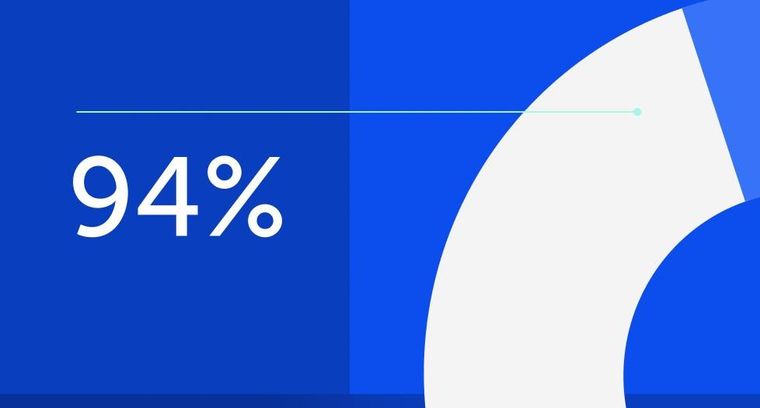
94% of researchers rate our articles as excellent or good
Learn more about the work of our research integrity team to safeguard the quality of each article we publish.
Find out more
REVIEW article
Front. Neurosci., 13 October 2023
Sec. Translational Neuroscience
Volume 17 - 2023 | https://doi.org/10.3389/fnins.2023.1210949
This article is part of the Research TopicThe Thalamus in Brain DisordersView all 5 articles
The thalamus acts as an interface between the periphery and the cortex, with nearly every sensory modality processing information in the thalamocortical circuit. Despite well-established thalamic nuclei for visual, auditory, and tactile modalities, the key thalamic nuclei responsible for innocuous thermosensation remains under debate. Thermosensory information is first transduced by thermoreceptors located in the skin and then processed in the spinal cord. Temperature information is then transmitted to the brain through multiple spinal projection pathways including the spinothalamic tract and the spinoparabrachial tract. While there are fundamental studies of thermal transduction via thermosensitive channels in primary sensory afferents, thermal representation in the spinal projection neurons, and encoding of temperature in the primary cortical targets, comparatively little is known about the intermediate stage of processing in the thalamus. Multiple thalamic nuclei have been implicated in thermal encoding, each with a corresponding cortical target, but without a consensus on the role of each pathway. Here, we review a combination of anatomy, physiology, and behavioral studies across multiple animal models to characterize the thalamic representation of temperature in two proposed thermosensory information streams.
The somatosensory system is a complex network of sensory receptors and neural pathways that enable us to perceive and respond to stimuli from the external environment. Somatosensation refers to the collective experience of these sensory modalities, including touch, temperature, itch, proprioception, and pain. Although touch has been extensively studied, the same cannot be said for temperature, particularly in the context of innocuous stimuli. The ability to perceive changes in temperature is crucial for maintaining homeostasis, detecting potential danger, and responding appropriately to environmental stimuli by serving as necessary information used during active haptic exploration. Hence, investigating the neural mechanisms underlying non-painful thermal stimulation is critical for understanding the broader context of somatosensation. In this article, we focus on the neural pathways implicated in thermosensation and highlight the relevance of two parallel thalamic nuclei and their cortical targets in thermal perception.
Thermal information is transduced by Aδ and C primary afferent fibers innervating the skin that terminate primarily in laminae I and II of the ipsilateral dorsal horn of the spinal cord for sensations arising on the body surface or the spinal trigeminal nucleus for sensations on the face (Light et al., 1979; Light and Perl, 1979a,b; Cervero et al., 1984; Cervero and Connell, 1984; Maxwell and Rethlyi, 1987). Lamina I spinal cord neurons are driven by nociceptive, thermal, and itch stimuli [mouse: (Chisholm et al., 2021); cat: (Craig and Kniffki, 1985; Craig and Hunsley, 1991; Andrew and Craig, 2001; Craig and Dostrovsky, 2001); primate: (Kumazawa and Perl, 1978), rat: (Zhang and Giesler, 2005)]. Spinothalamic projection neurons originating in the superficial laminae of the spinal cord target multiple thalamic nuclei in the mouse (Davidson et al., 2010), the rat (Lund and Webster, 1967; Giesler et al., 1979; Granum, 1986; Ma et al., 1986; Burstein et al., 1990; Iwata et al., 1992; Gauriau and Bernard, 2004a), the cat (Boivie, 1971; Carstens and Trevino, 1978; Jones et al., 1987; Craig et al., 1989; Stevens et al., 1989; Katter et al., 1991; Craig, 2003a; Klop et al., 2005), and the primate (Boivie, 1979; Willis et al., 1979; Hayes and Rustioni, 1980; Apkarian and Hodge, 1989a,b; Gingold et al., 1991; Craig, 2004), in addition to several other non-thalamic targets (Kuner and Kuner, 2021) including the lateral parabrachial nucleus of the brainstem (Light et al., 1993; Bester et al., 2000).
The lateral parabrachial nucleus is responsive to cutaneous thermal inputs (Nakamura, 2011), receives collateral projections from spinothalamic neurons (Hylden et al., 1989; Li et al., 2006; Al-Khater and Todd, 2009), has distinct subregions for cold and warm activated neurons (Nakamura and Morrison, 2008, 2010; Geerling et al., 2016), and projects to the thermoregulatory center of the preoptic area of the hypothalamus (Nakamura and Morrison, 2008, 2010) as well as the central amygdaloid nucleus (Yahiro et al., 2023). Inactivation of lateral parabrachial neurons eliminates autonomic thermoregulatory responses, such as skin cooling-evoked brown fat and shivering thermogenesis and skin warming-evoked cutaneous vasodilation (Kobayashi and Osaka, 2003; Nakamura and Morrison, 2008, 2010). Further, lesions of the lateral parabrachial nucleus (Yahiro et al., 2023), but not the thalamus (Yahiro et al., 2017), can lead to drastic impairments on thermoregulatory behavioral assays. Therefore, while the thalamus is important for sensory perception (Wolff et al., 2021), the spinoparabrachial pathway is considered critical for thermoregulatory behavior independent of the thalamic representation of temperature, and therefore is not the focus of this perspective (for a review of the thermoregulatory pathway, see Nakamura, 2011; Tan and Knight, 2018).
While there are notable differences in the spinothalamic tract across species (Hodge and Apkarian, 1990), the two major thalamic subdivisions targeted by spinothalamic information traveling to cortex are the medial and the lateral thalamus (Craig, 2004). The lamina I—medial thalamus pathway, or the limbic pathway, sends input to the submedial nucleus to the thalamus [multi-species: (Craig and Burton, 1981); rat: (Yoshida et al., 1991; Iwata et al., 1992; Gauriau and Bernard, 2004a), cat:(Craig and Dostrovsky, 1991), primate: (Craig, 2004; Craig and Zhang, 2006)] which then projects to the anterior cingulate cortex (Xue et al., 2022). The lamina I—medial thalamus pathway is associated with pain processing and emotion (Vertes et al., 2015; Bliss et al., 2016), but has not been implicated in innocuous sensing. Rather, there is evidence that the submedial nucleus of the thalamus is exclusively driven by nociceptive stimuli (Dostrovsky and Guilbaud, 1988), and therefore is not the focus of this perspective on innocuous thermal perception (for a review of thalamocortical involvement in pain, see Albe-Fessar et al., 1985; Yen and Lu, 2013; Groh et al., 2017).
The lamina I—lateral thalamus pathway, or the sensorimotor pathway, is associated with nociceptive and thermal perception in rodents (Milenkovic et al., 2014; Ziegler et al., 2023), cats (Craig et al., 2001), and primates (Craig and Blomqvist, 2002). Within the lamina I—lateral thalamus pathway, there are two distinct subdivisions traveling through anterior and posterior somatosensory thalamic nuclei [rodent: (Lund and Webster, 1967; Ma et al., 1986; Zhang and Giesler, 2005), cat: (Boivie, 1971; Mantyh, 1983), non-human primate: (Pearson and Haines, 1980; Apkarian and Hodge, 1989c), human: (Hong et al., 2011)]. Although the anterior and the posterior thalamic nuclei are characterized by distinct thermal representations and cortical targets, the relevance for one pathway over the other for innocuous thermal sensation remains under debate (Craig et al., 1994; Willis et al., 2002; Montes et al., 2005). Here, we propose that the anterior and posterior thalamic thermosensory streams represent two parallel processing pathways for distinct features of thermosensation.
The anterior thermosensory stream is characterized by the ventral somatosensory thalamic nuclei targeted by spinothalamic neurons. Across species, lamina I spinothalamic tract neurons send axon collaterals to the ventral posterolateral (VPL) and ventral posteromedial (VPM) nuclei as well as to the ventral posterior inferior (VPI) nucleus in primates (Willis et al., 2001). These ventral somatosensory thalamic nuclei are characterized by their robust somatotopic map (Emmers, 1965) and axonal projections to primary (Bernardo and Woolsey, 1987; Shi and Apkarian, 1995) and secondary (Krubitzer and Kaas, 1992) somatosensory cortex for VPL/VPM and VPI, respectively. Therefore, the anterior thermosensory stream is defined as lamina I spinothalamic neurons projecting to ventral somatosensory thalamus and then on to somatosensory cortex.
