- 1Laboratoire d’Imagerie Biomédicale Multimodale (BioMaps), CEA, CNRS, Inserm, Service Hospitalier Frédéric Joliot, Université Paris-Saclay, Orsay, France
- 2Department of Toxicology and Chemical Risks, Armed Forces Biomedical Research Institute, Bretigny sur Orge, France
Aim: Buprenorphine mainly acts as an agonist of mu-opioid receptors (mu-OR). High dose buprenorphine does not cause respiratory depression and can be safely administered to elicit typical opioid effects and explore pharmacodynamics. Acute buprenorphine, associated with functional and quantitative neuroimaging, may therefore provide a fully translational pharmacological challenge to explore the variability of response to opioids in vivo. We hypothesized that the CNS effects of acute buprenorphine could be monitored through changes in regional brain glucose metabolism, assessed using 18F-FDG microPET in rats.
Materials and methods: First, level of receptor occupancy associated with a single dose of buprenorphine (0.1 mg/kg, s.c) was investigated through blocking experiments using 11C-buprenorphine PET imaging. Behavioral study using the elevated plus-maze test (EPM) was performed to assess the impact of the selected dose on anxiety and also locomotor activity. Then, brain PET imaging using 18F-FDG was performed 30 min after injection of unlabeled buprenorphine (0.1 mg/kg, s.c) vs. saline. Two different 18F-FDG PET acquisition paradigms were compared: (i) 18F-FDG injected i.v. under anesthesia and (ii) 18F-FDG injected i.p. in awake animals to limit the impact of anesthesia.
Results: The selected dose of buprenorphine fully blocked the binding of 11C-buprenorphine in brain regions, suggesting complete receptor occupancy. This dose had no significant impact on behavioral tests used, regardless of the anesthetized/awake handling paradigm. In anesthetized rats, injection of unlabeled buprenorphine decreased the brain uptake of 18F-FDG in most brain regions except in the cerebellum which could be used as a normalization region. Buprenorphine treatment significantly decreased the normalized brain uptake of 18F-FDG in the thalamus, striatum and midbrain (p < 0.05), where binding of 11C-buprenorphine was the highest. The awake paradigm did not improve sensitivity and impact of buprenorphine on brain glucose metabolism could not be reliably estimated.
Conclusion: Buprenorphine (0.1 mg/kg, s.c) combined with 18F-FDG brain PET in isoflurane anesthetized rats provides a simple pharmacological imaging challenge to investigate the CNS effects of full receptor occupancy by this partial mu-OR agonist. Sensitivity of the method was not improved in awake animals. This strategy may be useful to investigate de desensitization of mu-OR associated with opioid tolerance in vivo.
1. Introduction
Strong mu-opioid receptor (MOR) agonists form the most powerful class of analgesic drugs. Their clinical management is however difficult due to several side effects and to significant risk for respiratory depression and overdose (Trescot et al., 2008). Prolonged use of opioids is associated with tolerance and addictive properties which may lead to misuse and drug abuse as exemplified by the current opioid crisis (Volkow and Collins, 2017; Volkow and Blanco, 2021).
There is a crucial need for in vivo methods to explore the molecular determinants of opioid response, tolerance and dependence in animals and humans (Morgan and Christie, 2011). In neuropharmacology, pharmacological challenges are essential to explore the pharmacokinetics (PK) and pharmacodynamics (PD) of opioids in animals and humans. However, management of pharmacological challenges using strong opioid may be poorly tolerated and appear risky given the high variability in terms of side effects experienced, which is difficult to predict, especially in healthy volunteers (Drewes et al., 2013).
Among strong opioid drugs, buprenorphine (BUP) benefits from a unique safety profile (Wakeman and Barnett, 2018). In vitro, buprenorphine is a high affinity agonist of MOR but is also described as antagonist of κ-OR, δ-OR, and agonist of nociceptin/ORL-1 receptors (Lutfy and Cowan, 2004; Cami-Kobeci et al., 2011). In vivo, buprenorphine mainly acts as a high-affinity and high-potency partial agonist (i.e., intermediate intrinsic efficacy) of MOR, although affinity for other opioid receptor subtypes may account for its neuropharmacological profile (Infantino et al., 2021). Low-dose buprenorphine offers potent analgesia for the treatment of moderate to severe pain in patients (Lutfy and Cowan, 2004). High dose buprenorphine, which is associated with almost full MOR occupancy in humans (Greenwald et al., 2003), does not cause respiratory depression and can be safely administered for maintenance therapy in patients with opioid use disorder (Helm et al., 2008; Wakeman and Barnett, 2018). A “ceiling” analgesic dose–response has been described in animals, suggesting limited efficacy of buprenorphine at stimulating MOR, which refers to a partial agonist profile. However, in humans, buprenorphine displays ceiling in respiratory effect but none in analgesic effect, thus behaving as a full agonist (Dahan et al., 2006). Because of buprenorphine’s high MOR affinity, its acute effects may drastically depend on the baseline pharmacologic conditions and individual differences in MOR availability and signaling (Greenwald et al., 2022). Single dose buprenorphine can therefore be considered a safe pharmacological challenge to safely explore individual differences in the effects of high level of MOR occupancy in animals and humans.
Conventional PK/PD studies offer limited insight into the impact of opioids at the CNS level, which is essential to understand the brain mechanisms involved in their neuropharmacology (Ing Lorenzini et al., 2012). In this framework, functional neuroimaging techniques such as positron emission tomography (PET) or functional magnetic resonance imaging (fMRI) offer minimally-invasive methods to investigate the impact of opioids on brain function in animals and humans (Cumming et al., 2019; Moningka et al., 2019). PET imaging using the MOR-agonist 11C-carfentanyl has been extensively used to estimate the target engagement associated with clinical doses of MOR ligands including buprenorphine (Cumming et al., 2019). Recent preclinical studies using carbon-11 radiolabeled buprenorphine (11C-buprenorphine) in rats and non-human primates have been reported as a method to simultaneously explore the neuropharmacokinetics of buprenorphine and its specific neuroreceptor binding, mainly at MORs (Auvity et al., 2021). Pharmacological functional MRI (phMRI) has been validated in animals and humans to describe the effects of buprenorphine on brain function (Upadhyay et al., 2011; Becerra et al., 2013; Seah et al., 2014). However, this preclinical work raised important questions regarding the impact of anesthesia which abolished the significance of phMRI measurements in response to buprenorphine (Seah et al., 2014).
In the present study, we hypothesized that the CNS effects of full receptor occupancy by buprenorphine could be safely estimated through changes in brain glucose metabolism in rats, assessed using 18F-2-fluoro-2-deoxy-D-glucose (18F-FDG) PET imaging. First, a dose of buprenorphine with minimal effects on animal behavior and full receptor occupancy was validated. Then, 18F-FDG PET imaging was performed in anesthetized animals or using an alternative acquisition protocol which aimed at limiting the impact of anesthesia on the outcome of brain PET data.
2. Materials and methods
2.1. Chemicals, radiochemicals
Buprenorphine hydrochloride for injection (0.1 mg/mL) was obtained from Coveto (France). 18F-2-fluoro-2-deoxy-D-glucose (18F-FDG) for injection was obtained from Cyclopharma (France). Isoflurane was obtained from Abbvie (Rungis, France). 11C-Buprenorphine was synthesized in-house from 3-O-triyl-6-O-desmethylbuprenorphine (ABX, Germany) in two steps using a C-11 Pro-2 module (iPhase Technologie, Australia). Ready-to-inject 11C-buprenorphine (1.8–3.5 GBq, decay-corrected radiochemical yield:15%) was obtained with a radiochemical purity above 98% and a mean molar activity of 93 ± 10 GBq/μmol EOB (End of bombarding).
