- 1Department of Physiology and Pharmacology, Schulich School of Medicine and Dentistry, The University of Western Ontario, London, ON, Canada
- 2Department of Anatomy and Cell Biology, Schulich School of Medicine and Dentistry, The University of Western Ontario, London, ON, Canada
- 3Department of Psychology, The University of Western Ontario, London, ON, Canada
Introduction: Autism Spectrum Disorder (ASD) has been associated with a wide variety of genetic and environmental risk factors in both human and preclinical studies. Together, findings support a gene-environment interaction hypothesis whereby different risk factors independently and synergistically impair neurodevelopment and lead to the core symptoms of ASD. To date, this hypothesis has not been commonly investigated in preclinical ASD models. Mutations in the Contactin-associated protein-like 2 (Cntnap2) gene and exposure to maternal immune activation (MIA) during pregnancy have both been linked to ASD in humans, and preclinical rodent models have shown that both MIA and Cntnap2 deficiency lead to similar behavioral deficits.
Methods: In this study, we tested the interaction between these two risk factors by exposing Wildtype, Cntnap2+/–, and Cntnap2–/– rats to Polyinosinic: Polycytidylic acid (Poly I:C) MIA at gestation day 9.5.
Results: Our findings showed that Cntnap2 deficiency and Poly I:C MIA independently and synergistically altered ASD-related behaviors like open field exploration, social behavior, and sensory processing as measured through reactivity, sensitization, and pre-pulse inhibition (PPI) of the acoustic startle response. In support of the double-hit hypothesis, Poly I:C MIA acted synergistically with the Cntnap2–/– genotype to decrease PPI in adolescent offspring. In addition, Poly I:C MIA also interacted with the Cntnap2+/– genotype to produce subtle changes in locomotor hyperactivity and social behavior. On the other hand, Cntnap2 knockout and Poly I:C MIA showed independent effects on acoustic startle reactivity and sensitization.
Discussion: Together, our findings support the gene-environment interaction hypothesis of ASD by showing that different genetic and environmental risk factors could act synergistically to exacerbate behavioral changes. In addition, by showing the independent effects of each risk factor, our findings suggest that ASD phenotypes could be caused by different underlying mechanisms.
Introduction
In recent decades, the rise of Autism Spectrum Disorder (ASD) prevalence has prompted many studies into its underlying etiology. In the field of genetics, the rapid advancement of DNA sequencing and genetic techniques has led to the discovery of many rare and common genetic variants associated with ASD (Manoli and State, 2021). Following a similar trend, various environmental factors that can influence neurodevelopment and increase the risk for ASD have been identified (Modabbernia et al., 2017). However, neither genetic nor environmental risk factors alone can account for all cases of the disorder, providing support for the gene-environment interaction hypothesis (Chaste and Leboyer, 2012). In this hypothesis, genetic and environmental risk factors produce a combined effect that alters prenatal and early postnatal brain development and lead to the hallmark ASD behavioral features of altered social interaction, communication, and repetitive behavior. Unfortunately, our current understanding of gene-environment interactions in the pathophysiology of ASD is limited, as studies have traditionally focused on studying individual risk factors (Varghese et al., 2017).
Beyond the complex etiology, deciphering precisely how genetic or environmental insults lead to the behavioral features of ASD has proven difficult. Although many advances have been made in developing ASD-related behavioral tests in preclinical models, it is still difficult for these models to replicate the human repertoire of social interaction, communication, and restricted or repetitive patterns of behavior (Crawley, 2012). To circumvent these limitations, one potential solution is to study basic sensory processing mechanisms that are more directly translatable between species. This avenue has been explored more closely in recent years, following the publication of the fifth edition of the Diagnostic and Statistical Manual of Mental Disorders (DSM V). In this new edition, the DSM emphasized hypo- or hyperreactivity to sensory input as a feature that can be associated with restricted, repetitive patterns of behavior, interests, or activities (American Psychiatric Association, 2013). Similarly, altered sensory processing can be linked with social behavior and communication, which often require integrating large amounts of sensory information (Thye et al., 2018).
In this study, our primary goal was to study sensory processing behaviors in a multifactorial gene-environment interaction rodent model of ASD. The two risk factors in our model were maternal immune activation (MIA) during pregnancy and a genetic deficiency in Contactin associated protein-like 2 (Cntnap2). A homozygous loss-of-function mutation in the human CNTNAP2 leads to a syndromic form of ASD, with the syndrome also including cortical dysplasia, seizures, language regression, and cognitive delays (Strauss et al., 2006; Jackman et al., 2009; Zweier, 2011; Peñagarikano and Geschwind, 2012; Rodenas-Cuadrado et al., 2016). These findings prompted the development of Cntnap2 knockout animal models to study ASD-related pathophysiology. On the other hand, exposure to various types of maternal infection during pregnancy is known to increase the offspring’s risk of developing ASD, which implicates the maternal immune response, a common factor between different infections, as a disruptor of neurodevelopment (Jiang et al., 2016; Haddad et al., 2020b). Following these epidemiological associations, animal models were developed to investigate how MIA, induced through injection of an immune stimulant at various times during pregnancy, impacts neurodevelopment and postnatal phenotypes.
Preclinical studies investigating the effects of Cntnap2 knockout and MIA have shown strong face validity in relation to ASD and potential for interaction. For example, both Cntnap2–/– mice and MIA offspring show deficits in social behavior, increased repetitive behavior, and increased sensory reactivity (Peñagarikano et al., 2011, 2015; Brimberg et al., 2016; Richetto et al., 2017; Vuillermot et al., 2017; Weber-Stadlbauer et al., 2017; Hui et al., 2018; Scott et al., 2018, 2020; Haddad et al., 2020a). Furthermore, a study by Schaafsma et al. (2017) combined MIA and Cntnap knockout in mice and demonstrated a sex-specific gene-environment interaction in ultrasonic vocalizations, social behavior, and locomotor hyperactivity. Beyond behavioral phenotypes, both Poly I:C MIA offspring and Cntnap2–/– mice show similar cellular and molecular brain phenotypes including disorganized cortical layering (Peñagarikano et al., 2011; Choi et al., 2016; Coutinho et al., 2017), decreased expression of Parvalbumin in the hippocampus (Abazyan et al., 2010; Peñagarikano et al., 2011; Lipina et al., 2013; Brunner et al., 2015), decreased cortical and hippocampal dendritic arborization (Li et al., 2014; Brimberg et al., 2016; Fernandes et al., 2019; Xu et al., 2019), and increased microglial activation (Coutinho et al., 2017; Hui et al., 2018).
This study sought to explore the Cntnap2-MIA interaction in the context of sensory processing, as measured by reactivity, sensitization, and pre-pulse inhibition (PPI) of the acoustic startle response. These startle phenotypes are also measurable in humans and provide a direct comparison to human ASD studies (Kohl et al., 2014; Takahashi et al., 2017). In addition, our study utilized a different MIA protocol with Polyinosinic: polycytidylic acid (Poly I:C) as the immunogen of choice, injected at gestation day (GD) 9.5, which is one of the most commonly used protocols in the MIA literature (Haddad et al., 2020b). Finally, we were interested in determining whether MIA would interact with a partial Cntnap2 deletion in heterozygous animals, which typically resemble Wildtype animals (Scott et al., 2020).
Materials and methods
Animals
This study was conducted using Wildtype (WT), Cntnap2 heterozygous (Cntnap2+/–) and homozygous (Cntnap2–/–) knockout rats on a Sprague Dawley background. The Cntnap2 rat model contains a 5 base pair frameshift deletion in exon 6 of the Cntnap2 gene, resulting in total functional loss of the Cntnap2 protein CASPR2, as confirmed via Western blot (ENVIGO). Original breeders were purchased from ENVIGO in vivo services and then licensed for in-house breeding. In this experiment, genotyping was performed using genomic DNA collected at weaning. The genomic DNA was amplified using PCR and then sequenced to detect the 5-base-pair deletion in Cntnap2+/– and Cntnap2–/– offspring (see Supplementary Figure 1 for representative sequencing results). All animal procedures were approved by the Western Animal Care Committee and adhered to the guidelines of the Canadian Council on Animal Care.
Rats were housed in open cages with sawdust bedding, given ad libitum food and water, and kept on a 12 h light–12 h dark cycle with lights turning on at 7:00 am. Cages were enriched with polycarbonate huts and wrinkled paper. Cage changes took place once a week except during behavioral testing procedures, during which cage changes were carried out at the end of the 5-day startle protocols to ensure startle measures were not influenced by cage change stress. All behavioral testing and cage changes took place during the light phase (between 7:00 and 19:00 h).
Timed breeding
We used the same male rat to generate all 18 litters in this study over 6 months to minimize the impact of variation in paternal characteristics and ensure our results can be more specifically linked to the maternal immune response. In each breeding round, the Cntnap2+/– breeding male (aged 6 months at the start of the experiment and 12 months by the end of the experiment) was paired with 2–3 Cntnap2+/– females (aged 3–9 months). Additionally, one litter in this experiment was accidentally generated by pairing a Cntnap2–/– female with the Cntnap2+/– male. Statistical inspection of behavioral data revealed that offspring from this litter were not different from those obtained by full heterozygous breeding and were therefore included in all the results. Breeding rounds were at least 1 week apart. There were six breeding rounds in total. Five of these rounds contained at least one saline and one Poly I:C dam. At the end of these five rounds, the WT saline offspring group was the smallest, so a sixth breeding round containing only saline-treated dams with three females was performed to increase the sample size for this group. The breeding females across all breeding rounds originated from four different genetic families (4–5 females per family) that were all unrelated to the breeding male.
After pairing, a vaginal smear was collected from each female at 8 a.m. every morning and inspected under a light microscope to track the estrus cycle and check for the presence of sperm. If sperm was detected in the smear, the female was considered pregnant, and that day was considered Gestation Day (GD) 0.5. Pregnant females were separated from the male on GD0.5 and transferred into a single cage, where they were left undisturbed until injection day (GD9.5). All breeding females were primiparous at the start of the experiment. Only one female was bred twice in this experiment, all other females were bred only once. For the female that was bred twice, visual inspection of startle and open field behavioral data, stratified by age, sex, and genotype, confirmed that the second litter showed similar phenotypes to primiparous litters in the same experimental group.
Maternal immune activation
Pregnant females were randomly assigned to receive either Poly I:C or saline injections. MIA was induced using Poly I:C (Sigma lot #118M4035V), which had been previously aliquoted and stored at −20°C. Poly I:C aliquots were diluted in 0.9% saline to obtain a concentration of 4 mg/ml. At around 10 a.m. on GD9.5, pregnant females were weighed and had their rectal temperature measured. Then, they underwent isoflurane anesthesia (5% induction, 2% maintenance), during which they were injected with either 0.9% saline or 4 mg/kg of Poly I:C into the tail vein. The injection procedure took an average of 5 min and rats were returned to their cages afterward and were left undisturbed besides blood collection, temperature/weight measurement, and weekly cage change. Temperature measurements were taken 3-, 24-, and 48- h following injection, whereas maternal weight was recorded 24- and 48- h following injection. Similar to our previous Poly I:C MIA experiment (Haddad et al., 2020a), we found no significant differences in post-injection temperature and weight gain data between the Poly I:C and saline dams (data not shown). Since body temperature and weight gain following Poly I:C MIA seem to be unreliable indicators of the maternal immune response (Haddad et al., 2020b), we confirmed the immune response by measuring the level of proinflammatory cytokines in the maternal circulation.
On the day of injection, maternal blood was collected from the dams 3 h following injection. Under isoflurane anesthesia, 0.5–1 mL of blood was drawn from the tail vein and stored in serum separation tubes (SARSTEDT Microvette). The blood was allowed to clot for 30 min at room temperature and then spun at 2000 g and 4°C for 10 min. Serum was then collected and stored at −20°C to later be used in the ELISA cytokine experiment to assess cytokine expression.
The day of parturition was designated as postnatal day (PND) 0. Offspring were weaned at PND21, and littermates were separated based on sex (but not genotype) and housed in groups of 2–4 rats per cage. This resulted in the cohousing of sex-matched littermates possessing different Cntnap2 genotypes. At the end of the experiment, the final number of litters in the saline group was as follows: Ten litters were born from saline dams, and eight litters were born from Poly I:C dams.
Quantification of the immune response—Enzyme-linked immunosorbent assay (ELISA)
Post-injection serum was used to quantify the maternal immune response to Poly I:C using DuoSet Interleukin (IL)-6 (Catalog# DY506; Lot P216627; R&D Systems) and Tumor Necrosis Factor (TNF)-α (Catalog# DY510; Lot P237489; R&D Systems) ELISAs supplemented with the ELISA ancillary kit (Catalog# DY008B; LotP240500; R&D Systems). The procedure was conducted according to the manufacturer’s instructions with a couple of modifications. Due to the presence of matrix effects in the serum that could disrupt the signal produced by the cytokines of interest, samples were diluted 10-fold as opposed to the twofold dilution recommended by the Duoset instruction manual (10% serum, 90% buffer solution). Additionally, 10% control serum was obtained from a control rat to establish the cytokine standards used to generate the standard curve, so that the curve is representative of a signal containing 10% serum and would help more accurately determine the true concentration of IL-6 in the samples. A standard curve was generated using linear regression of the log concentration plotted against the log optical density for positive and negative controls. This curve was then used to interpolate cytokine concentrations in the serum samples.
