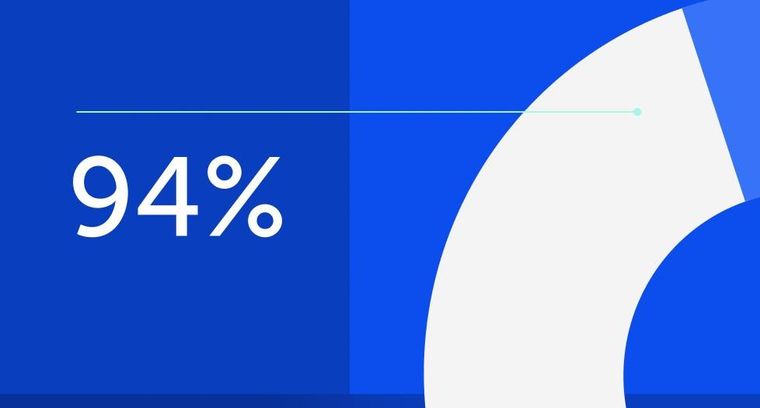
94% of researchers rate our articles as excellent or good
Learn more about the work of our research integrity team to safeguard the quality of each article we publish.
Find out more
REVIEW article
Front. Neurosci., 02 May 2023
Sec. Neuroprosthetics
Volume 17 - 2023 | https://doi.org/10.3389/fnins.2023.1156796
This article is part of the Research TopicInsights in Neuroprosthetics: 2023View all 4 articles
Objective: Noisy galvanic vestibular stimulation (nGVS) has been used to facilitate vestibular function and improve gait and balance in people with poor postural control. The aim of this scoping review is to collate, summarize and report on the nGVS parameters that have been used to augment postural control.
Method: A systematic scoping review was conducted up to December 2022. Data were extracted and synthesized from 31 eligible studies. Key nGVS parameters were identified, and the importance of these parameters and their influence on postural control evaluated.
Results: A range of nGVS parameters have been used to augment postural control, including; noise waveform, amplitude, frequency band, duration of stimulation, method of amplitude optimization, size and composition of electrodes and the electrode skin interface.
Conclusion: Systematic evaluation of the individual parameters that can be manipulated in the nGVS waveform identified that a broad array of settings have been utilized in each parameter across the studies. Choices made around the electrode and electrode-skin interface, as well as the amplitude, frequency band, duration and timing of the waveform are likely to influence the efficacy of nGVS. The ability to draw robust conclusions about the selection of optimal nGVS parameters to improve postural control, is hindered by a lack of studies that directly compare parameter settings or consider the variability in individuals’ response to nGVS. We propose a guideline for the accurate reporting of nGVS parameters, as a first step toward establishing standardized stimulation protocols.
Loss of postural control is a common consequence of both normal aging and pathological processes such as vestibular or other neurological disease (Agrawal et al., 2020a). Postural control requires appropriate and timely motor responses and is dependent on inputs from multiple sensory systems (vestibular, visual, and proprioceptive), integrated in the central nervous system (Agrawal et al., 2020b). In situations where a loss of postural control is attributed to a failure of vestibular information, there is an increased dependance on visual and proprioceptive information for balance (Sprenger et al., 2017). This can be inadequate in situations where visual and proprioceptive information is less available, or less accurate (Sprenger et al., 2017).
Noisy galvanic vestibular stimulation (nGVS), also known as stochastic vestibular stimulation (SVS), has been investigated as a treatment to improve postural control in standing and walking in healthy adults (Fujimoto et al., 2016; Wuehr et al., 2016b; Temple et al., 2018; Inukai et al., 2018a,b; Fujimoto et al., 2019; Inukai et al., 2020a,b,c; Nooristani et al., 2021), and in people with bilateral vestibulopathy (Iwasaki et al., 2014; Wuehr et al., 2016a; Fujimoto et al., 2018; Iwasaki et al., 2018; Ko et al., 2020; Sprenger et al., 2020; Chen et al., 2021; Eder et al., 2022), Parkinson’s disease (Pal et al., 2009; Samoudi et al., 2015; Wuehr et al., 2022) and multiple sclerosis (Lotfi et al., 2021). nGVS is a zero mean noisy galvanic current applied to the vestibular apparatus and its afferent nerves via electrodes placed bilaterally over the mastoid processes (Lajoie et al., 2021). It has been demonstrated to be safe, with infrequent adverse effects that have been of a mild nature (Wilkinson et al., 2009; Utz et al., 2011; Dilda et al., 2012; Lotfi et al., 2021; Matsugi et al., 2021).
While the exact mechanism of nGVS facilitation of postural control is unknown, it is assumed that there is an element of sensory restoration via stochastic resonance. nGVS boosts the weak physiological signal from the vestibular apparatus, reducing the firing threshold of vestibular irregular afferent neurons (Figure 1) (Moss et al., 2004; Yu et al., 2012; Schniepp et al., 2018; Wuehr et al., 2018; Dlugaiczyk et al., 2019). However, this response is dependent on the parameters of the noisy signal. Optimal noise parameters will enhance the physiological signal, whereas too weak a noise signal will have no effect, and too high a noise signal will degrade the information content of the physiological signal (Figure 1)(Moss et al., 2004).
Figure 1. Conceptual drawing illustrating the theoretical stochastic effect of increasing levels of noise on firing of a neuron (Created with BioRender.com). (A) A sine wave in which the signal fails to reach the threshold for neural firing. (B) A sine wave enhanced by an optimum noise signal, boosting the signal past the threshold for firing. (C) Excessive noise boosts the sine wave indiscriminately, increasing neural firing, but losing the temporal information.
While recent reviews have confirmed the beneficial effect of nGVS in healthy people (Stefani et al., 2020), people with BVP (McLaren et al., 2022), and Parkinson’s disease (Mahmud et al., 2022), improvements have not been uniform. Sprenger et al. (2020), and Nooristani et al. (2019b) found no improvement in standing balance with nGVS compared to sham controls, and Matsugi et al. (2020) and Sprenger et al. (2020) even found nGVS increased sway while standing on foam.
To develop clinical applications for nGVS it is critical that we understand which nGVS parameters will consistently and sustainably improve postural control. To date, limited systematic evaluation of parameter settings, and the variability in individual’s response to nGVS, make it unclear how parameter choice influences responsiveness and outcomes. This has resulted in a lack of consensus on the specific nGVS parameter settings to optimize postural control; frequency band, amplitude, noise waveform, electrode size, shape and composition, optimisation procedure and duration of stimulation (Dlugaiczyk et al., 2019; Fujimoto et al., 2019; Herssens and McCrum, 2019; Stefani et al., 2020; Lajoie et al., 2021).
While a plethora of parameters have been used in research, confusion remains around those providing the most favorable effect. To our knowledge, this is the first scoping review to collate, summarize and report on the different nGVS parameters used to improve postural control. Consensus on the optimum parameters has been identified as a critical next step to further this research (Herssens and McCrum, 2019; Haxby et al., 2020; Stefani et al., 2020; Lajoie et al., 2021; Pires et al., 2022). In this review, we look at the current evidence around parameter choice and give recommendations on future reporting to add rigor to nGVS research and facilitate meaningful comparison between studies.
The present scoping review was undertaken using the Arksey and O’Malley (2005) approach. A literature search was undertaken using: EBSCO (CINAHL plus, SPORTDiscus), Scopus, Ovid (AMED) and PubMed (Medline). We used the search terms (nGVS OR noisy galvanic stimulation OR Noisy vestibular stimulation OR galvanic vestibular stimulation OR GVS OR SVS OR stochastic vestibular stimulation) AND (gait OR walk* OR ambulation OR locomotion OR mobility OR balance OR stability OR posture*). Additional references were found by hand searching the reference lists of key articles. Studies were restricted to peer reviewed journals with full text available in English; no limit was placed on the publication date or study design.
