- 1Nantes Université, INSERM, CHU Nantes, The Enteric Nervous System in Gut and Brain Disorders, Nantes, France
- 2Nantes Université, INRAE, IMAD, CRNH-O, UMR 1280, PhAN, Nantes, France
Background and objective: There is mounting evidence to suggest that the gut-brain axis is involved in the development of Parkinson’s disease (PD). In this regard, the enteroendocrine cells (EEC), which faces the gut lumen and are connected with both enteric neurons and glial cells have received growing attention. The recent observation showing that these cells express alpha-synuclein, a presynaptic neuronal protein genetically and neuropathologically linked to PD came to reinforce the assumption that EEC might be a key component of the neural circuit between the gut lumen and the brain for the bottom-up propagation of PD pathology. Besides alpha-synuclein, tau is another key protein involved in neurodegeneration and converging evidences indicate that there is an interplay between these two proteins at both molecular and pathological levels. There are no existing studies on tau in EEC and therefore we set out to examine the isoform profile and phosphorylation state of tau in these cells.
Methods: Surgical specimens of human colon from control subjects were analyzed by immunohistochemistry using a panel of anti-tau antibodies together with chromogranin A and Glucagon-like peptide-1 (two EEC markers) antibodies. To investigate tau expression further, two EEC lines, namely GLUTag and NCI-H716 were analyzed by Western blot with pan-tau and tau isoform specific antibodies and by RT-PCR. Lambda phosphatase treatment was used to study tau phosphorylation in both cell lines. Eventually, GLUTag were treated with propionate and butyrate, two short chain fatty acids known to sense EEC, and analyzed at different time points by Western blot with an antibody specific for tau phosphorylated at Thr205.
Results: We found that tau is expressed and phosphorylated in EEC in adult human colon and that both EEC lines mainly express two tau isoforms that are phosphorylated under basal condition. Both propionate and butyrate regulated tau phosphorylation state by decreasing its phosphorylation at Thr205.
Conclusion and inference: Our study is the first to characterize tau in human EEC and in EEC lines. As a whole, our findings provide a basis to unravel the functions of tau in EEC and to further investigate the possibility of pathological changes in tauopathies and synucleinopathies.
Introduction
The enteroendocrine cells (EEC), which are scatterly distributed along the entire gastrointestinal (GI) mucosa representing around 1% of the total gut epithelium cell population, are key components of the gut-brain axis. They are classically regarded as specialized hormone-secreting cells with an apical surface that is exposed to gut lumen and a basal portion that contains secretory granules (Gribble and Reimann, 2019). Such an orientation allows EEC to respond to intraluminal signals such as nutrients or microbiota-derived metabolites. This aspect has been particularly well documented for short-chain fatty acids (SCFA), such as butyrate and propionate, which induce the release of the peptide hormone YY (PYY) (Larraufie et al., 2018). Morphologically, EEC were shown to exhibit neuron-like features including the expression of pre- and post-synaptic proteins together with the presence of neurite-like processes, called neuropods (Bohórquez et al., 2015). These neuropods are in close contact with the two components of the enteric nervous system (ENS), namely enteric glial cells (Bohórquez et al., 2014) and enteric neurons (Chandra et al., 2017) as well as vagal neurons (Kaelberer et al., 2018). The recent findings, which showed that EEC contain alpha-synuclein (Chandra et al., 2017), a presynaptic neuronal protein genetically and neuropathologically linked to Parkinson’s disease (PD) led to the assumption that they might be involved in the development of PD. By facing the gut lumen and being directly connected with alpha-synuclein positive enteric neurons (Chandra et al., 2017), the EEC might be a key component of the neural circuit between the gut lumen and the brain for the bottom-up propagation of PD pathology as initially hypothesized by Braak et al. (2006) and more recently by Borghammer and Van Den Berge (2019).
Alpha-synuclein accumulates in a group of neurodegenerative diseases collectively known as synucleinopathies with PD being the most common, while the accumulation of tau is a defining feature of tauopathies classically found in the brains of patients with Alzheimer’s disease (AD) or progressive supranuclear palsy (PSP) (Dugger and Dickson, 2017). Nevertheless, in numerous cases alpha-synuclein positive inclusions are also described in tauopathies and vice versa, suggesting a co-existence or crosstalk of these proteinopathies (Arai et al., 2001; Coughlin et al., 2019). Tau, like alpha-synuclein is expressed by enteric neurons (Lionnet et al., 2018), thereby suggesting that enteric tau might be involved in neurodegenerative disorders and/or enteric neuropathies (Derkinderen et al., 2021). In contrast to alpha-synuclein, no data are available about the distribution and phosphorylation pattern of tau isoforms in EEC. Here, we first examined the expression levels of tau isoforms and their phosphorylation profile in full thickness segments of human colon and in EEC lines. We then studied the regulation of tau phosphorylation by SCFA in EEC. Our results show the presence of phosphorylated tau in both mouse and human colonic EEC and the expression of one main tau isoform in EEC lines. We also show that EEC tau is phosphorylated under basal condition and that its phosphorylation state can be modified by SCFA. These data provide the first detailed characterization of EEC tau in human adult colonic tissues and in cell lines. Further investigation of tau modifications in EEC in pathological conditions may provide valuable information about the possible role of EEC tau in neurodegenerative diseases.
Materials and methods
Human, mouse, and rat tissues
Specimens of human colon were obtained from eight neurologically unimpaired subjects who underwent colon resection for colorectal cancer (5 men, 71 ± 7.6 years). For all tissues specimens, sampling was performed in macroscopically normal segments of uninvolved resection margins. The sampling of human colon was approved by the Fédération des Biothèques of the University Hospital of Nantes, according to the guidelines of the French Ethics Committee for Research on Humans and registered under the no. DC-2008-402. Written informed consent was obtained from each subject. Hippocampi were taken from a 2-month-old wild type C57BL/6J mouse and sciatic nerve sections were taken from one pregnant Sprague-Dawley rats (used for the generation of primary culture of rat ENS in our lab) in order to identify tau isoforms in GLUTag cells and to serve as a positive control for big tau experiments, respectively. These samples were stored at −80°C until further analysis by Western blot.
EEC lines and reagents
NCI-H716 cells (ATCC, LGC Standards, Molsheim, France, CCL-251) were maintained in RPMI-1640 (Gibco, Life Technologies, Villebon-sur-Yvette, France) whereas GLUTag (a gift from Professor Daniel J. Drucker of University of Toronto) and Caco-2 cells were maintained in DMEM (Gibco), all supplemented with 10% fetal bovine serum, 2 mM L-Glutamine, 50 IU/ml penicillin and 50 μg/ml streptomycin (all from Merck-Sigma, Saint-Quentin Fallavier, France) in a humidified incubator at 37 °C with 5% of CO2. Propionate and butyrate were from Merck-Sigma.
Dephosphorylation of tissues and cell lysates
For dephosphorylation experiments, cells and hippocampi were homogenized in a buffer containing 100 mM NaCl and 50 mM Tris-Cl at pH 7.4 with 1% (v/v) IGEPAL® CA-630 (ThermoFisher, Saint-Herblain, France) and a protease inhibitors cocktail without EDTA (Roche, Neuilly sur Seine, France) using either a “Precellys 24” (Bertin technologies, St Quentin-en-Yvelines, France) tissue homogenizer and followed by sonication with “vibracell 75 186” device (Sonics, Newton, CT, USA). Homogenates were centrifuged at 16,100 g for 20 min at 4°C with an Eppendorf 5415R centrifuge (Eppendorf, Hamburg, Germany), sonicated for 10 s and protein amounts normalized following a bicinchoninic acid protein assay (ThermoFisher). Samples were diluted to 1.0 mg/ml protein using homogenization buffer and incubated with 20 U/μl lambda phosphatase in MnCl2 and enzyme buffer as supplied with the lambda protein phosphatase kit (New England Biolabs, Evry-Courcouronnes, France) for 3 h at 30°C. The reaction was stopped by the addition of sample buffer (Life Technologies) and heating to 95°C for 5 min. Control samples were treated identically without the addition of lambda phosphatase.