While traditionally viewed as mechanosensory nuclei, ventral somatosensory thalamus encodes pain (Kenshalo et al., 1980; Casey and Morrow, 1983; Chung et al., 1986; Apkarian and Shi, 1994; Lenz et al., 1994), temperature (Bushnell et al., 1993), and proprioception (Francis et al., 2008). Discriminative mechanosensory information reaches somatosensory ventral thalamus via the dorsal column nuclei (Turecek et al., 2022) while temperature and nociceptive information arrives via the lamina I spinothalamic tract (Hodge and Apkarian, 1990; Willis et al., 2001). The density of the spinothalamic innervation of ventral somatosensory thalamic nuclei is less than that seen for posterior somatosensory thalamus (Al-Khater et al., 2008) which has led to the hypothesis that ventral thalamus is not critical for thermosensation (Craig et al., 1994). However, there is significant evidence that this pathway is also involved in thermosensation beyond mechanosensation (Willis et al., 2002). First, thermally evoked responses have been recorded at each stage of the anterior thermosensory pathway: in VPL-projecting spinothalamic neurons (Chung et al., 1979; Ferrington et al., 1987; Zhang et al., 2006; Davidson et al., 2008), in ventral somatosensory thalamus (Kenshalo et al., 1980; Bushnell et al., 1993; Lenz et al., 1993a; Apkarian and Shi, 1994; Lenz and Dougherty, 1998; Lee et al., 1999), and downstream in primary somatosensory cortex in mice (Milenkovic et al., 2014; Vestergaard et al., 2023) and humans (Guest et al., 2007). Second, human microstimulation studies have elicited cool percepts when the stimulation electrode is located within the ventral caudal thalamus (Lenz et al., 1993b; Ohara and Lenz, 2003). Finally, in monkeys, inactivation of ventral thalamus impaired behavioral performance on both thermosensory and nociceptive tasks, further supporting a role for ventral somatosensory thalamic nuclei in thermal perception (Bushnell et al., 1993). Taken together, this suggests that the anterior thermosensory stream is relevant for thermosensory encoding and perception.
The posterior thermosensory stream is defined by the posterior somatosensory thalamic nuclei targeted by spinothalamic neurons and their cortical projection target. Identification of this nucleus has required considerable effort across species. In primates, the posterior thalamic nucleus that receives the majority of spinothalamic tract inputs is the posterior part of the ventral medial nucleus, or the VMpo (located posteromedial to the VPL/VPm; previously considered part of PO; see Willis et al., 2002), as identified through both anterograde tracing from superficial spinal cord (Craig, 2004) and retrograde tracing from VMpo (Craig and Zhang, 2006) (for anatomical location of VMPo in humans, see Figure 1 from Montes et al., 2005; for anatomical location of VMPo in non-human primates, from Craig et al., 1994). Lamina I spinothalamic neurons targeting the posterior thalamic nuclei are responsive to thermal stimuli (Gauriau and Bernard, 2004b) and electrophysiology recordings from VMpo in primates have identified thermally responsive neurons (Craig et al., 1994, 1999). Anterograde tracing from VMpo has identified dorsal posterior insular cortex as the primary projection target (Craig, 2014) and posterior insular cortex has been identified as a thermally responsive cortical region in human fMRI studies (Craig et al., 2000). Therefore, the posterior thermosensory stream is defined as Lamina I spinothalamic tract neurons projecting to posterior somatosensory thalamus and then onto posterior insular cortex.
Figure 1. Visualization of horizontal (A) and coronal (B) sections from the Allen Mouse Brain Common Coordinate Framework (Wang et al., 2020). (A) Horizontal sections arranged from dorsal to ventral (left to right) depict thalamic nuclei outlined in black and key thalamic nuclei in color. (B) Coronal sections arranged from anterior to posterior (left to right) depict thalamic nuclei outlined in black and key thalamic nuclei in color.
The functional homolog of the primate VMpo has been identified in other species by the dense Lamina I spinal tract innervation, nociceptive and thermosensory response properties, and axonal projections to posterior insular cortex (Craig, 2014). In cats, the ventral aspect of VMb has been described as the VMpo equivalent because this nucleus receives input from lamina I spinothalamic neurons (Craig and Dostrovsky, 2001), is involved in thermosensory behavior (Norrsell and Craig, 1999), and projects to insular cortex (Clasca et al., 1997). In rodents, it was originally believed that the VMpo—insular cortex circuit did not exist (Craig, 2009), but this view has shifted given recent findings depicting a robust thermal representation in mouse posterior insular cortex (pIC) (Vestergaard et al., 2023). In rats, there was initially evidence for VPMpc as the candidate nucleus equivalent to cat VMb (Cliffer et al., 1991; Iwata et al., 1992; Jasmin et al., 2004), but PoT has emerged as the primary candidate (Gauriau and Bernard, 2004a). Similar to VMPo in primates, the PoT nucleus is located posteromedial to the VPL at the caudal end of the PO nucleus (Figure 1). PoT receives the highest proportion of spinothalmic input (Al-Khater et al., 2008), has thermosensitive/nociceptive neurons, and projects to a posterior cortical field we now associate as posterior insular cortex (Gauriau and Bernard, 2004b). Similar to rats, mouse PoT receives spinothalamic input (Davidson et al., 2010) and thalamocortical projections target posterior insular cortex without sending any projections to primary somatosensory cortex (Bokiniec et al., 2023). Therefore, we propose that the rodent functional homolog to VMpo is the PoT nucleus.
The human homolog to VMpo has been identified using cytoarchitecture characteristics of non-human primate VMpo (Blomqvist, 2000). Microstimulation in this region, which is defined as posterior and inferior to the core somatosensory nucleus in human electrophysiology experiments, has evoked thermosensory and nociceptive percepts in humans (Davis et al., 1999). Further, nociceptive-specific units (Davis et al., 1996; Dostrovsky, 2000) as well as cool-responsive units (Davis et al., 1999) have been recorded in this region in humans as well. Localized lesions exist in this thalamic area that is believed to be VMpo (Craig, 2014) in patients experiencing thermanesthesia and thalamic pain (Sprenger et al., 2012). However, it is important to note that this does not appear to be the sole thalamic representation as there are patients with thalamic lesions that do not include VMpo that have still led to abnormal cold sensation and pain (Montes et al., 2005; Kim et al., 2007). There have also been recordings and stimulations in human thalamus that are located much more lateral than VMpo that are cool-responsive (Lenz et al., 1993a; Lenz and Dougherty, 1998), supporting the idea that there are at least two streams for discriminative thermosensory information. Further, retrograde anatomical tracing from thermosensitive cortical regions of the mouse have identified an anterior–posterior distribution in the thalamocortical projection neurons to S1 and pIC, respectively (Figure 2). Therefore, the posterior thermosensory stream is a key pathway for thermal encoding, but not the exclusive pathway for thermosensation.
Figure 2. Functionally targeted retrograde tracers injected into forepaw primary somatosensory cortex (fS1) and forepaw posterior insular cortex (pIC) reveal thalamic populations. (A) Population injection sites and functional responses of fS1 (n = 10 mice). Left plots show 80% contours of the widefield thermal response to cool stimuli (blue) and fluorescence of the tracer (green) (n = 10 mice, 5 retrograde and 5 anterograde injections) aligned to peak temperature response in fS1. Image shows outlines of all injection sites localized on a coronal brain slice (ABA Plate: 576987753) from the Allen Brain Atlas. Left plots, Same as for right plots, but for fpIC and including response to 8 kHz sound stimulation (gray). (ABA Plate: 576990141). Scale bars: 500 μm. (B) Representative example brain slices of inputs to fS1 (green) or fpIC (magenta) from selected thalamic subregions. The full list of abbreviations is shown in Supplementary Table 1 of Bokiniec et al. (2023). Scale bars: 250 μm. (C) Left: Representative micrograph of a coronal brain section showing the VPL nucleus with CTB positive cells projecting to fS1 (i) or fpIC (ii), and one identified cell (iii - white, highlighted by arrowhead) that projects to both fS1 (green) and fpIC (magenta). Right: Percentage of dual labeled cells projecting to fS1 and fpIC in and thalamic nuclei (mean ± SEM, n = 5 mice). (D) Representation of reconstructed VPL, PO, and PoT inputs to fS1 (green) or fpIC (magenta) (n = 5 mice). Left, Plotted as a horizontal view (rostrocaudal vs. mediolateral). Right shows coronal sections highlighting (i) a rostral region with input to fS1 only, (ii) an intermediate rostrocaudal region with input to both fS1 and fpIC, and (iii) a caudal region with inputs to fpIC only. Reproduced from Bokiniec et al. (2023).
The existence of two parallel streams of thermosensory information suggests distinct roles of each thalamic nucleus in thermal encoding. In the context of temperature, the putative function of distinct representations have been considered in the frameworks of the sensory and the hedonic aspect of the stimulus (Sewards and Sewards, 2002) as well as exteroception and interoception (Craig, 2002). Here, we first consider the distinctions in the sensory representation itself and then expand on the proposed functional roles of these two parallel streams. While there are multiple stimulus dimensions that could differ, the most salient appears to be the representation of warm and cool in each pathway.
