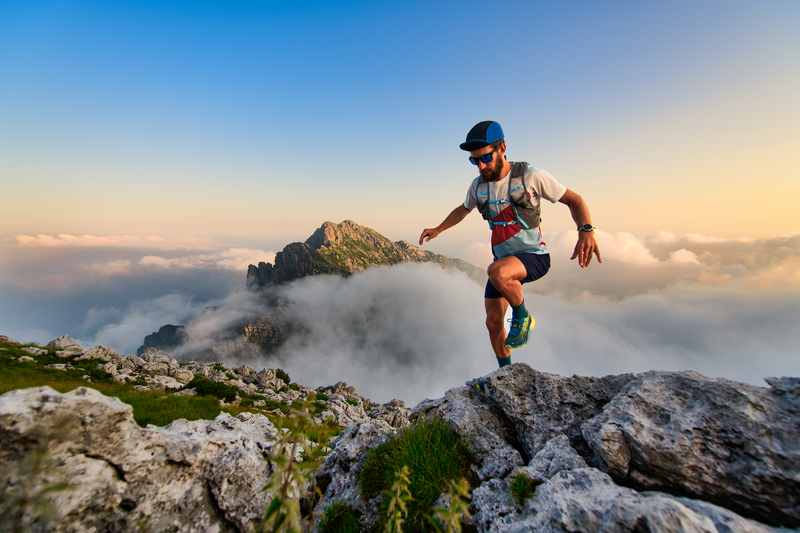
95% of researchers rate our articles as excellent or good
Learn more about the work of our research integrity team to safeguard the quality of each article we publish.
Find out more
REVIEW article
Front. Neurosci. , 26 May 2023
Sec. Neurodegeneration
Volume 17 - 2023 | https://doi.org/10.3389/fnins.2023.1188065
This article is part of the Research Topic Insights in Neurodegeneration: 2022 View all 9 articles
Alzheimer’s Disease (AD) and related dementias are a leading cause of death globally and are predicted to increase in prevalence. Despite this expected increase in the prevalence of AD, we have yet to elucidate the causality of the neurodegeneration observed in AD and we lack effective therapeutics to combat the progressive neuronal loss. Throughout the past 30 years, several non-mutually exclusive hypotheses have arisen to explain the causative pathologies in AD: amyloid cascade, hyper-phosphorylated tau accumulation, cholinergic loss, chronic neuroinflammation, oxidative stress, and mitochondrial and cerebrovascular dysfunction. Published studies in this field have also focused on changes in neuronal extracellular matrix (ECM), which is critical to synaptic formation, function, and stability. Two of the greatest non-modifiable risk factors for development of AD (aside from autosomal dominant familial AD gene mutations) are aging and APOE status, and two of the greatest modifiable risk factors for AD and related dementias are untreated major depressive disorder (MDD) and obesity. Indeed, the risk of developing AD doubles for every 5 years after ≥ 65, and the APOE4 allele increases AD risk with the greatest risk in homozygous APOE4 carriers. In this review, we will describe mechanisms by which excess ECM accumulation may contribute to AD pathology and discuss pathological ECM alterations that occur in AD as well as conditions that increase the AD risk. We will discuss the relationship of AD risk factors to chronic central nervous system and peripheral inflammation and detail ECM changes that may follow. In addition, we will discuss recent data our lab has obtained on ECM components and effectors in APOE4/4 and APOE3/3 expressing murine brain lysates, as well as human cerebrospinal fluid (CSF) samples from APOE3 and APOE4 expressing AD individuals. We will describe the principal molecules that function in ECM turnover as well as abnormalities in these molecular systems that have been observed in AD. Finally, we will communicate therapeutic interventions that have the potential to modulate ECM deposition and turnover in vivo.
In this review, we will describe mechanisms by which excess ECM accumulation may contribute to AD pathology and discuss pathological ECM alterations that occur in AD as well as conditions that increase the AD risk. We will discuss the relationship of AD risk factors to chronic central nervous system and peripheral inflammation and detail ECM changes that may follow. In addition, we will discuss recent data our lab has obtained on ECM components and effectors in APOE4/4 and APOE3/3 expressing murine brain lysates, as well as human cerebrospinal fluid (CSF) samples from APOE3 and APOE4 expressing AD individuals. We will describe the principal molecules that function in ECM turnover as well as abnormalities in these molecular systems that have been observed in AD. Finally, we will communicate therapeutic interventions that have the potential to modulate ECM deposition and turnover in vivo.
The neuronal ECM is structurally similar to ECM in other organ systems such as the cardiac myocardium. Both cardiac and neuronal ECMs have a framework composed of fibrous proteins such as collagen, fibronectin, laminin, and elastin. Furthermore within the framework, hyaluronic acid (HA) is abundant and serves as a substrate for proteoglycans with glycosaminoglycan (GAG) attachments such as chondroitin sulfate proteoglycans (CSPGs; Ex: versican). Finally, link proteins such as Tenascin-C and HAPLNs associate CSPGs with HA and with each other to impart stability. The major differences between the non-neural ECM and neural ECM are (1) the presence of CNS specific proteoglycans such as brevican and neurocan and (2) the cell types that are important to the formation and maturation of the ECM (3) the relative abundance of the individual ECM components and (4) the sulphation pattern of CSPGs.
The function of ECM in the CNS is also somewhat unique. CSPG components function to inhibit neuronal plasticity through varied mechanisms. These include inhibition of axonal growth and increased firing of select GABAergic neurons that dampen excitatory neurotransmission (Bozzelli et al., 2018). ECM proteins can also promote inflammation through their ability to interact with microglial CD44 and Toll-like Receptors (Jang et al., 2020). In contrast, CSPGs have been shown to possess neuroprotective properties including anti-inflammatory and anti-oxidant effects (Egea et al., 2010). These divergent properties of CSPGs may occur due to varied sulphation states and bioactive fragments of CSPG proteolysis (Jang et al., 2020).
Perineural Nets (PNNs) are a specialized type of compact brain ECM which predominantly surrounds the soma and proximal dendrites of parvalbumin (PV)-expressing GABAergic interneurons (Bozzelli et al., 2018) (See Figure 1). PNNs generally increase the ability of PV neurons to fire, and thus PNNs can indirectly inhibit the activity of glutamatergic neurons. For example, PNNs have been shown to decrease PV neuron membrane capacitance and increase GluA receptor insertion (Favuzzi et al., 2017; Tewari et al., 2018). PNNs also prevent lateral diffusion of GluA receptors (Frischknecht et al., 2009). Moreover, PNNs may help to localize presynaptic glutamate that is released onto PV expressing cells (Tewari et al., 2023). Consistent with these effects, disruption of PNNs has been linked to an increase in pyramidal cell activity at the single cell and population level (Slaker et al., 2015; Lensjø et al., 2017; Bozzelli et al., 2018; Alaiyed et al., 2020). Removal of PNNs has also been found to increase inhibitory synapses on PV neurons and to inhibit protein tyrosine phosphatase sigma, both of which promote pyramidal disinhibition (Lesnikova et al., 2021; Yang et al., 2021).
In the CNS, several systems modulate ECM including matrix metalloproteases and their inhibitors, molecules such as cytokines and chemokines that increase ECM component expression, and sulfatases that influence the susceptibility of PNNs to proteolysis.
The matrix metalloproteinases fall under two major families: matrix metalloproteinases (MMPs) and A Disintegrin and Metalloproteinases with or without ThromboSpondin Motifs ADAM(TS). A third family of cysteine proteases termed, Cathepsins (CTs), have also emerged as contributors to ECM homeostasis (Pantazopoulos et al., 2020). Furthermore, serine proteases (uPa, tPa, and plasmin) play a role in direct ECM degradation as well as modulation of the MMP (Tsuji et al., 2005; Zhao et al., 2008) and ADAMTS (Lemarchant et al., 2014) activation cascades.
Endogenous inhibitors of the MMPs and ADAMTSs are tissue inhibitor of metalloproteinases (TIMPs) and alpha-2-macroglobulin (α2M). SERPINs can instead inhibit serine proteases to indirectly reduce levels of active MMPs (Tsuji et al., 2005; Zhao et al., 2008) and ADAMTSs (Lemarchant et al., 2014).
While varied studies have performed in vitro digests to examine the susceptibility of ECM components to MMP mediated proteolysis, whether MMPs are expressed in proximity to these components is an unanswered question. However, data from in vivo studies with knockouts or specific inhibitors suggests that MMP-9 can attenuate PNN levels in association with a serotonin norepinephrine reuptake inhibitor (Alaiyed et al., 2020) or light reintroduction after visual deprivation/dark exposure (Murase et al., 2017). Of interest, levels of MMP-9 and/or MMP-13 are increased after kainite induced seizures or status epilepticus in which PNN attenuation is also observed (Szklarczyk et al., 2002; Rankin-Gee et al., 2015; Dubey et al., 2017).
ADAMTS proteins have also been implicated in PNN processing. For example, ADAMTS-12 homozygous knock-out (KO) mice show increased neurocan in cortical lysates compared to wild-type (WT) control mice (western blot; Fontanil et al., 2019). In another study of ADAMTS proteins, Demircan et al., found no difference in aggrecan cleavage fragments (~50 and ~60 kDa) amongst the three groups (ADAMTS-4 KO, ADAMTS-5 KO, and WT) following spinal cord injury (SCI) but a decrease in an ~50 kDa brevican fragment was observed at 7 days post-SCI in ADAMTS-4 and ADAMTS-5 KO mice compared to WT. Furthermore, they found a lack of a ~70 kDa versican fragment in both ADAMTS-4 and ADAMTS-5 KO groups which was not observed in the WT group at 7 days post-SCI (Demircan et al., 2014).
Select signaling molecules can also regulate ECM levels and, in particular, promote ECM deposition. These include TGF-β, which is believed to be one of the most potent endogenous regulators of ECM deposition. Following activation and translocation of SMAD transcription factors, both ECM depositing (collagens, fibronectins, TIMPs, PAIs) and degradation proteins (MMPs) are produced with net increased ECM deposition. Consistent with this, in primary rat astrocyte cultures treated with recombinant TGF-β full-length brevican production is increased, and this effect was not seen with treatment of recombinant IL-1β or VEGF (Hamel et al., 2005).
CCL5, which can upregulate TGF-β (Chang et al., 2012), is an additional important regulator of ECM deposition that is linked to fibrosis in varied endorgans (Berres et al., 2010). In a study with a human hepatic stellate cell line, the FDA approved CCR5 antagonist Maraviroc reduced deposition of ECM proteins including fibrillar collagens and it also reduced levels of TGF-β and TIMP-1 (Coppola et al., 2018). Of note, hepatic stellate cells share many characteristics and homeostatic functions with astrocytes including GFAP expression (Schachtrup et al., 2011; Liao et al., 2013).
PNN sulfation also regulates ECM levels. For example, sulfation can significantly impact PNN susceptibility to proteolysis (Foscarin et al., 2017). In the CNS, there are five chondroitin sulfate (CS) sulphation isomers: non-sulfated-CS, two mono-sulphated isomers (4-CS and 6-CS), and two di-sulphated isomers (4,6-CS or 2,6-CS). The mono sulphated chondroitin sulfate proteoglycans (CSPGs) are the major forms in the CNS with a lesser 4/6-CS ratio during development that gradually increases throughout adulthood (Kitagawa et al., 1997). It has been shown that the 4-CS imparts rigidity on the adult CNS and that 6-CS is permissive to axonal growth and plasticity (Lin et al., 2011; Yang et al., 2021) In a recent study, deletion of chondroitin-6 sulfotransferase led to a reduction in 6-CS levels and relatively early-onset age-related memory impairment (Yang et al., 2021).
