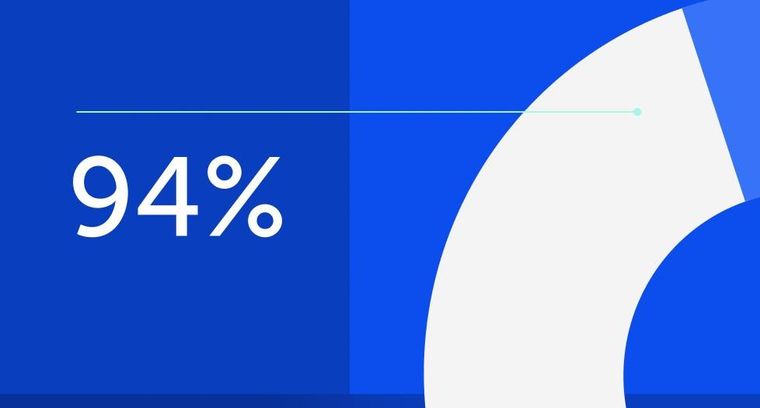
94% of researchers rate our articles as excellent or good
Learn more about the work of our research integrity team to safeguard the quality of each article we publish.
Find out more
REVIEW article
Front. Neurosci., 11 July 2023
Sec. Visual Neuroscience
Volume 17 - 2023 | https://doi.org/10.3389/fnins.2023.1216489
This article is part of the Research TopicOcular Neurodegenerative Diseases: Novel Mechanisms, Diagnosis, and Therapeutic StrategiesView all 18 articles
Age-related macular degeneration (AMD) is a chronic and progressive inflammatory disease of the retina characterized by photoceptor loss and significant central visual impairment due to either choroidal neovascularization or geographic atrophy. The pathophysiology of AMD is complex and multifactorial, driven by a combination of modifiable and non-modifiable risk factors, molecular mechanisms, and cellular processes that contribute to overall disease onset, severity, and progression. Unfortunately, due to the structural, cellular, and pathophysiologic complexity, therapeutic discovery is challenging. While purinergic signaling has been investigated for its role in the development and treatment of ocular pathologies including AMD, the potential crosstalk between known contributors to AMD, such as the complement cascade and inflammasome activation, and other biological systems, such as purinergic signaling, have not been fully characterized. In this review, we explore the interactions between purinergic signaling, ATP release, and known contributors to AMD pathogenesis including complement dysregulation and inflammasome activation. We begin by identifying what is known about purinergic receptors in cell populations of the outer retina and potential sources of extracellular ATP required to trigger purinergic receptor activation. Next, we examine evidence in the literature that the purinergic system accelerates AMD pathogenesis leading to apoptotic and pyroptotic cell death in retinal cells. To fully understand the potential role that purinergic signaling plays in AMD, more research is needed surrounding the expression, distribution, functions, and interactions of purinergic receptors within cells of the outer retina as well as potential crosstalk with other systems. By determining how these processes are affected in the context of purinergic signaling, it will improve our understanding of the mechanisms that drive AMD pathogenesis which is critical in developing treatment strategies that prevent or slow progression of the disease.
Purinergic signaling is a form of extracellular signaling involving purine and pyrimidine nucleotides and nucleosides that act on purinergic receptors to mediate numerous cellular functions (Burnstock, 2008). In total, there are three distinct classes of purinergic receptors known as P1, P2Y, and P2X receptors. P1 receptors are G protein-coupled receptors (GPCRs) that respond to adenosine (Burnstock, 2018). P2Y receptors are GPCRs that respond to nucleotides such as adenosine triphosphate (ATP), adenosine diphosphate (ADP), adenosine monophosphate (AMP), adenosine, uridine triphosphate (UTP), uridine diphosphate (UDP), and UDP-glucose (Burnstock, 2018). P2X receptors are unique and function as ligand-gated ion channels that respond exclusively to extracellular ATP (eATP) (Burnstock, 2018). Purinergic signaling represents a set of phylogenetically ancient pathways that play a critical role in numerous cellular processes, bodily systems, and developmental stages, including proliferation, differentiation, migration, apoptosis, embryogenesis, organogenesis, and aging (Burnstock and Dale, 2015; Huang et al., 2021). Within the eye, purinergic signaling has been investigated for its role in the development and treatment of ocular pathologies such as age-related macular degeneration (AMD), glaucoma, and diabetic retinopathy (Ye et al., 2021).
AMD is a leading cause of visual impairment and accounts for approximately 9% of all cases of blindness worldwide (Wong et al., 2014). The pathophysiology of AMD is complex and multifactorial, driven by a combination of non-modifiable risk factors (e.g., aging, genetic predisposition) and modifiable risk factors (e.g., smoking, hypertension, body mass index, hypercholesterolemia, nutritional intake, UV light) that contribute to overall disease onset, severity, and progression (Ambati and Fowler, 2012; Wong et al., 2014; Mitchell et al., 2018; de Jong et al., 2020; Stahl, 2020). AMD can be subdivided into early, intermediate, and advanced stages, with the advanced forms characterized by photoreceptor loss in the macula, the region of the retina responsible for central vision. There are two forms of advanced AMD. Wet (or exudative) AMD (~10% of cases) develops due to choroidal neovascularization (CNV), a form of abnormal angiogenesis in the choriocapillaris layers of the choroid, which results in the growth of neo-vessels breaching Bruch’s membrane, causing photoreceptor death (Mitchell et al., 2018). Dry (or non-exudative) AMD (~90% of cases) is a slowly progressing degenerative process whereby regions of retinal pigment epithelium (RPE), a monolayer of cells that combine with Bruch’s membrane and form the outer blood-eye barrier, undergo cell death leading to geographic atrophy (GA) (Mitchell et al., 2018). The early stages of both forms are characterized by the buildup of drusen deposits, which are composed of glycoproteins, lipids, and immunogenic factors. The drusen accumulate in the extracellular space between the RPE and Bruch’s membrane and provoke a chronic proinflammatory milieu that triggers the development of AMD (Mitchell et al., 2018).
Despite the emergence of anti-vascular endothelial growth factor (VEGF) agents to treat wet AMD, there are currently no therapies available to prevent the development of dry AMD aside from nutritional supplementation (AREDS formulation) (Age-Related Eye Disease Study Research Group, 2001). However, in 2023, the FDA approved pegcetacoplan, a complement C3 inhibitor, to slow the progression of GA, providing some promise for those with late-stage GA. It is also possible for a portion of patients with wet AMD to develop severe vision loss and blindness by developing GA over time, further emphasizing the complex nature of AMD pathogenesis (Holz et al., 2014; Rasmussen and Sander, 2014; Agarwal et al., 2015; Chen and Kaiser, 2020). Thus, understanding the multiple mechanisms that drive AMD and the interplay between wet and dry forms, is crucial in developing treatment strategies that not only slow the progression of the disease, but ultimately prevent its development.
In the present review, we explore purinergic signaling and mechanisms of unregulated ATP release in the outer retina, and its potential significance in AMD pathogenesis. While there is evidence for an interplay between purinergic signaling and the mechanisms associated with AMD pathogenesis such as complement dysregulation, inflammasome activation, and sublytic membrane attack complex (MAC) deposition, few studies have addressed their detailed interactions. We first begin by identifying what is known about purinergic receptors in cell populations of the outer retina along with potential sources of eATP required to trigger purinergic receptor activation. Next, we examine evidence in the literature that the purinergic system accelerates AMD pathogenesis leading to apoptotic and pyroptotic cell death in RPE, photoreceptors, and choroidal cells.
The purinergic system is a form of cell signaling in which both purine and pyrimidine nucleotides and nucleosides act on extracellular purinergic receptors (Burnstock, 2008). As outlined above, purinergic receptors are divided into two classes known as P1 and P2 receptors. P1 receptors are GPCRs that respond to the nucleoside adenosine (Burnstock, 2018). In contrast, P2 receptors are nucleotide receptors that are further subdivided into P2X and P2Y receptors. P2Y receptors are GPCRs that respond to several nucleotides such as ATP, ADP, AMP, UTP, UDP, and UDP-glucose (Burnstock, 2018). P2X receptors are ligand-gated ion channels that respond exclusively to eATP (Burnstock, 2018). This review will focus on P2X receptors which are composed of two transmembrane domains with cytoplasmic amino-and carboxyl-terminals that polymerize to form homotrimeric or heterotrimeric channels permeable to cations such as sodium, potassium, and calcium (Jacobson et al., 2020). In total, seven homotrimeric P2X receptors (P2X1-7) and several heterotrimeric P2X channels with hybrid properties exist (Ralevic and Burnstock, 1998; Jacobson et al., 2020; Santiago et al., 2020; Illes et al., 2021). Table 1 outlines the unique features of P2X1-7 receptors based on their functional and pharmacological properties (Illes et al., 2021). For a comprehensive review of purinergic signaling and current developments in this field, please see Burnstock (2006, 2017).
Table 1. P2X receptors showing their molecular, pharmacologic, and functional properties [Adapted from Illes et al. (2021)].