SDS-PAGE and Western blot
For dephosphorylation experiments, cells or tissues were processed as described above. For experiments that did not require dephosphorylation, cells or tissues were lysed in RIPA lysis buffer (Merck Millipore, Fontenay sous Bois, France) containing 2 mM orthovanadate (Merck-Sigma, Molsheim, France), phosphatase inhibitor cocktail II (Merck-Sigma) and a protease inhibitors cocktail (Merck-Sigma). Western blots were performed as we previously described using NuPAGE™ 10% Bis-Tris Protein Gels (Life Technologies). The primary anti-tau antibodies used are listed in Table 1. β-Actin antibodies (Abcam, France, 1:1,000 dilution) were used as loading control. Tau ladder (six human tau recombinant isoforms, Sigma-Merck) was used to identify tau isoforms in NCI-H716 cells. For quantification, the relevant immunoreactive bands were quantified with laser-scanning densitometry and analyzed with Image Lab software (Biorad, Marnes-la-Coquette, France) or ImageJ software (NIH; version 1.51). To allow comparison between different films, the density of the bands was expressed as a percentage of the average of controls. pThr205 tau immunoreactive bands were measured, normalized to the optical densities of total tau, and expressed as percentage of controls.
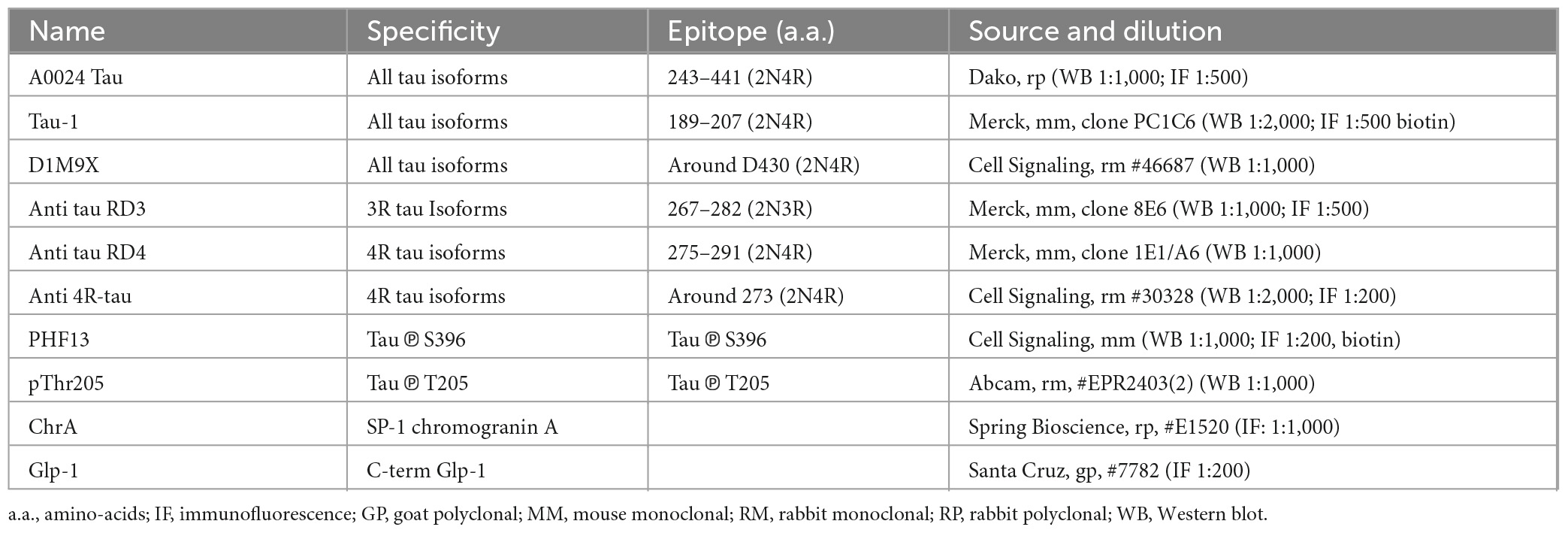
Table 1. The name, specificity, epitope, source, and dilution of the antibodies used in this study are shown.
Immunofluorescence
Human colonic tissues were embedded in paraffin using an embedding station (LEICA EG1150C) and sections (3 μm) were cut using a microtome (LEICA RM2255). The sections were deparaffinized by bathing twice in xylene (for 5 min each) and taken through graded concentrations of ethanol (100, 95, 70, and 50%, respectively, for 3 min each). After a rinse in distilled water, slides were washed in PBS and antigen retrieval was performed using a Sodium Citrate solution (2.94 g Sodium Citrate Tribase; 1 L distilled water; 500 μl Tween 20; pH 6) at 95°C for 20 min. Slides were incubated in NH4Cl (100 mM) for 15 min before incubation in PBS-0.5% triton X-100 (Merck-Sigma) for 1 h and blocking for 2 h in 10% (v/v) horse serum in PBS-0.5% triton X-100. Primary antibodies (Table 1) were incubated overnight at 4°C, and following washing, secondary antibodies were added for 2 h at room temperature. Secondary antibodies were anti-mouse Biotin (A24522, Thermo Fisher Scientific), Alexa Fluor 647-conjugated goat anti-rabbit (711-606-152, Jackson Immunoresearch, Ely, UK), Alexa Fluor 568-conjugated Streptavidin (S11226, Invitrogen, Thermo Fisher Scientific), Alexa Fluor 568-conjugated goat anti-mouse (Molecular Probes, Thermo Scientific). DAPI (1:10,000) was added to counterstain nuclei. Tissues sections were mounted in Prolong Gold anti-fading medium (Molecular Probes, Thermo Scientific). Images were acquired with a Zeiss, Axio Imager M2m fluorescence microscope coupled to a digital camera (Axiocam 503 mono).
RNA extraction and RT-PCR
Total RNA extraction from EEC and Caco-2 cells was carried out using a QIAamp RNA Blood Mini kit (Qiagen, Courtaboeuf, France) following the manufacturer’s instructions. 1 μg RNA per sample was reverse transcribed using a Superscript III reverse transcriptase assay kit (Thermo Fisher Scientific) according to the manufacturer’s instructions. To examine the alternate splicing of the microtubule binding domain repeat region encoded by exon 10, primers were used that specifically recognize mouse or human exons 9 and 11 as described by Duff et al. (2000). Primer sequences were: mouse exon 9F 5′-CCCCCTAAGTCACCATCAGCTAGT, mouse exon 11R 5′-CACTTTGCTCAGGTCCACCGGC, human exon 9F 5′-CTCCAAAATCAGGGGATCGC, human exon 11R 5′-CCTTGCTCAGGTCAACTGGT. Splicing around the N terminal insert domain encoded by exons 2 and 3 was detected using primers that recognize exons 1 and 5. Primer sequences used were: mouse exon 1F 5′-TCCGCTGTCCTCTTCTGTC, mouse exon 5R 5′-TTCTCGTCATTTCCTGTCC, human exon 1F 5′-TGAACCAGGATGGCTGAGC, human exon 5R 5′-TTGTCATCGCTTCCAGTCC. Annealing temperatures were 64°C (all MAPT primers), 62°C (M1F/M5R) and 68°C (M9F/M11R). Thirty-five reaction cycles were used for all. Mouse and human-specific RT-PCR products were analyzed by agarose gel electrophoresis. Products corresponding to exon 10+ tau mRNA (4R) are 390 base pairs (bp), while products corresponding to exon 10- mRNA (3R) are 297 bp. RT-PCR products containing tau mRNA with exons 2 and 3 (2N) are 428 bp, 2 + 3- mRNA products (1N) are 341 bp, and 2–3- mRNA products (0N) are 253 bp.
Statistics
All quantitative data shown are mean ± standard error of the mean (SEM). Mann–Whitney test or Kruskal–Wallis then Dunn’s multiple comparisons tests were performed for two or multiple groups comparison, respectively. Differences were deemed statistically significant if p < 0.05. GraphPad Prism software version 9.1.1 (GraphPad Software Inc., La Jolla, CA, United States) was used for statistical analyses and for designing figures.