Developmentally regulated deposition of PNNs closes critical periods of neuronal plasticity and tends to stabilize neuronal synapses and networks (Christensen et al., 2021). This stabilizing function may be impaired in CNS disorders where PNNs are disrupted or diminished including schizophrenia (SCZ; Mauney et al., 2013; Enwright et al., 2016), bipolar depression (BPD; Pantazopoulos et al., 2007; Alcaide et al., 2019), and autism spectrum disorder (ASD; Brandenburg and Blatt, 2022). Alternatively, excessive PNN deposition can impair the ability for new learning and may also detrimentally affect neuronal population activity important to learning and memory. With respect to the latter, excess PNN, as determined based on increased fluorescence following WFA immunostaining and an increased percentage of neurons with detectable PNN, has been linked to reductions in the power of gamma oscillations and the abundance of sharp wave ripples, high frequency oscillations that play a role in working memory and memory consolidation (Bozzelli et al., 2018; Alaiyed et al., 2020). In addition, attenuation of PNNs increases gamma power and SWR abundance (Lensjø et al., 2017; Alaiyed et al., 2020), possibly by diminishing PV neuron-mediated GABAergic inhibition of pyramidal cells (Slaker et al., 2015).
Importantly, gamma power is reduced in AD and in animal models of the same (Klein et al., 2016; Murty et al., 2021; Traikapi and Konstantinou, 2021). Gamma power and sharp wave ripple abundance are also reduced in human APOE4-knock-in (KI) mice and the magnitude of gamma power reduction is correlated with the level of subsequent cognitive decline. In a more recent study using wild type or TgF344-AD rats, low and high frequency gamma power were reduced at an early stage in the latter (6 months; low levels of amyloid accumulation; Stoiljkovic et al., 2019; Moradi et al., 2022). PNN and ECM components may also bind amyloid so that its clearance is impaired. For example, an association between CSPGs and plaques have been described in AD brain (DeWitt et al., 1993; Lepelletier et al., 2017; Hebisch et al., 2023). And while ECMs can also sequester and localize beneficial growth factors, recent studies suggest that a variety of profibrotic chemokines including CCL2 and CCL5 are sequestered as well (Hirose et al., 2001; Masuda et al., 2013).
ECM changes with age may arise from diverse causes including cell senescence, which occurs in association with chronic fibrotic diseases (Blokland et al., 2020). A senescence-associated-secretory phenotype (SASP) has been described (Sikora et al., 2021), in which secretory products may contribute to direct and indirect ECM pathology (dysregulation of proteases, ECM components, cytokines and chemokines). Indeed, a variety of profibrotic molecules are elevated in association with aging including TGF-β (Tominaga and Suzuki, 2019).
Consistent with this, there are several findings that show alterations in ECM properties with aging. For example, Mafi et al. found an increase in PNN density in aged Norway rats in the inferior colliculus (IC; Mafi et al., 2020), while Brewton et al. found a decrease in PNN density in the auditory cortex of aged mice (Brewton et al., 2016). In aged Octodon degus rodents, which can develop AD like pathology, a slight increase in the intensity and the number of both PNN-and PV-positive cells is detected in the entorhinal cortex of those with AD-like pathology compared to aged degus without AD like pathology (Tan et al., 2022). In addition, the degus with AD-like pathology also express increased levels of glial fibrillary acidic protein (GFAP), which is of interest in that activated astrocytes may express increased levels of PNN components.
As discussed in the section on ECM effectors, there is also an age-related reduction in C6S which renders PNNs more inhibitory (Baidoe-Ansah et al., 2022). This is supported by mechanistic studies in which deletion of chondroitin 6-sulfotransferase simulates aspects of brain aging (Yang et al., 2021). It has been shown that cortical 4-CS increases with ageing in rats and chickens (Kitagawa et al., 1997; Foscarin et al., 2017). Interestingly, in a study of healthy human adults (14–89 years of age), authors noted a significant positive correlation of 4-CS/6-CS ratio with age in synovial joint fluid (Uesaka et al., 2004) and it would be important to determine if this relationship also exists in CSF an overview of ECM effector changes that may occur with aging and inflammation is shown in Figure 2.
ECM changes may also occur with APOE expression. The targeted replacement (TR) and KI human APOE mouse models permit us to investigate ECM dynamics independent of Aβ deposition since these mice do not show accumulation of Aβ plaque with age. Astrocytes from these and other mice have been a focus of APOE-directed research as they are the major producers and secretors of brain APOE (Liao et al., 2017). Microglia produce APOE to a significantly lesser extent than astrocytes, though they likely contribute to APOE4-dependent pathology (Shi et al., 2019; Henningfield et al., 2022). Importantly, APOE4 glia produce and secrete less APOE protein than APOE3 and APOE2 glia (Riddell et al., 2008; Lanfranco et al., 2021), which is relevant since APOE is thought to reduce inflammation, a significant driver of overall ECM accumulation (Ulbrich et al., 2021). Our lab has observed increased PNN deposition in retro-splenial cortex and hippocampus of APOE4-TR compared to APOE3-TR (Blanco et al., unpublished observations). Furthermore, we also demonstrated that APOE4-TR mice have decreased ~50 kDa brevican cleavage fragment in CSF samples from human APOE4/4 s compared to APOE3/3 s (Greco et al., 2023). A reduction in this cleavage fragment has also been observed in a murine model of AD (Ajmo et al., 2010).
In other work examining ECM changes with APOE4, Keable et al. found that astrocytes cultured from KI APOE4 mice pups secreted significantly greater amount of fibronectin than KI APOE3 astrocytes and that increased fibronectin could enhance Aβ aggregation in the cerebral microvasculature (Keable et al., 2020). Of interest is that APOE4 murine microglia exhibit significantly increased SERPINA3 mRNA than APOE3 murine microglia that was found in two separate studies (Machlovi et al., 2022; Sepulveda et al., 2022). Serpins can inhibit the activity of ECM degrading proteases. Furthermore, in the same transcriptomic study (Machlovi et al., 2022), they found significantly decreased matrix metalloproteinase-2 (MMP-2) mRNA in APOE4-TR compared to APOE3-TR. Given MMP-2’s role in ECM homeostasis, this evidence supports increased ECM deposition in APOE4 carriers.
Work from our lab has shown increased TIMP-1 and CCL5 in CSF samples from humans expressing APOE4 and brain lysates from mice with a targeted replacement of this allele (Greco et al., 2023). In addition, recently published work from the Goate lab shows chemokine and matrisome (ECM protein and associated factors) increases in human IPSC-derived APOE4 astrocytes. This work also shows that APOE4 microglia are enriched for ECM, chemokine and cytokine signaling pathways in multiple brain regions (Tcw et al., 2022).
Of additional interest, ECM changes at the level of the blood brain barrier basement membrane may occur with APOE4 (Jackson et al., 2022). The risk of anti-amyloid antibody associated microhemorrhages is increased in APOE4 expressing individuals (Withington and Turner, 2022) and this could be due in part to increased amyloid sequestration by excess basement membrane matrix deposition.
Obesity is a major risk factor for the development of disorders characterized by excess ECM deposition in endorgans. These include Type II diabetes (T2D) and non-alcoholic fatty liver disease (NAFLD). Furthermore, obesity increases the risk for cognitive impairment, with several studies noting deficits in cognitive domains (working memory, verbal learning, episodic memory) in obese compared to non-obese individuals (Cournot et al., 2006; Gunstad et al., 2006; Fergenbaum et al., 2009; Coppin et al., 2014).
Adverse ECM effects due to obesity may be attributed to metabolic dysfunction, chronic inflammation, and mechanical stress. In T2D ECM pathology may occur in varied endorgans and lead to disorders including diabetic retinopathy, characterized by pathological ECM deposition and basement membrane thickening with progressive blindness (Giblin et al., 2022). In NAFLD, a disorder in which approximately 25% of patients will progress to liver cirrhosis. NAFLD-associated cirrhosis is characterized by intense collagen deposition by activated stellate cells (Fernando et al., 2019), a cell type which shares many common features with CNS astrocytes (Schachtrup et al., 2011). Moreover, in rodent models of NAFLD, activated stellate cells deposit CSPGs such as versican which can inhibit hepatocyte regeneration and recovery from injury (Bukong et al., 2016). Of interest, NAFLD is associated with an increased risk of developing dementia (Filipović et al., 2018; Shang et al., 2022). In addition, advanced liver fibrosis has been associated with increased rhinal Tau(Weinstein et al., 2022). Though the reasons for increased dementia risk in NAFLD are likely multifactorial, with a potential role for impaired cerebral perfusion and hepatic clearance of toxic molecules (Hadjihambi, 2022), it is tempting to speculate that chronic intracerebral inflammation may contribute.
Whether obesity itself is associated excess ECM deposition in the brain warrants further study. The idea is, however, supported by the link between obesity and neuroinflammation, with increased expression of pro-fibrotic molecules such as TGF-β (Yan et al., 2014; Salas-Venegas et al., 2022). It is also supported by studies that have examined effects of obesity in animal models of AD. For example, in a study using a murine model of AD, a high fat diet (HFD) was associated with increases in hippocampal levels of TIMP-1, CCL2, and CCL5 and increased hippocampal astrocyte and microgliosis. Furthermore, these markers correlated with hippocampal TSPO PET signal, a marker of inflammation (Barron et al., 2016). In two separate studies with AD-obesity mouse models, significantly increased cerebrovascular amyloid deposition was also observed (Takeda et al., 2010; Vargas-Soria et al., 2022). Dual AD-obesity mouse models also perform significantly worse on hippocampal-dependent memory tasks (Takeda et al., 2010; Barron et al., 2016). Interestingly, it has also been demonstrated that obese AD mice have increased hippocampal tau hyperphosphorylation compared to obese, AD, and control groups (Platt et al., 2016). Together these studies emphasize potent synergistic effects between obesity and AD pathology that results in amplified amyloid deposition, tau phosphorylation, and neuroinflammation.
While depression is often an initial symptom of AD, long standing recurrent untreated major-depressive disorder (MDD) is thought to be an independent risk factor for AD (Holmquist et al., 2020; Nedelec et al., 2022). While several mechanisms may be at play including corticosterone mediated reductions in hippocampal dendritic arbor (Watanabe et al., 1992; Magarinos and McEwen, 1995; Conrad et al., 1999; Kleen et al., 2006; McLaughlin et al., 2007), it should be noted that recent studies suggest that ECM deposition in increased in the hippocampus with rodent models of the same (Riga et al., 2017; Alaiyed et al., 2020). For example, in a rat model of chronic social defeat–induced persistent stress ECM increases were observed in the hippocampus. In addition, associated cognitive deficits were normalized by chondroitinase ABC (ChABC) injections that attenuated PNN levels (Riga et al., 2017). Moreover, in a murine model of chronic corticosterone induced depression. PNN increases were also observed in the hippocampus and venlafaxine, a serotonin-norepinephrine reuptake inhibitor could normalize PNN levels and excitatory/inhibitory balance (Alaiyed et al., 2020).