Upon discovery of discrete cold and warm spots in the skin (Blix, 1882), cool and warm encoding have been explored almost as two separate entities with the majority of studies reporting on the representation of cool stimuli. Historically, electrophysiological recordings investigating cooling sensation were targeting the ventral basal complex (VB), or the anterior stream as we describe here. Cool-sensitive neurons were identified within VB in response to cooling of the tongue (Poulos and Benjamin, 1968; Auen et al., 1980) or limb (Burton et al., 1970; Schingnitz and Werner, 1980). In human studies, cool-evoked neural responses were also identified in ventral somatosensory thalamus (Lenz and Dougherty, 1998; Lee et al., 1999). Cool-evoked thalamic responses showed either transient or sustained response dynamics (Burton et al., 1970). Some VB neurons showed high sensitivity to cool with step-like tuning functions, while others had a more graded response across increasing stimulus intensities (Sakata et al., 1989). Responses in VB to warm stimulation of the limb or tongue were incredibly rare, with some studies reporting no warming cells (Poulos and Benjamin, 1968). Responses to warming have also been uncommon relative to cooling at the spinal cord (Kenshalo et al., 1979; Ferrington et al., 1987; Dado et al., 1994). The notable exception to this lack of warm stimulus encoding in the thalamus was found in response to scrotal stimulation (Hellon and Misra, 1973; Jahns, 1975; Schingnitz and Werner, 1980). While warming the scrotum could elicit a strong evoked response in VB, this response required cortical involvement (Hellon and Taylor, 1982) and was later described as a non-specific activation (Kanosue et al., 1985). Therefore, warm-evoked sensory responses in VB remain elusive. Downstream, in somatosensory cortex, there is a similar lack of warm representation. In human EEG, cooling the glabrous skin of the hand elicited an evoked response over somatosensory cortex, while no evoked potential of any form was measured following rapid warming of the hand (Duclaux et al., 1974). This is consistent with recent imaging studies from somatosensory cortex in mice showing a robust cool response (Milenkovic et al., 2014), but a minimal warm response (Vestergaard et al., 2023).
However, the absence of warm encoding in the anterior thermosensory stream sets up an obvious concern – why is warm not represented here? At the periphery, cool-responsive neurons greatly outnumber the warm-responsive neurons (McGlone and Reilly, 2010). Therefore, the sparse encoding of warm in the anterior thermosensory stream could simply be predicted from the distribution of peripheral receptors and would suggest that warm is not well represented in whole organisms. However, warm is encoded robustly in the hypothalamus, independent of the thalamocortical circuit (Nakamura, 2011; Tan et al., 2016), demonstrating that warm can be represented in the central nervous system. While behavioral sensitivity to warm is lower than to cold (Paricio-Montesinos et al., 2020), humans are fully capable of detecting both transient warm and cool stimulation (Stevens and Choo, 1998) suggesting a discriminative representation of warm must exist in the somatosensory pathway. Alternatively, it is also possible that the cold representation seen in the anterior thermosensory stream is mediated exclusively by slowly adapting mechanoreceptors with thermal sensitivity (Burton et al., 1972) via the dorsal column nuclei (Abraira and Ginty, 2013). While this could underlie some of the thermotactile-responsive neurons in the anterior thermosensory stream, it would not account for the cool-selective units that have been recorded throughout the nucleus. Another possibility is that warm encoding in the anterior stream could be mediated via suppression rather than excitation. Warming stimulation can elicit suppressed responses in cool-responsive primary sensory afferents (Burton et al., 1970; Paricio-Montesinos et al., 2020). Warm-evoked suppression could be difficult to identify in anesthetized recordings because the spontaneous firing rate is artificially low. This must be investigated further to identify whether a robust warm-evoked suppression exists in the anterior thermosensory stream. Overall, the evidence suggests that the anterior stream is primarily involved in cool encoding with minimal impact on the discriminative encoding of warm.
The investigation of the posterior thermosensory stream is far more recent, which has resulted in even fewer investigations of thermosensation in posterior thalamus than ventral thalamus. However, there are electrophysiological recordings that have measured innocuous thermosensory responses in posterior thalamus (Craig et al., 1994) in addition to other modalities such as touch, itch, and pain (Lipshetz et al., 2018), further confirming its involvement in somatosensory processing. Spinothalamic Lamina I terminations in macaque VMPo display a coherent topographic map as assessed using anterograde (Craig, 2004) and retrograde (Craig and Zhang, 2006) tracing techniques suggesting this thalamic region has somatotopy. Additionally, anterograde tracing from the VMPo has also suggested a somatotopic map in the thalamocortical projections (Craig, 2014) to cortex. Microstimulation studies in humans found that while the cool percepts could be evoked in both the core region of somatosensory thalamus (anterior stream) and the posterior region of somatosensory thalamus (posterior stream), warm percepts were evoked more frequently in the posterior region (Ohara and Lenz, 2003). Microstimulation in insular cortex has also elicited non-painful warming percept in humans (Ostrowsky et al., 2000) and recordings in posterior insular cortex of mice have also shown a robust warm representation (Vestergaard et al., 2023). While further research is needed to better understand the neural mechanisms underlying the processing of warm stimuli and its representation in the brain, this suggests that the posterior stream is involved in both cool and warm thermal representation.
Parallel pathways for processing sensory information are the rule rather than the exception. In the visual cortical pathway, the ventral stream is believed to underlie visual discrimination while the dorsal pathway is associated with spatial vision and visually guided behaviors (Ungerleider and Mishkin, 1982). The ventral and dorsal visual streams are colloquially known as the “what” and “where” pathways for vision. Similarly, in the auditory circuits, the ventral pathway is associated with sound recognition while the dorsal pathway is associated with sound localization and speech production (Wang et al., 2008). As with vision, these pathways are also referred to as the “what” and “where” pathways for audition.
While these parallel cortical pathways are not considered entirely independent, the identification of two pathways that underlie sensory discrimination and action guidance has led to a shift in the perspective for visual and auditory processing. A similar search for guiding principles in somatosensory processing is underway. Here, we outline the major proposed roles of the distinct thermosensory pathways in somatosensation, with a particular focus on thermosensation.
While these auditory and visual pathways are cortico-cortical circuits that likely vary critically from thalamocortical pathways, it is tempting to try to draw direct parallels between the visual and auditory themes of sensing-for-discrimination and sensing-for-action in the “what” and “where” cortical pathways, respectively, to the proposed thermosensory information streams.
Posterior insular cortex, or the cortical target of the posterior thermosensory pathway, encodes a thermal representation of both warm and cool stimuli (Vestergaard et al., 2023) that is correlated with the perceived temperature rather than the valence of the thermosensation (Craig et al., 2000). This representation of temperature would lead to a more discriminable characteristic (Craig, 2011) than that seen for somatosensory cortex where a warm representation is largely absent (Vestergaard et al., 2023) and therefore could be compatible with a “what” stream of information for thermal encoding (Dijkerman and De Haan, 2007).
In contrast, the somatosensory cortex, or the cortical target of the anterior thermosensory pathway, is highly interconnected with regions involved in sensorimotor processing including motor cortex, and contains a precise somatotopic map of the body surface (Krubitzer and Kaas, 1990). A thermal stimulus is typically provided in concert with a tactile stimulus when interacting with objects or surfaces. Therefore, the more restricted thermal representation here combined with the robust activation of somatosensory cortex by the thermotactile stimulus could provide spatial localization to be primarily associated with the “where” pathway.
Similar to the visual and auditory pathways, these parallel pathways would not contain completely independent information. For example, cool information would be discriminable in the “where” pathway (anterior stream) and spatial information could be decoded from the somatotopic maps of temperature in posterior insular cortex (Vestergaard et al., 2023) in the “what” pathway (posterior stream). Yet the same hypothesis would not be drawn for tactile information. Somatosensory cortex is considered requisite for discriminative tactile information and spatial localization, making it critical for both the “what” and the “where” touch pathway (Diamond et al., 2008). However, as discussed below, it is entirely possible that touch and temperature should not necessarily be considered comparable sensations with identical neural pathways.
As described thus far, somatosensation is the compilation of multiple submodalities including touch, itch, proprioception, temperature, and pain that sense exteroceptive stimuli, or stimuli that are external to the body. However, it has been proposed that temperature and pain have been categorically misclassified as exteroceptive instead of interoceptive, or relating to stimuli that are internal to the body (Craig, 2002). In this view, interoceptive explicitly includes both visceral sensation and homeostatic sensory capacity (Ceunen et al., 2016). Therefore, the posterior thermosensory stream could be responsible for the interoceptive representation of the body state (Craig, 2003b) including thermosensory encoding.
As such, thermosensation is described as a homeostatic emotion because the valence of the thermal stimulus is dependent on the homeostatic state (Craig, 2007). Practically speaking, this would be equivalent to the pleasant feeling of a cool breeze on a warm sunny day compared to the unpleasant feeling of the same cool breeze during a frigid winter day. Therefore, the thermal representation in the posterior insular cortex, or the cortical target of the posterior thermosensory pathway, is referred to as both the primary thermosensory cortex and the limbic sensory cortex. This is because the posterior insular cortex is highly interconnected with regions involved in valence and homeostasis including the amygdala, hypothalamus, orbitofrontal cortex, and parabrachial nucleus (Yasui et al., 1991; Gehrlach et al., 2020; Bokiniec et al., 2023). In a positron emission tomography (PET) study, orbitofrontal cortex and anterior insular cortex thermal-evoked responses were correlated with subjective thermal experience (i.e., valence) while posterior insular cortex was correlated with objective thermal stimulation (i.e., applied temperature) (Craig et al., 2000). Therefore, it has been proposed that the sensory representation in posterior insular cortex is the limbic sensory substrate for subjective/homeostatic feelings and emotions (Craig, 2002).