Due to its well-established role in several inflammatory processes, a significant quantity of research has been performed surrounding P2X7. However, normal extracellular concentrations of ATP are approximately 10 nM under steady-state conditions, and the half maximal effective concentration (EC50) for P2X7 receptor activation is approximately 100 μM. Furthermore, once ATP is released, it can be rapidly degraded by ecto-enzymes yielding ADP, AMP, and adenosine, further decreasing the concentrations of ATP available for P2X activation. This means that the concentration of ATP required to activate P2X7 receptors is considerably higher than that found under physiological conditions leading to past debate surrounding its physiological relevance, especially in early stages of disease or inflammatory processes (Yang et al., 2011). However, with the novel introduction of plasma membrane luciferase (pmeLUC) which has made direct measurements of eATP possible, the concentration of eATP has been shown to reach 100–200 μM (Pellegatti et al., 2005, 2008; Morciano et al., 2017; Romagnani et al., 2020). Additionally, positive allosteric modulators acting at P2X7 are released into circulation during inflammation, thus further increasing the affinity of P2X7 for ATP (Tomasinsig et al., 2008; Kahlenberg and Kaplan, 2013; Di Virgilio et al., 2018). Secreted or membrane-bound ecto-kinases such as adenylate kinase, nucleoside monophosphokinases, and nucleoside diphosphokinases can also phosphorylate nucleosides to produce AMP, ADP, and ATP (Schwiebert and Zsembery, 2003). Taken together, there are several mechanisms whereby the concentration of eATP is capable of activating any and all of the P2X family of receptors. While questions remain surrounding the underlying pathways of P2X7 signaling in AMD, there is a significant gap in the literature surrounding other members of the P2X family of receptors, including novel and hybrid properties of heterotrimers, that may play an important role in various cellular and inflammatory processes.
The organization of the retina has been well studied for its complex synaptic circuitry of retinal neurons, supported by two vascular beds, an inner (retinal) and outer (choroidal) retinal supply. The outer vascular bed is comprised of the choroidal circulation that supports the outer retina and plays an important homeostatic role for the retinal pigment epithelium (RPE) and photoreceptors (Figure 1). For cells of the inner neuroretina, RPE, choroid, and retinal vasculature, purinergic receptors from each receptor class are present (Yang et al., 2011; Wagner et al., 2013; Jacobson and Civan, 2016). This is important, as purinergic signaling has been implicated in the proliferation, survival, death, migration, and differentiation of retinal cells throughout development, aging, and in disease states. For a review on purinergic signaling in the inner retina, please see Ventura et al. (2019) and Sanderson et al. (2014). Here, we discuss the distribution and functions of purinergic receptors on cells present within the outer retina, including the RPE and choroid, that may influence and contribute to AMD pathogenesis (Figure 2).
Figure 1. Complex architecture of the human retina. A schematic diagram of a cross-section through the retina. The layers of the neuroretina from inner to outer include: the retinal nerve fiber layer (RNFL), ganglion cell layer (GCL), inner plexiform layer (IPL), inner neuronal layer (INL), outer plexiform layer (OPL), and outer neuronal layer (ONL). Below the neuroretina is the retinal pigment epithelial layer (RPE) which is a monolayer of cells that sit on the extracellular matrix basement membrane, Bruch’s membrane (BM). The outer retinal is supplied by the choroidal blood circulatory system, comprised of the choriocapillaris (CC), the small vessel layer (SL) and the large vessel layer (not shown). Blood vessels (BV) are depicted within the figure. The retinal neurons in the inner retina include bipolar cells (BC, dark green), ganglion cells (GC, pink), amacrine cells (AC, light green), horizontal cells (HC, red), and rod and cone photoreceptors (orange). Microglia (blue), astrocytes (purple) and the Muller cells, a specialized astrocyte (MC, black) are supportive glial cell types that provide homeostasis and metabolic support for the retina.
Figure 2. (A) Complement activation with the formation of the C5b-9(n) leading to the formation of a MAC/PFTs complex. The fully formed MAC creates large, 10 nm-wide pores initiating an ion flux cumulating in a calcium dependent cell lysis. As a result of cell lysis, high concentrations of ATP may be released into the local environment which can simulate P2X and activate downstream purinergic modification. Extracellular ATP similarly can activate P2Y. Extracellular adenosine is re-cycled back into the cytoplasm via the nucleoside transporters (NT). (B) Pathogen-associated molecular patterns (PAMPs) and damage-associated molecular patterns (DAMPs) such as toxins, pathogens, metabolites, crystalline substances, nucleic acids, ion flux, reactive oxygen species, and ATP stimulate toll-like receptors (TLRs) and c type lectin receptors (CLRs) leading to activation of NF-κB followed by the subsequent transcriptional upregulation of NOD-like receptor family pyrin domain containing 3 (NLRP3) and pro-interleukin-1β. The cascade as depicted leads to the formation of gasdermin-D (GSDMD) and pyroptotic cell death through activation of caspase-4/5, capase-1, and GSDMD-NT along with release of ATP and other pro-inflammatory factors such as IL-1b, IL-18, and TFN-α.
The RPE is considered a primary site of pathology in AMD (Strauss, 2005; Bhutto and Lutty, 2012). It is supported by Bruch’s membrane, an important extracellular matrix that separates the RPE from the choroidal blood supply. The RPE is positioned between the overlying outer segments of photoreceptors and the choroidal blood supply and combines the functions of epithelial and glial cells to act as both a barrier and supporting tissue for overlying photoreceptors (Strauss, 2005; Bhutto and Lutty, 2012). Indeed, communication between photoreceptors and the RPE is critical to retinal function and occurs through a small extracellular space that exists between the apical membrane of RPE cells and photoreceptors (Mitchell and Reigada, 2008). Located within this space is an abundance of enzymes and a highly structured extracellular matrix that allows for many functional interactions between the RPE and photoreceptors to take place (Mitchell and Reigada, 2008). For example, the RPE delivers nutrients from the choroidal blood supply to the photoreceptors, removes metabolic end products from photoreceptors, produces melanin granules to absorb stray light, and recycles molecules important for maintenance of the visual cycle (Strauss, 2005; Mitchell and Reigada, 2008; Bhutto and Lutty, 2012). The RPE also plays an integral role in the daily renewal of photoreceptors through the recycling and resynthesis of spent outer segments (Strauss, 2005; Mitchell and Reigada, 2008; Bhutto and Lutty, 2012).
While RPE cells have been found on exon level profiling to express all subtypes of purinergic receptors (Wagner et al., 2013), the distribution of P2X receptors in human RPE cells is not completely known and few studies have explored the role of P2X receptors within the outer retina. For example, Yang et al. (2011) found P2X7 receptor mRNA in human RPE cells and functional data indicating that in addition to P2X7, other P2X receptors such as P2X1, P2X2, P2X3, P2X4, and P2X5 may also be present. However, further studies are needed to understand the expression, localization, and functions of these receptors within RPE cells.
Perhaps the most well-characterized of these receptors in the RPE to date is the P2X7 receptor, which induces calcium signaling and the activation of numerous cellular pathways that lead to subsequent apoptosis in both native and cultured human RPE cells (Yang et al., 2011). RPE cell death also results in the release of proinflammatory cytokines and further unregulated release of ATP thereby increasing the vulnerability of other cells to ATP-induced apoptosis (Yang et al., 2011). Increasing concentrations of eATP released from stressed, injured, or damaged outer retinal cells (in the case of AMD and other retinal diseases) also influence aspects of their overall function as support cells for the inner neuroretina through cytokine and growth factor release and as a stimulant for proliferation and differentiation (Notomi et al., 2011; Niyadurupola et al., 2013; Notomi et al., 2013; Clapp et al., 2019; Platania et al., 2019).
An important aspect of P2X signaling in RPE cells is that under physiological circumstances, these cells can maintain homeostasis and prevent ATP-induced apoptosis by expressing high levels of the enzymes CD39 and CD73 within their membranes. CD39 is an ectonucleoside triphosphate diphosphohydrolase (NTPDase) that rapidly hydrolyzes ATP and ADP to AMP, while CD73 is an ectonucleotidase that degrades AMP to adenosine (Kukulski et al., 2011; Dwyer et al., 2020). Interestingly, after being exposed to inflammatory factors, Zhang et al. (2018) demonstrated that RPE cells can rapidly become CD73-negative. This was found to be the result of matrix metalloproteinase-9 (MMP-9)-mediated shedding of CD73 from the cell membrane of RPE after exposure to inflammatory factors in vitro, leading to impaired immune suppression, increased concentrations of eATP, and accelerated local inflammation in the AMD environment (Zhang et al., 2018).
All immune cells, whether of the myeloid or lymphoid lineage, express at least one P2X receptor subtype, and many express all seven subtypes (Burnstock, 2016). Within the outer retina and choroid, these immune cells include resident retinal microglia, mast cells, lymphocytes, monocytes, and dendritic cells (Sarma and Ward, 2011; Merle et al., 2015a,b; Di Virgilio et al., 2017; Behnke et al., 2020; Di Virgilio et al., 2020; Ogura et al., 2020).
Microglia are resident professional phagocytes of the CNS, similar in function to blood-borne peripheral immune cells including monocytes, macrophages, and lymphocytes. They possess a high density of P2X7 receptors (Illes et al., 2017, 2020) in addition to P2X1 and P2X4 (Di Virgilio et al., 2020). Further, their ability to function as scavengers by migrating toward and clearing insoluble photo-oxidized material found in drusen has been shown to result from purinergic signaling interactions, such as in the case of ATP and P2X7 receptor activation (Gu and Wiley, 2018). Indeed, the heterogeneous expression of P2X1, P2X4, and P2X7 receptors on the surface of macrophages with varying ATP affinities may enable fine-tuning of macrophage responses to ATP (Guo et al., 2007; Adinolfi et al., 2018). This can result in distinct desensitization kinetics and diverse intracellular transduction pathways that contribute to numerous pro-inflammatory pathways in a concentration-dependent manner (Adinolfi et al., 2018).