In contrast to MDD, BPD is has been associated with reduced PNN levels (Pantazopoulos et al., 2007). Indeed, SSRIs are often contraindicated in BPD as they can precipitate manic episodes (Patel et al., 2015). First line therapy for bipolar disorder (mood stabilizers) differs from that for major depressive disorder, possibly due to differences in the underlying pathology.
Consistent with MDD findings, it is increasingly appreciated that acute stress can upregulate ECM remodeling while chronic stress, a significant risk factor for depression, is instead associated with excess ECM deposition (Ulbrich et al., 2021). ECM deposition and remodeling involves a complex variety of players, as discussed in an earlier section of this review, with players that favor degradation upregulated at early time points following stress or injury and players that shift the balance towards increased ECM deposition expressed as the insult moves to the chronic stage. In support of this, with acute CNS injury, microglia produce a wide array of proteases that function to breakdown ECM components. These include MMP-9, ADAMs and ADAMTSs (Kaushik et al., 2021). Astrocytes instead produce and secrete a variety of ECM components such as collagens, laminins, GAG-linked lecticans such as CSPGs, as well as link proteins and hyaluronan synthase (Ulbrich et al., 2021). Astrocytes also express MMP inhibitors including TIMP-1 (Crocker et al., 2006; Hasel et al., 2021), and this cell type forms the glial scar following traumatic brain injury (TBI; Mira et al., 2021) or stroke (Huang et al., 2014). Thus, a simplified perspective on glia and ECM is that with chronic injury or stress astrocytes serve a “building” role while with acute injury microglia serve a “deconstructing” role in the CNS ECM (Ulbrich et al., 2021).
There are several findings that show alterations in ECM properties and/or ECM effector levels with AD. ECM changes have been hypothesized to alter Aβ clearance, glial responses to amyloid, and neuronal sensitivity to amyloid (DeWitt et al., 1993; Lepelletier et al., 2017; Hebisch et al., 2023). In autopsy-based studies, CSPGs have been associated with amyloid plaques (DeWitt et al., 1993) and tau tangles. Furthermore, increases in collagen IV, perlecan and fibronectin were found to correlate with amyloid levels (Lepelletier et al., 2017). Palu and Liesi showed increased α1 and γ1 laminins in AD frontal cortices that co-localized with reactive astrocytes (Palu and Liesi, 2002). Shimizu et al., observed a 1.6-fold increase in total proteoglycans in the hippocampus and 4.3 fold increase in total proteoglycans in the superior frontal gyrus of AD brains compared to age-matched, healthy controls (Shimizu et al., 2009). In addition, they showed co-localization of proteoglycans with amyloid plaques. Interestingly, in a separate study, a positive correlation between amyloid plaque deposition and ECM components in AD individuals was observed (Damodarasamy et al., 2020).
PNN changes in AD, including altered sulfation patterns are well reviewed, in (Sun et al., 2021; Ali et al., 2022; Fawcett et al., 2022; Logsdon et al., 2022; Scarlett et al., 2022). Logsdon et al., found a significant increase in CS-GAGs in PFC in AD compared to healthy controls (Logsdon et al., 2022). Liddelow’s lab showed increased levels of 4-sulfotransferase, in AD brain-derived astrocytes (Sadick et al., 2022). This likely increases 4S-CSPG levels which are less susceptible than 6S-CSPGs to proteolysis.
While a recent review detailing PNN changes in AD found that a majority of PNN components are upregulated in AD (HA, HSPGs, CSPGs, DSPGs, TNC, and TNR) with two components decreased (reelin and keratin sulfate proteoglycans; Sun et al., 2021), Bruckner and colleagues showed no change in fluorescent intensity using pan-Anti-CSPG antibody in AD frontal and temporal sections compared to controls (ntotal = 12; Brückner et al., 1999). Morawski and colleagues also demonstrated no change in WFA, parvalbumin, brevican, and aggrecan IHC labeling in AD brains (n = 12) vs. controls (n = 12; Morawski et al., 2012).Differing observations may be explained by the tools used to detect the PNNs. Baig et al. utilized WFA, a lectin stain that binds N-acteylgalactosamine residues of the lecticans (Baig et al., 2005), and Lendvai used an antibody to the protein component of brevican (Lendvai et al., 2013). Furthermore, it is important to recognize that PNN lectican antibodies have epitopes to different parts of the PNN glycoproteins and thus immunohistochemical data may vary across studies due to PNN structural variability coupled with the heterogeneity of antibody epitope recognition sites. Taken together, the observed differences in these human studies may represent an aspect of changing PNN quality and not quantity. Furthermore, this notion of changing quality in AD brains but not quantity of PNNs or PV+ neurons was supported in a recent study of human AD brains where the sulphation code is implicated (Logsdon et al., 2022). Indeed, a recent review proposed an updated model of PNN pathology in AD where it is believed that reduced WFA+ intensity seen in postmortem AD brains is due to structural and sulphation alterations that reduce WFA’s affinity to PNNs; thus, giving the illusion of reduced PNN density (Scarlett et al., 2022). These studies suggest that future investigations examining PNNs in AD humans and rodent models need to address PNN quality changes that cannot be assessed with traditional methods of IHC labeling or Western blot detection.
With respect to ECM changes with amyloid or tau Tg mouse models of AD, two separate studies have found increased hippocampal brevican levels in these models (Ajmo et al., 2010; Végh et al., 2014a). In addition, one group showed a concomitant decrease in the proteolytic-generated ~50 kDa brevican cleavage fragment in hippocampal lysates from Tg mice (APP/Swe) compared to WT controls (Ajmo et al., 2010). It has also been shown that ChABC intrahippocampal injection in AD mouse models alleviates both neuropathology and cognitive deficits (Howell et al., 2015; Yang et al., 2015). In particular, contextual-fear learning and hippocampal slice long term potentiation (LTP) are increased in ChABC treated APP/PS1 versus penicillinase treated controls (Végh et al., 2014b). ChABC also reduced amyloid load and increased synaptic density in the APP/PS1 mice (Howell et al., 2015). In two separate AD-tau mouse models, ChABC treatment also improved cognitive deficits as assessed by object recognition test and increased field excitatory synaptic potential amplitudes in perirhinal cortex (Yang et al., 2015).
In terms of ECM effectors, analysis of CSF samples from sporadic cerebral amyloid angiopathy (CAA), which shares parallels with AD and can be coincident with the same (Vervuurt et al., 2023), shows a significant reduction in the MMP-2/TIMP-2 ratio, a change that would favor ECM deposition. Indeed, individuals who are afflicted with CAA have increased deposition of matrix at the blood brain barrier basement membrane. Previous studies also suggest that MMP-9 levels may be unchanged or reduced in AD and/or APOE4 patients as compared to controls (Adair et al., 2004; Mroczko et al., 2014). And though other reports show that MMP-9 may be elevated at the blood brain barrier with APOE4 or aggressive mouse models of AD (Halliday et al., 2016; Weekman and Wilcock, 2016; Montagne et al., 2020), potential confounds include elevated amyloid levels in aggressive murine models and/or select APOE4 patient populations (Deb and Gottschall, 1996). In a recent study, we did not see changes in MMP-9 with APOE4 genotype (Greco et al., 2023). Importantly, the ability of MMP-9 to ameliorate or exacerbate disease pathology is likely a function of quantity as well as localization. For example, increased expression of MMP-9 by activated microglia or pericytes at the blood brain barrier could have detrimental effects, while neuronal-derived and localized MMP activity may target preferentially target PNNs and synaptic adhesion molecules to enhance plasticity (Tian et al., 2007; Conant et al., 2015; Martin-de-Saavedra et al., 2022). This is supported by animal studies in which neuronal expression of MMP-9 was associated with an increase in non-amyloidogenic alpha-secretase cleaved amyloid precursor protein and well as plaque reduction and improved cognition in 6 month old female 5xFAD mice (Fragkouli et al., 2014). In contrast, another study showed that a pharmacological inhibitor of MMP-9, which would also target microglial and pericyte-derived enzyme activity, improved cognition but had no effect on plaque load in the 5XFAD model (Ringland et al., 2021).
Figure 1. Schematic representation of general PNN structure. Hyaluronic acid (HA) serves as the backbone of PNNs. The lecticans (aggrecan, versican, neurocan, and brevican) associate with HA, link proteins (HAPLNs) stabilize this association, and tenascins cross-links lecticans. TGF-β signaling promotes astrocytic production and secretion of CSPGs. Deposited CSPGs can sequester amyloid plaques and hinder degradation. This image was created using Biorender graphic software (https://Biorender.com).
Following the experiments that provided mounting evidence of PNN’s inhibitory role in neuroplasticity, there is clinical interest in targeting PNNs for disruption to promote recovery in neurological pathology. Intracerebral injection of ChABC in aged rodent models improves memory-dependent behaviors in a variety of AD models. Furthermore, in a spinal-cord injury (SCI) model, local ChABC injection improved functional outcome and recovery (Bradbury et al., 2002). However, ChABC is a bacterial enzyme, thermally instable, and requires continuous injections for maintenance of PNN levels. In this review it is beyond our scope to address all avenues of therapeutic PNN manipulation.
Figure 2. Schematic representation illustrating increase in specific PNN components and effectors with age and inflammation: TGF-β (Fessel, 2019; Yan et al., 2014; Tominaga and Suzuki, 2019), TIMP-1 (Baird et al., 2012; Hasel et al., 2021), 4/6-CS ratio (Foscarin et al., 2017; Baidoe-Ansah et al., 2022), and PNNs (Karetko-Sysa et al., 2014; Végh, Rausell, et al., 2014b; Mafi et al., 2020). This image was created using Biorender graphic software (https://Biorender.com).
Selective serotonin reuptake inhibitor (SSRIs) and selective serotonin-norepinephrine reuptake inhibitors (SNRIs) are typically used for management of anxiety and depressive symptoms; however, a full understanding of therapeutic mechanisms is lacking. Of interest, these medications have been shown to modulation PNN integrity. In accordance with this hypothesis, our lab has previously shown that MMP-9 dependent attenuation of PNNs can impact physiological markers associated with learning and memory deficits. Using a corticosterone-based mouse model of stress, Alaiyed et al. (2020) demonstrated an increase in expression of PSD-95, an increase in expression of MMP-9, increased pyramidal cell arborization, and increased gamma power in male C57BL/6 J mice treated with the antidepressant Venlafaxine (VFX). In VFX treated MMP-9 null mice, these effects were not seen (Alaiyed et al., 2020). These findings support a requirement for MMP-9 in effecting physiological PNN remodeling in vivo.
The successful use of antidepressant medications for modulation of PNNs has also been shown with SSRIs including fluoxetine (FLX). In two separate studies (Ohira et al., 2013; Guirado et al., 2014), FLX treated mice showed decreased PNN density and PV+ neurons in both mPFC and in hippocampus compared to vehicle. In concurrence with these findings, Mukhopadhyay et al., 2021 found a decrease in CA1 and CA3 hippocampal PNNs following FLX treatment in Sprague Dawley rats (Mukhopadhyay et al., 2021). Moreover, Ohira et al., 2019 found reduction of DG and CA3 hippocampal PNN density and PV+ interneurons in FLX treated marmosets (Ohira et al., 2019). Through both attenuation of PNNs and consequent effects on PV interneuron excitability, antidepressant medications may be of use in a combination treatment for the maintenance of plasticity as well as the prevention/alleviation of depressive symptoms commonly associated with AD (Dityatev et al., 2010; Donato et al., 2013).
