- 1College of Medicine, Chungnam National University, Daejeon, Republic of Korea
- 2Major of Biomedical Engineering, Department of Electrical, Electronic, and Computer Engineering, University of Ulsan, Ulsan, Republic of Korea
- 3Department of Physical and Rehabilitation Medicine, Chungnam National University Hospital, College of Medicine, Chungnam National University, Daejeon, Republic of Korea
Currently, neurointervention, surgery, medication, and central nervous system (CNS) stimulation are the main treatments used in CNS diseases. These approaches are used to overcome the blood brain barrier (BBB), but they have limitations that necessitate the development of targeted delivery methods. Thus, recent research has focused on spatiotemporally direct and indirect targeted delivery methods because they decrease the effect on nontarget cells, thus minimizing side effects and increasing the patient’s quality of life. Methods that enable therapeutics to be directly passed through the BBB to facilitate delivery to target cells include the use of nanomedicine (nanoparticles and extracellular vesicles), and magnetic field-mediated delivery. Nanoparticles are divided into organic, inorganic types depending on their outer shell composition. Extracellular vesicles consist of apoptotic bodies, microvesicles, and exosomes. Magnetic field-mediated delivery methods include magnetic field-mediated passive/actively-assisted navigation, magnetotactic bacteria, magnetic resonance navigation, and magnetic nanobots—in developmental chronological order of when they were developed. Indirect methods increase the BBB permeability, allowing therapeutics to reach the CNS, and include chemical delivery and mechanical delivery (focused ultrasound and LASER therapy). Chemical methods (chemical permeation enhancers) include mannitol, a prevalent BBB permeabilizer, and other chemicals—bradykinin and 1-O-pentylglycerol—to resolve the limitations of mannitol. Focused ultrasound is in either high intensity or low intensity. LASER therapies includes three types: laser interstitial therapy, photodynamic therapy, and photobiomodulation therapy. The combination of direct and indirect methods is not as common as their individual use but represents an area for further research in the field. This review aims to analyze the advantages and disadvantages of these methods, describe the combined use of direct and indirect deliveries, and provide the future prospects of each targeted delivery method. We conclude that the most promising method is the nose-to-CNS delivery of hybrid nanomedicine, multiple combination of organic, inorganic nanoparticles and exosomes, via magnetic resonance navigation following preconditioning treatment with photobiomodulation therapy or focused ultrasound in low intensity as a strategy for differentiating this review from others on targeted CNS delivery; however, additional studies are needed to demonstrate the application of this approach in more complex in vivo pathways.
1. Introduction
The main central nervous system (CNS) treatments used today, including neurointervention, surgery, medication, and CNS stimulation (Daroff et al., 2016), are often invasive, extending the patient’s recovery and rehabilitation time. While the injection of neurotropic drugs such as chondroitinase (Kasinathan et al., 2016), anti-nogo (Zörner and Schwab, 2010), and Rho antagonists (Bertrand et al., 2005) has been considered a replacement for these other treatments in in vitro/in vivo CNS injury models, this method affects not only diseased tissues but also nonrelated healthy tissues (Perez-Herrero and Fernandez-Medarde, 2015). Therefore, CNS therapeutics have recently focused on targeted spatiotemporal therapy to rapidly induce the accumulation of high-concentration therapeutics in damaged areas, thereby making the treatment more intelligent, safer, more effective, and more efficient than the aforementioned methods.
To achieve targeted delivery into the CNS, it is essential for therapeutic agents to pass directly through the blood brain barrier (BBB; Chacko et al., 2013). The BBB is a semipermeable layer of endothelial cells between the circulatory system and the CNS. While the BBB functions to protect the CNS from toxic substances and regulate ions, molecules, and cells for proper neuronal function (Daneman and Prat, 2015), its high selectivity, in which enzymatic and physical barriers are involved, also prevents therapeutics from reaching the CNS (Tewabe et al., 2021). Therefore, direct delivery methods such as nanoparticles (NPs; Dong, 2018; Tewabe et al., 2021), extracellular vesicles (EVs), and magnetic-field mediated delivery (Yu et al., 2018), and indirect delivery methods such as chemical and physical delivery methods are being developed to pass through the BBB and directly affect the CNS: direct delivery methods that allow therapeutics to pass through the BBB as a vehicle or a navigator versus indirect delivery methods that increase the permeability of the BBB as a permeabilizer (Figure 1).
While the direct and indirect methods have been mainly used separately, the authors of this review seek to shed light upon cases where the two methods are used together to create a synergistic effect and introduce the limitations and/or remaining unknowns in the field in regard to their combination. The direct and indirect CNS-targeted delivery strategies introduced in this article have demonstrated promise in in vitro and in animal studies as the next-generation platform for the treatment of CNS diseases ranging from Alzheimer’s disease to brain tumors. Therefore, this review aims to describe various direct and indirect CNS-targeted delivery methods and how they relate to one another to stimulate more interest and research in CNS-targeted delivery by defining the mechanism of each delivery method, discussing their advantages and disadvantages, and analyzing future perspectives for neuroscientists who may aim to understand or even use some of the ideas discussed here in the context of their own work. In addition, although numerous types of therapeutic agent administration, such as subcutaneous, intramuscular, intravascular, transintestinal, transperitoneal, and intrathecal ones, have been tried, all of these routes are currently being evaluated in pioneering studies and thus demand further investigation prior to discussion about whether these approaches have any validity on the CNS-targeted delivery of therapeutics. Just one type of administration, nose-to-CNS route, has been discovered as a promising tool in animal and human models, especially for neurological disease treatment (Mittal et al., 2014). Hence, it should be in mind that the current review is full of examples depicting nose-to-CNS delivery, using viral vectors for delivery, etc.
2. Direct targeted delivery
In direct CNS-targeted delivery, therapeutics may be preserved by methods such as encapsulation to permeabilize through the BBB safely, arrive in the CNS, and take effect. Among variety of direct methods in progress, nanomedicine and magnetic field-mediated deliveries are the most commonly used methods in a field of CNS disease treatment. Nanomedicine allows sustainable selective delivery to the target tissue (Dong, 2018; Tewabe et al., 2021), and magnetic field-mediated deliveries incorporate an external or internal magnetic field to directly navigate and accumulate magnetic therapeutics to the targeted area, resulting in a higher controllability than nanomedicine (Yu et al., 2018). Both nanomedicine and magnetic field-mediated deliveries could be seen as effective, promising delivery platforms for CNS diseases ranging from acute stroke to neurodegenerative disease to brain tumors, which have been most affected by the advancements made in direct delivery methods (Tiebosch et al., 2012; Tietze et al., 2013; Tentillier et al., 2016).
2.1. Nanomedicine
There are three types of namomedicine: NPs, EVs, and hybrid. NP structures, including 1D nanowires, 2D nanosheets, and 3D structures (Huang et al., 2020), such as carbon nanotubes (CNTs), liposomes, micelles, dendrimers, nanocapsules/nanospheres, and polymeric NPs (Perez-Herrero and Fernandez-Medarde, 2015). This review focuses on 3D structures since they allow for easier encapsulation and additional protection of therapeutics. The current review divides NPs into organic and inorganic type. Such classification of NPs focuses on their biocompatibility and stability depending on the consistency of the drug-containing shell. Also, this review introduces EVs, especially exosomes, which are the most up-to-date nanomedicine. Recent studies have focused on hybrids, as they minimize the disadvantages and maximize the advantages of organic, inorganic NPs, and EVs by combining the two or more (Ferreira Soares et al., 2020). Additionally, nanomedicine may be magnetized and incorporated in magnetic field-mediated delivery.
2.2. Organic nanoparticles
Organic NPs consist of organic materials, including lipids and aqueous molecules (Xie et al., 2019), allowing for high biocompatibility. Usually, NPs (liposomes, dendrimers, etc.), are prepared with functional moieties such as polyethylene glycol (PEG). For example, organic NPs made of lipids break down into lactic and glycolic acids after the loaded therapeutics are released into the target area, which can then be used up in the metabolic cycle (Vanden-Hehir et al., 2019). Additionally, organic NPs such as dendrimers, which are polymeric molecules with numerous branch structures enabling their surface to be easily modified, exhibit high bioavailability (Palmerston Mendes et al., 2017). When combined with organic NPs, the unstable hydrophobic drugs showed improved dissolution and stability (Pentek et al., 2017). For instance, the 100% dissolution time of resveratrol, a compound often known as an antioxidant, was found to be approximately 4.5 h when tested alone, while it took approximately 0.5 h in dendrimer-drug complexes (Chauhan, 2018). Additionally, 90% of resveratrol remained in dendrimer-resveratrol complexes compared to 10% for pure resveratrol, which indicates that dendrimers can mitigate stability issues (Pentek et al., 2017). Furthermore, organic NPs have been designed to mitigate the issue of crossing the BBB so that such liposomes and micelles have been reported to protect their vehicles from degradation and transport their loaded therapeutics across the BBB (Spuch and Navarro, 2011; Zou et al., 2017). For another example, the CD163 receptor-targeted liposomes incorporating dexamethasone showed a 3-fold higher delivery rate in the brain than sham group in 6-hydroxydopamine Parkinson’s disease rat models (Tentillier et al., 2016). The solid lipid NPs encapsulating acetylcholinesterase reactivators with the help of multiple emulsion technique presented a 9-fold higher delivery rate than acetylcholinesterase reactivator only group 45 min after injection in organophosphate-induced brain injury rat models (Pashirova et al., 2017).
Another notable advantage of organic NPs is their allowance for active cellular targeting; ligand tagging techniques allow the attachment of ligands, such as antibodies, peptides, aptamers, and folate molecules to the NP surface. The ligands make the organic NPs bind to target cell receptors, leading to efficiently selective delivery with high therapeutic efficacy (Mullen and Banaszak Holl, 2011); the L-carnitine-conjugated poly lactic-co-glycolic acid NPs containing paclitaxel showed a 10-fold higher accumulation in the brain than paclitaxel only injection 2 h after administration in brain tumor mouse models (Kou et al., 2018). In a review article in 2021, apolipoprotein E-conjugated polymeric NPs were emphasized regarding their merits such as the improved transport across the BBB, accumulation in the brain, and inhibition of the accumulation of neurodegenerative disease-related factors (Hartl et al., 2021). Similar to the protein derivative conjugation, glucose-coated micelles piled up in the mouse brains 56-fold higher than micelles without glucose conjugation (Anraku et al., 2017).
Despite exhibiting high biocompatibility, high bioavailability, high penetrability of the BBB, and high targetability, the use of organic NPs still faces several obstacles. One major problem is the difficulty of large-scale production. For example, liposome production currently involves numerous steps, increasing the complexity of the entire manufacturing process and making commercialization difficult (Roces et al., 2020). Moreover, light, temperature, and metal ions may trigger chemical changes in the NPs such as lipid oxidation, leading to permeability changes when these NPs could be exposed to such changes (e.g., during transportation, in storage, in the body after administration; Guimarães et al., 2021). Although promising results—the variety of polymer coatings has been developed to prevent the removal of NPs by mononuclear phagocyte systems, which almost recognize the administered NPs as a foreign body and make those defending systems activated, before they would arrive in the targeted CNS (Owens and Peppas, 2006) and coating NPs with PEG is the most effective manner to prohibit nonspecific protein adherence and subsequent phagocytosis—have been reported (Vonarbourg et al., 2006), potential problem with organic NPs such as liposomes is that they can still accumulate in the liver and spleen; this accumulation triggers phagocyte uptake by mononuclear phagocyte systems, dominantly by Kupffer cells in the liver, and 99% of the administered NPs can be eliminated (Campbell et al., 2018). Additionally, it is another issue that microglia facilitate NP clearance in the CNS, capturing even PEGylated NPs (Gu et al., 2020).
Many promising studies are in progress to overcome these limitations (Guimarães et al., 2021). For example, microfluidics used in liposome manufacturing can simplify and scale up production while enhancing drug encapsulation using “bottom-up” approaches, which have no needs of additional size reduction process (Forbes et al., 2019). Moreover, controlling cholesterol, phosphatidic acid, and PEG content in liposomes has been shown to improve stability (Szabová et al., 2021). However, studies on these areas are limited, these may be described as possible future directions for the development of organic NPs. Despite the efforts to mitigate the limitations of organic NPs, recent studies have focused on hybrid NPs and indirect methods (Shah et al., 2020). But still, the biocompatible, bioavailable, BBB-penetrable characteristics of organic NPs are noteworthy (Table 1).