Offspring testing outline
Startle, open field, and social behavior testing occurred in adolescence and adulthood. Adolescent testing began around PND38-39, and adult testing began around PND90-100. Animals were weighed and behaviorally tested in both adolescence and adulthood, with the whole battery of tests taking 9 days to complete at each age timepoint. Tests were performed in the following order: Startle acclimation and handling (Day 1), social behavior (Days 2 and 3), open field and startle reactivity (Day 4), startle sensitization (Days 5–8), and PPI (Day 9). To get animals acclimated to the experimenter, adolescent animals were handled at least twice, 3–5 min each time, before startle acclimation. The full experimental timeline is shown in Figure 1.1
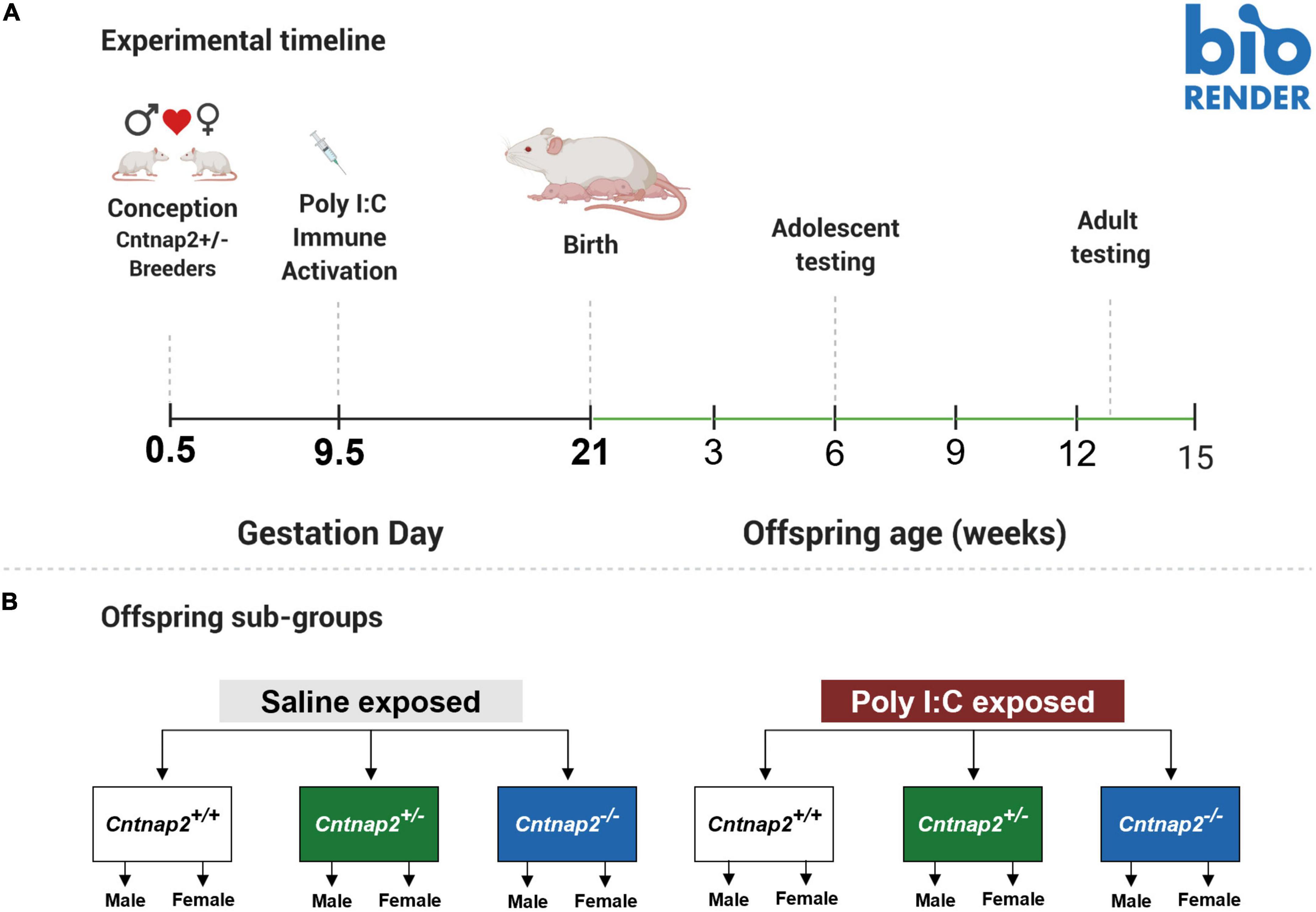
Figure 1. Summary of experimental timeline and groups. (A) Illustration showing the experimental timeline, including the timing of MIA and behavioral experiments. (B) A schematic showing the different MIA and genotype subgroups. There were 6 MIA-genotype groups, and 12 total subgroups when accounting for sex.
Acoustic startle response
Acclimation
Startle testing was performed as described by Valsamis and Schmid (2011). After handling, rats were initially acclimated to the experimental procedure, Plexiglas animal holders, and startle chambers (Med Associates, VT, USA) by undergoing three 5-min acclimation sessions, at least 6 h apart, with 65 dB sound pressure level (SPL) white noise background. The Plexiglass animal holders were washed with unscented soap between acclimation runs, and the same washing procedure was used for all the startle testing described below.
Startle Reactivity After the acclimation sessions, startle reactivity in response to startle stimuli of varying intensities was measured. This test consisted of white noise startle stimuli at intensities ranging from 65 to 120 dB in 5 dB increments, presented in a pseudorandomized order. The startle reactivity session used a constant platform sensitivity for all animals. Based on data from this test, platform sensitivity was then adjusted for each rat to optimally detect the startle reflex in all remaining startle tests.
Sensitization
Animals were exposed to a 5-min acclimation period with background noise, followed by a block of 30 trials. In this block, each trial consisted of a 20 ms-long, 100 dB white noise stimulus after which the startle amplitude was measured. A relatively long ITI was used (1 min) to minimize short-term habituation effects, thereby exposing sensitization effects (Azzopardi et al., 2018). To compare sensitization between groups, sensitization curves for day 1 of startle testing were generated, depicting the startle amplitude for each of the 30 trials in the session. However, these curves showed that animals across all groups showed a large degree of between-trial variability. Therefore, we decided to bin all trials following trial 1 into bins of 3 or 4 trials. Bin 1 was the average of the first 4 trials after trial 1 (trials 2–5), whereas bin 9 was the average of the last 4 trials in the session (trials 27–30). Bins 2–8 each consisted of the average of 3 trials and encompassed trials 6–26. Finally, a sensitization score (Bin 1 / Trial 1) was calculated for each animal, which produced a normalized value that accounted for each animal’s baseline startle at trial 1.
PPI
The PPI session consisted of an initial block of 20 trials (110 dB, 20 ms duration), followed by a PPI block. In the PPI block, animals were exposed to either startle-only trials (110 dB, 20 ms duration), or pre-pulse trials, which included a 4 ms white noise pre-pulse of either 75, 80, or 85 dB preceding the startle stimulus by an interstimulus interval (ISI) of either 30 or 100 ms. ISI was determined as the time between the onset of the pre-pulse to the onset of the startle stimulus. In total, the 60 pre-pulse trials were divided into 10 of each of the following pre-pulse-ISI combinations: 75–30, 75–100, 80–30, 80–100, 85–30, and 85–100. The trials were presented in a pseudorandomized order and separated by a 12–18 s randomized ITI. After the presentation of the startle stimulus in each startle-only or pre-pulse trial, the startle amplitude was measured. PPI was calculated as the amount of inhibition of startle in pre-pulse trials compared to startle-only trials in the PPI block:
Open field test
The day following the PPI test, rats were placed in a square open field of 45.7 cm × 45.7 cm dimension for 30 min as described previously (Fulcher et al., 2020). This test was used to determine locomotor activity by measuring the total distance traveled throughout the test. Additionally, anxiety-like behavior was measured through the time spent in the center of the chamber. The animal’s head location was monitored by an overhead camera as they freely explored the box; the data was collected and analyzed using ANYMAZE software (V4.99, Stoelting, Wood Dale, IL, USA). The apparatus was cleaned with 70% ethanol between experiments.
Social behavior
Each rat’s preference for a conspecific over an inanimate object (sociability), as well as its preference for a stranger rat over a familiar rat (social novelty), were assessed using the previously established 3-chamber assay (Moy et al., 2004; Crawley, 2007; Scott et al., 2020).
The apparatus was 115 cm long and 58 cm wide, with transparent walls that were 45 cm high. The apparatus was split equally into 3 chambers, and two gates (10 cm wide) separated the center chamber from the left and right side chambers. The gates were always open, and the animal was free to explore and move between chambers at all points during the test. In the middle of each of the side chambers, a plexiglass animal holder that allowed nose contact was placed.
The test began by placing the test rat in the center chamber, with empty animal holders in the left and right chambers. The test rat was given 10 min to explore and habituate to the entire apparatus. Following habituation, sociability preference was determined by placing a stranger rat (stranger 1) in one of the side chambers and allowing the test rat to explore for 10 min. The stranger was a sex-matched adult animal between 3 and 6 months of age. The strangers were housed in the same holding room as the experimental animals. To account for potential side bias that animals sometimes exhibit in this kind of test, the side where stranger 1 was placed was chosen randomly, such that roughly half of the animals had stranger 1 placed in the left chamber, and the other half had stranger 1 placed in the right chamber. Then, social novelty preference was determined by placing a novel stranger rat (stranger 2) in the opposing side chamber and allowing the test rat to explore for 10 min. During social novelty, stranger 1 is considered familiar to the test rat, and stranger 2 is considered novel. The test rat was not removed from the apparatus while placing the strangers into the side chambers to avoid additional stress, which can alter social and exploratory behavior. Between different test rats, the animal holders were washed with unscented soap, and the entire apparatus was wiped with 70% ethanol.
The test rat’s exploration was tracked using a camera and its activity in each chamber was quantified using ANYMAZE software (V4.99, Stoelting, Wood Dale, IL, USA). Additionally, the animal’s close exploration (sniffing) of the animal holders was tracked, which was specified as the animal’s nose (as detected by ANYMAZE) being within 3 cm of the animal holder.
Statistical analysis
Maternal serum cytokines
A one-tailed independent t-test was conducted for the levels of circulating proinflammatory cytokines (measured in pg/mL for both IL-6 and TNF-α) comparing 8 saline and 8 Poly I:C dams. These tests were adjusted to account for the violation of the assumption of equal variance since there was more variability in the levels of serum cytokines in Poly I:C dams compared to saline dams.
Offspring behavioral data
Before conducting analysis, outliers were systematically removed to exclude extreme values and to allow the data distributions to better approximate a normal distribution that is required for the parametric statistical tests described later in this section.
Data were scanned using the SPSS Explore function on each genotype-MIA-sex group in each age time point to detect outliers (for each age time point, 12 groups in total: 3 genotypes x 2 MIA groups × 2 sexes). Within each genotype-MIA-sex group, outliers were defined as either moderate or extreme: Moderate outliers were those that had a value of 1.5 interquartile ranges above the 75th percentile or below the 25th percentile; Extreme outliers were those that had a value of 3 interquartile ranges above the 75th percentile or below the 25th percentile.
Within each test at each age time point (open field, sociability, social novelty, startle), animals that were extreme outliers in any of the different measures or were moderate outliers in two or more measures were excluded from the data analysis for that test at that age time point. These exclusion criteria were chosen because many measures within each test are interdependent (e.g., an animal’s locomotor activity influences that probability of them spending time in the center of the open field).In the startle tests, additional outlier exclusion was conducted for separate measures (reactivity, sensitization curves, sensitization scores, PPI), as these measures were conducted on separate days and were influenced by trial-to-trial variability. For example, some animals that did not startle on trial 1 in the sensitization sessions showed extremely high sensitization scores that are not representative of their typical behavior or their respective groups. These animals were excluded for sensitization score statistical analysis, but not necessarily for the PPI statistical analysis, given that PPI was conducted on a separate day when these animals showed values comparable to their respective groups. This resulted in slightly different sample sizes for different startle measures.
The final animal numbers per group for each test at each age time point are summarized in Table 1. For each behavioral test, a three or four-way analysis of variance (ANOVA) was conducted separately for adolescent and adult data for each dependent variable. In the startle test, the dependent variables were startle reactivity (unit: arbitrary units), sensitization (no unit, normalized value), and PPI (no unit, normalized value). In the open field test, the dependent variables were total distance traveled in the 30 min session (unit: meters) and the total time spent in the center of the open field (unit: seconds). In the social behavior test, the dependent variables were time spent sniffing the animal holders in the sociability stage (unit: seconds) and time spent sniffing the animal holders in the social novelty stage (unit: seconds). All ANOVAs included genotype, sex and MIA as between-subject factors. Additional within-subject factors were included based on the measure of interest: Stimulus intensity for startle reactivity, trial/bin number for sensitization curves, pre-pulse intensity and ISI for PPI, and animal holder side for social behavior. All post-hoc testing was adjusted using the Bonferroni adjustment for multiple comparison.