Targeting PNN sulphation has also shown promise in two separate studies. Pearson et al., found that administration of Aryl-Sulfatase B (ARSB), selectively cleaves 4-CS groups on CSPGs, improved neurite outgrowth in vitro and regeneration of optic nerve lesion in vivo (Pearson et al., 2018). Furthermore, they found that post-fixed mouse brain sections incubated with ChABC drastically decreased PNN density whereas incubation with ASRB did not change PNN density. In a more recent study Yang et al., stereotaxically delivered an AAV-chst3 (encodes 6-sulfonotransferase) to perirhinal cortex (PRh) of aged C57BL/6 mice, and found recovery in memory impairment (Yang et al., 2021). In addition, they demonstrated reduction in PV+ neurons and PNN density in AAV-chst3 compared to AAV-GFP control group.
4-methylumbelliferone (4-MU) is an HA synthesis inhibitor approved in Europe for treatment of biliary spasms and demonstrates well-tolerance at high doses in humans. 4-MU functions to inhibit hyaluronic acid (HA) synthesis. Dubisova et al., examined its effects on PNNs in healthy adult C57BL/6JOlaHsd mice given 6-months of oral 4-MU in chow (Dubisova et al., 2022). They found a 72% reduction in GAG content in the brain and 50% reduction in spinal cord compared to controls. Furthermore, 4-MU treated mice showed improved hippocampal-dependent memory performance (spontaneous object recognition task and spontaneous alteration test).
In several studies, ketamine has shown ability to modulate PNNs in vivo. Matuszko et al., showed a reduction in PNN density and PV expression in mPFC of low-dose ketamine injected male SD rats (Matuszko et al., 2017). In a follow-up study, the same group demonstrated that PNNs were more numerous but immature in structure (less circular and smaller; Kaushik et al., 2021). Venturino et al., demonstrated that a single, high-dose ketamine injection was sufficient to decrease PNN density in C57BL/6 mice and this effect was increased with frequency of ketamine injections (Venturino et al., 2021). The authors attributed this mechanism of PNN reduction to increased microglia phagocytosis and proteolysis of PNNs; moreover, this was supported by lack of PNN reduction with ketamine when microglia were pharmacologically depleted (PLX5622) or inhibited (clopidogrel). In addition, they showed that 60 Hz gamma entrainment (2 h/day of light flickering) for 5 days drastically reduced PNN density and increased neuronal and microglia MMP-9 immunoreactivity proximal to PNNs.
An alternative approach to gamma entrainment, for non-invasive manipulation of PNNs in vivo, is repetitive transcranial magnetic stimulation (rTMS). Zheng et al., demonstrated efficacy of this approach in rats in which rTMS decreased cortical PNN density compared to the sham stimulated group (Zheng et al., 2023).
Given the role of inflammatory soluble mediators in driving ECM deposition and remodeling: inhibition of the CCL5/CCR5 signaling axis may show potential in modulating PNNs. This is supported by recent studies showing maraviroc’s (CCR5 antagonist) ability to attenuate liver fibrosis in a murine model of chronic liver failure. In vitro treatment of hepatic stellate cells with maraviroc drastically decreased PNN effectors including TIMP-1, TIMP-2, and TGF-β (Coppola et al., 2018). We found decreased hippocampal TIMP-1 in APOE4/CCR5KO heterozygous mice as compared to age-matched APOE4/WT mice, which supports a physiologic role of the CCR5 axis in modulating TIMP-1 levels (Greco et al., 2023). Interestingly it was shown that humans treated with maraviroc or with the CCR5 mutation specific null-allele (delta32) have improved cognitive and functional recovery following stroke compared to non-carriers (Joy et al., 2019). Future studies should address maraviroc’s clinical application in neurodegenerative disorders such as AD where ECM homeostasis is affected.
Potential therapies to target PNN levels are summarized in Table 1.
Numerous epidemiological and genetic studies have implicated a variety of common risk factors for late onset AD. These include age and APOE genotype as well as untreated MDD and obesity.
In this review, we have highlighted chronic inflammation with increased ECM deposition as a shared feature of these predisposing conditions. Since changes in ECM quality and quantity can have adverse physiological effects, we further suggest that excess ECM deposition can restrict neuroplasticity to in turn diminish cognitive reserve. Moreover, increased PNN deposition may alter excitatory/inhibitory balance to impair gamma oscillations and working memory. We have also touched on studies that suggest some ECM proteins may sequester amyloid and thus impair its clearance. Future studies are warranted to test ECM specific interventions in AD and AD risk factor models. Studies could also characterize specific ECM and PNN changes following traumatic brain injury (TBI), which also increases AD risk (Griffiths et al., 2020; Livingston et al., 2020). Of interest, TBI has been associated with chronic inflammation, increased TGF-β, and increased C-4S levels (Bhattacharyya et al., 2015). Future studies are also warranted to determine whether additional conditions associated with chronic brain inflammation, such as long COVID or HIV infection, impair cognition in part through effects on brain ECM.
MA, SD, and KC conducted the literature search, drafted the manuscript, and prepared the figures. RT provided the human AD CSF samples and assisted in intellectual content. All authors contributed to the article and approved the submitted version.
This work was supported by the National Institutes of Health under R01AG077002.
The authors declare that the research was conducted in the absence of any commercial or financial relationships that could be construed as a potential conflict of interest.
All claims expressed in this article are solely those of the authors and do not necessarily represent those of their affiliated organizations, or those of the publisher, the editors and the reviewers. Any product that may be evaluated in this article, or claim that may be made by its manufacturer, is not guaranteed or endorsed by the publisher.
Adair, J. C., Charlie, J., Dencoff, J. E., Kaye, J. A., Quinn, J. F., Camicioli, R. M., et al. (2004). Measurement of gelatinase B (MMP-9) in the cerebrospinal fluid of patients with vascular dementia and Alzheimer disease. Stroke 35, e159–e162. doi: 10.1161/01.STR.0000127420.10990.76
Ajmo, J. M., Bailey, L. A., Howell, M. D., Cortez, L. K., Pennypacker, K. R., Mehta, H. N., et al. (2010). Abnormal post-translational and extracellular processing of brevican in plaque-bearing mice over-expressing APPsw. J. Neurochem. 113, 784–795. doi: 10.1111/j.1471-4159.2010.06647.x
Alaiyed, S., McCann, M., Mahajan, G., Rajkowska, G., Stockmeier, C. A., Kellar, K. J., et al. (2020). Venlafaxine stimulates an MMP-9-dependent increase in excitatory/inhibitory balance in a stress model of depression. J. Neurosci. 40, 4418–4431. doi: 10.1523/JNEUROSCI.2387-19.2020
Alcaide, J., Guirado, R., Crespo, C., Blasco-Ibáñez, J. M., Varea, E., Sanjuan, J., et al. (2019). Alterations of perineuronal nets in the dorsolateral prefrontal cortex of neuropsychiatric patients. Int. J. Bipolar Disord. 7:24. doi: 10.1186/s40345-019-0161-0
Ali, A. B., Islam, A., and Constanti, A. (2022). The fate of interneurons, GABAA receptor sub-types and perineuronal nets in Alzheimer’s disease. Brain Pathol. 33:e13129. doi: 10.1111/bpa.13129
Almeida, O. P., Hankey, G. J., Yeap, B. B., Golledge, J., and Flicker, L. (2017). Depression as a modifiable factor to decrease the risk of dementia. Transl. Psychiatry 7:e1117. doi: 10.1038/tp.2017.90
Alonge, K. M., Dorfman, M. D., Scarlett, J. M., Melhorn, S. J., Ye, Y., Hu, S. J. W., et al. (2022). 152-OR: evidence linking loss of hypothalamic perineuronal nets to obesity pathogenesis. Diabetes 71:152-OR. doi: 10.2337/db22-152-OR
Baidoe-Ansah, D., Sakib, S., Jia, S., Mirzapourdelavar, H., Strackeljan, L., Fischer, A., et al. (2022). Aging-associated changes in cognition, expression and epigenetic regulation of chondroitin 6-sulfotransferase Chst3. Cells 11:13. doi: 10.3390/cells11132033
Baig, S., Wilcock, G. K., and Love, S. (2005). Loss of perineuronal net N-acetylgalactosamine in Alzheimer’s disease. Acta Neuropathol. 110, 393–401. doi: 10.1007/s00401-005-1060-2
Baird, G. S., Nelson, S. K., Keeney, T. R., Stewart, A., Williams, S., Kraemer, S., et al. (2012). Age-Dependent Changes in the Cerebrospinal Fluid Proteome by Slow Off-Rate Modified Aptamer Array. The American Journal of Pathology 180, 446–456. doi: 10.1016/j.ajpath.2011.10.024
Barron, A. M., Tokunaga, M., Zhang, M.-R., Ji, B., Suhara, T., and Higuchi, M. (2016). Assessment of neuroinflammation in a mouse model of obesity and β-amyloidosis using PET. J. Neuroinflammation 13:221. doi: 10.1186/s12974-016-0700-x
Berres, M.-L., Koenen, R. R., Rueland, A., Zaldivar, M. M., Heinrichs, D., Sahin, H., et al. (2010). Antagonism of the chemokine Ccl5 ameliorates experimental liver fibrosis in mice. J. Clin. Invest. 120, 4129–4140. doi: 10.1172/JCI41732
Bhattacharyya, S., Zhang, X., Feferman, L., Johnson, D., Tortella, F. C., Guizzetti, M., et al. (2015). Decline in arylsulfatase B and increase in chondroitin 4-sulfotransferase combine to increase chondroitin 4-sulfate in traumatic brain injury. J. Neurochem. 134, 728–739. doi: 10.1111/jnc.13156
Blokland, K. E. C., Pouwels, S. D., Schuliga, M., Knight, D. A., and Burgess, J. K. (2020). Regulation of cellular senescence by extracellular matrix during chronic fibrotic diseases. Clin. Sci. 134, 2681–2706. doi: 10.1042/CS20190893
Bozzelli, P. L., Alaiyed, S., Kim, E., Villapol, S., and Conant, K. (2018). Proteolytic remodeling of perineuronal nets: effects on synaptic plasticity and neuronal population dynamics. Neural Plast. 2018:5735789. doi: 10.1155/2018/5735789
Bradbury, E. J., Moon, L. D. F., Popat, R. J., King, V. R., Bennett, G. S., Patel, P. N., et al. (2002). Chondroitinase ABC promotes functional recovery after spinal cord injury. Nature 416, 636–640. doi: 10.1038/416636a