2.3. Inorganic nanoparticles
Inorganic NPs made of materials such as gold, iron oxide, and carbon have a high surface area to volume ratio (Yao et al., 2020), which enables a high drug-carrying capacity. For example, CNTs have a diameter of 0.4–3.0 nm and a length of 20.0–1000.0 nm, resulting in a high carrying capacity; when the antitumor agent 10-hydroxycamptothecin, which has been investigated for the treatment of brain glioma, was covalently attached to the surface of a multiwalled CNT, it showed a sufficient loading efficiency of approximately 16% (Kostarelos et al., 2005; Wu et al., 2009; Perez-Herrero and Fernandez-Medarde, 2015). Additionally, mesoporous silica NPs have a large inner pore amount, making them advantageous drug-carrying agents (Yao et al., 2020) with a carrying capacity of 100 wt% for anticancer drugs in mouse models (Cheng et al., 2019). The surface modifiability of inorganic NPs enables effective targeting; for example, attaching a folate-containing compound to CNTs enabled specific delivery of therapeutics to folate-receptor + cancer cells (Dhar et al., 2008; Perez-Herrero and Fernandez-Medarde, 2015). Gold NPs, Au-SMCC linker-doxorubicin nanoconjugates, also displayed surface modifiability made by connecting doxorubicin to the surface of gold NPs and showed effective drug accumulation in HepG2-R cancer cells (Cheng et al., 2013). Like aforementioned findings, modified gold NPs on strategies for CNS-targeted delivery have gained considerable attention as the relevance to the context of CNS disease treatment including brain tumors. In addition to the high drug-carrying capability, inorganic NPs such as silver, gold, or quantum dots can cross the BBB with their own mechanisms; gold NPs increased the BBB permeability by disrupting the endothelial tight junctions, in which Na, K, and/or Ca ion channels are be involved, and reached their the peak concentration in the cerebrospinal fluid (CSF) 3-fold higher than that in the blood 6 h after transperitoneal injection in rats, and quantum dots conjugated with fluorescein hydrochloride, which uses glucose transporters to penetrate the BBB, accumulated in the central canal and in the cervical spinal cord, especially in the gray matter, following transcardic and intravenous injection in zebrafishes and rats, respectively (Sela et al., 2015; Seven et al., 2021). Also, multi-walled CNTs, which use energy-dependent transcytosis, completely crossed the BBB and remained persistently in the brain even 24 h following intravascular injection in rats while notable decrement occurred in the peripheral capillaries (Kafa et al., 2015).
Despite the advantages of inorganic NPs, they exhibit immunotoxicity, genotoxicity, or specific tissue toxicities such as neurotoxicity, leading to inflammation, carcinogenesis, fibrosis, cardiovascular diseases, etc. (Mohammadpour et al., 2019). For example, after a month of accumulation in the spleen, gold NPs have been shown to downregulate the expression of genes relevant to wound healing, responses to external stimuli, and defense responses (Balasubramanian et al., 2010). In the liver, genes relevant to lipid metabolism or the cell cycle process were upregulated (e.g., squalene epoxidase expression showed a fold change of 2.9; Balasubramanian et al., 2010). Additionally, cells incubated with polymer-coated multiwalled nanotube F127 for 24 and 48 h showed 60 and 40% cell viability, respectively, indicating cytotoxicity (Ali-Boucetta et al., 2011).
However, inorganic NPs can be controlled by regulating size, morphology, dosage, and chemical factors to regulate their toxicity (Madani et al., 2011). During the generation of NPs, doping, a technique in which impurities are added to the NPs for chemical/physical improvements, may be used to reduce cytotoxicity (George et al., 2010). In doping methods, three types are commonly used; first, elemental doping is conducted at atomic level, and divided into metal doping using such as cerium, cobalt, copper, iron, lanthanum, manganese, potassium, silver, and zinc as dopants, among which manganese is widely used, and nonmetal doping using such as boron, carbon, fluorine, nitrogen, phosphorus and sulfur, among which nitrogen is the mostly applied dopant. Second, it can be performed at molecular level (molecular doping), in which active molecules are encapsulated into vehicles such as metal–organic frameworks, polymers, quantum dots, and silica, among which silica is the mostly used matrix. Third, codoping combines the benefits of codoped elements, through which metal combination, such as iron and sulfur, nitrogen and sulfur, copper and manganese, as well as combination of metal and nonmetal dopants have been achieved (Wang et al., 2022). However, such relationship between the matched dopants and NP matrix is not clearly known so that further studies will be required to establish a guideline for reliable manufacture of doped NPs.
Additionally, iron oxide NPs can be degraded into Fe ions in lysosomes of cells or under other acidic conditions, reducing the potential long-term toxicity (Yu et al., 2012; Mitchell et al., 2021). In a long-term (approximately 13 months) study regarding the safety of ferumoxytol, an FDA-approved iron oxide NP, in the treatment of iron-deficient anemia patients with chronic kidney disease on hemodialysis, no patients experienced intravenous ferumoxytol replacement treatment-related serious adverse events (Macdougall et al., 2019), indicating the relevance of the safety of iron NPs in the context of long-term administration. If toxicity issues can be resolved, inorganic NPs could have a high potential for use in effective direct delivery methods in the treatment of CNS disease due to their ability to easily cross the BBB and their efficient drug loading capacity (Table 1).
2.4. Extracellular vesicles/exosomes
While NPs are in common synthetic drug delivery vehicles (Sun et al., 2022), EVs are innate, nano-sized, phospholipid bilayer-enclosed vesicles (Elsharkasy et al., 2020) that can be, in theory, released by all kinds of cells (Rufino-Ramos et al., 2017) through exocytosis for intercellular communication. Recently, EVs have gained attention as a delivery tool of therapeutics owing to their groundbreaking preclinical success in brain-targeted delivery. Though the degree may vary depending on the type, EVs hold notable advantages for CNS-targeted delivery of therapeutics because of their noninvasiveness, high biocompatibility, permeability across the BBB, and high stability in vivo (Elsharkasy et al., 2020). Moreover, the surface of EVs can be engineered to increase brain targetability (Marie et al., 2021). Three main types of EVs include apoptotic bodies (ApoBDs), microvesicles (MVs), and exosomes. Major difference among the three types include their formation process, size, and origin (Shao et al., 2018; Nederveen et al., 2021). ApoBDs and most MVs have a large particle size (Elmore, 2007; Shao et al., 2018), making them not ideal candidates for targeted delivery to the CNS. Therefore, authors briefly introduce ApoBDs and MVs, and then concentrate on describing exosomes—the smallest EV type, which may easily pass through the BBB so that it holds a superior potential for CNS-targeted delivery than other EVs (Shao et al., 2018).
Apoptotic bodies are the largest of EVs (500–4,000 nm; Elmore, 2007) that are formed during apoptotic cell disassembly where fragmented cell contents such as organelles and nuclear content are enclosed in membrane-bound vesicles (Santavanond et al., 2021). ApoBDs successfully trigger clearance of damaged cells in tissue development and regeneration of the kidney and bone (Li et al., 2020). MVs are EV subtypes that are released directly from the cell surface of platelets, red blood cells, and endothelial cells (Yang J. et al., 2021) and range in size from 200 to 2,000 nm (Shao et al., 2018). MVs may transport proteins and miRNA between cells and have been used for the diagnosis of autoimmune diseases, treatment of acute kidney injury, etc. (Liu et al., 2016). While ApoBDs and MVs may be tried for CNS-targeted therapy with the help of indirect, BBB penetration-assisting methods, there is limited evidences on the two methods.
Exosomes are the smallest EVs (40–200 nm) that are formed in all types of cells when vesicles bud into endosomes inside cells (Shao et al., 2018). Exosomes may directly penetrate the BBB, are highly stable in peripheral circulation, and thus may protect disease-specific therapeutic molecules, unlike ApoBDs and MVs. At the beginning of researches, exosomes were studied as a biomarker to monitor disease development, allowing early diagnosis and treatment optimization in such stroke, glioma, Parkinson’s diseases, Alzheimer’s diseases, Huntington’s diseases, and amyotrophic scleroses (Liu et al., 2019; Fan et al., 2022). After that period, exosome trials have been extended to therapeutic role for CNS disease as well (Fan et al., 2022). In a Martins et al.’s research, the ability of two Aβ-binding proteins—alpha-1-antichymotrypsin and C4b-binding protein alpha chains—was analyzed to validate exosome levels in patients by using antibody-based methods, indicating significant correlations between alpha-1-antichymotrypsin exosomal concentrations and mini-mental state examination scores and clinical dementia rating scores, indicators of cognitive alterations. Moreover, C4b-binding protein alpha chains was reported to limit the complement activation by Aβ and dead cells in Alzheimer’s disease brains, potentially protecting the neurons from immune responses (Soares Martins et al., 2022). Recently, another notable advantage of exosomes was discovered; their inherited membrane proteins—such as CD9, CD63, prostaglandin F2 receptor negative regulator, and Lamp2b—can be genetically and/or chemically engineered to increase tissue-targetability, showing promising results in preclinical studies for CNS targeting (Sun et al., 2022). In a study by Alvarez-Erviti et al., Lamp2b-RVG plasmids were created to transfect autologous-derived dendritic cells, stimulating the secretion of engineered exosomes. Following that engineering, the exosomes were intravenously injected to mice, resulting in specific delivery of siRNA to neurons, oligodendrocytes, and microglia in the brain, ultimately leading to specific gene (BACE1) knockdown for Alzheimer’s diseases (Alvarez-Erviti et al., 2011). As such, exosomes may be used for targeted delivery of various therapeutics, such as proteins, siRNAs, miRNAs and even drug ingredients of low molecular weight, to injured CNS neurons with the help of genetically and/or chemically engineered surface modification (Sun et al., 2022; Zhou et al., 2022).
Despite such advantages, however, clinically approved CNS-targeted exosomes would require further investigation regarding detailed methods in pointed view of penetration efficiency of the BBB as well as the development of quantitative and qualitative monitoring and imaging tracking methods about targeted delivery to the CNS neurons (Hojun Choi et al., 2022). Moreover, in a research on wound healing promotion using adipose cell-derived exosomes, it was found that the metabolic condition of exosome donors affects the biogenesis, biological activity, and composition of the adipose stem cells (Cianfarani et al., 2013), thus affecting the adipose stem cell exosomes. Technically, isolation and purification methods should also be validated for reliable production in large scale (Rufino-Ramos et al., 2017).
2.5. Hybrid nanomedicine
Hybrid NPs are a combination of organic and inorganic NPs, which enable the combined advantages of both types of NPs to be leveraged for the treatment of CNS disease, and these NPs exhibit tunable size and surface charge based on the components included. The size and surface chemistry of NPs are critical since they affect the efficacy of NP delivery to diseased tissues, redistribution of NPs within the body, and potential toxicity (Walkey et al., 2012). One example of the tunability of the size of hybrid NPs is the use of different lipids; in a study comparing antibiotic-loaded lipid-polymer hybrid NPs (LPHNPs) engineered with cationic and zwitterionic lipids, the diameter of cationic LPHNPs was 203 ± 6.6 nm and that of zwitterionic LPHNPs was 191 ± 5.4 nm (Bose et al., 2020). Moreover, when cationic or zwitterionic LPHNPs were introduced into the LPHNP formulation, the charge was reduced by −29 ± 2.1 mV, indicating that LPHNPs are more advantageous in terms of their ability to interact with surrounding cells, their penetration of the BBB, and their colloidal stability (Bose et al., 2020).
High drug-loading yield and sustained drug release are other advantages of hybrid NPs; when the encapsulation efficiencies (%EE) of doxycycline-bare NPs and cationic or zwitterionic lipid layered LPHNPs loaded with doxycycline were compared, the results corresponded to a %EE of 63, 71, and 79%, respectively. Moreover, the antibiotic release rates of cationic LPHNPs at 12, 24, and 48 h were 38, 54, and 66% when zwitterionic LPHNPs were 32, 47, and 61% and doxycycline-bare NPs were 65, 69, and 75%, respectively (Bose et al., 2020).
Hybrid NPs also exhibit high stability and biocompatibility because their outer shells are combinations of the organic and inorganic shells. In an in vivo gene delivery study, bare pCas9/methylguanine methyltransferase degraded within 3 min of incubation with DNase I, and pCas9/MGMT plasmids loaded in LPHNPs remained intact throughout varying durations of incubation with DNAse I (Yang Q. et al., 2021). This result indicates that LPHNPs successfully protected pCas9/methylguanine methyltransferase from enzyme degradation and can be used as a stable delivery vehicle (Yang Q. et al., 2021). In addition, the application of LPHNPs after exposure to varying concentrations of cisplatin and curcumin demonstrated a cell viability of >80% based on CCK-9 assay results in A2780 tumor cells (Khan et al., 2020). Nevertheless, for the hybrid NPs, there were only in vitro performed studies that depicted in vitro or plainly brain cell update; for instance, Fe3O4 LPHNPs manufactured by self-assembly technique penetrated a BBB model composed of human brain microvascular endothelial cells (BMVECs) under magnetic field guidance (Qiao et al., 2020), and mesoporous silica liposome of poly(acryl acid)-hybrid NPs conjugated with angiopep-2 showed the enhanced penetration rate of the BBB model of BMVECs (Tao et al., 2019). Yet, without in vivo studies, it becomes difficult to explain the validity of the targeting experiment.