Importantly, this view essentially excludes the role of the anterior thermosensory stream in thermal encoding and highlights posterior insular cortex as the key structure for interoception. While posterior insular cortex is tightly interconnected with limbic sensory structures and likely is involved in valence encoding (Gogolla, 2017), it is not necessarily required for homeostatic thermoregulation. As described above, the spinoparabrachial tract is crucial for thermoregulation (Yahiro et al., 2017), independent of the thalamic representation which presumably drives the cortical representation in the posterior insular. This would suggest that posterior insular cortex could constitute the primary discriminative thermal representation, but does not clearly delineate whether thermosensation should be classified as exclusively interoceptive. In comparison, tactile encoding would be considered both interoceptive and exteroceptive. Interoceptive tactile signaling could be stomach distension when feeling ‘full’ after eating while exteroceptive tactile signaling could be the deformation of the skin when grasping an object. Exteroception is inherently important for somatosensation as it would include direct interactions with the external environment. The importance of particular regions of the body, such as the hand and mouth, for exteroception can be visualized in the innervation density of the primary sensory afferents in the skin, as measured anatomically and by perceptual acuity. Both mechanoreceptors and thermally responsive fibers densely innervate skin used for actively sensing the environment, such as the hands or the lips, relative to other body segments that may provide more critical measures of homeostatic state or body temperature, such as the abdomen (Stevens and Choo, 1998; Corniani and Saal, 2020). Taken together, we would propose that thermosensation is involved in both interoception and exteroception and that it is unique from touch in that it has a clear autonomic function in thermal homeostasis.
The definition of somatosensory encoding has also been considered in the framework of discriminative sensory features and hedonic, or valence, sensory features. While this view has been discussed in the context of pain, and particularly whether pain should be treated as a separate submodality of somatosensation or as a negative valence signal associated with other modalities such as touch, it has parallels to temperature sensing (Sewards and Sewards, 2002). Similar to the natural valence of thermal encoding described above, tactile encoding has a similar separation between sensory discrimination and sensory valence. For touch, a specific class of mechanosensitive fibers has been proposed to mediate affective, or rewarding touch properties, as opposed to sensory discriminative properties (McGlone et al., 2014). Within thermal encoding, human subjects can differentiate between thermal stimuli regardless of homeostatic body temperature while unpleasantness varied with body temperatures, suggesting that valence and sensory features could be represented by the activity of two neuronal populations (Mower, 1976). It has been proposed that the hedonic and discriminative sensory representations can both exist within the ventrobasal and posterior complex of the somatosensory thalamus, but the cortical encoding in primates contains only sensory representations in somatosensory cortex and has both sensory and hedonic representations in the insular cortex (Sewards and Sewards, 2002). In this view, the anterior thermosensory stream would be primarily driven by discriminative sensory features, while the posterior thermosensory stream would be primarily driven by the valence of the thermal stimulation.
However, the anterior thermosensory stream does not contain the full thermosensory representation and therefore would not be the ideal candidate region for thermal discriminative features. Further, we would actually delineate valence into two subcategories. The first classification of valence would be attributed only to the stimulus qualities. In touch, for example, this could be defined as a positive valence associated with a gentle stroke using silk compared to the negative valence associated with a hard stroke using sandpaper. The second classification of valence would be attributed to the integration of the stimulus qualities with the internal state. In touch, this could be described as the positive emotional response generated when tickled while happy compared to the negative response generated when tickled while crying. In this consideration, the first classification of valence based on stimulus properties could exist within each pathway. However, aforementioned human studies found that activity in posterior insular cortex does not correlate with valence, but only with stimulus intensity (Craig et al., 2000), suggesting that the posterior thermosensory pathway is not necessarily representing the first classification of valence. However, consistent with the theory of interoception (Craig, 2002), the second classification of valence would likely be developed in downstream structures from the posterior thermosensory stream. The combination of the reduced sensory representation in the anterior pathway and the evidence against valence representation in either pathway suggests there is insufficient evidence to predict that thermal encoding in these two thermosensory pathways is split well into these two categories of valence and discriminative features.
Instead, we propose a synthesis of these theories to suggest that thermal pathways are actually characterized by two distinct functional roles, both of which impact thermosensation. These two functional roles are thermotactile sensing and isolated temperature sensation (Figure 3). Somatosensory cortex has been implicated as a key structure in active sensing, or haptic exploration. The anterior thermosensory pathway has a restricted thermal encoding paired with robust tactile encoding. We propose that the thermal representation in this pathway is primarily involved in thermotactile behaviors. Given that mammalian species are homeotherms, the environmental temperature is usually lower than that of the body. Therefore, the statistics of the natural thermosensory environment are heavily cold biased, which could explain the limited sensory representation in the anterior thermosensory stream.
Figure 3. Parallel processing in ventral and posterior thermosensory streams. Thermal information is encoded in two distinct thalamocortical pathways defined as the ventral (blue) and posterior (yellow) thermosensory streams. Thalamic nuclei associated with each stream for limb stimulation are indicated for, from bottom to top: humans, non-human primates, cats, and rodents.
In contrast, the posterior thermosensory pathway contains the relevant information for both warm and cool encoding to have discriminative thermosensory capabilities. In the cortical target of this pathway, touch and temperature are represented in distinct subpopulations (Vestergaard et al., 2023) which would further enable precise thermosensory encoding. All evidence suggests that the posterior thermosensory pathway is crucial for temperature discrimination, but extensive studies into the thalamic nucleus responsible for this pathway will be required to elucidate its classification within the thalamic hierarchy. This would suggest that posterior thalamus and posterior insular cortex could encode both interoceptive and exteroceptive thermosensory information while providing discriminative thermal information to both the anterior thermosensory stream and the limbic thermosensory pathway. Taken together, we believe this framework could provide a functional segregation between the pathways while remaining consistent with prior proposed models.
The relevant pathways for thermosensory processing in the central nervous system remain an area of active debate. Here we propose a synthesis of research across model organisms to describe two pathways for innocuous thermal perception: the anterior and the posterior thermosensory streams. The anterior thermosensory pathway transmits thermosensory information from the superficial laminae of the spinal cord to the ventral nuclei of the lateral thalamus and ultimately somatosensory cortex. The posterior thermosensory pathway transmits thermosensory information from the superficial laminae of the spinal cord to the posterior nuclei of the lateral thalamus and ultimately posterior insular cortex. While there is an extensive body of work on somatosensory encoding in the ventral somatosensory thalamic nuclei, there is a paucity of data in the posterior somatosensory thalamic nuclei. Anatomical tracing has shown that the thalamic neurons of the anterior and posterior streams are completely non-overlapping (Bokiniec et al., 2023), suggesting parallel information processing in the thalamocortical circuit, but comparative studies investigating thermal encoding properties across these thermal pathways will be required to support, or disclaim, the hypothetical frameworks presented here. While it has been proposed that the anterior and posterior thermosensory streams are designated for sensory-discriminative functions (Vestergaard et al., 2023), interoception (Craig, 2002), or hedonic identity (Sewards and Sewards, 2002), the one organizing principle that remains evident is that temperature is not touch. It is a distinct modality that requires further exploration and future work is required to carefully disentangle the role of these two thalamic pathways on thermal encoding.
TL and CW contributed to conception and design of the article, developing the literature review, and building a cohesive framework. CW wrote initial manuscript draft. All authors contributed to the article and approved the submitted version.
CW was supported by Human Frontier Science Program (HFSP LT000359/2018-L).
We thank Phillip Bokiniec for constructive comments on earlier versions of the manuscript.
The authors declare that the research was conducted in the absence of any commercial or financial relationships that could be construed as a potential conflict of interest.
All claims expressed in this article are solely those of the authors and do not necessarily represent those of their affiliated organizations, or those of the publisher, the editors and the reviewers. Any product that may be evaluated in this article, or claim that may be made by its manufacturer, is not guaranteed or endorsed by the publisher.
Vc, Ventral caudal; VPL, Ventral posterolateral nucleus; VP, Ventral posterior nucleus; VPI, Ventral margin of the ventral tier nuclei; VB, Ventrobasal complex; PoT, Posterior triangular nucleus; VMb, Basal part of the ventral medial nucleus; VMpo, Posterior part of the ventral medial nucleus; S1, Primary somatosensory cortex; pIC, Posterior insular cortex.
