- Department of Neurology, Division of Sleep Medicine, Beth Israel Deaconess Medical Center, Harvard Medical School, Boston, MA, United States
Opioids provide analgesia, as well as modulate sleep and respiration, all by possibly acting on the μ-opioid receptors (MOR). MOR’s are ubiquitously present throughout the brain, posing a challenge for understanding the precise anatomical substrates that mediate opioid induced respiratory depression (OIRD) that ultimately kills most users. Sleep is a major modulator not only of pain perception, but also for changing the efficacy of opioids as analgesics. Therefore, sleep disturbances are major risk factors for developing opioid overuse, withdrawal, poor treatment response for pain, and addiction relapse. Despite challenges to resolve the neural substrates of respiratory malfunctions during opioid overdose, two main areas, the pre-Bötzinger complex (preBötC) in the medulla and the parabrachial (PB) complex have been implicated in regulating respiratory depression. More recent studies suggest that it is mediation by the PB that causes OIRD. The PB also act as a major node in the upper brain stem that not only receives input from the chemosensory areas in medulla, but also receives nociceptive information from spinal cord. We have previously shown that the PB neurons play an important role in mediating arousal from sleep in response to hypercapnia by its projections to the forebrain arousal centers, and it may also act as a major relay for the pain stimuli. However, due to heterogeneity of cells in the PB, their precise roles in regulating, sleep, analgesia, and respiratory depression, needs addressing. This review sheds light on interactions between sleep and pain, along with dissecting the elements that adversely affects respiration.
Introduction
Physical pain is a guaranteed experience of just about any existence, yet opioids, one of the most effective treatments for pain, kills about 220 people per day in the United States (Centers for Disease Control Prevention [CDC], 2022). Initially declared an epidemic by the president in 2017, the opioid crisis has only gotten worse since then with opioid-related overdose deaths increasing by 44% in the following 3 years (National Institutes of Health [NIH], 2015). This is no surprise given that 1 in 5 people in the US, and globally, suffer from chronic pain (Goldberg and McGee, 2011; Yong et al., 2022). Used for thousands of years for pain and sedation, opioids first became commonly prescribed after new standards and regulations of pain management were established by the United States in 2000. Combined with incentives from the pharmaceutical manufacturing companies, and a lack of suitable alternatives, opioid prescribing quickly turned into over-prescribing (Jones et al., 2018). Significant research has since looked at opioids and the effect of prolonged use, finding that 1 in 4 patients receiving long term opioid treatment become addicted (Centers for Disease Control Prevention [CDC], 2017). Most commonly, long term users develop an opioid use disorder (OUD), which is a chronic relapsing disorder caused by intense cravings, increased opioid tolerance, and avoidance of withdrawal symptoms (Strang et al., 2020; Dydyk et al., 2022). The addictive quality of opioids have been attributed to the feeling of euphoria, in addition to analgesia, that the user experiences (White and Irvine, 1999). Over 16 million people worldwide, including 2.1 million in the US, suffer from an OUD of which 20% are estimated to eventually die from an overdose (Dydyk et al., 2022). Respiratory depression, specifically a decrease in respiratory rate and tidal volume, is ultimately what causes fatality from opioids (White and Irvine, 1999; Dahan et al., 2001; Pattinson, 2008). Overdose fatalities are common because, with repeated use, chronic opioid users gradually increase their dosage amounts (opioid tolerance) to achieve the same level of pain relief, slowly approaching amounts (White and Irvine, 1999) that depress all phases of respiratory activity (rate, minute volume, and tidal exchange) and produce irregular breathings (Pattinson, 2008; Dahan et al., 2010; Ramirez et al., 2021). Opioid analgesics including fentanyl depress respiration primarily by reducing the responsiveness of brain−stem respiratory centers to carbon dioxide (CO2) (Kirby and McQueen, 1986), therefore, opioids are also well-known to be associated with increased incidence of sleep-disordered breathing (SDB) pathology (Webster et al., 2008; Correa et al., 2015). Due to this, the infants less than 6 months old, opioid−naïve patients, the elderly, and those who have coexisting conditions such as chronic pulmonary disease and major organ failure, or are receiving other central nervous system (CNS) depressants, are some of the sub-populations that are at greater risk of opioid induced respiratory depression (OIRD).
In addition to respiratory depression, opioids also increase cardiovascular events, sleep disorders, clinical depression, hyperalgesia, risk of bone fractures, hormone dysregulation, immunosuppression, constipation, sedation, and dizziness among others (Baldini et al., 2012). In fact, sleep disruption (SD) occurs even in acute administration of opioids in healthy individuals, and contributes not only to the development of opioid dependence, but also relapse (Tripathi et al., 2020). A side effect with its own serious complications, sleep apnea is commonly experienced by opioid users and increases patients’ risk of coronary artery disease, heart attacks, heart failure, and strokes (Guilleminault et al., 2010; Schwarzer et al., 2015). On the contrary, treating OUD is in itself an effective treatment for sleep apnea, demonstrating the high interconnectivity of the opioid-induced analgesia and respiration pathways (Schwarzer et al., 2015). Further understanding of the bidirectional relationships shared between opioids, sleep, and respiration will help develop targeted therapies for pain management.
Opioid receptors–their role in analgesia and respiration
Opioids act on opioid receptors, which are g-couple protein receptors (GPCRs) and are located throughout the body, both in the peripheral and central nervous system. There are four different opioid receptors that are structurally and functionally different. The delta (δ) opioid receptor, located mostly in the brain and mesenteric plexus, is responsible for spinal and supra-spinal analgesia, motor integration, thermoregulation (Mansour et al., 1988; Al-Hasani and Bruchas, 2011; Dietis et al., 2011). The kappa (κ) opioid receptor, in, spinal cord, and mesenteric plexus, produces analgesia, diuresis, food intake, neuroendocrine function and (Mansour et al., 1988; Al-Hasani and Bruchas, 2011; Dietis et al., 2011). The nociceptin opioid receptor (NOP), located in the spinal cord, depending on the concentration of opioids administered causes analgesia, hyperalgesia, and allodynia. Finally, the mu (μ) opioid receptor (MOR) derived from the Oprm1 gene, is located throughout the brain, spinal cord, mesenteric plexus, and submucosal plexus (Dahan et al., 2001; Dietis et al., 2011). The MOR is responsible for analgesia, sedation, respiratory depression, bradycardia, nausea, vomiting, and reduction in gastric motility. MORs alone are responsible for both pain and respiratory effects of opioids, which are the most adverse and clinically relevant effects of opioids for prescribing them as analgesics (Dahan et al., 2001). Other receptor subtypes show minimal effects on pain or respiration in the absence of MOR, therefore, this review will focus exclusively on the MOR (Meunier et al., 1995; Matthes et al., 1996).