Despite such advantages, further studies are needed to determine the proper balance of the ratio of the components of the outer shell (i.e., cholesterol). This ratio is important in CNS targeting, as it affects the stability of antigen release, lipid layer stability, and the protection of the integrity of the hybrid structure during long-term storage (Hu et al., 2010). Therefore, recent studies have focused on manufacturing hybrid NPs in an appropriate ligand/receptor density ratio, as this ratio substantially affects their targeting abilities (Alkilany et al., 2019). For example, there was a study in which equimolar amounts of five different lipids—ethyl arachidate, myristic acid, stearic acid, ethyl myristate, and glycerol monostearate—were tested in the form of BBB-crossing terpolymer lipid-hybrid NP, in which polysorbate 80 was covalently attached to poly(methacrylic acid)-grafted-starch, for colloidal stability and in vitro application when delivering doxorubicin to glioblastoma multiform (GBM) brain tumor cells. Of the different lipids tested, the ethyl arachidate doxorubicin-hybrid NP showed the most ideal colloidal properties, stability, and highest cytotoxicity against GBM cells (Ahmed et al., 2021). To address the remaining challenges in identifying the ideal out shell composition of hybrid NPs for different CNS therapies, additional studies should focus on determining the proper ratio of the outer shell components in correspondence with degree of in vivo stability, targetability, and bioavailability, respectively, in individual types of CNS disease and therapeutics (Table 1).
Similar to the hybrid NPs, hybrid nanomedicine, a combination of NPs and exosomes, has been recently tried, especially in cancer researches; for example, a CD47-expressing exosome cRGD-modified liposome-hybrid nanomedicine inhibited activation of mTOR pathway in ovarian cancer cells indicating targeted codelivery of triptolide and miR497 (Li et al., 2022), and another hybrid nanomedicine combining granulocyte-macrophage colony-stimulating factor-overexpressing exosomes and docetaxel-loaded liposomes showed enhanced codelivery of granulocyte-macrophage colony-stimulating factor and docetaxel in metastatic peritoneal cancer rats (Lv et al., 2020). Meanwhile, reported are just few studies showing a potential of hybrid nanomedicine application to CNS-targeted delivery (Rufino-Ramos et al., 2017); for instance, enveloped protein nanocages precisely matching the targeted scaffold via viral glycoprotein were inserted into the EV (Votteler et al., 2016), and exosome-mimetic nanovesicle, in which therapeutic drugs were contained through repetitive macrophage and monocyte breakdown using sequential filtering of extrusion (Jang et al., 2013). However, application of hybrid nanomedicine to CNS-targeted delivery is at its initial stage of development so that it would require further approval in feasibility and validity as the limitations of hybrid NPs do.
In addition to researches on improving the hybrid.nanomedicine themselves, it is expected that future studies on hybrid nanomedicine will be focused on their combination with other indirect methods, such as focused ultrasound (FUS; Ahmed et al., 2021) and photodynamic therapy (PDT; Zhang et al., 2020), approaches of which will hold promise despite the need for additional studies.
3. Magnetic field-mediated delivery
This review introduces magnetic delivery in chronological order of their development: magnetic field-mediated passive navigation, magnetotactic bacteria (MTB), magnetic resonance navigation (MRN), and magnetic nanobots. It is important to note that due to preexisting categorical limitations of targeted delivery, this review classify magnetic field-mediated delivery as a direct method, although it actually has indirect characteristics in nature.
Magnetic navigation is a delivery method that accumulates magnetic micro/nanodrug carriers in the targeted area by controlling the direction and propulsion of the carriers with externally provided permanent magnets or electromagnets; this technique has the advantages of navigational delivery to an injury site and exclusive localization within the injury site, allowing therapeutics to escape from emulous binding with non-in situ receptors, specifically in the context of CNS-targeted delivery (Chorny et al., 2011). In vitro and in vivo studies involving centralizing scattered ferromagnetic rods/microcapsules have shown that magnetic forces may be used to successfully manipulate magnetic materials for directional navigation (Nacev et al., 2015; Voronin et al., 2017). Furthermore, magneto-nanobots can be utilized as magnetic field-mediated, actively assisted navigational vehicles that enable cell-specific targeting; in a study where an external magnetic field and magnetic nanobots consisting of magnetic NPs (Fe3O4NPs) were used to deliver anticancer drugs, the nanobots were shown to gain thrust from O2 emissions produced from the decomposition of H2O2 released from tumor cells (Andhari et al., 2020). Attaching Fe3O4 to the NPs provides autonomous propulsion and superparamagnetic properties to the nanobot system (Andhari et al., 2020), suggesting a potential of the targeted delivery to deeply located lesions within the CNS. However, using an external magnetic field is difficult in tissues greater than 2 cm deep within the body, as the aforementioned advantages of magnetic field-mediated directional navigation sharply decrease with increasing distance between the magnets and the carriers (Hwang, 2020). Although internal methods such as the implantation of magnets within the body have been devised as an alternative (e.g., intrathecal implant; Lueshen et al., 2015), implants can trigger side effects such as infection and intolerance to them (Ganz, 2017). Thus, these disadvantages of external and internal methods should be augmented to promise better magnetic field-mediated delivery.
Magnetotactic bacteria are groups of bacteria that contain magnetosomes, which are intracellular structures that consist of biological materials such as magnetite (Fe3O4) and greigite (Fe3S4); MTB-derived magnetosomes thus have higher biocompatibility than artificial NPs due to their biological properties (Alphandery et al., 2017). Magnetosomes can be isolated from MTB and used as drug delivery carriers, and, as an alternative to paramagnetic/superparamagnetic NPs, MTB themselves can be loaded with drug-carrying vehicles for magnetic field-mediated navigation into target tissues. In one recent study, when magnetosome toxicity was evaluated in mice, complete blood counts and the results of a basic metabolic panel were within the normal range, and no change in the composition of urine or weight was found (Nan et al., 2021). Additionally, drug-carrying MTB with long actin-like filaments encoded by the mamK gene exhibit magneto-aerotaxis, which enables them to align with the direction of an applied magnetic field, move toward the hypoxic regions of targeted cells, and directly navigate the MTB-loaded vehicles to the CNS (Marcuello et al., 2018). In addition, magnetosomes have been shown to promote the axonal outgrowth of neurons through stretch growing when magnetic fields are applied (De Vincentiis et al., 2021). In one study, a total of 500–700 μg of magnetosomes coated with poly-L-lysine and magnetic fields of 202 kHz and 27 mT were used to treat GBM brain tumor cell models, and living GBM cells in all mice completely disappeared after 68 days (Alphandery et al., 2017). Although MTB have many advantages, they may adversely affect the human immune system by decreasing the level of lymphocyte proliferation (Nan et al., 2021). The composition of magnetosomes produced in MTB is often difficult to reproduce, leading to their low production yield (Alphandery et al., 2017). To overcome these limitations, MRN was developed.
Magnetic resonance navigation is a delivery method that uses a magnetic resonance imaging (MRI) scanner to provide propulsion to microcarriers (up to 400 mT/m) and to make them follow a preprogrammed route to the targeted area (Martel, 2010). Administrating MRN consists of three steps—steering, creating a magnetic gradient, and real-time tracking imaging—that are repeated until the carrier reaches the target (Pouponneau et al., 2014; Li et al., 2019). In a study testing MRN targeting in a two-level bifurcation, magnetic drug-eluting beads aggregated with targeting success rates of 84, 100, 84, and 92% for the four different ends of the branch routes (Li et al., 2019). In another study on rabbit hepatic arteries, the MRN successfully steered therapeutic magnetic microcarriers to the left lobes (Pouponneau et al., 2014). In these studies, MRN showed targeting effectivity both in the one-level bifurcation in an animal model and in the higher level of bifurcation of artificial pathways; these findings demonstrate its potential use in targeting more complex pathways in the human body (Pouponneau et al., 2014; Li et al., 2019). However, the references currently cited are based on the treatment of hepatocellular carcinoma by liver chemoembolization. Hence, it is unsure how will it perform well for the brain, which should be evaluated in future studies.
Furthermore, factors such as pulsatile flow can currently limit the use of MRN in in vivo studies to even simple pathways, such as one or two Y-bifurcations and 2D vascular phantoms (Folio and Ferreira, 2017). Moreover, some nonspherical microparticles navigated using MRN occasionally attach to the surface of nontarget tissues (Ghosh et al., 2021). However, this problem may be solved by increasing the magnetic gradient to overcome friction and by administering orthogonal pulses (Ghosh et al., 2021). While limitations exist, these studies demonstrate the targeting effectivity and potential of MRN for use in more complex pathways, making it one of the most promising tools for CNS-targeted therapy (Table 2). However, it should be in mind that it comes from the authors’ personal extrapolation from the current information and their use in clinical paradigms cannot be justified yet due to such major limitations.
4. Indirect targeted delivery
The most considerable barrier to the use of direct targeted delivery has been their inability to allow therapeutics that exceed 400 Da to pass through the BBB (Pardridge, 2012; Cui and Cho, 2022). To overcome this disadvantage of the direct targeted delivery, development of indirect targeted methods has been demanded. In this section, the authors aim to introduce indirect chemical and physical methods that open the BBB, the mechanisms of each method, their advantages and disadvantages, and discussion of the recent findings with important implications. Chemical and physical methods can increase the permeability of the BBB using chemicals and physical energy, respectively. Mannitol has been widely used to decrease intracranial pressure (Chengyan Chu et al., 2018), but shown side effects such as edema and renal failure (Visweswaran et al., 1997) and a short efficacy time (Cosolo et al., 1989). Therefore, other chemicals, such as bradykinin and 1-O-pentylglycerol, have been studied. After briefly introducing chemical methods, this section mainly focuses on describing two main physical methods that are being developed: FUS and LASER therapy.
4.1. Chemical delivery
Indirect chemical targeted delivery is a method that chemically increases the permeability of the BBB, thereby allowing therapeutics to pass the BBB and take effect in the CNS (Pardridge, 2012; Dong, 2018; Cui and Cho, 2022). Hyperosmotic mannitol is the most widely used BBB permeabilizer (Chengyan Chu et al., 2018). It triggers dehydration of BBB endothelial tissues and deflates cell bodies, causing loosening of tight junctions, which enhances the permeability of drugs, stem cells, liposomal vehicles, and antibodies (Hendricks et al., 2015). However, when mannitol was injected into adult Sprague–Dawley rats at the optimum rate of 0.25 ml.s-1.kg-1 for 20 s, BBB disruption has been shown to last for approximately 5 min at maximum and then rapidly reverse (Cosolo et al., 1989), and administering 1,171 ± 376 g mannitol to a patient with normal baseline renal function resulted in acute renal failure (Dorman et al., 1990). Moreover, intracarotid arterial hyperosmolar mannitol increased the production of cytokines, chemokines, and trophic factors, leading to a neuroinflammatory response (Burks et al., 2021). Therefore, as mannitol has a short efficacy time and potential provocation of renal failure and systemic toxicity, and causes a neuroinflammatory response, other chemical methods are being developed to overcome such limitations.
Bradykinin is another agent used to permeabilize the BBB. Bradykinin is a vasoactive compound that selectively increases the permeability of abnormal brain capillaries (Black, 1995). A study using an RG2 rat glioma model demonstrated that stimulating the B2 bradykinin receptor rapidly increases the permeability of the BBB, especially in brain-tumor-associated tissue (Bartus et al., 1996). Moreover, treating female Wistar rats with bradykinin caused a 3.3-fold increase in the number of small arterioles, a 2.1-fold increase in the number of medium arterioles and a 1.5-fold increase in the number of large arterioles, alluding to an increase in pinocytotic vesicle density and BBB permeability (Raymond et al., 1986). However, it has a short half-life (27 ± 10 s; Cyr et al., 2001), may decrease the blood flow to the cerebrum, and causes extravasation, necrosis, edema, and BBB breakdown at high dosages or pathological conditions, since it is a potent vasoactive compound for arterial dilation (Wahl et al., 1999). To overcome such limitations, bradykinin receptor agonist NG291 is being developed to induce a rapid onset of transient BBB disruption without early brain injury. In a Sprague Dawley rat and CD-1 mouse model study, notable NG291-induced increase in BBB permeability was revealed in a localized, reversible, dose-dependent and P-gp efflux transport-mediated manner. Moreover, NG291 did not evoke short-term neurotoxicity, nor the increase of brain water content, the number of Fluoro-Jade C positive cells, and astrocyte activation (Rodríguez-Massó et al., 2021), indicating that biased agonism may play a role in enabling therapeutics to specifically target BBB opening without causing brain injury.