Results
Human colonic EEC express tau
To determine if tau is expressed in EEC, we examined chromogranin A and glucagon-like peptide 1 (GLP-1)-containing cells of the human colon. Chromogranin A is an acidic glycoprotein located in secretory vesicles of endocrine cells, which is classically used as a pan-EEC marker (Wilson and Lloyd, 1984), whereas GLP-1 is a marker of L-type EEC (Eissele et al., 1992), the most abundant EEC population in the human colon (Latorre et al., 2016). Using the pan-tau antibody A0024, we identified tau in GLP-1-positive cells in the epithelial lining (Figure 1A). When human colonic tissue was immunolabelled with isoform-specific tau antibodies, we showed that 3R-tau was expressed in chromogranin A immunoreactive cells (Figure 1B); no specific staining was observed when a 4R-tau was used (data not shown). Tau phosphorylation at multiple serine and threonine sites is the predominant mechanism by which its biological activity is regulated (Guo et al., 2017). We therefore examined the phosphorylation state of tau with a phospho-specific antibody that detect tau phosphorylated at Ser396 and showed that phospho-tau was observed within chromogranin A-positive cells in colon epithelium (Figure 1C). Approximately 73 and 80% of chromogranin A immunoreactive cells in the colon contained 3R- and phosphorylated-tau, respectively (Table 2). When taken together, these results show that tau is expressed and phosphorylated in EEC in human colon, with 3R-tau likely being most abundant isoforms.
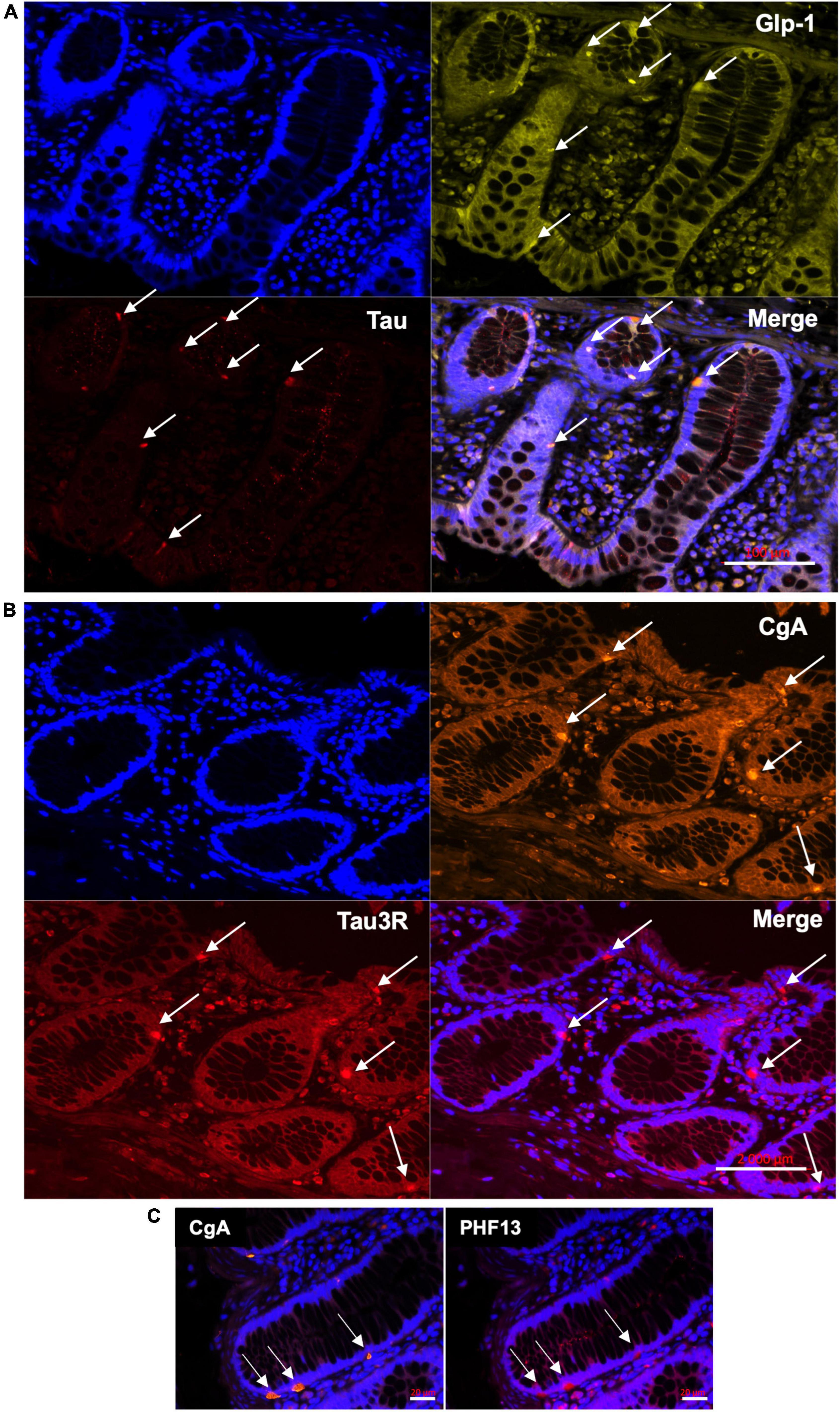
Figure 1. Distribution of tau in the epithelial lining in adult human colon. (A) Total tau antibody A0024 (Table 1) was used to detect tau in epithelium lining of human colonic samples (arrows). An antibody specific to Glucagon-like peptide 1 (GLP-1) was used to specifically label L-type EEC (arrows). DAPI was used to counterstain nuclei. Scale bar is 100 μm. (B) Isoform-specific antibody (tau RD3, Table 1) was used to detect 3R-tau and an antibody specific to chromogranin A (CgA) was used to specifically label EEC (arrows). DAPI was used to counterstain nuclei. Scale bar is 2 mm. (C) Human colonic samples were co-labeled with an antibody specific for tau phosphorylated at Ser396 (PHF13, Table 1) and an antibody specific to chromogranin A (CgA). Arrows show cells immunoreactive for both PS396 and CgA. DAPI was used to counterstain nuclei. Scale bar is 20 μm. Representative photomicrographs are shown.
Tau is expressed and phosphorylated in EEC lines
There are multiple cell lines used as models for EEC research, including GLUTag, a mouse endocrine tumor-adherent cell line and NCI-H716, a human-derived suspension cell line. These two cell lines have been widely used as models for GLP-1–producing L-cells and proved to be useful for in vitro screening bioassays (Goldspink et al., 2018). As a first approach, we compared the banding pattern on Western blots of total tau as evaluated with the A0024 pan-tau antibody between non-dephosphorylated lysates of GLUTag cells and hippocampus of 2-month-old mouse. Total tau antibody detected several bands in 2-month-old mouse brain migrating between 50 and 60 kDa (Figure 2A). In GLUTag cells, the observed banding pattern was markedly different with a doublet of 48 and 50 kDa bands, the latter showing the most intense labeling (Figure 2A). In adult mouse brain, primary CNS/ENS neurons and neuronal cell lines, tau isoforms are phosphorylated on multiple tau and serine residues resulting in reduced electrophoretic mobility on SDS-PAGE compared to non-phosphorylated tau (Goedert et al., 1992a; Davis et al., 1995; Lionnet et al., 2018). In order to determine the phosphorylation state of tau in GLUTag cells, cell lysates treated or not with lambda phosphatase (Davis et al., 1995) were analyzed by Western blot using three different pan-tau antibodies. Treatment with lambda phosphatase caused tau dephosphorylation, as evidenced by a significant downward shift in mobility of the tau doublet detected with either the pan-Tau A0024, D1M9X, or Tau-1 (Figure 2B). Of note, this downward shift was associated with increased Tau immunoreactivity when the Tau-1 antibody was used (Figure 2B). These findings are in line with previous observations showing that Tau-1 binds preferentially to tau when dephosphorylated at serine residues 195, 198, 199, and 202 (Liu et al., 1993; Szendrei et al., 1993). In order to determine which tau isoforms are expressed in GLUTag cells, we used two commercially available isoform-specific tau antibodies directed against 3R and 4R-tau, which have been shown to be highly specific in a recent comprehensive study that tested the specificity of tau antibodies using immunoblotting (Ercan et al., 2017). GLUTag lysates were compared to dephosphorylated brain samples (hippocampus of 2-month-old wild-type mice), which express all 4R isoforms and to a lesser extent the 0N3R isoform (McMillan et al., 2008; Liu and Götz, 2013; Figure 2C). After dephosphorylation, the 3R and 4R antibodies detected one single band in cell lysates that comigrated with 0N3R and 0N4R in the hippocampus, respectively (McMillan et al., 2008; Liu and Götz, 2013; Figure 2C). When taken together, these results show that 0N3R and 0N4R are the two main tau isoforms that are expressed in GLUTag cells and these two isoforms are phosphorylated under basal conditions.