Search results were imported into Covidence systematic review software (Veritas Health Innovation, Melbourne, Australia. Available at www.covidence.org). Articles were screened by title and abstract. The full text publication was reviewed independently by two reviewers (RM and DT) and eligibility determined according to the criteria in Table 1. If there was any uncertainty about inclusion, a third reviewer (PS) was consulted until a consensus was reached. The literature search was last performed on the 22/01/23.
Data were extracted directly from the text and tables into a customized template generated on Covidence software. Data extracted included: author, year of publication, study design, population, and the following nGVS parameters: skin preparation, conductive medium at the electrode skin interface, impedance restriction, electrodes used (material, size, surface area), electrode placement, waveform, frequency band, amplitude, method of optimization, duration of the stimulation and time period of assessment. Data were charted and grouped to synthesize key information regarding parameters, and a summary of the results was generated.
After removal of duplicates, the electronic search yielded 1,242 citations. The title and abstract were screened against the inclusion and exclusion criteria (Table 1), excluding 1,182 studies. Two reviewers (RM, DT) completed a full text review on 60 studies. Twenty-nine studies were excluded, leaving 31 studies for data extraction (Figure 2). Table 2 contains a summary of the studies included in this review. Review findings are reported and discussed in an integrated analysis and discussion.
The parameters used in nGVS studies are described and how these may affect the efficacy of nGVS when used to treat people with postural control deficits, are evaluated.
Electrodes are the conductive surface delivering stimulation to the skin (Table 3). Manufactured from carbon rubber or silver/silver chloride, circular, oval or rectangular in shape, they have varied in size between a surface area of 0.5cm2 to 50cm2. The effect of electrode size during nGVS has been examined in only one study. Nooristani et al. (2019a) used identical stimulation parameters applied via an electrode of 35cm2 and an electrode of 3cm2. This generated the same stimulus, but different current densities at the skin-electrode interface. The smaller sized electrodes, providing higher current density over a smaller area, resulted in a significant improvement in balance compared to the 35 cm2 electrodes, that provided results that were no different to sham stimulation. Truong et al. (2023) modeled the electrical effect of both 3, and 35cm2 electrodes and reported the smaller electrode provided a more focal stimulation of the vestibular apparatus and temporal cortex, whereas there was more current loss via the skull and cerebrospinal fluid using a 35cm2 electrode. To afford further support for the observation that smaller electrodes provide more specific, localized stimulation of the vestibular system, the size, shape and material of electrodes, and details around the electrode-skin interface, should be clearly reported.
The connection between the skin and the electrode is affected by impedance. To deliver the desired current, impedance is kept at the lowest level possible, by skin preparation to reduce the resistance of dry skin cells and the stratum corneum, combined with the use of a conductive medium between the electrode and the skin (Table 3; McAdams et al., 1996; Abe and Nishizawa, 2021). At electrical frequencies lower than 1 Hz the impedance at the interface between the electrode and the electrolytic medium provides the greatest impedance to current, whereas at frequencies between 1 and 10,000 Hz the skin, and particularly the stratum corneum, produces the most impedance (McAdams et al., 1996). Fixed bandwidth nGVS contains frequencies within both these ranges (Table 4). While skin preparation and the skin/ electrode interface are paramount in electrical stimulation protocols, they are often unreported in the nGVS literature (Table 3). The conductive interface between the electrode and skin is either a saline soaked sponge (Wuehr et al., 2016a,b; Nooristani et al., 2019b; Eder et al., 2022) or electrode gel (Mulavara et al., 2011; Goel et al., 2015; Mulavara et al., 2015; Samoudi et al., 2015; Temple et al., 2018; Ko et al., 2020; Piccolo et al., 2020; Asslander et al., 2021; Lotfi et al., 2021). Most nGVS machines measure impedance and will signal to the user if it becomes too high. Higher impedance will either reduce the current that a machine is able to deliver to the vestibular system or, will draw a higher voltage to maintain the current amplitude (it is assumed that below the specified impedance threshold the relationship between frequency and impedance should not affect the current amplitude). Only seven studies mention the level and whether impedance is controlled: keeping impedance below 600 Ohms (Mulavara et al., 2011) or 1,000 Ohms (Goel et al., 2015; Mulavara et al., 2015; Samoudi et al., 2015; Temple et al., 2018) in five studies and below 10,000 Ohms in two (Nooristani et al., 2021; Mitsutake et al., 2022). Mulavara et al. (2011) provided a frequency analysis of their signal at the machine but to our knowledge no one has investigated the waveform frequency at the skin level to determine how the conductive interface at the skin influences the final stimulation output.
Overall, details of the electrodes and electrode skin interface are not consistently reported. With the limited data available it appears that smaller electrodes arranged in a bipolar configuration may yield better postural control (Nooristani et al., 2019a). Basic science suggests that skin preparation and an interface medium to reduce skin impedance are a critical part of the stimulation process (McAdams et al., 1996; Abe and Nishizawa, 2021). As we move toward investigating the use of nGVS as either an orthotic to improve postural control or a means of enhancing rehabilitation, the features within the interface between the technology and the person are going to become more important (McLaren et al., 2016). In the people most likely to benefit from an nGVS intervention, the ear can already be a “busy” place, potentially housing hearing aids, glasses, mask loops and earrings. Small, unobtrusive, and tidy interfaces are likely to be more acceptable for regular or long-term use. This will need to be considered if this technology progresses to a consumer usability and commercial development stage.
Noisy galvanic stimulation (nGVS) is a zero-mean noisy, alternating current delivered via electrodes over the bilateral mastoid processes. The zero-mean characteristic ensures that the stimulation does not introduce a constant bias or offset to the vestibular system (Stirzaker, 2005). nGVS and stochastic vestibular stimulation (SVS) are the most commonly used terms to describe this waveform in the literature. While there are numerous types of noise, the noise referred to in the nGVS literature is primarily white noise. White noise is a random signal with a flat power spectrum, meaning its power is distributed equally across all frequencies. This is similar to white light, which contains all visible wavelengths with the same energy (Marmarelis, 2012). The most common type of white noise referred to in relation to nGVS is Gaussian distribution (Figure 3). In the context of white noise, “Gaussian” refers to the amplitude distribution of the signal, which follows a Gaussian or normal distribution (Figure 3)(Marmarelis, 2012). Both the frequency distribution and distribution of current amplitude will influence the shape of the waveform (Figures 3, 4), and mathematically we can expect this to influence stochastic resonance (Hanggi et al., 1993). While not investigated in the postural control literature, Soma et al. (2003) found pink noise nGVS out- performed white noise when used to sensitize venous baroreceptors in response to postural change. Pink noise has also improved fine motor control in people with Parkinson’s disease (Kuatsjah et al., 2019). However, lack of clarity around the waveform used, imprecise use of nomenclature, and simultaneous variation of other parameters, make the influence of noise waveform on the efficacy of nGVS unclear.
Figure 3. 1 mA, 0–30 Hz Gaussian white noise nGVS signal (Created with BioRender.com). (A) Spectral frequency graph, the waveform contains frequencies between 0 and 30 Hz with equal intensity at each frequency. (B) Time plot of the signal. The amplitude of 1 mA means that 99% of all generated amplitude values are between 0.5 mA and −0.5 mA. (C) A histogram of the distribution of the signal amplitude. A random level of current generated for every sample is normally distributed, thus the probability density function follows a bell-shaped curve.
Figure 4. Representative figure comparing the effect of frequency bandwidth on a Gaussian white noise signal (Created with BioRender.com).
nGVS current is delivered across a variety of frequency bandwidths, in which all frequencies within the bandwidth are represented. The benefits of nGVS for postural control have been seen over all these frequency bands, although it is possible that different frequency bands may be optimal for different people (Lee et al., 2021).