1-O-pentylglycerol is an alkylglycerol that has also been investigated for its ability to permeabilize the BBB. 1-O-pentylglycerol affects the endothelial cell morphology of the barrier and disrupts the BBB structure (Hülper et al., 2013). For example, an in vivo tumor-free and C6 glioma-bearing rat experiment indicated that intracarotid injection of 1-O-pentylglycerol caused a transfer of fluorescein and lissamine-rhodamine B200 across the BBB, as the mean ratio of ipsilateral to contralateral fluorescence intensity in the coronal sections for fluorescein was 6.45 ± 1.4, and the mean ratio of lissamine-rhodamine B200–albumin was 2.66 ± 1.0 (Erdlenbruch et al., 2003), which indicates applicability as one of brain tumor therapeutics. Additionally, no 1-O-pentylglycerol accumulation was found in the brain, and more than 70% of the administered dose was excreted within 270 min after administration, which suggests rapid renal elimination and the nontoxicity of 1-O-pentylglycerol (Erdlenbruch et al., 2005). While 1-O-pentylglycerol raised concerns regarding immunogenicity and thus adverse effects in nontarget cells, incubating bEnd3 mouse brain cells with poly(alkyl cyanoacrylate)-alkylglyceryl dextran did not show significant toxicity at concentrations <25 μg/mL (Ibegbu et al., 2017).
Although various indirect chemical targeted delivery methods exist and are under development, it is not easy to reach a consensus regarding a specific chemical to recommend. The most researched and used chemicals—mannitol, bradykinin, and 1-O-pentylglycerol—each has unignorable shortcomings, such as short efficacy time (Erdlenbruch et al., 2005), drug leakage (Rodríguez-Massó et al., 2021), and systemic toxicity (Dorman et al., 1990). Due to the aforementioned significant side effects, their use in clinical paradigms cannot be justified yet because of such major limitations. Therefore, indirect physical targeted delivery methods are being developed to remedy such limitations.
4.2. Physical delivery
4.2.1. Focused ultrasound
Focused ultrasound is a noninvasive augmentation method that opens the BBB through the application of high-intensity focused ultrasound (HIFU), which helps deliver therapeutics to the targeted brain region. When the FUS wave is applied in a pulsed manner, tissues experience a cycle of high and low pressure (Timbie et al., 2015). FUS is used to generate microbubbles, which act as contrast media, and these bubbles function as echo-enhancers that pass through the ultrasound field, resulting in stable cavitation (Lee et al., 2017). Then, the blood vessels are mechanically stimulated by the microbubbles, which leads to opening of the BBB. Since the microbubbles can concentrate the ultrasonic energy, the initial ultrasound pressure needed for opening the BBB is considerably reduced, leading to the BBB opening with lesser risks of skull bone heating (Burgess et al., 2015).
Focused ultrasound increases the bioavailability of therapeutics in the target area and helps them maintain their bioactivity throughout the delivery processes (Huttunen and Saarma, 2019). In a recent mouse study of Parkinson’s disease, FUS was used with two sonication locations in regions that showed severe damage in Parkinson’s disease: the caudate-putamen and substantia nigra (Dauer and Przedborski, 2003), and varied pulse lengths of 5,500 cycles (3.3 ms) to 10,000 cycles (6.6 ms) were used. When neurturin, a type of neurotrophic factors, was directly injected, its bioavailability area was limited to an average of 0.20 ± 0.05 mm2. However, with FUS, the bioavailability of neurturin was 5.07 ± 0.64 mm2 in the caudate-putamen and 2.25 ± 1.14 mm2 in the substantia nigra, showing a maximum 25-fold increase due to BBB opening, allowing neurotrophic factors to pass through (Samiotaki et al., 2015). Additionally, FUS causes minimal damage to the nervous system while increasing the delivery rate of therapeutics passing through the BBB. In a study of nonhuman primates in which the short-term (2 days) and long-term (18 days) effects on FUS-mediated BBB opening and the secondary effect on visual-motor cognitive task-related touch accuracy or reaction time were assessed, response accuracy did not decrease in the short term (from 0.76 ± 0.08 to 0.80 ± 0.03) and nor in the long term (from 0.62 ± 0.08 to 0.68 ± 0.05), which suggests that cognitive performance dose not worsen following the FUS-induced BBB opening in nonhuman primates. In addition, no observable damage, microhemorrhage, or necrosis was observed in the histological test after the nonhuman primates were euthanized (Pouliopoulos et al., 2021). The aforementioned results suggest that FUS should be evaluated in healthy human populations to check whether it causes deterioration of cognitive functions or additional damage to the nervous system.
Although FUS has advantages, there are also limitations in applying FUS in clinical practices, especially regarding intensity. HIFU treatment with a frequency of 20 MHz generating a focal intensity ranging of 1,000–10,000 W/cm2 may induce skin burns and neuronal damage due to thermal injuries caused by the inverse piezoelectric effect, leading to irreversible coagulative necrosis (Fan et al., 2012). In contrast to HIFU, low-intensity focused ultrasound (LIFU) with a peak intensity ranging of 10–500 W/cm2 can increase the penetration of the therapeutics but may not cause side effects such as microhemorrhage and ischemia (Meng et al., 2018). Regarding the safety margin of FUS, LIFU treatment with a frequency ranging of 220–650 KHz producing a focal intensity of 80 W/cm2 through an intact cranium induced no significant hemorrhage or necrosis in the necropsic cortex of eight 3–4-month-old swine (Zibly et al., 2014). Therefore, controlling the intensity and establishing clinical evidence is necessary for FUS to be an effective treatment. In authors’ personal opinion, the focal peak intensity ranging of 10–80 W/cm2 is ideal for FUS-based indirect delivery of therapeutics into the CNS in term of safety and efficiency: the stronger intensity, the better permeability but more adverse effects. Nevertheless, if more animal studies can be performed and the results applied to humans, FUS may be an effective way to help deliver therapeutics in a minimally invasive manner.
4.2.2. Light amplification by the stimulated emission of radiation therapy
Light amplification by the stimulated emission of radiation (LASER) therapy has been used in neurosurgery to destroy unhealthy brain tissue by creating heat. However, excess heat can induce damage to neighboring nontarget tissues. Therefore, various studies have been conducted to increase the spatial precision of LASER therapy, leading to the development of CNS-targeted therapy that includes using LASER to change cells into a specific state in which LASER increases the permeability of the BBB and subsequently deliver therapeutics. Currently, LASER interstitial therapy (LITT), PDT, and photobiomodulation therapy (PBM) are three approaches to LASER therapy that are studied for CNS-targeted delivery. LITT is a technique used to increase local BBB permeability by directing a LASER to the target area, transmitting light energy through a thin fiber, which is then converted into thermal energy, and creating a local disruption of the BBB (Melnick et al., 2021). LITT has been applied to the treatment of CNS diseases such as GBM (Patel et al., 2020). LITT-induced hyperthermia disrupts the BBB, with peak permeability for anti-neoplastic drugs happening by 1–2 weeks following ablation and disappearing by 4–6 weeks (Leuthardt et al., 2016). However, there are difficulties in controlling temperature to prevent thermal damage to nearby organs or structures (Salem et al., 2019); specifically, high temperature—close to or above 100°C—at the tissue can cause water to boil, and undesirable air bubbles can be produced (Zhu et al., 2017). To overcome this problem, thermal MRI has been introduced to monitor the heating process in real time and deactivate the system when the temperature reaches a critical level (Salem et al., 2019; Chen et al., 2021). However, well-designed prospective clinical trials and appropriate follow-up time are required to check patient outcomes with using LITT.
Another type of LASER therapy is PDT, which consists of three major elements: photosensitizers, light energy, and tissue oxygen. When a specific wavelength of light activates the photosensitive agent, the photosensitizer generates cytotoxic reactive oxygen species (ROS) such as hydroxyl radicals and singlet oxygen. With the help of photosensitizers such as 5-aminolevulinic acid and phthalocyanines, PDT can site-selectively open the BBB by destroying tight junction proteins and increasing the tight junction gap (Semyachkina-Glushkovskaya et al., 2020). However, although PDT can increase BBB permeability, it cannot utilize near-infrared light, making it difficult to allow deep penetration and low phototoxicity to normal tissues (Lan et al., 2019). Therefore, two-photon excitation and upconversion NPs, such as angiopep-2 conjugated NPs, are being studied to minimize invasiveness (Tsai et al., 2018).
The mechanism of PBM action in cells is the same as that observed in the use of PDT, but the two have apparent differences. PDT requires exogenous photosensitizers, and its high level of ROS generation causes cellular damage, while PBM utilizes endogenous cellular photosensitizers, resulting in low ROS levels that may not deteriorate cellular functions (Lubart and Friedmann, 2011). Furthermore, the combination of PDT and PBM can act as a synergistic therapeutic tool with the help of increased efficacy of anti-neoplastic treatment by increasing ATP production in mitochondria and increasing the production and homogeneity of protoporphyrin X, which is an endogenous photosensitizer required in PDT (Joniová et al., 2021). Additionally, in a study, PDT (660 nm) combined with PBM (850 nm) in which the Ru complex was used as a photosensitizer, increased photocytotoxicity was observed in A375 tumor cells (Negri et al., 2019). However, in the transcranial PBM approach, light cannot penetrates the brain more than 20mm from the cortex, making it difficult to use in delivering sufficient doses to the targeted region (Moro et al., 2014). Further study and clinical tests are required to fully understand the mechanism of PBM, solve these limitations, and improve the efficacy of PBM.
5. Limitations/challenges
Direct and indirect targeted deliveries have many advantages and a great potential for use in CNS disease treatments, but have some limitations to be overwhelmed. Organic NPs require complicated process in production, and accumulate in the liver and spleen, and have a short half-life, and inorganic NPs have systemic toxicity involving multiple organs to be solved. Exosomes need further studies to figure the dependency of their characteristics on progenitor cells and nonstandardization of isolation and purification out. Hybrid nanomedicine demands the appropriately balanced ratio of the outer shell components, such as ligand/receptor density ratio, for the stability. Also, magnetic field-mediated delivery has difficulty in maintaining magnetic field greater than 2 cm deep within the body and navigational ability properly with increasing distance, MTB may trigger activation of the human immune system, and MRN has the obligation to use pulsatile flow, which disturbs its constant magnetic guidance even in simple pathways, and the tendency of nonspherical microparticles to attach to nontarget tissues surfaces. Regarding challenges of indirect methods, chemical deliveries, such as bradykinin and 1-O pentylglyceol, have numerous disadvantages; a short half-life, extravasation, adverse effects in nontarget organs. FUS has safety issues, intensity-related thermal injury by the inverse piezoelectric effect, and LASER therapy has problems of phototoxicity and generation of local hyperthermia and ROS to be solved.
Meanwhile, many of the delivery methods, especially NPs, described in the current manuscript have been known to reduce libido; for example, silver NPs compromised reproductive activity such as sperm motility 7 days after intravascular injection in rabbits (Castellini et al., 2014). Similarly, treatment of unconjugated titanium, gold, and silver NPs reduced the motility and viability of cryopreserved bovine spermatozoa from post-equilibration to post-thaw period following ejaculation (Pande et al., 2022). Furthermore, gender difference was reported in hepatic toxicity, especially dominant in female sex, after oral exposure of titanium NPs over 90 days in rats (Chen et al., 2019). Also, male sex-dominant differences in histopathology and mortality were noticed after single oral administration of copper NPs at higher dose in rats (Lee et al., 2016). To the contrary, no gender difference was revealed in bone marrow toxicity after oral exposure of silver NPs for 28 days in rats (Kim et al., 2008). Now, there are few clinical studies investigating gender difference of NPs; interestingly, in a randomized controlled clinical trial in which 19 patients with carious teeth were recruited, subgroup analysis showed no gender difference in marginal integrity restoration of resin composites following pretreatment with silver and gold NPs (Nemt-Allah et al., 2021). Hence, it requires further studies prior to discussion about their gender based effect.