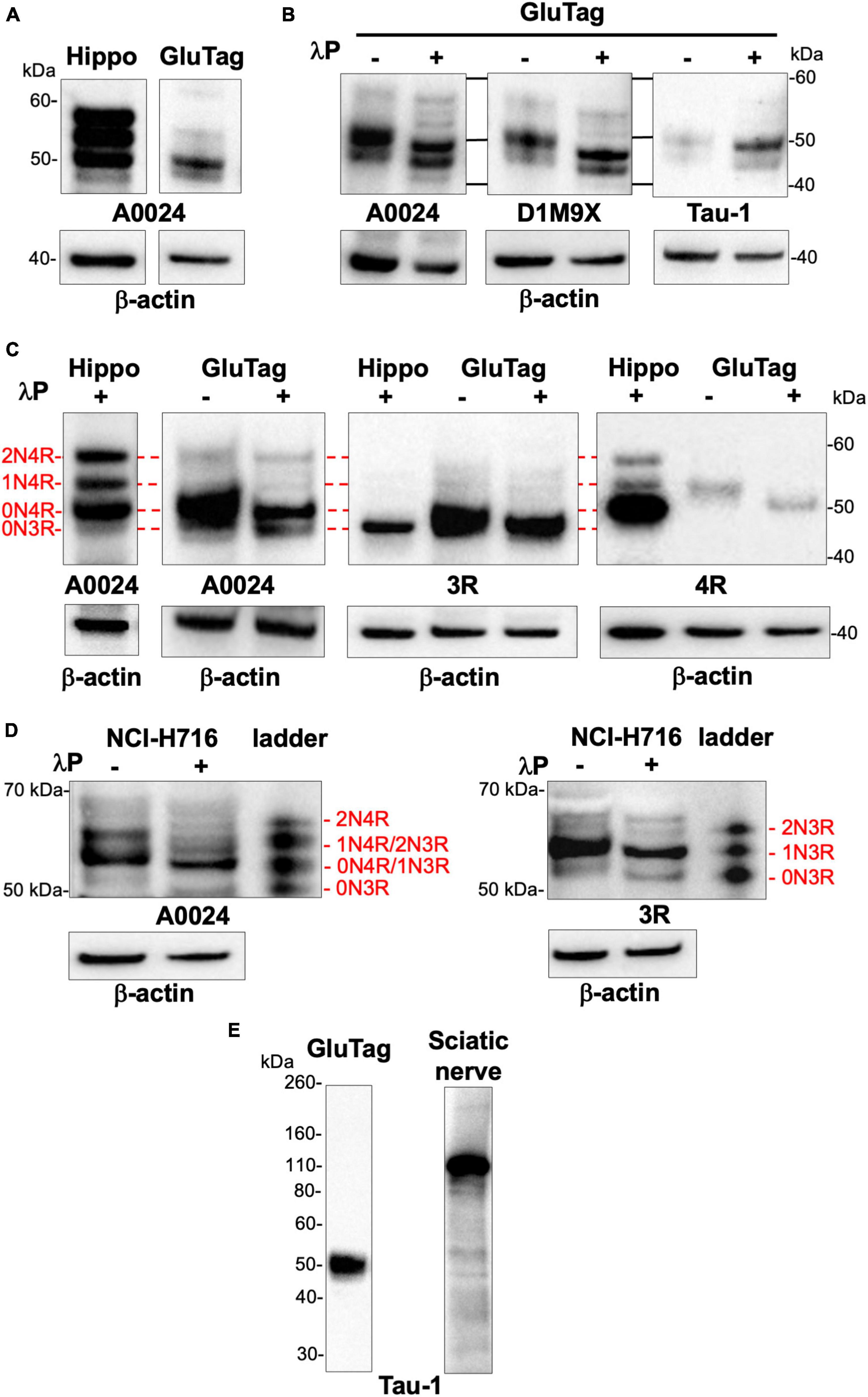
Figure 2. Tau protein isoforms and phosphorylation in EEC lines. (A) Hippocampus (2-month-old mice, Hippo) and GLUTag cell lysates were subjected to immunoblot analysis using the pan-Tau antibody A0024. (B) Lysates of GLUTag cell were treated (+) or not (–) with lambda phosphatase (λP) before immunoblotting with the pan-tau antibodies A0024, D1M9X, and Tau-1. (C) GLUTag cells lysates were treated (+) or not (–) with lambda phosphatase (λP) before immunoblotting with the tau isoform-specific antibodies 3R and 4R and the pan-tau antibody A0024. Hippocampus lysate from 2-month old mice dephosphorylated with lambda phosphatase (λP+), which contains all 4R isoforms and 0N3R isoform (McMillan et al., 2008; Liu and Götz, 2013) was used as a ladder to determine mouse tau isoform profile in GLUTag cells; the red lines show comigration. (D) NCI-H716 cell lysates were treated (+) or not (–) with lambda phosphatase (λP) before immunoblotting with the tau isoform-specific antibody 3R and the pan-tau antibody A0024. Tau ladder, which contains all 6 dephosphorylated human tau isoforms was used to determine tau isoform profile. (E) GLUTag cells lysates were subjected to immunoblot analysis using Tau-1 antibody. Rat sciatic nerve lysates were used as positive control to detect big tau. In all experiments, β-actin immunoblot was used as a loading control. The results shown in panels (A,C) are representative of 3 and 2 independent experiments, respectively. The results shown in panel (B) are representative of 7, 2, and 3 independent experiments for A0024, DM19X, and Tau-1 antibodies, respectively. The results shown in panels (D,E) are representative of 3 and 2 independent experiments, respectively.
Similar experiments were conducted with the NCI-H716 cell line. Treatment with lambda phosphatase caused tau dephosphorylation, as evidenced by a significant downward shift in mobility of the tau doublet detected when the pan-tau A0024 antibody was used (Figure 2D). After dephosphorylation, this tau doublet at 50 and 55 kDa comigrated with 0N3R and 1N3R/0N4R from the tau human ladder (Figure 2D). The isoform specific 3R antibody detected one major at band at 58 kDa and a fainter one around 55 kDa in dephosphorylated samples that comigrated with 1N3R and 0N3R isoforms of recombinant human tau, respectively (Figure 2D). No major bands were observed when the 4R-tau antibody was used (data not shown). As a whole, these results show that 1N3R and to a lesser extent 0N3R are the two main isoforms expressed in NCI-H716 cell.
“Big” or peripheral tau is a tau isoform specifically expressed in the peripheral nervous system, including sciatic nerve (Taleghany and Oblinger, 1992). It differs from the 2N4R tau isoform by a 254 amino-acid insert located in the amino-terminal half and migrates at 110 kDa on SDS/PAGE (Goedert et al., 1992b). As shown in Supplementary Figure 1, the uncropped images of the Western blots performed from Figure 2 with total tau antibodies (A0024, D1M9X, and Tau-1) did not show any additional bands at 110 kDa in either GLUTag and NCI-H716 cells, thereby suggesting that EEC do not express big tau. Additional experiments were performed with Tau-1 antibody, which has been previously shown to detect big tau (Davis and Johnson, 1999) and rat sciatic nerve to serve as positive controls (Taleghany and Oblinger, 1992). Tau-1 detected the expected low molecular weight tau isoforms around 50 kDa in GLUTag cells, however, a 110 kDa migrating band was only observed with rat sciatic nerve lysates (Figure 2E).
To further refine the analysis of tau isoforms in EEC, tau expression was analyzed at the mRNA level. To this end, RNA from both GLUTag and NCI-H716 was reverse transcribed to cDNA and amplified with PCR. The human epithelial cell line Caco-2 was used for comparison. Primers were designed, based on those previously described by Duff et al. (2000), to detect splicing of human and mouse tau exons 2, 3, and 10. This allowed amplification of products corresponding to 0N tau in GLUTag, 1N (and to a lesser extent 2N) in NCI-H716 (Figures 3A, B). No transcripts were observed in Caco-2 cells. Transcripts of 3R tau were also observed in GLUTag and NCI-H716, but not in Caco-2 cells when inclusion of exon 10 was assessed using primers specific to human tau exons 9 and 11 (Figure 3). Thus, the results obtained at the mRNA level confirm those obtained at the protein level and show that 0N3R and 1N3R are the mains isoforms expressed by GLUTag and NCI-H716 cells, respectively. They also show that human intestinal epithelial cells do not express tau.
