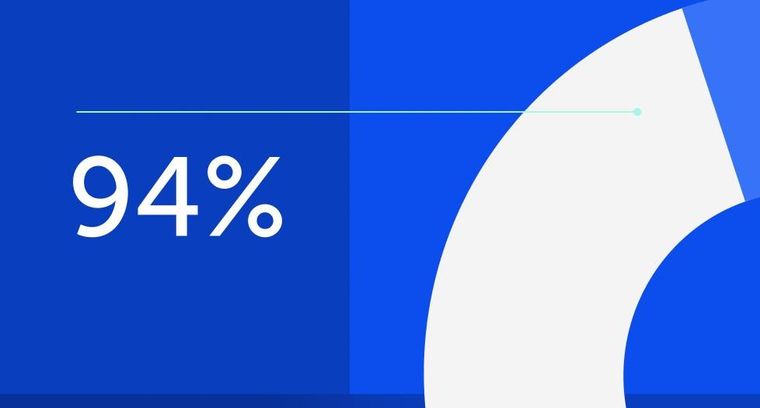
94% of researchers rate our articles as excellent or good
Learn more about the work of our research integrity team to safeguard the quality of each article we publish.
Find out more
MINI REVIEW article
Front. Neurosci., 25 April 2023
Sec. Sleep and Circadian Rhythms
Volume 17 - 2023 | https://doi.org/10.3389/fnins.2023.1165230
This article is part of the Research TopicDevelopment of Circadian Clock Functions, volume IIView all 11 articles
Glucocorticoids (GCs) are essential drivers of mammalian tissue growth and maturation during one of the most critical developmental windows, the perinatal period. The developing circadian clock is shaped by maternal GCs. GC deficits, excess, or exposure at the wrong time of day leads to persisting effects later in life. During adulthood, GCs are one of the main hormonal outputs of the circadian system, peaking at the beginning of the active phase (i.e., the morning in humans and the evening in nocturnal rodents) and contributing to the coordination of complex functions such as energy metabolism and behavior, across the day. Our article discusses the current knowledge on the development of the circadian system with a focus on the role of GC rhythm. We explore the bidirectional interaction between GCs and clocks at the molecular and systemic levels, discuss the evidence of GC influence on the master clock in the suprachiasmatic nuclei (SCN) of the hypothalamus during development and in the adult system.
Circadian rhythms are controlled by a clock system that conveys ~24 h rhythmicity to almost every physiological function in a living organism. When (and how) does the clock system really start ticking, and more specifically, which cells and mechanisms are involved, are long-standing questions in the field of chronophysiology. In adult mammals, circadian timekeeping is organized hierarchically with a central clock synchronizing subordinated clocks in all tissues to ensure that complex functions such as activity (Moore and Eichler, 1972), sleep (Collins et al., 2020), food intake (Nagai et al., 1978; Koch et al., 2020), and stress responses (Oster et al., 2006; Son et al., 2008) are efficiently adapted to a rhythmic environment.
The master pacemaker of the circadian system is located in the hypothalamic suprachiasmatic nucleus (SCN) (Lehman et al., 1987; Romero et al., 1993; Silver et al., 1996). Environmental factors that set the time (or entrain) internal clocks are called zeitgebers (from the German term for “time givers”). The main zeitgeber for the SCN is ambient light which reaches the retina and, in turn, the SCN through the retino-hypothalamic tract (RHT) (Ibuka et al., 1977; Hannibal et al., 2000).
The SCN has been traditionally described as a nucleus of around 20,000 neurons localized on each side of the third ventricle. It is topologically divided into “ventral core” and “dorsal shell” (Hastings et al., 2018). Neurons in the core receive direct photic input from the retina and transmit this signal to the surrounding shell. The neurons in the SCN shell, that in absence of light show autonomous activity with an endogenous period of ~24 h, are then entrained and translate their synchrony by efferent connections to subordinated clocks in multiple brain regions including, importantly, clocks within the medial hypothalamus, where key cell groups organize hormone release and set the tone of the autonomic nervous system (Dibner et al., 2010; Kalsbeek et al., 2011). Interestingly, this neuron-centered view changed recently when astrocyte clocks were found to be partially necessary and completely sufficient to restore neuronal circadian function in the SCN (Brancaccio et al., 2019). Rather than being “mere support cells” for the SCN neuronal circuit, they play an essential role as time-keepers of the adult master clock (Barca-Mayo et al., 2017; Brancaccio et al., 2017, 2019; Tso et al., 2017).
Glucocorticoids (GC) are examples of rhythmic hormonal outputs tightly regulated by the SCN and well-known mediators of circadian entrainment in the peripheral tissues (Pevet and Challet, 2011; Pezük et al., 2012). The circadian regulation of GC release is a result of close interaction between the SCN and clocks along the hypothalamus-pituitary–adrenal (HPA) axis (Oster et al., 2006; Son et al., 2008). Efferences from the SCN influence the release of corticotropin-releasing hormone (CRH) by the paraventricular nucleus of the hypothalamus (PVN), both inhibitory and stimulatory inputs from the SCN, depending on fluctuating arginine vasopressin (AVP) levels, are responsible of generating the complete daily profile of GCs (Kalsbeek et al., 1992, 1996; Buijs et al., 1993). The PVN controls the rhythmic secretion of adrenocorticotropic hormone (ACTH) from the pituitary and, consequently, GC production by the adrenal gland. Via autonomic pathways the SCN-PVN synchronize adrenal clocks regulating the time-of-day-dependent sensitivity of the adrenal to ACTH stimulation (Oster et al., 2006; Son et al., 2008). As a result, GCs are peaking at the beginning of the active phase to coordinate complex functions when high energy demands are expected (Balsalobre et al., 2000). For instance, GCs promote glucose and lipid mobilization through gluconeogenesis in the liver and the release of free fatty acids from adipocytes. GC rhythms are key regulators of mood and cognition, demonstrated by the improvement of learning skills during GC peak (Liston et al., 2013) and the impairment of memory retrieval when circadian GC rise is blocked pharmacologically (Rimmele et al., 2010).