2.2. Animals
Thirty-two male Sprague–Dawley rats were used for this study. Eighteen rats were used for PET imaging experiments. Different 14 rats were used for behavioral experiments. Rats were housed under standard experimental conditions: room temperature (20 ± 2°C); light/dark cycle (14 h light/10 h dark); water and food ad libitum and kept in social groups of two rats per cage. An acclimation period of 7 days was respected before any experiment. All procedures were in accordance with European directives on the protection and use of laboratory animals (Council Directive 2010/63/UE, French decree 2013–118). The experimental protocol was evaluated and validated by a local ethic committee for animal use and approved by the French government (n° APAFIS#34694–2,022,011,718,132,961 v1). Anaesthesia was induced and maintained using isoflurane 2–2.5% in O2.
2.3. MicroPET acquisition
Brain microPET acquisitions were performed in anesthetized rats using an Inveon, microPET (Siemens Healthcare, Knoxville, TN, United States, spatial resolution 1.6 mm).
2.4. 11C-Buprenorphine PET study
First, level of receptor occupancy associated with the selected dose of buprenorphine (0.1 mg/kg, s.c) was investigated using 11C-buprenorphine PET imaging in anesthetized rats. Rats were s.c injected with unlabeled buprenorphine (0.1 mg/kg (n = 3)), 10 mg/kg (n = 2) or vehicle (Saline vehicle, n = 3). After 30 min, 11C-buprenorphine was i.v injected followed by dynamic PET acquisition.
2.5. 18F-FDG PET study
2.5.1. Anesthetized rat paradigm
First, awake rats received either s.c injection of buprenorphine (0.1 mg/kg s.c., n = 5) or vehicle (n = 5). After 25 min, anaesthesia was induced using isoflurane 2–2.5% in O2 and a catheter was inserted in the caudal vein. At 30 min, 18F-FDG was i.v injected (37.62 ± 2.3 MBq), followed by 30 min static PET acquisition starting after 20 min of 18F-FDG uptake (20–50 min).
2.5.2. Awake rat paradigm
A customized PET protocol was used to limit the impact of anaesthesia on the estimation of brain function using 18F-FDG PET (Schiffer et al., 2007). This protocol takes advantage of the irreversible uptake of 18F-FDG by the brain. Thirty min after s.c injection of buprenorphine (0.1 mg/kg s.c. n = 4) or vehicle (n = 4) in awake animals, 18F-FDG (1 ml; mean dose = 33.6 ± 4.1 MBq) was injected intraperitoneally (i.p), in awake rats. Then, rats were then transferred in a cage to allow for the brain uptake of 18F-FDG in awake and freely moving animals. It takes 30 min for i.p administered 18F-FDG to reach the plateau brain uptake of i.v administered 18F-FDG (Schiffer et al., 2007). Therefore, after 35 min uptake, anaesthesia was induced before transferring rat to the microPET scanner for a 30 min static PET acquisition (40–70 min).
2.5.3. PET data reconstruction and analysis
PET images were reconstructed by the 2D OSEM/FORE algorithm and corrected for attenuation, random coincidences and scatter. The voxel size was 0.2 × 0.2 × 0.2 mm3. Brain PET images were corrected for radioactive decay, injected dose and body weight and was expressed as standardized uptake values (SUV), with SUV = tissue activity (kBq/cc)/[injected dose (kBq)/body weight (g)]. SUV-normalized PET images were spatially co-registered to a standard rat 18F-FDG PET template using Pmod software (version 4.2, PMOD Technologies Ltd., Zurich, Switzerland). Quantitative SUV values were determined through a volume-of-interest (VOI) analysis in selected brain regions which size is relevant to the spatial resolution of the microPET scanner (~1.6 mm) which included thalamus, cerebellum, striatum, hippocampus, hypothalamus, midbrain, septum, ventral tegmental area (VTA), pons and cortex.
For dynamic 11C-buprenorphine PET images, SUV-normalized time-activity curves were obtained in selected brain regions. Summed PET images from 40–60 min were generated for regional analysis. Regional SUV values were obtained from static 18F-FDG PET images.
For both the 18F-FDG and the 11C-buprenorphine PET images, regional SUV values were then divided by respective SUV values in the cerebellum to obtain SUVR.
2.6. Anxiety and locomotor activity
We hypothesized that animal handling to perform i.p injection of 18F-FDG in awake rat may impact animal behavior with consequences for the pharmacological imaging challenge. The impact of animal handling and/or pre-treatment by unlabeled buprenorphine on animal anxiety and activity was investigated using the elevated plus-maze (EPM) test (Etaee et al., 2017). Rats were assigned to 4 groups (n = 7 in per group) which recapitulated buprenorphine treatment and the type of 18F-FDG injection. Rats used to simulate the awake paradigm did not require anaesthesia and the EPM was performed 40 min after i.p injection of 0.5 mL saline. Animals used to simulate the anesthetized paradigm underwent the EPM test 20 min after the end of anaesthesia during which a venous catheter was inserted and 1 mL saline was injected. After treatment by buprenorphine 0.1 mg/kg or vehicle (saline), each rat was installed at the center of the maze and allowed to explore all arms freely. Behavior was controlled for 5 min over the maze using a dedicated digital camera. The maze was cleaned after each experiment in each animal. The time spent in the open arms, as well as the total distance covered by the rat were determined.
2.7. Statistical analysis
A two-way ANOVA with Turkey’s post hoc test was performed to compare SUV or SUVR values between groups for each brain regions. Statistical analysis was performed using GraphPad software (version 9.0).
3. Results
3.1. 11C-Buprenorphine PET study
In control rats, s.c injected with saline 30 min before PET, brain distribution of microdose 11C-buprenorphine was consistent with previous report (Auvity et al., 2021; Vodovar et al., 2022). SUV-normalized PET images showed higher uptake in subcortical brain regions compared with the cortex (Figure 1). Significant uptake by these regions contrasted with the very low uptake of 11C-buprenorphine by the cerebellum (Figures 2, 3). Time-activity curves obtained in the different brain regions showed a relatively slow uptake of 11C-buprenorphine, which peaked at ~7 min, followed slow washout of brain radioactivity (Figure 2A). The lowest uptake was found in the cerebellum which is assumed to be devoid of MOR in rats (Tempel and Zukin, 1987) (Figures 2, 3). Pseudo-equilibrium of concentration of 11C-buprenorphine in brain regions relative to the cerebellum was almost reached at ~40 min (Figure 2B). Summed PET images (40–60 min) were therefore used for further analyses as a compromise between pseudo-equilibrium and the short radioactive half-life of carbon-11 (20.4 min), to allow for quantitative determination of the PET signal in brain regions, including the cerebellum. Highest SUV values were found in the hypothalamus, midbrain, VTA and thalamus.