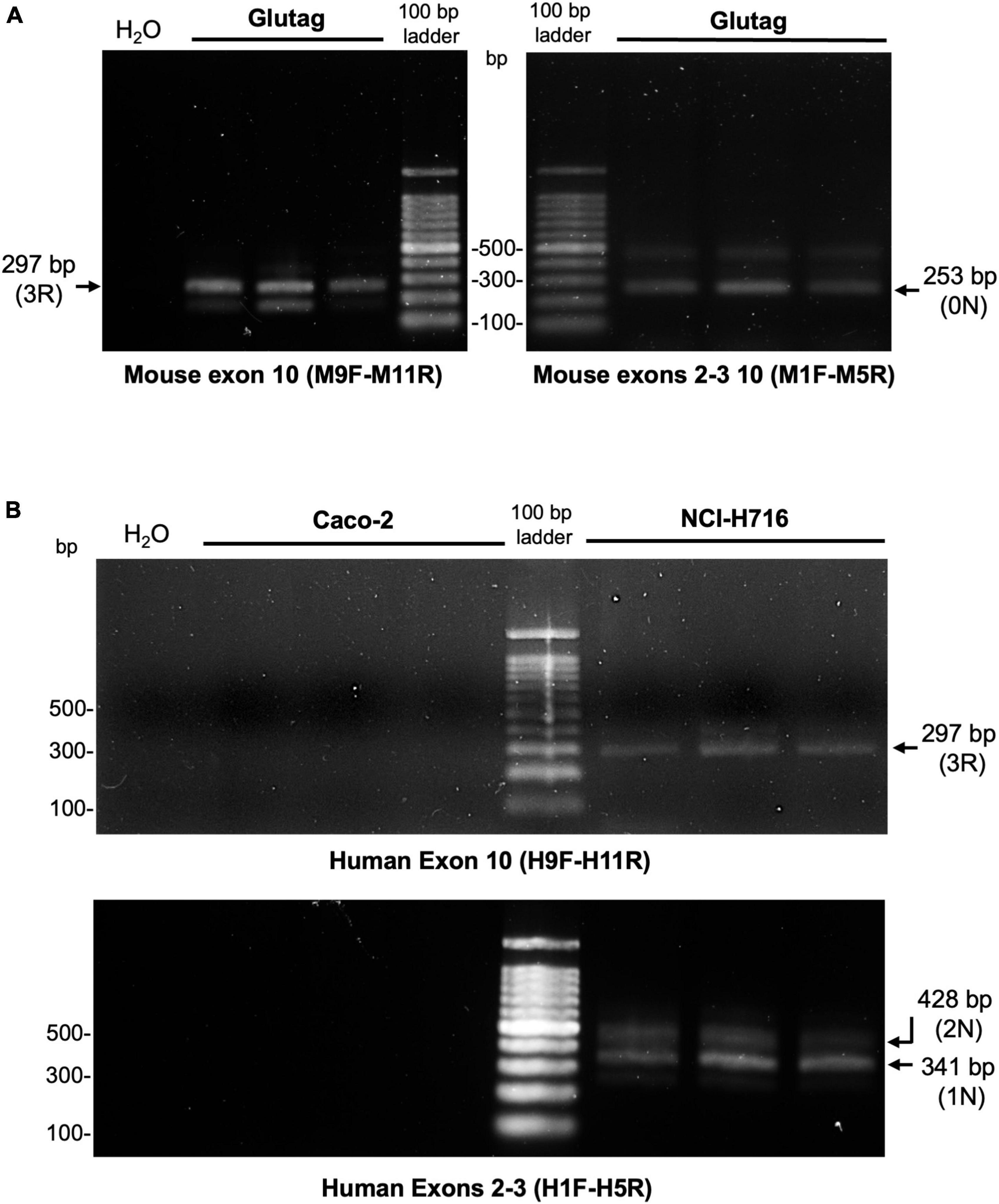
Figure 3. Detection of tau mRNA in EEC lines. Mtau and htau cDNA were amplified using PCR with mouse- and human-tau specific primers to detect the expression of exon 2–3- (0 N), 2 + 3- (1 N), 2 + 3 + (2 N), 10- (3R), and 10 + (4R) tau isoforms in GLUTag (A) and NCI-H716 cells (B), respectively. Gel (2% agarose) images are shown. cDNA from colonic Caco-2 cell line were used as negative control. Numbers correspond to base pairs of a DNA ladder. The expected positions of PCR products are indicated by an arrow. n = 3.
Tau phosphorylation is regulated by SCFA in EEC lines
Short-chain fatty acids act as ligands for G protein coupled receptors, which are expressed in EEC, where they mediate hormone release, such as PYY and GLP-1 (reviewed in Martin-Gallausiaux et al., 2021). The phosphorylation of tau at multiple serine and threonine sites has been described in both developing and adult brain and is the predominant mechanism by which tau functions are regulated (Guo et al., 2017). This logically led us to study the effects of SCFA on tau phosphorylation in EEC. To this end, GLUTag cell lines were treated with 1 mM of either propionate or butyrate at different time points from 10 to 180 min and cell lysates were analyzed by Western blot with an antibody specific for tau phosphorylated at Thr205. Treatment with propionate or butyrate for 180 min caused tau dephosphorylation at Thr205 when compared to control conditions (Figures 4A, B), without significant changes in tau expression (Figures 4A, B).
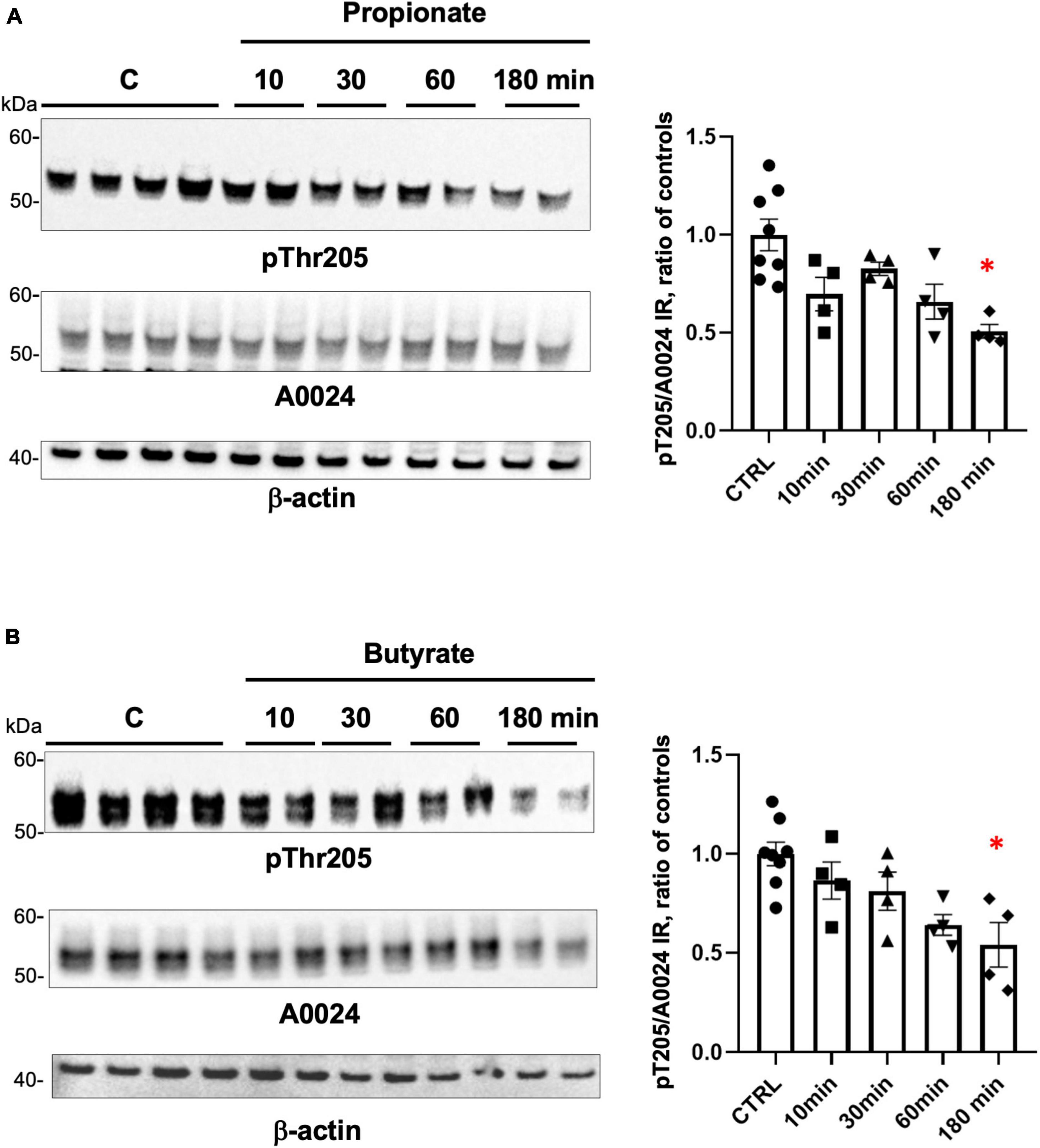
Figure 4. Regulation of tau phosphorylation by SCFA in GLUTag cells. (A) GLUTag cells were treated with 1 mM propionate for the indicated periods of time. Cell lysates were subjected to immunoblot analysis using antibodies against tau phosphorylated at Thr205 (pThr205) and total tau (A0024). Membranes were also probed with an anti-β-actin antibody to ensure equal protein loading. pThr205 immunoreactive bands were measured, normalized to the optical densities of total tau (A0024), and expressed as ratio of controls. Data correspond to mean ± SEM (n = 4–8, n indicates the number of wells; *p < 0.05, 180 min-treated vs control). (B) GLUTag cells were treated with 1 mM butyrate for the indicated periods of time. Cell lysates were analyzed as in panel (A) and quantification was performed as in panel (A). Data correspond to mean ± SEM (n = 4–8, n indicates the number of wells; *p < 0.05, 180 min-treated vs. control).