Genetic association studies have also investigated this link by identifying a unique haplotype containing a heterotrimeric combination of P2X4 and P2X7 subunits that increases an individual’s risk of developing AMD due to impaired P2X7 function. This results in reduced phagocytic capacity of macrophages, delayed clearance of apoptotic cells, and leakage of ATP from necrotic cells (Gu et al., 2013). Furthermore, P2X7-null mice models demonstrated reduced blood-borne macrophage phagocytosis activity resulting in thickening of Bruch’s membrane, RPE dysfunction, and retinal stress at 12 months of age (Vessey et al., 2017), followed by Bruch’s membrane thickening, RPE cell loss, retinal functional deficits, and signs of inflammation between the RPE and photoreceptors at 18 months of age – phenotypic characteristics consistent with early AMD (Vessey et al., 2017). Taken together, communication between macrophages occurs, in part, via purinergic signaling.
Other studies also support the role of ATP in regulating macrophage chemotaxis and macrophage activation (Kronlage et al., 2010; Junger, 2011; Sakaki et al., 2013). This outlines a potential mechanism for the role of purinergic signaling in the pathogenesis of AMD, whereby immune cells present within the outer retina are no longer able to manage the task of removing the constant supply of photoreceptor debris, leading to the progressive and damaging accumulation of drusen within Bruch’s membrane, activation of bystander cells, worsening nutrient and oxygen support for the RPE, and a vicious cycle of RPE failure, neuronal cell death, and central vision loss (Strauss, 2005; Zumerle et al., 2019).
Mast cells (MCs) are recognized as key components of inflammatory reactions and are implicated in several inflammatory diseases. They are responsive to toxins and microbes, as well as substances such as advanced glycation end products, complement factors, C-reactive protein, and ATP, all of which are implicated in AMD (Ogura et al., 2020). Of the seven P2X receptors, only five (P2X1, P2X3, P2X4, P2X6, and P2X7) are expressed by MCs (Wareham et al., 2009; Wareham and Seward, 2016). These receptors play an important role in regulating MC activities, such as calcium influx and degranulation that results in the release of many pre-stored inflammatory mediators (Bulanova and Bulfone-Paus, 2010). Inflammatory mediators include IL-1β, Nuclear factor kappa B (NF-κB), tumor necrosis factor α (TNF-α), serotonin, and kinins, along with the synthesis and secretion of an array of cytokines, chemokines, prostaglandins, leukotrienes, and growth and angiogenesis factors (i.e., platelet-derived growth factor and VEGF) (Galli and Tsai, 2008; Bulanova and Bulfone-Paus, 2010; Kurashima et al., 2012; Theoharides et al., 2012; Shieh et al., 2014; Salcman et al., 2021). These MC components can modulate the activity of cells in their proximity and lead to the generation of reactive oxygen species (ROS), promotion of chemotaxis, altered phagocytosis, degradation of underlying extracellular matrix (ECM), and other events contributing to an overall increase in inflammation (Bhutto et al., 2016; Kempuraj et al., 2016; Caraffa et al., 2018).
In the pathogenesis of AMD, MC-derived tryptase release also results in the breakdown of collagens and activation of MMPs that degrade choroidal stroma and Bruch’s membrane. This leads to thinning of the choroid and degeneration of the RPE, both of which are hallmarks of GA (Ogura et al., 2020). Additionally, MC activation has been implicated in choroidal neovascularization through granzyme B release through intracellular immune-mediated cell death and extracellular ECM degradation (Matsubara et al., 2020). This results in remodeling of the ECM in Bruch’s membrane, breakdown of the blood-retina barrier, and slowing of metabolite transport between the choroidal blood supply and retina, which can contribute to drusen deposition, vascular leakage, disruption of choroidal endothelial cell function, and the release of sequestered VEGF from Bruch’s membrane (Matsubara et al., 2020).
The retina is nourished by two independent vascular supplies (Figure 1). The outer retina and photoreceptors are fed by the choroidal vasculature that lies directly beneath the photoreceptors and the RPE, while the inner retina is served by intrinsic retinal vasculature, branches of the central retinal artery that enter at the optic disc (Newman, 2015). As autonomic innervation is absent in the generation of retinal vascular tone, the tone of these vessels must be generated by intrinsic mechanisms such as the release of vasoactive agents from neurons, glial cells, and vascular endothelial cells (Kur et al., 2012; Newman, 2015). For this reason, purinergic signaling involving ATP has been explored as a mechanism to generate tone in retinal arterioles (Kur and Newman, 2014). Indeed, experiments have demonstrated that a reduction of endogenous eATP levels leads to arteriole dilation, while an increase in eATP levels leads to vessel constriction through altered P2X1 receptor activity (Kur et al., 2012; Newman, 2015).
Purinergic signaling also results in choroidal and retinal neovascularization through remodeling of existing vasculature and proteolytic degradation of the endothelial basal membrane and surrounding extracellular matrix via MMP-2 and MMP-9 activation (Yancopoulos et al., 2000; Berglin et al., 2003). Additionally, stimulation of P2X receptors promotes VEGF release and alters endothelial barrier properties depending on the type of receptors present and the local concentration of the nucleotides within the vasculature (Adinolfi et al., 2018). For instance, chronic P2X receptor activation with ATP acting as a danger-associated molecular pattern (DAMP) at high concentrations leads to endothelial barrier destabilization and edema formation through impaired Müller cell function in the induction, maintenance, and proper functioning of the blood–retinal barrier (Shen et al., 2012; Wakx et al., 2016). Together, these processes can contribute to retinal degeneration under pathologic conditions such as the proinflammatory environment seen in AMD.
Broadly, mechanisms of intermittent ATP release can be the result of (1) cell damage or cell death (e.g., complement activation and MAC deposition, osmotic swelling, ischemia, inflammation, or apoptosis leading to the passive leakage of ATP from cells), (2) vesicular release, or (3) channel-mediated release (Bulanova and Bulfone-Paus, 2010). However, sustained ATP release which is likely the ATP release of pathophysiological significance can also result from a multiplicity of pathways (Lazarowski et al., 2003; Dale et al., 2023; Di Virgilio et al., 2023; Shinozaki et al., 2023). Within the outer retina, several processes that contribute to AMD pathogenesis lead to ATP release (Figure 2). For example, activation of the complement cascade results in ATP release from MAC deposition leading to inflammasome activation, the release of pore-forming gasdermins, and pyroptosis. ATP release can also act as a feedforward method to trigger P2X receptors and further promote cell degeneration in the AMD outer retina. Recently, apoptosis has also been shown to release ATP as a “find me” signal through Pannexin 1 channels (Medina et al., 2020).
The complement system plays a central role in AMD pathogenesis, along with aspects of cellular immunity and homeostasis. It consists of a network of proteins that can be sequentially cleaved and activated through any of three distinct pathways: the classical pathway, the lectin pathway, and/or the alternative pathway (Ricklin et al., 2010; Sarma and Ward, 2011; Merle et al., 2015a,b). Each of these pathways converge in the terminal pathway of the complement system, which results in the formation of the C5b-9(n) MAC complex. Fully formed MAC creates large, 10 nm-wide, pores in the membranes of pathogens and vulnerable host cells and can result in calcium dependent cell lysis (Sarma and Ward, 2011; Merle et al., 2015a,b). For a comprehensive review of complement and its role in AMD, please see Armento et al. (2021).
As a result of cell lysis, high concentrations of ATP may be released into the local environment. This can stimulate P2X receptors and influence the recruitment and activation of numerous inflammatory cells such as retinal microglia, mast cells, and circulating lymphocytes, monocytes, and macrophages, as described above (Ricklin et al., 2010; Sarma and Ward, 2011; Merle et al., 2015a,b; Behnke et al., 2020; Ogura et al., 2020). Ultimately, this amplification loop can: (1) induce changes in the composition of Bruch’s membrane, the choriocapillaris, and ECM, (2) impair transport properties, alter lipid metabolism, and result in the accumulation of drusen, and (3) lead to chronic inflammation, oxidative stress, and altered energy metabolism as seen in the pathogenesis of AMD (Armento et al., 2021).
These findings are echoed by genetic studies where over 33 different loci associated with aspects of the complement system, ECM remodeling, and other pathways such as cholesterol metabolism have demonstrated an increased risk for the development of AMD (Klein et al., 2005; Schramm et al., 2014; Black and Clark, 2016). Complement activation can also alter the expression of MMP-2 and MMP-9 in various cell types, including RPE (Bandyopadhyay and Rohrer, 2012). As discussed above, this can result in ECM turnover, neovascularization due to imbalances in VEGF secretion, altered ATP metabolism due to interactions with extracellular nucleosides, and increased purinergic signaling.
MAC deposition does not always result in lysis of host cells due to the presence of regulatory proteins and active repair processes. For example, active repair processes such as MAC plugging, exocytosis, and endocytosis repair cell membranes and remove MAC pores before lysis can take place to limit sustained elevations in intracellular calcium (Kunchithapautham and Rohrer, 2011). Other regulatory processes include CD59, a membrane-bound GPI-anchored protein that inhibits the addition of C9 into the C5b-8/9 complex on host cells, which limits mean MAC lesion size (Kunchithapautham and Rohrer, 2011). Soluble inhibitors such as vitronectin or clusterin that bind to the C5b-7 structure of the MAC can also prevent its attachment to cell membranes, rendering it water-soluble and inactive (Kunchithapautham and Rohrer, 2011). Notably, these changes in MAC lesion size and binding affect the kinetics of ATP release and ion flux thereby influencing aspects of purinergic signaling.