Results
Maternal serum cytokine response
To confirm the efficacy of our Poly I:C MIA, the maternal serum cytokine response was measured 3 h post-injection. The proinflammatory cytokines of interest were IL-6 and TNFα, commonly elevated following Poly I:C exposure (Esslinger et al., 2016; Goeden et al., 2016; Mueller et al., 2019; Openshaw et al., 2019). There was a significant increase in serum IL-6 (t = 3.065; df = 7, p = 0.0091) and TNFα (t = 4.186; df = 7, p = 0.002) 3 h after Poly I:C administration, confirming that the protocol was successful in inducing a strong maternal immune response (Figure 2). Cytokine levels in saline dams were all just above the detection threshold of the ELISA. For both cytokines, data from Poly I:C dams were variable, but even the least responsive Poly I:C dam had an elevated level of IL-6 and TNFα compared to all saline dams (IL-6 highest saline dam–65 pg/mL; IL6 lowest Poly I:C dam–110 pg/mL; TNFα highest saline dam–21 pg/mL; TNFα lowest Poly I:C dam–29 pg/mL).
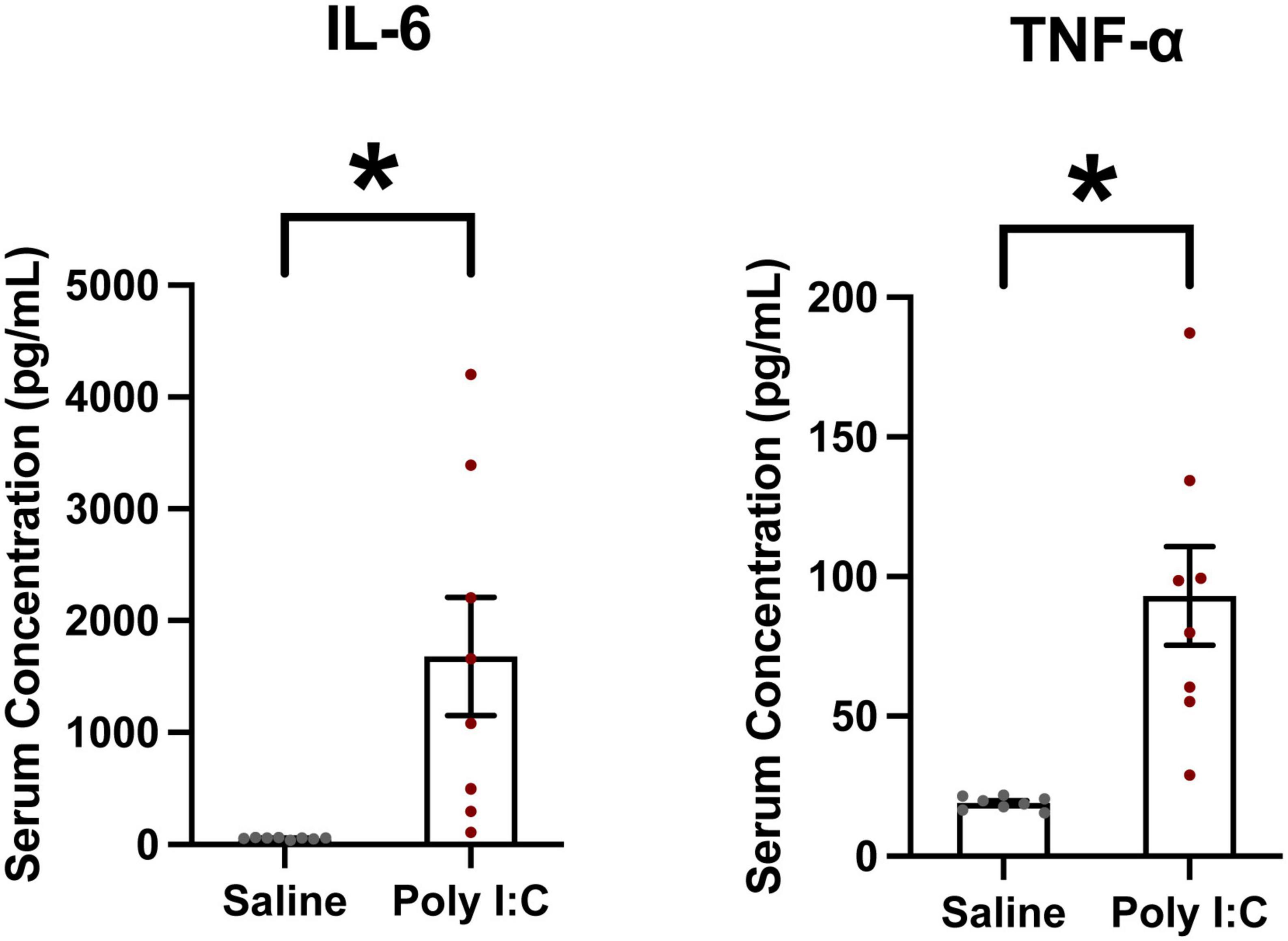
Figure 2. Poly I:C MIA significantly increases circulating levels of proinflammatory cytokines Interleukin-6 (IL-6) and Tumor Necrosis Factor α (TNFα) in maternal serum. Serum samples were collected 3 h following Poly I:C injection and quantified using ELISA. Both IL-6 and TNFα were significantly elevated in serum from Poly I:C-treated dams compared to saline-treated dams. Data are shown as mean ± standard error, *p < 0.05. Individual dots represent individual dams (n = 8 per group).
Acoustic startle phenotypes
Reactivity to various stimulus intensities
A separate repeated measures ANOVA was conducted at each age time point with stimulus intensity (65 to 120 dB in 5 dB steps) as a within subject factor and genotype, MIA, and sex as between subject factors. In adolescence, the ANOVA revealed a significant main effect of genotype [F(2,161) = 77.791, p < 0.001] on startle response amplitudes, and a stimulus intensity*genotype interaction [F(13.1,1053) = 10.096, p < 0.001]. Following post-hoc testing, Cntnap2–/– offspring exhibited significantly increased startle reactivity compared to both Cntnap2+/– and WT offspring, regardless of MIA status, at all stimulus intensities except 70 and 85 dB (adjusted p < 0.05 for each significant comparison, indicated by the # symbol in Figures 3A, B).
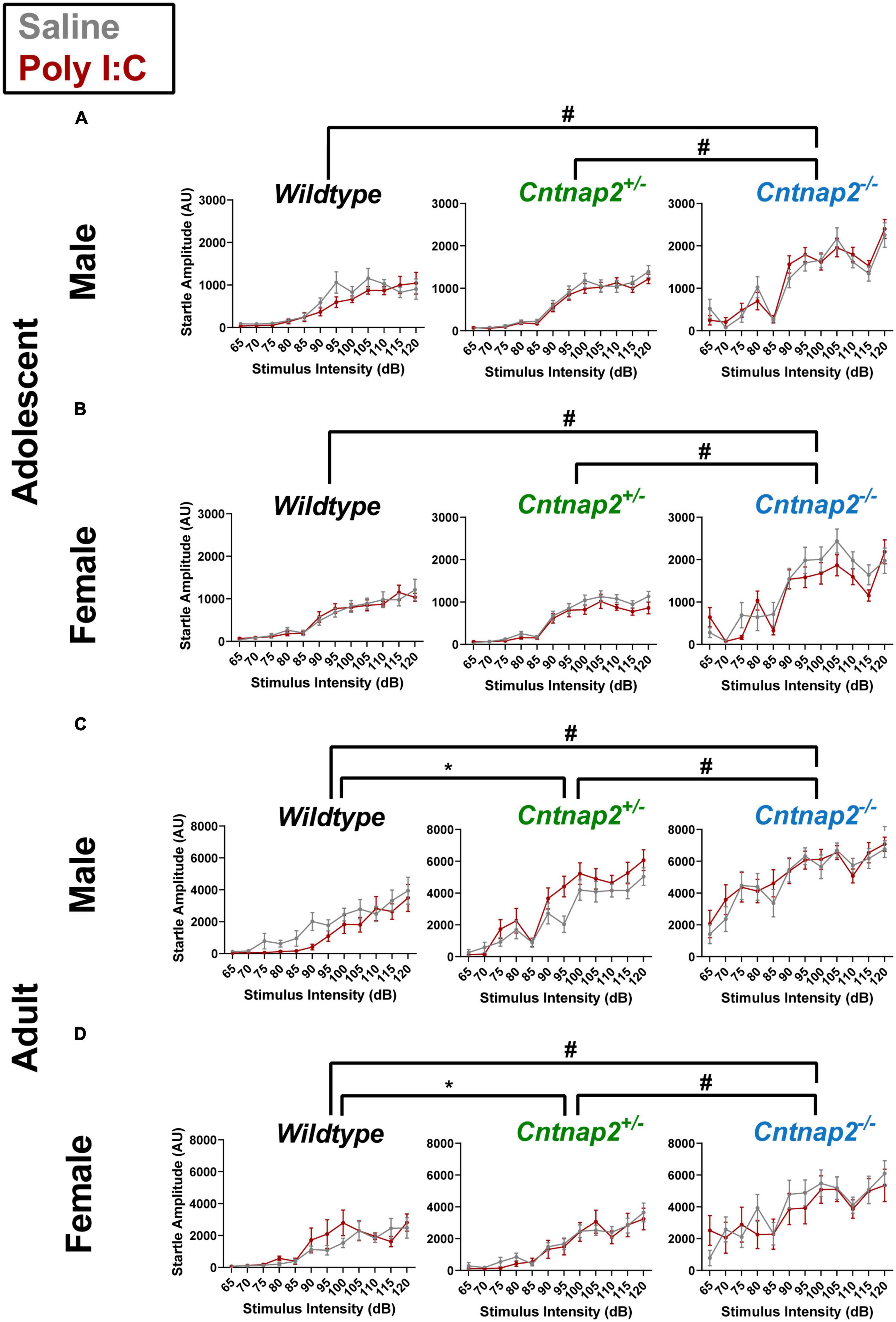
Figure 3. Partial and full Cntnap2 knockout show age-dependent effects on startle reactivity. (A,B) Cntnap2–/– adolescent animals show a general increase in startle reactivity compared to Cntnap2+/– animals at all stimulus intensities except at 70 and 85 dB (adjusted #p < 0.05 for each stimulus intensity comparison). (C,D) In adulthood, Cntnap2–/– offspring startled significantly higher than Cntnap2+/– and WT offspring at all intensities (#p < 0.05 for each stimulus intensity comparison), whereas Cntnap2+/– offspring startled significantly higher than WT offspring at 100 dB or higher intensities (adjusted *p < 0.05 for each stimulus intensity comparison). Data are shown as mean ± standard error. For information on sample sizes for each group, please see Table 1.
In adulthood, there was also a significant main effect of genotype [F(2,152) = 57.377, p < 0.001] and a significant stimulus intensity*genotype interaction [F(15.0,1143) = 10.096, p < 0.001]. Post-hoc testing revealed that Cntnap2–/– offspring startled more than Cntnap2+/– and WT offspring at all stimulus intensities (adjusted p < 0.05 for each significant comparison, indicated by the # symbol in Figures 3C, D). In addition, Cntnap2+/– offspring startled more than WT offspring at all decibels louder than 95 dB (adjusted p < 0.05 for each significant comparison, indicated by the * symbol in Figures 3C, D). Although the curves in Figures 3C, D suggest that males were influenced differently from females, this effect did not reach statistical significance in the form of a genotype*sex interaction [F(2,152) = 1.890, p = 0.155] or a stimulus intensity*genotype*sex interaction [F(15.03,1143) = 1.163, p = 0.295].
Overall, these data show that Cntnap2–/– offspring, regardless of Poly I:C MIA or sex, exhibit increased startle reactivity to a broad range of stimulus intensities in both adolescence and adulthood. In addition, there is an intermediate reactivity phenotype in Cntnap2+/– offspring that manifests in adulthood.
Sensitization of startle upon repeated stimulation
A feature that makes the acoustic startle response a great measure of pre-attentive sensory processing is how it can be modulated in various conditions. In the case of repeated stimulation to the same startle stimulus, the response can either sensitize (increase) or habituate (decrease), with each of these processes having distinct cellular and molecular correlates (Koch, 1999). Therefore, the startle amplitude in every consecutive trial is a sum of an individual’s baseline reactivity, habituation, and sensitization during that particular trial.
One of the outstanding questions from previous experiments in both humans and preclinical models is whether the increase in startle amplitude is due to an increase in baseline reactivity or an increase in sensitization. Closer inspection of our data from Figures 3A, B indicated that sensitization is playing a role in our results. Specifically, we noted a marked decrease in the startle response to 85- and 115 dB trials in adolescent Cntnap2–/– offspring. In our startle protocol, these 2 trials were presented earlier in the session compared to other trials of comparable stimulus durations and intensities (80 and 90 dB; 110 and 120 dB). To further investigate whether our startle reactivity phenotype was due to altered sensitization, we conducted a follow-up experiment where animals were exposed to 30 trials of 100 dB stimuli. We used an ITI of 1 min to minimize short-term habituation and therefore induce a robust sensitization effect. In addition, to account for inter-trial variability, we binned the trials after trial 1 into 9 separate bins of 3–4 trials each, with trial 1 representing the baseline reactivity and the different bins representing reactivity influenced by sensitization and habituation throughout the session.
A repeated measures ANOVA was conducted with trial 1/bins 1–9 as a within subject factor and genotype, MIA, and sex as between subject factors. In adolescence, there was a significant genotype*MIA*sex interaction [F(2,161) = 3.453, p = 0.034] on startle response amplitudes. To follow up on this interaction, separate ANOVAs were conducted for each sex. In males, there was a significant genotype*MIA interaction [F(2,83) = 5.308, p = 0.007]. Post-hoc testing revealed that Poly I:C Cntnap2–/– males startled significantly higher throughout the session compared to saline Cntnap2–/– males (p < 0.001; indicated by the * symbol in Figure 4A). This effect was not present in adolescent Cntnap2–/– females (Figure 4C). In addition, both males and females showed a significant main effect of genotype, whereby Cntnap2–/– offspring started significantly higher throughout the session compared to both Cntnap2–/– and WT offspring (adjusted p < 0.001 for both comparisons; indicated by the # symbol in both Figures 4A, C).