Compared to the knowledge we have on the adult circadian system, little is known about its assembly and function during development. The view of the fetal hypothalamic clock as a “dependent” oscillator entrained by maternal rhythmic signals has been questioned by our own findings and by others (Landgraf et al., 2015; Astiz et al., 2020; Lužná et al., 2020; Greiner et al., 2022). In mice, the SCN circuit develops, matures, and connects to afferent and efferent pathways around birth (Munekawa et al., 2000; Sekaran et al., 2005; Kabrita and Davis, 2008). However, some early rhythmic genes of the molecular clock machinery, such as RevErbα, are already expressed in the hypothalamus earlier (Čečmanová et al., 2019; Astiz et al., 2020; Carmona-Alcocer et al., 2020). Since both, astrocytes and neurons, display strong circadian rhythmicity, it seems possible that the two could promote the gain of autonomous function of the developing SCN and participate in organizing the coupling between the SCN and the HPA axis, essential for the adaptation to the environment after birth (Astiz and Oster, 2018). Extensive evidence suggests that during this critical developmental window, the fetal/neonatal SCN and HPA axis are susceptible to GCs. An excess, a deficit or even the exposure at the wrong time of day can have persisting effects later on (Moisiadis and Matthews, 2014a; Busada and Cidlowski, 2017; Astiz et al., 2020).
The strong coupling between the clock and the stress system is nourished by a solid bidirectional interaction at the molecular level. GCs activate, in target cells, intracellular GC receptors (GR) and mineralocorticoid receptors (MR). The primary ligands of GR are cortisol and other GCs (i.e., corticosterone, the main GC in rodents), while the primary ligand of MR is aldosterone in the periphery and corticosterone in the central nervous system (CNS) (Fuller et al., 2012). While GR is widely distributed within the body and especially abundant in the brain and the pituitary, MR is prominently present in peripheral tissues and in the brain, the expression is high in the magnocellular neurons and pre-sympathetic neurons of the hypothalamic paraventricular nuclei (PVN) as well as in the hippocampus and cerebral cortex (Oyamada et al., 2008; Fuller et al., 2019). Since MR has a 10-fold higher affinity for GCs than GR, it becomes active even at nadir GC levels (Kolber et al., 2008; Daskalakis et al., 2022). In contrast, GR is only activated at higher GC concentrations, such as those reached during the circadian peak or after stress-induced HPA axis activation (Kolber et al., 2008). The presence of both GR and MR in the neurons of the SCN of adult mice and humans remains a controversial (Rosenfeld et al., 1988; Olejníková et al., 2018). The genes encoding for GR and MR are not expressed in adult SCN neurons in rodents and humans.1
GR acts as a ligand-activated transcription factor. In the inactive/unbound state, GR locates in the cytosol bound to the N-terminal and middle region of a chaperone complex containing Heat shock protein 90 (HSP90) (Antunica-Noguerol et al., 2016; Fries et al., 2017; Mazaira and Galigniana, 2019). HSP90 does not form part of the active receptor complex, but is important for correct GR protein folding and stability (Biebl and Buchner, 2019). The HSP90 complex is also formed by co-chaperones important for GR stabilization and feedback, such as FK506-binding protein 5 (FKBP5) (Binder, 2009; Biebl and Buchner, 2019). Fkbp5 is transcriptionally induced by GC-GR and, in turn, regulates GR sensitivity through an intracellular negative feedback loop, essentially keeping inactive GR in the cytosol (Pratt and Toft, 1997; Nicolaides et al., 2000; Binder, 2009; Klengel et al., 2013; Criado-Marrero et al., 2018). Upon ligand binding, GR monomers dimerize (either with other GR monomer or with MR) and translocate to the nucleus where the dimer binds the DNA at GC response elements (GREs), regulatory regions of target genes (George et al., 2017; Oakley et al., 2021). Through binding of GREs, the transcription of GR target genes is modulated positively or negatively (Reddy et al., 2009; Surjit et al., 2011). Besides this canonical signaling cascade, GR and MR activation have shown rapid non-genomic changes, mediated by membrane-anchored GR/MR that might mediate the coordination of rapid adaptive responses to stress (Groeneweg et al., 2012).
The clock gene machinery is present in almost every cell of the body and reciprocal interaction with GR is found at transcriptional, translational, and post-translational levels (summarized in Figure 1; Nader et al., 2010). The molecular clock machinery works as an interlocked transcriptional-translational-feedback loop (Takahashi, 2017). The positive members of the loop: BMAL1 (brain and muscle aryl hydrocarbon receptor nuclear translocator-like 1) and CLOCK (circadian locomotor output cycles kaput) heterodimerize and activate the transcription of their repressors Per1/2 (Period 1/2), Cry1/2 (Cryptochrome 1/2) through binding of E-Box promoter elements. Upon translation, PER and CRY form complexes in the cytosol, translocate into the nucleus and repress CLOCK/BMAL1 activity, shutting down their own transcription. After clearance of PER/CRY complexes, the inhibition of CLOCK/BMAL1 is released and a new circadian (~24 h) cycle begins. The cycle is further stabilized by accessory loops in which CLOCK/BMAL1 drive the circadian expression of Dec 1/2 and nuclear receptors of the ROR and the REV-ERB family (Takahashi, 2017).
Figure 1. Scheme of the reciprocal molecular interaction between GR and the clock machinery at transcriptional, translational, and post-translational levels as described in detail in the text. BMAL1: brain and muscle aryl hydrocarbon receptor nuclear translocator-like 1. CLOCK: circadian locomotor output cycles kaput. Pers: Periods. Dec2: differentially expressed in Chondrocytes 2. Npas2: Neuronal PAS Domain-Containing Protein 2. RevErb-beta: Nuclear Receptor Subfamily 1 Group D Member 2. AC: acetyl group. GR: Glucocorticoid receptor. RevERb-Alpha: Nuclear Receptor Subfamily 1 Group D Member 1. CRY1/2 (Cryptochrome 1/2). HSP90: Heat shock protein 90. FKBP5: FK506-binding protein 5. CORT: Corticosterone.
As mentioned above, GR through its ability to respond to GCs’ circadian oscillations, is an ideal coupling partner of the molecular clock (Lambrou et al., 2021). Activated GR is able to influence the transcription of various clock genes via binding to GREs in their promoter regions, among them Per2, Rev-ERBß, Dec2, NPAS2, and E4BP4/NFIL3 (So et al., 2009; Nader et al., 2010). The clock gene Per1 contains both, E-Box and GRE elements in its promoter; it is therefore responsive to both, BMAL1/CLOCK and GR binding (Conway-Campbell et al., 2010). Moreover, the nuclear receptor Rev-ERBα is repressed by GR activity through negative GREs present in its promoter (Surjit et al., 2011).