Under sublytic conditions, several effects have been described that are hypothesized to contribute to the development and progression of both dry and wet forms of AMD. For instance, sublytic MAC formation can activate signaling pathways related to calcium, receptor tyrosine kinases, phospholipase C, protein kinase C, phospholipase 2α, and other extracellular signal-regulated kinases (Cybulsky et al., 2005; Fosbrink et al., 2005). This can lead to changes in cellular response including secretion, adherence, aggregation, chemotaxis, cell division, and impacts on membrane function (Bohana-Kashtan et al., 2004). In RPE cells, sublytic MAC increases the production of cytokines IL-6, IL-8, and MCP-1, which may contribute to early AMD (Lueck et al., 2011). Increased expression of MMP-2 and MMP-9 and VEGF are also associated with sublytic MAC formation on RPE and correlate with both remodeling of the choriocapillaris and neovascular processes seen in wet AMD (Thurman et al., 2009; Lueck et al., 2011). This is because VEGF, present in granular vesicles, is secreted via exocytosis following depolarization of cell membranes through activation of voltage-gated calcium channels. Calcium influx also activates the Ras/Erk pathway known to be involved in the regulated secretion of VEGF (Kunchithapautham and Rohrer, 2011). Additionally, P2X7 receptor activation also triggers VEGF release (Hill et al., 2010; Adinolfi et al., 2012). Thus, sublytic MAC formation and purinergic signaling influences intracellular signaling pathways that result in growth factor secretion (Lueck et al., 2011).
Various membrane pore-forming toxins, such as α-haemolysin, leukotoxin, and α-toxin, have also been shown to exert their toxic effects through autocrine and paracrine signaling in human erythrocytes (Birke et al., 2013) leading to complement-mediated lysis amplified by ATP release and P2X receptor activation (Birke et al., 2013). Additionally, amyloid-β protein aggregates and other pore forming toxins may lead to sublytic membrane damage and subsequent release of cellular components such as ATP, IL-1β, and IL-18 (Sanz et al., 2009; Ciudad et al., 2020). However, the underlying mechanisms and processes surrounding complement amplification, MAC deposition, and P2X receptor activation are not yet fully understood. We hypothesize that MAC deposition leads to an increase in eATP and subsequent P2X receptor activation. This results in an enhancement of ion flux, which has an impact on mitochondrial potential, the formation of ROS, inflammasome activation, and other intracellular changes leading to a feedback loop that allows for more MAC deposition. Further research is needed to establish these connections.
Overall, sublytic MAC and pore formation results in the remodeling of the choriocapillaris which contributes to the buildup of drusen, enhances complement activation and NLRP3 inflammasome activity, and leads to increased inflammation through cytokine release and recruitment of immune cells. Chronic inflammatory changes impact the overlying RPE, and the outer retina responds through additional signaling resulting in CNV, or regression of the choriocapillaris forming “ghost” vessels, subsequent RPE loss, and photoreceptor death in GA (Kumar-Singh, 2019).
Inflammasomes are multimolecular complexes comprised of three protein constituents: a NOD-like receptor, the adaptor protein apoptosis-associated speck-like protein containing a caspase recruitment domain (ASC), and pro-caspase 1 (Latz et al., 2013). Their activation consists of a two-step process in which both an initial priming signal and an activating signal are required (Latz et al., 2013). The initial priming signal is initiated by pathogen-associated molecular patterns (PAMPs) that stimulate toll-like receptors (TLRs) leading to the activation of NF-κB followed by the subsequent transcriptional upregulation of NOD-like receptor family pyrin domain containing 3 (NLRP3) and pro-interleukin-1β (Latz et al., 2013; Kelley et al., 2019). This is especially important in non-immune cells such as the RPE where basal expression levels are considered insufficient to initiate inflammasome assembly (Tseng et al., 2013; Narendran et al., 2021). Next, an activation signal is provided by a broad variety of molecules classified as either PAMPs or damage-associated molecular patterns (DAMPs) such as toxins, pathogens, metabolites, crystalline substances, nucleic acids, ion flux, reactive oxygen species, and ATP (Latz et al., 2013; Malik and Kanneganti, 2017; Zheng et al., 2020). In the case of P2X7 activation, eATP acting as a DAMP is detected by P2X7. Following activation, inflammasomes lead to a unique inflammatory programmed cell death pathway known as pyroptosis (Kelley et al., 2019).
Pyroptosis is executed by a family of pore-forming proteins known as gasdermins (GSDMs) (Shi et al., 2015). In humans, the current members of the GSDM family include GSDMA, GSDMB, GSDMC, GSDMD, and GSDME, which contain an autoinhibitory carboxyterminal domain and a pore-forming amino-terminal domain responsible for perforating the plasma membrane of cells (Orning et al., 2019; Broz et al., 2020; Liu et al., 2021). Typically, pyroptotic cell death initiates following the activation of the NLRP3 inflammasome and most often results in GSDMD pore formation and release of ATP (He et al., 2015; Kayagaki et al., 2015; Shi et al., 2015). Proinflammatory cytokines such as IL-1β and IL-18 are also released through the nonselective 10–14 nM gasdermin pore (Latz et al., 2013; Yu et al., 2021) which induce both inflammatory an apoptotic effects (Martinon et al., 2002; Ambati et al., 2013). However, while there are cytotoxic effects of IL-18 and IL-1β on the RPE, studies have also shown beneficial effects of inflammasome-mediated IL-18 release through the inhibition of neovascularization in an acute laser-induced injury model of neovascular AMD (Doyle et al., 2012). These contrasting findings imply that a single factor (IL-18) or pathway (NLRP3 inflammasome activation) can be simultaneously anti-angiogenic and destructive to the RPE and that Toll-like receptor 3 (TLR3) activation may be beneficial in terms of decreasing choroidal neovascularization while also promoting RPE degeneration (Ambati et al., 2013). In contrast to the reported anti-angiogenic effects of IL-18, IL-1β promotes neovascularization (Lavalette et al., 2011).
Based on the mechanisms described above, activation of the NLRP3 inflammasome and various gasdermin proteins have been implicated in the pathogenesis of AMD and several pathways have been suggested to trigger inflammasome activation in the outer retina including lipofuscin component A2E, accumulated Alu RNA, drusen components, amyloid-β, lipid peroxidation products, photooxidative damage, lysosomal destabilizations, particulate matter, overexpression of VEGF, and eATP (Ambati et al., 2013; Kerur et al., 2013). For example, the formation of amyloid-β oligomers (AβOs), which are aggregates of amyloid-β peptides and a major proinflammatory component of drusen (Luibl et al., 2006), can lead to RPE degeneration and GA through AβO-induced priming, assembly, and activation of the NLRP3 inflammasome in RPE cells. This occurs through a P2X7-mediated pathway, in which amyloid-β protein aggregates form a conductivity pore resulting in membrane damage and subsequent release of cellular components such as ATP and inflammatory mediators (Sanz et al., 2009; Ciudad et al., 2020). Like other mechanisms of NLRP3 inflammasome activation, AβO-induced AMD models have been demonstrated to result in the expression of GSDMD (Sun et al., 2018), along with RPE cytotoxicity driven by mitochondrial dysfunction and ROS formation (Sorbara and Girardin, 2011; Zhou et al., 2011).
Additionally, repetitive element-derived Alu RNA transcripts, non-canonical targets of DICER1-mediated enzymatic degradation, accumulate in human GA following the loss of DICER1 expression and are capable of activating P2X7 and the NLRP3 inflammasome to cause cell death of the retinal pigment epithelium in GA (Fowler et al., 2014). This is because Alu RNA transcripts can function as both priming and activating signals for inflammasome signaling (Ambati et al., 2013; Kerur et al., 2013). As a result of this pathway and the fact that Alu RNA transcripts require reverse transcriptase, multiple nucleoside reverse transcriptase inhibitors (NRTIs) have been investigated and found to be efficacious in inhibiting P2X7-mediated NLRP3 inflammasome activation in mouse models of GA, CNV, and other P2X7 driven diseases (Fowler et al., 2014).
Links between purinergic signaling and NLRP3 inflammasome activation are also well-defined as a result of ATP acting as a paracrine or autocrine signal in response to cell death or other stimuli (i.e., increased pressure, hypoxic injury, or complement-mediated damage). In these scenarios, the high amount of passive ATP release from cells activates the inflammasome through a P2X7R-dependent pyroptotic cell death pathway (Yang et al., 2015). Activated caspase-11 may also cleave pannexin-1 channels, inducing ATP release and P2X7R-related pyroptosis (Yang et al., 2015). Other nucleotide metabolites such as ADP, UTP, UDP, UDP glucose, and adenosine, along with other members of the purinergic receptor family (i.e., P2X, P2Y, and P1 receptors), may also contribute through complex purinergic signaling networks (Gombault et al., 2013).
Taken together, an increasing body of evidence suggests that the retina can respond to diverse danger signals including unregulated ATP release via purinergic signaling leading to NLRP3 inflammasome activation (Gombault et al., 2013; Gao et al., 2015; Yang et al., 2020), GSDM pore formation, and pyroptosis. Therefore, inhibition of P2X receptors and NLRP3 activation has been identified as putative drug targets in several models of AMD progression by delaying RPE degeneration in GA and/or slowing RPE barrier breakdown and neovascularization in CNV.
Purinergic signaling has been investigated for its role in the development of ocular pathologies such as AMD, glaucoma, and diabetic retinopathy. Despite the emergence of anti-VEGF agents to treat the wet form of late AMD, and the recently FDA-approved pegcetacoplan, a complement C3 inhibitor, to slow the progression of the dry form of late AMD, there are no approved drugs available to prevent the development of wet or dry AMD. For this reason, potential crosstalk between known contributors to AMD, such as complement dysregulation and inflammasome activation, and other cellular systems, such as purinergic signaling, must be considered (Figure 3).
Figure 3. Overview of mechanisms of ATP release and purinergic signaling in the pathogenesis of AMD.