6. Future directions and conclusion
In direct CNS-targeted delivery, organic NPs show high biocompatibility and low immunogenicity but are difficult to produce at a large scale, lack stability, and are eliminated too early. Inorganic NPs show a high drug-carrying capacity, surface modifiability, and magnetic field-mediated navigability, but have toxic side effects. To overcome these limitations, efforts have been made to control the components of outer shells for better stability and reduce factors that cause toxicity. Although hybrid NPs, a combination of organic and inorganic NPs, have many advantages, such as tunable size, high stability, and high drug-loading yield, they have side effects, including harmful tissue deposition patterns, unintended activation of the host immune response, and damage to nontarget cells. Among EVs, exosomes show a notable potential for CNS-targeted delivery of therapeutics because of genetically and/or chemically engineered targetability of inherited surface proteins as well as biocompatibility and permeability across the BBB. Therefore, studies are in progress to manufacture NPs, exosomes, and ligands in an appropriate ratio, and contingencies such as PEG stealth technology have evolved, making hybrid nanomedicine, a combination of NPs and EVs, an effective direct delivery method. Another advantageous direct delivery method is magnetic field-mediated delivery, which utilizes magneto-nanobots, MTB, and MRN. However, methods using an external magnetic field have difficulty reaching deep targeted regions, and MTB negatively affect immune system of the human body and have a low production yield. Therefore, MRN was developed to overcome these issues, showing successful targeting efficacy in simple pathways in in vivo studies. While further studies are needed to apply MRN in more complex pathways, due to its pulsatile flow-induced navigability weakening in real time, it is one of the most promising direct CNS-targeted delivery methods.
As there are obstacles to overcome in the use of direct CNS-targeted delivery methods, indirect delivery methods have been developed and are used together with direct methods to create a synergistic effect. Chemicals such as mannitol and bradykinin effectively increase the BBB permeability but have a short half-life and show toxicity when injected. Although short-chain alkylglycerols do not have the same drawbacks as mannitol and bradykinin, they lead to complications related to immunogenicity problems and can invade nontarget cells. While FUS, one of the physical targeted delivery methods, increases and maintains the bioavailability of therapeutics and causes minimal damage to the CNS, it has problems related to delivery intensity that need to be solved to make this method more advantageous. LASER therapies, such as LITT, PDT, and PBM, have recently gained prominence and have been increasingly used for disrupting the BBB. However, they still have limitations—thermal damage, shallow penetration, and phototoxicity—to overcome, so thermal MRI is being introduced in LASER therapies, and two-photon excitation and upconversion NPs are being studied. Based on the outcomes, such as anti-neoplastic activity and validity of endogenous photosensitizers, LASER therapy may be one of the most promising indirect CNS-targeted therapy methods. While this article has recommended the most promising direct and indirect targeted therapies, it is important to note that combining the direct and indirect methods, such as hybrid nanomedicine of organic, inorganic NPs, and exosomes via MRN following preconditioning treatment with either PBM or LIFS, will be more effective and focused in the future (Figure 2).
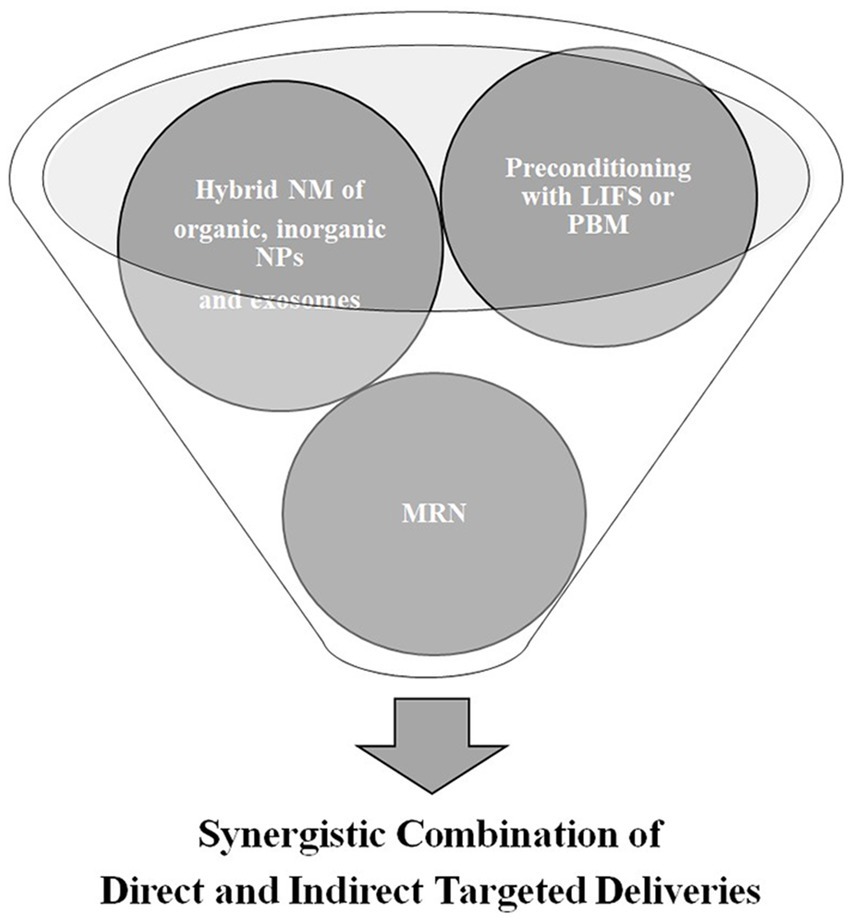
Figure 2. Future directions of targeted delivery of therapeutics in central nervous system diseases; low intensity focused ultrasound (LIFS); photobiomodulation therapy (PBM); nanomedicine (NM); nanoparticles (NPs); and magnetic resonance navigation (MRN).
Author contributions
JA, SW, HS, SI, GY, SL, K-iK, and CH substantially contributed to all of the following aspects of this study: (1) study conception and design, acquisition of data, or analysis and interpretation of data, (2) drafting or critical revision of the article for important intellectual content, (3) final approval of the version to be published, and (4) agreement to be accountable for all aspects of the work in ensuring that questions related to the accuracy or integrity of any part of the work are appropriately investigated and resolved. All authors contributed to the article and approved the submitted version.
Funding
This work was supported by the 2022 Research Fund of University of Ulsan, Ulsan, Republic of Korea. The study sponsor had no involvement in the study design; in the collection, analysis, or interpretation of data; in the writing of the manuscript; or in the decision to submit the manuscript for publication.
Conflict of interest
The authors declare that the research was conducted in the absence of any commercial or financial relationships that could be construed as a potential conflict of interest.
Publisher’s note
All claims expressed in this article are solely those of the authors and do not necessarily represent those of their affiliated organizations, or those of the publisher, the editors and the reviewers. Any product that may be evaluated in this article, or claim that may be made by its manufacturer, is not guaranteed or endorsed by the publisher.
References
Ahmed, T., Liu, F. F., He, C., Abbasi, A. Z., Cai, P., Rauth, A. M., et al. (2021). Optimizing the Design of Blood-Brain Barrier-Penetrating Polymer-Lipid-Hybrid Nanoparticles for delivering anticancer drugs to Glioblastoma. Pharm. Res. 38, 1897–1914. doi: 10.1007/s11095-021-03122-9
Ali-Boucetta, H., Al-Jamal, K. T., Müller, K. H., Li, S., Porter, A. E., Eddaoudi, A., et al. (2011). Cellular uptake and cytotoxic impact of chemically functionalized and polymer-coated carbon nanotubes. Small 7, 3230–3238. doi: 10.1002/smll.201101004
Alkilany, A. M., Zhu, L., Weller, H., Mews, A., Parak, W. J., Barz, M., et al. (2019). Ligand density on nanoparticles: a parameter with critical impact on nanomedicine. Adv. Drug Deliv. Rev. 143, 22–36. doi: 10.1016/j.addr.2019.05.010
Alphandery, E., Idbaih, A., Adam, C., Delattre, J. Y., Schmitt, C., Guyot, F., et al. (2017). Development of non-pyrogenic magnetosome minerals coated with poly-l-lysine leading to full disappearance of intracranial U87-Luc glioblastoma in 100% of treated mice using magnetic hyperthermia. Biomaterials 141, 210–222. doi: 10.1016/j.biomaterials.2017.06.026
Alvarez-Erviti, L., Seow, Y., Yin, H., Betts, C., Lakhal, S., and Wood, M. J. A. (2011). Delivery of siRNA to the mouse brain by systemic injection of targeted exosomes. Nat. Biotechnol. 29, 341–345. doi: 10.1038/nbt.1807
Andhari, S. S., Wavhale, R. D., Dhobale, K. D., Tawade, B. V., Chate, G. P., Patil, Y. N., et al. (2020). Self-propelling targeted magneto-Nanobots for deep tumor penetration and pH-responsive intracellular drug delivery. Sci. Rep. 10:4703. doi: 10.1038/s41598-020-61586-y
Anraku, Y., Kuwahara, H., Fukusato, Y., Mizoguchi, A., Ishii, T., Nitta, K., et al. (2017). Glycaemic control boosts glucosylated nanocarrier crossing the BBB into the brain. Nat. Commun. 8:1001. doi: 10.1038/s41467-017-00952-3
Balasubramanian, S. K., Jittiwat, J., Manikandan, J., Ong, C. N., Yu, L. E., and Ong, W. Y. (2010). Biodistribution of gold nanoparticles and gene expression changes in the liver and spleen after intravenous administration in rats. Biomaterials 31, 2034–2042. doi: 10.1016/j.biomaterials.2009.11.079
Bartus, P. E. R. T., Elliott, P., Hayward, N., Dean, R., McEwen, E. L., and Fisher, S. K. (1996). Permeability of the blood brain barrier by the bradykinin agonist, RMP-7: evidence for a sensitive, auto-regulated, receptor-mediated system. Immunopharmacology 33, 270–278. doi: 10.1016/0162-3109(96)00070-7
Bertrand, J., Winton, M. J., Rodriguez-Hernandez, N., Campenot, R. B., and McKerracher, L. (2005). Application of rho antagonist to neuronal cell bodies promotes neurite growth in compartmented cultures and regeneration of retinal ganglion cell axons in the optic nerve of adult rats. J. Neurosci. 25, 1113–1121. doi: 10.1523/JNEUROSCI.3931-04.2005
Black, K. L. (1995). Biochemical opening of the blood-brain barrier. Adv. Drug Deliv. Rev. 15, 37–52. doi: 10.1016/0169-409X(95)00004-Q
Bose, R. J. C., Tharmalingam, N., Choi, Y., Madheswaran, T., Paulmurugan, R., McCarthy, J. R., et al. (2020). Combating intracellular pathogens with Nanohybrid-facilitated antibiotic delivery. Int. J. Nanomedicine 15, 8437–8449. doi: 10.2147/IJN.S271850
Burgess, A., Shah, K., Hough, O., and Hynynen, K. (2015). Focused ultrasound-mediated drug delivery through the blood-brain barrier. Expert. Rev. Neurother. 15, 477–491. doi: 10.1586/14737175.2015.1028369
Burks, S. R., Kersch, C. N., Witko, J. A., Pagel, M. A., Sundby, M., Muldoon, L. L., et al. (2021). Blood-brain barrier opening by intracarotid artery hyperosmolar mannitol induces sterile inflammatory and innate immune responses. Proc. Natl. Acad. Sci. U. S. A. 118: e2021915118. doi: 10.1073/pnas.2021915118
Campbell, F., Bos, F. L., Sieber, S., Arias-Alpizar, G., Koch, B. E., Huwyler, J., et al. (2018). Directing nanoparticle biodistribution through evasion and exploitation of Stab2-dependent nanoparticle uptake. ACS Nano 12, 2138–2150. doi: 10.1021/acsnano.7b06995
Castellini, C., Ruggeri, S., Mattioli, S., Bernardini, G., Macchioni, L., Moretti, E., et al. (2014). Long-term effects of silver nanoparticles on reproductive activity of rabbit buck. Syst Biol Reprod Med 60, 143–150. doi: 10.3109/19396368.2014.891163
Chacko, A. M., Li, C., Pryma, D. A., Brem, S., Coukos, G., and Muzykantov, V. (2013). Targeted delivery of antibody-based therapeutic and imaging agents to CNS tumors: crossing the blood-brain barrier divide. Expert Opin. Drug Deliv. 10, 907–926. doi: 10.1517/17425247.2013.808184
Chauhan, A. S. (2018). Dendrimers for Drug Delivery. Molecules 23:938. doi: 10.3390/molecules23040938
Chen, C., Lee, I., Tatsui, C., Elder, T., and Sloan, A. E. (2021). Laser interstitial thermotherapy (LITT) for the treatment of tumors of the brain and spine: a brief review. J. Neuro-Oncol. 151, 429–442. doi: 10.1007/s11060-020-03652-z
Chen, Z., Zhou, D., Zhou, S., and Jia, G. (2019). Gender difference in hepatic toxicity of titanium dioxide nanoparticles after subchronic oral exposure in Sprague-Dawley rats. J. Appl. Toxicol. 39, 807–819. doi: 10.1002/jat.3769
Cheng, C. A., Deng, T., Lin, F. C., Cai, Y., and Zink, J. I. (2019). Supramolecular Nanomachines as stimuli-responsive gatekeepers on Mesoporous silica nanoparticles for antibiotic and Cancer drug delivery. Theranostics 9, 3341–3364. doi: 10.7150/thno.34576
Cheng, J., Gu, Y. J., Cheng, S. H., and Wong, W. T. (2013). Surface functionalized gold nanoparticles for drug delivery. J. Biomed. Nanotechnol. 9, 1362–1369. doi: 10.1166/jbn.2013.1536
Chengyan Chu, G. L., Janowski, M., Bulte, J. W. M., Li, S., Pearl, M., and Walczak, P. (2018). Real-time MRI guidance for reproducible hyperosmolar opening of the blood-brain barrier in mice. Front. Neurol.