Opioid alternatives–blocking adverse effects of MOR agonists on respiration
Therapies that rescue respiratory depression following opioid administration are currently being investigated. Similar to Naloxone, the widely known non-specific opioid antagonist used to quickly reverse opioid overdose, non-opioid treatments like the potassium channel blocker (GAL021), ampakine (CX717), or 5-HT4(a) receptor antagonists have been shown to rescue opioid-induced respiratory depression, but not without affecting analgesia (Manzke et al., 2003; Oertel et al., 2010; Roozekrans et al., 2014). Though interventions are important to treat opioid overdose, alternative pain management therapies would be far more beneficial as they could replace opioids altogether. Novel GPCRs that primarily activate the G protein pathway with limited arrestin recruitment, as β-arrestin recruitment by the MOR appears to contribute to some of the unwanted effects of classical opioids (Mores et al., 2019), and therefore, these ligands are particularly interesting as potential targets for pain therapy. For instance, G-protein-biased μ-opioid receptor (MOR) activation using the ligands like TRV130, PZM21, and SR17018, may provide analgesia without the associated side effects of opioids including respiratory depression (Viscusi et al., 2016; Dahan et al., 2018; Elango et al., 2021). Additionally, use of poly-pharmacological ligands, which affect multiple receptor types that act on several different pain receptors or pathways, which could eventually summate to the same analgesic strength of opioids, without affecting respiration and sleep (Pasquinucci et al., 2021) is also promising. However, to accurately predict poly-pharmacology would also require high level of data curation, integration, and methodology development from various drug delivery disciplines. Peripherally restricted analgesics that target only peripheral opioid receptors, but not those in the central nervous system, could provide sufficient analgesia (Che and Roth, 2021) without the potential adverse effects. While these therapies seem promising, the lack of effective alternatives so far to opioids demonstrate the continued importance of further dissecting different elements of the pain and respiration pathways that are so much interwoven.
Sleep loss/deprivation as a modulator of the opioid mediated analgesia
Numerous studies have investigated the impact of experimental sleep disturbances on pain perception (Moldofsky, 1995; Iaboni and Moldofsky, 2008; Finan et al., 2013; Schrimpf et al., 2015), and despite highly heterogeneous SD protocols, the overall results point to an increase in pain responses and behaviors when the sleep is disturbed in otherwise healthy humans, rats, or mice. Studies in rodents show that sleep loss either in the form of total sleep (Alexandre et al., 2020) or rapid eye movement (REM) sleep loss (Nascimento et al., 2007; Skinner et al., 2011) not only increases pain perception, but also decreases the analgesic efficacy of opioids. Furthermore, sleep apnea which is commonly experienced by opioid users, that also results in fragmented sleep, and may be an additional risk factor in developing opioid tolerance, OUD and susceptibility to relapse (Doufas et al., 2013; Jaoude et al., 2016; Ardon et al., 2020).
The loss of opioid efficacy in sleep-deprived individuals represents a potential major risk at the clinical level that could likely accelerate the development of tolerance, leading to dose escalation, and the risk of dependence or overdose. Interestingly, administration of the non-selective COX1/2 inhibitor ibuprofen failed to prevent both mechanical and heat hypersensitivity induced by 9 h of total sleep deprivation in mice and rats (Wodarski et al., 2015; Alexandre et al., 2020). This was reversed by caffeine and modafinil, two wake-promoting agents that have no analgesic activity in rested mice, but immediately normalize pain sensitivity in sleep-deprived animals, without affecting sleep debt (Alexandre et al., 2017). A similar study in rats also confirms an unexpected role for alertness in setting hyperalgesia (Hambrecht-Wiedbusch et al., 2017). Also studies in humans show that the increase in prostaglandin production, especially PGE2 is the potential mediator in sleep-loss induced changes in nociceptive processing (Haack et al., 2009; Simpson et al., 2020), and sleep loss produces an apparent loss of drug efficacy when compared to well-rested individuals. Therefore, there is an urgent need to identify the brain circuits that are primarily affected by sleep deprivation (e.g., over-activated wake circuits), which could be targeted to alter the efficacy of MORs in providing pain relief without producing opioid tolerance.
Anatomical substrates for opioid induced respiratory depression (OIRD) and analgesia
To understand how pain and respiration pathways are interconnected, significant research has been done to determine which brain structures are important in facilitating respiration and, therefore, are most important in mediating OIRD. Both the analgesic and respiratory effects of opioid signaling are mediated by μ receptor 1 (Oprm1), which are inhibitory (Ramirez et al., 2021). The abundance of Oprm1 expression in the majority of the respiratory control areas of the brainstem has made it more challenging to resolve the neural substrates of respiratory malfunctions during overdose. Two main areas, the pre-Bötzinger complex (preBötC) in the ventral medulla and the PB complex in the upper brain stem area (Stucke et al., 2015; Miller et al., 2017; Varga et al., 2020) are implicated in regulating OIRD. The medullary ventral respiratory group (VRG) (Onimaru and Homma, 2006) that regulates inspiration and respiratory rhythmogenesis (preBötC) receives descending inputs from various areas including the PB and Kölliker Fuśe (KF) nuclei which exert significant additional regulatory control (Wang et al., 1993). Among the medullary VRG, only the preBötC is considered critical for depressive action of opioids (Mellen et al., 2003; Del Negro et al., 2018; Sun et al., 2019; Norris et al., 2021). In fact, additional research has shown that while the preBötC is responsible for respiratory rhythm generation, it is the PB, specifically, that has a modulatory effect, especially during OIRD (Eguchi et al., 1987; Bachmutsky et al., 2020). Further, MORs located in the PB, not the preBötC or KF, mediate the respiratory response to opioid administration as inhibition of the PB MORs mimic OIRD, while activation reverses it (Lalley et al., 2014; Liu et al., 2021). While the preBötC is important for generating respiration, it is the mediation by the PB that causes opioid-induced respiratory depression, making the PB, a structure of focus to dissect the elements that transmit pain, modulate respiration and sleep.
The PB, located in the rostral hindbrain at the midline of the pons and hindbrain, is split, medially and laterally, by a large fiber tract called the superior cerebellar peduncle (Palmiter, 2018). The PB has diverse neuronal populations that express MORs (PBOprm1) and mediate normal breathing, affected by modified breathing during hypercapnia challenge (Kaur et al., 2017; Kaur and Saper, 2019, 2021) and by the OIRD (Hurlé et al., 1983). MOR-expressing neurons in the PB have been shown to be specifically important in cardiovascular, gustatory, and pain functions, which is understandable given their specific projection patterns to the amygdala, basal forebrain, amygdalo-piriform transition area, hindbrain reticular formation, and cranial motor nuclei (Chamberlin et al., 1999; Wilson et al., 2003; Huang et al., 2021a). Part of the initial difficulty in studying the role of PB MOR-expressing neurons (PBOprm1) is their high levels of expression throughout the PB and that opiates can cause both sedation and wakefulness, depending on the site of action, receptor type, and dosage (De Andrés and Caballero, 1989).
Direct administration of opioid agonists locally into the PB results in suppression of respiratory rate, directly contrasting the results observed following local opioid administration within the VRG, particularly the preBötC (Krause et al., 2009; Mustapic et al., 2010; Stucke et al., 2015). In mice lacking the MOR, morphine-induced decrease in ventilation was abolished, suggesting that MOR is the site of action for respiratory effects of morphine (Dahan et al., 2001). In awake mice, removal of MORs from PB/KF neurons significantly rescues morphine-induced respiratory rate depression (Bachmutsky et al., 2020; Varga et al., 2020). This occurs at a therapeutically relevant analgesic dose and, importantly also at a very high dose that adversely affects respiration. By comparison, removal of MORs from preBötC neurons only rescues morphine-induced rate depression at lower doses, but at high doses further increases the occurrence of apneas (Varga et al., 2020). More evidence for the involvement of PBOprm1 neurons in OIRD pathogenesis comes from a recent study, where it was shown that PBOprm1 neuronal activity is tightly correlated with respiratory rate, and this correlation is abolished following morphine injection (Liu et al., 2021, 2022). Chemogenetic inhibition of PBOprm1 neurons mimics OIRD in mice, whereas their activation following morphine injection rescues respiratory rhythms to baseline levels (Liu et al., 2021). This suggests that PBOprm1 neurons may play a larger role in OIRD (Varga et al., 2020; Liu et al., 2021). However, their activation would inadvertently disrupt sleep and also increase pain sensitivity (Alexandre et al., 2017, 2020), as activation of most PBOprm1 neurons promotes wakefulness (Kaur et al., 2013, 2017; Qiu et al., 2016; Kaur and Saper, 2019). Sleep disruption and increased pain sensitivity are major contributors to the development of increased opioid dependence, overuse and addiction (Hartwell et al., 2014; Koller et al., 2019; Huhn, 2021). Therefore, a better understanding of the PB neuronal subtypes is needed to prevent OIRD while preserving analgesia and sleep.