As outlined in this review, the current literature surrounding purinergic signaling and AMD pathogenesis has focused primarily on the role of P2X7 receptor signaling. However, P2X7 has the highest EC50 for ATP in the P2X receptor family and may not be physiologically relevant throughout all stages of the development and progression of AMD, especially in the early stages of the disease. On the other hand, in advanced stages and under certain conditions, mechanisms may allow for P2X7 receptor activation through altered regulatory proteins, repair processes, and interactions between mediators such as MMPs and ectonucleosides leading to increased concentrations of ATP, increased purinergic signaling, and accelerated local inflammation contributing to the AMD pathogenesis.
To fully understand the potential roles that purinergic signaling plays in AMD, more research is needed surrounding the expression, distribution, functions, and interactions of P2X receptors with other systems, such as complement activation, within cells of the outer retina, RPE, choroid, retinal vasculature, and the immune system. This must include further characterization of both homotrimeric purinergic receptors, such as P2X1, P2X2, P2X3, P2X4, P2X5, and P2X6, along with heterotrimeric receptors that can exhibit novel properties and functions.
Finally, while there are numerous mechanisms for ATP release within the outer retina, the role of purinergic signaling in both lytic and sublytic processes should be explored in the context of how these processes may amplify complement-induced lysis, a mechanism that has not yet been fully elucidated. This may involve processes that make cells more vulnerable to MAC deposition following P2X activation, such as crosstalk between complement and P2X receptor signaling, MMP-9 activation, and other spatial and temporal aspects of ATP release. In determining how these processes can influence and be influenced by purinergic signaling, it will improve our understanding of the mechanisms that drive AMD pathogenesis, which is critical in developing treatment strategies that prevent or slow the progression of the disease.
HM performed the data collection and analysis. HM, KJ, and JM wrote the manuscript. JM and CC conceived the review, obtained funding, and critically revised the manuscript. All authors have read and approved the final manuscript.
The authors would like to thank Lucas Chang for help in data collection and analysis in early stages of the review process.
CC was employed by Paragon Ventures Inc.
The remaining authors declare that the research was conducted in the absence of any commercial or financial relationships that could be construed as a potential conflict of interest.
All claims expressed in this article are solely those of the authors and do not necessarily represent those of their affiliated organizations, or those of the publisher, the editors and the reviewers. Any product that may be evaluated in this article, or claim that may be made by its manufacturer, is not guaranteed or endorsed by the publisher.
Adinolfi, E., Giuliani, A. L., De Marchi, E., Pegoraro, A., Orioli, E., and Di Virgilio, F. (2018). The P2X7 receptor: a main player in inflammation. Biochem. Pharmacol. 151, 234–244. doi: 10.1016/j.bcp.2017.12.021
Adinolfi, E., Raffaghello, L., Giuliani, A. L., Cavazzini, L., Capece, M., Chiozzi, P., et al. (2012). Expression of P2X7 receptor increases in vivo tumor growth. Cancer Res. 72, 2957–2969. doi: 10.1158/0008-5472.CAN-11-1947
Agarwal, A., Rhoades, W. R., Hanout, M., Soliman, M. K., Sarwar, S., Sadiq, M. A., et al. (2015). Management of neovascular age-related macular degeneration: current state-of-the-art care for optimizing visual outcomes and therapies in development. Clin. Ophthalmol. 9, 1001–1015. doi: 10.2147/OPTH.S74959
Age-Related Eye Disease Study Research Group (2001). A randomized, placebo-controlled, clinical trial of high-dose supplementation with vitamins C and E, beta carotene, and zinc for age-related macular degeneration and vision loss: AREDS report no. 8. Arch. Ophthalmol. 119, 1417–1436. doi: 10.1001/archopht.119.10.1417
Ambati, J., Atkinson, J. P., and Gelfand, B. D. (2013). Immunology of age-related macular degeneration. Nat. Rev. Immunol. 13, 438–451. doi: 10.1038/nri3459
Ambati, J., and Fowler, B. J. (2012). Mechanisms of age-related macular degeneration. Neuron 75, 26–39. doi: 10.1016/j.neuron.2012.06.018
Armento, A., Ueffing, M., and Clark, S. J. (2021). The complement system in age-related macular degeneration. Cell. Mol. Life Sci. 78, 4487–4505. doi: 10.1007/s00018-021-03796-9
Bandyopadhyay, M., and Rohrer, B. (2012). Matrix metalloproteinase activity creates pro-angiogenic environment in primary human retinal pigment epithelial cells exposed to complement. Investigative ophthalmology & visual science 53, 1953–1961. doi: 10.1167/iovs.11-8638
Behnke, V., Wolf, A., and Langmann, T. (2020). The role of lymphocytes and phagocytes in age-related macular degeneration (AMD). Cell. Mol. Life Sci. 77, 781–788. doi: 10.1007/s00018-019-03419-4
Berglin, L., Sarman, S., van der Ploeg, I., Steen, B., Ming, Y., Itohara, S., et al. (2003). Reduced choroidal neovascular membrane formation in matrix metalloproteinase-2-deficient mice. Invest. Ophthalmol. Vis. Sci. 44, 403–408. doi: 10.1167/iovs.02-0180
Bhutto, I., and Lutty, G. (2012). Understanding age-related macular degeneration (AMD): relationships between the photoreceptor/retinal pigment epithelium/Bruch's membrane/choriocapillaris complex. Mol. Asp. Med. 33, 295–317. doi: 10.1016/j.mam.2012.04.005
Bhutto, I. A., McLeod, D. S., Jing, T., Sunness, J. S., Seddon, J. M., and Lutty, G. A. (2016). Increased choroidal mast cells and their degranulation in age-related macular degeneration. Br. J. Ophthalmol. 100, 720–726. doi: 10.1136/bjophthalmol-2015-308290
Birke, K., Lipo, E., Birke, M. T., and Kumar-Singh, R. (2013). Topical application of PPADS inhibits complement activation and choroidal neovascularization in a model of age-related macular degeneration. PLoS One 8:e76766. doi: 10.1371/journal.pone.0076766
Black, J. R., and Clark, S. J. (2016). Age-related macular degeneration: genome-wide association studies to translation. Genet. Med. 18, 283–289. doi: 10.1038/gim.2015.70
Bohana-Kashtan, O., Ziporen, L., Donin, N., Kraus, S., and Fishelson, Z. (2004). Cell signals transduced by complement. Mol. Immunol. 41, 583–597. doi: 10.1016/j.molimm.2004.04.007
Broz, P., Pelegrín, P., and Shao, F. (2020). The gasdermins, a protein family executing cell death and inflammation. Nat. Rev. Immunol. 20, 143–157. doi: 10.1038/s41577-019-0228-2
Bulanova, E., and Bulfone-Paus, S. (2010). P2 receptor-mediated signaling in mast cell biology. Puriner. Signal. 6, 3–17. doi: 10.1007/s11302-009-9173-z
Burnstock, G. (2008). “Purinergic System” in Encyclopedia of molecular pharmacology. eds. S. Offermanns and W. Rosenthal (Berlin, Heidelberg: Springer)
Burnstock, G. (2016). P2X ion channel receptors and inflammation. Purinergic Signal. 12, 59–67. doi: 10.1007/s11302-015-9493-0
Burnstock, G. (2017). Purinergic signalling: therapeutic developments. Front. Pharmacol. 8:661. doi: 10.3389/fphar.2017.00661
Burnstock, G. (2018). Purine and purinergic receptors. Brain Neurosci. Adv. 2:2398212818817494. doi: 10.1177/2398212818817494
Burnstock, G., and Dale, N. (2015). Purinergic signalling during development and ageing. Purinergic Signal. 11, 277–305. doi: 10.1007/s11302-015-9452-9
Caraffa, A. L., Conti, C., D Ovidio, C., Gallenga, C. E., Tettamanti, L., Mastrangelo, F., et al. (2018). New concepts in neuroinflammation: mast cells pro-inflammatory and anti-inflammatory cytokine mediators. J. Biol. Regul. Homeost. Agents 32, 449–454.
