-0321-9
Yang, W., Liu, Y., Tu, Z., Xiao, C., Yan, S., Ma, X., et al. (2019b). CRISPR/Cas9-mediated PINK1 deletion leads to neurodegeneration in rhesus monkeys. Cell Res. 29, 334–336. doi: 10.1038/s41422-019-0142-y
Yang, Y., Xu, J., Ge, S., and Lai, L. (2021). CRISPR/Cas: advances, limitations, and applications for precision cancer research. Front. Med. 8:649896. doi: 10.3389/fmed.2021.649896
Yao, J., Huang, J., Hai, T., Wang, X., Qin, G., Zhang, H., et al. (2014). Efficient bi-allelic gene knockout and site-specific knock-in mediated by TALENs in pigs. Sci. Rep. 4:6926. doi: 10.1038/srep06926
Yoon, H. H., Ye, S., Lim, S., Jo, A., Lee, H., Hong, F., et al. (2022). CRISPR-Cas9 gene editing protects from the A53T-SNCA overexpression-induced pathology of Parkinson’s disease in vivo. CRISPR J 5, 95–108. doi: 10.1089/crispr.2021.0025
Zhang, B., Lin, J., Perčulija, V., Li, Y., Lu, Q., Chen, J., et al. (2022). Structural insights into target DNA recognition and cleavage by the CRISPR-Cas12c1 system. Nucleic Acids Res. 50, 11820–11833. doi: 10.1093/nar/gkac987
Zhang, X. H., Tee, L. Y., Wang, X. G., Huang, Q. S., and Yang, S. H. (2015). Off-target effects in CRISPR/Cas9-mediated genome engineering. Mol. Ther. Nucleic Acids 4:e264. doi: 10.1038/mtna.2015.37
Zhang, X., Zhu, B., Chen, L., Xie, L., Yu, W., Wang, Y., et al. (2020). Dual base editor catalyzes both cytosine and adenine base conversions in human cells. Nat. Biotechnol. 38, 856–860. doi: 10.1038/s41587-020-0527-y
Zhou, H., Su, J., Hu, X., Zhou, C., Li, H., Chen, Z., et al. (2020). Glia-to-neuron conversion by CRISPR-CasRx alleviates symptoms of neurological disease in mice. Cells 181, 590–603.e16. doi: 10.1016/j.cell.2020.03.024
Zhou, X., Xin, J., Fan, N., Zou, Q., Huang, J., Ouyang, Z., et al. (2015). Generation of CRISPR/Cas9-mediated gene-targeted pigs via somatic cell nuclear transfer. Cell. Mol. Life Sci. 72, 1175–1184. doi: 10.1007/s00018-014-1744-7
Zhou, H., Zhang, J., Shi, H., Li, P., Sui, X., Wang, Y., et al. (2022). Downregulation of CDK5 signaling in the dorsal striatum alters striatal microcircuits implicating the association of pathologies with circadian behavior in mice. Mol. Brain 15:53. doi: 10.1186/s13041-022-00939-2
Keywords: Parkinson’s disease, CRISPR Cas9, model animals, precision treatment, gene editing
Citation: Qu J, Liu N, Gao L, Hu J, Sun M and Yu D (2023) Development of CRISPR Cas9, spin-off technologies and their application in model construction and potential therapeutic methods of Parkinson’s disease. Front. Neurosci. 17:1223747. doi: 10.3389/fnins.2023.1223747
Edited by:
Sen Yan, Jinan University, ChinaReviewed by:
Shunliang Xu, The Second Hospital of Shandong University, China Sameh A. Abdelnour, Zagazig University, EgyptCopyright © 2023 Qu, Liu, Gao, Hu, Sun and Yu. This is an open-access article distributed under the terms of the Creative Commons Attribution License (CC BY). The use, distribution or reproduction in other forums is permitted, provided the original author(s) and the copyright owner(s) are credited and that the original publication in this journal is cited, in accordance with accepted academic practice. No use, distribution or reproduction is permitted which does not comply with these terms.
*Correspondence: Dongyi Yu, ZG9uZ3lpX3l1QDE2My5jb20=