The clock machinery and GR also interact at the protein level. It has been shown that Rev-ERBα, GRs and HSP90 interact in the cytosol (Okabe et al., 2016). Moreover, Rev-ERBα and GR distribute differentially between the nuclei and the cytosol depending on the phase of the clock, suggesting a possible influence of each other with regard to intracellular trafficking (Surjit et al., 2011; Okabe et al., 2016). The above-mentioned interaction has been shown in cultured fibroblast, whether this mechanism is also present in brain cells needs to be determined. CLOCK harbors a histone-acetyl-transferase activity which is able to alter the affinity of GR to GREs through acetylation of lysine clusters in its hinge region (Nader et al., 2010). Also, CRY proteins are able to decrease GR’s transactivation potential by interacting directly with GR (Lamia et al., 2011). These multiple and reciprocal molecular interactions stress the relevance of both pathways for the cellular and systemic regulation of circadian rhythms.
During pregnancy, maternal physiology including the circadian system has to adapt to fulfill fetal requirements. During the first two thirds of pregnancy, the embryo/fetus is protected by the placenta from excessive levels of GCs by expressing (among others) the enzyme 11β-Hydroxysteroid dehydrogenase (11β-HSD2) which inactivates GCs converting them into an inactive metabolite, cortisone (O’Donnell et al., 2012). Even though only 10% of maternal GCs reach the fetus, a circadian rhythmicity of GCs can still be detected in fetal blood (Astiz et al., 2020). During the last third of pregnancy, there is a gradual increase of GC levels in fetal blood (Atkinson and Waddell, 1995) that helps the fetus to continue developing and growing (Fowden et al., 2022). This is partly due to a down-regulation of 11β-HSD2 (Wieczorek et al., 2019). Therefore, even if GC concentration changes over pregnancy, the circadian rhythmicity is always present, and since GR is expressed at high levels in the fetal hypothalamus (including the SCN during that period), it could be a key signal driving the development and maturation of the circadian system and its coupling to the HPA axis (Astiz et al., 2020; Greiner et al., 2022).
Indeed, extensive evidence shows that maternal stress or circadian rhythm disruption (e.g., through altered photoperiod, sleep deprivation, shift work) leads to a higher risk of developing metabolic, behavioral, and sleep disorders later in life (Moisiadis and Matthews, 2014b; Vilches et al., 2014; Marín, 2016; Mendez et al., 2016; Logan and McClung, 2019; Lužná et al., 2020). Interestingly, due to the close SCN-HPA axis relationship most of the experimental paradigms assessing the long-term effects of maternal stress used in nocturnal rodents entail some degree of circadian disruption because animals are subjected to different manipulations during their normal rest/light phase. Reciprocally, circadian disruption paradigms entail a certain degree of activation of the stress system. This issue was disentangled recently by our lab, when we demonstrated that the offspring from mothers exposed to GCs during the rest phase show worse circadian and stress-related behavioral phenotypes than those from mothers exposed to the same GC concentration, but during the active phase. This means that the effect of prenatal GCs activating epigenetic mechanisms and programming offspring behavior depends on the maternal exposure time (Astiz et al., 2020). We have found that when GC exposure happens, GR in the fetal hypothalamus is activated only if the signal reaches the tissue during the inactive phase. This time-dependent sensitivity to GCs in the fetal hypothalamus was gated by an autonomous function of the fetal molecular clock (Astiz et al., 2020). Then, the maturation of the clock and stress axis of the newborn seems to depend not only on maternal GCs but also on GR sensitivity, which is time-dependent.
From a physiological point of view, an efficient synchronization of the circadian system would require that central or peripheral signals feedback to the SCN to adjust its function according to the internal environment. In the adult, SCN hypothalamic efferences reach the sub-PVN, PVN, supraoptic nuclei (SON), dorsomedial nucleus (DMH), and the arcuate nucleus (ARC) among many others (Saeb-Parsy et al., 2000; Kalsbeek et al., 2004; Postnova et al., 2013; Buijs et al., 2017) and some of those connections are reciprocal (e.g., with the ARC). However, the SCN clock is quite resistant to re-setting signals. This property, so far attributed to the robust network feature of the SCN circuit, might be important to prevent the impact of Zeitgeber noise such as sporadic light exposure, acute stress, or miss-timed food intake on the central pacemaker (Schibler et al., 2015).
GCs, as one of the main outputs of the circadian system, might feedback to the SCN, however, it is still under discussion whether the influence is direct or indirect (Balsalobre et al., 2000). In the early 90s, it was demonstrated that SCN neurons are among the few cell types in adult rodents that do not express GR (Rosenfeld et al., 1988). Interestingly, experiments manipulating GC circadian phase have shown that, GCs have a key role in circadian resynchronization of locomotor activity after jetlag, indicating a clear influence on the SCN (Kiessling et al., 2010). Furthermore, either the removal of the adrenal glands or the restitution of the hormone within physiological limits in adrenalectomized rats, demonstrated that glucocorticoids are involved in plastic rearrangements of the SCN circuit (Maurel et al., 2000). These ultrastructural arrangements have been observed over the 24 h cycle characterized by day/night changes of the glial, axon terminal, and/or somato-dendritic coverage of neurons expressing arginine vasopressin (AVP) or vasoactive intestinal peptide (VIP) (Becquet et al., 2008).
As mentioned before, we know now that astrocytes play a key role as time-keepers of the adult SCN. Astrocytes express the molecular clock machinery with self-sustained circadian rhythms that persist even in constant environmental conditions (Prolo et al., 2005; Marpegan et al., 2009; Barca-Mayo et al., 2017). The molecular clock in SCN astrocytes is entrainable by neuronal signals (Marpegan et al., 2009), temperature changes (Prolo et al., 2005), and they reciprocally modulate neuronal rhythms (Barca-Mayo et al., 2017). Additionally, several astroglial functions are under circadian control such as, intracellular Ca2+ waves (Brancaccio et al., 2017) and the release of gliotransmitters, ATP (Burkeen et al., 2011) and glutamate (Svobodova et al., 2018) and even their morphological changes during the day (Becquet et al., 2008). Interestingly, we have found GR expression in the SCN of adult mice colocalizing with glial fibrillary acidic protein (GFAP) a marker of mature astrocytes (Figure 2) confirming previous observations (Moriya et al., 2000; Leone et al., 2006). Although this needs further investigation it opens the possibility that not only neurons but also astrocytes might play an essential role in the complex cooperation between the SCN, the HPA axis and the systemic coordination of circadian rhythms.