Discussion
Tau is a microtubule-associated protein for which the physiological functions are still a topic of intense investigation (Guo et al., 2017). Additionally, in pathological conditions tau is a key player in the pathogenesis of several diseases collectively referred to as tauopathies including AD and PSP (Guo et al., 2017). In the adult brain, tau is classically described as a neuronal protein specifically localized and highly enriched in axons but precise localization studies showed that tau distribution in the mature CNS is more widespread than initially thought, with expression in the somatodendritic compartment of neurons as well as in glial cells (Kanaan and Grabinski, 2021). Besides the CNS, the presence of tau has been demonstrated in several non-neuronal cells, such as monocytes (Kim et al., 1991), lymphocytes (Kvetnoy et al., 2000), testicular spermatids (Ashman et al., 1992), podocytes (Vallés-Saiz et al., 2022), pancreatic beta cells (Maj et al., 2016) as well as in peripheral neurons, including enteric neurons (Lionnet et al., 2018). Here, we show for the first time that tau is expressed in EEC, not only in the adult human colon but also in two EEC lines, namely GLUTag and NCI-H716.
Earlier studies showed that EEC exhibit neuronal features with the presence of axon-like basal processes and the expression of neuronal proteins such as synapsin 1, PGP9.5 and neurofilaments (Bohórquez et al., 2014, 2015). Our identification of tau in EEC further expands the neuronal repertoire of EEC and echoes recent publications which showed that alpha-synuclein is also expressed in EEC (Chandra et al., 2017; Casini et al., 2021; Amorim Neto et al., 2022). The observation that alpha-synuclein EEC lie in close proximity to alpha-synuclein–expressing enteric neurons led Liddle and collaborators to posit that the EEC might be critically involved in the circuit between the gut lumen and the brain for the bottom-up propagation of PD pathology (Chandra et al., 2017). Our current findings together with our previous data showing that enteric neurons express tau (Lionnet et al., 2018) suggest that such a scenario could also occur in tauopathies. It could thus be suggested that an hitherto unidentified substance from the gut lumen could induce changes in tau conformation in EEC, leading to a prion-like spreading of tau from EEC to enteric neurons, as already described between CNS neurons (Clavaguera et al., 2009; Frost et al., 2009). It should be however borne in mind that, unlike PD and synucleinopathies (De Guilhem De Lataillade et al., 2020), all existing studies suggest that pathological tau species are not observed in the gut of subjects with either AD or PSP (Shankle et al., 1993; Lionnet et al., 2018; Dugger et al., 2019). Further studies are therefore necessary to determine if pathological tau species are present in the gut of patients with tauopathies, either in enteric nerves or EEC (Derkinderen et al., 2021). Regarding the EEC, the identification of these potential pathological tau species in the gut could greatly benefit from novel approaches, for example by combining laser capture microdissection of EEC (Blatt and Srinivasan, 2008) with ultrasensitive amplification techniques of aggregated proteins such as real-time quaking-induced conversion (Wu et al., 2022).
Six isoforms of tau are expressed in adult human brain by alternative splicing from a single gene. Regulated inclusion of exons 2 and 3 yields tau isoforms with 0, 1, or 2 N-terminal inserts (0N, 1N, and 2N, respectively), whereas exclusion or inclusion of exon 10 leads to expression of tau isoforms with three (3R) or four (4R) microtubule-binding repeats. The various splice combinations of tau are thus abbreviated-0N3R, 0N4R, 1N3R, 1N4R, 2N3R, 2N4R-encoding six proteins isoforms ranging from 352 to 441 amino acids in length (Goedert and Jakes, 1990). In the current study, we identified 0N3R/0N4R and 0N3R/1N3R as the two main tau isoforms expressed in GLUTag and NCI-H716 cell lines. The reasons why EEC lines only express a subset of tau isoforms remains to be determined but the observation showing that the intracellular sorting of tau in different cell compartments is isoform-dependent may provide a clue (Liu and Götz, 2013). It has been indeed reported that 0N, 1N and 2N isoforms are primarily localized to the cell bodies, nucleus, and axons, respectively (Liu and Götz, 2013), thereby suggesting that tau in EEC lines is primarily cytoplasmic. In order to properly compare the isoform profile of tau between human adult brain and EEC from human adult colon, it would have been useful to study in more detail tau isoforms in human EEC. We were however unable to do so for two main reasons: first, apart from 3R and 4R antibodies, other specific antibodies for 0N, 1N, and 2N isoforms did not work well for immunohistochemistry on paraffin sections, at least in our hands; second, in contrast to our previous research on the ENS, the use of frozen colonic sections for Western blot analysis was not possible because of the sparse distribution of EEC along the intestinal epithelium (Lionnet et al., 2018). Again, this precise characterization could benefit from laser microdissection approaches (Blatt and Srinivasan, 2008).
The phosphorylation of tau at multiple serine and threonine sites is the predominant mechanism by which tau functions are regulated (Guo et al., 2017). Dephosphorylation of tau from EEC lines produced a downward shift demonstrating that EEC-tau is, like CNS tau, phosphorylated (Hanger et al., 2002). Using a phospho-specific antibody, we further showed that tau was phosphorylated at Thr205 in GLUTag cells under basal condition and that phosphorylation at this residue was regulated by SCFA. What can be the role of tau and the consequences of tau phosphorylation from a functional point of view? In this regard, it is tempting to compare our findings to those obtained in pancreatic β-cells. EEC and pancreatic β-cells share similar pathways of differentiation during embryonic development (Ryu et al., 2018). Remarkably, like EEC, pancreatic β-cells, also express tau (Maj et al., 2016; Wijesekara et al., 2018) and several studies showed that tau is critically involved in pancreatic β-cells function, insulin secretion and glucose homeostasis (Maj et al., 2016; Wijesekara et al., 2018). One might therefore suggest that, EEC-tau similarly regulates the secretion of peptide-hormones and that such a regulation is mediated via tau phosphorylation. Further experiments performed after silencing tau in EEC will be needed to answer this question.
Conclusion
In conclusion, we have characterized tau in the human colon and in EEC lines and we show that EEC tau phosphorylation can be regulated by SCFA. The data we have acquired on tau in EEC strongly supports additional future studies aimed at expanding our knowledge of peripheral pathology in tauopathies and at deciphering the physiological role of tau in CEE.
Data availability statement
The raw data supporting the conclusions of this article will be made available by the authors, without undue reservation.