Opioids also reduce sensitivity to hypercapnic, hypoxic ventilatory responses, by acting on the MORs (Dahan et al., 2001; Pattinson, 2008; Lam et al., 2016) that possibly inhibit the PBOprm1 neurons. Patients with opioid overdose lack hypercapnic arousal responses (Dahan et al., 2001; Pattinson, 2008; Lam et al., 2016). Under normal conditions, cessation of breathing during sleep (apneas) with obstructive sleep apnea (OSA) activates the respiratory chemosensory nuclei in the pons, namely, retrotrapezoid (RTN) and nucleus of solitary tract (NTS) that serve as relay centers for blood gas information that converges onto PBOprm1 neurons, which promote arousal and also provide feed-back to respiratory control centers to increase respiratory drive. Distinct output pathways of the lateral PB neurons (dorsal vs. external lateral) may selectively affect the relay of the noxious input for mediating analgesic and sedative effects of opioids (Chiang et al., 2020). Calcitonin gene related peptide (CGRP) neurons in the external lateral part of PB (PBCGRP) are not only critical for relaying pain signals to the central nucleus of amygdala (CeA) (Neugebauer and Weidong, 2002; Han et al., 2015; Chen et al., 2017; Bowen et al., 2020) but this pathway may also transduce affective waking and cortical arousal in response to hypercapnia (Kaur et al., 2013, 2017; Qiu et al., 2016; Kaur and Saper, 2019).
Heterogeneity in the PB
The neuronal subtypes in the PB are both diverse and intermixed (Geerling et al., 2011, 2016, 2017; Huang et al., 2021a; Yeghiazarians et al., 2021), a difficult combination when trying to determine the function of each in distinct sensory pathway. Prior research has relied on structural or location identifiers to distinguish different cell populations, which is now replaced by the use of the precise genetic markers that accurately identify the pivotal structure. Transcription factors like, Lmxb1 and Atoh1 mark two distinct developmental macro-populations (Karthik et al., 2022) in the PB, where the Lmxb1 population contains gene markers for FoxP2, Calca and Sat2b, while the Atoh1 contains pro-dynorphin (pdyn), g-protein coupled receptor (GPR) and FoxP2, and are located more ventrally (Karthik et al., 2022). Of those, Calca (encoding CGRP) has been linked to control over pain and hypercapnia induced arousal (PBCGRP). Fork head box protein transcription factor (FoxP2) expressing neurons (PBFoxP2) are located ventrally to that of the Atoh1 population, and are possibly associated with hypercapnia induced increase in respiration or with insufficient respiration as in OSA (Kaur and Saper, 2019, 2021; Karthik et al., 2022).
Advanced molecular tools provide more directed approach to dissect selective cell types. Use of targeted viral vectors (cre-dependent) and optogenetics, that specifically act on selective neuronal subtype and allow us to manipulate (activate or inhibit) them, while recording the animals for respiration, electroencephalogram/electromyography (EEG/EMG) signals, allows us to objectively investigate role of each cell types in pain, sleep and hypercapnia induced arousal (Kaur et al., 2013, 2017; Chiang et al., 2019, 2020).
The PB contains a population of glutamatergic neurons that are part of a chemosensory relay circuit projecting to forebrain arousal centers (Kaur et al., 2013; Yokota et al., 2015; Qiu et al., 2016; Saper and Kaur, 2018; Chiang et al., 2019). Calca-expressing neurons in the PBel (PBCGRP), which have the densest MORs expression in the PB (Wolinsky et al., 1996; Chamberlin et al., 1999; Miller et al., 2017; Huang et al., 2021b) regulate both pain-induced arousal (Kuner and Kuner, 2021) and respiration (Kaur et al., 2013, 2017; Qiu et al., 2016; Kaur and Saper, 2019). Thus opioid-induced inhibition of these neurons likely contributes to the sedative effects of opioids and can possibly explain their interconnected role in regulating pain, sleep and respiratory depression to opioids. Stimulation of PBelCGRP neurons affect cortical arousal, while their inhibition decreased hypercapnic arousal responses without affecting the ventilatory drive (Kaur et al., 2013, 2017, 2020; Kaur and Saper, 2019, 2021). In addition, the neurons located in the dorsal PB and caudal to the KF (that are FoxP2 or pdyn positive) also express Oprm1 (Chamberlin et al., 1999; Huang et al., 2021a). Our preliminary studies with manipulating and recording neuronal activity of the PBFoxP2 neurons shows that they are activated in response to hypercapnia, and further that their activity correlates with breathing and inhibiting these neurons reduces the minute ventilation in response to hypercapnia, suggests that PBFoxP2 neurons could likely mediate OIRD. Hypercapnia also activates a subset of FoxP2 neurons in the centro-lateral that express pro-dynorphin (PBpdyn). Thus, differential expression of Oprm1 on these three types of PB neurons (Huang et al., 2021a,b; Karthik et al., 2022) may contribute to the differential response to opioids, critical in defining respiratory depression and analgesic effects (Figure 1). Genetic silencing of PBCGRP neurons also block pain responses and memory formation, whereas their optogenetic stimulation produces hyperalgesia aversive memory (Chen et al., 2017; Campos et al., 2018; Sun et al., 2020). PBpdyn expressing neurons in the dorsal nucleus of the PB regulate body temperature (Norris et al., 2021) and also provide cellular substrate for transmission of nociceptive information to the PBCGRP efferent (Chiang et al., 2019, 2020), which is important in pain processing.