Figure 2. Representative double fluorescence immunostainings in the adult SCN. GFAP (green) used as an astrocyte marker, GR (red) and DAPI (blue) as a nuclear marker. Brain was collected from a 90-day old mouse at ZT (Zeitgeber time) 7, immediately frozen on dry ice, and sectioned (12 μm) in a cryostat. After fixation (20 min at room temperature (RT)), sections were blocked with goat serum 4% in phosphate buffer (PBS) containing 0.4% of Triton X-100 for 1 h at RT. Sections were incubated with anti-GR (Abcam Ab183127, 1:200 in blocking) for 2 nights at 4°C. Sections were incubated afterwards with anti-GFAP (ThermoFisher 14–9,892-82, 1:200 in blocking) for 1 night at 4°C. The day after, sections were incubated with secondary antibodies – goat anti-rabbit Alexa 594 (ThermoFisher A11012, 1:500) and goat anti-mouse Alexa 488 (ThermoFisher A11029, 1:500) in a dark chamber for 2 h at RT. DAPI staining was performed to stain nuclei by incubating slides with 300 nM DAPI (ThermoFisher D1306) in PBS for 2 min in the dark. Images of immunofluorescent staining were made using Nikon Ts2R-FL (10X (insets) and 40X magnification).
In summary, accumulating evidence suggests that GCs play an important role in the development and maturation of the circadian system. This, in turn, feeds back on stress axis regulation in the adult. Glial clocks may play an important role in this context, but experimental evidence for this is still sparse. To causally address such interaction, it would be important to study clock system development in animals with alterations in glial clock function and how glial disruption affects clock and stress regulation in the offspring. With regard to humans, the relevance of perinatal rhythms for shaping circadian system regulation and stress responses offers tremendous potential for therapeutic interventions. Can stabilization of GC rhythms during the end of pregnancy lead to increased resilience of the offspring against stress and stress-associated disorders, and what type of interventions beyond GC treatment are efficient in this context? To which extent is GC perinatal programming reversible later in life, and—against the background of the broad impact of stress hormones on physiological functions—what other systems are affected? Finally, how does GC programming affect glial function later in life, and what does this mean for neuro-immunologic disorders? First steps in this direction have been taken with the studies on perinatal GC treatments (Astiz et al., 2020). It will be interesting to further decipher the underlying mechanisms of GC-fetal rhythm interaction and search for additional factors that impinge on this crosstalk.
All authors listed have made a substantial, direct, and intellectual contribution to the work and approved it for publication.
This work was supported by the German Research Foundation (DFG) grant AS547-1/2 (to ML and MA) and OS353-10/1 (to HO), by Spanish Research Grants PID2021-122694OB-I00 funded by Ministerio de Ciencia e Innovacion (MCIN) to MA and KH, by Achucarro Basque Center for Neuroscience and Basque Foundation of Science (Ikerbasque) to MA, and by the Federation of the European Biochemical Societies (FEBS) Summer Fellowship 2022 (to KH).
The authors would like to thank Beatrix Mahnke and Cecile Demarez, students at the Institute of Neurobiology, University of Lübeck for their contribution optimizing the immunofluorescence protocol.
The authors declare that the research was conducted in the absence of any commercial or financial relationships that could be construed as a potential conflict of interest.
All claims expressed in this article are solely those of the authors and do not necessarily represent those of their affiliated organizations, or those of the publisher, the editors and the reviewers. Any product that may be evaluated in this article, or claim that may be made by its manufacturer, is not guaranteed or endorsed by the publisher.
Antunica-Noguerol, M., Budziñski, M. L., Druker, J., Gassen, N. C., Sokn, M. C., Senin, S., et al. (2016). The activity of the glucocorticoid receptor is regulated by SUMO conjugation to FKBP51. Cell Death Differ. 23, 1579–1591. doi: 10.1038/cdd.2016.44
Astiz, M., Heyde, I., Fortmann, M. I., Bossung, V., Roll, C., Stein, A., et al. (2020). The circadian phase of antenatal glucocorticoid treatment affects the risk of behavioral disorders. Nat. Commun. 11:3593. doi: 10.1038/s41467-020-17429-5
Astiz, M., and Oster, H. (2018). Perinatal programming of circadian clock-stress crosstalk. Neural Plast. 2018, 1–12. doi: 10.1155/2018/5689165
Atkinson, H. C., and Waddell, B. J. (1995). The hypothalamic-pituitary-adrenal axis in rat pregnancy and lactation: circadian variation and interrelationship of plasma adrenocorticotropin and corticosterone. Endocrinology 136, 512–520. doi: 10.1210/endo.136.2.7835284