Abraira, V. E., and Ginty, D. D. (2013). The sensory neurons of touch. Neuron 79, 618–639. doi: 10.1016/j.neuron.2013.07.051
Albe-Fessar, D., Berkley, K. J., Kruger, L., Ralston, H. J., and Willis, W. D. (1985). Diencephalic mechanisms of pain sensation. Brain Res. Rev. 9, 217–296. doi: 10.1016/0165-0173(85)90013-X
Al-Khater, K. M., Kerr, R., and Todd, A. J. (2008). A quantitative study of spinothalamic neurons in laminae I, III, and IV in lumbar and cervical segments of rat spinal cord. J. Comp. Neurol. 511, 1–18. doi: 10.1002/cne.21811
Al-Khater, K. M., and Todd, A. J. (2009). Collateral projections of neurons in laminae I, III, and IV of rat spinal cord to thalamus, periaqueductal gray matter, and lateral parabrachial area. J. Comp. Neurol. 515, 629–646. doi: 10.1002/cne.22081
Andrew, D., and Craig, A. D. (2001). Spinothalamic lamina I neurons selectively sensitive to histamine: a central neural pathway for itch. Nat. Neurosci. 4, 72–77. doi: 10.1038/82924
Apkarian, A. V., and Hodge, C. J. (1989a). Primate spinothalamic pathways: I. a quantitative study of the cells of origin of the spinothalamic pathway. J. Comp. Neurol. 288, 447–473. doi: 10.1002/cne.902880307
Apkarian, A. V., and Hodge, C. J. (1989b). Primate spinothalamic pathways: II. The cells of origin of the dorsolateral and ventral spinothalamic pathways. J. Comp. Neurol. 288, 474–492. doi: 10.1002/cne.902880308
Apkarian, A. V., and Hodge, C. J. (1989c). Primate spinothalamic pathways: III. Thalamic terminations of the dorsolateral and ventral spinothalamic pathways. J. Comp. Neurol. 288, 493–511. doi: 10.1002/cne.902880309
Apkarian, A., and Shi, T. (1994). Squirrel monkey lateral thalamus. I. Somatic nociresponsive neurons and their relation to spinothalamic terminals. J. Neurosci. 14, 6779–6795. doi: 10.1523/JNEUROSCI.14-11-06779.1994
Auen, E. L., Poulos, D. A., Hirata, H., and Molt, J. T. (1980). Location and organization of thalamic thermosensitive neurons responding to cooling the cat oral-facial regions. Brain Res. 191, 260–264. doi: 10.1016/0006-8993(80)90330-3
Bernardo, K. L., and Woolsey, T. A. (1987). Axonal trajectories between mouse somatosensory thalamus and cortex. J. Comp. Neurol. 258, 542–564. doi: 10.1002/cne.902580406
Bester, H., Chapman, V., Besson, J.-M., and Bernard, J.-F. (2000). Physiological properties of the Lamina I Spinoparabrachial neurons in the rat. J. Neurophysiol. 83, 2239–2259. doi: 10.1152/jn.2000.83.4.2239
Bliss, T. V. P., Collingridge, G. L., Kaang, B.-K., and Zhuo, M. (2016). Synaptic plasticity in the anterior cingulate cortex in acute and chronic pain. Nat. Rev. Neurosci. 17, 485–496. doi: 10.1038/nrn.2016.68
Blix, M. (1882). Experimentela bidrag till losning af fragan om hudnervernas specifika energi. Upps. Lakfor Forh 18, 87–102.
Blomqvist, A. (2000). Cytoarchitectonic and immunohistochemical characterization of a specific pain and temperature relay, the posterior portion of the ventral medial nucleus, in the human thalamus. Brain 123, 601–619. doi: 10.1093/brain/123.3.601
Boivie, J. (1971). The termination of the spinothalamic tract in the cat an experimental study with silver impregnation methods. Exp. Brain Res. 112, 331–353. doi: 10.1007/BF00234489
Boivie, J. (1979). An anatomical reinvestigation of the termination of the spinothalamic tract in the monkey. J. Comp. Neurol. 186, 343–369. doi: 10.1002/cne.901860304
Bokiniec, P., Whitmire, C. J., Leva, T. M., and Poulet, J. F. A. (2023). Brain-wide connectivity map of mouse thermosensory cortices. Cereb. Cortex 33, 4870–4885. doi: 10.1093/cercor/bhac386
Burstein, R., Dado, R. J., and Giesler, G. J. (1990). The cells of origin of the spinothalamic tract of the rat: a quantitative reexamination. Brain Res. 511, 329–337. doi: 10.1016/0006-8993(90)90179-F
Burton, H., Forbes, D. J., and Benjamin, R. M. (1970). Thalamic neurons responsive to temperature changes of glabrous hand and foot skin in squirrel monkey. Brain Res. 24, 179–190. doi: 10.1016/0006-8993(70)90099-5
Burton, H., Terashima, S.-I., and Clark, J. (1972). Response properties of slowly adapting mechanoreceptors to temperature stimulation in cats. Brain Res. 45, 401–416. doi: 10.1016/0006-8993(72)90471-4
Bushnell, M. C., Duncan, G. H., and Tremblay, N. (1993). Thalamic VPM nucleus in the behaving monkey. I. Multimodal and discriminative properties of thermosensitive neurons. J. Neurophysiol. 69, 739–752. doi: 10.1152/jn.1993.69.3.739
Carstens, E., and Trevino, D. L. (1978). Laminar origins of spinothalamic projections in the cat as determined by the retrograde transport of horseradish peroxidase. J. Comp. Neurol. 182, 151–165. doi: 10.1002/cne.901820110
Casey, K. L., and Morrow, T. J. (1983). Ventral posterior thalamic neurons differentially responsive to noxious stimulation of the awake monkey. Science 221, 675–677. doi: 10.1126/science.6867738
Cervero, F., and Connell, L. A. (1984). Distribution of somatic and visceral primary afferent fibres within the thoracic spinal cord of the cat. J. Comp. Neurol. 230, 88–98. doi: 10.1002/cne.902300108
Cervero, F., Connell, L. A., and Lawson, S. N. (1984). Somatic and visceral primary afferents in the lower thoracic dorsal root ganglia of the cat. J. Comp. Neurol. 228, 422–431. doi: 10.1002/cne.902280309
Ceunen, E., Vlaeyen, J. W. S., and Van Diest, I. (2016). On the origin of interoception. Front. Psychol. 7:743. doi: 10.3389/fpsyg.2016.00743
Chisholm, K. I., Lo Re, L., Polgár, E., Gutierrez-Mecinas, M., Todd, A. J., and McMahon, S. B. (2021). Encoding of cutaneous stimuli by lamina I projection neurons. Pain 162, 2405–2417. doi: 10.1097/j.pain.0000000000002226
Chung, J. M., Kenshalo, D. R., Gerhart, K. D., and Willis, W. D. (1979). Excitation of primate spinothalamic neurons by cutaneous C-fiber volleys. J. Neurophysiol. 42, 1354–1369. doi: 10.1152/jn.1979.42.5.1354
Chung, J. M., Lee, K. H., Surmeier, D. J., Sorkin, L. S., Kim, J., and Willis, W. D. (1986). Response characteristics of neurons in the ventral posterior lateral nucleus of the monkey thalamus. J. Neurophysiol. 56, 370–390. doi: 10.1152/jn.1986.56.2.370
Clasca, F., Llamas, A., and Reinoso-Suarez, F. (1997). Insular cortex and neighboring fields in the cat: a redefinition based on cortical microarchitecture and connections with the thalamus. J. Comp. Neurol. 384, 456–482. doi: 10.1002/(SICI)1096-9861(19970804)384:3<456::AID-CNE10>3.0.CO;2-H
Cliffer, K., Burstein, R., and Giesler, G. (1991). Distributions of spinothalamic, spinohypothalamic, and spinotelencephalic fibers revealed by anterograde transport of PHA-L in rats. J. Neurosci. 11, 852–868. doi: 10.1523/JNEUROSCI.11-03-00852.1991
Corniani, G., and Saal, H. P. (2020). Tactile innervation densities across the whole body. J. Neurophysiol. 124, 1229–1240. doi: 10.1152/jn.00313.2020
Craig, A. D. (2002). How do you feel? Interoception: the sense of the physiological condition of the body. Nat. Rev. Neurosci. 3, 655–666. doi: 10.1038/nrn894
Craig, A. D. (2003a). Distribution of trigeminothalamic and spinothalamic lamina I terminations in the cat. Somatosens. Mot. Res. 20, 209–222. doi: 10.1080/08990220310001623013
Craig, A. D. (2003b). Interoception: the sense of the physiological condition of the body. Curr. Opin. Neurobiol. 13, 500–505. doi: 10.1016/S0959-4388(03)00090-4
Craig, A. D. (2007). “Interoception and emotion: a neuroanatomical perspective” in Handbook of emotion
Craig, A. D. (2009). A rat is not a monkey is not a human: comment on Mogil (nature rev. Neurosci. 10, 283–294 (2009)). Nat. Rev. Neurosci. 10:466. doi: 10.1038/nrn2606-c1
Craig, A. D. (2011). Interoceptive cortex in the posterior insula: comment on Garcia-Larrea et al. 2010 brain 133, 2528. Brain 134:e166. doi: 10.1093/brain/awq308
Craig, A. D., and Blomqvist, A. (2002). Is there a specific lamina I spinothalamocortical pathway for pain and temperature sensations in primates? J. Pain 3, 95–101. doi: 10.1054/jpai.2002.122953
Craig, A. D. (2004). Distribution of trigeminothalamic and spinothalamic lamina I terminations in the macaque monkey. J. Comp. Neurol. 477, 119–148. doi: 10.1002/cne.20240