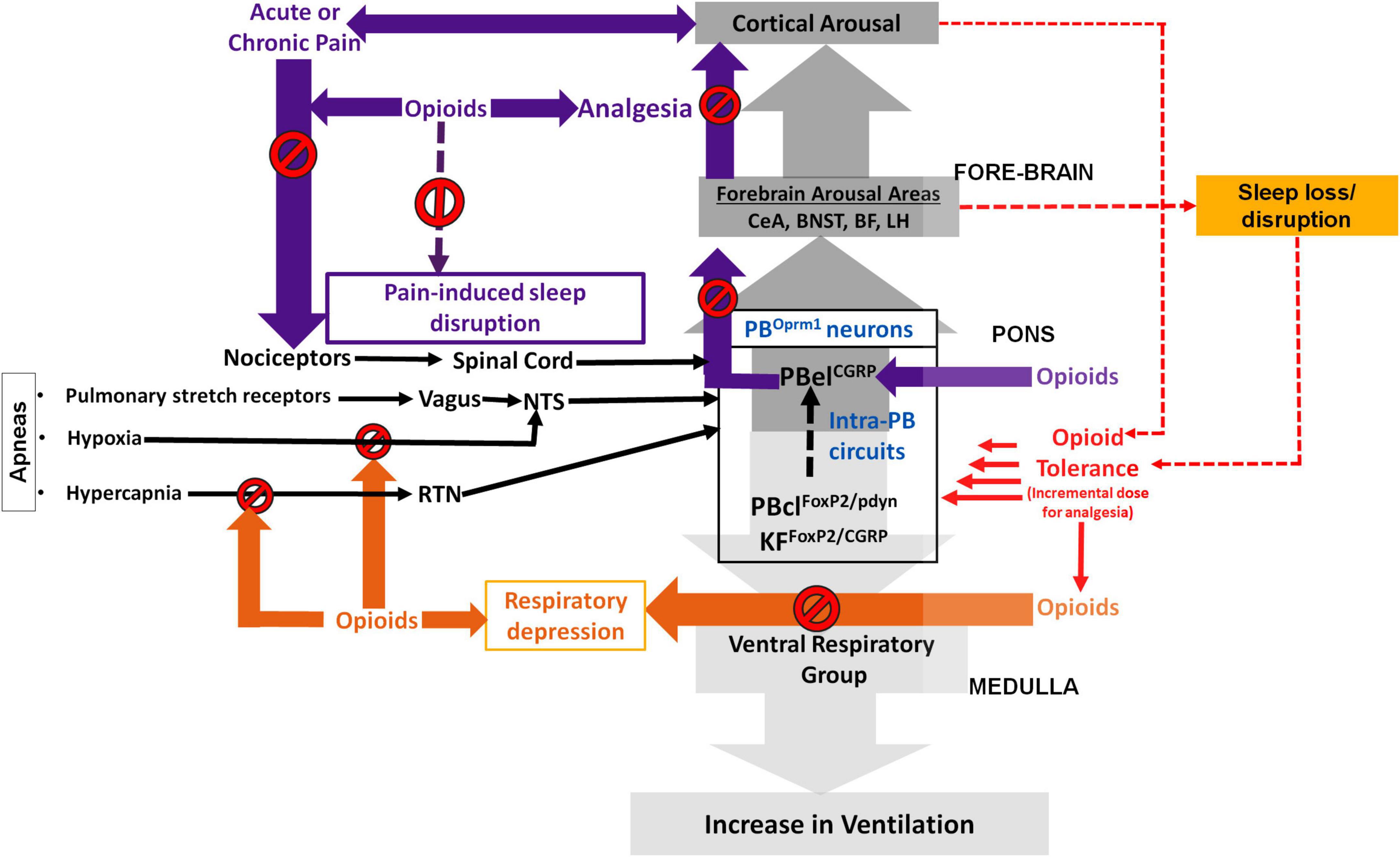
Figure 1. Schematic showing the possible role of the parabrachial μ-opioid receptor (MOR) expressing neurons (PBOprm1) in regulating opioid induced respiratory depression (OIRD), analgesia, and sleep: The neurons in the centro lateral sub-nucleus of parabrachial area (PBcl) and Kölliker Fuśe (KF) that express FoxP2 (PBclFoxP2) and those that express pro-dynorphin (PBclpdyn) may critically modulate respiration through their descending projections to the medullary ventral respiratory group (VRG). Opioids at incrementally higher dose (due to developing opioid tolerance) may act by inhibiting the PBclFoxP2/PBclpdyn neurons resulting in a continuous cycle of progressively depressed respiration that can prove to be fatal. Both hypercapnia and hypoxia stimuli (during apneic events) are conveyed to the PB via the retrotrapezoid (RTN) and nucleus of solitary tract (NTS), and these pathways are also inhibited by opioids use and tolerance. The interconnectivity of the PBOprm1 neurons, specifically between the PBclFoxP2/PBclpdyn and PBelCGRP subpopulations may explain cortical arousal that results from respiratory stress during sleep apnea. The PB neurons that express CGRP (PBelCGRP) regulate waking up in response to pain, hypercapnia and aversive stimuli through their projections to the forebrain arousal areas such as, central nucleus of amygdala (CeA); the bed nucleus of stria terminalis (BNST); the basal forebrain (BF) and the lateral hypothalamus (LH). Opioids may provide analgesia and prevent sleep disruption (SD) by inhibiting the PBelCGRP neurons that act as relay node for the pain stimulus which is transmitted to these neurons via the spinal cord. In contrast, inadequate sleep over-activate this cortical arousal circuit, inclusive of the PBelCGRP neurons, which causes decreased sensitivity to inhibition from opioids, also known as opioid tolerance. Sleep disruption, in itself, also cause increased pain sensitivity, with decreasing levels of opioid induced analgesia. The resulting continuous cycle accelerates opioid tolerance while progressively reducing opioid analgesia.
Insomnia, pain induced sleep loss and apnea induced sleep fragmentation may cause over activation of the arousal circuits, that includes all the wake-active PB neurons (Figure 1) and their projection targets, which are usually inhibited by opioids to produce potent analgesia (Jasmin et al., 1994; Pieh et al., 2011; Hartwell et al., 2014; Jaoude et al., 2016; Bertz et al., 2019). In addition, opioids also enhance the inhibition of descending pain-modulating pathways contributing to anti-nociception (Millan, 2002; Lueptow et al., 2018). Sleep-loss alters these pathways as well altering the nociceptive processing, contributing to the development of opioid tolerance. Higher opioid doses further exacerbates sleep disturbances (O’Brien et al., 2021) increasing the risk for the associated respiratory depression.
With further increase in opioid-related deaths during the COVID-19 pandemic, it’s clear that the opioid crisis continues to be a significant health concern for unforeseeable future. Since sleep, respiration, and pain are so interconnected, pain-induced arousal and opioid-induced respiratory depression pathways possibly intersect at the PB, making this particularly vital to dissect its heterogeneous cell population and its role in regulating pain, respiration, sleep as well as their interaction with one another. This will also provide insight into the additive effects of alcohol use disorder, which may employ the same neuronal pathway, as is evidenced by growing research on the use of MOR antagonists to treat alcoholism (Drobes et al., 2003; Ripley et al., 2015; Ben Hamida et al., 2019). As opioids and alcohol both cause systemic depression, largely acting on MOR and GABA, respectively, to mediate inhibition (Chamberlin et al., 1999; Morrow et al., 2001; Ben Hamida et al., 2019). Frequent users of both alcohol and opioids also experience chronic pain and hyperalgesia which is further exacerbated by use of these substances together (Witkiewitz and Vowles, 2018).
More studies are required targeted at characterizing the role of different PB neuronal subsets in mediating the OIRD, which will help design better therapeutics that will prevent not only respiratory depression, but will also help spare opioid induced analgesia and prevent pain induced SD and fragmentation. Alternatively, given the bidirectional relationship between pain and sleep, treating the disturbed sleep may also be the key to preventing opioid overuse, withdrawal, poor treatment response for pain and addiction relapse.
Author contributions
NL: conceptualization, research, first draft, and editing. JL and RS: research support and editing. SK: conceptualization, research, supervision, and editing. All authors contributed to the article and approved the submitted version.
Funding
This work was supported by NIH/NINDS-NS112175 grant awarded to SK.
Conflict of interest
The authors declare that the research was conducted in the absence of any commercial or financial relationships that could be construed as a potential conflict of interest.
Publisher’s note
All claims expressed in this article are solely those of the authors and do not necessarily represent those of their affiliated organizations, or those of the publisher, the editors and the reviewers. Any product that may be evaluated in this article, or claim that may be made by its manufacturer, is not guaranteed or endorsed by the publisher.