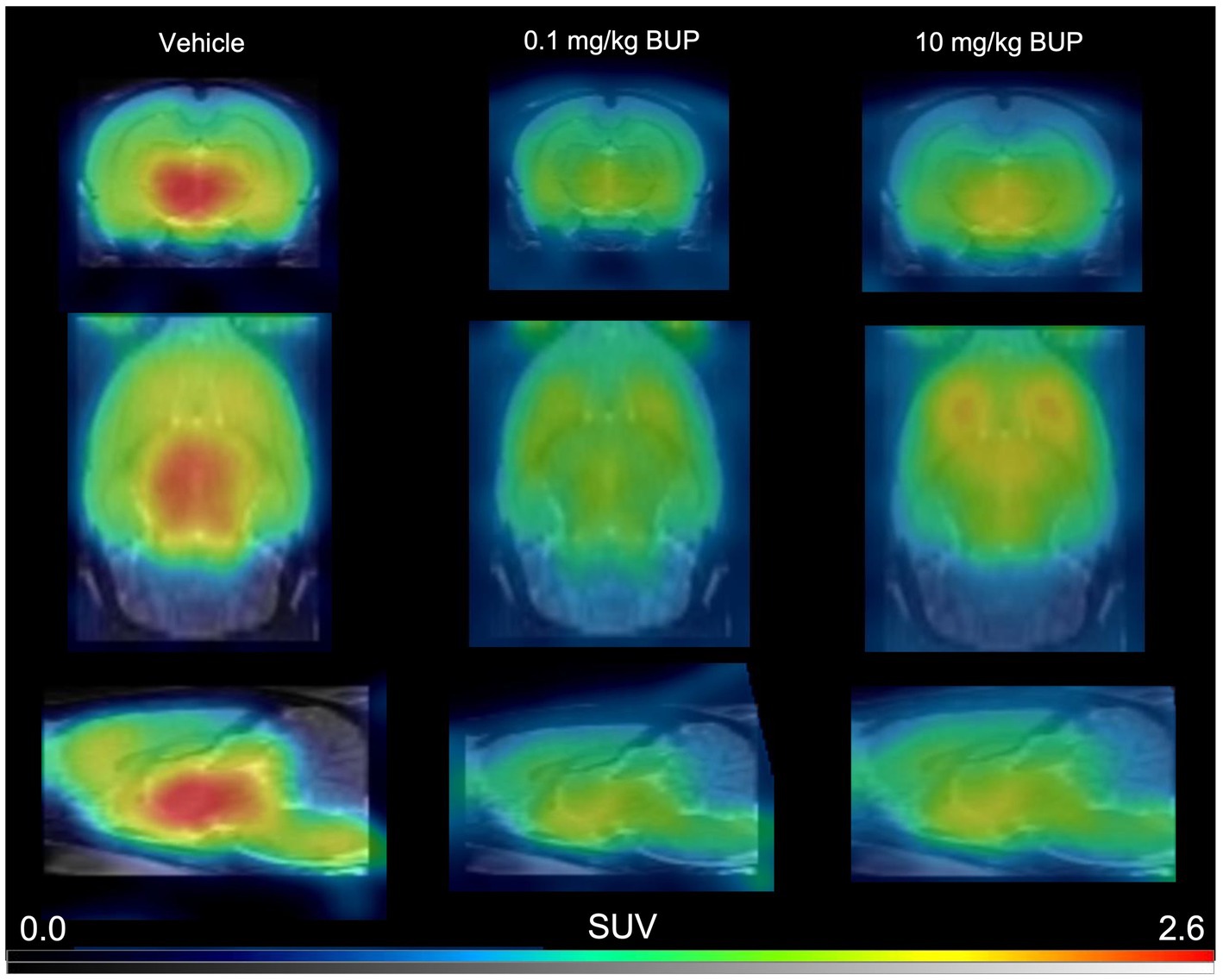
Figure 1. Representative 11C-buprenorphine PET images. These are summed PET images (40–60 min) obtained in the rat brain without (vehicle) or after pharmacological blocking using unlabelled buprenorphine (BUP) 0.1 mg/kg or 10 mg/kg injected s.c 30 min before 11C-buprenorphine.
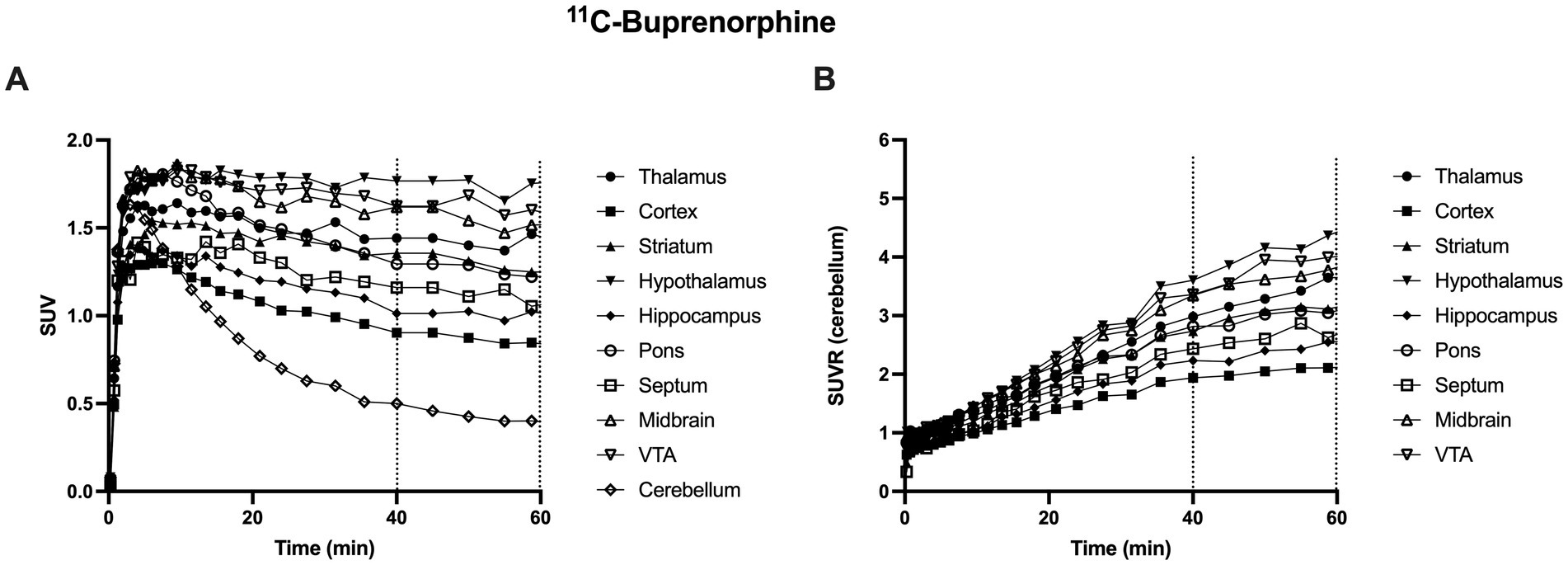
Figure 2. Representative brain kinetics of 11C-buprenorphine in selected brain regions in rats. In A, data are expressed as standardized uptake value (SUV) versus time (min). Corresponding data, normalized by SUV values in the cerebellum (SUVR) are shown in B. Dashed lines show the time frame used for 11C-buprenorphine PET data analysis (from 40 to 60 min) VTA: ventral tegmental area.
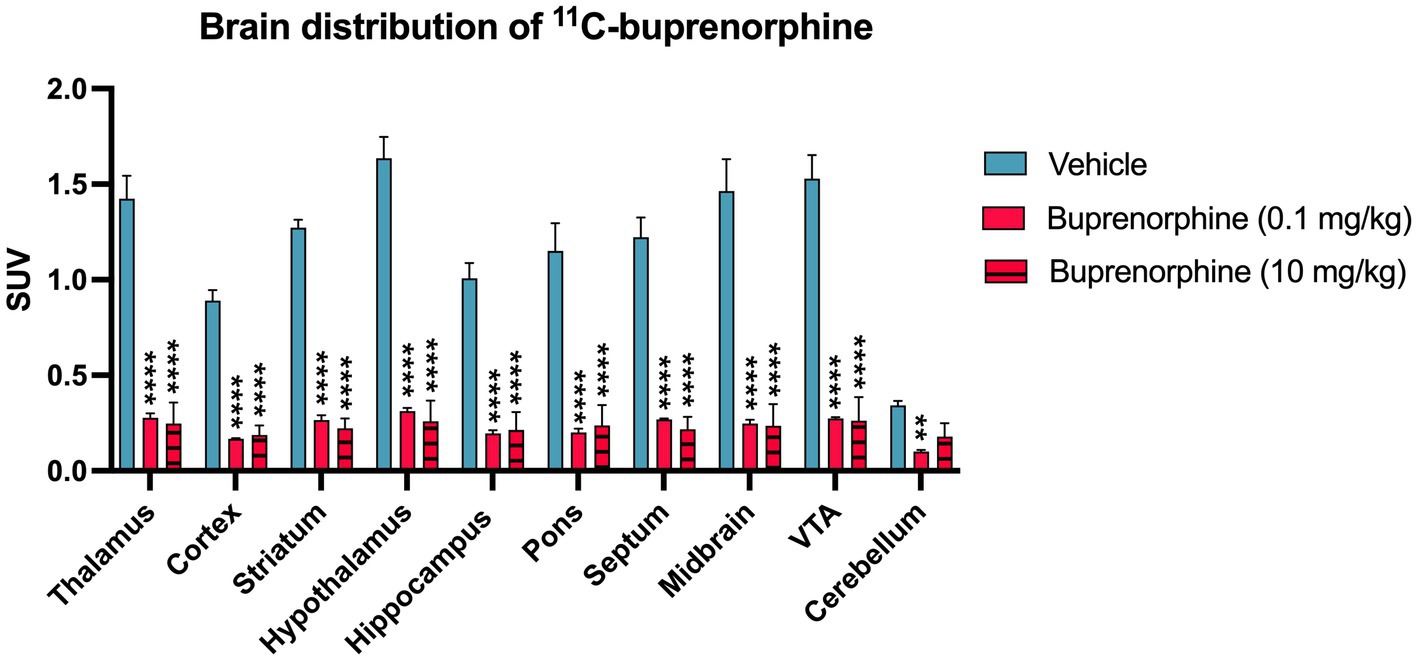
Figure 3. Impact of pharmacological blocking using unlabelled buprenorphine on the regional brain uptake of 11C-buprenorphine uptake of 11C-buprenorphine. Data are expressed as standardized uptake value (SUV) and reported as mean ± S.D. obtained from summed (40–60 min) PET images obtained in the rat brain without (vehicle) or after pharmacological blocking using unlabelled buprenorphine (BUP) 0.1 mg/kg or 10 mg/kg injected s.c 30 min before 11C-buprenorphine. VTA: ventral tegmental area. Statistical significance was set at p < 0.05 with **p < 0.01 and ****p < 0.0001.