Brandenburg, C., and Blatt, G. J. (2022). Region-specific alterations of perineuronal net expression in postmortem autism brain tissue. Front. Mol. Neurosci. 15:838918. doi: 10.3389/fnmol.2022.838918
Brewton, D. H., Kokash, J., Jimenez, O., Pena, E. R., and Razak, K. A. (2016). Age-related deterioration of perineuronal nets in the primary auditory cortex of mice. Front. Aging Neurosci. 8:270. doi: 10.3389/fnagi.2016.00270
Brückner, G., Hausen, D., Härtig, W., Drlicek, M., Arendt, T., and Brauer, K. (1999). Cortical areas abundant in extracellular matrix chondroitin sulphate proteoglycans are less affected by cytoskeletal changes in Alzheimer’s disease. Neuroscience 92, 791–805. doi: 10.1016/S0306-4522(99)00071-8
Bukong, T. N., Maurice, S. B., Chahal, B., Schaeffer, D. F., and Winwood, P. J. (2016). Versican: a novel modulator of hepatic fibrosis. Lab. Investig. 96:Article 3. doi: 10.1038/labinvest.2015.152
Chang, L.-Y., Lin, Y.-C., Mahalingam, J., Huang, C.-T., Chen, T.-W., Kang, C.-W., et al. (2012). Tumor-derived chemokine CCL5 enhances TGF-β-mediated killing of CD8(+) T cells in colon cancer by T-regulatory cells. Cancer Res. 72, 1092–1102. doi: 10.1158/0008-5472.CAN-11-2493
Christensen, A. C., Lensjø, K. K., Lepperød, M. E., Dragly, S.-A., Sutterud, H., Blackstad, J. S., et al. (2021). Perineuronal nets stabilize the grid cell network. Nat. Commun. 12:253. doi: 10.1038/s41467-020-20241-w
Conant, K., Allen, M., and Lim, S. T. (2015). Activity dependent CAM cleavage and neurotransmission. Frontiers in Cellular Neuroscience 9 https://www.frontiersin.org/articles/10.3389/fncel.2015.00305
Conrad, C. D., Magariños, A. M., LeDoux, J. E., and McEwen, B. S. (1999). Repeated restraint stress facilitates fear conditioning independently of causing hippocampal CA3 dendritic atrophy. Behav. Neurosci. 113, 902–913. doi: 10.1037/0735-7044.113.5.902
Coppin, G., Nolan-Poupart, S., Jones-Gotman, M., and Small, D. M. (2014). Working memory and reward association learning impairments in obesity. Neuropsychologia 65, 146–155. doi: 10.1016/j.neuropsychologia.2014.10.004
Coppola, N., Perna, A., Lucariello, A., Martini, S., Macera, M., Carleo, M. A., et al. (2018). Effects of treatment with Maraviroc a CCR5 inhibitor on a human hepatic stellate cell line. J. Cell. Physiol. 233, 6224–6231. doi: 10.1002/jcp.26485
Cournot, M., Marquié, J. C., Ansiau, D., Martinaud, C., Fonds, H., Ferrières, J., et al. (2006). Relation between body mass index and cognitive function in healthy middle-aged men and women. Neurology 67, 1208–1214. doi: 10.1212/01.wnl.0000238082.13860.50
Crocker, S. J., Milner, R., Pham-Mitchell, N., and Campbell, I. L. (2006). Cell and agonist-specific regulation of genes for matrix metalloproteinases and their tissue inhibitors by primary glial cells. J. Neurochem. 98, 812–823. doi: 10.1111/j.1471-4159.2006.03927.x
Damodarasamy, M., Vernon, R. B., Pathan, J. L., Keene, C. D., Day, A. J., Banks, W. A., et al. (2020). The microvascular extracellular matrix in brains with Alzheimer’s disease neuropathologic change (ADNC) and cerebral amyloid angiopathy (CAA). Fluids Barriers CNS 17:60. doi: 10.1186/s12987-020-00219-y
Deb, S., and Gottschall, P. E. (1996). Increased production of matrix metalloproteinases in enriched astrocyte and mixed hippocampal cultures treated with beta-amyloid peptides. Journal of Neurochemistry 66, 1641–1647. doi: 10.1046/j.1471-4159.1996.66041641.x
Demircan, K., Topcu, V., Takigawa, T., Akyol, S., Yonezawa, T., Ozturk, G., et al. (2014). ADAMTS4 and ADAMTS5 knockout mice are protected from versican but not aggrecan or brevican proteolysis during spinal cord injury. Biomed. Res. Int. 2014:e693746. doi: 10.1155/2014/693746
DeWitt, D. A., Silver, J., Canning, D. R., and Perry, G. (1993). Chondroitin sulfate proteoglycans are associated with the lesions of Alzheimer’s disease. Exp. Neurol. 121, 149–152. doi: 10.1006/exnr.1993.1081
Dingess, P. M., Harkness, J. H., Slaker, M., Zhang, Z., Wulff, S. S., Sorg, B. A., et al. (2018). Consumption of a high-fat diet alters perineuronal nets in the prefrontal cortex. Neural Plast. 2018, 1–8. doi: 10.1155/2018/2108373
Dingess, P. M., Zhang, Z., Sorg, B. A., Ferrario, C. R., and Brown, T. E. (2020). Sex and region-specific effects of high fat diet on PNNs in obesity susceptible rats. Physiol. Behav. 222:112963. doi: 10.1016/j.physbeh.2020.112963
Dityatev, A., Schachner, M., and Sonderegger, P. (2010). The dual role of the extracellular matrix in synaptic plasticity and homeostasis. Nature Reviews. Neuroscience 11, 735–746. doi: 10.1038/nrn2898
Donato, F., Rompani, S. B., and Caroni, P. (2013). Parvalbumin-expressing basket-cell network plasticity induced by experience regulates adult learning. Nature 504:Article 7479. doi: 10.1038/nature12866
Dubey, D., McRae, P. A., Rankin-Gee, E. K., Baranov, E., Wandrey, L., Rogers, S., et al. (2017). Increased metalloproteinase activity in the hippocampus following status epilepticus. Epilepsy Res. 132, 50–58. doi: 10.1016/j.eplepsyres.2017.02.021
Dubisova, J., Burianova, J. S., Svobodova, L., Makovicky, P., Martinez-Varea, N., Cimpean, A., et al. (2022). Oral treatment of 4-methylumbelliferone reduced perineuronal nets and improved recognition memory in mice. Brain Res. Bull. 181, 144–156. doi: 10.1016/j.brainresbull.2022.01.011
Egea, J., García, A. G., Verges, J., Montell, E., and López, M. G. (2010). Antioxidant, antiinflammatory and neuroprotective actions of chondroitin sulfate and proteoglycans. Osteoarthr. Cartil. 18, S24–S27. doi: 10.1016/j.joca.2010.01.016
Enwright, J. F., Sanapala, S., Foglio, A., Berry, R., Fish, K. N., and Lewis, D. A. (2016). Reduced labeling of Parvalbumin neurons and Perineuronal nets in the dorsolateral prefrontal cortex of subjects with schizophrenia. Neuropsychopharmacology 41, 2206–2214. doi: 10.1038/npp.2016.24
Favuzzi, E., Marques-Smith, A., Deogracias, R., Winterflood, C. M., Sánchez-Aguilera, A., Mantoan, L., et al. (2017). Activity-dependent gating of parvalbumin interneuron function by the perineuronal net protein brevican. Neuron 95, 639–655.e10. doi: 10.1016/j.neuron.2017.06.028
Fawcett, J. W., Fyhn, M., Jendelova, P., Kwok, J. C. F., Ruzicka, J., and Sorg, B. A. (2022). The extracellular matrix and perineuronal nets in memory. Mol. Psychiatry 27:Article 8. doi: 10.1038/s41380-022-01634-3
Fessel, J. (2019). Ineffective levels of transforming growth factors and their receptor account for old age being a risk factor for Alzheimer’s disease. Alzheimer’s & Dementia : Translational Research & Clinical Interventions 5, 899–905. doi: 10.1016/j.trci.2019.11.007
Fergenbaum, J. H., Bruce, S., Lou, W., Hanley, A. J. G., Greenwood, C., and Young, T. K. (2009). Obesity and lowered cognitive performance in a Canadian first nations population. Obesity 17, 1957–1963. doi: 10.1038/oby.2009.161
Fernando, D. H., Forbes, J. M., Angus, P. W., and Herath, C. B. (2019). Development and progression of non-alcoholic fatty liver disease: the role of advanced glycation end products. Int. J. Mol. Sci. 20:5037. doi: 10.3390/ijms20205037
Filipović, B., Marković, O., Đurić, V., and Filipović, B. (2018). Cognitive changes and brain volume reduction in patients with nonalcoholic fatty liver disease. Can. J. Gastroenterol. Hepatol. 2018:9638797. doi: 10.1155/2018/9638797
Fontanil, T., Mohamedi, Y., Moncada-Pazos, A., Cobo, T., Vega, J. A., Cobo, J. L., et al. (2019). Neurocan is a new substrate for the ADAMTS12 Metalloprotease: potential implications in neuropathies. Cell. Physiol. Biochem. 52, 1003–1016. doi: 10.33594/000000069
Foscarin, S., Raha-Chowdhury, R., Fawcett, J. W., and Kwok, J. C. F. (2017). Brain ageing changes proteoglycan sulfation, rendering perineuronal nets more inhibitory. Aging 9, 1607–1622. doi: 10.18632/aging.101256
Fragkouli, A., Tsilibary, E. C., and Tzinia, A. K. (2014). Neuroprotective role of MMP-9 overexpression in the brain of Alzheimer’s 5xFAD mice. Neurobiol. Dis. 70, 179–189. doi: 10.1016/j.nbd.2014.06.021
Frischknecht, R., Heine, M., Perrais, D., Seidenbecher, C. I., Choquet, D., and Gundelfinger, E. D. (2009). Brain extracellular matrix affects AMPA receptor lateral mobility and short-term synaptic plasticity. Nat. Neurosci. 12, 897–904. doi: 10.1038/nn.2338
GBD 2019 CollaboratorsNichols, E., Abd-Allah, F., Abdoli, A., Abosetugn, A. E., Abrha, W. A., et al. (2021). Global mortality from dementia: application of a new method and results from the global burden of disease study 2019. Alzheimers Dement. 7:e12200. doi: 10.1002/trc2.12200
Giblin, M. J., Ontko, C. D., and Penn, J. S. (2022). Effect of cytokine-induced alterations in extracellular matrix composition on diabetic retinopathy-relevant endothelial cell behaviors. Sci. Rep. 12:Article 1. doi: 10.1038/s41598-022-12683-7
Greco, G. A., Rock, M., Amontree, M., Lanfranco, M. F., Korthas, H., Hong, S. H., et al. (2023). CCR5 deficiency normalizes TIMP levels, working memory, and gamma oscillation power in APOE4 targeted replacement mice. Neurobiol. Dis. 179:106057. doi: 10.1016/j.nbd.2023.106057
Griffiths, D. R., Jenkins, T. M., Addington, C. P., Stabenfeldt, S. E., and Lifshitz, J. (2020). Extracellular matrix proteins are time-dependent and regional-specific markers in experimental diffuse brain injury. Brain Behav. 10:e01767. doi: 10.1002/brb3.1767
Guirado, R., Perez-Rando, M., Sanchez-Matarredona, D., Castrén, E., and Nacher, J. (2014). Chronic fluoxetine treatment alters the structure, connectivity and plasticity of cortical interneurons. Int. J. Neuropsychopharmacol. 17, 1635–1646. doi: 10.1017/S1461145714000406
Gunstad, J., Paul, R. H., Cohen, R. A., Tate, D. F., and Gordon, E. (2006). Obesity is associated with memory deficits in young and middle-aged adults. Eat. Weight Disord. 11, e15–e19. doi: 10.1007/BF03327747
Hadjihambi, A. (2022). Cerebrovascular alterations in NAFLD: is it increasing our risk of Alzheimer’s disease? Anal. Biochem. 636:114387. doi: 10.1016/j.ab.2021.114387
Halliday, M. R., Rege, S. V., Ma, Q., Zhao, Z., Miller, C. A., Winkler, E. A., et al. (2016). Accelerated pericyte degeneration and blood-brain barrier breakdown in apolipoprotein E4 carriers with Alzheimer’s disease. Journal of Cerebral Blood Flow and Metabolism: Official Journal of the International Society of Cerebral Blood Flow and Metabolism 36, 216–227. doi: 10.1038/jcbfm.2015.44