Balsalobre, A., Brown, S. A., Marcacci, L., Tronche, F., Kellendonk, C., Reichardt, H. M., et al. (2000). Resetting of circadian time in peripheral tissues by glucocorticoid signaling. Science (New York, N.Y.) 289, 2344–2347. doi: 10.1126/science.289.5488.2344
Barca-Mayo, O., Pons-Espinal, M., Follert, P., Armirotti, A., Berdondini, L., and De Pietri Tonelli, D. (2017). Astrocyte deletion of Bmal1 alters daily locomotor activity and cognitive functions via GABA signalling. Nat. Commun. 8:14336. doi: 10.1038/ncomms14336
Becquet, D., Girardet, C., Guillaumond, F., François-Bellan, A.-M., and Bosler, O. (2008). Ultrastructural plasticity in the rat suprachiasmatic nucleus. Possible involvement in clock entrainment. Glia 56, 294–305. doi: 10.1002/glia.20613
Biebl, M. M., and Buchner, J. (2019). Structure, function, and regulation of the Hsp90 machinery. Cold Spring Harb. Perspect. Biol. 11:a034017. doi: 10.1101/cshperspect.a034017
Binder, E. B. (2009). The role of FKBP5, a co-chaperone of the glucocorticoid receptor in the pathogenesis and therapy of affective and anxiety disorders. Psychoneuroendocrinology 34, S186–S195. doi: 10.1016/j.psyneuen.2009.05.021
Brancaccio, M., Edwards, M. D., Patton, A. P., Smyllie, N. J., Chesham, J. E., Maywood, E. S., et al. (2019). Cell-autonomous clock of astrocytes drives circadian behavior in mammals. Science (New York, N.Y.) 363, 187–192. doi: 10.1126/science.aat4104
Brancaccio, M., Patton, A. P., Chesham, J. E., Maywood, E. S., and Hastings, M. H. (2017). Astrocytes control circadian timekeeping in the Suprachiasmatic nucleus via Glutamatergic signaling. Neuron 93, 1420–1435.e5. doi: 10.1016/j.neuron.2017.02.030
Buijs, F. N., Guzmán-Ruiz, M., León-Mercado, L., Basualdo, M. C., Escobar, C., Kalsbeek, A., et al. (2017). Suprachiasmatic nucleus interaction with the arcuate nucleus; essential for organizing physiological rhythms. ENeuro 4:ENEURO.0028-17.2017. doi: 10.1523/ENEURO.0028-17.2017
Buijs, R. M., Kalsbeek, A., van der Woude, T. P., van Heerikhuize, J. J., and Shinn, S. (1993). Suprachiasmatic nucleus lesion increases corticosterone secretion. Am. J. Phys. 264, R1186–R1192. doi: 10.1152/ajpregu.1993.264.6.R1186
Burkeen, J. F., Womac, A. D., Earnest, D. J., and Zoran, M. J. (2011). Mitochondrial calcium signaling mediates rhythmic extracellular ATP accumulation in suprachiasmatic nucleus astrocytes. J. Neurosci. Off. J. Soc. Neurosci. 31, 8432–8440. doi: 10.1523/JNEUROSCI.6576-10.2011
Busada, J. T., and Cidlowski, J. A. (2017). Mechanisms of glucocorticoid action during development. Curr. Top. Dev. Biol. 125, 147–170. doi: 10.1016/bs.ctdb.2016.12.004
Carmona-Alcocer, V., Rohr, K. E., Joye, D. A. M., and Evans, J. A. (2020). Circuit development in the master clock network of mammals. Eur. J. Neurosci. 51, 82–108. doi: 10.1111/ejn.14259
Čečmanová, V., Houdek, P., Šuchmanová, K., Sládek, M., and Sumová, A. (2019). Development and entrainment of the fetal clock in the Suprachiasmatic nuclei: the role of glucocorticoids. J. Biol. Rhythm. 34, 307–322. doi: 10.1177/0748730419835360
Collins, B., Pierre-Ferrer, S., Muheim, C., Lukacsovich, D., Cai, Y., Spinnler, A., et al. (2020). Circadian VIPergic neurons of the Suprachiasmatic nuclei sculpt the sleep-wake cycle. Neuron 108, 486–499.e5. doi: 10.1016/j.neuron.2020.08.001
Conway-Campbell, B. L., Sarabdjitsingh, R. A., McKenna, M. A., Pooley, J. R., Kershaw, Y. M., Meijer, O. C., et al. (2010). Glucocorticoid Ultradian rhythmicity directs cyclical gene pulsing of the clock gene period 1 in rat hippocampus. J. Neuroendocrinol. 22, 1093–1100. doi: 10.1111/j.1365-2826.2010.02051.x
Criado-Marrero, M., Rein, T., Binder, E. B., Porter, J. T., Koren, J., and Blair, L. J. (2018). Hsp90 and FKBP51: complex regulators of psychiatric diseases. Philos. Trans. R. Soc. Lond. Ser. B Biol. Sci. 373:20160532. doi: 10.1098/rstb.2016.0532
Daskalakis, N. P., Meijer, O. C., and de Kloet, E. R. (2022). Mineralocorticoid receptor and glucocorticoid receptor work alone and together in cell-type-specific manner: implications for resilience prediction and targeted therapy. Neurobiology of Stress 18:100455. doi: 10.1016/j.ynstr.2022.100455
Dibner, C., Schibler, U., and Albrecht, U. (2010). The mammalian circadian timing system: organization and coordination of central and peripheral clocks. Annu. Rev. Physiol. 72, 517–549. doi: 10.1146/annurev-physiol-021909-135821
Fowden, A. L., Vaughan, O. R., Murray, A. J., and Forhead, A. J. (2022). Metabolic consequences of glucocorticoid exposure before birth. Nutrients 14:2304. doi: 10.3390/nu14112304
Fries, G. R., Gassen, N. C., and Rein, T. (2017). The FKBP51 glucocorticoid receptor co-chaperone: regulation, function, and implications in health and disease. Int. J. Mol. Sci. 18:2614. doi: 10.3390/ijms18122614
Fuller, P. J., Yang, J., and Young, M. J. (2019). “Chapter three—mechanisms of mineralocorticoid receptor signaling” in Vitamins and Hormones. ed. G. Litwack, vol. 109 (Academic Press), 37–68.