Blocking experiment by unlabeled buprenorphine (0.1 mg/kg) visually decreased the brain PET signal (Figure 1). Blocking significantly decreased the binding of 11C-buprenorphine in all selected brain regions including, in a lower extent, in the cerebellum. Higher dose of unlabeled buprenorphine (10 mg/kg) did not further block the binding of 11C-buprenorphine in any brain region, thus suggesting full receptor occupancy by buprenorphine 0.1 mg/kg (Figure 3).
3.2. 18F-FDG PET study
The impact of full receptor occupancy by buprenorphine 0.1 mg/kg on brain glucose metabolism was then investigated using 18F-FDG PET (Figure 4). In anesthetized rats, SUV-normalized 18F-FDG PET images revealed a lower 18F-FDG uptake in buprenorphine-treated animals compared with control (saline) rats. The decrease in 18F-FDG uptake was significant in all brain regions (p < 0.01 to <0.0001) except in the cerebellum (p > 0.05, Figure 4A). The highest level of significance for the impact of buprenorphine on 18F-FDG uptake was observed in the thalamus, striatum and midbrain (Figure 4A). Cerebellum was then used as a normalization region to generate regional SUVRs which confirmed the trend in reduced relative 18F-FDG uptake which was significant in the thalamus and striatum (p < 0.05) (Figure 4B).
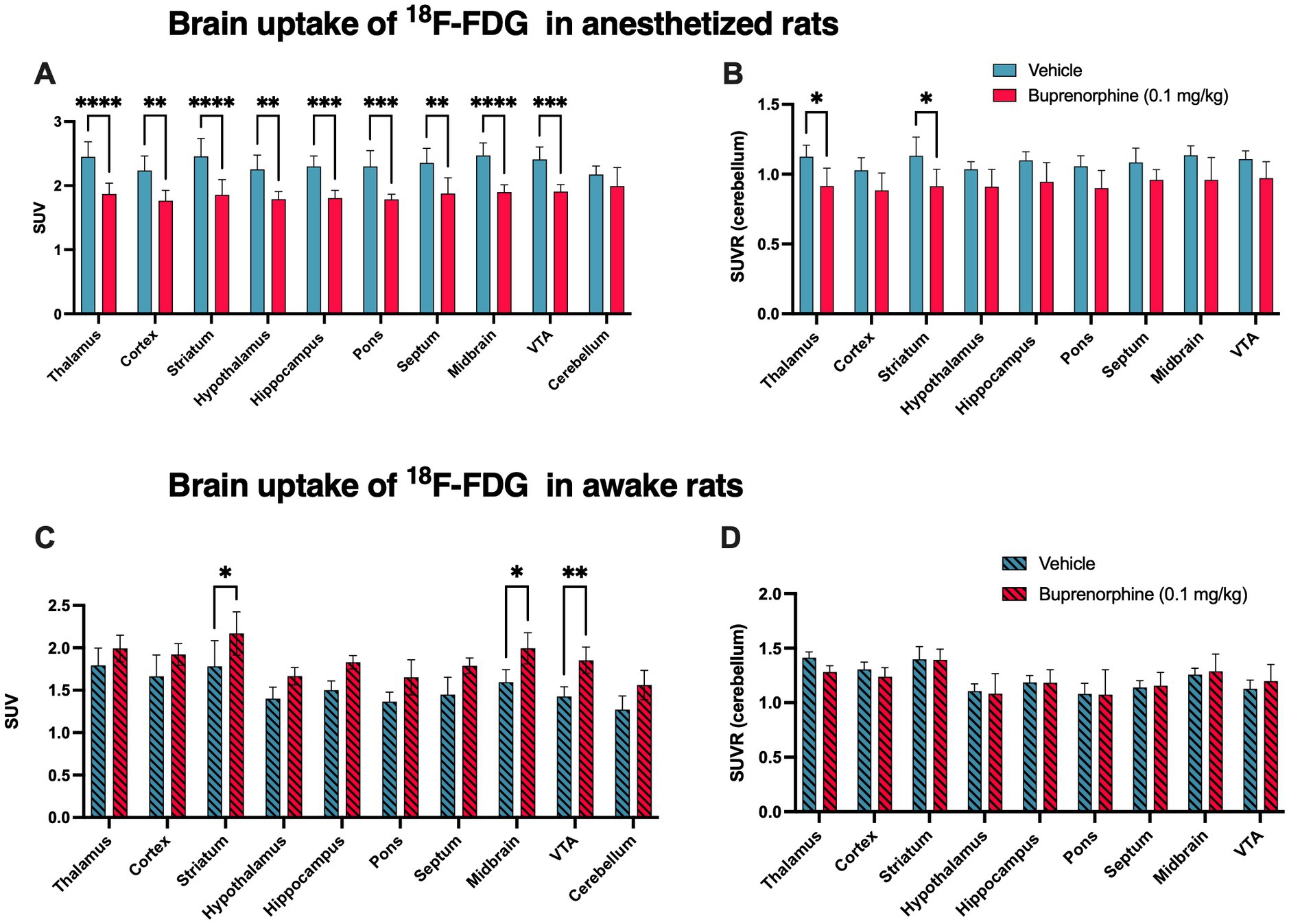
Figure 4. Impact of the buprenorphine challenge on brain function estimated using 18F-FDG PET imaging in rats. The uptake of 18F-FDG Data in selected brain regions is expressed as standardized uptake value (SUV) and reported as mean ± S.D. (n = 4–5 per group). Brain 18F-FDG PET data were obtained without (vehicle) or after pharmacological challenge using unlabelled buprenorphine 0.1 mg/kg in anesthetized (in A) or in awake animals (in C). SUVR corresponds to the regional uptake of 18F-FDG in brain region divided by the cerebellar uptake obtained in anesthetized (in B) or in awake animals (in D). VTA: ventral tegmental area. Statistical significance was set at *p < 0.05 with **p < 0.01, ***p < 0.001 and ****p < 0.0001.
In awake animals, the SUV-normalized uptake of 18F-FDG tended to be higher in BUP treated rats compared with vehicle-treated rats, with a similar impact across brain regions, including the cerebellum. Increase in 18F-FDG SUV values was significant only in the striatum, midbrain and VTA (p < 0.05, Figure 4C). However, in the awake situation, SUVR values were strikingly similar in buprenorphine-treated and control rats with no significant difference (Figure 4D).