Chen, E. R., and Kaiser, P. K. (2020). Therapeutic potential of the Ranibizumab port delivery system in the treatment of AMD: evidence to date. Clin. Ophthalmol. 14, 1349–1355. doi: 10.2147/OPTH.S194234
Ciudad, S., Puig, E., Botzanowski, T., Meigooni, M., Arango, A. S., Do, J., et al. (2020). Aβ(1-42) tetramer and octamer structures reveal edge conductivity pores as a mechanism for membrane damage. Nat. Commun. 11:3014. doi: 10.1038/s41467-020-16566-1
Clapp, C., Diaz-Lezama, N., Adan-Castro, E., Ramirez-Hernandez, G., Moreno-Carranza, B., Sarti, A. C., et al. (2019). Pharmacological blockade of the P2X7 receptor reverses retinal damage in a rat model of type 1 diabetes. Acta Diabetol. 56, 1031–1036. doi: 10.1007/s00592-019-01343-4
Cybulsky, A. V., Takano, T., Papillon, J., Bijian, K., and Guillemette, J. (2005). Activation of the extracellular signal-regulated kinase by complement C5b-9. Am. J. Physiol. Renal Physiol. 289, F593–F603. doi: 10.1152/ajprenal.00066.2005
Dale, N., Butler, J., Dospinescu, V. M., and Nijjar, S. (2023). Channel-mediated ATP release in the nervous system. Neuropharmacology 227:109435. doi: 10.1016/j.neuropharm.2023.109435
de Jong, E. K., Geerlings, M. J., and den Hollander, A. I. (2020). Age-related macular degeneration. Genet. Genom. Eye Disease, 1, 155–180. doi: 10.1016/B978-0-12-816222-4.00010-1
Di Virgilio, F., Dal Ben, D., Sarti, A. C., Giuliani, A. L., and Falzoni, S. (2017). The P2X7 receptor in infection and inflammation. Immunity 47, 15–31. doi: 10.1016/j.immuni.2017.06.020
Di Virgilio, F., Giuliani, A. L., Vultaggio-Poma, V., Falzoni, S., and Sarti, A. C. (2018). Non-nucleotide agonists triggering P2X7 receptor activation and pore formation. Front. Pharmacol. 9:39. doi: 10.3389/fphar.2018.00039
Di Virgilio, F., Sarti, A. C., and Coutinho-Silva, R. (2020). Purinergic signaling, DAMPs, and inflammation. Am. J. Physiol. Cell Physiol. 318, C832–C835. doi: 10.1152/ajpcell.00053.2020
Di Virgilio, F., Vultaggio-Poma, V., Falzoni, S., and Giuliani, A. L. (2023). Extracellular ATP: A powerful inflammatory mediator in the central nervous system. Neuropharmacology 224:109333. doi: 10.1016/j.neuropharm.2022.109333
Doyle, S. L., Campbell, M., Ozaki, E., Salomon, R. G., Mori, A., Kenna, P. F., et al. (2012). NLRP3 has a protective role in age-related macular degeneration through the induction of IL-18 by drusen components. Nat. Med. 18, 791–798. doi: 10.1038/nm.2717
Dwyer, K. M., Kishore, B. K., and Robson, S. C. (2020). Conversion of extracellular ATP into adenosine: a master switch in renal health and disease. Nat. Rev. Nephrol. 16, 509–524. doi: 10.1038/s41581-020-0304-7
Fosbrink, M., Niculescu, F., and Rus, H. (2005). The role of c5b-9 terminal complement complex in activation of the cell cycle and transcription. Immunol. Res. 31, 37–46. doi: 10.1385/IR:31:1:37
Fowler, B. J., Gelfand, B. D., Kim, Y., Kerur, N., Tarallo, V., Hirano, Y., et al. (2014). Nucleoside reverse transcriptase inhibitors possess intrinsic anti-inflammatory activity. Science 346, 1000–1003. doi: 10.1126/science.1261754
Galli, S. J., and Tsai, M. (2008). Mast cells: versatile regulators of inflammation, tissue remodeling, host defense and homeostasis. J. Dermatol. Sci. 49, 7–19. doi: 10.1016/j.jdermsci.2007.09.009
Gao, J., Liu, R. T., Cao, S., Cui, J. Z., Wang, A., et al. (2015). NLRP3 inflammasome: activation and regulation in age-related macular degeneration. Mediat. Inflamm. 2015, 1–11. doi: 10.1155/2015/690243
Gombault, A., Baron, L., and Couillin, I. (2013). ATP release and purinergic signaling in NLRP3 inflammasome activation. Front. Immunol. 3:414. doi: 10.3389/fimmu.2012.00414
Gu, B. J., Baird, P. N., Vessey, K. A., Skarratt, K. K., Fletcher, E. L., Fuller, S. J., et al. (2013). A rare functional haplotype of the P2RX4 and P2RX7 genes leads to loss of innate phagocytosis and confers increased risk of age-related macular degeneration. FASEB J. 27, 1479–1487. doi: 10.1096/fj.12-215368
Gu, B. J., and Wiley, J. S. (2018). P2X7 as a scavenger receptor for innate phagocytosis in the brain. Br. J. Pharmacol. 175, 4195–4208. doi: 10.1111/bph.14470
Guo, C., Masin, M., Qureshi, O. S., and Murrell-Lagnado, R. D. (2007). Evidence for functional P2X4/P2X7 heteromeric receptors. Mol. Pharmacol. 72, 1447–1456. doi: 10.1124/mol.107.035980
He, W. T., Wan, H., Hu, L., Chen, P., Wang, X., Huang, Z., et al. (2015). Gasdermin D is an executor of pyroptosis and required for interleukin-1β secretion. Cell Res. 25, 1285–1298. doi: 10.1038/cr.2015.139
Hill, L. M., Gavala, M. L., Lenertz, L. Y., and Bertics, P. J. (2010). Extracellular ATP may contribute to tissue repair by rapidly stimulating purinergic receptor X7-dependent vascular endothelial growth factor release from primary human monocytes. J. Immunol. 185, 3028–3034. doi: 10.4049/jimmunol.1001298
Holz, F. G., Schmitz-Valckenberg, S., and Fleckenstein, M. (2014). Recent developments in the treatment of age-related macular degeneration. J. Clin. Invest. 124, 1430–1438. doi: 10.1172/JCI71029
Huang, Z., Xie, N., Illes, P., Di Virgilio, F., Ulrich, H., Semyanov, A., et al. (2021). From purines to purinergic signalling: molecular functions and human diseases. Signal Transduct. Target. Ther. 6:162. doi: 10.1038/s41392-021-00553-z
Illes, P., Khan, T. M., and Rubini, P. (2017). Neuronal P2X7 receptors revisited: do they really exist? J. Neurosci. Off. J. Soc. Neurosci. 37, 7049–7062. doi: 10.1523/JNEUROSCI.3103-16.2017
Illes, P., Müller, C. E., Jacobson, K. A., Grutter, T., Nicke, A., Fountain, S. J., et al. (2021). Update of P2X receptor properties and their pharmacology: IUPHAR review 30. Br. J. Pharmacol. 178, 489–514. doi: 10.1111/bph.1529
Illes, P., Rubini, P., Ulrich, H., Zhao, Y., and Tang, Y. (2020). Regulation of microglial functions by purinergic mechanisms in the healthy and diseased CNS. Cells 9:1108. doi: 10.3390/cells9051108
Jacobson, K. A., and Civan, M. M. (2016). Ocular purine receptors as drug targets in the eye. J. Ocul. Pharmacol. Therapeut. 32, 534–547. doi: 10.1089/jop.2016.0090
Jacobson, K. A., Delicado, E. G., Gachet, C., Kennedy, C., von Kügelgen, I., Li, B., et al. (2020). Update of P2Y receptor pharmacology: IUPHAR review 27. Br. J. Pharmacol. 177, 2413–2433. doi: 10.1111/bph.15005
Junger, W. G. (2011). Immune cell regulation by autocrine purinergic signalling. Nat. Rev. Immunol. 11, 201–212. doi: 10.1038/nri2938
Kahlenberg, J. M., and Kaplan, M. J. (2013). Little peptide, big effects: the role of LL-37 in inflammation and autoimmune disease. J. Immunol. 191, 4895–4901. doi: 10.4049/jimmunol.1302005
Kayagaki, N., Stowe, I. B., Lee, B. L., O'Rourke, K., Anderson, K., Warming, S., et al. (2015). Caspase-11 cleaves gasdermin D for non-canonical inflammasome signalling. Nature 526, 666–671. doi: 10.1038/nature15541
Kelley, N., Jeltema, D., Duan, Y., and He, Y. (2019). The NLRP3 inflammasome: an overview of mechanisms of activation and regulation. Int. J. Mol. Sci. 20:3328. doi: 10.3390/ijms20133328
Kempuraj, D., Thangavel, R., Natteru, P. A., Selvakumar, G. P., Saeed, D., Zahoor, H., et al. (2016). Neuroinflammation induces neurodegeneration. J. Neurol. Neurosurg. Spine 1:1003.