Fuller, P. J., Yao, Y., Yang, J., and Young, M. J. (2012). Mechanisms of ligand specificity of the mineralocorticoid receptor. J. Endocrinol. 213, 15–24. doi: 10.1530/JOE-11-0372
George, C. L., Birnie, M. T., Flynn, B. P., Kershaw, Y. M., Lightman, S. L., and Conway-Campbell, B. L. (2017). Ultradian glucocorticoid exposure directs gene-dependent and tissue-specific mRNA expression patterns in vivo. Mol. Cell. Endocrinol. 439, 46–53. doi: 10.1016/j.mce.2016.10.019
Greiner, P., Houdek, P., Sládek, M., and Sumová, A. (2022). Early rhythmicity in the fetal suprachiasmatic nuclei in response to maternal signals detected by omics approach. PLoS Biol. 20:e3001637. doi: 10.1371/journal.pbio.3001637
Groeneweg, F. L., Karst, H., de Kloet, E. R., and Joëls, M. (2012). Mineralocorticoid and glucocorticoid receptors at the neuronal membrane, regulators of nongenomic corticosteroid signalling. Mol. Cell. Endocrinol. 350, 299–309. doi: 10.1016/j.mce.2011.06.020
Hannibal, J., Møller, M., Ottersen, O. P., and Fahrenkrug, J. (2000). PACAP and glutamate are co-stored in the retinohypothalamic tract. J. Comp. Neurol. 418, 147–155. doi: 10.1002/(SICI)1096-9861(20000306)418:2<147::AID-CNE2>3.0.CO;2-#
Hastings, M. H., Maywood, E. S., and Brancaccio, M. (2018). Generation of circadian rhythms in the suprachiasmatic nucleus. Nat. Rev. Neurosci. 19, 453–469. doi: 10.1038/s41583-018-0026-z
Ibuka, N., Inouye, S. I., and Kawamura, H. (1977). Analysis of sleep-wakefulness rhythms in male rats after suprachiasmatic nucleus lesions and ocular enucleation. Brain Res. 122, 33–47. doi: 10.1016/0006-8993(77)90660-6
Kabrita, C. S., and Davis, F. C. (2008). Development of the mouse suprachiasmatic nucleus: determination of time of cell origin and spatial arrangements within the nucleus. Brain Res. 1195, 20–27. doi: 10.1016/j.brainres.2007.12.020
Kalsbeek, A., Buijs, R. M., van Heerikhuize, J. J., Arts, M., and van der Woude, T. P. (1992). Vasopressin-containing neurons of the suprachiasmatic nuclei inhibit corticosterone release. Brain Res. 580, 62–67. doi: 10.1016/0006-8993(92)90927-2
Kalsbeek, A., La Fleur, S., Van Heijningen, C., and Buijs, R. M. (2004). Suprachiasmatic GABAergic inputs to the paraventricular nucleus control plasma glucose concentrations in the rat via sympathetic innervation of the liver. J. Neurosci. Off. J. Soc. Neurosci. 24, 7604–7613. doi: 10.1523/JNEUROSCI.5328-03.2004
Kalsbeek, A., Heerikhuize, J. J.Van, Wortel, J., and Buijs, R. M. (1996). A diurnal rhythm of stimulatory input to the Hypothalamo–pituitary–adrenal system as revealed by timed Intrahypothalamic administration of the vasopressin V1Antagonist. J. Neurosci., 16, 5555–5565, doi: 10.1523/JNEUROSCI.16-17-05555.1996
Kalsbeek, A., Yi, C.-X., Cailotto, C., la Fleur, S. E., Fliers, E., and Buijs, R. M. (2011). Mammalian clock output mechanisms. Essays Biochem. 49, 137–151. doi: 10.1042/bse0490137
Kiessling, S., Eichele, G., and Oster, H. (2010). Adrenal glucocorticoids have a key role in circadian resynchronization in a mouse model of jet lag. J. Clin. Invest. 120, 2600–2609. doi: 10.1172/JCI41192
Klengel, T., Mehta, D., Anacker, C., Rex-Haffner, M., Pruessner, J. C., Pariante, C. M., et al. (2013). Allele-specific FKBP5 DNA demethylation mediates gene–childhood trauma interactions. Nat. Neurosci. 16, 33–41. doi: 10.1038/nn.3275
Koch, C. E., Begemann, K., Kiehn, J. T., Griewahn, L., Mauer, J., Hess, M. E., et al. (2020). Circadian regulation of hedonic appetite in mice by clocks in dopaminergic neurons of the VTA. Nat. Commun. 11:3071. doi: 10.1038/s41467-020-16882-6
Kolber, B. J., Wieczorek, L., and Muglia, L. J. (2008). Hypothalamic-pituitary-adrenal axis dysregulation and behavioral analysis of mouse mutants with altered glucocorticoid or mineralocorticoid receptor function. Stress (Amsterdam, Netherlands) 11, 321–338. doi: 10.1080/10253890701821081
Lambrou, G. I., Kino, T., Koide, H., Ng, S. S. M., Geronikolou, S. A., Bacopoulou, F., et al. (2021). Bioinformatics analyses of spatial peripheral circadian clock-mediated gene expression of glucocorticoid receptor-related genes. Adv. Exp. Med. Biol. 1338, 67–79. doi: 10.1007/978-3-030-78775-2_9
Lamia, K. A., Papp, S. J., Yu, R. T., Barish, G. D., Uhlenhaut, N. H., Jonker, J. W., et al. (2011). Cryptochromes mediate rhythmic repression of the glucocorticoid receptor. Nature 480, 552–556. doi: 10.1038/nature10700
Landgraf, D., Achten, C., Dallmann, F., and Oster, H. (2015). Embryonic development and maternal regulation of murine circadian clock function. Chronobiol. Int. 32, 416–427. doi: 10.3109/07420528.2014.986576
Lehman, M. N., Silver, R., Gladstone, W. R., Kahn, R. M., Gibson, M., and Bittman, E. L. (1987). Circadian rhythmicity restored by neural transplant. Immunocytochemical characterization of the graft and its integration with the host brain. J. Neurosci. Off. J. Soc. Neurosci. 7, 1626–1638.