Chorny, M., Fishbein, I., Forbes, S., and Alferiev, I. (2011). Magnetic nanoparticles for targeted vascular delivery. IUBMB Life 63, 613–620. doi: 10.1002/iub.479
Cianfarani, F., Toietta, G., Di Rocco, G., Cesareo, E., Zambruno, G., and Odorisio, T. (2013). Diabetes impairs adipose tissue-derived stem cell function and efficiency in promoting wound healing. Wound Repair Regen. 21, 545–553. doi: 10.1111/wrr.12051
Cosolo, W. C., Martinello, P., Louis, W. J., and Christophidis, N. (1989). Blood-brain barrier disruption using mannitol: time course and electron microscopy studies. Am. J. Phys. Regul. Integr. Comp. Phys. 256, R443–R447. doi: 10.1152/ajpregu.1989.256.2.R443
Cui, B., and Cho, S. W. (2022). Blood-brain barrier-on-a-chip for brain disease modeling and drug testing. BMB Rep. 55, 213–219. doi: 10.5483/BMBRep.2022.55.5.043
Cyr, M., Lepage, Y., Blais, C. Jr., Gervais, N., Cugno, M., Rouleau, J. L., et al. (2001). Bradykinin and des-Arg(9)-bradykinin metabolic pathways and kinetics of activation of human plasma. Am. J. Physiol. Heart Circ. Physiol. 281, H275–H283. doi: 10.1152/ajpheart.2001.281.1.H275
Daneman, R., and Prat, A. (2015). The blood-brain barrier. Cold Spring Harb. Perspect. Biol. 7:a020412. doi: 10.1101/cshperspect.a020412
Daroff, J. J., Mazziotta, J. C., and Pomeroy, S. L. (2016). “Management of neurological disease” in Bradley’s Neurology in Clinical Practice. eds. J. J. D. RB, J. C. Mazziotta, and S. K. Pomeroy (Philadelphia, PA: Elsevier)
Dauer, W., and Przedborski, S. (2003). Parkinson's disease. Neuron 39, 889–909. doi: 10.1016/S0896-6273(03)00568-3
De Vincentiis, S., Falconieri, A., Mickoleit, F., Cappello, V., Schuler, D., and Raffa, V. (2021). Induction of axonal outgrowth in mouse hippocampal neurons via bacterial Magnetosomes. Int. J. Mol. Sci. 22:4126. doi: 10.3390/ijms22084126
Dhar, S., Liu, Z., Thomale, J., Dai, H., and Lippard, S. J. (2008). Targeted single-wall carbon nanotube-mediated Pt(IV) prodrug delivery using folate as a homing device. J. Am. Chem. Soc. 130, 11467–11476. doi: 10.1021/ja803036e
Dong, X. (2018). Current strategies for brain drug delivery. Theranostics 8, 1481–1493. doi: 10.7150/thno.21254
Dorman, H. R., Sondheimer, J. H., and Cadnapaphornchai, P. (1990). Mannitol-induced acute renal failure. Medicine (Baltimore) 69, 153–159. doi: 10.1097/00005792-199005000-00003
Elmore, S. (2007). Apoptosis: a review of programmed cell death. Toxicol. Pathol. 35, 495–516. doi: 10.1080/01926230701320337
Elsharkasy, O. M., Nordin, J. Z., Hagey, D. W., de Jong, O. G., Schiffelers, R. M., Andaloussi, S. E., et al. (2020). Extracellular vesicles as drug delivery systems: why and how? Adv. Drug Deliv. Rev. 159, 332–343. doi: 10.1016/j.addr.2020.04.004
Erdlenbruch, B., Alipour, M., Fricker, G., Miller, D. S., Kugler, W., Eibl, H., et al. (2003). Alkylglycerol opening of the blood-brain barrier to small and large fluorescence markers in normal and C6 glioma-bearing rats and isolated rat brain capillaries. Br. J. Pharmacol. 140, 1201–1210. doi: 10.1038/sj.bjp.0705554
Erdlenbruch, B., Kugler, W., Schinkhof, C., Neurath, H., Eibl, H., and Lakomek, M. (2005). Blood-brain barrier opening with alkylglycerols: biodistribution of 1-O-pentylglycerol after intravenous and intracarotid administration in rats. J. Drug Target. 13, 143–150. doi: 10.1080/10611860400029085
Fan, Y., Chen, Z., and Zhang, M. (2022). Role of exosomes in the pathogenesis, diagnosis, and treatment of central nervous system diseases. J. Transl. Med. 20:291. doi: 10.1186/s12967-022-03493-6
Fan, T.-Y., Zhang, L., Chen, W., Liu, Y., He, M., Huang, X., et al. (2012). Feasibility of MRI-guided high intensity focused ultrasound treatment for adenomyosis. Eur. J. Radiol. 81, 3624–3630. doi: 10.1016/j.ejrad.2011.05.036
Ferreira Soares, D. C., Domingues, S. C., Viana, D. B., and Tebaldi, M. L. (2020). Polymer-hybrid nanoparticles: current advances in biomedical applications. Biomed. Pharmacother. 131:110695. doi: 10.1016/j.biopha.2020.110695
Folio, D., and Ferreira, A. (2017). Two-dimensional robust magnetic resonance navigation of a ferromagnetic microrobot using Pareto optimality. IEEE Trans. Robot. 33, 583–593. doi: 10.1109/TRO.2016.2638446
Forbes, N., Hussain, M. T., Briuglia, M. L., Edwards, D. P., Horst, J. H. T., Szita, N., et al. (2019). Rapid and scale-independent microfluidic manufacture of liposomes entrapping protein incorporating in-line purification and at-line size monitoring. Int. J. Pharm. 556, 68–81. doi: 10.1016/j.ijpharm.2018.11.060
Ganz, R. A. (2017). A modern magnetic implant for Gastroesophageal reflux disease. Clin. Gastroenterol. Hepatol. 15, 1326–1337. doi: 10.1016/j.cgh.2016.12.019
George, S., Pokhrel, S., Xia, T., Gilbert, B., Ji, Z., Schowalter, M., et al. (2010). Use of a rapid cytotoxicity screening approach to engineer a safer zinc oxide nanoparticle through iron doping. ACS Nano 4, 15–29. doi: 10.1021/nn901503q
Ghosh, A., Liu, Y., Artemov, D., and Gracias, D. H. (2021). Magnetic resonance guided navigation of untethered microgrippers. Adv. Healthc. Mater. 10:2000869. doi: 10.1002/adhm.202000869
Gu, X., Song, Q., Zhang, Q., Huang, M., Zheng, M., Chen, J., et al. (2020). Clearance of two organic nanoparticles from the brain via the paravascular pathway. J. Control. Release 322, 31–41. doi: 10.1016/j.jconrel.2020.03.009
Guimarães, D., Cavaco-Paulo, A., and Nogueira, E. (2021). Design of liposomes as drug delivery system for therapeutic applications. Int. J. Pharm. 601:120571. doi: 10.1016/j.ijpharm.2021.120571
Hartl, N., Adams, F., and Merkel, O. M. (2021). From adsorption to covalent bonding: Apolipoprotein E functionalization of polymeric nanoparticles for drug delivery across the blood-brain barrier. Adv. Ther. 4:2000092. doi: 10.1002/adtp.202000092
Hendricks, B. K., Cohen-Gadol, A. A., and Miller, J. C. (2015). Novel delivery methods bypassing the blood-brain and blood-tumor barriers. Neurosurg. Focus. 38:E10. doi: 10.3171/2015.1.FOCUS14767
Hojun Choi, K. C., Kim, D.-H., Byung-Koo, O., Yim, H., Jo, S., and Choi, C. (2022). Strategies for targeted delivery of Exosomes to the brain: advantages and challenges. Pharmaceutics.
Howard, M. D., Hood, E. D., Zern, B., Shuvaev, V. V., Grosser, T., and Muzykantov, V. R. (2014). Nanocarriers for vascular delivery of anti-inflammatory agents. Annu. Rev. Pharmacol. 54, 205–226. doi: 10.1146/annurev-pharmtox-011613-140002
Hu, C. M., Kaushal, S., Tran Cao, H. S., Aryal, S., Sartor, M., and Esener, S. (2010). Half-antibody functionalized lipid-polymer hybrid nanoparticles for targeted drug delivery to carcinoembryonic antigen presenting pancreatic cancer cells. Mol. Pharm.
Huang, C., Chen, X., Xue, Z., and Wang, T. (2020). Effect of structure: a new insight into nanoparticle assemblies from inanimate to animate. Sci. Adv. 6:eaba1321. doi: 10.1126/sciadv.aba1321
Hülper, P., Veszelka, S., Walter, F. R., Wolburg, H., Fallier-Becker, P., Piontek, J., et al. (2013). Acute effects of short-chain alkylglycerols on blood-brain barrier properties of cultured brain endothelial cells. Br. J. Pharmacol. 169, 1561–1573. doi: 10.1111/bph.12218
Huttunen, H. J., and Saarma, M. (2019). CDNF protein therapy in Parkinson's disease. Cell Transplant. 28, 349–366. doi: 10.1177/0963689719840290
Hwang, C. H. (2020). Targeted delivery of erythropoietin hybridized with magnetic Nanocarriers for the treatment of central nervous system injury: a literature review. Int. J. Nanomedicine 15, 9683–9701. doi: 10.2147/IJN.S287456
Ibegbu, D. M., Boussahel, A., Cragg, S. M., Tsibouklis, J., and Barbu, E. (2017). Nanoparticles of alkylglyceryl dextran and poly(ethyl cyanoacrylate) for applications in drug delivery: preparation and characterization. Int. J. Polym. Mater. Polym. Biomater. 66, 265–279. doi: 10.1080/00914037.2016.1201827
Jang, S. C., Kim, O. Y., Yoon, C. M., Choi, D. S., Roh, T. Y., Park, J., et al. (2013). Bioinspired exosome-mimetic nanovesicles for targeted delivery of chemotherapeutics to malignant tumors. ACS Nano 7, 7698–7710. doi: 10.1021/nn402232g
Joniová, J., Kazemiraad, C., Gerelli, E., and Wagnières, G. (2021). Stimulation and homogenization of the protoporphyrin IX endogenous production by photobiomodulation to increase the potency of photodynamic therapy. J. Photochem. Photobiol. B Biol. 225:112347. doi: 10.1016/j.jphotobiol.2021.112347
Kafa, H., Wang, J. T.-W., Rubio, N., Venner, K., Anderson, G., Pach, E., et al. (2015). The interaction of carbon nanotubes with an in vitro blood-brain barrier model and mouse brain in vivo. Biomaterials 53, 437–452. doi: 10.1016/j.biomaterials.2015.02.083
Kasinathan, N., Volety, S. M., and Josyula, V. R. (2016). Chondroitinase: a promising therapeutic enzyme. Crit. Rev. Microbiol. 42, 474–484.
Khan, M. M., Madni, A., Tahir, N., Parveen, F., Khan, S., Jan, N., et al. (2020). Co-delivery of Curcumin and Cisplatin to enhance cytotoxicity of Cisplatin using lipid-chitosan hybrid nanoparticles. Int. J. Nanomedicine 15, 2207–2217. doi: 10.2147/IJN.S247893
Kim, Y. S., Kim, J. S., Cho, H. S., Rha, D. S., Kim, J. M., Park, J. D., et al. (2008). Twenty-eight-day oral toxicity, genotoxicity, and gender-related tissue distribution of silver nanoparticles in Sprague-Dawley rats. Inhal. Toxicol. 20, 575–583. doi: 10.1080/08958370701874663
Kostarelos, K., Lacerda, L., Partidos, C. D., Prato, M., and Bianco, A. (2005). Carbon nanotube-mediated delivery of peptides and genes to cells: translating nanobiotechnology to therapeutics. J. Drug Deliv. Sci. Technol. 15, 41–47. doi: 10.1016/S1773-2247(05)50005-4
Kou, L., Hou, Y., Yao, Q., Guo, W., Wang, G., Wang, M., et al. (2018). L-Carnitine-conjugated nanoparticles to promote permeation across blood-brain barrier and to target glioma cells for drug delivery via the novel organic cation/carnitine transporter OCTN2. Artif. Cells Nanomed. Biotechnol. 46, 1605–1616.