References
Alexandre, C., Latremolier, A., and Finan, P. (2020). “Effect of sleep loss on pain,” in The Oxford Handbook of the Neurobiology of Pain, ed. J. N. Wood (Oxford: Oxford University Press), 556–608.
Alexandre, C., Latremoliere, A., Ferreira, A., Miracca, G., Yamamoto, M., Scammell, T. E., et al. (2017). Decreased alertness due to sleep loss increases pain sensitivity in mice. Nat. Med. 23, 768–774. doi: 10.1038/nm.4329
Al-Hasani, R., and Bruchas, M. R. (2011). Molecular mechanisms of opioid receptor-dependent signaling and behavior. Anesthesiology 115, 1363–1381.
Ardon, A., Gillespie, N., Kolli, S., Shilling, A. M., and Warrick, M. (2020). Patient-controlled analgesia in high-risk populations: Implications for safety. Curr. Anesthesiol. Rep. 10, 463–472.
Bachmutsky, I., Wei, X. P., Kish, E., and Yackle, K. (2020). Opioids depress breathing through two small brainstem sites. Elife 9:e52694. doi: 10.7554/eLife.52694
Baldini, A., Von Korff, M., and Lin, E. H. (2012). A review of potential adverse effects of long-term opioid therapy: A practitioner’s guide. Prim. Care Compan. J Clin. Psychiatry 14:PCC.11m01326.
Ben Hamida, S., Boulos, L. J., McNicholas, M., Charbogne, P., and Kieffer, B. L. (2019). Mu opioid receptors in GABAergic neurons of the forebrain promote alcohol reward and drinking. Addict. Biol. 24, 28–39. doi: 10.1111/adb.12576
Bertz, J. W., Epstein, D. H., Reamer, D., Kowalczyk, W. J., Phillips, K. A., Kennedy, A. P., et al. (2019). Sleep reductions associated with illicit opioid use and clinic-hour changes during opioid agonist treatment for opioid dependence: Measurement by electronic diary and actigraphy. J. Subst. Abuse Treat. 106, 43–57. doi: 10.1016/j.jsat.2019.08.011
Bowen, A. J., Chen, J. Y., Huang, Y. W., Baertsch, N. A., Park, S., and Palmiter, R. D. (2020). Dissociable control of unconditioned responses and associative fear learning by parabrachial CGRP neurons. Elife 9:e59799. doi: 10.7554/eLife.59799
Campos, C. A., Bowen, A. J., Roman, C. W., and Palmiter, R. D. (2018). Encoding of danger by parabrachial CGRP neurons. Nature 555, 617–620.
Centers for Disease Control and Prevention [CDC] (2017). Prescription Opioids | Opioids | CDC. Available online at: https://www.cdc.gov/opioids/basics/prescribed.html (accessed December 18, 2022).
Centers for Disease Control and Prevention [CDC] (2022). National Center for Health Statistics, Office of Communication (301) 458-4800. U.S. Overdose Deaths In 2021 Increased Half as Much as in 2020 – But Are Still Up 15%. Hyattsville, ND: National Center for Health Statistics.
Chamberlin, N. L., Mansour, A., Watson, S. J., and Saper, C. B. (1999). Localization of mu-opioid receptors on amygdaloid projection neurons in the parabrachial nucleus of the rat. Brain Res. 827, 198–204. doi: 10.1016/s0006-8993(99)01168-3
Che, T., and Roth, B. L. (2021). Structural insights accelerate the discovery of opioid alternatives. Annu. Rev. Biochem. 90, 739–761. doi: 10.1146/annurev-biochem-061620-044044
Chen, Q., Roeder, Z., Li, M. H., Zhang, Y., Ingram, S. L., and Heinricher, M. M. (2017). Optogenetic evidence for a direct circuit linking nociceptive transmission through the parabrachial complex with pain-modulating neurons of the rostral ventromedial medulla (RVM). eNeuro 4:ENEURO.0202–17.2017. doi: 10.1523/ENEURO.0202-17.2017
Chiang, M. C., Bowen, A., Schier, L. A., Tupone, D., Uddin, O., and Heinricher, M. M. (2019). Parabrachial complex: A hub for pain and aversion. J. Neurosci. 39, 8225–8230. doi: 10.1523/JNEUROSCI.1162-19.2019
Chiang, M. C., Nguyen, E. K., Canto-Bustos, M., Papale, A. E., Oswald, A. M., and Ross, S. E. (2020). Divergent neural pathways emanating from the lateral parabrachial nucleus mediate distinct components of the pain response. Neuron 106, 927.e5–939.e5. doi: 10.1016/j.neuron.2020.03.014
Correa, D., Farney, R. J., Chung, F., Prasad, A., Lam, D., and Wong, J. (2015). Chronic opioid use and central sleep apnea. Anesth. Analg. 120, 1273–1285.
Dahan, A., Aarts, L., and Smith, T. W. (2010). Incidence, reversal, and prevention of opioid-induced respiratory depression. Anesthesiology 112, 226–238.
Dahan, A., Sarton, E., Teppema, L., Olievier, C., Nieuwenhuijs, D., Matthes, H. W., et al. (2001). Anesthetic potency and influence of morphine and sevoflurane on respiration in μ-opioid receptor knockout mice. Anesthesiology 94, 824–832. doi: 10.1097/00000542-200105000-00021
Dahan, A., van der Schrier, R., Smith, T., Aarts, L., van Velzen, M., and Niesters, M. (2018). Averting opioid-induced respiratory depression without affecting analgesia. Anesthesiology 128, 1027–1037. doi: 10.1097/ALN.0000000000002184
De Andrés, I., and Caballero, A. (1989). Chronic morphine administration in cats: Effects on sleep and EEG. Pharmacol. Biochem. Behav. 32, 519–526.
Del Negro, C. A., Funk, G. D., and Feldman, J. L. (2018). Breathing matters. Nat. Rev. Neurosci. 19, 351–367.
Dietis, N., Rowbotham, D. J., and Lambert, D. G. (2011). Opioid receptor subtypes: Fact or artifact? Br. J. Anaesthesia 107, 8–18.
Doufas, A. G., Tian, L., Padrez, K. A., Suwanprathes, P., Cardell, J. A., Maecker, H. T., et al. (2013). Experimental pain and opioid analgesia in volunteers at high risk for obstructive sleep apnea. PLoS One 8:e54807. doi: 10.1371/journal.pone.0054807
Drobes, D. J., Anton, R. F., Thomas, S. E., and Voronin, K. (2003). A clinical laboratory paradigm for evaluating medication effects on alcohol consumption: Naltrexone and nalmefene. Neuropsychopharmacol. 28, 755–764. doi: 10.1038/sj.npp.1300101
Dydyk, A. M., Jain, N. K., and Gupta, M. (2022). Opioid Use Disorder. Treasure Island, FL: StatPearls Publishing.
Eguchi, K., Tadaki, E., Simbulan, D., and Kumazawa, T. (1987). Respiratory depression caused by either morphine microinjection or repetitive electrical stimulation in the region of the nucleus parabrachialis of cats. Pflügers Arch. Eur. J. Physiol. 409, 367–373.