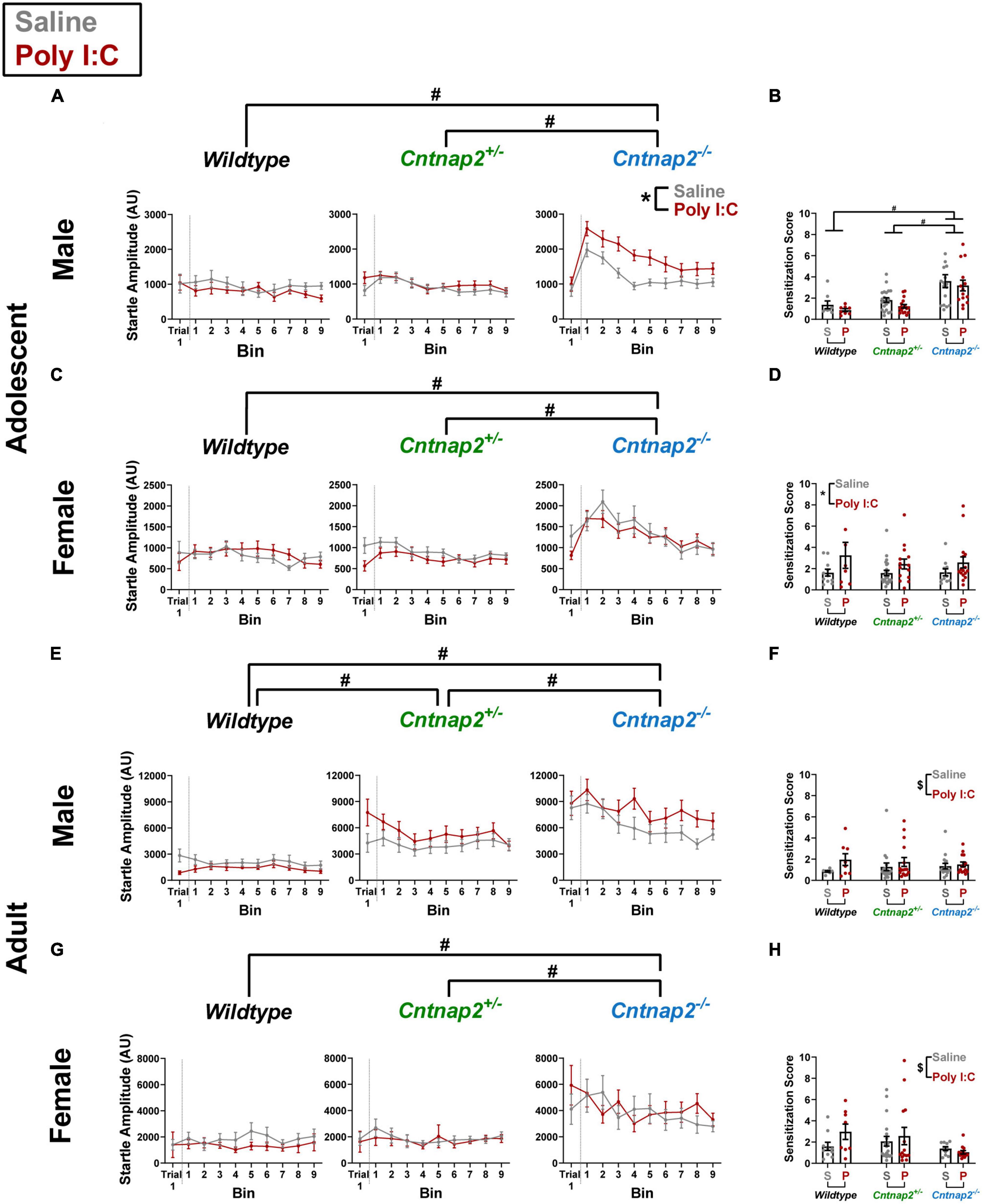
Figure 4. Independent and synergistic effects of Cntnap2 knockout and Poly I:C MIA on sensitization of startle that manifest in an age, sex, and measurement-specific manner. (A) Adolescent Cntnap2–/– males show increased startle reactivity compared to both Cntnap2+/– and WT males across the entire session (adjusted p < 0.001 for both comparisons; independent genotype effect indicated by the # symbol). In addition, Cntnap2–/– Poly I:C males show increased startle reactivity compared to Cntnap2–/– saline males (p < 0.001; synergistic genotype-MIA effect indicated by the * symbol). (B) Sensitization scores show that only the independent genotype effect remains after normalizing the baseline startle response amplitude (adjusted p < 0.001; indicated by the # symbol). (C) Adolescent Cntnap2–/– females how increased startle reactivity compared to both Cntnap2+/– and WT females across the entire session (adjusted p < 0.001 for both comparisons; independent genotype effect indicated by the # symbol). (D) Adolescent Poly I:C females show increased sensitization scores compared to adolescent saline females regardless of genotype (p = 0.04; indicated by the * symbol). (E) Adult Cntnap2–/– and Cntnap2+/– males both show increased startle reactivity compared to WT males across the entire session (adjusted p < 0.001 for both comparisons; independent genotype effect indicated by the # symbol). (F) Genotype does not alter sensitization scores in adult males, but Poly I:C offspring sensitize more than saline offspring regardless of sex and genotype (p = 0.047 indicated by the $ symbol). (G) Adult Cntnap2–/– females how increased startle reactivity compared to both Cntnap2+/– and WT females across the entire session (adjusted p < 0.001 for both comparisons; indicated by the # symbol). (H) Genotype does not alter sensitization scores in adult females, but Poly I:C offspring sensitize more than saline offspring regardless of sex and genotype (p = 0.047 indicated by the $ symbol). Data are shown as mean ± standard error. For information on sample sizes for each group, please see Table 1.
In adulthood, there were no significant effects associated with Poly I:C MIA but there was a significant genotype*sex interaction [F(2,152) = 5.747, p = 0.004]. Post-hoc testing revealed that both Cntnap2–/– and Cntnap2+/– males startled significantly higher throughout the session compared to WT males (adjusted p < 0.001 for both comparisons; indicated by the # symbol in Figure 4E). In contrast, Cntnap2+/– females were not significantly different from WT females, and the only difference in female offspring was that of increased startle amplitude across the session in Cntnap2–/– females compared to both Cntnap2+/– and WT females (adjusted p = 0.001 and 0.002, respectively; indicated by the # symbol in Figure 4G).
A challenge that arises when comparing sensitization curves between groups is that they do not properly account for each animal’s baseline reactivity, which can lead to false sensitization effects if animals of one group startled more intensely, as is the case for Cntnap2 knockouts. It is known that the biggest sensitization effect is typically measured in early trials (Groves and Thompson, 1970). Therefore, we calculated a sensitization score by dividing each animal’s average startle amplitude in bin 1 by its amplitude in trial 1 and compared the resulting scores between groups.
A separate univariate ANOVA was conducted for the sensitization scores at each age with genotype, MIA, and sex as between subject factors. In adolescence, there was a significant genotype*sex interaction [F(2,157) = 7.695, p = 0.001], a significant MIA*sex interaction [F(1,157) = 9.1, p = 0.003], as well as a main effect of genotype [F(2,157) = 6.583, p = 0.002]. Post-hoc testing showed that Cntnap2–/– males sensitized significantly more than Cntnap2+/– (p < 0.001) and WT males (adjusted p < 0.001; indicated by the # symbol in Figure 4B), and this effect was absent in females (Figure 4D). Moreover, Poly I:C females sensitized significantly more than saline females regardless of genotype, and this effect was absent in males (p = 0.04; indicated by the * symbol in Figure 4D).
In adulthood, the ANOVA showed a significant main effect of Poly I:C MIA [F(1,141) = 4.019, p = 0.047] where Poly I:C offspring showed higher sensitization compared to saline offspring regardless of genotype (indicated by the $ symbol in Figures 4F, H). In contrast to adolescent data, there were no genotype-related effects on sensitization in adulthood.
These data show that during adolescence, Poly I:C MIA increases reactivity over 30 trials in Cntnap2–/– males, but this increase was not reflected in the sensitization score, suggesting that differences in baseline reactivity may have skewed the results. Moreover, Cntnap2–/– offspring regardless of MIA, as well as MIA female offspring regardless of genotype, showed increased sensitization scores in adolescence. In adulthood, startle reactivity was increased due to complete Cntnap2 knockout, similar to what was seen in the previous experiments, and Cntnap2+/– males showed an intermediate reactivity phenotype. Cntnap2 knockout rats did not show increased sensitization, whereas Poly I:C MIA offspring, regardless of genotype, showed increased sensitization.
Pre-pulse inhibition
A repeated measures ANOVA was conducted at each age with pre-pulse intensity and ISI as within subject factors, and genotype, MIA, and sex as between subject factors. In adolescence, there was a significant ISI*genotype*MIA interaction [F(2,155) = 4.447, p = 0.013]. To further investigate this interaction, 2 follow-up ANOVAs were conducted for each ISI. For 30 ms ISI, there were significant main effects of genotype [F(2,155) = 30.001 p < 0.001; adjusted p < 0.001 for both Cntnap2–/– vs. Cntnap2+/– and Cntnap2–/– vs. WT; indicated by the # symbol in Figure 5A] and MIA [F(1,155) = 6.247, p = 0.013; Poly I:C < saline; indicated by the * symbol in Figure 5A], as well as a significant genotype*MIA interaction [F(2,155) = 6.054, p = 0.003]. Post-hoc testing revealed that Poly I:C Cntnap2–/– offspring have decreased PPI compared to saline Cntnap2–/– offspring (adjusted p = 0.001; indicated by the $ symbol in Figure 5A). For 100 ms ISI, there was a main effect of genotype [F(2,155) = 13.873], where Cntnap2–/– offspring showed decreased PPI regardless of MIA (adjusted p < 0.001 for both comparisons; indicated by the # symbol in Figure 5C).
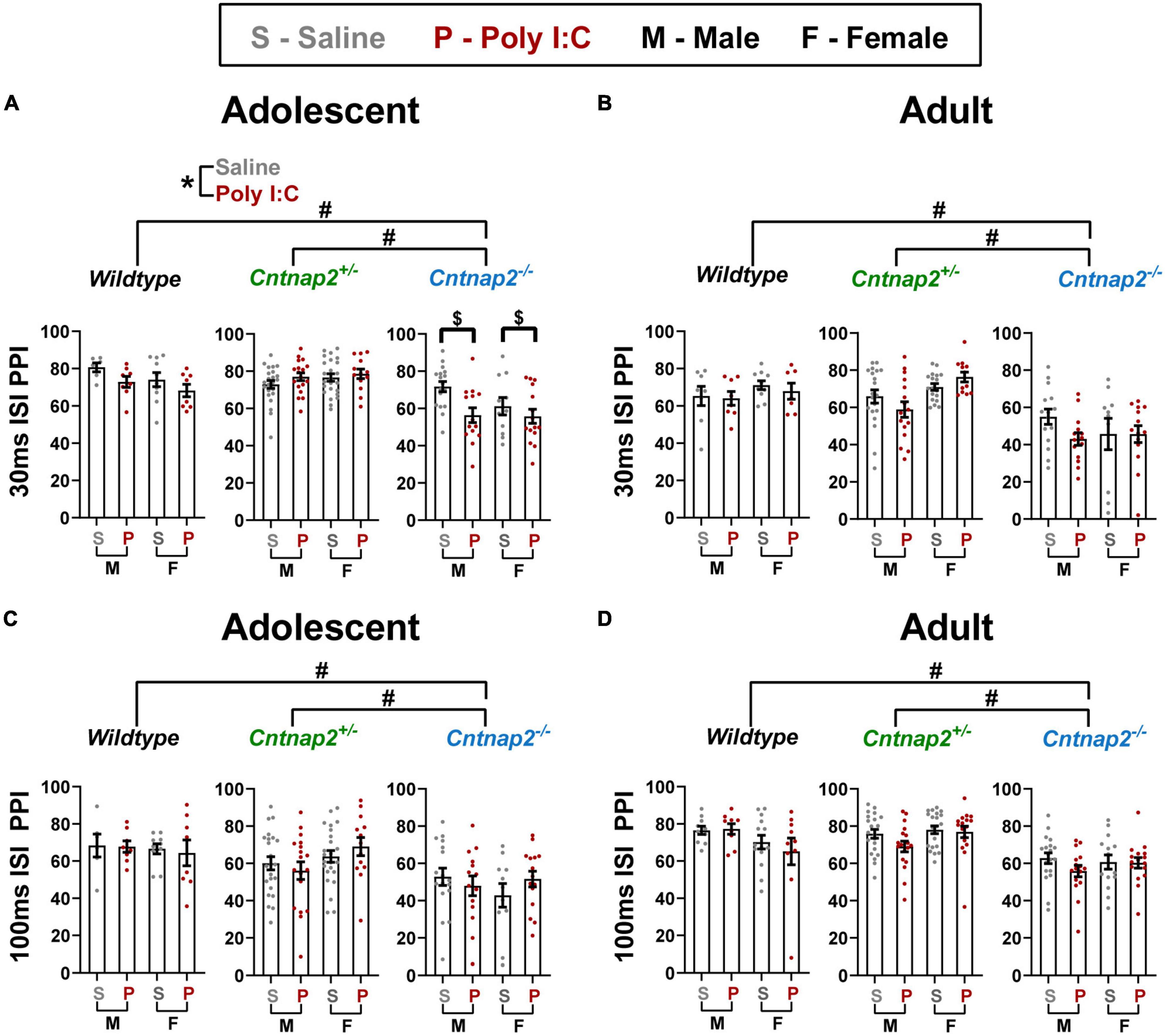
Figure 5. Cntnap2 deficiency impairs PPI at all stimulus parameters, but Poly I:C MIA leads to further reduction in PPI in Cntnap2–/– adolescent offspring with 30 ms ISIs. (A) Adolescent Cntnap2–/– offspring show significantly reduced PPI at 30 ms ISI compared to both WT and Cntnap2+/– animals (adjusted p < 0.001; indicated by the # symbol), and Poly I:C MIA leads to further reduction of PPI in an independent manner across all genotypes (p = 0.013; indicated by the * symbol) and in a synergistic manner in Cntnap2–/– males (p = 0.003; indicated by the $ symbol). (B) In adulthood, Poly I:C MIA has no influence on PPI, but Cntnap2–/– offspring still show greatly reduced PPI at 30 ms ISI (adjusted p < 0.001; indicated by the # symbol). (C,D) At 100 ms ISI, Cntnap2–/– offspring show reduced PPI in both adolescence and adulthood (p < 0.001; indicated by the # symbol) and Poly I:C MIA does not influence PPI in any of the genotype groups. Data are shown as mean ± standard error. For information on sample sizes for each group, please see Table 1.