Lan, M., Zhao, S., Liu, W., Lee, C. S., Zhang, W., and Wang, P. (2019). Photosensitizers for photodynamic therapy. Adv. Healthc. Mater. 8:1900132. doi: 10.1002/adhm.201900132
Lee, H., Kim, H., Han, H., Lee, M., Lee, S., Yoo, H., et al. (2017). Microbubbles used for contrast enhanced ultrasound and theragnosis: a review of principles to applications. Biomed. Eng. Lett. 7, 59–69. doi: 10.1007/s13534-017-0016-5
Lee, I. C., Ko, J. W., Park, S. H., Lim, J. O., Shin, I. S., Moon, C., et al. (2016). Comparative toxicity and biodistribution of copper nanoparticles and cupric ions in rats. Int. J. Nanomedicine 11, 2883–2900. doi: 10.2147/IJN.S106346
Leuthardt, E. C., Duan, C., Kim, M. J., Campian, J. L., Kim, A. H., Miller-Thomas, M. M., et al. (2016). Hyperthermic laser ablation of recurrent Glioblastoma leads to temporary disruption of the Peritumoral blood brain barrier. PLoS One 11:e0148613. doi: 10.1371/journal.pone.0148613
Li, L., He, D., Guo, Q., Zhang, Z., Ru, D., Wang, L., et al. (2022). Exosome-liposome hybrid nanoparticle codelivery of TP and miR497 conspicuously overcomes chemoresistant ovarian cancer. J Nanobiotechnol 20:50. doi: 10.1186/s12951-022-01264-5
Li, N., Jiang, Y., Plantefève, R., Michaud, F., Nosrati, Z., Tremblay, C., et al. (2019). Magnetic resonance navigation for targeted embolization in a two-level bifurcation phantom. Ann. Biomed. Eng. 47, 2402–2415. doi: 10.1007/s10439-019-02317-x
Li, M., Liao, L., and Tian, W. (2020). Extracellular vesicles derived from apoptotic cells: an essential link between death and regeneration. Front. Cell Dev. Biol. 8:573511. doi: 10.3389/fcell.2020.573511
Liu, W., Bai, X., Zhang, A., Huang, J., Xu, S., and Zhang, J. (2019). Role of Exosomes in central nervous system diseases. Front. Mol. Neurosci. 12:240. doi: 10.3389/fnmol.2019.00240
Liu, M. L., Williams, K. J., and Werth, V. P. (2016). Chapter four—Microvesicles in autoimmune diseases. Advances in Clinical Chemistry. 125–175. doi: 10.1016/bs.acc.2016.06.005
Lueshen, E., Venugopal, I., Soni, T., Alaraj, A., and Linninger, A. (2015). Implant-assisted Intrathecal magnetic drug targeting to aid in therapeutic nanoparticle localization for potential treatment of central nervous system disorders. J. Biomed. Nanotechnol. 11, 253–261. doi: 10.1166/jbn.2015.1907
Lv, Q., Cheng, L., Lu, Y., Zhang, X., Wang, Y., Deng, J., et al. (2020). Thermosensitive exosome-liposome hybrid nanoparticle-mediated Chemoimmunotherapy for improved treatment of metastatic peritoneal Cancer. Adv Sci 7:2000515. doi: 10.1002/advs.202000515
Macdougall, I. C., Strauss, W. E., Dahl, N. V., Bernard, K., and Li, Z. (2019). Ferumoxytol for iron deficiency anemia in patients undergoing hemodialysis. Clin. Nephrol. 91, 237–245. doi: 10.5414/CN109512
Madani, S. Y., Naderi, N., Dissanayake, O., Tan, A., and Seifalian, A. M. (2011). A new era of cancer treatment: carbon nanotubes as drug delivery tools. Int. J. Nanomedicine 6, 2963–2979. doi: 10.2147/IJN.S16923
Marcuello, C., Chambel, L., Rodrigues, M. S., Ferreira, L. P., and Cruz, M. M. (2018). Magnetotactic Bacteria: magnetism beyond Magnetosomes. IEEE Trans. Nanobiosci. 17, 555–559. doi: 10.1109/TNB.2018.2878085
Marie, C. V., Pauwels, J., and Vandenbroucke, R. E. (2021). Special delEVery: extracellular vesicles as promising delivery platform to the brain. Biomedicine
Martel, S. (2010). Microrobotic navigable entities for magnetic resonance targeting. Annu. Int. Conf. IEEE Eng. Med. Biol. Soc. 2010, 1942–1945.
Melnick, K., Shin, D., Dastmalchi, F., Kabeer, Z., Rahman, M., Tran, D., et al. (2021). Role of laser interstitial thermal therapy in the Management of Primary and Metastatic Brain Tumors. Curr. Treat. Options in Oncol. 22:108. doi: 10.1007/s11864-021-00912-6
Meng, Y., Suppiah, S., Surendrakumar, S., Bigioni, L., and Lipsman, N. (2018). Low-intensity MR-guided focused ultrasound mediated disruption of the blood-brain barrier for intracranial metastatic diseases. Front. Oncol. 8:338. doi: 10.3389/fonc.2018.00338
Mitchell, M. J., Billingsley, M. M., Haley, R. M., Wechsler, M. E., Peppas, N. A., and Langer, R. (2021). Engineering precision nanoparticles for drug delivery. Nat. Rev. Drug Discov. 20, 101–124. doi: 10.1038/s41573-020-0090-8
Mittal, D., Ali, A., Md, S., Baboota, S., Sahni, J. K., and Ali, J. (2014). Insights into direct nose to brain delivery: current status and future perspective. Drug Deliv. 21, 75–86. doi: 10.3109/10717544.2013.838713
Mohammadpour, R., Dobrovolskaia, M. A., Cheney, D. L., Greish, K. F., and Ghandehari, H. (2019). Subchronic and chronic toxicity evaluation of inorganic nanoparticles for delivery applications. Adv. Drug Deliv. Rev. 144, 112–132. doi: 10.1016/j.addr.2019.07.006
Moro, C., Massri, N. E., Torres, N., Ratel, D., De Jaeger, X., Chabrol, C., et al. (2014). Photobiomodulation inside the brain: a novel method of applying near-infrared light intracranially and its impact on dopaminergic cell survival in MPTP-treated mice. J. Neurosurg. 120, 670–683. doi: 10.3171/2013.9.JNS13423
Mullen, D. G., and Banaszak Holl, M. M. (2011). Heterogeneous ligand-nanoparticle distributions: a major obstacle to scientific understanding and commercial translation. Acc. Chem. Res. 44, 1135–1145. doi: 10.1021/ar1001389
Nacev, A., Weinberg, I. N., Stepanov, P. Y., Kupfer, S., Mair, L. O., Urdaneta, M. G., et al. (2015). Dynamic inversion enables external magnets to concentrate ferromagnetic rods to a central target. Nano Lett. 15, 359–364. doi: 10.1021/nl503654t
Nan, X., Teng, Y., Tian, J., Hu, Z., and Fang, Q. (2021). A comprehensive assessment of the biocompatibility of Magnetospirillum gryphiswaldense MSR-1 bacterial magnetosomes in vitro and in vivo. Toxicology 462:152949. doi: 10.1016/j.tox.2021.152949
Nederveen, J. P., Warnier, G., Di Carlo, A., Nilsson, M. I., and Tarnopolsky, M. A. (2021). Extracellular vesicles and Exosomes: insights from exercise science. Front. Physiol. 11:604274. doi: 10.3389/fphys.2020.604274
Negri, L. B., Martins, T. J., da Silva, R. S., and Hamblin, M. R. (2019). Photobiomodulation combined with photodynamic therapy using ruthenium phthalocyanine complexes in A375 melanoma cells: effects of nitric oxide generation and ATP production. J. Photochem. Photobiol. B Biol. 198:111564. doi: 10.1016/j.jphotobiol.2019.111564
Nemt-Allah, A. A., Ibrahim, S. H., and El-Zoghby, A. F. (2021). Marginal integrity of composite restoration with and without surface pretreatment by gold and silver nanoparticles vs Chlorhexidine: a randomized controlled trial. J. Contemp. Dent. Pract. 22, 1087–1097. doi: 10.5005/jp-journals-10024-3200
Owens, D. E. 3rd, and Peppas, N. A. (2006). Opsonization, biodistribution, and pharmacokinetics of polymeric nanoparticles. Int. J. Pharm. 307, 93–102. doi: 10.1016/j.ijpharm.2005.10.010
Palmerston Mendes, L., Pan, J., and Torchilin, V. P. (2017). Dendrimers as Nanocarriers for nucleic acid and drug delivery in Cancer therapy. Molecules 22:1401. doi: 10.3390/molecules22091401
Pande, M., Tyagi, S., Kumar, S., Soni, Y. K., Chand, N., Sirohi, A. S., et al. (2022). Effects of unconjugated gold, silver and titanium dioxide nanoparticles on bovine spermatozoa at various stages of cryopreservation. Cryo-Letters 43, 150–157. doi: 10.54680/fr22310110512
Pardridge, W. M. (2012). Drug transport across the blood-brain barrier. J. Cereb. Blood Flow Metab. 32, 1959–1972. doi: 10.1038/jcbfm.2012.126
Pashirova, T. N., Zueva, I. V., Petrov, K. A., Babaev, V. M., Lukashenko, S. S., Rizvanov, I. K., et al. (2017). Nanoparticle-delivered 2-PAM for rat brain protection against Paraoxon central toxicity. ACS Appl. Mater. Interfaces 9, 16922–16932. doi: 10.1021/acsami.7b04163
Patel, B., Yang, P. H., and Kim, A. H. (2020). The effect of thermal therapy on the blood-brain barrier and blood-tumor barrier. Int. J. Hyperth. 37, 35–43. doi: 10.1080/02656736.2020.1783461
Pentek, T., Newenhouse, E., O'Brien, B., and Chauhan, A. S. (2017). Development of a topical resveratrol formulation for commercial applications using Dendrimer nanotechnology. Molecules 22:137. doi: 10.3390/molecules22010137
Perez-Herrero, E., and Fernandez-Medarde, A. (2015). Advanced targeted therapies in cancer: drug nanocarriers, the future of chemotherapy. Eur. J. Pharm. Biopharm. 93, 52–79. doi: 10.1016/j.ejpb.2015.03.018
Pouliopoulos, A. N., Kwon, N., Jensen, G., Meaney, A., Niimi, Y., Burgess, M. T., et al. (2021). Safety evaluation of a clinical focused ultrasound system for neuronavigation guided blood-brain barrier opening in non-human primates. Sci. Rep. 11:15043. doi: 10.1038/s41598-021-94188-3
Pouponneau, P., Bringout, G., and Martel, S. (2014). Therapeutic magnetic microcarriers guided by magnetic resonance navigation for enhanced liver chemoembilization: a design review. Ann. Biomed. Eng. 42, 929–939. doi: 10.1007/s10439-014-0972-1
Qiao, L., Qin, Y., Wang, Y., Liang, Y., Zhu, D., Xiong, W., et al. (2020). A brain glioma gene delivery strategy by angiopep-2 and TAT-modified magnetic lipid-polymer hybrid nanoparticles. RSC Adv. 10, 41471–41481. doi: 10.1039/D0RA07161G
Raymond, J., Robertson, D., and Dinsdale, H. (1986). Pharmacological modification of Bradykinin induced breakdown of the blood-brain barrier. Can. J. Neurol. Sci. 13, 214–220. doi: 10.1017/S0317167100036301
Roces, C. B., Port, E. C., Daskalakis, N. N., Watts, J. A., Aylott, J. W., Halbert, G. W., et al. (2020). Rapid scale-up and production of active-loaded PEGylated liposomes. Int. J. Pharm. 586:119566. doi: 10.1016/j.ijpharm.2020.119566
Rodríguez-Massó, S. R., Erickson, M. A., Banks, W. A., Ulrich, H., and Martins, A. H. (2021). The Bradykinin B2 receptor agonist (NG291) causes rapid onset of transient blood-brain barrier disruption without evidence of early brain injury. Front. Neurosci. 15:791709. doi: 10.3389/fnins.2021.791709
Rufino-Ramos, D., Albuquerque, P. R., Carmona, V., Perfeito, R., Nobre, R. J., and Pereira de Almeida, L. (2017). Extracellular vesicles: novel promising delivery systems for therapy of brain diseases. J. Control. Release 262, 247–258. doi: 10.1016/j.jconrel.2017.07.001