Kerur, N., Hirano, Y., Tarallo, V., Fowler, B. J., Bastos-Carvalho, A., Yasuma, T., et al. (2013). TLR-independent and P2X7-dependent signaling mediate Alu RNA-induced NLRP3 inflammasome activation in geographic atrophy. Invest. Ophthalmol. Vis. Sci. 54, 7395–7401. doi: 10.1167/iovs.13-12500
Klein, R. J., Zeiss, C., Chew, E. Y., Tsai, J. Y., Sackler, R. S., Haynes, C., et al. (2005). Complement factor H polymorphism in age-related macular degeneration. Science 308, 385–389. doi: 10.1126/science.1109557
Kronlage, M., Song, J., Sorokin, L., Isfort, K., Schwerdtle, T., Leipziger, J., et al. (2010). Autocrine purinergic receptor signaling is essential for macrophage chemotaxis. Sci. Signal. 3:ra55. doi: 10.1126/scisignal.2000588
Kukulski, F., Lévesque, S. A., and Sévigny, J. (2011). Impact of ectoenzymes on p2 and p1 receptor signaling. Adv. Pharmacol. 61, 263–299. doi: 10.1016/B978-0-12-385526-8.00009-6
Kumar-Singh, R. (2019). The role of complement membrane attack complex in dry and wet AMD – from hypothesis to clinical trials. Exp. Eye Res. 184, 266–277. doi: 10.1016/j.exer.2019.05.006
Kunchithapautham, K., and Rohrer, B. (2011). Sublytic membrane-attack-complex (MAC) activation alters regulated rather than constitutive vascular endothelial growth factor (VEGF) secretion in retinal pigment epithelium monolayers. J. Biol. Chem. 286, 23717–23724. doi: 10.1074/jbc.M110.214593
Kur, J., and Newman, E. A. (2014). Purinergic control of vascular tone in the retina. J. Physiol. 592, 491–504. doi: 10.1113/jphysiol.2013.267294
Kur, J., Newman, E. A., and Chan-Ling, T. (2012). Cellular and physiological mechanisms underlying blood flow regulation in the retina and choroid in health and disease. Prog. Retin. Eye Res. 31, 377–406. doi: 10.1016/j.preteyeres.2012.04.004
Kurashima, Y., Amiya, T., Nochi, T., Fujisawa, K., Haraguchi, T., Iba, H., et al. (2012). Extracellular ATP mediates mast cell-dependent intestinal inflammation through P2X7 purinoceptors. Nat. Commun. 3:1034. doi: 10.1038/ncomms2023
Latz, E., Xiao, T. S., and Stutz, A. (2013). Activation and regulation of the inflammasomes. Nat. Rev. Immunol. 13, 397–411. doi: 10.1038/nri3452
Lavalette, S., Raoul, W., Houssier, M., Camelo, S., Levy, O., Calippe, B., et al. (2011). Interleukin-1β inhibition prevents choroidal neovascularization and does not exacerbate photoreceptor degeneration. Am. J. Pathol. 178, 2416–2423. doi: 10.1016/j.ajpath.2011.01.013
Lazarowski, E. R., Boucher, R. C., and Harden, T. K. (2003). Mechanisms of release of nucleotides and integration of their action as P2X-and P2Y-receptor activating molecules. Mol. Pharmacol. 64, 785–795. doi: 10.1124/mol.64.4.785
Liu, X., Xia, S., Zhang, Z., Wu, H., and Lieberman, J. (2021). Channelling inflammation: gasdermins in physiology and disease. Nat. Rev. Drug Discov. 20, 384–405. doi: 10.1038/s41573-021-00154-z
Lueck, K., Wasmuth, S., Williams, J., Hughes, T. R., Morgan, B. P., Lommatzsch, A., et al. (2011). Sub-lytic C5b-9 induces functional changes in retinal pigment epithelial cells consistent with age-related macular degeneration. Eye 25, 1074–1082. doi: 10.1038/eye.2011.109
Luibl, V., Isas, J. M., Kayed, R., Glabe, C. G., Langen, R., and Chen, J. (2006). Drusen deposits associated with aging and age-related macular degeneration contain nonfibrillar amyloid oligomers. J. Clin. Invest. 116, 378–385. doi: 10.1172/JCI25843
Malik, A., and Kanneganti, T. D. (2017). Inflammasome activation and assembly at a glance. J. Cell Sci. 130, 3955–3963. doi: 10.1242/jcs.207365
Martinon, F., Burns, K., and Tschopp, J. (2002). The inflammasome: a molecular platform triggering activation of inflammatory caspases and processing of proIL-beta. Mol. Cell 10, 417–426. doi: 10.1016/s1097-2765(02)00599-3
Matsubara, J. A., Tian, Y., Cui, J. Z., Zeglinski, M. R., Hiroyasu, S., Turner, C. T., et al. (2020). Retinal distribution and extracellular activity of granzyme B: a serine protease that degrades retinal pigment epithelial tight junctions and extracellular matrix proteins. Front. Immunol. 11:574. doi: 10.3389/fimmu.2020.00574
Medina, C. B., Mehrotra, P., Arandjelovic, S., Perry, J. S. A., Guo, Y., Morioka, S., et al. (2020). Metabolites released from apoptotic cells act as tissue messengers. Nature 580, 130–135. doi: 10.1038/s41586-020-2121-3
Merle, N. S., Church, S. E., Fremeaux-Bacchi, V., and Roumenina, L. T. (2015a). Complement system part I - molecular mechanisms of activation and regulation. Front. Immunol. 6:262. doi: 10.3389/fimmu.2015.00262
Merle, N. S., Noe, R., Halbwachs-Mecarelli, L., Fremeaux-Bacchi, V., and Roumenina, L. T. (2015b). Complement system part II: role in immunity. Front. Immunol. 6:257. doi: 10.3389/fimmu.2015.00257
Mitchell, P., Liew, G., Gopinath, B., and Wong, T. Y. (2018). Age-related macular degeneration. Lancet 392, 1147–1159. doi: 10.1016/S0140-6736(18)31550-2
Mitchell, C. H., and Reigada, D. (2008). Purinergic signalling in the subretinal space: a role in the communication between the retina and the RPE. Purinergic signalling 4, 101–107. doi: 10.1007/s11302-007-9054-2
Morciano, G., Sarti, A. C., Marchi, S., Missiroli, S., Falzoni, S., Raffaghello, L., et al. (2017). Use of luciferase probes to measure ATP in living cells and animals. Nat. Protoc. 12, 1542–1562. doi: 10.1038/nprot.2017.052
Narendran, S., Pereira, F., Yerramothu, P., Apicella, I., Wang, S. B., Ambati, K., et al. (2021). Nucleoside reverse transcriptase inhibitors and Kamuvudines inhibit amyloid-β induced retinal pigmented epithelium degeneration. Signal Transduct. Target. Ther. 6:149. doi: 10.1038/s41392-021-00537-z
Newman, E. A. (2015). Glial cell regulation of neuronal activity and blood flow in the retina by release of gliotransmitters. Philos. Trans. R. Soc. Lond. Ser. B Biol. Sci. 370:20140195. doi: 10.1098/rstb.2014.0195
Niyadurupola, N., Sidaway, P., Ma, N., Rhodes, J. D., Broadway, D. C., and Sanderson, J. (2013). P2X7 receptor activation mediates retinal ganglion cell death in a human retina model of ischemic neurodegeneration. Invest. Ophthalmol. Vis. Sci. 54, 2163–2170. doi: 10.1167/iovs.12-10968
Notomi, S., Hisatomi, T., Kanemaru, T., Takeda, A., Ikeda, Y., Enaida, H., et al. (2011). Critical involvement of extracellular ATP acting on P2RX7 purinergic receptors in photoreceptor cell death. Am. J. Pathol. 179, 2798–2809. doi: 10.1016/j.ajpath.2011.08.035
Notomi, S., Hisatomi, T., Murakami, Y., Terasaki, H., Sonoda, S., Asato, R., et al. (2013). Dynamic increase in extracellular ATP accelerates photoreceptor cell apoptosis via ligation of P2RX7 in subretinal hemorrhage. PLoS ONE 8:e53338. doi: 10.1371/journal.pone.0053338
Ogura, S., Baldeosingh, R., Bhutto, I. A., Kambhampati, S. P., Scott McLeod, D., Edwards, M. M., et al. (2020). A role for mast cells in geographic atrophy. FASEB J. 34, 10117–10131. doi: 10.1096/fj.202000807R
Orning, P., Lien, E., and Fitzgerald, K. A. (2019). Gasdermins and their role in immunity and inflammation. J. Exp. Med. 216, 2453–2465. doi: 10.1084/jem.20190545
Pellegatti, P., Falzoni, S., Pinton, P., Rizzuto, R., and Di Virgilio, F. (2005). A novel recombinant plasma membrane-targeted luciferase reveals a new pathway for ATP secretion. Mol. Biol. Cell 16, 3659–3665. doi: 10.1091/mbc.e05-03-0222
Pellegatti, P., Raffaghello, L., Bianchi, G., Piccardi, F., Pistoia, V., and Virgilioa, F.. (2008). Increased level of extracellular ATP at tumor sites: in vivo imaging with plasma membrane luciferase. PloS one, 3, e2599. doi: 10.1371/journal.pone.0002599
Platania, C. B. M., Lazzara, F., Fidilio, A., Fresta, C. G., Conti, F., Giurdanella, G., et al. (2019). Blood-retinal barrier protection against high glucose damage: the role of P2X7 receptor. Biochem. Pharmacol. 168, 249–258. doi: 10.1016/j.bcp.2019.07.010
Ralevic, V., and Burnstock, G. (1998). Receptors for purines and pyrimidines. Pharmacol. Rev. 50, 413–492.