Hamel, M. G., Mayer, J., and Gottschall, P. E. (2005). Altered production and proteolytic processing of brevican by transforming growth factor β in cultured astrocytes. J. Neurochem. 93, 1533–1541. doi: 10.1111/j.1471-4159.2005.03144.x
Hasel, P., Rose, I. V. L., Sadick, J. S., Kim, R. D., and Liddelow, S. A. (2021). Neuroinflammatory astrocyte subtypes in the mouse brain. Nat. Neurosci. 24, 1475–1487. doi: 10.1038/s41593-021-00905-6
Hebisch, M., Klostermeier, S., Wolf, K., Boccaccini, A. R., Wolf, S. E., Tanzi, R. E., et al. (2023). The impact of the cellular environment and aging on modeling Alzheimer’s disease in 3D cell culture models. Adv. Sci. 10:2205037. doi: 10.1002/advs.202205037
Henningfield, C. M., Arreola, M. A., Soni, N., Spangenberg, E. E., and Green, K. N. (2022). Microglia-specific ApoE knock-out does not alter Alzheimer’s disease plaque pathogenesis or gene expression. Glia 70, 287–302. doi: 10.1002/glia.24105
Hirose, J., Kawashima, H., Yoshie, O., Tashiro, K., and Miyasaka, M. (2001). Versican interacts with chemokines and modulates cellular responses*. J. Biol. Chem. 276, 5228–5234. doi: 10.1074/jbc.M007542200
Holmquist, S., Nordström, A., and Nordström, P. (2020). The association of depression with subsequent dementia diagnosis: a Swedish nationwide cohort study from 1964 to 2016. PLoS Med. 17:e1003016. doi: 10.1371/journal.pmed.1003016
Howell, M. D., Bailey, L. A., Cozart, M. A., Gannon, B. M., and Gottschall, P. E. (2015). Hippocampal administration of chondroitinase ABC increases plaque-adjacent synaptic marker and diminishes amyloid burden in aged APPswe/PS1dE9 mice. Acta Neuropathol. Commun. 3:54. doi: 10.1186/s40478-015-0233-z
Huang, L., Wu, Z.-B., ZhuGe, Q., Zheng, W., Shao, B., Wang, B., et al. (2014). Glial scar formation occurs in the human brain after ischemic stroke. Int. J. Med. Sci. 11, 344–348. doi: 10.7150/ijms.8140
Jackson, R. J., Meltzer, J. C., Nguyen, H., Commins, C., Bennett, R. E., Hudry, E., et al. (2022). APOE4 derived from astrocytes leads to blood–brain barrier impairment. Brain 145, 3582–3593. doi: 10.1093/brain/awab478
Jang, D. G., Sim, H. J., Song, E. K., Kwon, T., and Park, T. J. (2020). Extracellular matrixes and neuroinflammation. BMB Rep. 53, 491–499. doi: 10.5483/BMBRep.2020.53.10.156
Joy, M. T., Ben Assayag, E., Shabashov-Stone, D., Liraz-Zaltsman, S., Mazzitelli, J., Arenas, M., et al. (2019). CCR5 is a therapeutic target for recovery after stroke and traumatic brain injury. Cells 176, 1143–1157.e13. doi: 10.1016/j.cell.2019.01.044
Karetko-Sysa, M., Skangiel-Kramska, J., and Nowicka, D. (2014). Aging somatosensory cortex displays increased density of WFA-binding perineuronal nets associated with GAD-negative neurons. Neuroscience 277, 734–746. doi: 10.1016/j.neuroscience.2014.07.049
Kaushik, R., Lipachev, N., Matuszko, G., Kochneva, A., Dvoeglazova, A., Becker, A., et al. (2021). Fine structure analysis of perineuronal nets in the ketamine model of schizophrenia. Eur. J. Neurosci. 53, 3988–4004. doi: 10.1111/ejn.14853
Keable, A., O’Neill, R., MacGregor Sharp, M., Gatherer, M., Yuen, H. M., Johnston, D. A., et al. (2020). ApoE4 astrocytes secrete basement membranes rich in Fibronectin and poor in Laminin compared to ApoE3 astrocytes. Int. J. Mol. Sci. 21:4371. doi: 10.3390/ijms21124371
Kitagawa, H., Tsutsumi, K., Tone, Y., and Sugahara, K. (1997). Developmental regulation of the sulfation profile of chondroitin sulfate chains in the chicken embryo brain. J. Biol. Chem. 272, 31377–31381. doi: 10.1074/jbc.272.50.31377
Kleen, J. K., Sitomer, M. T., Killeen, P. R., and Conrad, C. D. (2006). Chronic stress impairs spatial memory and motivation for reward without disrupting motor ability and motivation to explore. Behav. Neurosci. 120, 842–851. doi: 10.1037/0735-7044.120.4.842
Klein, A. S., Donoso, J. R., Kempter, R., Schmitz, D., and Beed, P. (2016). Early cortical changes in gamma oscillations in Alzheimer’s disease. Front. Syst. Neurosci. 10:83. doi: 10.3389/fnsys.2016.00083
Knuesel, I., Nyffeler, M., Mormède, C., Muhia, M., Meyer, U., Pietropaolo, S., et al. (2009). Age-related accumulation of Reelin in amyloid-like deposits. Neurobiol. Aging 30, 697–716. doi: 10.1016/j.neurobiolaging.2007.08.011
Lanfranco, M. F., Sepulveda, J., Kopetsky, G., and Rebeck, G. W. (2021). Expression and secretion of apoE isoforms in astrocytes and microglia during inflammation. Glia 69, 1478–1493. doi: 10.1002/glia.23974
Lemarchant, S., Pruvost, M., Hébert, M., Gauberti, M., Hommet, Y., Briens, A., et al. (2014). TPA promotes ADAMTS-4-induced CSPG degradation, thereby enhancing neuroplasticity following spinal cord injury. Neurobiol. Dis. 66, 28–42. doi: 10.1016/j.nbd.2014.02.005
Lendvai, D., Morawski, M., Négyessy, L., Gáti, G., Jäger, C., Baksa, G., et al. (2013). Neurochemical mapping of the human hippocampus reveals perisynaptic matrix around functional synapses in Alzheimer’s disease. Acta Neuropathol. 125, 215–229. doi: 10.1007/s00401-012-1042-0
Lensjø, K. K., Lepperød, M. E., Dick, G., Hafting, T., and Fyhn, M. (2017). Removal of Perineuronal nets unlocks juvenile plasticity through network mechanisms of decreased inhibition and increased gamma activity. J. Neurosci. 37, 1269–1283. doi: 10.1523/JNEUROSCI.2504-16.2016
Lepelletier, F.-X., Mann, D. M. A., Robinson, A. C., Pinteaux, E., and Boutin, H. (2017). Early changes in extracellular matrix in Alzheimer’s disease. Neuropathol. Appl. Neurobiol. 43, 167–182. doi: 10.1111/nan.12295
Lesnikova, A., Casarotto, P. C., Fred, S. M., Voipio, M., Winkel, F., Steinzeig, A., et al. (2021). Chondroitinase and antidepressants promote plasticity by releasing TRKB from dephosphorylating control of PTPσ in parvalbumin neurons. J. Neurosci. 41, 972–980. doi: 10.1523/JNEUROSCI.2228-20.2020
Liao, R., Wu, H., Yi, Y., Wang, J.-X., Cai, X.-Y., He, H.-W., et al. (2013). Clinical significance and gene expression study of human hepatic stellate cells in HBV related-hepatocellular carcinoma. J. Exp. Clin. Cancer Res. 32:22. doi: 10.1186/1756-9966-32-22
Liao, F., Yoon, H., and Kim, J. (2017). Apolipoprotein E metabolism and functions in brain and its role in Alzheimer’s disease. Curr. Opin. Lipidol. 28, 60–67. doi: 10.1097/MOL.0000000000000383
Lin, R., Rosahl, T. W., Whiting, P. J., Fawcett, J. W., and Kwok, J. C. F. (2011). 6-Sulphated chondroitins have a positive influence on axonal regeneration. PLoS One 6:e21499. doi: 10.1371/journal.pone.0021499
Livingston, G., Huntley, J., Sommerlad, A., Ames, D., Ballard, C., Banerjee, S., et al. (2020). Dementia prevention, intervention, and care: 2020 report of the lancet commission. Lancet 396, 413–446. doi: 10.1016/S0140-6736(20)30367-6
Logsdon, A. F., Francis, K. L., Richardson, N. E., Hu, S. J., Faber, C. L., Phan, B. A., et al. (2022). Decoding perineuronal net glycan sulfation patterns in the Alzheimer’s disease brain. Alzheimers Dement. 18, 942–954. doi: 10.1002/alz.12451
Machlovi, S. I., Neuner, S. M., Hemmer, B. M., Khan, R., Liu, Y., Huang, M., et al. (2022). APOE4 confers transcriptomic and functional alterations to primary mouse microglia. Neurobiol. Dis. 164:105615. doi: 10.1016/j.nbd.2022.105615
Mafi, A. M., Hofer, L. N., Russ, M. G., Young, J. W., and Mellott, J. G. (2020). The density of Perineuronal nets increases with age in the inferior Colliculus in the Fischer Brown Norway rat. Front. Aging Neurosci. 12:27. doi: 10.3389/fnagi.2020.00027
Magarinos, A. M., and McEwen, B. S. (1995). Stress-induced atrophy of apical dendrites of hippocampal CA3c neurons: involvement of glucocorticoid secretion and excitatory amino acid receptors. Neuroscience 69, 89–98. doi: 10.1016/0306-4522(95)00259-L
Martín-de-Saavedra, M. D., Dos Santos, M., Culotta, L., Varea, O., Spielman, B. P., Parnell, E., et al. (2022). Shed CNTNAP2 ectodomain is detectable in CSF and regulates Ca2+ homeostasis and network synchrony via PMCA2/ATP2B2. Neuron 110, 627–643.e9. doi: 10.1016/j.neuron.2021.11.025
Masuda, A., Yasuoka, H., Satoh, T., Okazaki, Y., Yamaguchi, Y., and Kuwana, M. (2013). Versican is upregulated in circulating monocytes in patients with systemic sclerosis and amplifies a CCL2-mediated pathogenic loop. Arthritis Res. Ther. 15:R74. doi: 10.1186/ar4251
Matuszko, G., Curreli, S., Kaushik, R., Becker, A., and Dityatev, A. (2017). Extracellular matrix alterations in the ketamine model of schizophrenia. Neuroscience 350, 13–22. doi: 10.1016/j.neuroscience.2017.03.010
Mauney, S. A., Athanas, K. M., Pantazopoulos, H., Shaskan, N., Passeri, E., Berretta, S., et al. (2013). Developmental pattern of perineuronal nets in the human prefrontal cortex and their deficit in schizophrenia. Biol. Psychiatry 74, 427–435. doi: 10.1016/j.biopsych.2013.05.007
McLaughlin, K. J., Gomez, J., Baran, S. E., and Conrad, C. D. (2007). The effects of chronic stress on hippocampal morphology and function: an evaluation of chronic restraint paradigms. Brain Res. 1161, 56–64. doi: 10.1016/j.brainres.2007.05.042
Mira, R. G., Lira, M., and Cerpa, W. (2021). Traumatic brain injury: mechanisms of glial response. Front. Physiol. 12:740939. doi: 10.3389/fphys.2021.740939
Montagne, A., Nation, D. A., Sagare, A. P., Barisano, G., Sweeney, M. D., Chakhoyan, A., et al. (2020). APOE4 leads to blood-brain barrier dysfunction predicting cognitive decline. Nature 581, 71–76. doi: 10.1038/s41586-020-2247-3
Mroczko, B., Groblewska, M., Zboch, M., Kulczyńska, A., Koper, O. M., Szmitkowski, M., et al. (2014). Concentrations of matrix metalloproteinases and their tissue inhibitors in the cerebrospinal fluid of patients with Alzheimer’s disease. Journal of Alzheimer’s Disease: JAD 40, 351–357. doi: 10.3233/JAD-131634
Moradi, F., van den Berg, M., Mirjebreili, M., Kosten, L., Verhoye, M., Amiri, M., et al. (2022). Early electrophysiological aberrations in the Hippocampus of the TgF344-AD rat model as a potential biomarker for Alzheimer’s disease prognosis. Neuroscience doi:doi: 10.1101/2022.07.01.498373 [Preprint].