Craig, A. D. (2014). Topographically organized projection to posterior insular cortex from the posterior portion of the ventral medial nucleus in the long-tailed macaque monkey: VMpo input to posterior insula. J. Comp. Neurol. 522, 36–63. doi: 10.1002/cne.23425
Craig, A. D., and Zhang, E.-T. (2006). Retrograde analyses of spinothalamic projections in the macaque monkey: input to posterolateral thalamus. J. Comp. Neurol. 499, 953–964. doi: 10.1002/cne.21155
Craig, A. D., and Burton, H. (1981). Spinal and medullary lamina I projection to nucleus submedius in medial thalamus: a possible pain center. J. Neurophysiol. 45, 443–466. doi: 10.1152/jn.1981.45.3.443
Craig, A. D., Bushnell, M. C., Zhang, E.-T., and Blomqvist, A. (1994). A thalamic nucleus specific for pain and temperature sensation. Nature 372, 770–773. doi: 10.1038/372770a0
Craig, A. D., Chen, K., Bandy, D., and Reiman, E. M. (2000). Thermosensory activation of insular cortex. Nat. Neurosci. 3, 184–190. doi: 10.1038/72131
Craig, A. D., and Dostrovsky, J. O. (1991). Thermoreceptive lamina I trigeminothalamic neurons project to the nucleus submedius in the cat. Exp. Brain Res. 85, 470–474. doi: 10.1007/BF00229424
Craig, A. D., and Dostrovsky, J. O. (2001). Differential projections of Thermoreceptive and nociceptive Lamina I Trigeminothalamic and spinothalamic neurons in the cat. J. Neurophysiol. 86, 856–870. doi: 10.1152/jn.2001.86.2.856
Craig, A. D., and Hunsley, S. J. (1991). Morphine enhances the activity of thermoreceptive cold-specific lamina I spinothalamic neurons in the cat. Brain Res. 558, 93–97. doi: 10.1016/0006-8993(91)90719-C
Craig, A. D., and Kniffki, K. D. (1985). Spinothalamic lumbosacral lamina I cells responsive to skin and muscle stimulation in the cat. J. Physiol. 365, 197–221. doi: 10.1113/jphysiol.1985.sp015767
Craig, A. D., Krout, K., and Andrew, D. (2001). Quantitative response characteristics of Thermoreceptive and nociceptive Lamina I spinothalamic neurons in the cat. J. Neurophysiol. 86, 1459–1480. doi: 10.1152/jn.2001.86.3.1459
Craig, A. D., Linington, A. J., and Kniffki, K.-D. (1989). Cells of origin of spinothalamic tract projections to the medial and lateral thalamus in the cat. J. Comp. Neurol. 289, 568–585. doi: 10.1002/cne.902890404
Craig, A. D., Zhang, E.-T., and Blomqvist, A. (1999). A distinct thermoreceptive subregion of lamina I in nucleus caudalis of the owl monkey. J. Comp. Neurol. 404, 221–234. doi: 10.1002/(SICI)1096-9861(19990208)404:2<221::AID-CNE7>3.0.CO;2-N
Dado, R. J., Katter, J. T., and Giesler, G. J. (1994). Spinothalamic and spinohypothalamic tract neurons in the cervical enlargement of rats. II. Responses to innocuous and noxious mechanical and thermal stimuli. J. Neurophysiol. 71, 981–1002. doi: 10.1152/jn.1994.71.3.981
Davidson, S., Truong, H., and Giesler, G. J. (2010). Quantitative analysis of spinothalamic tract neurons in adult and developing mouse. J. Comp. Neurol. 518, 3193–3204. doi: 10.1002/cne.22392
Davidson, S., Zhang, X., Khasabov, S. G., Simone, D. A., and Giesler, G. J. (2008). Termination zones of functionally characterized spinothalamic tract neurons within the primate posterior thalamus. J. Neurophysiol. 100, 2026–2037. doi: 10.1152/jn.90810.2008
Davis, K. D., Kiss, Z. H., Tasker, R. R., and Dostrovsky, J. O. (1996). Thalamic stimulation-evoked sensations in chronic pain patients and in nonpain (movement disorder) patients. J. Neurophysiol. 75, 1026–1037. doi: 10.1152/jn.1996.75.3.1026
Davis, K. D., Lozano, A. M., Manduch, M., Tasker, R. R., Kiss, Z. H. T., and Dostrovsky, J. O. (1999). Thalamic relay site for cold perception in humans. J. Neurophysiol. 81, 1970–1973. doi: 10.1152/jn.1999.81.4.1970
Diamond, M. E., von Heimendahl, M., Knutsen, P. M., Kleinfeld, D., and Ahissar, E. (2008). “Where” and “what” in the whisker sensorimotor system. Nat. Rev. Neurosci. 9, 601–612. doi: 10.1038/nrn2411
Dijkerman, H. C., and De Haan, E. H. F. (2007). Somatosensory processes subserving perception and action. Behav. Brain Sci. 30, 189–201. doi: 10.1017/S0140525X07001392
Dostrovsky, J. O. (2000). Role of thalamus in pain. Prog. Brain Res 129, 245–257. doi: 10.1016/S0079-6123(00)29018-3
Dostrovsky, J. O., and Guilbaud, G. (1988). Noxious stimuli excite neurons in nucleus submedius of the normal and arthritic rat. Brain Res. 460, 269–280. doi: 10.1016/0006-8993(88)90372-1
Duclaux, R., Franzen, O., Chatt, A. B., Kenshalo, D. R., and Stowell, H. (1974). Responses recorded from human scalp evoked by cutaneous thermal stimulation. Brain Res. 78, 279–290. doi: 10.1016/0006-8993(74)90552-6
Emmers, R. (1965). Organization of the first and the second somesthetic regions (SI and SII) in the rat thalamus. J. Comp. Neurol. 124, 215–227. doi: 10.1002/cne.901240207
Ferrington, D. G., Sorkin, L. S., and Willis, W. D. (1987). Responses of spinothalamic tract cells in the superficial dorsal horn of the primate lumbar spinal cord. J. Physiol. 388, 681–703. doi: 10.1113/jphysiol.1987.sp016638
Francis, J. T., Xu, S., and Chapin, J. K. (2008). Proprioceptive and cutaneous representations in the rat ventral posterolateral thalamus. J. Neurophysiol. 99, 2291–2304. doi: 10.1152/jn.01206.2007
Gauriau, C., and Bernard, J.-F. (2004a). A comparative reappraisal of projections from the superficial laminae of the dorsal horn in the rat: the forebrain. J. Comp. Neurol. 468, 24–56. doi: 10.1002/cne.10873
Gauriau, C., and Bernard, J.-F. (2004b). Posterior triangular thalamic neurons convey nociceptive messages to the secondary somatosensory and insular cortices in the rat. J. Neurosci. 24, 752–761. doi: 10.1523/JNEUROSCI.3272-03.2004
Geerling, J. C., Kim, M., Mahoney, C. E., Abbott, S. B. G., Agostinelli, L. J., Garfield, A. S., et al. (2016). Genetic identity of thermosensory relay neurons in the lateral parabrachial nucleus. Am. J. Physiol. Regul. Integr. Comp. Physiol. 310, R41–R54. doi: 10.1152/ajpregu.00094.2015
Gehrlach, D. A., Weiand, C., Gaitanos, T. N., Cho, E., Klein, A. S., Hennrich, A. A., et al. (2020). A whole-brain connectivity map of mouse insular cortex. elife 9:e55585. doi: 10.7554/eLife.55585
Giesler, G. J., Menétrey, D., and Basbaum, A. I. (1979). Differential origins of spinothalamic tract projections to medial and lateral thalamus in THE rat: NEURONS OF THE RAT SPINOTHALAMIC TRACT. J. Comp. Neurol. 184, 107–125. doi: 10.1002/cne.901840107
Gingold, S. I., Greenspan, J. D., and Apkarian, A. V. (1991). Anatomic evidence of nociceptive inputs to primary somatosensory cortex: relationship between spinothalamic terminals and thalamocortical cells in squirrel monkeys. J. Comp. Neurol. 308, 467–490. doi: 10.1002/cne.903080312
Granum, S. L. (1986). The spinothalamic system of the rat. I. Locations of cells of origin. J. Comp. Neurol. 247, 159–180. doi: 10.1002/cne.902470204
Groh, A., Mease, R., and Krieger, P. (2017). Pain processing in the thalamocortical system. e-Neuroforum 23, 117–122. doi: 10.1515/nf-2017-A019
Guest, S., Grabenhorst, F., Essick, G., Chen, Y., Young, M., McGlone, F., et al. (2007). Human cortical representation of oral temperature. Physiol. Behav. 92, 975–984. doi: 10.1016/j.physbeh.2007.07.004
Hayes, N. L., and Rustioni, A. (1980). Spinothalamic and spinomedullary neurons in macaques: a single and double retrograde tracer study. Neuroscience 5, 861–874. doi: 10.1016/0306-4522(80)90155-4
Hellon, R. F., and Misra, N. K. (1973). Neurones in the dorsal horn of the rat responding to scrotal skin temperature changes. J. Physiol. 232, 375–388. doi: 10.1113/jphysiol.1973.sp010275
Hellon, R. F., and Taylor, D. C. M. (1982). An analysis of a thermal afferent pathway in the rat. J. Physiol. 326, 319–328. doi: 10.1113/jphysiol.1982.sp014195
Hong, J. H., Kwon, H. G., and Jang, S. H. (2011). Probabilistic Somatotopy of the spinothalamic pathway at the Ventroposterolateral nucleus of the thalamus in the human brain. Am. J. Neuroradiol. 32, 1358–1362. doi: 10.3174/ajnr.A2497
Hylden, J. L. K., Anton, F., and Nahin, R. L. (1989). Spinal lamina I projection neurons in the rat: collateral innervation of parabrachial area and thalamus. Neuroscience 28, 27–37. doi: 10.1016/0306-4522(89)90229-7