While the frequency band width has an influence on the shape of the noisy signal (Figure 4), to date there has not been a comprehensive comparison of the effect of different frequencies on performance (Stefani et al., 2020; Lajoie et al., 2021). Whether there is an optimum frequency band that will provide consistently improved results, and whether these frequency bands have different effects depending on the task (i.e., standing versus walking) is unknown (Wuehr et al., 2017; Inukai et al., 2018a; Lajoie et al., 2021).
Various rationales around head motion, physiology of the vestibular system or CNS function support the choice of different frequencies (Table 4). Specifically, the frequency of head motion during gait is reported as occurring around 0- 2 Hz (Hirasaki et al., 1999), whereas, the frequency of body sway is considered to sit at frequencies between 0.02-10 Hz (Asslander et al., 2021; Lajoie et al., 2021). A frequency band of 0- 30 Hz is the natural frequency of the vestibular system and is proposed to stimulate vestibular hair cells (Mulavara et al., 2011; Goel et al., 2015; Mulavara et al., 2015; Dlugaiczyk et al., 2019); and a frequency band of 0–640 Hz has been used to increase excitability when applied to the cortex (Terney et al., 2008).
In the only study comparing nGVS frequency bands, Mulavara et al. (2011) found that 8/15 participants improved their postural control with a 1–2 Hz bandwidth noise signal and 10/15 responded to a 0–30 Hz bandwidth white noise signal. There was no significant difference in the balance performance between the stimulation in the two frequency ranges. Stefani et al. (2020) found that the percentage improvement in balance was significantly larger for frequencies of 0–30 Hz compared to 0.1–640 Hz. However, the small number of studies, variation in stimulation parameters aside from frequency band, and inconsistency in outcome measures used, highlight the challenges of understanding optimum stimulation parameters.
An electric current is measured in amperes (A) and can be measured as peak amplitude (the highest value), peak-to-peak (the change from the highest to the lowest amplitude value) or as root mean squared (rms). A limitation of the literature is the failure in most cases to specify how amplitude has been measured, making it difficult to determine how comparable amplitudes are between studies.
nGVS to improve balance function has typically been delivered at a low or subthreshold amplitude; at an insufficient level to induce any cutaneous or vestibular (motion) afferent information. The amplitude must be set to a level that enhances the subthreshold neural signal without interfering with the ability of the network to detect suprathreshold signals (Collins et al., 1995). Regardless of whether nGVS works via the mechanism of stochastic resonance or direct stimulation of the vestibular afferents or hair cells, augmentation of the neural signal is only advantageous when it arrives at the appropriate rate at the precise time to enable accurate decoding in the central nervous system (Rieke, 1997). An amplitude that is too low will not facilitate a weak physiological signal, whereas an amplitude that is too high will provide too much stimulation to the vestibular system, making the signal nonsensical and risking the central nervous system degrading the weighting given to the afferent signal (Stacey and Durand, 2000).
Current amplitudes used in nGVS studies designed to improve postural control have varied from 100 to 1,200 mA (Table 5). Amplitude has been reported in the majority of studies and is generally considered a key variable in the optimization of the nGVS signal (Wuehr et al., 2018). A variety of methods have been employed to determine amplitude. While it is generally accepted that amplitude is important, the literature is divided on whether amplitude should be determined at a group level or individualized to each participant.
Using a set amplitude at a value that is most likely to be subthreshold for the group is the most straightforward method of deciding on the amplitude. Using this method, a machine can be pre-set and used with minimal training. The disadvantage of a set amplitude is that it may not be facilitatory for all. Ten studies set amplitude at a group level. One study used a 1,200 μA amplitude (Matsugi et al., 2022), four studies used a 1,000 μA amplitude (Inukai et al., 2018b; Nooristani et al., 2019a,b; Matsugi et al., 2020), one at 600 μA (Matsugi et al., 2022), five studies have used a set amplitude of 400 μA (Inukai et al., 2018a,b, 2020a,b,c), and three studies 200 μA (Inukai et al., 2020a; Nooristani et al., 2021; Matsugi et al., 2022). The varying nature of study designs and the sensory conditions utilized during assessment make it difficult to draw credible conclusions between studies (Matsugi et al., 2020; McLaren et al., 2022). Of the current amplitudes set at a group level, 200 μA demonstrated significantly reduced postural sway in two studies (Inukai et al., 2020a; Nooristani et al., 2021), and no change in a further study (Matsugi et al., 2022); 400 μA provided noted improvements in balance in four studies (Inukai et al., 2018a,b, 2020b,c) with no effect in one study. At 1,000 and 1,200 μA, the results were variable; balance improved in one study (Inukai et al., 2018b), deteriorated in one study (Matsugi et al., 2020) but had no effect (Nooristani et al., 2019b; Matsugi et al., 2022), or had limited effect (Nooristani et al., 2019a) in three other studies.
In their ground-breaking primate work investigating individual sensory thresholds to sinusoidal galvanic vestibular stimulation, Kwan et al. (2019) recorded stimulation thresholds between 400 and 600 μA. Although there are limitations in applying the findings of sinusoidal galvanic vestibular stimulation to nGVS, this highlights uncertainty about whether set amplitudes used in some nGVS studies may be suprathreshold and are thus unlikely to induce stochastic resonance. In addition, while using a set threshold for all participants is appealing it may not be sensitive enough to provide optimisation of the signal to gain the most favorable result for individual participants.
In keeping with the theory of stochastic resonance, other studies have used various methods to individualize the amplitude to participants. This has been done by testing an array of amplitudes and choosing the amplitude that improves standing sway measures the most (Mulavara et al., 2011; Iwasaki et al., 2014, 2018; Mulavara et al., 2015; Fujimoto et al., 2016, 2018; Asslander et al., 2021; Chen et al., 2021), or by calculating amplitude at a percentage of the cutaneous sensory threshold (Wuehr et al., 2016a,b; Piccolo et al., 2020; Lotfi et al., 2021; Mitsutake et al., 2022) or a percentage of the vestibular (motion) threshold of 1 Hz sinusoidal GVS stimulation, to determine the optimum signal (Goel et al., 2015; Mulavara et al., 2015; Samoudi et al., 2015; Temple et al., 2018; Piccolo et al., 2020; Sprenger et al., 2020).
The underlying pathology also appears to influence the current amplitude that is optimal for individuals. Studies using individualized optimization have had a mean amplitude of 397 +/−167 μA, which is close to the value most commonly used in studies that set the amplitude at a group level. Optimized studies involving healthy subjects used a mean current amplitude of 309 +/− 83 μA whereas the mean amplitude for people with bilateral vestibulopathy (BVP) was 494 +/− 131 μA. A higher mean amplitude for people with an underlying vestibular diagnosis suggests that the integrity of the vestibular system should be considered when planning stimulation of clinical populations, to give the greatest chance of success.
Examining the means of individualizing the amplitude using a wide range of amplitudes and testing the sway parameters of each amplitude to find the optimum amplitude, could be the most specific method of determining optimal amplitude and has been the gold standard against which other methods have been measured (Iwasaki et al., 2014, 2018; Goel et al., 2015; Mulavara et al., 2015). However, it is time consuming and requires a laboratory-based setting to determine the optimum parameters, meaning it cannot be translated easily into the clinical setting. Fujimoto et al. (2018) used this technique in sessions 2 weeks apart. Of 13 participants, only 3 participants had the same optimum amplitude in both sessions, suggesting there are factors involved in the application of the stimulation or neural excitability that affect response to stimulation on a day-to-day basis.