In adulthood, there was a significant ISI*genotype interaction [F(1,141) = 41.149, p < 0.001] where Cntnap2–/– offspring showed decreased PPI compared to Cntnap2+/– (adjusted p < 0.001) and WT (adjusted p < 0.001) offspring at both ISIs, but the extent of the decrease was much higher at 30 ISI (approximately 28% less PPI; indicated by the # symbol in Figure 5B) than at 100 ISI (approximately 7% less PPI; indicated by the # symbol in Figure 5D). Similarly, there was a significant genotype*sex interaction [F(2,141) = 4.637, p = 0.011], whereby both sexes showed decreased PPI in Cntnap2–/– offspring compared to Cntnap2+/– (adjusted p < 0.001) and WT (adjusted p < 0.001) offspring, but the extent of decrease in females was higher than that in males (approximately 21% decrease in females compared to 13.5% in males; data not shown).
Overall, PPI data show that Poly I:C MIA and Cntnap2–/– genotype both independently and synergistically decrease PPI in adolescent male offspring. The Cntnap2–/– genotype effect persists into adulthood, whereas the independent and synergistic Poly I:C MIA effects do not.
Open field exploration
Total distance traveled
Here, we used the open field test to assess locomotor hyperactivity in saline and Poly I:C MIA offspring across age, sex, and Cntnap2 genotypes by measuring the total distance traveled throughout the 30-min test session. A separate univariate ANOVA was conducted for each age with genotype, MIA, and sex as between-subject factors. In adolescence, there was a main effect of genotype [F(2,175) = 18.363, p < 0.001], and post-hoc testing revealed a significant increase in total distance traveled in Cntnap2–/– offspring compared to both Cntnap2+/– offspring (p < 0.001 for both comparisons, indicated by the # symbol in Figure 6A).
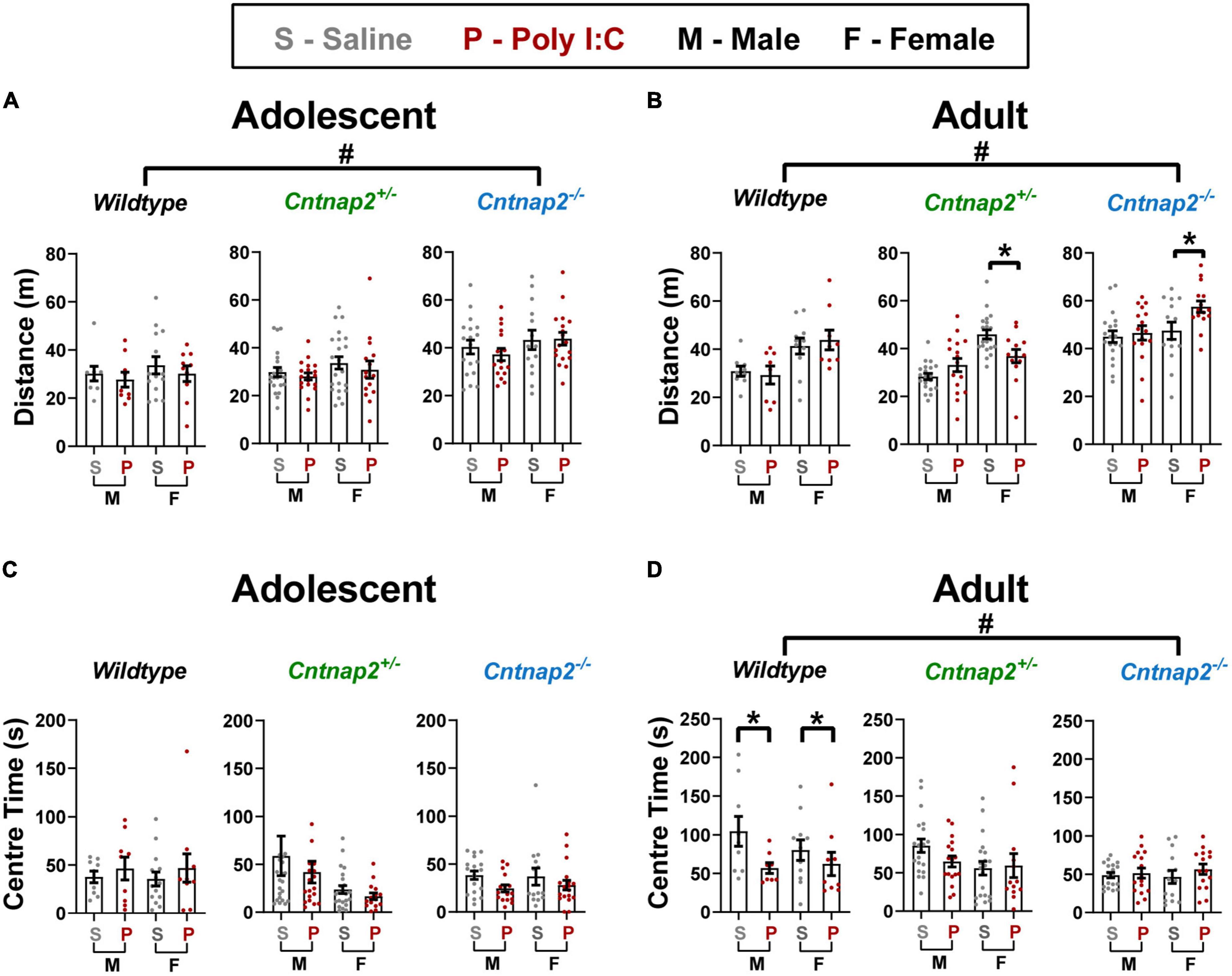
Figure 6. Poly I:C MIA influences locomotor activity and anxiety in the open field in a sex and genotype-dependent manner, whereas full Cntnap2 deficiency induces changes in open field exploration in both adolescence and adulthood. (A) Adolescent Cntnap2–/– offspring show hyperactivity measured by total distance traveled in the open field compared to WT and Cntnap2+/– offspring at 6 weeks of age (p < 0.001 for both comparisons; indicated by the # symbol). (B) In adulthood, Cntnap2–/– hyperactivity persists (p < 0.001 for both comparisons; indicated by the # symbol), but Poly I:C MIA leads to reduced activity in Cntnap2+/– females (p = 0.019) and increased activity in Cntnap2–/– females (0.013; both Poly I:C effects indicated by the * symbol). (C) Anxiety as measured by time spent in the center is not altered by either Cntnap2 deficiency or Poly I:C MIA in adolescence. (D) In adulthood, Poly I:C WT offspring show reduced center time compared to WT saline offspring (p = 0.008; indicated by the * symbol), but Poly I:C MIA has no effects on Cntnap2+/– or Cntnap2–/– offspring. Additionally, Cntnap2–/– offspring show significantly decreased center time compared to WT offspring (p = 0.049 and p = 0.004, respectively; indicated by the # symbol). Data are shown as mean ± standard error. For information on sample sizes for each group, please see Table 1.
In adulthood, the ANOVA revealed a significant main effect of genotype [F(2,159) = 31.767, p < 0.001; Figure 6B] and a significant genotype*MIA*sex interaction [F(2,159) = 5.346, p = 0.006]. To further investigate this interaction, two follow-up ANOVAs for each sex revealed a significant genotype*MIA interaction in females only [F(2,82) = 6.218, p = 0.003], where Cntnap2+/– Poly I:C females traveled less than Cntnap2+/– saline females (p = 0.019) and Cntnap2–/– Poly I:C females traveled more than Cntnap2–/– saline females (p = 0.013; both Poly I:C effects indicated by the * symbol in Figure 6B). In contrast, there was only a main effect of genotype in males [F(2,83) = 25.346, p < 0.001] where Cntnap2–/– males showed higher distance traveled compared to both Cntnap2+/– and WT males (p < 0.001 for both comparisons, indicated by the # symbol in Figure 6B). In summary, we confirmed that Cntnap2–/– rats are hyperlocomotive, with mild and opposing effects of MIA in female adult Cntnap2+/– and Cntnap2–/– rats.
Center time
Next, we tested the separate and combined effects of Cntnap2 genotype, Poly I:C MIA, sex age on the time spent in the center of the open field, a rodent measure of anxiety behavior. A separate univariate ANOVA was conducted for each age with genotype, MIA, and sex as between-subject factors. In adolescence, there were no significant main effects or interactions associated with genotype or MIA (Figure 6C). In adulthood, there was a main effect of genotype [F(2,159) = 6.040, p = 0.003], where Cntnap2–/– animals spent significantly less time in the center of the open field compared to Cntnap2+/– and WT offspring (p = 0.049 and p = 0.004, respectively; indicated by the # symbol in Figure 6D). Additionally, there was a significant genotype*MIA interaction [F(2,159) = 3.302, p = 0.039] and post-hoc testing revealed that WT Poly I:C offspring spent less time in the center of the open field compared to WT saline offspring regardless of sex (p = 0.008; indicated by the * symbol in Figure 6D).
Social behavior in the 3-chamber test
For the habituation stage of the test, a repeated measures ANOVA was conducted to test for animal side preference before introducing the social stimuli. This ANOVA had side as within subject factor and genotype, MIA, and sex as between subject factors. In adolescence, there was a significant main effect of side, where animals generally preferred spending more time in the left chamber compared to the right chamber [F(1,182) = 3.96, p = 0.048; average of 257 s in the left chamber compared to 241 s in the right chamber]. However, there were no significant interactions between side preference and any of the between subject factors. In adulthood, a similar ANOVA also showed a main effect of side, where animals generally preferred spending more time in the left chamber compared to the right chamber [F(1,172) = 71.4, p < 0.001; 267 s in the left chamber compared to 218 s in the right chamber]. In addition, the ANOVA also showed an interaction between MIA and side [F(1,172) = 4.719, p = 0.031], where Poly I:C animals preferred the left chamber slightly more than saline animals by spending approximately 11 s more in that chamber compared to saline animals, regardless of genotype or sex. Together, these data indicate that our experimental setup induced a left side preference in all animals. However, it is unlikely that this side preference influenced our sociability and social novelty results presented below given (1) the lack of interaction with genotype, MIA, and sex in adolescence, (2) the relatively small magnitude of the interaction with MIA in adulthood, and (3) the fact that we randomized side selection such that the placement of the social stimuli occurred with roughly the same probability in the left and right chambers.
For each of the sociability and social novelty tests, a repeated measures ANOVA was conducted at each age with side as a repeated factor and genotype, MIA, and sex as between subject factors. The social side is considered the one which control animals typically prefer, that is, the side with the conspecific in the sociability test and the side with the novel conspecific in the social novelty test.
For the sociability test, adolescent statistical testing showed a significant side*genotype*MIA interaction [F(2,173) = 4.443, p = 0.003]. To follow up on this interaction, a separate analysis was conducted for each genotype which showed a significant side*MIA interaction for Cntnap2+/– offspring [F(1,76) = 5.305, p = 0.024]. Together, these data show that Poly I:C Cntnap2+/– offspring spent less time sniffing the social animal holder compared to saline Cntnap2+/– offspring (p = 0.013; indicated by the * symbol in Figure 7A). In adulthood, there were no significant main effects or interactions associated with either genotype or MIA for time spent sniffing the social vs. non-social animal holders (Figure 7B). Overall, these data show that Poly I:C MIA reduces but does not abolish sociability preference in Cntnap2+/– offspring, which can be considered a relatively mild social deficit in this experimental protocol.