Iwata, K., Kenshalo, D. R., Dubner, R., and Nahin, R. L. (1992). Diencephalic projections from the superficial and deep laminae of the medullary dorsal horn in the rat. J. Comp. Neurol. 321, 404–420. doi: 10.1002/cne.903210308
Jahns, R. (1975). Types of neuronal responses in the rat thalamus to peripheral temperature changes. Exp. Brain Res. 23, 157–166. doi: 10.1007/BF00235458
Jasmin, L., Granato, A., and Ohara, P. T. (2004). Rostral agranular insular cortex and pain areas of the central nervous system: a tract-tracing study in the rat. J. Comp. Neurol. 468, 425–440. doi: 10.1002/cne.10978
Jones, M. W., Apkarian, A. V., Stevens, R. T., and Hodge, C. J. (1987). The spinothalamic tract: an examination of the cells of origin of the dorsolateral and ventral spinothalamic pathways in cats. J. Comp. Neurol. 260, 349–361. doi: 10.1002/cne.902600303
Kanosue, K., Nakayama, T., Ishikawa, Y., Hosono, T., Kaminaga, T., and Shosaku, A. (1985). Responses of thalamic and hypothalamic neurons to scrotal warming in rats: non-specific responses? Brain Res. 328, 207–213. doi: 10.1016/0006-8993(85)91031-5
Katter, J. T., Burstein, R., and Giesler, G. J. (1991). The cells of origin of the spinohypothalamic tract in cats. J. Comp. Neurol. 303, 101–112. doi: 10.1002/cne.903030109
Kenshalo, D. R., Giesler, G. J., Leonard, R. B., and Willis, W. D. (1980). Responses of neurons in primate ventral posterior lateral nucleus to noxious stimuli. J. Neurophysiol. 43, 1594–1614. doi: 10.1152/jn.1980.43.6.1594
Kenshalo, D. R., Leonard, R. B., Chung, J. M., and Willis, W. D. (1979). Responses of primate spinothalamic neurons to graded and to repeated noxious heat stimuli. J. Neurophysiol. 42, 1370–1389. doi: 10.1152/jn.1979.42.5.1370
Kim, J. H., Greenspan, J. D., Coghill, R. C., Ohara, S., and Lenz, F. A. (2007). Lesions limited to the human thalamic principal somatosensory nucleus (ventral caudal) are associated with loss of cold sensations and central pain. J. Neurosci. 27, 4995–5004. doi: 10.1523/JNEUROSCI.0716-07.2007
Klop, E. M., Mouton, L. J., and Holstege, G. (2005). Segmental and laminar organization of the spinothalamic neurons in cat: evidence for at least five separate clusters. J. Comp. Neurol. 493, 580–595. doi: 10.1002/cne.20777
Kobayashi, A., and Osaka, T. (2003). Involvement of the parabrachial nucleus in thermogenesis induced by environmental cooling in the rat. Pflüg. Arch. Eur. J. Physiol. 446, 760–765. doi: 10.1007/s00424-003-1119-7
Krubitzer, L., and Kaas, J. (1990). The organization and connections of somatosensory cortex in marmosets. J. Neurosci. 10, 952–974. doi: 10.1523/JNEUROSCI.10-03-00952.1990
Krubitzer, L. A., and Kaas, J. H. (1992). The somatosensory thalamus of monkeys: cortical connections and a redefinition of nuclei in marmosets. J. Comp. Neurol. 319, 123–140. doi: 10.1002/cne.903190111
Kumazawa, T., and Perl, E. R. (1978). Excitation of marginal and substantia gelatinosa neurons in the primate spinal cord: indications of their place in dorsal horn functional organization. J. Comp. Neurol. 177, 417–434. doi: 10.1002/cne.901770305
Kuner, R., and Kuner, T. (2021). Cellular circuits in the brain and their modulation in acute and chronic pain. Physiol. Rev. 101, 213–258. doi: 10.1152/physrev.00040.2019
Lee, J.-I., Dougherty, P. M., Antezana, D., and Lenz, F. A. (1999). Responses of neurons in the region of human thalamic principal somatic sensory nucleus to mechanical and thermal stimuli graded into the painful range. J. Comp. Neurol. 410, 541–555. doi: 10.1002/(SICI)1096-9861(19990809)410:4<541::AID-CNE3>3.0.CO;2-8
Lenz, F. A., and Dougherty, P. M. (1998). Neurons in the human thalamic somatosensory nucleus (Ventralis Caudalis) respond to innocuous cool and mechanical stimuli. J. Neurophysiol. 79, 2227–2230. doi: 10.1152/jn.1998.79.4.2227
Lenz, F. A., Gracely, R. H., Rowland, L. H., and Dougherty, P. M. (1994). A population of cells in the human thalamic principal sensory nucleus respond to painful mechanical stimuli. Neurosci. Lett. 180, 46–50. doi: 10.1016/0304-3940(94)90910-5
Lenz, F. A., Seike, M., Lin, Y. C., Baker, F. H., Rowland, L. H., Gracely, R. H., et al. (1993a). Neurons in the area of human thalamic nucleus ventralis caudalis respond to painful heat stimuli. Brain Res. 623, 235–240. doi: 10.1016/0006-8993(93)91433-S
Lenz, F. A., Seike, M., Richardson, R. T., Lin, Y. C., Baker, F. H., Khoja, I., et al. (1993b). Thermal and pain sensations evoked by microstimulation in the area of human ventrocaudal nucleus. J. Neurophysiol. 70, 200–212. doi: 10.1152/jn.1993.70.1.200
Li, J., Xiong, K., Pang, Y., Dong, Y., Kaneko, T., and Mizuno, N. (2006). Medullary dorsal horn neurons providing axons to both the parabrachial nucleus and thalamus. J. Comp. Neurol. 498, 539–551. doi: 10.1002/cne.21068
Light, A. R., and Perl, E. R. (1979a). Spinal termination of functionally identified primary afferent neurons with slowly conducting myelinated fibers. J. Comp. Neurol. 186, 133–150. doi: 10.1002/cne.901860203
Light, A. R., and Perl, E. R. (1979b). Reexamination of the dorsal root projection to the spinal dorsal horn including observations on the differential termination of coarse and fine fibers. J. Comp. Neurol. 186, 117–131. doi: 10.1002/cne.901860202
Light, A. R., Sedivec, M. J., Casale, E. J., and Jones, S. L. (1993). Physiological and morphological characteristics of spinal neurons projecting to the parabrachial region of the cat. Somatosens. Mot. Res. 10, 309–325. doi: 10.3109/08990229309028840
Light, A. R., Trevino, D. L., and Perl, E. R. (1979). Morphological features of functionally defined neurons in the marginal zone and substantia gelatinosa of the spinal dorsal horn. J. Comp. Neurol. 186, 151–171. doi: 10.1002/cne.901860204
Lipshetz, B., Khasabov, S. G., Truong, H., Netoff, T. I., Simone, D. A., and Giesler, G. J. (2018). Responses of thalamic neurons to itch- and pain-producing stimuli in rats. J. Neurophysiol. 120, 1119–1134. doi: 10.1152/jn.00264.2018
Lund, R. D., and Webster, K. E. (1967). Thalamic afferents from the spinal cord and trigeminal nuclei. An experimental anatomical study in the rat. J. Comp. Neurol. 130, 313–327. doi: 10.1002/cne.901300404
Ma, W., Peschanski, M., and Besson, J.-M. (1986). The overlap of spinothalamic and dorsal column nuclei projections in the ventrobasal complex of the rat thalamus: a double anterograde labeling study using light microscopy analysis. J. Comp. Neurol. 245, 531–540. doi: 10.1002/cne.902450408
Mantyh, P. W. (1983). The terminations of the spinothalamic tract in the cat. Neurosci. Lett. 38, 119–124. doi: 10.1016/0304-3940(83)90027-7
Maxwell, D., and Rethlyi, M. (1987). Ultrastructure and synapticconnections of cutaneous afferent fibres in the spinal cord. TINS 10, 117–123. doi: 10.1016/0166-2236(87)90056-7
McGlone, F., and Reilly, D. (2010). The cutaneous sensory system. Neurosci. Biobehav. Rev. 34, 148–159. doi: 10.1016/j.neubiorev.2009.08.004
McGlone, F., Wessberg, J., and Olausson, H. (2014). Discriminative and affective touch: sensing and feeling. Neuron 82, 737–755. doi: 10.1016/j.neuron.2014.05.001
Milenkovic, N., Zhao, W.-J., Walcher, J., Albert, T., Siemens, J., Lewin, G. R., et al. (2014). A somatosensory circuit for cooling perception in mice. Nat. Neurosci. 17, 1560–1566. doi: 10.1038/nn.3828
Montes, C., Magnin, M., Maarrawi, J., Frot, M., Convers, P., Mauguière, F., et al. (2005). Thalamic thermo-algesic transmission: ventral posterior (VP) complex versus VMpo in the light of a thalamic infarct with central pain. Pain 113, 223–232. doi: 10.1016/j.pain.2004.09.044
Mower, G. D. (1976). Perceived intensity of peripheral thermal stimuli is independent of internal body temperature. J. Comp. Physiol. Psychol. 90, 1152–1155. doi: 10.1037/h0077284
Nakamura, K. (2011). Central circuitries for body temperature regulation and fever. Am. J. Physiol. Regul. Integr. Comp. Physiol. 301, R1207–R1228. doi: 10.1152/ajpregu.00109.2011
Nakamura, K., and Morrison, S. F. (2008). A thermosensory pathway that controls body temperature. Nat. Neurosci. 11, 62–71. doi: 10.1038/nn2027
Nakamura, K., and Morrison, S. F. (2010). A thermosensory pathway mediating heat-defense responses. Proc. Natl. Acad. Sci. 107, 8848–8853. doi: 10.1073/pnas.0913358107