Rasmussen, A., and Sander, B. (2014). Long-term longitudinal study of patients treated with ranibizumab for neovascular age-related macular degeneration. Curr. Opin. Ophthalmol. 25, 158–163. doi: 10.1097/ICU.0000000000000050
Ricklin, D., Hajishengallis, G., Yang, K., and Lambris, J. D. (2010). Complement: a key system for immune surveillance and homeostasis. Nat. Immunol. 11, 785–797. doi: 10.1038/ni.1923
Romagnani, A., Rottoli, E., Mazza, E. M. C., Rezzonico-Jost, T., De Ponte Conti, B., Proietti, M., et al. (2020). P2X7 receptor activity limits accumulation of T cells within tumors. Cancer Res. 80, 3906–3919. doi: 10.1158/0008-5472.CAN-19-3807
Sakaki, H., Tsukimoto, M., Harada, H., Moriyama, Y., and Kojima, S. (2013). Autocrine regulation of macrophage activation via exocytosis of ATP and activation of P2Y11 receptor. PLoS One 8:e59778. doi: 10.1371/journal.pone.0059778
Salcman, B., Affleck, K., and Bulfone-Paus, S. (2021). P2X receptor-dependent modulation of mast cell and glial cell activities in Neuroinflammation. Cells 10:2282. doi: 10.3390/cells10092282
Sanderson, J., Dartt, D. A., Trinkaus-Randall, V., Pintor, J., Civan, M. M., Delamere, N. A., et al. (2014). Purines in the eye: recent evidence for the physiological and pathological role of purines in the RPE, retinal neurons, astrocytes, Müller cells, lens, trabecular meshwork, cornea and lacrimal gland. Exp. Eye Res. 127, 270–279. doi: 10.1016/j.exer.2014.08.009
Santiago, A., Madeira, M., Boia, R., Aires, I., Rodrigues-Neves, A., Santos, P., et al. (2020). Keep an eye on adenosine: its role in retinal inflammation. Pharmacol. Ther. 210, 1–22. doi: 10.1016/j.pharmthera.2020.107513
Sanz, J. M., Chiozzi, P., Ferrari, D., Colaianna, M., Idzko, M., Falzoni, S., et al. (2009). Activation of microglia by amyloid {beta} requires P2X7 receptor expression. J. Immunol. (Baltimore, Md.: 1950). 182(7), 4378–4385. doi: 10.4049/jimmunol.0803612
Sarma, J. V., and Ward, P. A. (2011). The complement system. Cell Tissue Res. 343, 227–235. doi: 10.1007/s00441-010-1034-0
Schramm, E. C., Clark, S. J., Triebwasser, M. P., Raychaudhuri, S., Seddon, J., and Atkinson, J. P. (2014). Genetic variants in the complement system predisposing to age-related macular degeneration: a review. Mol. Immunol. 61, 118–125. doi: 10.1016/j.molimm.2014.06.032
Schwiebert, E. M., and Zsembery, A. (2003). Extracellular ATP as a signaling molecule for epithelial cells. Biochim. Biophys. Acta 1615, 7–32. doi: 10.1016/s0005-2736(03)00210-4
Shen, W., Fruttiger, M., Zhu, L., Chung, S. H., Barnett, N. L., Kirk, J. K., et al. (2012). Conditional Muller cell ablation causes independent neuronal and vascular pathologies in a novel transgenic model. J. Neurosci. 32, 15715–15727. doi: 10.1523/JNEUROSCI.2841-12.2012
Shi, J., Zhao, Y., Wang, K., Shi, X., Wang, Y., Huang, H., et al. (2015). Cleavage of GSDMD by inflammatory caspases determines pyroptotic cell death. Nature 526, 660–665. doi: 10.1038/nature15514
Shieh, C. H., Heinrich, A., Serchov, T., van Calker, D., and Biber, K. (2014). P2X7-dependent, but differentially regulated release of IL-6, CCL2, and TNF-α in cultured mouse microglia. Glia 62, 592–607. doi: 10.1002/glia.22628
Shinozaki, Y., Saito, K., Kashiwagi, K., and Koizumi, S. (2023). Ocular P2 receptors and glaucoma. Neuropharmacology 222:109302. doi: 10.1016/j.neuropharm.2022.109302
Sorbara, M. T., and Girardin, S. E. (2011). Mitochondrial ROS fuel the inflammasome. Cell Res. 21, 558–560. doi: 10.1038/cr.2011.20
Stahl, A. (2020). The diagnosis and treatment of age-related macular degeneration. Deutsch. Arztebl. Int. 117, 513–520. doi: 10.3238/arztebl.2020.0513
Strauss, O. (2005). The retinal pigment epithelium in visual function. Physiol. Rev. 85, 845–881. doi: 10.1152/physrev.00021.2004
Sun, Y., Zheng, Y., Wang, C., and Liu, Y. (2018). Glutathione depletion induces ferroptosis, autophagy, and premature cell senescence in retinal pigment epithelial cells. Cell Death Dis. 9:753. doi: 10.1038/s41419-018-0794-4
Theoharides, T. C., Alysandratos, K. D., Angelidou, A., Delivanis, D. A., Sismanopoulos, N., Zhang, B., et al. (2012). Mast cells and inflammation. Biochim. Biophys. Acta 1822, 21–33. doi: 10.1016/j.bbadis.2010.12.014
Thurman, J. M., Renner, B., Kunchithapautham, K., Ferreira, V. P., Pangburn, M. K., Ablonczy, Z., et al. (2009). Oxidative stress renders retinal pigment epithelial cells susceptible to complement-mediated injury. J. Biol. Chem. 284, 16939–16947. doi: 10.1074/jbc.M808166200
Tomasinsig, L., Pizzirani, C., Skerlavaj, B., Pellegatti, P., Gulinelli, S., Tossi, A., et al. (2008). The human cathelicidin LL-37 modulates the activities of the P2X7 receptor in a structure-dependent manner. J. Biol. Chem. 283, 30471–30481. doi: 10.1074/jbc.M802185200
Tseng, W. A., Thein, T., Kinnunen, K., Lashkari, K., Gregory, M. S., D'Amore, P. A., et al. (2013). NLRP3 inflammasome activation in retinal pigment epithelial cells by lysosomal destabilization: implications for age-related macular degeneration. Invest. Ophthalmol. Vis. Sci. 54, 110–120. doi: 10.1167/iovs.12-10655
Ventura, A. L. M., Mitchell, C. H., and Faillace, M. P. (2019). Purinergic signaling in the retina: from development to disease. Brain Res. Bull. 151, 92–108. doi: 10.1016/j.brainresbull.2018.10.016
Vessey, K. A., Gu, B. J., Jobling, A. I., Phipps, J. A., Greferath, U., Tran, M. X., et al. (2017). Loss of function of P2X7 receptor scavenger activity in aging mice: a novel model for investigating the early pathogenesis of age-related macular degeneration. Am. J. Pathol. 187, 1670–1685. doi: 10.1016/j.ajpath.2017.04.016
Wagner, A. H., Anand, V. N., Wang, W. H., Chatterton, J. E., Sun, D., Shepard, A. R., et al. (2013). Exon-level expression profiling of ocular tissues. Exp. Eye Res. 111, 105–111. doi: 10.1016/j.exer.2013.03.004
Wakx, A., Dutot, M., Massicot, F., Mascarelli, F., Limb, G. A., and Rat, P. (2016). Amyloid β peptide induces apoptosis through P2X7 cell death receptor in retinal cells: modulation by marine Omega-3 fatty acid DHA and EPA. Appl. Biochem. Biotechnol. 178, 368–381. doi: 10.1007/s12010-015-1878-6
Wareham, K. J., and Seward, E. P. (2016). P2X7 receptors induce degranulation in human mast cells. Purinergic Signal. 12, 235–246. doi: 10.1007/s11302-016-9497-4
Wareham, K., Vial, C., Wykes, R., Bradding, P., and Seward, E. (2009). Functional evidence for the expression of P2X1, P2X4 and P2X7 receptors in human lung mast cells. Br. J. Pharmacol. 157, 1215–1224. doi: 10.1111/j.1476-5381.2009.00287.x
Wong, W. L., Su, X., Li, X., Cheung, C. M., Klein, R., Cheng, C. Y., et al. (2014). Global prevalence of age-related macular degeneration and disease burden projection for 2020 and 2040: a systematic review and meta-analysis. Lancet Glob. Health 2, e106–e116. doi: 10.1016/S2214-109X(13)70145-1
Yancopoulos, G. D., Davis, S., Gale, N. W., Rudge, J. S., Wiegand, S. J., and Holash, J. (2000). Vascular-specific growth factors and blood vessel formation. Nature 407, 242–248. doi: 10.1038/35025215
Yang, D., Elner, S. G., Clark, A. J., Hughes, B. A., Petty, H. R., and Elner, V. M. (2011). Activation of P2X receptors induces apoptosis in human retinal pigment epithelium. Invest. Ophthalmol. Vis. Sci. 52, 1522–1530. doi: 10.1167/iovs.10-6172
Yang, D., He, Y., Muñoz-Planillo, R., Liu, Q., and Núñez, G. (2015). Caspase-11 requires the Pannexin-1 channel and the purinergic P2X7 pore to mediate pyroptosis and endotoxic shock. Immunity 43, 923–932. doi: 10.1016/j.immuni.2015.10.009
Yang, M., So, K. F., Lam, W. C., and Lo, A. (2020). Novel programmed cell death as therapeutic targets in age-related macular degeneration? Int. J. Mol. Sci. 21:7279. doi: 10.3390/ijms21197279
Ye, S. S., Tang, Y., and Song, J. T. (2021). ATP and adenosine in the retina and retinal diseases. Front. Pharmacol. 12:654445. doi: 10.3389/fphar.2021.654445
Yu, P., Zhang, X., Liu, N., Tang, L., Peng, C., and Chen, X. (2021). Pyroptosis: mechanisms and diseases. Signal Transduct. Target. Ther. 6:128. doi: 10.1038/s41392-021-00507-5
Zhang, W., Zhou, S., Liu, G., Kong, F., Chen, S., and Yan, H. (2018). Multiple steps determine CD73 shedding from RPE: lipid raft localization, ARA1 interaction, and MMP-9 up- regulation. Purinergic Signal. 14, 443–457. doi: 10.1007/s11302-018-9628-1
Zheng, D., Liwinski, T., and Elinav, E. (2020). Inflammasome activation and regulation: toward a better understanding of complex mechanisms. Cell Discov. 6:36. doi: 10.1038/s41421-020-0167-x
Zhou, R., Yazdi, A. S., Menu, P., and Tschopp, J. (2011). A role for mitochondria in NLRP3 inflammasome activation. Nature 469, 221–225. doi: 10.1038/nature09663
Keywords: purinergic signaling, age-related macular degeneration, ATP, complement, inflammasome, P2X
Citation: Molcak H, Jiang K, Campbell CJ and Matsubara JA (2023) Purinergic signaling via P2X receptors and mechanisms of unregulated ATP release in the outer retina and age-related macular degeneration. Front. Neurosci. 17:1216489. doi: 10.3389/fnins.2023.1216489
Received: 03 May 2023; Accepted: 26 June 2023;
Published: 11 July 2023.
Edited by:
Dan Wen, Central South University, ChinaReviewed by:
Michael Risner, Vanderbilt University, United StatesCopyright © 2023 Molcak, Jiang, Campbell and Matsubara. This is an open-access article distributed under the terms of the Creative Commons Attribution License (CC BY). The use, distribution or reproduction in other forums is permitted, provided the original author(s) and the copyright owner(s) are credited and that the original publication in this journal is cited, in accordance with accepted academic practice. No use, distribution or reproduction is permitted which does not comply with these terms.
*Correspondence: Joanne A. Matsubara, am1zQG1haWwudWJjLmNh
Disclaimer: All claims expressed in this article are solely those of the authors and do not necessarily represent those of their affiliated organizations, or those of the publisher, the editors and the reviewers. Any product that may be evaluated in this article or claim that may be made by its manufacturer is not guaranteed or endorsed by the publisher.
Research integrity at Frontiers
Learn more about the work of our research integrity team to safeguard the quality of each article we publish.