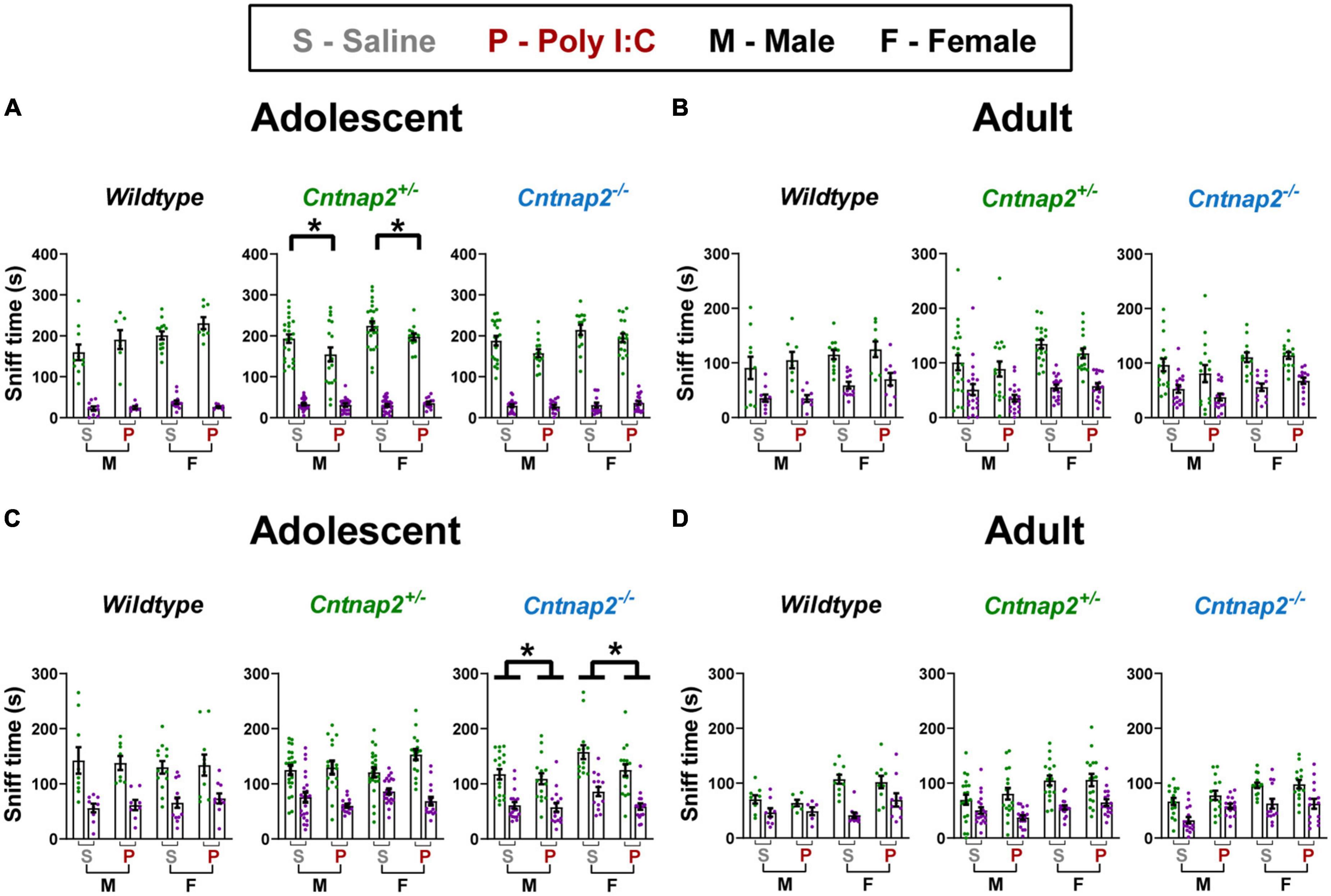
Figure 7. Poly I:C reduces social exploration but not social preference in adolescent Cntnap2+/– and Cntnap2–/– offspring. In all groups at both ages, time spent in the social chamber/exploring the social animal holder was significantly more than the time spent in the non-social chamber/exploring the non-social animal holder, indicating that all groups showed strong preference for the social side/stimulus. (A) Poly I:C MIA reduced time spent sniffing the social stimulus in adolescent Cntnap2+/– but not WT or Cntnap2–/– offspring (p = 0.013; indicated by the * symbol). (B) There were no significant effects associated with Poly I:C MIA or Cntnap2 genotype in adult sociability exploration. (C) Cntnap2–/– Poly I:C offspring spent significantly less time sniffing animal holders on both the social and non-social sides compared to their Cntnap2–/– saline offspring. (D) There were no significant effects associated with Poly I:C MIA or Cntnap2 genotype in adult social novelty exploration. Data are shown as mean ± standard error. For information on sample sizes for each group, please see Table 1.
For the social novelty test, adolescent statistical testing showed a significant genotype*MIA effect [F(2,170) = 3.445 p = 0.034] in the total time spent sniffing both social and non-social animal holders. Post-hoc tests revealed that Poly I:C Cntnap2–/– offspring spent less time sniffing both the novel stranger animal holder and the familiar animal holder compared to saline Cntnap2–/– offspring (p = 0.003; indicated by the * symbol in Figure 7C). In adulthood, there were no significant main effects or interactions associated with either Genotype or MIA for the time spent sniffing the social vs. non-social animal holders (Figure 7D). Overall, these data show that Poly I:C MIA reduces social novelty exploration time but not preference in adolescent Cntnap2–/– offspring, which can be considered a mild social deficit in this experimental protocol.
Discussion
The findings of this study demonstrate how genetic and environmental risk factors can act both independently and synergistically to alter ASD-related behavioral phenotypes in a sex and age-specific manner. The synergistic effects induced by Poly I:C MIA in Cntnap2+/– and Cntnap2–/– animals provide support for the gene-environment interaction hypothesis by showing that the genetic deficiency made animals more susceptible to the effects of the environmental insult. One of the primary goals of our study was to characterize the sensory processing phenotype of our double-hit model through startle phenotypes, which are known for their translatability across species (Koch, 1999; Swerdlow et al., 1999; Braff et al., 2001).
Effects on startle reactivity and sensitization
Several studies have shown that autistic individuals exhibit increased reactivity to acoustic stimuli, even those as quiet as 65 dB (Kohl et al., 2014; Takahashi et al., 2014, 2018; Ebishima et al., 2019). Similarly, our group has shown that both the Cntnap2–/– animals and Poly I:C MIA offspring show increased startle reactivity (Scott et al., 2018, 2020; Haddad et al., 2020a). We sought to extend these findings by attempting to decipher whether the increased reactivity is due to increased baseline startle or increased sensitization. Firstly, our results replicated the increased startle reactivity in Cntnap2–/– offspring that has been associated with altered brainstem auditory processing and was ameliorated by systemic injection of the Gamma-Aminobutyric Acid (GABA)B receptor agonist R-baclofen (Scott et al., 2018, 2020; Möhrle et al., 2021). In contrast, Poly I:C MIA did not alter startle reactivity, which conflicts with one of our previous findings using the same MIA protocol (Haddad et al., 2020a). This difference in results could be attributed to various factors that have been shown to alter susceptibility to MIA, including Poly I:C batch, rat strain, and housing conditions, all of which were different compared to our previous study (Schwartzer et al., 2013; Careaga et al., 2018; Morais et al., 2018; Mueller et al., 2018).
The startle reactivity findings strongly suggested that the increased startle response amplitude may be attributed at least partly to sensitization. Interestingly, our follow-up experiment that was designed to optimally detect sensitization showed that both Cntnap2 knockout and Poly I:C MIA independently led to increased sensitization in an age and sex-specific manner. However, combined with the previous experiment, we concluded that only the increased reactivity in Cntnap2 adolescent males was due to increased sensitization.
An important implication of these findings is that the same sensory processing phenotype—increased sensitization—can be caused by different pathophysiological mechanisms—Poly I:C MIA or Cntnap2 deficiency—that manifest differently across age and sex. This could partly explain why it has been challenging to develop treatments for ASD, as therapeutics may not be able to simultaneously target multiple pathophysiological mechanisms (McCracken et al., 2021). Another implication of these findings is the importance of determining why an autistic individual develops sensory hyperreactivity. In a clinical setting, understanding whether hyperreactivity is due to an increase in baseline reactivity or an increase in sensitization can be used to more precisely inform the management of the disorder.
Effects on pre-pulse inhibition
Decreased PPI has been reported in autistic individuals and many ASD animal models, including Poly I:C MIA offspring and Cntnap2 knockout animals (Vuillermot et al., 2011; Meehan et al., 2017; Sinclair et al., 2017; Scott et al., 2018, 2020; Haddad et al., 2020b; Möhrle et al., 2021; Zheng et al., 2023). In line with these previous studies, both Cntnap2 knockout and Poly I:C MIA led to decreased PPI. In addition, the two factors also interacted in adolescent offspring to further decrease 30 ms ISI PPI in Cntnap2–/– offspring, indicating that a deficiency in Cntnap2 leaves animals susceptible to Poly I:C MIA’s detrimental effects on sensorimotor gating.
The ISI-specific changes in PPI point toward specific brain structures and molecular mechanisms involved in the MIA-Cntnap2–/– interaction that can be investigated in future studies. For instance, short ISI PPI (30 ms) is presumably mediated by projections from the ventral nucleus of the trapezoid body or the locus coeruleus to the cochlear root neurons, whereas longer ISI PPI (100 ms) is mediated by a more comprehensive circuit involving multiple brainstem nuclei that integrate multimodal pre-pulses (Gómez-Nieto et al., 2020). From a molecular perspective, it is hypothesized that PPI with short ISI is more dependent on the activation of ionotropic receptors, whereas PPI with longer ISIs depends on metabotropic neurotransmitter receptors (Jones and Shannon, 2000; Yeomans et al., 2010).
Effects on open field exploration and social behavior
Hyperactivity is linked to both core symptoms and comorbidities in ASD. The DSM V diagnostic criteria for ASD include observations of repetitive patterns of behavior, which may manifest as hyperactivity (American Psychiatric Association, 2013). Furthermore, attention deficit hyperactivity disorder is a common comorbidity in individuals with ASD (Lai et al., 2019; Mutluer et al., 2022). Interestingly, locomotor hyperactivity measured in the open field test has been reported in both Cntnap2–/– animals and Poly I:C MIA offspring (Peñagarikano et al., 2011; Tang et al., 2013; Zhu et al., 2014; da Silveira et al., 2017; Scott et al., 2020). On the other hand, anxiety is one of the comorbidities commonly associated with ASD (van Steensel et al., 2011; Hollocks et al., 2019; Lai et al., 2019; Mutluer et al., 2022) and was assessed in our study by measuring time spent in the center of the open field. Although Cntnap2–/– animals have previously shown normal open field center time compared to controls, Poly I:C MIA is known to decrease center time in this paradigm, which is linked to increased anxiety (Meyer et al., 2005; Meyer, 2006; Brunner et al., 2015; Schaafsma et al., 2017; Kim et al., 2018).
Our purpose for including the social behavior tests follows from human studies associating atypical sensory processing across various modalities with social impairments (Thye et al., 2018). Similarly, past research has shown an association between sensory over-responsivity and anxiety, the latter being a common comorbidity in autistic individuals (van Steensel et al., 2011; Green et al., 2012; Hollocks et al., 2019). Lastly, hyperactivity could be linked to increased responsivity or impaired habituation to the sensory environment.
Contrary to our prediction, the results of the open field and social behavior tests showed a different pattern of effects compared to the startle experiments. For instance, unlike the genotype-independent increase in sensitization in adult Poly I:C MIA offspring, anxiety-like behavior was only increased in Wildtype but not Cntnap2-deficient MIA offspring. Upon further inspection, this discrepancy in the center time phenotype could be explained by a floor effect in Cntnap2–/– animals. After all, complete Cntnap2 knockout substantially reduced center time, possibly to a point that masks the effects of Poly I:C MIA.
In terms of social behavior phenotypes, our experiments showed that there were subtle gene-environment interaction effects that reduced sociability and social novelty exploration but did not abolish social preference. Combined with our other findings, these subtle differences indicate that sensory phenotypes measured through the acoustic startle response may not play a role in social exploration as measured by the 3-chamber test. For instance, direct social interaction where conspecific animals are free to move and physically interact provides a richer set of sensory stimulation than in our testing paradigm. In such a scenario, sensitization or impaired PPI could stand in the way of typical social interaction, which would not necessarily be captured in the restricted 3-chamber social apparatus. Indeed, our regular interactions with Cntnap2–/– animals have shown that these animals are exceptionally alert and react excessively to handling by experimenters, even at the end of a 10-day experimental protocol during which they are handled daily.
Another key takeaway from both the locomotor hyperactivity and social behavior measures is the potential for a gene-environment interaction with Cntnap2+/– animals. This heterozygous genotype can be likened to a common gene variant that, on its own, offers a slight increase in the chance of developing ASD compared to rare variants that cause syndromic forms of ASD (Choi and An, 2021). Indeed, behavioral phenotyping has shown that Cntnap2+/– animals are comparable to Wildtype animals in many regards (Scott et al., 2020). An interaction effect with Poly I:C MIA suggests that this partial genetic deficiency could increase susceptibility to environmental insults such as maternal infection during pregnancy (Clarke et al., 2009; Meyer, 2019). Interestingly, Poly I:C MIA interactions with the Cntnap2+/– genotype present differently compared to those with Cntnap-/- genotype, as seen by our locomotor hyperactivity results.
Experimental considerations
Immune response variability
An important consideration in Poly I:C experiments is the extent of the maternal immune response that is elicited by the mother. In this experiment, the maternal immune response was confirmed through the detection of increased IL-6 and TNF-α 3 h after Poly I:C MIA. However, the cytokine response was highly variable between dams. Given that the same batch and dose of Poly I:C was used throughout our experiment, this variability could be attributed to our use of dams from four different unrelated genetic backgrounds, each with a unique immune profile that could have altered the course of the immune response mounted against Poly I:C.