Norrsell, U., and Craig, A. D. (1999). Behavioral Thermosensitivity after lesions of thalamic target areas of a Thermosensory spinothalamic pathway in the cat. J. Neurophysiol. 82, 611–625. doi: 10.1152/jn.1999.82.2.611
Ohara, S., and Lenz, F. A. (2003). Medial lateral extent of thermal and pain sensations evoked by microstimulation in somatic sensory nuclei of human thalamus. J. Neurophysiol. 90, 2367–2377. doi: 10.1152/jn.00450.2003
Ostrowsky, K., Isnard, J., Ryvlin, P., Guenot, M., Fischer, C., and Mauguiere, F. (2000). Functional mapping of the insular cortex: clinical implication in temporal lobe epilepsy. Epilepsia 41, 681–686. doi: 10.1111/j.1528-1157.2000.tb00228.x
Paricio-Montesinos, R., Schwaller, F., Udhayachandran, A., Rau, F., Walcher, J., Evangelista, R., et al. (2020). The sensory coding of warm perception. Neuron 106, 830–841.e3. doi: 10.1016/j.neuron.2020.02.035
Pearson, J. C., and Haines, D. E. (1980). Somatosensory thalamus of a prosimian primate (Galago senegalensis). I. Configuration of nuclei and termination of spinothalamic fibers. J. Comp. Neurol. 190, 533–558. doi: 10.1002/cne.901900309
Poulos, D. A., and Benjamin, R. M. (1968). Response of thalamic neurons to thermal stimulation of the tongue. J. Neurophysiol. 31, 28–43. doi: 10.1152/jn.1968.31.1.28
Sakata, Y., Morimoto, A., and Murakami, N. (1989). Responses of thalamic neurons in rats to skin cooling and hypothalamic temperature. Am. J. Physiol. Regul. Integr. Comp. Physiol. 256, R1293–R1298. doi: 10.1152/ajpregu.1989.256.6.R1293
Schingnitz, G., and Werner, J. (1980). Responses of thalamic neurons to thermal stimulation of the limbs, scrotum and tongue in the rat. J. Therm. Biol. 5, 53–61. doi: 10.1016/0306-4565(80)90040-6
Sewards, T. V., and Sewards, M. (2002). Separate, parallel sensory and hedonic pathways in the mammalian somatosensory system. Brain Res. Bull. 58, 243–260. doi: 10.1016/S0361-9230(02)00783-9
Shi, T., and Apkarian, A. V. (1995). Morphology of thalamocortical neurons projecting to the primary somatosensory cortex and their relationship to spinothalamic terminals in the squirrel monkey. J. Comp. Neurol. 361, 1–24. doi: 10.1002/cne.903610102
Sprenger, T., Seifert, C. L., Valet, M., Andreou, A. P., Foerschler, A., Zimmer, C., et al. (2012). Assessing the risk of central post-stroke pain of thalamic origin by lesion mapping. Brain 135, 2536–2545. doi: 10.1093/brain/aws153
Stevens, J. C., and Choo, C. (1998). Temperature sensitivity of the body surface over the life span. Somatosens. Mot. Res. 15, 13–28. doi: 10.1080/08990229870925
Stevens, R. T., Hodge, C. J., and Apkarian, A. V. (1989). Medial, Intralaminar, and lateral terminations of lumbar spinothalamic tract neurons: a fluorescent double-label study. Somatosens. Mot. Res. 6, 285–308. doi: 10.3109/08990228909144678
Tan, C. L., Cooke, E. K., Leib, D. E., Lin, Y.-C., Daly, G. E., Zimmerman, C. A., et al. (2016). Warm-sensitive neurons that control body temperature. Cells 167, 47–59.e15. doi: 10.1016/j.cell.2016.08.028
Tan, C. L., and Knight, Z. A. (2018). Regulation of body temperature by the nervous system. Neuron 98, 31–48. doi: 10.1016/j.neuron.2018.02.022
Turecek, J., Lehnert, B. P., and Ginty, D. D. (2022). The encoding of touch by somatotopically aligned dorsal column subdivisions. Nature 612, 310–315. doi: 10.1038/s41586-022-05470-x
Ungerleider, L., and Mishkin, M. (1982). “Two cortical visual systems” in Analysis of visual behavior (MIT Press), 549–586.
Vertes, R. P., Linley, S. B., and Hoover, W. B. (2015). Limbic circuitry of the midline thalamus. Neurosci. Biobehav. Rev. 54, 89–107. doi: 10.1016/j.neubiorev.2015.01.014
Vestergaard, M., Carta, M., Güney, G., and Poulet, J. F. A. (2023). The cellular coding of temperature in the mammalian cortex. Nature 614, 725–731. doi: 10.1038/s41586-023-05705-5
Wang, Q., Ding, S.-L., Li, Y., Royall, J., Feng, D., Lesnar, P., et al. (2020). The Allen mouse brain common coordinate framework: a 3D reference atlas. Cells 181, 936–953.e20. doi: 10.1016/j.cell.2020.04.007
Wang, W.-J., Wu, X.-H., and Li, L. (2008). The dual-pathway model of auditory signal processing. Neurosci. Bull. 24, 173–182. doi: 10.1007/s12264-008-1226-8
Willis, W. D., Kenshalo, D. R., and Leonard, R. B. (1979). The cells of origin of the primate spinothalamic tract. J. Comp. Neurol. 188, 543–573. doi: 10.1002/cne.901880404
Willis, W. D., Zhang, X., Honda, C. N., and Giesler, G. J. (2001). Projections from the marginal zone and deep dorsal horn to the ventrobasal nuclei of the primate thalamus. Pain 92, 267–276. doi: 10.1016/S0304-3959(01)00268-8
Willis, W. D., Zhang, X., Honda, C. N., and Giesler, G. J. (2002). A critical review of the role of the proposed VMpo nucleus in pain. J. Pain 3, 79–94. doi: 10.1054/jpai.2002.122949
Wolff, M., Morceau, S., Folkard, R., Martin-Cortecero, J., and Groh, A. (2021). A thalamic bridge from sensory perception to cognition. Neurosci. Biobehav. Rev. 120, 222–235. doi: 10.1016/j.neubiorev.2020.11.013
Xue, M., Shi, W.-T., Zhou, S.-B., Li, Y.-N., Wu, F.-Y., Chen, Q.-Y., et al. (2022). Mapping thalamic-anterior cingulate monosynaptic inputs in adult mice. Mol. Pain 18:174480692210870. doi: 10.1177/17448069221087034
Yahiro, T., Kataoka, N., and Nakamura, K. (2023). Two ascending Thermosensory pathways from the lateral parabrachial nucleus that mediate behavioral and autonomous thermoregulation. J. Neurosci. 43, 5221–5240. doi: 10.1523/JNEUROSCI.643-23.2023
Yahiro, T., Kataoka, N., Nakamura, Y., and Nakamura, K. (2017). The lateral parabrachial nucleus, but not the thalamus, mediates thermosensory pathways for behavioural thermoregulation. Sci. Rep. 7:5031. doi: 10.1038/s41598-017-05327-8
Yasui, Y., Breder, C. D., Safer, C. B., and Cechetto, D. F. (1991). Autonomic responses and efferent pathways from the insular cortex in the rat. J. Comp. Neurol. 303, 355–374. doi: 10.1002/cne.903030303
Yen, C.-T., and Lu, P.-L. (2013). Thalamus and pain. Acta Anaesthesiol. Taiwanica 51, 73–80. doi: 10.1016/j.aat.2013.06.011
Yoshida, A., Dostrovsky, J. O., Sessle, B. J., and Chiang, C. Y. (1991). Trigeminal projections to the nucleus submedius of the thalamus in the rat. J. Comp. Neurol. 307, 609–625. doi: 10.1002/cne.903070408
Zhang, X., Davidson, S., and Giesler, G. J. (2006). Thermally identified subgroups of marginal zone neurons project to distinct regions of the ventral posterior lateral nucleus in rats. J. Neurosci. 26, 5215–5223. doi: 10.1523/JNEUROSCI.0701-06.2006
Zhang, X., and Giesler, G. J. (2005). Response Characterstics of spinothalamic tract neurons that project to the posterior thalamus in rats. J. Neurophysiol. 93, 2552–2564. doi: 10.1152/jn.01237.2004
Keywords: thermosensation, thalamus, thalamocortical, spinothalamic, somatosensory
Citation: Leva TM and Whitmire CJ (2023) Thermosensory thalamus: parallel processing across model organisms. Front. Neurosci. 17:1210949. doi: 10.3389/fnins.2023.1210949
Received: 23 April 2023; Accepted: 15 September 2023;
Published: 13 October 2023.
Edited by:
Lucy Maree Palmer, University of Melbourne, AustraliaReviewed by:
Dieter Jaeger, Emory University, United StatesCopyright © 2023 Leva and Whitmire. This is an open-access article distributed under the terms of the Creative Commons Attribution License (CC BY). The use, distribution or reproduction in other forums is permitted, provided the original author(s) and the copyright owner(s) are credited and that the original publication in this journal is cited, in accordance with accepted academic practice. No use, distribution or reproduction is permitted which does not comply with these terms.
*Correspondence: Clarissa J. Whitmire, Yy53aGl0bWlyZUB1cS5lZHUuYXU=
Disclaimer: All claims expressed in this article are solely those of the authors and do not necessarily represent those of their affiliated organizations, or those of the publisher, the editors and the reviewers. Any product that may be evaluated in this article or claim that may be made by its manufacturer is not guaranteed or endorsed by the publisher.
Research integrity at Frontiers
Learn more about the work of our research integrity team to safeguard the quality of each article we publish.