Five studies have used vestibular motion perception to set amplitude. The theory behind this method of optimization is that it tests the excitability and integrity of the vestibular nerve and sets the current amplitude accordingly. A 1 Hz sinusoidal GVS waveform is delivered and the amplitude at which an individual senses mediolateral motion, or is observed moving on a force plate, is taken as the sensory threshold. A percentage of this threshold amplitude (between 50% and 100%) is then applied to a nGVS waveform. This could be done in a clinical setting as the perception of movement has been found to be as sensitive as measuring movement of the center of mass on a forceplate (Goel et al., 2015). However, a disadvantage is that this method requires a machine that can deliver both a GVS and nGVS waveform, and the risk of falls - particularly in those with impaired balance already (BVP and older adults). Studies using a 100% motion perception threshold have resulted in a deterioration in balance (Pavlik et al., 1999) or had no effect on postural control, and led to nausea in people with Parkinson’s disease who were medicated (Samoudi et al., 2015). nGVS at 80% motion sensation perception had no effect when standing on a firm surface with eyes open or closed (Woll et al., 2019; Sprenger et al., 2020) and led to increased sway standing on a foam surface (Sprenger et al., 2020). However, studies using an amplitude of 50% of motion perception were effective at improving postural control (Temple et al., 2018; Piccolo et al., 2020). Mulavara et al. (2015) investigated balance at a range of nGVS amplitudes and compared their results with the motion perception threshold, finding no significant correlation (p > 0.05) between optimal nGVS stimulation and perceptual threshold amplitude. In their study the average peak current amplitude that resulted in improved balance performance was 35% of the motion perception threshold. By contrast, Goel et al. (2015) found the optimal amplitude determined by exposure to multiple current amplitudes to be equivalent to 50% of the motion perception threshold. While conceptually sound as a method that investigates the responsiveness of the vestibular nerve to stimulation and sets the stimulation current amplitude accordingly, results have been inconsistent and further research is needed to establish the adequacy of this method of individualization.
The cutaneous threshold, or the point at which nGVS starts to cause cutaneous sensation under the electrodes, has also been used as a method of optimization (Wuehr et al., 2016a,b; Lotfi et al., 2021; Mitsutake et al., 2022). The advantage of using cutaneous sensory threshold to determine the optimum amplitude is that it can be easily done outside a laboratory setting, it only requires one waveform and there is a low risk of delivering stimulation at a level that may cause unexpected imbalance. At 80% of the cutaneous threshold, studies have demonstrated improvements in gait stability in both older healthy adults and those with BVP. Studies using balance measures at a range of amplitudes to find the optimum that have also tested the sensory threshold, support this finding. Iwasaki et al. (2014, 2018), found the optimum amplitude to be 83 and 85% of the sensory threshold, respectively. While this method has the benefit of providing a quick and easy method of optimization, the relationship between cutaneous sensation and vestibular function has not been established and the theoretical underpinnings of this method require validation.
Amplitudes of nGVS between 100 and 1,200 μA have been used to improve postural control, with the most beneficial amplitudes appearing to sit in the lower end of this range. Individualisation of the stimulus amplitude appears to be important, particularly in participants with impaired vestibular function who may require a stronger stimulus intensity to receive the benefits of nGVS (Iwasaki et al., 2014, 2018; Sprenger et al., 2020). There is no one single method of tuning the signal amplitude to the individual that is obviously superior at this point.
A variety of frequency bands have been used to create the noisy signal, to date there has been insufficient systematic research investigating nGVS frequency bands to determine if there is an optimum frequency band that produces superior postural control (Lee et al., 2021). The solitary review on this topic determined that, a narrower bandwidth nGVS (0-30 Hz), demonstrated significantly greater postural control performance when compared to a wider bandwidth (0.1–640 Hz) (Stefani et al., 2020). Uniformity of terminology and provision of frequency and amplitude characteristics of the stochastic signals used, when reporting on nGVS will aid future inquiry into the effect of the waveforms.
Most studies have looked at the coincident effect of nGVS and its effect on balance while actively stimulating the vestibular system (Table 6). The consensus from these studies is that nGVS can immediately reduce sway in standing (Pal et al., 2009; Mulavara et al., 2011; Iwasaki et al., 2014, 2018; Goel et al., 2015; Samoudi et al., 2015; Fujimoto et al., 2018; Inukai et al., 2018a,b; Nooristani et al., 2019a,b; Inukai et al., 2020a,b; Ko et al., 2020; Piccolo et al., 2020; Asslander et al., 2021) and improve gait parameters (Mulavara et al., 2015; Wuehr et al., 2016a,b; Iwasaki et al., 2018; Temple et al., 2018; Ko et al., 2020; Piccolo et al., 2020; Chen et al., 2021). These findings support the potential for nGVS to be used as an orthotic to support balance.
A limitation in studies to date is the short duration of application - seconds to minutes. Only one study has investigated the effect of long duration nGVS over the stimulation period. Fujimoto et al. (2016), applied nGVS for 3 h and assessed balance at 1 and 2 h during the application. While there was a significant reduction in sway velocity after 1 h of nGVS, after 2 h this change was no longer significant. This is an important area for further investigation to determine whether the ameliorating effects of nGVS continue during prolonged application.
Residual effects on standing balance immediately after nGVS stimulation demonstrate a continued positive effect on standing balance and sway velocity (Fujimoto et al., 2016, 2018; Inukai et al., 2020c; Nooristani et al., 2021). Significant enhancement of balance has been observed 10 min after stimulation (Inukai et al., 2020b,c) and 30-min post-stimulation (Fujimoto et al., 2016, 2018), with improvements continuing to be significant for 3 hours after a 30-min period of stimulation (Fujimoto et al., 2016, 2018).
Two studies have used nGVS over a period of weeks (Lotfi et al., 2021; Eder et al., 2022). While neither of these studies have found a significant change in postural control with nGVS compared to their control groups, both studies contribute to our understanding of how we could make repeated application of nGVS more effective. Lotfi et al. (2021), investigated 30 min nGVS twice a week for 6 weeks in people with Multiple Sclerosis. They compared the treatment to vestibular rehabilitation and a no treatment group. They found vestibular rehabilitation significantly improved balance and there was no significant difference between receiving nGVS and no treatment. However, the subthreshold nGVS treatment was given while individuals lay supine. As the vestibular apparatus is relatively inactive in this stationary well supported position, it is possible that there was little vestibular afferent activity for subthreshold stimulation to augment in this treatment protocol. This pattern has also been seen in an MRI study. Helmchen et al. (2019) found no evidence of a change in cortical activity when participants received nGVS while lying supine with the head immobilized within an MRI machine. This supports the theoretical basis of stochastic resonance as the means behind nGVS, as without head movement there is little vestibular afferent signal to facilitate.
In comparison, Eder et al. (2022) investigated whether nGVS applied during vestibular rehabilitation over a 2-week period produced a synergistic effect compared to standard vestibular rehabilitation, in a randomized controlled pilot study. While nGVS augmentation of treatment was feasible, they found no difference between groups. Notably, while there was a reduction in base of support in both groups during closed eye walking, neither group demonstrated a significant change in postural sway measurements standing on foam with eyes closed. It is possible that this test is too challenging for people with bilateral vestibulopathy (Iwasaki et al., 2014; Sprenger et al., 2020; McLaren et al., 2022). Alternatively, this may indicate a difference in the way the CNS processes vestibular information in standing and walking or a targeted effect of vestibular rehabilitation toward mobility.
Short duration coincident nGVS appears to improve postural control in standing (Mulavara et al., 2011; Goel et al., 2015; Inukai et al., 2018a,b; Iwasaki et al., 2018; Inukai et al., 2020a,b,c; Asslander et al., 2021) and walking (Mulavara et al., 2015; Wuehr et al., 2016a,b; Temple et al., 2018; Piccolo et al., 2020; Chen et al., 2021), with significant effects lasting for hours after stimulation (Fujimoto et al., 2016, 2018). Intermittent nGVS over a period of weeks appears to be a safe treatment that is well tolerated (Lotfi et al., 2021; Eder et al., 2022). The efficacy of longer periods of nGVS is yet to be established.