3.3. Behavioral test
The EPM test showed that treatment by buprenorphine 0.1 mg/kg did not significantly impact animal behavior in terms of anxiety or locomotor activity (p > 0.05, Figure 5). This was similarly observed in both the awake or the anaesthetized paradigm.
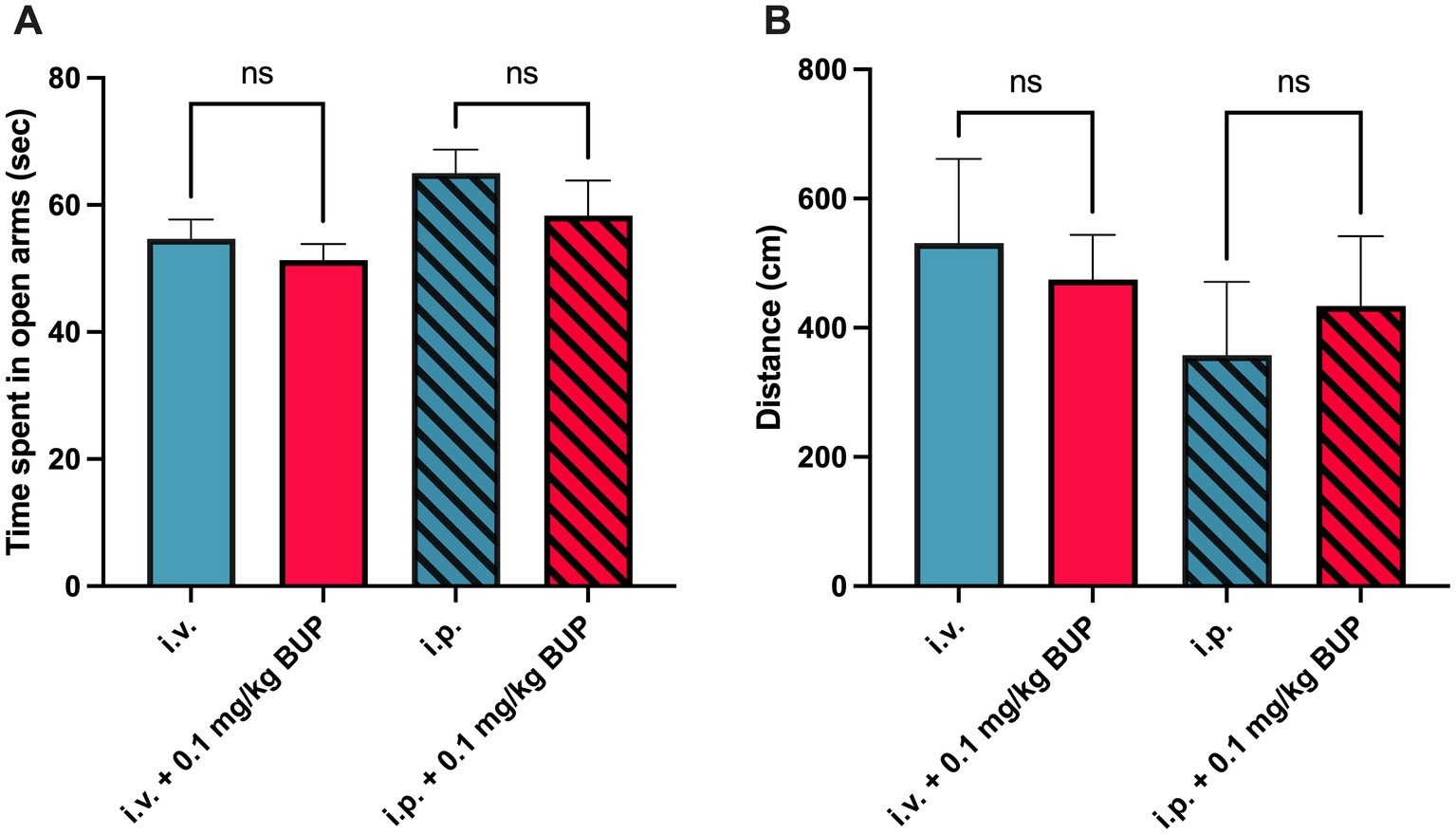
Figure 5. Behavioral analysis for elevated plus maze. Corresponding time spent in open arms (in sec, n = 7 per group) in awake paradigm (full bars) and after the anesthetized paradigm (hatched bars) associated with animal handling during the anesthetized paradigm (i.v injection) or awake paradigm, i.p injection) is shown in A. This was measured without or with administration of buprenorphine (0.1 mg/kg, BUP). Corresponding locomotor activity in both the open and walled arms was determined (in cm) is shown in B. n.s: non-significant.
4. Discussion
Innovative pharmacological challenges associated with appropriate pharmacological neuroimaging techniques are needed to explore the complexity of the neuropharmacology of opioids and combat the opioid pandemic (Volkow and Collins, 2017; Moningka et al., 2019). The aim of this study was to validate a reproducible pharmacological challenge, safely enabling full receptor occupancy of MOR, which CNS effects can be quantitatively monitored using brain 18F-FDG PET in rats.
First, we used brain 11C-buprenorphine PET imaging in rats to show that full saturation of neuroreceptors was achieved with the challenge dose of buprenorphine (0.1 mg/kg, s.c). Target engagement is better correlated with plasma concentration of buprenorphine rather than injected dose (Auvity et al., 2021). Pharmacological challenge associated with full receptor occupancy is therefore preferable to limit the impact of pharmacokinetic variability, which may lead to different level of receptor occupancy, on PD measurements. The results obtained after injection with a 100-fold higher dose of buprenorphine (10 mg/kg) did not further block the binding of 11C-buprenorphine to any brain region confirming that full saturation was achieved with buprenorphine 0.1 mg/kg. Receptor occupancy was estimated using 11C-buprenorphine PET imaging rather than a MOR-specific radioligand such as 11C-carfentanyl (Eriksson and Antoni, 2015). This enabled investigation of the binding of buprenorphine to any CNS target, which may not restrict to MOR (Lutfy and Cowan, 2004). However, very low binding of 11C-buprenorphine in the cerebellum compared with other brain regions is consistent with the low expression of MOR in the rat cerebellum (Tempel and Zukin, 1987), and was similarly observed in nonhuman primates (Galynker et al., 1996; Auvity et al., 2021). This cerebellar binding may either reflect low but significant expression or MOR in the cerebellum, or significant binding of 11C-buprenorphine to kappa-opioid receptor (KOR) which are widely expressed in the rat cerebellum (Tempel and Zukin, 1987; Peckys and Landwehrmeyer, 1999). However, the very low involvement of 11C-buprenorphine binding to the cerebellum compared with other brain regions supports its predominant binding to MOR, as previously demonstrated using blocking by either MOR-or KOR-specific ligands in rats (Auvity et al., 2021).
Dynamic PET data revealed that maximum brain uptake of 11C-buprenorphine was achieved ~10 min after i.v injection (Figure 2). The slow washout of radioactivity from the brain is consistent with the slow dissociation rate of buprenorphine to MOR in vitro (Bidlack et al., 2018). These PET data moreover show that buprenorphine binding to neuroreceptor is relatively stable over a prolonged time-frame which ensures stability of the MOR occupancy during pharmacodynamic or pharmacological imaging assessment. Brain 18F-FDG PET was therefore injected 30 min after the buprenorphine challenge to capture a time-frame during which MOR are fully occupied.
Buprenorphine-induced hyperactivity has been reported in certain rat strains (Burke et al., 2019). The EPM test was therefore conducted in our Sprague–Dawley rats and showed that the selected dose of buprenorphine did not impact animal behavior in terms of anxiety or locomotor activity. This means that change in brain function, as assessed from the outcome of brain 18F-FDG experiments, is not likely to reflect dramatic change in animal behavior or peripheral movement during the buprenorphine challenge in conscious animals. The limited impact of buprenorphine on animal behavior prompted us to test of a paradigm aiming at limiting the impact of anaesthesia on the outcome of brain 18F-FDG PET data.