Elango, D., Malathi, D. C., and Palanisamy, P. R. (2021). Oliceridine - Breakthrough in the management of pain. J. Pharmacol. Pharmacother. 12, 157–162. doi: 10.1358/dot.2020.56.4.3107707
Finan, P. H., Goodin, B. R., and Smith, M. T. (2013). The association of sleep and pain: An update and a path forward. J. Pain 14, 1539–1552. doi: 10.1016/j.jpain.2013.08.007
Geerling, J. C., Kim, M., Mahoney, C. E., Abbott, S. B., Agostinelli, L. J., Garfield, A. S., et al. (2016). Genetic identity of thermosensory relay neurons in the lateral parabrachial nucleus. Am. J. Physiol. Integr. Comp. Physiol. 310, R41–R54. doi: 10.1152/ajpregu.00094.2015
Geerling, J. C., Stein, M. K., Miller, R. L., Shin, J. W., Gray, P. A., and Loewy, A. D. (2011). FoxP2 expression defines dorsolateral pontine neurons activated by sodium deprivation. Brain Res. 1375, 19–27. doi: 10.1016/j.brainres.2010.11.028
Geerling, J. C., Yokota, S., Rukhadze, I., Roe, D., and Chamberlin, N. L. (2017). Kölliker–Fuse GABAergic and glutamatergic neurons project to distinct targets. J. Comp. Neurol. 525, 1844–1860. doi: 10.1002/cne.24164
Goldberg, D. S., and McGee, S. J. (2011). Pain as a global public health priority. BMC Public Health 11:770. doi: 10.1186/1471-2458-11-770
Guilleminault, C., Cao, M., Yue, H. J., and Chawla, P. (2010). Obstructive sleep apnea and chronic opioid use. Lung 188, 459–468. doi: 10.1007/s00408-010-9254-3
Haack, M., Lee, E., Cohen, D. A., and Mullington, J. M. (2009). Activation of the prostaglandin system in response to sleep loss in healthy humans: Potential mediator of increased spontaneous pain. Pain 145, 136–141. doi: 10.1016/j.pain.2009.05.029
Hambrecht-Wiedbusch, V. S., Gabel, M., Liu, L. J., Imperial, J. P., Colmenero, A. V., and Vanini, G. (2017). Preemptive caffeine administration blocks the increase in postoperative pain caused by previous sleep loss in the rat: A potential role for preoptic adenosine A2A receptors in sleep-pain interactions. Sleep 40:zsx116. doi: 10.1093/sleep/zsx116
Han, S., Soleiman, M. T., Soden, M. E., Zweifel, L. S., and Palmiter, R. D. (2015). Elucidating an affective pain circuit that creates a threat memory. Cell 162, 363–374. doi: 10.1016/j.cell.2015.05.057
Hartwell, E. E., Pfeifer, J. G., McCauley, J. L., Moran-Santa Maria, M., and Back, S. E. (2014). Sleep disturbances and pain among individuals with prescription opioid dependence. Addict. Behav. 39, 1537–1542. doi: 10.1016/j.addbeh.2014.05.025
Huang, D., Grady, F. S., Peltekian, L., and Geerling, J. C. (2021a). Efferent projections of Vglut2, Foxp2, and Pdyn parabrachial neurons in mice. J. Comp. Neurol. 529, 657–693. doi: 10.1002/cne.24975
Huang, D., Grady, F. S., Peltekian, L., Laing, J. J., and Geerling, J. C. (2021b). Efferent projections of CGRP/Calca -expressing parabrachial neurons in mice. J. Comp. Neurol. 529, 2911–2957. doi: 10.1002/cne.25136
Huhn, A. (2021). Dual orexin-receptor antagonist effects on sleep, opioid withdrawal, and craving in persons with opioid use disorder. Neuropsychopharmacology 46, 22.
Hurlé, M. A., Mediavilla, A., and Flórez, J. (1983). Participation of pontine structures in the respiratory action of opioids. Life Sci. 33(Suppl. 1) 571–574. doi: 10.1016/0024-3205(83)90567-2
Iaboni, A., and Moldofsky, H. (2008). “Sleep and medical disorders.” in Sleep medicine (Cambridge clinical guides), eds H. Smith, C. Comella, and B. Högl (Cambridge: Cambridge University Press), 186–207.
Jaoude, P., Lal, A., Vermont, L., Porhomayon, J., and El-Solh, A. A. (2016). Pain intensity and opioid utilization in response to CPAP therapy in veterans with obstructive sleep apnea on chronic opioid treatment. J. Clin. Sleep Med. 12, 1105–1111. doi: 10.5664/jcsm.6046
Jasmin, L., Wang, H., Tarczy-Hornoch, K., Levine, J. D., and Basbaum, A. I. (1994). Differential effects of morphine on noxious stimulus-evoked fos-like immunoreactivity in subpopulations of spinoparabrachial neurons. J. Neurosci. 14, 7252–7260. doi: 10.1523/JNEUROSCI.14-12-07252.1994
Jones, M. R., Viswanath, O., Peck, J., Kaye, A. D., Gill, J. S., and Simopoulos, T. T. (2018). A brief history of the opioid epidemic and strategies for pain medicine. Pain and Ther. 7, 13–21.
Karthik, S., Huang, D., Delgado, Y., Laing, J. J., Peltekian, L., Iverson, G. N., et al. (2022). Molecular ontology of the parabrachial nucleus. J. Comp. Neurol. 530, 1658–1699. doi: 10.1002/cne.25307
Kaur, S., De Luca, R., Khanday, M. A., Bandaru, S. S., Thomas, R. C., Broadhurst, R. Y., et al. (2020). Role of serotonergic dorsal raphe neurons in hypercapnia-induced arousals. Nat. Commun. 11, 2769. doi: 10.1038/s41467-020-16518-9
Kaur, S., Pedersen, N. P., Yokota, S., Hur, E. E., Fuller, P. M., Lazarus, M., et al. (2013). Glutamatergic signaling from the parabrachial nucleus plays a critical role in hypercapnic arousal. J. Neurosci. 33, 7627–7640. doi: 10.1523/JNEUROSCI.0173-13.2013
Kaur, S., and Saper, C. (2021). FoxP2 cells in the lateral parabrachial area may drive respiratory responses to hypercapnia. FASEB J. 35:fasebj.2021.35.S1.02023.
Kaur, S., and Saper, C. B. (2019). Neural circuitry underlying waking up to hypercapnia. Front. Neurosci. 13:401. doi: 10.3389/fnins.2019.00401
Kaur, S., Wang, J. L., Ferrari, L., Thankachan, S., Kroeger, D., Venner, A., et al. (2017). A genetically defined circuit for arousal from sleep during hypercapnia. Neuron 96, 1153.e5–1167.e5. doi: 10.1016/j.neuron.2017.10.009
Kirby, G. C., and McQueen, D. S. (1986). Characterization of opioid receptors in the cat carotid body involved in chemosensory depression in vivo. Br. J. Pharmacol. 88, 889–898. doi: 10.1111/j.1476-5381.1986.tb16263.x
Koller, G., Schwarzer, A., Halfter, K., and Soyka, M. (2019). Pain management in opioid maintenance treatment. Expert Opin. Pharmacother. 20, 1993–2005.
Krause, K. L., Neumueller, S. E., Marshall, B. D., Kiner, T., Bonis, J. M., Pan, L. G., et al. (2009). μ-Opioid receptor agonist injections into the presumed pre-Bötzinger complex and the surrounding region of awake goats do not alter eupneic breathing. J. Appl. Physiol. 107, 1591–1599.
Kuner, R., and Kuner, T. (2021). Cellular circuits in the brain and their modulation in acute and chronic pain. Physiol. Rev. 101, 213–258.