Morawski, M., Brückner, G., Jäger, C., Seeger, G., Matthews, R. T., and Arendt, T. (2012). Involvement of perineuronal and perisynaptic extracellular matrix in Alzheimer’s disease neuropathology. Brain Pathol. 22, 547–561. doi: 10.1111/j.1750-3639.2011.00557.x
Mukhopadhyay, S., Chatterjee, A., Tiwari, P., Ghai, U., and Vaidya, V. A. (2021). Postnatal fluoxetine treatment alters Perineuronal net formation and maintenance in the Hippocampus. Eneuro 8:ENEURO.0424-20.2021. doi: 10.1523/ENEURO.0424-20.2021
Murase, S., Lantz, C. L., and Quinlan, E. M. (2017). Light reintroduction after dark exposure reactivates plasticity in adults via perisynaptic activation of MMP-9. elife 6:e27345. doi: 10.7554/eLife.27345
Murty, D. V., Manikandan, K., Kumar, W. S., Ramesh, R. G., Purokayastha, S., Nagendra, B., et al. (2021). Stimulus-induced gamma rhythms are weaker in human elderly with mild cognitive impairment and Alzheimer’s disease. elife 10:e61666. doi: 10.7554/eLife.61666
Nedelec, T., Couvy-Duchesne, B., Monnet, F., Daly, T., Ansart, M., Gantzer, L., et al. (2022). Identifying health conditions associated with Alzheimer’s disease up to 15 years before diagnosis: an agnostic study of French and British health records. Lancet Digit. Health 4, e169–e178. doi: 10.1016/S2589-7500(21)00275-2
Nichols, E., Steinmetz, J. D., Vollset, S. E., Fukutaki, K., Chalek, J., Abd-Allah, F., et al. (2022). Estimation of the global prevalence of dementia in 2019 and forecasted prevalence in 2050: an analysis for the global burden of disease study 2019. Lancet Public Health 7, e105–e125. doi: 10.1016/S2468-2667(21)00249-8
Ohira, K., Hagihara, H., Miwa, M., Nakamura, K., and Miyakawa, T. (2019). Fluoxetine-induced dematuration of hippocampal neurons and adult cortical neurogenesis in the common marmoset. Mol. Brain 12:69. doi: 10.1186/s13041-019-0489-5
Ohira, K., Takeuchi, R., Iwanaga, T., and Miyakawa, T. (2013). Chronic fluoxetine treatment reduces parvalbumin expression and perineuronal nets in gamma-aminobutyric acidergic interneurons of the frontal cortex in adult mice. Mol. Brain 6:43. doi: 10.1186/1756-6606-6-43
Palu, E., and Liesi, P. (2002). Differential distribution of laminins in Alzheimer disease and normal human brain tissue. J. Neurosci. Res. 69, 243–256. doi: 10.1002/jnr.10292
Pantazopoulos, H., Gisabella, B., Rexrode, L., Benefield, D., Yildiz, E., Seltzer, P., et al. (2020). Circadian rhythms of perineuronal net composition. ENeuro 7:ENEURO.0034-19.2020. doi: 10.1523/ENEURO.0034-19.2020
Pantazopoulos, H., Lange, N., Baldessarini, R. J., and Berretta, S. (2007). Parvalbumin neurons in the entorhinal cortex of subjects diagnosed with bipolar disorder or schizophrenia. Biol. Psychiatry 61, 640–652. doi: 10.1016/j.biopsych.2006.04.026
Patel, R., Reiss, P., Shetty, H., Broadbent, M., Stewart, R., McGuire, P., et al. (2015). Do antidepressants increase the risk of mania and bipolar disorder in people with depression? A retrospective electronic case register cohort study. BMJ Open 5:e008341. doi: 10.1136/bmjopen-2015-008341
Pearson, C. S., Mencio, C. P., Barber, A. C., Martin, K. R., and Geller, H. M. (2018). Identification of a critical sulfation in chondroitin that inhibits axonal regeneration. elife 7:e37139. doi: 10.7554/eLife.37139
Platt, T. L., Beckett, T. L., Kohler, K., Niedowicz, D. M., and Murphy, M. P. (2016). Obesity, diabetes, and leptin resistance promote tau pathology in a mouse model of disease. Neuroscience 315, 162–174. doi: 10.1016/j.neuroscience.2015.12.011
Qiu, C., Kivipelto, M., and von Strauss, E. (2009). Epidemiology of Alzheimer’s disease: occurrence, determinants, and strategies toward intervention. Dialogues Clin. Neurosci. 11, 111–128. doi: 10.31887/DCNS.2009.11.2/cqiu
Rankin-Gee, E. K., McRae, P. A., Baranov, E., Rogers, S., Wandrey, L., and Porter, B. E. (2015). Perineuronal net degradation in epilepsy. Epilepsia 56, 1124–1133. doi: 10.1111/epi.13026
Reed, M. J., Damodarasamy, M., Pathan, J. L., Erickson, M. A., Banks, W. A., and Vernon, R. B. (2018). The effects of Normal aging on regional accumulation of hyaluronan and chondroitin sulfate proteoglycans in the mouse brain. J. Histochem. Cytochem. 66, 697–707. doi: 10.1369/0022155418774779
Reichelt, A. C., Gibson, G. D., Abbott, K. N., and Hare, D. J. (2019). A high-fat high-sugar diet in adolescent rats impairs social memory and alters chemical markers characteristic of atypical neuroplasticity and parvalbumin interneuron depletion in the medial prefrontal cortex. Food Funct. 10, 1985–1998. doi: 10.1039/C8FO02118J
Reichelt, A. C., Lemieux, C. A., Princz-Lebel, O., Singh, A., Bussey, T. J., and Saksida, L. M. (2021). Age-dependent and region-specific alteration of parvalbumin neurons, perineuronal nets and microglia in the mouse prefrontal cortex and hippocampus following obesogenic diet consumption. Sci. Rep. 11:5593. doi: 10.1038/s41598-021-85092-x
Riddell, D. R., Zhou, H., Atchison, K., Warwick, H. K., Atkinson, P. J., Jefferson, J., et al. (2008). Impact of apolipoprotein E (ApoE) polymorphism on brain ApoE levels. J. Neurosci. 28, 11445–11453. doi: 10.1523/JNEUROSCI.1972-08.2008
Riga, D., Kramvis, I., Koskinen, M. K., van Bokhoven, P., van der Harst, J. E., Heistek, T. S., et al. (2017). Hippocampal extracellular matrix alterations contribute to cognitive impairment associated with a chronic depressive-like state in rats. Sci. Transl. Med. 9:eaai8753. doi: 10.1126/scitranslmed.aai8753
Ringland, C., Schweig, J. E., Eisenbaum, M., Paris, D., Ait-Ghezala, G., Mullan, M., et al. (2021). MMP9 modulation improves specific neurobehavioral deficits in a mouse model of Alzheimer’s disease. BMC Neurosci. 22:39. doi: 10.1186/s12868-021-00643-2
Sadick, J. S., O’Dea, M. R., Hasel, P., Dykstra, T., Faustin, A., and Liddelow, S. A. (2022). Astrocytes and oligodendrocytes undergo subtype-specific transcriptional changes in Alzheimer’s disease. Neuron 110, 1788–1805.e10. doi: 10.1016/j.neuron.2022.03.008
Salas-Venegas, V., Flores-Torres, R. P., Rodríguez-Cortés, Y. M., Rodríguez-Retana, D., Ramírez-Carreto, R. J., Concepción-Carrillo, L. E., et al. (2022). The obese brain: mechanisms of systemic and local inflammation, and interventions to reverse the cognitive deficit. Front. Integr. Neurosci. 16:798995. doi: 10.3389/fnint.2022.798995
Scarlett, J. M., Hu, S. J., and Alonge, K. M. (2022). The loss of perineuronal nets in Alzheimer’s disease: missing or hiding in plain sight? Front. Integr. Neurosci. 16:896400. doi: 10.3389/fnint.2022.896400
Schachtrup, C., Le Moan, N., Passino, M. A., and Akassoglou, K. (2011). Hepatic stellate cells and astrocytes: stars of scar formation and tissue repair. Cell Cycle 10, 1764–1771. doi: 10.4161/cc.10.11.15828
Sepulveda, J., Luo, N., Nelson, M., Ng, C. A. S., and Rebeck, G. W. (2022). Independent APOE4 knock-in mouse models display reduced brain APOE protein, altered neuroinflammation, and simplification of dendritic spines. J. Neurochem. 163, 247–259. doi: 10.1111/jnc.15665
Shang, Y., Widman, L., and Hagström, H. (2022). Nonalcoholic fatty liver disease and risk of dementia: a population-based cohort study. Neurology 99, e574–e582. doi: 10.1212/WNL.0000000000200853
Shi, Y., Manis, M., Long, J., Wang, K., Sullivan, P. M., Remolina Serrano, J., et al. (2019). Microglia drive APOE-dependent neurodegeneration in a tauopathy mouse model. J. Exp. Med. 216, 2546–2561. doi: 10.1084/jem.20190980
Shimizu, H., Ghazizadeh, M., Sato, S., Oguro, T., and Kawanami, O. (2009). Interaction between β-amyloid protein and heparan sulfate proteoglycans from the cerebral capillary basement membrane in Alzheimer’s disease. J. Clin. Neurosci. 16, 277–282. doi: 10.1016/j.jocn.2008.04.009
Sikora, E., Bielak-Zmijewska, A., Dudkowska, M., Krzystyniak, A., Mosieniak, G., Wesierska, M., et al. (2021). Cellular senescence in brain aging. Front. Aging Neurosci. 13:646924. doi: 10.3389/fnagi.2021.646924
Slaker, M., Churchill, L., Todd, R. P., Blacktop, J. M., Zuloaga, D. G., Raber, J., et al. (2015). Removal of perineuronal nets in the medial prefrontal cortex impairs the acquisition and reconsolidation of a cocaine-induced conditioned place preference memory. J. Neurosci. 35, 4190–4202. doi: 10.1523/JNEUROSCI.3592-14.2015
Slomski, A. (2022). Obesity is now the top modifiable dementia risk factor in the US. JAMA 328:10. doi: 10.1001/jama.2022.10273
Stoiljkovic, M., Kelley, C., Stutz, B., Horvath, T. L., and Hajós, M. (2019). Altered cortical and hippocampal excitability in TgF344-AD rats modeling Alzheimer’s disease pathology. Cerebral Cortex 29, 2716–2727. doi: 10.1093/cercor/bhy140
Sun, Y., Xu, S., Jiang, M., Liu, X., Yang, L., Bai, Z., et al. (2021). Role of the extracellular matrix in Alzheimer’s disease. Front. Aging Neurosci. 13:707466. doi: 10.3389/fnagi.2021.707466
Szklarczyk, A., Lapinska, J., Rylski, M., McKay, R. D. G., and Kaczmarek, L. (2002). Matrix Metalloproteinase-9 undergoes expression and activation during dendritic remodeling in adult hippocampus. J. Neurosci. 22, 920–930. doi: 10.1523/JNEUROSCI.22-03-00920.2002
Takeda, S., Sato, N., Uchio-Yamada, K., Sawada, K., Kunieda, T., Takeuchi, D., et al. (2010). Diabetes-accelerated memory dysfunction via cerebrovascular inflammation and Aβ deposition in an Alzheimer mouse model with diabetes. Proc. Natl. Acad. Sci. U. S. A. 107, 7036–7041. doi: 10.1073/pnas.1000645107
Tan, Z., Garduño, B. M., Aburto, P. F., Chen, L., Ha, N., Cogram, P., et al. (2022). Cognitively impaired aged Octodon degus recapitulate major neuropathological features of sporadic Alzheimer’s disease. Acta Neuropathol. Commun. 10:182. doi: 10.1186/s40478-022-01481-x
Tcw, J., Qian, L., Pipalia, N. H., Chao, M. J., Liang, S. A., Shi, Y., et al. (2022). Cholesterol and matrisome pathways dysregulated in astrocytes and microglia. Cells 185, 2213–2233.e25. doi: 10.1016/j.cell.2022.05.017
Tewari, B. P., Chaunsali, L., Campbell, S. L., Patel, D. C., Goode, A. E., and Sontheimer, H. (2018). Perineuronal nets decrease membrane capacitance of peritumoral fast spiking interneurons in a model of epilepsy. Nat. Commun. 9:4724. doi: 10.1038/s41467-018-07113-0
Tewari, B., Woo, A., Prim, C., Chaunsali, L., Kimbrough, I., Engel, K., et al. (2023). Perineuronal nets support astrocytic ion and glutamate homeostasis at tripartite synapses. Review doi:doi: 10.21203/rs.3.rs-2501039/v1 [Preprint].