Salem, U., Kumar, V. A., Madewell, J. E., Schomer, D. F., de Almeida Bastos, D. C., Zinn, P. O., et al. (2019). Neurosurgical applications of MRI guided laser interstitial thermal therapy (LITT). Cancer Imaging 19:65. doi: 10.1186/s40644-019-0250-4
Samiotaki, G., Acosta, C., Wang, S., and Konofagou, E. E. (2015). Enhanced delivery and bioactivity of the Neurturin Neurotrophic factor through focused ultrasound—mediated blood—brain barrier Openingin vivo. J. Cereb. Blood Flow Metab. 35, 611–622. doi: 10.1038/jcbfm.2014.236
Santavanond, J. P., Rutter, S. F., Atkin-Smith, G. K., and Poon, I. K. H. (2021). Apoptotic bodies: mechanism of formation, isolation and functional relevance. Subcell. Biochem. 97, 61–88. doi: 10.1007/978-3-030-67171-6_4
Sela, H., Cohen, H., Elia, P., Zach, R., Karpas, Z., and Zeiri, Y. (2015). Spontaneous penetration of gold nanoparticles through the blood brain barrier (BBB). J. Nanobiotechnol. 13:71. doi: 10.1186/s12951-015-0133-1
Semyachkina-Glushkovskaya, O., Borisova, E., Mantareva, V., Angelov, I., Eneva, I., Terskov, A., et al. (2020). Photodynamic opening of the blood–brain barrier using different photosensitizers in mice. Appl. Sci. 10:33. doi: 10.3390/app10010033
Seven, E. S., Seven, Y. B., Zhou, Y., Poudel-Sharma, S., Diaz-Rucco, J. J., Kirbas Cilingir, E., et al. (2021). Crossing the blood–brain barrier with carbon dots: uptake mechanism andin vivocargo delivery. Nanoscale Adv. 3, 3942–3953. doi: 10.1039/D1NA00145K
Shah, S., Dhawan, V., Holm, R., Nagarsenker, M. S., and Perrie, Y. (2020). Liposomes: advancements and innovation in the manufacturing process. Adv. Drug Deliv. Rev. 154-155, 102–122. doi: 10.1016/j.addr.2020.07.002
Shao, H., Im, H., Castro, C. M., Breakefield, X., Weissleder, R., and Lee, H. (2018). New Technologies for Analysis of extracellular vesicles. Chem. Rev. 118, 1917–1950. doi: 10.1021/acs.chemrev.7b00534
Soares Martins, T., Marçalo, R., da Cruz e Silva, C. B., Trindade, D., Catita, J., Amado, F., et al. (2022). Novel exosome biomarker candidates for Alzheimer’s disease Unravelled through mass spectrometry analysis. Mol. Neurobiol. 59, 2838–2854. doi: 10.1007/s12035-022-02762-1
Spuch, C., and Navarro, C. (2011). Liposomes for targeted delivery of active agents against neurodegenerative diseases (Alzheimer's disease and Parkinson's disease). J. Drug. Deliv. 2011:469679
Sun, K., Zheng, X., Jin, H., Yu, F., and Zhao, W. (2022). Exosomes as CNS drug delivery tools and their applications. Pharmaceutics 14:2252. doi: 10.3390/pharmaceutics14102252
Szabová, J., Mišík, O., Havlíková, M., Lízal, F., and Mravec, F. (2021). Influence of liposomes composition on their stability during the nebulization process by vibrating mesh nebulizer. Colloids Surf. B: Biointerfaces 204:111793. doi: 10.1016/j.colsurfb.2021.111793
Tao, J., Fei, W., Tang, H., Li, C., Mu, C., Zheng, H., et al. (2019). Angiopep-2-conjugated "Core-Shell" hybrid Nanovehicles for targeted and pH-triggered delivery of arsenic trioxide into Glioma. Mol. Pharm. 16, 786–797. doi: 10.1021/acs.molpharmaceut.8b01056
Tentillier, N., Etzerodt, A., Olesen, M. N., Rizalar, F. S., Jacobsen, J., Bender, D., et al. (2016). Anti-inflammatory modulation of microglia via CD163-targeted glucocorticoids protects dopaminergic neurons in the 6-OHDA Parkinson's disease model. J. Neurosci. 36, 9375–9390. doi: 10.1523/JNEUROSCI.1636-16.2016
Tewabe, A., Abate, A., Tamrie, M., Seyfu, A., and Abdela Siraj, E. (2021). Targeted drug delivery—from magic bullet to Nanomedicine: principles, challenges, and future perspectives. J. Multidiscip. Healthc. 14, 1711–1724. doi: 10.2147/JMDH.S313968
Tiebosch, I. A., Crielaard, B. J., Bouts, M. J., Zwartbol, R., Salas-Perdomo, A., Lammers, T., et al. (2012). Combined treatment with recombinant tissue plasminogen activator and dexamethasone phosphate-containing liposomes improves neurological outcome and restricts lesion progression after embolic stroke in rats. J. Neurochem. 123, 65–74. doi: 10.1111/j.1471-4159.2012.07945.x
Tietze, R., Lyer, S., Dürr, S., Struffert, T., Engelhorn, T., Schwarz, M., et al. (2013). Efficient drug-delivery using magnetic nanoparticles--biodistribution and therapeutic effects in tumour bearing rabbits. Nanomedicine 9, 961–971. doi: 10.1016/j.nano.2013.05.001
Timbie, K. F., Mead, B. P., and Price, R. J. (2015). Drug and gene delivery across the blood-brain barrier with focused ultrasound. J. Control. Release 219, 61–75. doi: 10.1016/j.jconrel.2015.08.059
Tsai, Y.-C., Vijayaraghavan, P., Chiang, W.-H., Chen, H.-H., Liu, T.-I., Shen, M.-Y., et al. (2018). Targeted delivery of functionalized Upconversion nanoparticles for externally triggered Photothermal/photodynamic therapies of brain Glioblastoma. Theranostics 8, 1435–1448. doi: 10.7150/thno.22482
Vanden-Hehir, S., Tipping, W. J., Lee, M., Brunton, V. G., Williams, A., and Hulme, A. N. (2019). Raman imaging of Nanocarriers for drug delivery. Nano 9:341. doi: 10.3390/nano9030341
Visweswaran, P., Massin, E. K., and Dubose, T. D. Jr. (1997). Mannitol-induced acute renal failure. J. Am. Soc. Nephrol. 8, 1028–1033. doi: 10.1681/ASN.V861028
Vonarbourg, A., Passirani, C., Saulnier, P., and Benoit, J. P. (2006). Parameters influencing the stealthiness of colloidal drug delivery systems. Biomaterials 27, 4356–4373. doi: 10.1016/j.biomaterials.2006.03.039
Voronin, D. V., Sindeeva, O. A., Kurochkin, M. A., Mayorova, O., Fedosov, I. V., Semyachkina-Glushkovskaya, O., et al. (2017). In vitro and in vivo visualization and trapping of fluorescent magnetic microcapsules in a bloodstream. ACS Appl. Mater. Interfaces 9, 6885–6893. doi: 10.1021/acsami.6b15811
Votteler, J., Ogohara, C., Yi, S., Hsia, Y., Nattermann, U., Belnap, D. M., et al. (2016). Designed proteins induce the formation of nanocage-containing extracellular vesicles. Nature 540, 292–295. doi: 10.1038/nature20607
Wahl, M., Görlach, C., Hortobágyi, T., and Benyó, Z. (1999). Effects of bradykinin in the cerebral circulation. Acta Physiol. Hung. 86, 155–160.
Walkey, C. D., Olsen, J. B., Guo, H., Emili, A., and Chan, W. C. W. (2012). Nanoparticle size and surface chemistry determine serum protein adsorption and macrophage uptake. J. Am. Chem. Soc. 134, 2139–2147. doi: 10.1021/ja2084338
Wang, H., Abdussalam, A., and Xu, G. (2022). The role of doping strategy in nanoparticle-based electrochemiluminescence biosensing. Bioelectrochemistry 148:108249. doi: 10.1016/j.bioelechem.2022.108249
Wu, W., Li, R., Bian, X., Zhu, Z., Ding, D., Li, X., et al. (2009). Covalently combining carbon nanotubes with anticancer agent: preparation and antitumor activity. ACS Nano 3, 2740–2750. doi: 10.1021/nn9005686
Xie, J., Shen, Z., Anraku, Y., Kataoka, K., and Chen, X. (2019). Nanomaterial-based blood-brain-barrier (BBB) crossing strategies. Biomaterials 224:119491. doi: 10.1016/j.biomaterials.2019.119491
Yang, Q., Zhou, Y., Chen, J., Huang, N., Wang, Z., and Cheng, Y. (2021). Gene therapy for drug-resistant Glioblastoma via lipid-polymer hybrid nanoparticles combined with focused ultrasound. Int. J. Nanomedicine 16, 185–199. doi: 10.2147/IJN.S286221
Yang, J., Zou, X., Jose, P. A., and Zeng, C. (2021). “Chapter two—extracellular vesicles: potential impact on cardiovascular diseases” in Advances in Clinical Chemistry. ed. G. S. Makowski (Dovepress: Elsevier), 49–100.
Yao, Y., Zhou, Y., Liu, L., Xu, Y., Chen, Q., Wang, Y., et al. (2020). Nanoparticle-based drug delivery in cancer therapy and its role in overcoming drug resistance. Front. Mol. Biosci. 7:193. doi: 10.3389/fmolb.2020.00193
Yu, M. K., Park, J., and Jon, S. (2012). Targeting strategies for multifunctional nanoparticles in cancer imaging and therapy. Theranostics 2, 3–44. doi: 10.7150/thno.3463
Yu, H., Tang, W., Mu, G., Wang, H., Chang, X., Dong, H., et al. (2018). Micro-/Nanorobots propelled by oscillating magnetic fields. Micromachines 9:540. doi: 10.3390/mi9110540
Zhang, C., Chen, W., Zhang, T., Jiang, X., and Hu, Y. (2020). Hybrid nanoparticle composites applied to photodynamic therapy: strategies and applications. J. Mater. Chem. B 8, 4726–4737. doi: 10.1039/D0TB00093K
Zhou, L., Kodidela, S., Godse, S., Thomas-Gooch, S., Kumar, A., Raji, B., et al. (2022). Targeted drug delivery to the central nervous system using extracellular vesicles. Pharmaceuticals (Basel) 15:358. doi: 10.3390/ph15030358
Zhu, M., Sun, Z., and Ng, C. K. (2017). Image-guided thermal ablation with MR-based thermometry. Quant. Imaging Med. Surg. 7, 356–368. doi: 10.21037/qims.2017.06.06
Zibly, Z., Graves, C. A., Harnof, S., Hadani, M., and Cohen, Z. R. (2014). Sonoablation and application of MRI guided focused ultrasound in a preclinical model. J. Clin. Neurosci. 21, 1808–1814. doi: 10.1016/j.jocn.2014.04.008
Zörner, B., and Schwab, M. E. (2010). Anti-Nogo on the go: from animal models to a clinical trial. Ann. N. Y. Acad. Sci. 1198, E22–E34. doi: 10.1111/j.1749-6632.2010.05566.x
Keywords: therapeutics, central nervous system, wounds and injuries, targeted delivery, nanoparticles, exosomes, magnetics
Citation: Won S, An J, Song H, Im S, You G, Lee S, Koo K-i and Hwang CH (2023) Transnasal targeted delivery of therapeutics in central nervous system diseases: a narrative review. Front. Neurosci. 17:1137096. doi: 10.3389/fnins.2023.1137096
Edited by:
Na Li, Central South University, ChinaReviewed by:
Santosh Bashyal, The University of Texas at Austin, United StatesRupinder Kaur Sodhi, Panjab University, India
Copyright © 2023 Won, An, Song, Im, You, Lee, Koo and Hwang. This is an open-access article distributed under the terms of the Creative Commons Attribution License (CC BY). The use, distribution or reproduction in other forums is permitted, provided the original author(s) and the copyright owner(s) are credited and that the original publication in this journal is cited, in accordance with accepted academic practice. No use, distribution or reproduction is permitted which does not comply with these terms.
*Correspondence: Kyo-in Koo, a2lrb29AdWxzYW4uYWMua3I=; Chang Ho Hwang, Y2hod2FuZzEyMjBAY251LmFjLmty
†These authors have contributed equally to this work and share first authorship
‡ORCID: Kyo-in Koo https://orcid.org/0000-0003-4173-9218
Chang Ho Hwang https://orcid.org/0000-0003-0444-3602