Lalley, P. M., Pilowsky, P. M., Forster, H. V., and Zuperku, E. J. (2014). CrossTalk opposing view: The pre-Bötzinger complex is not essential for respiratory depression following systemic administration of opioid analgesics. J. Physiol. 592, 1163–1166. doi: 10.1113/jphysiol.2013.258830
Lam, K. K., Kunder, S., Wong, J., Doufas, A. G., and Chung, F. (2016). Obstructive sleep apnea, pain, and opioids: Is the riddle solved? Curr. Opin. Anaesthesiol. 29, 134–140. doi: 10.1097/ACO.0000000000000265
Liu, S., Kim, D. I., Oh, T. G., Pao, G. M., Kim, J. H., Palmiter, R. D., et al. (2021). Neural basis of opioid-induced respiratory depression and its rescue. Proc. Natl. Acad. Sci. 118, e2022134118. doi: 10.1073/pnas.2022134118
Liu, S., Ye, M., Pao, G. M., Song, S. M., Jhang, J., Jiang, H., et al. (2022). Divergent brainstem opioidergic pathways that coordinate breathing with pain and emotions. Neuron 110, 857.e9–873.e9. doi: 10.1016/j.neuron.2021.11.029
Lueptow, L. M., Fakira, A. K., and Bobeck, E. N. (2018). The contribution of the descending pain modulatory pathway in opioid tolerance. Front. Neurosci. 12:886. doi: 10.3389/fnins.2018.00886
Mansour, A., Khachaturian, H., Lewis, M. E., Akil, H., and Watson, S. J. (1988). Anatomy of CNS opioid receptors. Trends Neurosci. 11, 308–314.
Manzke, T., Guenther, U., Ponimaskin, E. G., Haller, M., Dutschmann, M., Schwarzacher, S., et al. (2003). 5-HT4(a) receptors avert opioid-induced breathing depression without loss of analgesia. Science 301, 226–229. doi: 10.1126/science.1084674
Matthes, H. W., Maldonado, R., Simonin, F., Valverde, O., Slowe, S., Kitchen, I., et al. (1996). Loss of morphine-induced analgesia, reward effect and withdrawal symptoms in mice lacking the mu-opioid-receptor gene. Nature 383, 822–823. doi: 10.1038/383819a0
Mellen, N. M., Janczewski, W. A., Bocchiaro, C. M., and Feldman, J. L. (2003). Opioid-induced quantal slowing reveals dual networks for respiratory rhythm generation. Neuron 37, 821–826. doi: 10.1016/s0896-6273(03)00092-8
Meunier, J. C., Mollereau, C., Toll, L., Suaudeau, C., Moisand, C., Alvinerie, P., et al. (1995). Isolation and structure of the endogenous agonist of opioid receptor-like ORL1 receptor. Nature 377, 532–535.
Miller, J. R., Zuperku, E. J., Stuth, E. A. E., Banerjee, A., Hopp, F. A., and Stucke, A. G. (2017). A subregion of the parabrachial nucleus partially mediates respiratory rate depression from intravenous remifentanil in young and adult rabbits. Anesthesiology 127, 502–514. doi: 10.1097/ALN.0000000000001719
Mores, K. L., Cummins, B. R., Cassell, R. J., and van Rijn, R. M. (2019). A review of the therapeutic potential of recently developed G protein-biased kappa agonists. Front. Pharmacol. 10:407. doi: 10.3389/fphar.2019.00407
Morrow, A. L., VanDoren, M. J., Penland, S. N., and Matthews, D. B. (2001). The role of GABAergic neuroactive steroids in ethanol action, tolerance and dependence. Brain Res. Rev. 37, 98–109.
Mustapic, S., Radocaj, T., Sanchez, A., Dogas, Z., Stucke, A. G., Hopp, F. A., et al. (2010). Clinically relevant infusion rates of μ-opioid agonist remifentanil cause bradypnea in decerebrate dogs but not via direct effects in the pre-bötzinger complex region. J. Neurophysiol. 103, 409–418.
Nascimento, D. C., Andersen, M. L., Hipólide, D. C., Nobrega, J. N., and Tufik, S. (2007). Pain hypersensitivity induced by paradoxical sleep deprivation is not due to altered binding to brain μ-opioid receptors. Sleep Med. 178, 216–220.
National Institutes of Health [NIH] (2015). Overdose Death Rates | National Institute on Drug Abuse (n.d.). National Institute on Drug Abuse (n.d.). Available online at: https://nida.nih.gov/research-topics/trends-statistics/overdose-death-rates (accessed December 18, 2022).
Neugebauer, V., and Weidong, L. I. (2002). Processing of nociceptive mechanical and thermal information in central amygdala neurons with knee-joint input. J. Neurophysiol. 87, 103–112.
Norris, A. J., Shaker, J. R., Cone, A. L., Ndiokho, I. B., and Bruchas, M. R. (2021). Parabrachial opioidergic projections to preoptic hypothalamus mediate behavioral and physiological thermal defenses. Elife 10:e60779. doi: 10.7554/eLife.60779
O’Brien, C. B., Locklear, C. E., Glovak, Z. T., Zebadúa Unzaga, D., Baghdoyan, H. A., and Lydic, R. (2021). Opioids cause dissociated states of consciousness in C57BL/6J mice. J. Neurophysiol. 126, 1265–1275. doi: 10.1152/jn.00266.2021
Oertel, B. G., Felden, L., Tran, P. V., Bradshaw, M. H., Angst, M. S., Schmidt, H., et al. (2010). Selective antagonism of opioid-induced ventilatory depression by an ampakine molecule in humans without loss of opioid analgesia. Clin. Pharmacol. Ther. 87, 204–211. doi: 10.1038/clpt.2009.194
Onimaru, H., and Homma, I. (2006). Point:Counterpoint: The parafacial respiratory group (pFRG)/pre-Bötzinger complex (preBötC) is the primary site of respiratory rhythm generation in the mammal. J. Appl. Physiol. 100, 2094–2098. doi: 10.1152/japplphysiol.00119.2006
Palmiter, R. D. (2018). The parabrachial nucleus: CGRP neurons function as a general alarm. Trends Neurosci. 41, 280–293. doi: 10.1016/j.tins.2018.03.007
Pasquinucci, L., Parenti, C., Georgoussi, Z., Reina, L., Tomarchio, E., and Turnaturi, R. (2021). Lp1 and lp2: Dual-target mopr/dopr ligands as drug candidates for persistent pain relief. Molecules 26:4168. doi: 10.3390/molecules26144168
Pattinson, K. T. S. (2008). Opioids and the control of respiration. Br. J. Anaesth. 100, 747–758. doi: 10.1093/bja/aen094
Pieh, C., Popp, R., Geisler, P., and Hajak, G. (2011). Sleep and pain: A bi-directional relation?. [German]\rSchlaf und schmerz: Ein wechselseitiger Zusammenhang? Psychiatr Prax 38, 166–170. doi: 10.1055/s-0030-1265949
Qiu, M. H., Chen, M. C., Fuller, P. M., and Lu, J. (2016). Stimulation of the pontine parabrachial nucleus promotes wakefulness via extra-thalamic forebrain circuit nodes. Curr. Biol. 26, 2301–2312. doi: 10.1016/j.cub.2016.07.054
Ramirez, J. M., Burgraff, N. J., Wei, A. D., Baertsch, N. A., Varga, A. G., Baghdoyan, H. A., et al. (2021). Neuronal mechanisms underlying opioid-induced respiratory depression: Our current understanding. J. Neurophysiol. 125, 1899–1919. doi: 10.1152/jn.00017.2021
Ripley, T. L., Sanchez-Roige, S., Bullmore, E. T., Mugnaini, M., Maltby, K., Miller, S. R., et al. (2015). The novel mu-opioid antagonist, GSK1521498, reduces ethanol consumption in C57BL/6J mice. Psychopharmacology (Berl). 232, 3431–3441. doi: 10.1007/s00213-015-3995-x
Roozekrans, M., van der Schrier, R., Okkerse, P., Hay, J., McLeod, J. F., and Dahan, A. (2014). Two studies on reversal of opioid-induced respiratory depression by BK-channel blocker GAL021 in human volunteers. Anesthesiology 121, 459–468. doi: 10.1097/ALN.0000000000000367
Saper, C. B., and Kaur, S. (2018). Brain circuitry for arousal from apnea. Cold Spring Harb. Symp. Quant. Biol. 83, 63–69. doi: 10.1101/sqb.2018.83.038125
Schrimpf, M., Liegl, G., Boeckle, M., Leitner, A., Geisler, P., Pieh, C., et al. (2015). The effect of sleep deprivation on pain perception: A meta-analysis. Psychother. Psychosom. 84:63.