Breeding scheme
Our experiment utilized a rather unique breeding scheme by generating all the litters using one male breeder and female breeders from four different families unrelated to each other and the male breeder. ASD has a strong hereditary component and paternal characteristics like age and genetic/epigenetic profiles are known to influence the risk for various neurodevelopmental disorders including ASD (Vervoort et al., 2022). More specific to this field of study, the effects of MIA are likely dependent not just on the maternal immune signals, but also on the fetal response to those signals, which could be influenced by immune-related genes passed on from the father. Therefore, to reduce the impact of paternal confounding variables and more precisely study the effects of MIA on offspring behavioral phenotypes, we decided to use the same breeding male. To our knowledge, this type of breeding scheme has not been previously performed in a Poly I:C MIA study, although it is difficult to evaluate such a claim given the paucity of breeding information provided in the majority of Poly I:C MIA studies.
Conclusion
Altogether, our study’s findings support the double-hit gene-environment interaction hypothesis of ASD by showing that Poly I:C MIA interacts with both partial and complete Cntnap2 deficiency to alter offspring behavior. By using the acoustic startle response and its modulations to measure sensory processing phenotypes, our findings can be directly translated into other preclinical ASD models and human studies. Future studies can explore the structural and functional brain correlates of these behavioral findings to locate vulnerable brain networks or molecular pathways impacted simultaneously by multiple ASD risk factors. Despite showing several double-hit interactions, it is important to consider that each risk factor also had independent effects on offspring phenotypes, which were at times additive in nature. This serves as a reminder that even if overlapping mechanisms between different risk factors are discovered, the effects of each genetic alteration or environmental exposure must still be considered in the context of ASD diagnosis, treatment, and management.
Data availability statement
The original contributions presented in this study are included in the article/Supplementary material, further inquiries can be directed to the corresponding author.
Ethics statement
This animal study was reviewed and approved by the Animal Care Committee, University of Western Ontario.
Author contributions
FH conducted experiments, analyzed data, made figures, and wrote the first draft of the manuscript. CD has helped with data acquisition and edited the manuscript. SS, FH, and CD conceptualized the study. All authors contributed to the article and approved the submitted version.
Funding
This work was supported by the Natural Sciences and Engineering Research Council of Canada (NSERC) 04472-2018 RGPIN, Canadian Institutes of Health Research (CIHR) PJF168866, and Jonathan & Joshua Memorial Funds.
Conflict of interest
The authors declare that the research was conducted in the absence of any commercial or financial relationships that could be construed as a potential conflict of interest.
Publisher’s note
All claims expressed in this article are solely those of the authors and do not necessarily represent those of their affiliated organizations, or those of the publisher, the editors and the reviewers. Any product that may be evaluated in this article, or claim that may be made by its manufacturer, is not guaranteed or endorsed by the publisher.
Supplementary material
The Supplementary Material for this article can be found online at: https://www.frontiersin.org/articles/10.3389/fnins.2023.1160243/full#supplementary-material
Footnotes
References
Abazyan, B., Nomura, J., Kannan, G., Ishizuka, K., Tamashiro, K. L., Nucifora, F., et al. (2010). Prenatal interaction of mutant DISC1 and immune activation produces adult psychopathology. Biol. Psychiatry 68, 1172–1181. doi: 10.1016/j.biopsych.2010.09.022
American Psychiatric Association (eds) (2013). Diagnostic and statistical manual of mental disorders: DSM-5, 5th Edn. Washington, DC: American Psychiatric Association.
Azzopardi, E., Louttit, A. G., DeOliveira, C., Laviolette, S. R., and Schmid, S. (2018). The role of cholinergic midbrain neurons in startle and prepulse inhibition. J. Neurosci. 38, 8798–8808. doi: 10.1523/JNEUROSCI.0984-18.2018
Braff, D. L., Geyer, M. A., and Swerdlow, N. R. (2001). Human studies of prepulse inhibition of startle: Normal subjects, patient groups, and pharmacological studies. Psychopharmacology 156, 234–258. doi: 10.1007/s002130100810
Brimberg, L., Mader, S., Jeganathan, V., Berlin, R., Coleman, T. R., Gregersen, P. K., et al. (2016). Caspr2-reactive antibody cloned from a mother of an ASD child mediates an ASD-like phenotype in mice. Mol. Psychiatry 21, 1663–1671. doi: 10.1038/mp.2016.165
Brunner, D., Kabitzke, P., He, D., Cox, K., Thiede, L., Hanania, T., et al. (2015). Comprehensive analysis of the 16p11.2 deletion and null Cntnap2 mouse models of autism spectrum disorder. PLoS One 10:e0134572. doi: 10.1371/journal.pone.0134572
Careaga, M., Taylor, S. L., Chang, C., Chiang, A., Ku, K. M., Berman, R. F., et al. (2018). Variability in PolyIC induced immune response: Implications for preclinical maternal immune activation models. J. Neuroimmunol. 323, 87–93. doi: 10.1016/j.jneuroim.2018.06.014
Chaste, P., and Leboyer, M. (2012). Autism risk factors: Genes, environment, and gene-environment interactions. Dialog. Clin. Neurosci. 14, 281–292.
Choi, G. B., Yim, Y. S., Wong, H., Kim, S., Kim, H., Kim, S. V., et al. (2016). The maternal interleukin-17a pathway in mice promotes autism-like phenotypes in offspring. Science 351, 933–939. doi: 10.1126/science.aad0314
Choi, L., and An, J.-Y. (2021). Genetic architecture of autism spectrum disorder: Lessons from large-scale genomic studies. Neurosci. Biobehav. Rev. 128, 244–257. doi: 10.1016/j.neubiorev.2021.06.028
Clarke, M. C., Tanskanen, A., Huttunen, M., Whittaker, J. C., and Cannon, M. (2009). Evidence for an interaction between familial liability and prenatal exposure to infection in the causation of schizophrenia. AJP 166, 1025–1030. doi: 10.1176/appi.ajp.2009.08010031
Coutinho, E., Menassa, D. A., Jacobson, L., West, S. J., Domingos, J., Moloney, T. C., et al. (2017). Persistent microglial activation and synaptic loss with behavioral abnormalities in mouse offspring exposed to CASPR2-antibodies in utero. Acta Neuropathol. 134, 567–583. doi: 10.1007/s00401-017-1751-5
Crawley, J. N. (2007). Mouse behavioral assays relevant to the symptoms of autism. Brain Pathol. 17, 448–459. doi: 10.1111/j.1750-3639.2007.00096.x
Crawley, J. N. (2012). Translational animal models of autism and neurodevelopmental disorders. Dialog. Clin. Neurosci. 14, 293–305. doi: 10.31887/DCNS.2012.14.3/jcrawley
da Silveira, V. T., Medeiros, D., de, C., Ropke, J., Guidine, P. A., Rezende, G. H., et al. (2017). Effects of early or late prenatal immune activation in mice on behavioral and neuroanatomical abnormalities relevant to schizophrenia in the adulthood. Int. J. Dev. Neurosci. 58, 1–8. doi: 10.1016/j.ijdevneu.2017.01.009
Ebishima, K., Takahashi, H., Stickley, A., Nakahachi, T., Sumiyoshi, T., and Kamio, Y. (2019). Relationship of the acoustic startle response and its modulation to adaptive and maladaptive behaviors in typically developing children and those with autism spectrum disorders: A pilot study. Front. Hum. Neurosci. 13:5. doi: 10.3389/fnhum.2019.00005
Esslinger, M., Wachholz, S., Manitz, M. P., Plumper, J., Sommer, R., Juckel, G., et al. (2016). Schizophrenia associated sensory gating deficits develop after adolescent microglia activation. Brain Behav. Immun. 58, 99–106. doi: 10.1016/j.bbi.2016.05.018
Fernandes, D., Santos, S. D., Coutinho, E., Whitt, J. L., Beltrão, N., Rondão, T., et al. (2019). Disrupted AMPA receptor function upon genetic- or antibody-mediated loss of autism-associated CASPR2. Cereb. Cortex 29, 4919–4931. doi: 10.1093/cercor/bhz032
Fulcher, N., Azzopardi, E., De Oliveira, C., Hudson, R., Schormans, A. L., Zaman, T., et al. (2020). Deciphering midbrain mechanisms underlying prepulse inhibition of startle. Prog. Neurobiol. 185:101734. doi: 10.1016/j.pneurobio.2019.101734
Goeden, N., Velasquez, J., Arnold, K. A., Chan, Y., Lund, B. T., Anderson, G. M., et al. (2016). Maternal inflammation disrupts fetal neurodevelopment via increased placental output of serotonin to the fetal brain. J. Neurosci. 36, 6041–6049. doi: 10.1523/JNEUROSCI.2534-15.2016
Gómez-Nieto, R., Hormigo, S., and López, D. E. (2020). Prepulse inhibition of the auditory startle reflex assessment as a hallmark of brainstem sensorimotor gating mechanisms. Brain Sci. 10:639. doi: 10.3390/brainsci10090639
Green, S. A., Ben-Sasson, A., Soto, T. W., and Carter, A. S. (2012). Anxiety and sensory over-responsivity in toddlers with autism spectrum disorders: Bidirectional effects across time. J. Autism Dev. Disord. 42, 1112–1119. doi: 10.1007/s10803-011-1361-3
Groves, P. M., and Thompson, R. F. (1970). Habituation: A dual-process theory. Psychol. Rev. 77, 419–450.
Haddad, F. L., Patel, S. V., and Schmid, S. (2020b). Maternal immune activation by Poly I:C as a preclinical model for neurodevelopmental disorders: A focus on autism and schizophrenia. Neurosci. Biobehav. Rev. 113, 546–567. doi: 10.1016/j.neubiorev.2020.04.012
Haddad, F. L., Lu, L., Baines, K. J., and Schmid, S. (2020a). Sensory filtering disruption caused by poly I:C – timing of exposure and other experimental considerations. Brain Behav. Immun. Health 9:100156. doi: 10.1016/j.bbih.2020.100156
Hollocks, M. J., Lerh, J. W., Magiati, I., Meiser-Stedman, R., and Brugha, T. S. (2019). Anxiety and depression in adults with autism spectrum disorder: A systematic review and meta-analysis. Psychol. Med. 49, 559–572. doi: 10.1017/S0033291718002283
Hui, C. W., St-Pierre, A., El Hajj, H., Remy, Y., Hébert, S. S., Luheshi, G. N., et al. (2018). Prenatal immune challenge in mice leads to partly sex-dependent behavioral, microglial, and molecular abnormalities associated with schizophrenia. Front. Mol. Neurosci. 11:13. doi: 10.3389/fnmol.2018.00013
Jackman, C., Horn, N. D., Molleston, J. P., and Sokol, D. K. (2009). Gene associated with seizures, autism, and hepatomegaly in an amish girl. Pediatr. Neurol. 40, 310–313. doi: 10.1016/j.pediatrneurol.2008.10.013
Jiang, H., Xu, L., Shao, L., Xia, R., Yu, Z., Ling, Z., et al. (2016). Maternal infection during pregnancy and risk of autism spectrum disorders: A systematic review and meta-analysis. Brain Behav. Immun. 58, 165–172. doi: 10.1016/j.bbi.2016.06.005
Jones, C. K., and Shannon, H. E. (2000). Muscarinic cholinergic modulation of prepulse inhibition of the acoustic startle reflex. J. Pharmacol. Exp. Ther. 294, 1017–1023.