This scoping review was limited to studies that have used a postural control measure that will be more responsive to the vestibular influence on the vestibulospinal reflexes (VSRs) and via the vestibulospinal tract. However, the vestibular system has a direct impact on postural control via both the VSRs and the vestibulo-ocular reflexes (VORs). While some studies have found the greatest benefit of nGVS was demonstrated with the eyes closed, eliminating the influence of the VOR on balance (McLaren et al., 2022), and others have found no effect of the visual condition (Matsugi et al., 2020), in most real-life situations the stabilizing role of the VOR for vision plays a critical role in balance and postural control. However, to date we do not have evidence that a change in postural control with nGVS is associated with a the change in the VOR (Matsugi et al., 2022).
Examining the effect of nGVS on vestibulo-ocular control can help us understand how nGVS influences the vestibular apparatus. As with the effect of nGVS on postural control, the effects on the VOR have also been contradictory. Some studies investigating the effects on otolith-ocular responses have demonstrated positive results. Iwasaki et al. (2017) found that nGVS significantly increased the amplitude of the ocular vestibular evoked myogenic potential (oVEMP) responses in 79% of ears. Serrador et al. (2018) found that imperceptible nGVS resulted in a significant increase in ocular counter roll gain in older adults, and Keywan et al. (2018) demonstrated a reduced vestibular perceptual threshold in the roll plane, and translational movement (Keywan et al., 2019). These studies indicate more sensitive vestibular function during active nGVS (Keywan et al., 2020), and support the theory that nGVS preferentially stimulates the irregularly firing afferents originating at the otolith organs (Keywan et al., 2019).
In contrast Matsugi et al. (2022) found that nGVS significantly reduced rather than increased the video head impulse test (vHIT) gain to horizontal head impulses, indicating suppression of the VOR with nGVS. The reasons for these contradictory results are not clear. As the frequency band of the nGVS stimulus in Matsugi et al. (2022) was not disclosed, and they used set amplitudes, there could be parameter based differences. Also, in both Matsugi et al. (2022) and Serrador et al. (2018), young healthy subjects demonstrated no change in ocular counter roll response to nGVS (Serrador et al., 2018) or vHIT (Matsugi et al., 2022). The young and healthy may demonstrate a ceiling effect and have little capacity for improvements in oculomotor control. This could also be an indication that the physiological response to nGVS is specific to the particular parts of the vestibular anatomy. The oVEMP and ocular counter roll following the initial head movement—both of which have shown a positive response to nGVS—primarily test the function of the utricle, whereas the horizontal vHIT is a test of the horizontal semicircular canals. Research looking at the effect of nGVS on ocular control provides helpful insight into the theoretical basis for this modality but needs context to be understood in relation to the influence on postural control. Future work looking at the role of nGVS-facilitated gaze stabilization and the influence this has on balance will help clarify the role of the vestibular system in postural and oculomotor control, and the physiological effects of nGVS on different anatomical sites of the vestibular system.
Task specificity during tuning of the nGVS signal has not been clearly addressed in the literature (Herssens and McCrum, 2019). To date, the optimization of nGVS for postural control has primarily been done in standing (Iwasaki et al., 2014; Goel et al., 2015; Mulavara et al., 2015; Chen et al., 2021) with only one study using gait velocity to optimize the nGVS amplitude (Iwasaki et al., 2018). In two comparable studies, Iwasaki et al. (2014, 2018) used the same 0.02–10 Hz frequency band tested across multiple amplitudes to optimize the stimulus amplitude. Iwasaki et al. (2014) used standing sway to determine the optimal amplitude and found that the mean optimum amplitude was 281 +/−40 μA in a healthy population and 456 +/− 82 μA in people with BVP. In a subsequent study Iwasaki et al. (2018) used the amplitude that improved gait velocity the most, to determine the optimum amplitude and found the mean optimum amplitude was much higher, 341 +/− 46.6 μA in a healthy population and 725 +/− 79.9 μA in people with BVP. This comparison suggests that the task may influence the choice of parameters, a gap in the literature that will be important to address as we move toward clinical trials of nGVS.
It is becoming evident that nGVS is unlikely to be a treatment suitable for improving postural control in every individual. Inukai et al. (2018a, 2020a), concluded that subjects who have a longer sway path at baseline appear to show a larger stimulation effect and Nooristani et al. (2021) found that nGVS induced significantly greater improvement in sway velocity in older adults with vestibular impairment (who would be assumed to have poorer balance), compared to older adults with normal vestibular function. Supporting this theory, in studies of healthy people, Fujimoto et al. (2019), Temple et al. (2018), and Goel et al. (2015) found only approximately 2/3 of healthy adults demonstrated an optimal response to nGVS. However, 45/46 participants with bilateral vestibulopathy demonstrated an improvement in stability when nGVS was applied (Iwasaki et al., 2014, 2018; Wuehr et al., 2016a,b; Fujimoto et al., 2018; Ko et al., 2020, Chen et al., 2021). This is hypothesized to be due to a ceiling effect, whereby nGVS is more effective in those with sub-optimal vestibular input such as vestibular disorders or older adults who are more likely to have age related presbyvestibulopathy (Inukai et al., 2018a, Nooristani et al., 2021). Or alternatively, non-responders may have an inherently low weighting for vestibular sensory contributions (Goel et al., 2015). Conversely, Schniepp et al. (2018) found that nGVS lowered the VSR threshold in individuals with vestibulopathy with residual function but no response to nGVS in those with complete loss of vestibular function bilaterally, indicating that some residual vestibular function is required for a response to nGVS. These findings highlight the importance of targeting nGVS to the populations that are most likely to benefit from this intervention, particularly those with poor balance or impaired vestibular function but without complete vestibular loss.
Overall, nGVS appears promising as a technology to facilitate vestibular function and improve gait and balance in people with balance deficits (Lajoie et al., 2021). However, a limitation of research to date is not only the vast array of different parameters that have been used, and a lack of consensus on optimum stimulus parameters, but also the complexities of the vestibular contribution to stability. CNS weighting of afferent inputs in the balance response, individual variances in responsiveness and the effects of the task all influence the benefits gained from nGVS (Herssens and McCrum, 2019).
As a promising adjunct to balance rehabilitation, the progress of nGVS as a therapeutic tool is limited while the optimal parameters are not understood. There is currently insufficient evidence to develop a clinical guideline (AGREE Next Steps Consortium, 2017). However, looking to the future, researchers can bring us closer to that position by ensuring complete and transparent reporting of the parameters likely to influence the stimulation effect (Table 7) as we work toward developing consensus on optimum stimulus parameters.
While nGVS parameters appear important, the ability to draw robust conclusions about the selection of optimal parameters is hindered by limited systematic evaluation of parameter settings within and across experiments; and variability in individuals’ response to nGVS. Understanding the optimum parameters to stimulate balance and gait responses will enable us to investigate the use of nGVS as a clinical tool to augment balance rehabilitation. However, before we can use nGVS as a therapeutic tool we need to sort out the science that will influence its clinical application. In response to the lack of research into the optimum parameters of nGVS, we propose a guideline (Table 7) for the accurate reporting of nGVS parameters, as a first step toward establishing standardized stimulation protocols.
RM and DT conceptualized the study. RM performed the literature search. RM and DT independently reviewed the articles. RM extracted and critically reviewed the data and wrote the first draft of the manuscript. IN provided his expertise and critical feedback in the signal processing elements of the paper. PS, RT, and DT provided feedback at all stages of manuscript development. All authors contributed to the article and approved the submitted version.
This work was supported by the Health Research Council of New Zealand grant numbers, HRC22/363 and HRC19/632.
Our thanks to Usman Ghani (New Zealand College of Chiropractic) for providing the data for Figures 3, 4.
The authors declare that the research was conducted in the absence of any commercial or financial relationships that could be construed as a potential conflict of interest.