Schwarzer, A., Aichinger-Hinterhofer, M., Maier, C., Vollert, J., and Walther, J. W. (2015). Sleep-disordered breathing decreases after opioid withdrawal: Results of a prospective controlled trial. Pain 156, 2167–2174. doi: 10.1097/j.pain.0000000000000279
Simpson, N., Sethna, N., Kaur, S., and Mullington, J. (2020). Sleep deficiency and chronic pain: Potential underlying mechanisms and clinical implications. Neuropsychopharmacology 45, 205–216. doi: 10.1038/s41386-019-0439-z
Skinner, G. O., Damasceno, F., Gomes, A., and de Almeida, O. M. (2011). Increased pain perception and attenuated opioid antinociception in paradoxical sleep-deprived rats are associated with reduced tyrosine hydroxylase staining in the periaqueductal gray matter and are reversed by L-DOPA. Pharmacol. Biochem. Behav. 99, 94–99. doi: 10.1016/j.pbb.2011.04.009
Strang, J., Volkow, N. D., Degenhardt, L., Hickman, M., Johnson, K., Koob, G. F., et al. (2020). Opioid use disorder. Nat. Rev. Dis. Prim. 6:3.
Stucke, A. G., Miller, J. R., Prkic, I., Zuperku, E. J., Hopp, F. A., and Stuth, E. A. (2015). Opioid-induced respiratory depression is only partially mediated by the prebötzinger complex in young and adult rabbits in vivo. Anesthesiology 122, 1288–1298. doi: 10.1097/ALN.0000000000000628
Sun, L., Liu, R., Guo, F., Wen, M. Q., Ma, X. L., Li, K. Y., et al. (2020). Parabrachial nucleus circuit governs neuropathic pain-like behavior. Nat. Commun. 11, 5974. doi: 10.1038/s41467-020-19767-w
Sun, X., Thörn Pérez, C., Halemani, D. N., Shao, X. M., Greenwood, M., Heath, S., et al. (2019). Opioids modulate an emergent rhythmogenic process to depress breathing. Elife 8:e50613. doi: 10.7554/eLife.50613
Tripathi, R., Rao, R., Dhawan, A., Jain, R., and Sinha, S. (2020). Opioids and sleep – a review of literature. Sleep Med. 67, 269–275.
Varga, A. G., Reid, B. T., Kieffer, B. L., and Levitt, E. S. (2020). Differential impact of two critical respiratory centres in opioid-induced respiratory depression in awake mice. J. Physiol. 598, 189–205. doi: 10.1113/JP278612
Viscusi, E., Minkowitz, H., Webster, L., Soergel, D., Burt, D., Subach, R., et al. (2016). Rapid onset of pain relief with oliceridine (TRV130), A novelμ receptor G protein pathway selective (μ-GPS) Modulator, VS. Morphine: A phase 2A/B study analysis. Anesth. Analg. 17, S82–S83.
Wang, W., Fung, M. L., and St John, W. M. (1993). Pontile regulation of ventilatory activity in the adult rat. J. Appl. Physiol. 74, 2801–2811. doi: 10.1152/jappl.1993.74.6.2801
Webster, L. R., Choi, Y., Desai, H., Webster, L., and Grant, B. J. (2008). Sleep-disordered breathing and chronic opioid therapy. Pain Med. 9, 425–432.
Wilson, J. D., Nicklous, D. M., Aloyo, V. J., and Simansky, K. J. (2003). An orexigenic role for μ-opioid receptors in the lateral parabrachial nucleus. Am. J. Physiol. Regul. Integr. Comp. Physiol. 285, R1055–R1065. doi: 10.1152/ajpregu.00108.2003
Witkiewitz, K., and Vowles, K. E. (2018). Alcohol and opioid use, co-use, and chronic pain in the context of the opioid epidemic: A critical review. Alcohol. Clin. Exp. Res. 42, 478–488. doi: 10.1111/acer.13594
Wodarski, R., Schuh-Hofer, S., Yurek, D. A., Wafford, K. A., Gilmour, G., Treede, R. D., et al. (2015). Development and pharmacological characterization of a model of sleep disruption-induced hypersensitivity in the rat. Eur. J. Pain (United Kingdom) 19, 554–566. doi: 10.1002/ejp.580
Wolinsky, T. D., Carr, K. D., Hiller, J. M., and Simon, E. J. (1996). Chronic food restriction alters mu and kappa opioid receptor binding in the parabrachial nucleus of the rat: A quantitative autoradiographic study. Brain Res. 706, 333–336. doi: 10.1016/0006-8993(95)01337-7
Yeghiazarians, Y., Jneid, H., Tietjens, J. R., Redline, S., Brown, D. L., El-Sherif, N., et al. (2021). Obstructive sleep apnea and cardiovascular disease: A scientific statement from the American Heart Association. Circulation 144, e56–e67.
Yokota, S., Kaur, S., VanderHorst, V. G., Saper, C. B., and Chamberlin, N. L. (2015). Respiratory-related outputs of glutamatergic, hypercapnia-responsive parabrachial neurons in mice. J. Comp. Neurol. 523, 907–920. doi: 10.1002/cne.23720
Keywords: analgesia, opioid induced respiratory depression, opioid use disorder, opioid tolerance, sleep-loss
Citation: Lynch N, Lima JD, Spinieli RL and Kaur S (2023) Opioids, sleep, analgesia and respiratory depression: Their convergence on Mu (μ)-opioid receptors in the parabrachial area. Front. Neurosci. 17:1134842. doi: 10.3389/fnins.2023.1134842
Received: 30 December 2022; Accepted: 21 March 2023;
Published: 06 April 2023.
Edited by:
Giancarlo Vanini, School of Medicine, University of Michigan, United StatesReviewed by:
Dinesh Pal, University of Michigan, United StatesCopyright © 2023 Lynch, Lima, Spinieli and Kaur. This is an open-access article distributed under the terms of the Creative Commons Attribution License (CC BY). The use, distribution or reproduction in other forums is permitted, provided the original author(s) and the copyright owner(s) are credited and that the original publication in this journal is cited, in accordance with accepted academic practice. No use, distribution or reproduction is permitted which does not comply with these terms.
*Correspondence: Satvinder Kaur, c2thdXJAYmlkbWMuaGFydmFyZC5lZHU=