In the anesthetized rat paradigm, unlabelled buprenorphine was injected in awake animal 30 min before 18F-FDG PET acquisition, which was performed under isoflurane anaesthesia. The buprenorphine challenge significantly decreased 18F-FDG uptake (SUV) in all tested brain region except the cerebellum. This suggests that conventional brain 18F-FDG PET, performed under isoflurane anaesthesia, can be used as a translational biomarker of the response to the buprenorphine challenge in the brain. This provides a simple pharmacological imaging paradigm to investigate the CNS effects of full receptor occupancy by this MOR agonist. These preclinical data are consistent with previously reported clinical PET data obtained in polydrug abusers. A single dose of buprenorphine (1 mg, intramuscularly) significantly decreased the brain glucose consumption by 21%, as assessed using brain 18F-FDG PET with kinetic modeling, which takes both the arterial input function and blood glucose level into account (Walsh et al., 1994).
Efforts were then made to improve the significance and/or the sensitivity of 18F-FDG PET to non-invasively investigate the CNS effects of buprenorphine. First, using the anesthetized rat paradigm, normalization of the regional PET data by the uptake by the cerebellum led to a global decrease in SUVR values in the brain. This strongly suggests that the decrease in 18F-FDG uptake by the brain induced by buprenorphine is not due to any change in the peripheral metabolism of glucose or 18F-FDG. However, regional difference in SUVR, as tested using post-hoc analysis, was significant only in the thalamus and striatum (p < 0.05). This may be explained by the relatively low number of rats in each group (n = 5). Moreover, our study used different individuals to compare 18F-FDG uptake without and after pre-treatment by buprenorphine, which did not take full advantage of PET imaging as a minimally invasive procedure (Lancelot and Zimmer, 2010; Zimmer, 2023). For future studies, comparison of the regional 18F-FDG uptake in the same individuals, before and after administration of buprenorphine, would enable paired comparison with higher statistical power to better capture and compare the effects of buprenorphine across brain regions.
It is often assumed that general anaesthesia may impede the CNS effects of drugs, thus supporting the use of neuroimaging technique in awake and conscious animals (Chang et al., 2016; Cavaliere et al., 2018). This particularly holds for preclinical phMRI studies for which neuroimaging teams have to develop a sophisticated expertise to prevent motion artifacts and reduce restraint-induced stress during acquisition (Ferrari et al., 2012). We hypothesized that the awake rat paradigm, which proved to be useful for pharmacological 18F-FDG PET studies in rodents (Levigoureux et al., 2019; Hugon et al., 2022), may offer enhanced sensitivity to detect the CNS effects of buprenorphine. Baseline SUV values in the brain were lower in the awake compared with the anesthetized rat paradigm. In anesthetized rats, limited impact of the injection route (i.v vs i.p injection) on late brain SUVs has been reported (Schiffer et al., 2007). Moreover, isoflurane anaesthesia used in the uptake phase of anesthetized rat paradigm, but not in the awake rat paradigm, was expected to decrease 18F-FDG uptake by the brain (Spangler-Bickell et al., 2016). Unexpectedly, in awake rats, a global increase in SUV was observed which was significant at the regional level in the striatum, midbrain (p < 0.05) and VTA (p < 0.01). However, normalization of regional SUVs by the cerebellar uptake strikingly abolished the global and regional effect of buprenorphine. This suggests that the increase in SUV may rather be due to an alteration in the peripheral metabolism of 18F-FDG. Our behavioral data suggest that this may not be linked with change in locomotor activity or anxiety in awake animal. It may be hypothesized that in the awake rat paradigm, buprenorphine may decrease the uptake of 18F-FDG by peripheral tissues, thus enhancing its brain availability. Altogether, these results do not support the use of the awake rat paradigm for the pharmacological imaging a buprenorphine in rats, which can be performed using a conventional procedure under isoflurane anaesthesia. The awake paradigm has been successfully used for pharmacological neuroimaging (Levigoureux et al., 2019; Hugon et al., 2022). This means that selection of either the awake or anesthetized paradigm requires preliminary validation and may depend on the investigated drug.
There are several limitations in this study. First, PET data have only been interpreted in the light of SUV and SUVR values, calculated from brain PET data only. Full kinetic modeling, which requires arterial blood sampling, is difficult to perform in rodents and has not been performed in neither the 11C-buprenorphine nor the 18F-FDG PET study. Buprenorphine is mainly metabolized into norbuprenorphine in rats (Yassen et al., 2012). However, it was shown that norbuprenorphine (Alhaddad et al., 2012), as well as 11C-norbuprenorphine (Auvity et al., 2020), negligibly cross the blood–brain barrier compared with parent buprenorphine/11C-buprenorphine. It can therefore be assumed that the brain PET signal associated with 11C-buprenorphine injection predominantly corresponds to parent, unmetabolized 11C-buprenorphine. Similarly, full kinetic modeling would have been useful to quantitatively address the effects of buprenorphine on the brain glucose metabolism using 18F-FDG PET. However, in anesthetized rat, the limited impact of buprenorphine on 18F-FDG uptake in the cerebellum enabled normalization of the brain PET signal. The cerebellum cannot be properly considered a reference region for 18F-FDG because it shows substantial brain uptake. However, normalization of PET data by the cerebellum uptake (SUVR) limits the possible impact of buprenorphine on blood glucose and peripheral kinetics of 18F-FDG for correct interpretation of brain PET data.
Our pharmacological imaging data for buprenorphine, obtained using 18F-FDG PET imaging, can be directly compared with buprenorphine challenge performed using phMRI and the same dose of buprenorphine in rodents (0.1 mg/kg i.v.). In conscious rats (but not in anesthetized rats), buprenorphine yielded phMRI activation, as assessed from change in the BOLD (blood-oxygen-level dependent) signal in cortex (i.e., cingulate, somatosensory cortex, and insula), subcortical structures (i.e., caudate-putamen, hippocampus, and thalamus) and cerebellum (Becerra et al., 2013). This activation profile corresponds with the brain distribution of 11C-buprenorphine except in the cerebellum. Moreover, phMRI deactivation was observed in the cingulate, mammillary nuclei, amygdala, dentate gyrus and brainstem. This complex phMRI response profile contrasts with our 18F-FDG PET data showing a decrease in brain glucose metabolism in MOR-rich regions in anesthetized rodent and humans (Walsh et al., 1994). Similar phMRI response profile, with predominant activation pattern, have been reported in awake nonhuman primates (Seah et al., 2014) and humans (Upadhyay et al., 2011), as assessed from change in CBV (cerebral brain volume). These discrepancies suggest that phMRI and 18F-FDG PET do not capture the same neuropharmacological parameters to describe the CNS effects of buprenorphine. It may be hypothesized that phMRI captures the direct activation of MORs whereas 18F-FDG PET captures the downstream MOR-related effects of buprenorphine which involves modulation of GABA-and glutamate-mediated processes, and results in a global decrease in neuronal activity (Reeves et al., 2022). It can therefore be assumed that 18F-FDG PET may reflect the outcome of fMRI data showing the global decrease in functional connectivity observed during a buprenorphine challenge in humans (Upadhyay et al., 2011). Finally, compared with fMRI, PET is a quantitative imaging modality, although only semi-quantitative analysis, based on the determination of SUVR in a limited number of rats, was performed in this study to compare the effect of full receptor occupancy by buprenorphine on brain function (Tournier et al., 2021). Further experiments are needed to explore the ability of 18F-FDG PET to distinguish different level of response to the buprenorphine challenge validated here.