All claims expressed in this article are solely those of the authors and do not necessarily represent those of their affiliated organizations, or those of the publisher, the editors and the reviewers. Any product that may be evaluated in this article, or claim that may be made by its manufacturer, is not guaranteed or endorsed by the publisher.
Abe, Y., and Nishizawa, M. (2021). Electrical aspects of skin as a pathway to engineering skin devices. APL Bioeng. 5:041509. doi: 10.1063/5.0064529
Agrawal, Y., Merfeld, D. M., Horak, F. B., Redfern, M. S., Manor, B., Westlake, K. P., et al. (2020b). Aging, vestibular function, and balance: proceedings of a National Institute on Aging/National Institute on Deafness and Other Communication Disorders Workshop. J. Gerontol. A Biol. Sci. Med. Sci. 75, 2471–2480. doi: 10.1093/Gerona/Glaa097
Agrawal, Y., and Smith, P.F., And Merfeld, D. (2020a). "Dizziness, imbalance and age-related vestibular loss, " In The senses: a comprehensive reference ., Eds. B. Fritzch and H. Straka. (Cambridge, MA: Elsevier, Academic Press).
Agree Next Steps Consortium. (2017). The Agree Ii Instrument [Electronic Version]. Available at: http://www.Agreetrust.org (Accessed 28 Aug 2022).
Arksey, H., and O'Malley, L. (2005). Scoping studies: towards a methodological framework. Int. J. Soc. Res. Methodol. 8, 19–32. doi: 10.1080/1364557032000119616
Asslander, L., Giboin, L.S., Gruber, M., and Schniepp, R., And Wuehr, M. (2021). No evidence for stochastic resonance effects on standing balance when applying Noisy galvanic vestibular stimulation in young healthy adults. Sci. Rep. 11,:12327. doi: 10.1038/S41598-021-91808-W
Chen, P. Y., Jheng, Y. C., Wang, C. C., Huang, S. E., Yang, T. H., Hsu, P. C., et al. (2021). Effect of Noisy galvanic vestibular stimulation on dynamic posture sway under visual deprivation in patients with bilateral vestibular hypofunction. Sci. Rep. 11:4229. doi: 10.1038/S41598-021-83206-Z
Collins, J., and Chow, C., And Imhoff, T. (1995). Stochastic resonance without tuning. Nature 376, 236–238, doi: 10.1038/376236a0
Dilda, V., Macdougall, H.G., and Curthoys, I.S., And Moore, S.T. (2012). Effects of galvanic vestibular stimulation on cognitive function. Exp. Brain Res. 216, 275–285. doi: 10.1007/S00221-011-2929-Z
Dlugaiczyk, J., and Gensberger, K.D., And Straka, H. (2019). Galvanic vestibular stimulation: from basic concepts to clinical applications. J. Neurophysiol. 121, 2237–2255. doi: 10.1152/Jn.00035.2019
Eder, J., Kellerer, S., Amberger, T., Keywan, A., Dlugaiczyk, J., Wuehr, M., et al. (2022). Combining vestibular rehabilitation with Noisy galvanic vestibular stimulation for treatment of bilateral Vestibulopathy. J. Neurol. 269, 5731–5737. doi: 10.1007/S00415-022-11033-X
Fujimoto, C., Egami, N., Kawahara, T., Uemura, Y., Yamamoto, Y., Yamasoba, T., et al. (2018). Noisy galvanic vestibular stimulation sustainably improves posture in bilateral Vestibulopathy. Front. Neurol. 9:900. doi: 10.3389/Fneur.2018.00900
Fujimoto, C., and Yagi, M., And Murofushi, T. (2019). Recent advances in idiopathic bilateral Vestibulopathy: a literature review. Orphanet J. Rare Dis. 14,:202. doi: 10.1186/S13023-019-1180-8
Fujimoto, C., Yamamoto, Y., Kamogashira, T., Kinoshita, M., Egami, N., Uemura, Y., et al. (2016). Noisy galvanic vestibular stimulation induces a sustained improvement in body balance in elderly adults. Sci. Rep. 6:37575. doi: 10.1038/Srep37575
Goel, R., Kofman, I., Jeevarajan, J., De Dios, Y., Cohen, H. S., Bloomberg, J. J., et al. (2015). Using low levels of stochastic vestibular stimulation to improve balance function. PLoS One 10:E0136335. doi: 10.1371/Journal.Pone.0136335
Hanggi, P., Jung, P., and Zerbe, C., And Moss, F. (1993). Can colored noise improve stochastic resonance? J. Stat. Phys. 70, 25–47, doi: 10.1007/BF01053952
Haxby, F., and Akrami, M., And Zamani, R. (2020). Finding a balance: a systematic review of the biomechanical effects of vestibular prostheses on stability in humans. J Funct Morphol Kinesiol 5,:23. doi: 10.3390/Jfmk5020023
Helmchen, C., Rother, M., and Spliethoff, P., And Sprenger, A. (2019). Increased brain responsivity to galvanic vestibular stimulation in bilateral vestibular failure. Neuroimage Clin 24,:101942. doi: 10.1016/J.Nicl.2019.101942
Herssens, N., And Mccrum, C. (2019). Stimulating balance: recent advances in vestibular stimulation for balance and gait. J. Neurophysiol. 122, 447–450. doi: 10.1152/Jn.00851.2018
Hirasaki, E., Moore, S.T., and Raphan, T., And Cohen, B. (1999). Effects of walking velocity on vertical head and body movements during locomotion. Exp. Brain Res. 127, 117–130, doi: 10.1007/s002210050781
Inukai, Y., Masaki, M., Otsuru, N., Saito, K., Miyaguchi, S., Kojima, S., et al. (2018a). Effect of Noisy galvanic vestibular stimulation in community-dwelling elderly people: a randomised controlled trial. J. Neuroeng. Rehabil. 15:63. doi: 10.1186/S12984-018-0407-6
Inukai, Y., Miyaguchi, S., Kobayashi, N., and Otsuru, N., And Onishi, H. (2020a). Noisy galvanic vestibular stimulation effect on center of pressure sway during one-legged standing. J. Clin. Neurosci. 82, 173–178. doi: 10.1016/J.Jocn.2020.10.050
Inukai, Y., Miyaguchi, S., Saito, M., and Otsuru, N., And Onishi, H. (2020b). Effects of different stimulation conditions on the stimulation effect of Noisy galvanic vestibular stimulation. Front. Hum. Neurosci. 14,:581405. doi: 10.3389/Fnhum.2020.581405
Inukai, Y., Otsuru, N., Masaki, M., Saito, K., Miyaguchi, S., Kojima, S., et al. (2018b). Effect of Noisy galvanic vestibular stimulation on center of pressure sway of static standing posture. Brain Stimul. 11, 85–93. doi: 10.1016/J.Brs.2017.10.007
Inukai, Y., Otsuru, N., Saito, K., Miyaguchi, S., Kojima, S., Yokota, H., et al. (2020c). The after-effect of Noisy galvanic vestibular stimulation on postural control in young people: a randomized controlled trial. Neurosci. Lett. 729:135009. doi: 10.1016/J.Neulet.2020.135009
Iwasaki, S., Fujimoto, C., Egami, N., Kinoshita, M., Togo, F., Yamamoto, Y., et al. (2018). Noisy vestibular stimulation increases gait speed in Normals and in bilateral Vestibulopathy. Brain Stimul. 11, 709–715. doi: 10.1016/J.Brs.2018.03.005
Iwasaki, S., Karino, T., Kamogashira, F., Togo, C., Fujimoto, Y., Yamamoto, Y., et al. (2017). Effect of Noisy galvanic vestibular stimulation on ocular vestibular-evoked myogenic potentials to bone-conducted vibration. Front. Neurol. 8:26. doi: 10.3389/Fneur.2017.00026
Iwasaki, S., Yamamoto, Y., Togo, F., Kinoshita, M., Yoshifuji, Y., Fujimoto, C., et al. (2014). Noisy vestibular stimulation improves body balance in bilateral Vestibulopathy. Neurology 82, 969–975. doi: 10.1212/Wnl.0000000000000215