Tian, L., Stefanidakis, M., Ning, L., Van Lint, P., Nyman-Huttunen, H., Libert, C., et al. (2007). Activation of NMDA receptors promotes dendritic spine development through MMP-mediated ICAM-5 cleavage. The Journal of Cell Biology 178, 687–700. doi: 10.1083/jcb.200612097
Tominaga, K., and Suzuki, H. I. (2019). TGF-β signaling in cellular senescence and aging-related pathology. Int. J. Mol. Sci. 20:5002. doi: 10.3390/ijms20205002
Traikapi, A., and Konstantinou, N. (2021). Gamma oscillations in Alzheimer’s disease and their potential therapeutic role. Front. Syst. Neurosci. 15:782399. doi: 10.3389/fnsys.2021.782399
Tsuji, K., Aoki, T., Tejima, E., Arai, K., Lee, S.-R., Atochin, D. N., et al. (2005). Tissue plasminogen activator promotes matrix metalloproteinase-9 upregulation after focal cerebral ischemia. Stroke 36, 1954–1959. doi: 10.1161/01.STR.0000177517.01203.eb
Uesaka, S., Miyazaki, K., and Ito, H. (2004). Age-related changes and sex differences in chondroitin sulfate isomers and hyaluronic acid in normal synovial fluid. Mod. Rheumatol. 14, 470–475. doi: 10.1007/s10165-004-0351-0
Ulbrich, P., Khoshneviszadeh, M., Jandke, S., Schreiber, S., and Dityatev, A. (2021). Interplay between perivascular and perineuronal extracellular matrix remodelling in neurological and psychiatric diseases. Eur. J. Neurosci. 53, 3811–3830. doi: 10.1111/ejn.14887
Vargas-Soria, M., Ramos-Rodriguez, J. J., del Marco, A., Hierro-Bujalance, C., Carranza-Naval, M. J., Calvo-Rodriguez, M., et al. (2022). Accelerated amyloid angiopathy and related vascular alterations in a mixed murine model of Alzheimer’s disease and type two diabetes. Fluids Barriers CNS 19:88. doi: 10.1186/s12987-022-00380-6
Végh, M. J., Heldring, C. M., Kamphuis, W., Hijazi, S., Timmerman, A. J., Li, K. W., et al. (2014a). Reducing hippocampal extracellular matrix reverses early memory deficits in a mouse model of Alzheimer’s disease. Acta Neuropathol. Commun. 2:76. doi: 10.1186/s40478-014-0076-z
Végh, M. J., Rausell, A., Loos, M., Heldring, C. M., Jurkowski, W., van Nierop, P., et al. (2014b). Hippocampal extracellular matrix levels and stochasticity in synaptic protein expression increase with age and are associated with age-dependent cognitive decline. Mol. Cell Proteomics 13, 2975–2985. doi: 10.1074/mcp.M113.032086
Venturino, A., Schulz, R., De Jesús-Cortés, H., Maes, M. E., Nagy, B., Reilly-Andújar, F., et al. (2021). Microglia enable mature perineuronal nets disassembly upon anesthetic ketamine exposure or 60-Hz light entrainment in the healthy brain. Cell Rep. 36:109313. doi: 10.1016/j.celrep.2021.109313
Vervuurt, M., de Kort, A. M., Jäkel, L., Kersten, I., Abdo, W. F., Schreuder, F. H. B. M., et al. (2023). Decreased ratios of matrix metalloproteinases to tissue-type inhibitors in cerebrospinal fluid in sporadic and hereditary cerebral amyloid angiopathy. Alzheimers Res. Ther. 15:26. doi: 10.1186/s13195-023-01171-3
Watanabe, Y., Gould, E., and McEwen, B. S. (1992). Stress induces atrophy of apical dendrites of hippocampal CA3 pyramidal neurons. Brain Res. 588, 341–345. doi: 10.1016/0006-8993(92)91597-8
Weekman, E. M., and Wilcock, D. M. (2016). Matrix Metalloproteinase in Blood-Brain Barrier Breakdown in Dementia. Journal of Alzheimer’s Disease: JAD 49, 893–903. doi: 10.3233/JAD-150759
Weinstein, G., O’Donnell, A., Davis-Plourde, K., Zelber-Sagi, S., Ghosh, S., DeCarli, C. S., et al. (2022). Non-alcoholic fatty liver disease, liver fibrosis, and regional amyloid-β and tau pathology in middle-aged adults: the Framingham study. J. Alzheimer's Dis. 86, 1371–1383. doi: 10.3233/JAD-215409
Withington, C. G., and Turner, R. S. (2022). Amyloid-related imaging abnormalities with anti-amyloid antibodies for the treatment of dementia due to Alzheimer’s disease. Front. Neurol. 13:862369. doi: 10.3389/fneur.2022.862369
Yamazaki, Y., Shinohara, M., Yamazaki, A., Ren, Y., Asmann, Y. W., Kanekiyo, T., et al. (2020). ApoE (Apolipoprotein E) in brain pericytes regulates endothelial function in an isoform-dependent manner by modulating basement membrane components. Arterioscler. Thromb. Vasc. Biol. 40, 128–144. doi: 10.1161/ATVBAHA.119.313169
Yan, J., Zhang, H., Yin, Y., Li, J., Tang, Y., Purkayastha, S., et al. (2014). Obesity-and aging-induced excess of central transforming growth factor-β potentiates diabetic development via an RNA stress response. Nat. Med. 20:Article 9. doi: 10.1038/nm.3616
Yang, S., Cacquevel, M., Saksida, L. M., Bussey, T. J., Schneider, B. L., Aebischer, P., et al. (2015). Perineuronal net digestion with chondroitinase restores memory in mice with tau pathology. Exp. Neurol. 265, 48–58. doi: 10.1016/j.expneurol.2014.11.013
Yang, S., Gigout, S., Molinaro, A., Naito-Matsui, Y., Hilton, S., Foscarin, S., et al. (2021). Chondroitin 6-sulphate is required for neuroplasticity and memory in ageing. Mol. Psychiatry 26, 5658–5668. doi: 10.1038/s41380-021-01208-9
Zhao, Y., Lyons, C. E. Jr., Xiao, A., Templeton, D. J., Sang, Q. A., Brew, K., et al. (2008). Urokinase directly activates matrix metalloproteinases-9: a potential role in glioblastoma invasion. Biochem. Biophys. Res. Commun. 369, 1215–1220. doi: 10.1016/j.bbrc.2008.03.038
Keywords: extracellular matrix, perineuronal net (PNNs), Alzheiemer’s disease, chondroitin sulfate proteoglycan (CSPGs), Matrix metalloproteinases (MMPs), major Depressive Disorder (MDD), Aging, APOE
Citation: Amontree M, Deasy S, Turner RS and Conant K (2023) Matrix disequilibrium in Alzheimer’s disease and conditions that increase Alzheimer’s disease risk. Front. Neurosci. 17:1188065. doi: 10.3389/fnins.2023.1188065
Received: 16 March 2023; Accepted: 20 April 2023;
Published: 26 May 2023.
Edited by:
Wendy Noble, University of Exeter, United KingdomReviewed by:
Harry Pantazopoulos, University of Mississippi Medical Center, United StatesCopyright © 2023 Amontree, Deasy, Turner and Conant. This is an open-access article distributed under the terms of the Creative Commons Attribution License (CC BY). The use, distribution or reproduction in other forums is permitted, provided the original author(s) and the copyright owner(s) are credited and that the original publication in this journal is cited, in accordance with accepted academic practice. No use, distribution or reproduction is permitted which does not comply with these terms.
*Correspondence: Katherine Conant, a2VjODRAZ2VvcmdldG93bi5lZHU=
Disclaimer: All claims expressed in this article are solely those of the authors and do not necessarily represent those of their affiliated organizations, or those of the publisher, the editors and the reviewers. Any product that may be evaluated in this article or claim that may be made by its manufacturer is not guaranteed or endorsed by the publisher.
Research integrity at Frontiers
Learn more about the work of our research integrity team to safeguard the quality of each article we publish.