5. Conclusion
In the present study, a pharmacological imaging challenge using buprenorphine 0.1 mg/kg was validated. This dose enabled full receptor occupancy with limited impact on rat locomotor activity or anxiety. CNS effects induced by this buprenorphine challenge can be monitored using 18F-FDG PET in anesthetized rats. This multi-tracer approach using minimally-invasive PET imaging could be combined with phMRI to provide a multiparametric insight into the functional determinants of the acute response to opioids. This may help untangling the neurophysiology of opioid tolerance and dependence in vivo.
Data availability statement
The raw data supporting the conclusions of this article will be made available by the authors, without undue reservation.
Ethics statement
The animal study was reviewed and approved by CETA n° APAFIS#34694-2022011718132961 v1.
Author contributions
CL, WS, KT, and NT designed the study. AS, SL, and MG acquired the data. AS, LB, CL, KT, and SG analysed the data. FC supervised the radiochemistry. CL, KT, GB, and MB contributed to the interpretation of the results. AS wrote the first draft. All authors contributed to the article and approved the submitted version.
Funding
This work was funded by Amélie Soyer received a PhD grant from the CEA (commissariat à l’énergie atomique et aux énergies alternatives) and the AID (Agence de l’innovation de défense - Ministère des Armées). This work was performed on a platform member of France Life Imaging network (grant ANR-11-INBS-0006).
Acknowledgments
The authors gratefully thank Maud Goislard for technical assistance and Philippe Gervais for providing 18F-FDG.
Conflict of interest
The authors declare that the research was conducted in the absence of any commercial or financial relationships that could be construed as a potential conflict of interest.
Publisher’s note
All claims expressed in this article are solely those of the authors and do not necessarily represent those of their affiliated organizations, or those of the publisher, the editors and the reviewers. Any product that may be evaluated in this article, or claim that may be made by its manufacturer, is not guaranteed or endorsed by the publisher.
References
Alhaddad, H., Cisternino, S., Declèves, X., Tournier, N., Schlatter, J., Chiadmi, F., et al. (2012). Respiratory toxicity of buprenorphine results from the blockage of P-glycoprotein-mediated efflux of norbuprenorphine at the blood-brain barrier in mice. Crit. Care Med. 40, 3215–3223. doi: 10.1097/CCM.0b013e318265680a
Auvity, S., Breuil, L., Goislard, M., Bottlaender, M., Kuhnast, B., Tournier, N., et al. (2020). An original radio-biomimetic approach to synthesize radiometabolites for PET imaging. Nucl. Med. Biol. 90-91, 10–14. doi: 10.1016/j.nucmedbio.2020.08.001
Auvity, S., Goutal, S., Caillé, F., Vodovar, D., Pruvost, A., Wimberley, C., et al. (2021). Pharmacokinetic neuroimaging to study the dose-related brain kinetics and target engagement of buprenorphine in vivo. Neuropsychopharmacology 46, 1220–1228. doi: 10.1038/s41386-021-00976-w
Becerra, L., Upadhyay, J., Chang, P.-C., Bishop, J., Anderson, J., Baumgartner, R., et al. (2013). Parallel buprenorphine phMRI responses in conscious rodents and healthy human subjects. J. Pharmacol. Exp. Ther. 345, 41–51. doi: 10.1124/jpet.112.201145
Bidlack, J. M., Knapp, B. I., Deaver, D. R., Plotnikava, M., Arnelle, D., Wonsey, A. M., et al. (2018). In vitro pharmacological characterization of buprenorphine, Samidorphan, and combinations being developed as an adjunctive treatment of major depressive disorder. J. Pharmacol. Exp. Ther. 367, 267–281. doi: 10.1124/jpet.118.249839
Burke, N. N., Ferdousi, M., Deaver, D. R., Finn, D. P., Roche, M., and Kelly, J. P. (2019). Locomotor and anti-immobility effects of buprenorphine in combination with the opioid receptor modulator samidorphan in rats. Neuropharmacology 146, 327–336. doi: 10.1016/j.neuropharm.2018.12.012
Cami-Kobeci, G., Polgar, W. E., Khroyan, T. V., Toll, L., and Husbands, S. M. (2011). Structural determinants of opioid and NOP receptor activity in derivatives of buprenorphine. J. Med. Chem. 54, 6531–6537. doi: 10.1021/jm2003238
Cavaliere, C., Kandeepan, S., Aiello, M., Ribeiro de Paula, D., Marchitelli, R., Fiorenza, S., et al. (2018). Multimodal neuroimaging approach to variability of functional connectivity in disorders of consciousness: a PET/MRI pilot study. Front. Neurol. 9:861. doi: 10.3389/fneur.2018.00861
Chang, P.-C., Procissi, D., Bao, Q., Centeno, M. V., Baria, A., and Apkarian, A. V. (2016). Novel method for functional brain imaging in awake minimally restrained rats. J. Neurophysiol. 116, 61–80. doi: 10.1152/jn.01078.2015
Cumming, P., Marton, J., Lilius, T. O., Olberg, D. E., and Rominger, A. (2019). A survey of molecular imaging of opioid receptors. Molecules 24:E4190. doi: 10.3390/molecules24224190
Dahan, A., Yassen, A., Romberg, R., Sarton, E., Teppema, L., Olofsen, E., et al. (2006). Buprenorphine induces ceiling in respiratory depression but not in analgesia. Br. J. Anaesth. 96, 627–632. doi: 10.1093/bja/ael051
Drewes, A. M., Jensen, R. D., Nielsen, L. M., Droney, J., Christrup, L. L., Arendt-Nielsen, L., et al. (2013). Differences between opioids: pharmacological, experimental, clinical and economical perspectives: differences between opioids. Br. J. Clin. Pharmacol. 75, 60–78. doi: 10.1111/j.1365-2125.2012.04317.x
Eriksson, O., and Antoni, G. (2015). [11C] Carfentanil binds preferentially to μ-opioid receptor subtype 1 compared to subtype 2. Mol. Imaging 14, 7290.2015.00019, 476–483. doi: 10.2310/7290.2015.00019
Etaee, F., Asadbegi, M., Taslimi, Z., Shahidi, S., Sarihi, A., Soleimani Asl, S., et al. (2017). The effects of methamphetamine and buprenorphine, and their interaction on anxiety-like behavior and locomotion in male rats. Neurosci. Lett. 655, 172–178. doi: 10.1016/j.neulet.2017.04.043
Ferrari, L., Turrini, G., Crestan, V., Bertani, S., Cristofori, P., Bifone, A., et al. (2012). A robust experimental protocol for pharmacological fMRI in rats and mice. J. Neurosci. Methods 204, 9–18. doi: 10.1016/j.jneumeth.2011.10.020
Galynker, I., Schlyer, D. J., Dewey, S. L., Fowler, J. S., Logan, J., Gatley, S. J., et al. (1996). Opioid receptor imaging and displacement studies with [6-O-[11C] methyl] buprenorphine in baboon brain. Nucl. Med. Biol. 23, 325–331. doi: 10.1016/0969-8051(95)02087-X
Greenwald, M. K., Herring, A. A., Perrone, J., Nelson, L. S., and Azar, P. (2022). A Neuropharmacological model to explain buprenorphine induction challenges. Ann. Emerg. Med. 80, 509–524. doi: 10.1016/j.annemergmed.2022.05.032
Greenwald, M. K., Johanson, C.-E., Moody, D. E., Woods, J. H., Kilbourn, M. R., Koeppe, R. A., et al. (2003). Effects of buprenorphine maintenance dose on mu-opioid receptor availability, plasma concentrations, and antagonist blockade in heroin-dependent volunteers. Neuropsychopharmacology 28, 2000–2009. doi: 10.1038/sj.npp.1300251