Leone, M. J., Marpegan, L., Bekinschtein, T. A., Costas, M. A., and Golombek, D. A. (2006). Suprachiasmatic astrocytes as an interface for immune-circadian signalling. J. Neurosci. Res. 84, 1521–1527. doi: 10.1002/jnr.21042
Liston, C., Cichon, J. M., Jeanneteau, F., Jia, Z., Chao, M. V., and Gan, W.-B. (2013). Circadian glucocorticoid oscillations promote learning-dependent synapse formation and maintenance. Nat. Neurosci. 16, 698–705. doi: 10.1038/nn.3387
Logan, R. W., and McClung, C. A. (2019). Rhythms of life: circadian disruption and brain disorders across the lifespan. Nat. Rev. Neurosci. 20, 49–65. doi: 10.1038/s41583-018-0088-y
Lužná, V., Houdek, P., Liška, K., and Sumová, A. (2020). Challenging the integrity of rhythmic maternal signals revealed gene-specific responses in the fetal Suprachiasmatic nuclei. Front. Neurosci. 14:613531. doi: 10.3389/fnins.2020.613531
Marín, O. (2016). Developmental timing and critical windows for the treatment of psychiatric disorders. Nat. Med. 22, 1229–1238. doi: 10.1038/nm.4225
Marpegan, L., Krall, T. J., and Herzog, E. D. (2009). Vasoactive intestinal polypeptide entrains circadian rhythms in astrocytes. J. Biol. Rhythm. 24, 135–143. doi: 10.1177/0748730409332042
Maurel, D., Sage, D., Mekaouche, M., and Bosler, O. (2000). Glucocorticoids up-regulate the expression of glial fibrillary acidic protein in the rat suprachiasmatic nucleus. Glia 29, 212–221. doi: 10.1002/(sici)1098-1136(20000201)29:3<212::aid-glia3>3.0.co;2-6
Mazaira, G. I., and Galigniana, M. D. (2019). Reconstitution of the steroid receptor Heterocomplex. Methods Mol. Biol. (Clifton, N.J.) 1966, 125–135. doi: 10.1007/978-1-4939-9195-2_10
Mendez, N., Halabi, D., Spichiger, C., Salazar, E. R., Vergara, K., Alonso-Vasquez, P., et al. (2016). Gestational Chronodisruption impairs circadian physiology in rat male offspring, increasing the risk of chronic disease. Endocrinology 157, 4654–4668. doi: 10.1210/en.2016-1282
Moisiadis, V. G., and Matthews, S. G. (2014a). Glucocorticoids and fetal programming part 1: outcomes. Nat. Rev. Endocrinol. 10, 391–402. doi: 10.1038/nrendo.2014.73
Moisiadis, V. G., and Matthews, S. G. (2014b). Glucocorticoids and fetal programming part 2: mechanisms. Nat. Rev. Endocrinol. 10, 403–411. doi: 10.1038/nrendo.2014.74
Moore, R. Y., and Eichler, V. B. (1972). Loss of a circadian adrenal corticosterone rhythm following suprachiasmatic lesions in the rat. Brain Res. 42, 201–206. doi: 10.1016/0006-8993(72)90054-6
Moriya, T., Yoshinobu, Y., Kouzu, Y., Katoh, A., Gomi, H., Ikeda, M., et al. (2000). Involvement of glial fibrillary acidic protein (GFAP) expressed in astroglial cells in circadian rhythm under constant lighting conditions in mice. J. Neurosci. Res. 60, 212–218. doi: 10.1002/(SICI)1097-4547(20000415)60:2<212::AID-JNR10>3.0.CO;2-P
Munekawa, K., Tamada, Y., Iijima, N., Hayashi, S., Ishihara, A., Inoue, K., et al. (2000). Development of astroglial elements in the suprachiasmatic nucleus of the rat: with special reference to the involvement of the optic nerve. Exp. Neurol. 166, 44–51. doi: 10.1006/exnr.2000.7490
Nader, N., Chrousos, G. P., and Kino, T. (2010). Interactions of the circadian CLOCK system and the HPA axis. Trends Endocrinol. Metab. 21, 277–286. doi: 10.1016/j.tem.2009.12.011
Nagai, K., Nishio, T., Nakagawa, H., Nakamura, S., and Fukuda, Y. (1978). Effect of bilateral lesions of the suprachiasmatic nuclei on the circadian rhythm of food-intake. Brain Res. 142, 384–389. doi: 10.1016/0006-8993(78)90648-0
Nicolaides, N. C., Chrousos, G., and Kino, T. (2000). “Glucocorticoid receptor” in Endotext. eds. K. R. Feingold, B. Anawalt, A. Boyce, G. Chrousos, W. W. de Herder, and K. Dhatariya, et al. (MDText.com, Inc.) Available at: http://www.ncbi.nlm.nih.gov/books/NBK279171/
O’Donnell, K. J., Bugge Jensen, A., Freeman, L., Khalife, N., O’Connor, T. G., and Glover, V. (2012). Maternal prenatal anxiety and downregulation of placental 11β-HSD2. Psychoneuroendocrinology 37, 818–826. doi: 10.1016/j.psyneuen.2011.09.014
Oakley, R. H., Whirledge, S. D., Petrillo, M. G., Riddick, N. V., Xu, X., Moy, S. S., et al. (2021). Combinatorial actions of glucocorticoid and mineralocorticoid stress hormone receptors are required for preventing neurodegeneration of the mouse hippocampus. Neurobiol. Stress 15:100369. doi: 10.1016/j.ynstr.2021.100369
Okabe, T., Chavan, R., Fonseca Costa, S. S., Brenna, A., Ripperger, J. A., and Albrecht, U. (2016). REV-ERBα influences the stability and nuclear localization of the glucocorticoid receptor. J. Cell Sci. 129, 4143–4154. doi: 10.1242/jcs.190959
Olejníková, L., Polidarová, L., and Sumová, A. (2018). Stress affects expression of the clock gene Bmal1 in the suprachiasmatic nucleus of neonatal rats via glucocorticoid-dependent mechanism. Acta Physiol. (Oxf.) 223:e13020. doi: 10.1111/apha.13020
Oster, H., Damerow, S., Kiessling, S., Jakubcakova, V., Abraham, D., Tian, J., et al. (2006). The circadian rhythm of glucocorticoids is regulated by a gating mechanism residing in the adrenal cortical clock. Cell Metab. 4, 163–173. doi: 10.1016/j.cmet.2006.07.002
Oyamada, N., Sone, M., Miyashita, K., Park, K., Taura, D., Inuzuka, M., et al. (2008). The role of mineralocorticoid receptor expression in brain remodeling after cerebral ischemia. Endocrinology 149, 3764–3777. doi: 10.1210/en.2007-1770
Pevet, P., and Challet, E. (2011). Melatonin: both master clock output and internal time-giver in the circadian clocks network. J. Physiol. Paris 105, 170–182. doi: 10.1016/j.jphysparis.2011.07.001
Pezük, P., Mohawk, J. A., Wang, L. A., and Menaker, M. (2012). Glucocorticoids as entraining signals for peripheral circadian oscillators. Endocrinology 153, 4775–4783. doi: 10.1210/en.2012-1486