Ethics statement
The studies involving human participants were reviewed and approved by the Fédération des Biothèques of the University Hospital of Nantes, according to the guidelines of the French Ethics Committee for Research on Humans and registered under the no. DC-2008-402. The patients/participants provided their written informed consent to participate in this study.
Author contributions
GC, NB, BC, IG, PC, and TO performed the experiments. MR-D managed the tissue sampling and biobanking. MR-D, GL, and PD supervised the study and wrote the final version of the manuscript. MN and HB provided critical feedback and helped shape the research. PD wrote the first draft of the manuscript. All authors contributed to the article and approved the submitted version.
Acknowledgments
We acknowledge the IBISA MicroPICell Facility (Biogenouest), Member of the National Infrastructure France-Bioimaging supported by the French National Research Agency (ANR-10-INBS-04) for confocal microscopy pictures.
Conflict of interest
The authors declare that the research was conducted in the absence of any commercial or financial relationships that could be construed as a potential conflict of interest.
Publisher’s note
All claims expressed in this article are solely those of the authors and do not necessarily represent those of their affiliated organizations, or those of the publisher, the editors and the reviewers. Any product that may be evaluated in this article, or claim that may be made by its manufacturer, is not guaranteed or endorsed by the publisher.
Supplementary material
The Supplementary Material for this article can be found online at: https://www.frontiersin.org/articles/10.3389/fnins.2023.1166848/full#supplementary-material
References
Amorim Neto, D. P., Bosque, B. P., Pereira de Godoy, J. V., Rodrigues, P. V., Meneses, D. D., Tostes, K., et al. (2022). Akkermansia muciniphila induces mitochondrial calcium overload and α -synuclein aggregation in an enteroendocrine cell line. iScience 25:103908. doi: 10.1016/j.isci.2022.103908
Arai, Y., Yamazaki, M., Mori, O., Muramatsu, H., Asano, G., and Katayama, Y. (2001). Alpha-synuclein-positive structures in cases with sporadic Alzheimer’s disease: Morphology and its relationship to tau aggregation. Brain Res. 888, 287–296. doi: 10.1016/s0006-8993(00)03082-1
Ashman, J. B., Hall, E. S., Eveleth, J., and Boekelheide, K. (1992). Tau, the neuronal heat-stable microtubule-associated protein, is also present in the cross-linked microtubule network of the testicular spermatid manchette. Biol. Reprod. 46, 120–129. doi: 10.1095/biolreprod46.1.120
Blatt, R., and Srinivasan, S. (2008). Defining disease with laser precision: Laser capture microdissection in gastroenterology. Gastroenterology 135, 364–369. doi: 10.1053/j.gastro.2008.06.054
Bohórquez, D. V., Samsa, L. A., Roholt, A., Medicetty, S., Chandra, R., and Liddle, R. A. (2014). An enteroendocrine cell-enteric glia connection revealed by 3D electron microscopy. PLoS One 9:e89881. doi: 10.1371/journal.pone.0089881
Bohórquez, D. V., Shahid, R. A., Erdmann, A., Kreger, A. M., Wang, Y., Calakos, N., et al. (2015). Neuroepithelial circuit formed by innervation of sensory enteroendocrine cells. J. Clin. Invest. 125, 782–786. doi: 10.1172/JCI78361
Borghammer, P., and Van Den Berge, N. (2019). Brain-first versus gut-first Parkinson’s Disease: A hypothesis. J. Parkinsons Dis. 9, S281–S295. doi: 10.3233/JPD-191721
Braak, H., de Vos, R. A. I., Bohl, J., and Del Tredici, K. (2006). Gastric alpha-synuclein immunoreactive inclusions in Meissner’s and Auerbach’s plexuses in cases staged for Parkinson’s disease-related brain pathology. Neurosci. Lett. 396, 67–72. doi: 10.1016/j.neulet.2005.11.012
Casini, A., Mancinelli, R., Mammola, C. L., Pannarale, L., Chirletti, P., Onori, P., et al. (2021). Distribution of α-synuclein in normal human jejunum and its relations with the chemosensory and neuroendocrine system. Eur. J. Histochem. 65:3310. doi: 10.4081/ejh.2021.3310
Chandra, R., Hiniker, A., Kuo, Y.-M., Nussbaum, R. L., and Liddle, R. A. (2017). α-Synuclein in gut endocrine cells and its implications for Parkinson’s disease. JCI Insight 2:92295. doi: 10.1172/jci.insight.92295
Clavaguera, F., Bolmont, T., Crowther, R. A., Abramowski, D., Frank, S., Probst, A., et al. (2009). Transmission and spreading of tauopathy in transgenic mouse brain. Nat. Cell Biol. 11, 909–913. doi: 10.1038/ncb1901
Coughlin, D., Xie, S. X., Liang, M., Williams, A., Peterson, C., Weintraub, D., et al. (2019). Cognitive and pathological influences of tau pathology in lewy body disorders. Ann. Neurol. 85, 259–271. doi: 10.1002/ana.25392
Davis, D. R., Brion, J. P., Couck, A. M., Gallo, J. M., Hanger, D. P., Ladhani, K., et al. (1995). The phosphorylation state of the microtubule-associated protein tau as affected by glutamate, colchicine and beta-amyloid in primary rat cortical neuronal cultures. Biochem. J. 309(Pt 3), 941–949. doi: 10.1042/bj3090941
Davis, P. K., and Johnson, G. V. (1999). Energy metabolism and protein phosphorylation during apoptosis: A phosphorylation study of tau and high-molecular-weight tau in differentiated PC12 cells. Biochem. J. 340(Pt 1), 51–58.
De Guilhem De Lataillade, A., Lebouvier, T., Noble, W., Leclair-Visonneau, L., and Derkinderen, P. (2020). Enteric synucleinopathy: Real entity or only a trendy concept? Free Neuropathol. 1:26. doi: 10.17879/FREENEUROPATHOLOGY-2020-2920
Derkinderen, P., Rolli-Derkinderen, M., Chapelet, G., Neunlist, M., and Noble, W. (2021). Tau in the gut, does it really matter? J. Neurochem. 158, 94–104. doi: 10.1111/jnc.15320
Duff, K., Knight, H., Refolo, L. M., Sanders, S., Yu, X., Picciano, M., et al. (2000). Characterization of pathology in transgenic mice over-expressing human genomic and cDNA tau transgenes. Neurobiol. Dis. 7, 87–98. doi: 10.1006/nbdi.1999.0279
Dugger, B. N., and Dickson, D. W. (2017). Pathology of neurodegenerative diseases. Cold Spring Harb. Perspect. Biol. 9:a028035. doi: 10.1101/cshperspect.a028035
Dugger, B. N., Hoffman, B. R., Scroggins, A., Serrano, G. E., Adler, C. H., Shill, H. A., et al. (2019). Tau immunoreactivity in peripheral tissues of human aging and select tauopathies. Neurosci. Lett. 696, 132–139. doi: 10.1016/j.neulet.2018.12.031
Eissele, R., Göke, R., Willemer, S., Harthus, H. P., Vermeer, H., Arnold, R., et al. (1992). Glucagon-like peptide-1 cells in the gastrointestinal tract and pancreas of rat, pig and man. Eur. J. Clin. Invest. 22, 283–291. doi: 10.1111/j.1365-2362.1992.tb01464.x
Ercan, E., Eid, S., Weber, C., Kowalski, A., Bichmann, M., Behrendt, A., et al. (2017). A validated antibody panel for the characterization of tau post-translational modifications. Mol. Neurodegener. 12:87. doi: 10.1186/s13024-017-0229-1
Frost, B., Jacks, R. L., and Diamond, M. I. (2009). Propagation of tau misfolding from the outside to the inside of a cell. J. Biol. Chem. 284, 12845–12852. doi: 10.1074/jbc.M808759200
Goedert, M., Spillantini, M. G., Cairns, N. J., and Crowther, R. A. (1992a). Tau proteins of Alzheimer paired helical filaments: Abnormal phosphorylation of all six brain isoforms. Neuron 8, 159–168. doi: 10.1016/0896-6273(92)90117-v
Goedert, M., and Jakes, R. (1990). Expression of separate isoforms of human tau protein: Correlation with the tau pattern in brain and effects on tubulin polymerization. EMBO J. 9, 4225–4230.