Helm, S., Trescot, A. M., Colson, J., Sehgal, N., and Silverman, S. (2008). Opioid antagonists, partial agonists, and agonists/antagonists: the role of office-based detoxification. Pain Physician 11, 225–235. doi: 10.36076/ppj.2008/11/225
Hugon, G., Goutal, S., Sarazin, M., Bottlaender, M., Caillé, F., Droguerre, M., et al. (2022). Impact of donepezil on brain glucose metabolism assessed using [18F]2-Fluoro-2-deoxy-D-glucose positron emission tomography imaging in a mouse model of Alzheimer’s disease induced by Intracerebroventricular injection of amyloid-Beta peptide. Front. Neurosci. 16:835577. doi: 10.3389/fnins.2022.835577
Infantino, R., Mattia, C., Locarini, P., Pastore, A. L., Maione, S., and Luongo, L. (2021). Buprenorphine: far beyond the “ceiling”. Biomol. Ther. 11:816. doi: 10.3390/biom11060816
Ing Lorenzini, K., Daali, Y., Dayer, P., and Desmeules, J. (2012). Pharmacokinetic-pharmacodynamic modelling of opioids in healthy human volunteers. A minireview. Basic Clin. Pharmacol. Toxicol. 110, 219–226. doi: 10.1111/j.1742-7843.2011.00814.x
Lancelot, S., and Zimmer, L. (2010). Small-animal positron emission tomography as a tool for neuropharmacology. Trends Pharmacol. Sci. 31, 411–417. doi: 10.1016/j.tips.2010.06.002
Levigoureux, E., Vidal, B., Fieux, S., Bouillot, C., Emery, S., Newman-Tancredi, A., et al. (2019). Serotonin 5-HT1A receptor biased agonists induce different cerebral metabolic responses: a [18F]-Fluorodesoxyglucose positron emission tomography study in conscious and anesthetized rats. ACS Chem. Neurosci. 10, 3108–3119. doi: 10.1021/acschemneuro.8b00584
Lutfy, K., and Cowan, A. (2004). Buprenorphine: a unique drug with complex pharmacology. Curr. Neuropharmacol. 2, 395–402. doi: 10.2174/1570159043359477
Moningka, H., Lichenstein, S., Worhunsky, P. D., DeVito, E. E., Scheinost, D., and Yip, S. W. (2019). Can neuroimaging help combat the opioid epidemic? A systematic review of clinical and pharmacological challenge fMRI studies with recommendations for future research. Neuropsychopharmacology 44, 259–273. doi: 10.1038/s41386-018-0232-4
Morgan, M. M., and Christie, M. J. (2011). Analysis of opioid efficacy, tolerance, addiction and dependence from cell culture to human. Br. J. Pharmacol. 164, 1322–1334. doi: 10.1111/j.1476-5381.2011.01335.x
Peckys, D., and Landwehrmeyer, G. B. (1999). Expression of mu, kappa, and delta opioid receptor messenger RNA in the human CNS: a 33P in situ hybridization study. Neuroscience 88, 1093–1135. doi: 10.1016/s0306-4522(98)00251-6
Reeves, K. C., Shah, N., Muñoz, B., and Atwood, B. K. (2022). Opioid receptor-mediated regulation of neurotransmission in the brain. Front. Mol. Neurosci. 15:919773. doi: 10.3389/fnmol.2022.919773
Schiffer, W. K., Mirrione, M. M., and Dewey, S. L. (2007). Optimizing experimental protocols for quantitative behavioral imaging with 18F-FDG in rodents. J. Nucl. Med. 48, 277–287.
Seah, S., Asad, A. B. A., Baumgartner, R., Feng, D., Williams, D. S., Manigbas, E., et al. (2014). Investigation of cross-species translatability of pharmacological MRI in awake nonhuman primate - a buprenorphine challenge study. PLoS One 9:e110432. doi: 10.1371/journal.pone.0110432
Spangler-Bickell, M. G., de Laat, B., Fulton, R., Bormans, G., and Nuyts, J. (2016). The effect of isoflurane on 18F-FDG uptake in the rat brain: a fully conscious dynamic PET study using motion compensation. EJNMMI Res. 6:86. doi: 10.1186/s13550-016-0242-3
Tempel, A., and Zukin, R. S. (1987). Neuroanatomical patterns of the mu, delta, and kappa opioid receptors of rat brain as determined by quantitative in vitro autoradiography. Proc. Natl. Acad. Sci. 84, 4308–4312. doi: 10.1073/pnas.84.12.4308
Tournier, N., Comtat, C., Lebon, V., and Gennisson, J.-L. (2021). Challenges and perspectives of the hybridization of PET with functional MRI or ultrasound for neuroimaging. Neuroscience 474, 80–93. doi: 10.1016/j.neuroscience.2020.10.015
Trescot, A. M., Datta, S., Lee, M., and Hansen, H. (2008). Opioid pharmacology. Pain Physician 11, S133–S153. doi: 10.36076/ppj.2008/11/S133
Upadhyay, J., Anderson, J., Schwarz, A. J., Coimbra, A., Baumgartner, R., Pendse, G., et al. (2011). Imaging drugs with and without clinical analgesic efficacy. Neuropsychopharmacology 36, 2659–2673. doi: 10.1038/npp.2011.156
Vodovar, D., Chevillard, L., Caillé, F., Risède, P., Pottier, G., Auvity, S., et al. (2022). Mechanisms of respiratory depression induced by the combination of buprenorphine and diazepam in rats. Br. J. Anaesth. 128, 584–595. doi: 10.1016/j.bja.2021.10.029
Volkow, N. D., and Blanco, C. (2021). The changing opioid crisis: development, challenges and opportunities. Mol. Psychiatry 26, 218–233. doi: 10.1038/s41380-020-0661-4
Volkow, N. D., and Collins, F. S. (2017). The role of science in addressing the opioid crisis. N. Engl. J. Med. 377, 391–394. doi: 10.1056/NEJMsr1706626
Wakeman, S. E., and Barnett, M. L. (2018). Primary care and the opioid-overdose crisis-buprenorphine myths and realities. N. Engl. J. Med. 379, 1–4. doi: 10.1056/NEJMp1802741
Walsh, S. L., Gilson, S. F., Jasinski, D. R., Stapleton, J. M., Phillips, R. L., Dannals, R. F., et al. (1994). Buprenorphine reduces cerebral glucose metabolism in polydrug abusers. Neuropsychopharmacology 10, 157–170. doi: 10.1038/npp.1994.18
Yassen, A., Olofsen, E., Kan, J., Dahan, A., and Danhof, M. (2012). Animal-to-human extrapolation of the pharmacokinetic and Pharmacodynamic properties of buprenorphine. Clin. Pharmacokinet. 46, 433–447. doi: 10.2165/00003088-200746050-00005
Keywords: addiction, neuroimaging, opioid, PET imaging, pharmacodynamics, pharmacokinetics, receptor occupancy, neuroreceptor imaging
Citation: Soyer A, Leterrier S, Breuil L, Goislard M, Leroy C, Saba W, Thibault K, Bo GD, Bottlaender M, Caillé F, Goutal S and Tournier N (2023) Validation of a pharmacological imaging challenge using 11C-buprenorphine and 18F-2-fluoro-2-deoxy-D-glucose positron emission tomography to study the effects of buprenorphine to the rat brain. Front. Neurosci. 17:1181786. doi: 10.3389/fnins.2023.1181786
Edited by:
Benjamin Vidal, Theranexus (France), FranceReviewed by:
Frode Willoch, University of Oslo, NorwayJeih-San Liow, National Institutes of Health (NIH), United States
Copyright © 2023 Soyer, Leterrier, Breuil, Goislard, Leroy, Saba, Thibault, Bo, Bottlaender, Caillé, Goutal and Tournier. This is an open-access article distributed under the terms of the Creative Commons Attribution License (CC BY). The use, distribution or reproduction in other forums is permitted, provided the original author(s) and the copyright owner(s) are credited and that the original publication in this journal is cited, in accordance with accepted academic practice. No use, distribution or reproduction is permitted which does not comply with these terms.
*Correspondence: Nicolas Tournier, bi50b3VybmllckB1bml2ZXJzaXRlLXBhcmlzLXNhY2xheS5mcg==