Kim, H.-J., Won, H., Im, J., Lee, H., Park, J., Lee, S., et al. (2018). Effects of Panax ginseng C.A. Meyer extract on the offspring of adult mice with maternal immune activation. Mol. Med. Rep. 18, 3834–3842. doi: 10.3892/mmr.2018.9417
Koch, M. (1999). The neurobiology of startle. Prog. Neurobiol. 59, 107–128. doi: 10.1016/S0301-0082(98)00098-7
Kohl, S., Wolters, C., Gruendler, T. O. J., Vogeley, K., Klosterkötter, J., and Kuhn, J. (2014). Prepulse inhibition of the acoustic startle reflex in high functioning autism. PLoS One 9:e92372. doi: 10.1371/journal.pone.0092372
Lai, M.-C., Kassee, C., Besney, R., Bonato, S., Hull, L., Mandy, W., et al. (2019). Prevalence of co-occurring mental health diagnoses in the autism population: A systematic review and meta-analysis. Lancet Psychiatry 6, 819–829. doi: 10.1016/S2215-0366(19)30289-5
Li, W.-Y., Chang, Y.-C., Lee, L. J.-H., and Lee, L.-J. (2014). Prenatal infection affects the neuronal architecture and cognitive function in adult mice. DNE 36, 359–370. doi: 10.1159/000362383
Lipina, T. V., Zai, C., Hlousek, D., Roder, J. C., and Wong, A. H. C. (2013). Maternal immune activation during gestation interacts with disc1 point mutation to exacerbate schizophrenia-related behaviors in mice. J. Neurosci. 33, 7654–7666. doi: 10.1523/JNEUROSCI.0091-13.2013
Manoli, D. S., and State, M. W. (2021). Autism spectrum disorder genetics and the search for pathological mechanisms. Am. J. Psychiatry 178, 30–38. doi: 10.1176/appi.ajp.2020.20111608
McCracken, J. T., Anagnostou, E., Arango, C., Dawson, G., Farchione, T., Mantua, V., et al. (2021). Drug development for autism spectrum disorder (ASD): Progress, challenges, and future directions. Eur. Neuropsychopharmacol. 48, 3–31. doi: 10.1016/j.euroneuro.2021.05.010
Meehan, C., Harms, L., Frost, J. D., Barreto, R., Todd, J., Schall, U., et al. (2017). Effects of immune activation during early or late gestation on schizophrenia-related behaviour in adult rat offspring. Brain Behav. Immun. 63, 8–20. doi: 10.1016/j.bbi.2016.07.144
Meyer, U. (2006). The time of prenatal immune challenge determines the specificity of inflammation-mediated brain and behavioral pathology. J. Neurosci. 26, 4752–4762. doi: 10.1523/JNEUROSCI.0099-06.2006
Meyer, U. (2019). Neurodevelopmental resilience and susceptibility to maternal immune activation. Trends Neurosci. 42, 793–806. doi: 10.1016/j.tins.2019.08.001
Meyer, U., Feldon, J., Schedlowski, M., and Yee, B. K. (2005). Towards an immuno-precipitated neurodevelopmental animal model of schizophrenia. Neurosci. Biobehav. Rev. 29, 913–947. doi: 10.1016/j.neubiorev.2004.10.012
Modabbernia, A., Velthorst, E., and Reichenberg, A. (2017). Environmental risk factors for autism: An evidence-based review of systematic reviews and meta-analyses. Mol. Autism 8:13. doi: 10.1186/s13229-017-0121-4
Möhrle, D., Wang, W., Whitehead, S. N., and Schmid, S. (2021). GABAB receptor agonist R-baclofen reverses altered auditory reactivity and filtering in the Cntnap2 knock-out rat. Front. Integr. Neurosci. 15:710593. doi: 10.3389/fnint.2021.710593
Morais, L. H., Felice, D., Golubeva, A. V., Moloney, G., Dinan, T. G., and Cryan, J. F. (2018). Strain differences in the susceptibility to the gut–brain axis and neurobehavioural alterations induced by maternal immune activation in mice. Behav. Pharmacol. 29, 181–198. doi: 10.1097/FBP.0000000000000374
Moy, S. S., Nadler, J. J., Perez, A., Barbaro, R. P., Johns, J. M., Magnuson, T. R., et al. (2004). Sociability and preference for social novelty in five inbred strains: An approach to assess autistic-like behavior in mice. Genes Brain Behav. 3, 287–302. doi: 10.1111/j.1601-1848.2004.00076.x
Mueller, F. S., Polesel, M., Richetto, J., Meyer, U., and Weber-Stadlbauer, U. (2018). Mouse models of maternal immune activation: Mind your caging system! Brain. Behav. Immun. 73, 643–660. doi: 10.1016/j.bbi.2018.07.014
Mueller, F. S., Richetto, J., Hayes, L. N., Zambon, A., Pollak, D. D., Sawa, A., et al. (2019). Influence of poly(I:C) variability on thermoregulation, immune responses and pregnancy outcomes in mouse models of maternal immune activation. Brain Behav. Immun. 80, 406–418. doi: 10.1016/j.bbi.2019.04.019
Mutluer, T., Aslan Genç, H., Özcan Morey, A., Yapici Eser, H., Ertinmaz, B., Can, M., et al. (2022). Population-based psychiatric comorbidity in children and adolescents with autism spectrum disorder: A meta-analysis. Front. Psychiatry 13:856208. doi: 10.3389/fpsyt.2022.856208
Openshaw, R. L., Kwon, J., McColl, A., Penninger, J. M., Cavanagh, J., Pratt, J. A., et al. (2019). JNK signalling mediates aspects of maternal immune activation: Importance of maternal genotype in relation to schizophrenia risk. J. Neuroinflammation 16, 1–11. doi: 10.1186/s12974-019-1408-5
Peñagarikano, O., Abrahams, B. S., Herman, E. I., Winden, K. D., Gdalyahu, A., Dong, H., et al. (2011). Absence of CNTNAP2 leads to epilepsy, neuronal migration abnormalities, and core autism-related deficits. Cell 147, 235–246. doi: 10.1016/j.cell.2011.08.040
Peñagarikano, O., and Geschwind, D. H. (2012). What does CNTNAP2 reveal about autism spectrum disorder? Trends Mol. Med. 18, 156–163. doi: 10.1016/j.molmed.2012.01.003
Peñagarikano, O., Lázaro, M. T., Lu, X.-H., Gordon, A., Dong, H., Lam, H. A., et al. (2015). Exogenous and evoked oxytocin restores social behavior in the Cntnap2 mouse model of autism. Sci. Transl. Med. 7:271ra8. doi: 10.1126/scitranslmed.3010257
Richetto, J., Massart, R., Weber-Stadlbauer, U., Szyf, M., Riva, M. A., and Meyer, U. (2017). Genome-wide DNA methylation changes in a mouse model of infection-mediated neurodevelopmental disorders. Biol. Psychiatry 81, 265–276. doi: 10.1016/j.biopsych.2016.08.010
Rodenas-Cuadrado, P., Pietrafusa, N., Francavilla, T., Neve, A. L., Striano, P., and Vernes, S. C. (2016). Characterisation of CASPR2 deficiency disorder – a syndrome involving autism, epilepsy and language impairment. BMC Med. Genet. 17:8. doi: 10.1186/s12881-016-0272-8
Schaafsma, W., Basterra, L. B., Jacobs, S., Brouwer, N., Meerlo, P., Schaafsma, A., et al. (2017). Maternal inflammation induces immune activation of fetal microglia and leads to disrupted microglia immune responses, behavior, and learning performance in adulthood. Neurobiol. Dis. 106, 291–300. doi: 10.1016/j.nbd.2017.07.017
Schwartzer, J. J., Careaga, M., Onore, C. E., Rushakoff, J. A., Berman, R. F., and Ashwood, P. (2013). Maternal immune activation and strain specific interactions in the development of autism-like behaviors in mice. Transl. Psychiatry 3, e240–e240. doi: 10.1038/tp.2013.16
Scott, K. E., Kazazian, K., Mann, R. S., Möhrle, D., Schormans, A. L., Schmid, S., et al. (2020). Loss of Cntnap2 in the rat causes autism-related alterations in social interactions, stereotypic behavior, and sensory processing. Autism Res. 13, 1698–1717. doi: 10.1002/aur.2364
Scott, K. E., Schormans, A. L., Pacoli, K. Y., Oliveira, C. D., Allman, B. L., and Schmid, S. (2018). Altered auditory processing, filtering, and reactivity in the Cntnap2 knock-out rat model for neurodevelopmental disorders. J. Neurosci. 38, 8588–8604. doi: 10.1523/JNEUROSCI.0759-18.2018
Sinclair, D., Oranje, B., Razak, K. A., Siegel, S. J., and Schmid, S. (2017). Sensory processing in autism spectrum disorders and Fragile X syndrome—from the clinic to animal models. Neurosci. Biobehav. Rev. 76, 235–253. doi: 10.1016/j.neubiorev.2016.05.029
Strauss, K. A., Puffenberger, E. G., Huentelman, M. J., Gottlieb, S., Dobrin, S. E., Parod, J. M., et al. (2006). Recessive symptomatic focal epilepsy and mutant contactin-associated protein-like 2. N. Engl. J. Med. 354, 1370–1377. doi: 10.1056/NEJMoa052773
Swerdlow, N. R., Braff, D. L., and Geyer, M. A. (1999). Cross-species studies of sensorimotor gating of the startle reflex. Ann. N. Y. Acad. Sci. 877, 202–216.
Takahashi, H., Nakahachi, T., Komatsu, S., Ogino, K., Iida, Y., and Kamio, Y. (2014). Hyperreactivity to weak acoustic stimuli and prolonged acoustic startle latency in children with autism spectrum disorders. Mol. Autism 5, 1–8. doi: 10.1186/2040-2392-5-23
Takahashi, H., Nakahachi, T., Stickley, A., Ishitobi, M., and Kamio, Y. (2017). Stability of the acoustic startle response and its modulation in children with typical development and those with autism spectrum disorders: A one-year follow-up. Autism Res. 10, 673–679. doi: 10.1002/aur.1710
Takahashi, H., Nakamura, T., Kim, J., Kikuchi, H., Nakahachi, T., Ishitobi, M., et al. (2018). Acoustic hyper-reactivity and negatively skewed locomotor activity in children with autism spectrum disorders: An exploratory study. Front. Psychiatry 9:355. doi: 10.3389/fpsyt.2018.00355
Tang, B., Jia, H., Kast, R. J., and Thomas, E. A. (2013). Epigenetic changes at gene promoters in response to immune activation in utero. Brain Behav. Immun. 30, 168–175. doi: 10.1016/j.bbi.2013.01.086
Thye, M. D., Bednarz, H. M., Herringshaw, A. J., Sartin, E. B., and Kana, R. K. (2018). The impact of atypical sensory processing on social impairments in autism spectrum disorder. Dev. Cogn. Neurosci. 29, 151–167. doi: 10.1016/j.dcn.2017.04.010
Valsamis, B., and Schmid, S. (2011). Habituation and prepulse inhibition of acoustic startle in rodents. J. Vis. Exp. 55:e3446. doi: 10.3791/3446
van Steensel, F. J. A., Bögels, S. M., and Perrin, S. (2011). Anxiety disorders in children and adolescents with autistic spectrum disorders: A meta-analysis. Clin. Child Fam. Psychol. Rev. 14:302. doi: 10.1007/s10567-011-0097-0
Varghese, M., Keshav, N., Jacot-Descombes, S., Warda, T., Wicinski, B., Dickstein, D. L., et al. (2017). Autism spectrum disorder: Neuropathology and animal models. Acta Neuropathol. 134, 537–566. doi: 10.1007/s00401-017-1736-4
Vervoort, I., Delger, C., and Soubry, A. (2022). A multifactorial model for the etiology of neuropsychiatric disorders: The role of advanced paternal age. Pediatr. Res. 91, 757–770. doi: 10.1038/s41390-021-01435-4
Vuillermot, S., Feldon, J., and Meyer, U. (2011). Nurr1 is not essential for the development of prepulse inhibition deficits induced by prenatal immune activation. Brain Behav. Immun. 25, 1316–1321. doi: 10.1016/j.bbi.2011.06.012
Vuillermot, S., Luan, W., Meyer, U., and Eyles, D. (2017). Vitamin D treatment during pregnancy prevents autism-related phenotypes in a mouse model of maternal immune activation. Mol. Autism 8:9. doi: 10.1186/s13229-017-0125-0
Weber-Stadlbauer, U., Richetto, J., Labouesse, M. A., Bohacek, J., Mansuy, I. M., and Meyer, U. (2017). Transgenerational transmission and modification of pathological traits induced by prenatal immune activation. Mol. Psychiatry 22, 102–112. doi: 10.1038/mp.2016.41
Xu, X., Chini, M., Bitzenhofer, S. H., and Hanganu-Opatz, I. L. (2019). Transient knock-down of prefrontal DISC1 in immune-challenged mice causes abnormal long-range coupling and cognitive dysfunction throughout development. J. Neurosci. 39, 1222–1235. doi: 10.1523/JNEUROSCI.2170-18.2018
Yeomans, J. S., Bosch, D., Alves, N., Daros, A., Ure, R. J., and Schmid, S. (2010). GABA receptors and prepulse inhibition of acoustic startle in mice and rats. Eur. J. Neurosci. 31, 2053–2061. doi: 10.1111/j.1460-9568.2010.07236.x
Zheng, A., Scott, K. E., Schormans, A. L., Mann, R., Allman, B. L., and Schmid, S. (2023). Differences in startle and prepulse inhibition in contactin-associated protein-like 2 knock-out rats are associated with sex-specific alterations in brainstem neural activity. Neuroscience 513, 96–110. doi: 10.1016/j.neuroscience.2023.01.020
Zhu, F., Zheng, Y., Liu, Y., Zhang, X., and Zhao, J. (2014). Minocycline alleviates behavioral deficits and inhibits microglial activation in the offspring of pregnant mice after administration of polyriboinosinic-polyribocytidilic acid. Psychiatry Res. 219, 680–686. doi: 10.1016/j.psychres.2014.06.046
Keywords: maternal immune activation, Cntnap2, Poly I:C, startle, pre-pulse inhibition, autism spectrum disorder, rat
Citation: Haddad FL, De Oliveira C and Schmid S (2023) Investigating behavioral phenotypes related to autism spectrum disorder in a gene-environment interaction model of Cntnap2 deficiency and Poly I:C maternal immune activation. Front. Neurosci. 17:1160243. doi: 10.3389/fnins.2023.1160243
Received: 06 February 2023; Accepted: 24 February 2023;
Published: 14 March 2023.
Edited by:
Junhua Yang, Guangdong Pharmaceutical University, ChinaReviewed by:
Cristian Perez Fernadez, University of Almería, SpainWeijie Xie, Shanghai Jiao Tong University, China
Copyright © 2023 Haddad, De Oliveira and Schmid. This is an open-access article distributed under the terms of the Creative Commons Attribution License (CC BY). The use, distribution or reproduction in other forums is permitted, provided the original author(s) and the copyright owner(s) are credited and that the original publication in this journal is cited, in accordance with accepted academic practice. No use, distribution or reproduction is permitted which does not comply with these terms.
*Correspondence: Susanne Schmid, susanne.schmid@schulich.uwo.ca