Keywan, A., Badarna, H., and Jahn, K., And Wuehr, M. (2020). No evidence for after-effects of Noisy galvanic vestibular stimulation on motion perception. Sci. Rep. 10,:2545. doi: 10.1038/S41598-020-59374-9
Keywan, A., and Jahn, K., And Wuehr, M. (2019). Noisy galvanic vestibular stimulation primarily affects otolith- mediated motion perception Neuroscience 399, 161–166. doi: 10.1016/J.Neuroscience.2018.12.031
Keywan, A., Wuehr, M., and Pradhan, C., And Jahn, K. (2018). Noisy galvanic stimulation improves roll-tilt vestibular perception in healthy subjects. Front. Neurol. 9,:83. d: doi: 10.3389/Fneur.2018.00083
Ko, L.W., Chikara, R.K., Chen, P.Y., Jheng, Y.C., Wang, C.C., Yang, Y.C., Li, L.P.H., Liao, K.K., Chou, L.W., and Kao, C.L. (2020). Noisy galvanic vestibular stimulation (stochastic resonance) changes electroencephalography activities and postural control in patients with bilateral vestibular hypofunction. Brain Sci. 10,:740. d: doi: 10.3390/Brainsci10100740
Kuatsjah, E., and Khoshnam, M., And Menon, C. (2019). Investigation on the effect of Noisy galvanic vestibular stimulation on fine motor skills during a Visuomotor task in healthy participants. PLoS One 14,:E0216214. doi: 10.1371/Journal.Pone.0216214
Kwan, A., Forbes, P.A., Mitchell, D.E., and Blouin, J.S., And Cullen, K.E. (2019). Neural substrates, dynamics and thresholds of galvanic vestibular stimulation in the behaving primate. Nat. Commun. 10,:1904. doi: 10.1038/S41467-019-09738-1
Lajoie, K., Marigold, D.S., and Valdes, B.A., And Menon, C. (2021). The potential of Noisy galvanic vestibular stimulation for optimizing and assisting human performance. Neuropsychologia 152:107751. doi: 10.1016/J.Neuropsychologia.2021.107751
Lee, S., Smith, P.F., and Lee, W.H., And Mckeown, M.J. (2021). Frequency-specific effects of galvanic vestibular stimulation on response-time performance in Parkinson's disease. Front. Neurol. 12,:758122. doi: 10.3389/Fneur.2021.758122
Lotfi, Y., Farahani, A., Azimiyan, M., and Moossavi, A., And Bakhshi, E. (2021). Comparison of efficacy of vestibular rehabilitation and Noisy galvanic vestibular stimulation to improve dizziness and balance in patients with multiple sclerosis. J. Vestib. Res. 31, 541–551. doi: 10.3233/Ves-201609
Mahmud, M., Hadi, Z., Prendergast, M., Ciocca, M., Saad, A. R., Pondeca, Y., et al. (2022). The effect of galvanic vestibular stimulation on postural balance in Parkinson's disease: a systematic review and meta-analysis. J. Neurol. Sci. 442:120414. doi: 10.1016/J.Jns.2022.120414
Marmarelis, V. (2012). “Appendix ii: Gaussian white noise” in Nonlinear dynamic modeling of physiological systems (Hoboken, New Jersey: Institute of Electrical and Electronics Engineers), 499–501.
Matsugi, A., Nagino, K., Shiozaki, T., Okada, Y., Mori, N., Nakamura, J., et al. (2021). No impact of stochastic galvanic vestibular stimulation on arterial pressure and heart rate variability in the elderly population. Front. Hum. Neurosci. 15:646127. doi: 10.3389/Fnhum.2021.646127
Matsugi, A., and Oku, K., And Mori, N. (2020). The effects of stochastic galvanic vestibular stimulation on body sway and muscle activity. Front. Hum. Neurosci. 14,:591671. doi: 10.3389/Fnhum.2020.591671
Matsugi, A., and Shiozaki, T., And Tanaka, H. (2022). Vestibulo-ocular reflex is modulated by Noisy galvanic vestibular stimulation. Front. Neurol. 13,:826739. doi: 10.3389/Fneur.2022.826739
Mcadams, E.T., Jossinet, J., and Lackermeier, A., And Risacher, F. (1996). Factors affecting electrode-gel-skin Interface impedance in electrical impedance tomography. Med. Biol. Eng. Comput. 34, 397–408, doi: 10.1007/BF02523842
Mclaren, R., Joseph, F., and Baguley, C., And Taylor, D. (2016). A review of E-textiles in neurological rehabilitation: how close are we? J. Neuroeng. Rehabil. 13,:59. doi: 10.1186/S12984-016-0167-0
Mclaren, R., Smith, P.F., Taylor, R.L., Ravindran, S., and Rashid, U., And Taylor, D. (2022). Efficacy of Ngvs to improve postural stability in people with bilateral Vestibulopathy: a systematic review and meta-analysis. Front. Neurosci. doi: 10.3389/Fnins.2022.1010239, 16,:1010239.
Mitsutake, T., Taniguchi, T., Nakazono, H., and Yoshizuka, H., And Sakamoto, M. (2022). Effects of Noisy galvanic vestibular stimulation on the muscle activity and joint movements in different standing postures conditions. Front. Hum. Neurosci. 16,:891669. doi: 10.3389/Fnhum.2022.891669
Moss, F., and Ward, L.M., And Sannita, W.G. (2004). Stochastic resonance and sensory information processing: a tutorial and review of application. Clin. Neurophysiol. 115, 267–281. doi: 10.1016/J.Clinph.2003.09.014
Mulavara, A. P., Fiedler, M. J., Kofman, I. S., Wood, S. J., Serrador, J. M., Peters, B., et al. (2011). Improving balance function using vestibular stochastic resonance: optimizing stimulus characteristics. Exp. Brain Res. 210, 303–312. doi: 10.1007/S00221-011-2633-Z
Mulavara, A. P., Kofman, I. S., De Dios, Y. E., Miller, C., Peters, B. T., Goel, R., et al. (2015). Using low levels of stochastic vestibular stimulation to improve locomotor stability. Front. Syst. Neurosci. 9:117. doi: 10.3389/Fnsys.2015.00117
Nooristani, M., Bigras, C., Lafontaine, L., Bacon, B.A., and Maheu, M., And Champoux, F. (2021). Vestibular function modulates the impact of Ngvs on postural control in older adults. J. Neurophysiol. 125, 489–495. doi: 10.1152/Jn.00512.2020
Nooristani, M., Maheu, M., and Bacon, B.A., And Champoux, F. (2019a). The importance of Ngvs current density for postural control enhancement. Brain Stimul. 12, 1592–1594. doi: 10.1016/J.Brs.2019.07.022
Nooristani, M., Maheu, M., Houde, M.S., and Bacon, B.A., And Champoux, F. (2019b). Questioning the lasting effect of galvanic vestibular stimulation on postural control. PLoS One 14,:E0224619. doi: 10.1371/Journal.Pone.0224619
Pal, S., and Rosengren, S.M., And Colebatch, J.G. (2009). Stochastic galvanic vestibular stimulation produces a small reduction in sway in Parkinson's disease. J. Vestib. Res. 19, 137–142. doi: 10.3233/Ves-2009-0360
Pavlik, A.E., Inglis, J.T., Lauk, M., and Oddsson, L., And Collins, J. (1999). The effects of stochastic galvanic vestibular stimulation on human postural sway. Exp. Brain Res. 124, 273–280, doi: 10.1007/s002210050623
Piccolo, C., and Bakkum, A., And Marigold, D.S. (2020). Subthreshold stochastic vestibular stimulation affects balance-challenged standing and walking. PLoS One 15,:E0231334. doi: 10.1371/Journal.Pone.0231334
Pires, A.P.B.D.Á., Silva, T.R., Torres, M.S., Diniz, M.L., and Tavares, M.C., And Gonçalves, D.U. (2022). Galvanic vestibular stimulation and its applications: a systematic review. Braz. J. Otorhinolaryngol. doi: 10.1016/J.Bjorl.2022.05.010, 88, S202–S211
Rieke, F. (1997). “Spikes: exploring the neural code” in Computational Neuroscience, eds. B. Bialek, R. de Ruyter van Steveninck, F. Rieke and R. Warland (Cambridge, MA: MIT Press).