Postnova, S., Fulcher, R., Braun, H. A., and Robinson, P. A. (2013). A minimal physiologically based model of the HPA axis under influence of the sleep-wake cycles. Pharmacopsychiatry 46 Suppl 1, S36–S43. doi: 10.1055/s-0033-1333763
Pratt, W. B., and Toft, D. O. (1997). Steroid receptor interactions with heat shock protein and immunophilin chaperones. Endocr. Rev. 18, 306–360. doi: 10.1210/edrv.18.3.0303
Prolo, L. M., Takahashi, J. S., and Herzog, E. D. (2005). Circadian rhythm generation and entrainment in astrocytes. J. Neurosci. Off. J. Soc. Neurosci. 25, 404–408. doi: 10.1523/JNEUROSCI.4133-04.2005
Reddy, T. E., Pauli, F., Sprouse, R. O., Neff, N. F., Newberry, K. M., Garabedian, M. J., et al. (2009). Genomic determination of the glucocorticoid response reveals unexpected mechanisms of gene regulation. Genome Res. 19, 2163–2171. doi: 10.1101/gr.097022.109
Rimmele, U., Meier, F., Lange, T., and Born, J. (2010). Suppressing the morning rise in cortisol impairs free recall. Learn. Mem. 17, 186–190. doi: 10.1101/lm.1728510
Romero, M. T., Lehman, M. N., and Silver, R. (1993). Age of donor influences ability of suprachiasmatic nucleus grafts to restore circadian rhythmicity. Brain Res. Dev. Brain Res. 71, 45–52. doi: 10.1016/0165-3806(93)90103-h
Rosenfeld, P., Van Eekelen, J. A. M., Levine, S., and De Kloet, E. R. (1988). Ontogeny of the type 2 glucocorticoid receptor in discrete rat brain regions: an immunocytochemical study. Dev. Brain Res. 42, 119–127. doi: 10.1016/0165-3806(88)90207-6
Saeb-Parsy, K., Lombardelli, S., Khan, F. Z., McDowall, K., Au-Yong, I. T., and Dyball, R. E. (2000). Neural connections of hypothalamic neuroendocrine nuclei in the rat. J. Neuroendocrinol. 12, 635–648. doi: 10.1046/j.1365-2826.2000.00503.x
Schibler, U., Gotic, I., Saini, C., Gos, P., Curie, T., Emmenegger, Y., et al. (2015). Clock-talk: interactions between central and peripheral circadian oscillators in mammals. Cold Spring Harb. Symp. Quant. Biol. 80, 223–232. doi: 10.1101/sqb.2015.80.027490
Sekaran, S., Lupi, D., Jones, S. L., Sheely, C. J., Hattar, S., Yau, K.-W., et al. (2005). Melanopsin-dependent photoreception provides earliest light detection in the mammalian retina. Curr. Biol. 15, 1099–1107. doi: 10.1016/j.cub.2005.05.053
Silver, R., LeSauter, J., Tresco, P. A., and Lehman, M. N. (1996). A diffusible coupling signal from the transplanted suprachiasmatic nucleus controlling circadian locomotor rhythms. Nature 382, 810–813. doi: 10.1038/382810a0
So, A. Y.-L., Bernal, T. U., Pillsbury, M. L., Yamamoto, K. R., and Feldman, B. J. (2009). Glucocorticoid regulation of the circadian clock modulates glucose homeostasis. Proc. Natl. Acad. Sci. U. S. A. 106, 17582–17587. doi: 10.1073/pnas.0909733106
Son, G. H., Chung, S., Choe, H. K., Kim, H.-D., Baik, S.-M., Lee, H., et al. (2008). Adrenal peripheral clock controls the autonomous circadian rhythm of glucocorticoid by causing rhythmic steroid production. Proc. Natl. Acad. Sci. U. S. A. 105, 20970–20975. doi: 10.1073/pnas.0806962106
Surjit, M., Ganti, K. P., Mukherji, A., Ye, T., Hua, G., Metzger, D., et al. (2011). Widespread negative response elements mediate direct repression by agonist- Liganded glucocorticoid receptor. Cells 145, 224–241. doi: 10.1016/j.cell.2011.03.027
Svobodova, I., Bhattaracharya, A., Ivetic, M., Bendova, Z., and Zemkova, H. (2018). Circadian ATP release in Organotypic cultures of the rat Suprachiasmatic nucleus is dependent on P2X7 and P2Y receptors. Front. Pharmacol. 9:192. doi: 10.3389/fphar.2018.00192
Takahashi, J. S. (2017). Transcriptional architecture of the mammalian circadian clock. Nat. Rev. Genet. 18, 164–179. doi: 10.1038/nrg.2016.150
Tso, C. F., Simon, T., Greenlaw, A. C., Puri, T., Mieda, M., and Herzog, E. D. (2017). Astrocytes regulate daily rhythms in the Suprachiasmatic nucleus and behavior. Curr. Biol. 27, 1055–1061. doi: 10.1016/j.cub.2017.02.037
Vilches, N., Spichiger, C., Mendez, N., Abarzua-Catalan, L., Galdames, H. A., Hazlerigg, D. G., et al. (2014). Gestational chronodisruption impairs hippocampal expression of NMDA receptor subunits Grin1b/Grin3a and spatial memory in the adult offspring. PLoS One 9:e91313. doi: 10.1371/journal.pone.0091313
Keywords: circadian system, hypothalamic-pituitary-adrenal axis, development, perinatal programming, glucocorticoids, glucocorticoid receptor, molecular clock
Citation: Lehmann M, Haury K, Oster H and Astiz M (2023) Circadian glucocorticoids throughout development. Front. Neurosci. 17:1165230. doi: 10.3389/fnins.2023.1165230
Received: 13 February 2023; Accepted: 06 April 2023;
Published: 25 April 2023.
Edited by:
Takahiro J. Nakamura, Meiji University, JapanReviewed by:
Russell D. Romeo, Columbia University, United StatesCopyright © 2023 Lehmann, Haury, Oster and Astiz. This is an open-access article distributed under the terms of the Creative Commons Attribution License (CC BY). The use, distribution or reproduction in other forums is permitted, provided the original author(s) and the copyright owner(s) are credited and that the original publication in this journal is cited, in accordance with accepted academic practice. No use, distribution or reproduction is permitted which does not comply with these terms.
*Correspondence: Mariana Astiz, bWFyaWFuYS5hc3RpekBhY2h1Y2Fycm8ub3Jn
†These authors have contributed equally to this work
Disclaimer: All claims expressed in this article are solely those of the authors and do not necessarily represent those of their affiliated organizations, or those of the publisher, the editors and the reviewers. Any product that may be evaluated in this article or claim that may be made by its manufacturer is not guaranteed or endorsed by the publisher.
Research integrity at Frontiers
Learn more about the work of our research integrity team to safeguard the quality of each article we publish.