Goedert, M., Spillantini, M. G., and Crowther, R. A. (1992b). Cloning of a big tau microtubule-associated protein characteristic of the peripheral nervous system. Proc. Natl. Acad. Sci. U. S. A. 89, 1983–1987. doi: 10.1073/pnas.89.5.1983
Goldspink, D. A., Reimann, F., and Gribble, F. M. (2018). Models and tools for studying enteroendocrine cells. Endocrinology 159, 3874–3884. doi: 10.1210/en.2018-00672
Gribble, F. M., and Reimann, F. (2019). Function and mechanisms of enteroendocrine cells and gut hormones in metabolism. Nat. Rev. Endocrinol. 15, 226–237. doi: 10.1038/s41574-019-0168-8
Guo, T., Noble, W., and Hanger, D. P. (2017). Roles of tau protein in health and disease. Acta Neuropathol. 133, 665–704. doi: 10.1007/s00401-017-1707-9
Hanger, D. P., Gibb, G. M., de Silva, R., Boutajangout, A., Brion, J.-P., Revesz, T., et al. (2002). The complex relationship between soluble and insoluble tau in tauopathies revealed by efficient dephosphorylation and specific antibodies. FEBS Lett. 531, 538–542. doi: 10.1016/s0014-5793(02)03611-6
Kaelberer, M. M., Buchanan, K. L., Klein, M. E., Barth, B. B., Montoya, M. M., Shen, X., et al. (2018). A gut-brain neural circuit for nutrient sensory transduction. Science 361:eaat5236. doi: 10.1126/science.aat5236
Kanaan, N. M., and Grabinski, T. (2021). Neuronal and glial distribution of tau protein in the adult rat and monkey. Front. Mol. Neurosci. 14:607303. doi: 10.3389/fnmol.2021.607303
Kim, H., Strong, T. V., and Anderson, S. J. (1991). Evidence for tau expression in cells of monocyte lineage and its in vitro phosphorylation by v-fms kinase. Oncogene 6, 1085–1087.
Kvetnoy, I. M., Hernandez-Yago, J., Kvetnaia, T. V., Khavinson, V. K., Malinin, V. V., Yarilin, A. A., et al. (2000). Tau-protein expression in human blood lymphocytes: A promising marker and suitable sample for life-time diagnosis of Alzheimer’s disease. Neuro Endocrinol. Lett. 21, 313–318.
Larraufie, P., Martin-Gallausiaux, C., Lapaque, N., Dore, J., Gribble, F. M., Reimann, F., et al. (2018). SCFAs strongly stimulate PYY production in human enteroendocrine cells. Sci. Rep. 8:74. doi: 10.1038/s41598-017-18259-0
Latorre, R., Sternini, C., De Giorgio, R., and Greenwood-Van Meerveld, B. (2016). Enteroendocrine cells: A review of their role in brain-gut communication. Neurogastroenterol. Motil. 28, 620–630. doi: 10.1111/nmo.12754
Lionnet, A., Wade, M. A., Corbillé, A.-G., Prigent, A., Paillusson, S., Tasselli, M., et al. (2018). Characterisation of tau in the human and rodent enteric nervous system under physiological conditions and in tauopathy. Acta Neuropathol. Commun. 6:65. doi: 10.1186/s40478-018-0568-3
Liu, C., and Götz, J. (2013). Profiling murine tau with 0N, 1N and 2N isoform-specific antibodies in brain and peripheral organs reveals distinct subcellular localization, with the 1N isoform being enriched in the nucleus. PLoS One 8:e84849. doi: 10.1371/journal.pone.0084849
Liu, W. K., Moore, W. T., Williams, R. T., Hall, F. L., and Yen, S. H. (1993). Application of synthetic phospho- and unphospho- peptides to identify phosphorylation sites in a subregion of the tau molecule, which is modified in Alzheimer’s disease. J. Neurosci. Res. 34, 371–376. doi: 10.1002/jnr.490340315
Maj, M., Hoermann, G., Rasul, S., Base, W., Wagner, L., and Attems, J. (2016). The microtubule-associated protein tau and its relevance for pancreatic beta cells. J. Diabetes Res. 2016:1964634. doi: 10.1155/2016/1964634
Martin-Gallausiaux, C., Marinelli, L., Blottière, H. M., Larraufie, P., and Lapaque, N. (2021). SCFA: Mechanisms and functional importance in the gut. Proc. Nutr. Soc. 80, 37–49. doi: 10.1017/S0029665120006916
McMillan, P., Korvatska, E., Poorkaj, P., Evstafjeva, Z., Robinson, L., Greenup, L., et al. (2008). Tau isoform regulation is region- and cell-specific in mouse brain. J. Comp. Neurol. 511, 788–803. doi: 10.1002/cne.21867
Ryu, G. R., Lee, E., Kim, J. J., Moon, S.-D., Ko, S.-H., Ahn, Y.-B., et al. (2018). Comparison of enteroendocrine cells and pancreatic β-cells using gene expression profiling and insulin gene methylation. PLoS One 13:e0206401. doi: 10.1371/journal.pone.0206401
Shankle, W. R., Landing, B. H., Ang, S. M., Chui, H., Villarreal-Engelhardt, G., and Zarow, C. (1993). Studies of the enteric nervous system in Alzheimer disease and other dementias of the elderly: Enteric neurons in Alzheimer disease. Mod. Pathol. 6, 10–14.
Szendrei, G. I., Lee, V. M., and Otvos, L. (1993). Recognition of the minimal epitope of monoclonal antibody Tau-1 depends upon the presence of a phosphate group but not its location. J. Neurosci. Res. 34, 243–249. doi: 10.1002/jnr.490340212
Taleghany, N., and Oblinger, M. M. (1992). Regional distribution and biochemical characteristics of high molecular weight tau in the nervous system. J. Neurosci. Res. 33, 257–265. doi: 10.1002/jnr.490330209
Vallés-Saiz, L., Peinado-Cahuchola, R., Ávila, J., and Hernández, F. (2022). Microtubule-associated protein tau in murine kidney: Role in podocyte architecture. Cell Mol. Life Sci. 79:97. doi: 10.1007/s00018-021-04106-z
Wijesekara, N., Gonçalves, R. A., Ahrens, R., De Felice, F. G., and Fraser, P. E. (2018). Tau ablation in mice leads to pancreatic β cell dysfunction and glucose intolerance. FASEB J. 32, 3166–3173. doi: 10.1096/fj.201701352
Wilson, B. S., and Lloyd, R. V. (1984). Detection of chromogranin in neuroendocrine cells with a monoclonal antibody. Am. J. Pathol. 115, 458–468.
Keywords: enteroendocrine cells, tau, short-chain fatty acids, gut-brain axis, tauopathies, gastrointestinal tract
Citation: Chapelet G, Béguin N, Castellano B, Grit I, de Coppet P, Oullier T, Neunlist M, Blottière H, Rolli-Derkinderen M, Le Dréan G and Derkinderen P (2023) Tau expression and phosphorylation in enteroendocrine cells. Front. Neurosci. 17:1166848. doi: 10.3389/fnins.2023.1166848
Received: 15 February 2023; Accepted: 17 May 2023;
Published: 02 June 2023.
Edited by:
Einar M. Sigurdsson, New York University, United StatesReviewed by:
Naruhiko Sahara, National Institutes for Quantum and Radiological Science and Technology, JapanErin Congdon, New York University, United States
Copyright © 2023 Chapelet, Béguin, Castellano, Grit, de Coppet, Oullier, Neunlist, Blottière, Rolli-Derkinderen, Le Dréan and Derkinderen. This is an open-access article distributed under the terms of the Creative Commons Attribution License (CC BY). The use, distribution or reproduction in other forums is permitted, provided the original author(s) and the copyright owner(s) are credited and that the original publication in this journal is cited, in accordance with accepted academic practice. No use, distribution or reproduction is permitted which does not comply with these terms.
*Correspondence: Malvyne Rolli-Derkinderen, bWFsdnluZS5kZXJraW5kZXJlbkB1bml2LW5hbnRlcy5mcg==; orcid.org/0000-0002-3535-8761; Pascal Derkinderen, cGFzY2FsLmRlcmtpbmRlcmVuQHVuaXYtbmFudGVzLmZy, ZGVya2luZGVyZW5wQHlhaG9vLmZy; orcid.org/0000-0001-8792-2582
†These authors have contributed equally to this work and share first authorship
‡These authors have contributed equally to this work and share last authorship