Samoudi, G., Jivegard, M., and Mulavara, A.P., And Bergquist, F. (2015). Effects of stochastic vestibular galvanic stimulation and Ldopa on balance and motor symptoms in patients with Parkinson's disease. Brain Stimul. 8, 474–480. doi: 10.1016/J.Brs.2014.11.019
Schniepp, R., Boerner, J.C., Decker, J., Jahn, K., and Brandt, T., And Wuehr, M. (2018). Noisy vestibular stimulation improves Vestibulospinal function in patients with bilateral Vestibulopathy. J. Neurol. 265, 57–62. doi: 10.1007/S00415-018-8814-Y
Serrador, J.M., Deegan, B.M., and Geraghty, M.C., And Wood, S.J. (2018). Enhancing vestibular function in the elderly with imperceptible electrical stimulation. Sci. Rep. 8,:336. doi: 10.1038/S41598-017-18653-8
Soma, R., Nozaki, D., and Kwak, S., And Yamamoto, Y. (2003). 1/F noise outperforms white noise in sensitizing Baroreflex function in the human brain. Phys. Rev. Lett. 91,:078101. doi: 10.1103/Physrevlett.91.078101
Sprenger, A., Spliethoff, P., Rother, M., and Machner, B., And Helmchen, C. (2020). Effects of perceptible and imperceptible galvanic vestibular stimulation on the postural control of patients with bilateral Vestibulopathy. J. Neurol. 267, 2383–2397. doi: 10.1007/S00415-020-09852-X
Sprenger, A., Wojak, J.F., and Jandl, N.M., And Helmchen, C. (2017). Postural control in bilateral vestibular failure: its relation to visual, proprioceptive, vestibular, and cognitive input, Front. Neurol. 8:444. doi: 10.3389/Fneur.2017.00444
Stacey, W.C., And Durand, D.M. (2000). Stochastic resonance improves signal detection in hippocampal Ca1 neurons. J. Neurophysiol. 83, 1394–1402. doi: 10.1152/Jn.2000.83.3.1394
Stefani, S.P., Serrador, J.M., and Breen, P.P., And Camp, A.J. (2020). Impact of galvanic vestibular stimulation-induced stochastic resonance on the output of the vestibular system: a systematic review. Brain Stimul. 13, 533–535. doi: 10.1016/J.Brs.2020.01.006
Temple, D.R., De Dios, Y.E., Layne, C.S., and Bloomberg, J.J., And Mulavara, A.P. (2018). Efficacy of stochastic vestibular stimulation to improve locomotor performance during adaptation to Visuomotor and somatosensory distortion. Front. Physiol. 9,:301. doi: 10.3389/Fphys.2018.00301
Terney, D., Chaieb, L., Moliadze, V., and Antal, A., And Paulus, W. (2008). Increasing human brain excitability by transcranial high-frequency random noise stimulation. J. Neurosci. 28, 14147–14155. doi: 10.1523/Jneurosci.4248-08.2008
Truong, D.Q., Guillen, A., Nooristani, M., Maheu, M., and Champoux, F., And Datta, A. (2023). Impact of galvanic vestibular stimulation electrode current density on brain current flow patterns: does electrode size matter? PLoS One 18,:E0273883. doi: 10.1371/Journal.Pone.0273883
Utz, K. S., Korluss, K., Schmidt, L., Rosenthal, A., Oppenlander, K., Keller, I., et al. (2011). Minor adverse effects of galvanic vestibular stimulation in persons with stroke and healthy individuals. Brain Inj. 25, 1058–1069. doi: 10.3109/02699052.2011.607789
Wilkinson, D., and Zubko, O., And Sakel, M. (2009). Safety of repeated sessions of galvanic vestibular stimulation following stroke: a single-case study. Brain Inj. 23, 841–845. doi: 10.1080/02699050903232541
Woll, J., and Sprenger, A., And Helmchen, C. (2019). Postural control during galvanic vestibular stimulation in patients with persistent perceptual-postural dizziness. J. Neurol. 266, 1236–1249. doi: 10.1007/S00415-019-09255-7
Wuehr, M., Boerner, J. C., Pradhan, C., Decker, J., Jahn, K., Brandt, T., et al. (2018). Stochastic resonance in the human vestibular system - noise-induced facilitation of Vestibulospinal reflexes. Brain Stimul. 11, 261–263. doi: 10.1016/J.Brs.2017.10.016
Wuehr, M., and Decker, J., And Schniepp, R. (2017). Noisy galvanic vestibular stimulation: an emerging treatment option for bilateral Vestibulopathy. J. Neurol. 264, 81–86. doi: 10.1007/S00415-017-8481-4
Wuehr, M., Nusser, E., Decker, J., Krafczyk, S., Straube, A., Brandt, T., et al. (2016a). Noisy vestibular stimulation improves dynamic walking stability in bilateral Vestibulopathy. Neurology 86, 2196–2202. doi: 10.1212/WNL.0000000000002748
Wuehr, M., Nusser, E., Krafczyk, S., Straube, A., Brandt, T., Jahn, K., et al. (2016b). Noise-enhanced vestibular input improves dynamic walking stability in healthy subjects. Brain Stimul. 9, 109–116. doi: 10.1016/J.Brs.2015.08.017
Wuehr, M., Schmidmeier, F., Katzdobler, S., Fietzek, U. M., Levin, J., and Zwergal, A. (2022). Effects of low-intensity vestibular noise stimulation on postural instability in patients with Parkinson's disease. J. Parkinsons Dis. 12, 1611–1618. doi: 10.3233/Jpd-213127
Keywords: neuromodulation, nGVS, posture, galvanic vestibular stimulation, balance, stochastic resonance, vestibular, parameters
Citation: McLaren R, Smith PF, Taylor RL, Niazi IK and Taylor D (2023) Scoping out noisy galvanic vestibular stimulation: a review of the parameters used to improve postural control. Front. Neurosci. 17:1156796. doi: 10.3389/fnins.2023.1156796
Received: 03 February 2023; Accepted: 04 April 2023;
Published: 02 May 2023.
Edited by:
Julia Dlugaiczyk, University Hospital Zürich, SwitzerlandReviewed by:
Max Wuehr, Ludwig Maximilian University of Munich, GermanyCopyright © 2023 McLaren, Smith, Taylor, Niazi and Taylor. This is an open-access article distributed under the terms of the Creative Commons Attribution License (CC BY). The use, distribution or reproduction in other forums is permitted, provided the original author(s) and the copyright owner(s) are credited and that the original publication in this journal is cited, in accordance with accepted academic practice. No use, distribution or reproduction is permitted which does not comply with these terms.
*Correspondence: Ruth McLaren, cnV0aC5tY2xhcmVuQGF1dC5hYy5ueg==
Disclaimer: All claims expressed in this article are solely those of the authors and do not necessarily represent those of their affiliated organizations, or those of the publisher, the editors and the reviewers. Any product that may be evaluated in this article or claim that may be made by its manufacturer is not guaranteed or endorsed by the publisher.
Research integrity at Frontiers
Learn more about the work of our research integrity team to safeguard the quality of each article we publish.