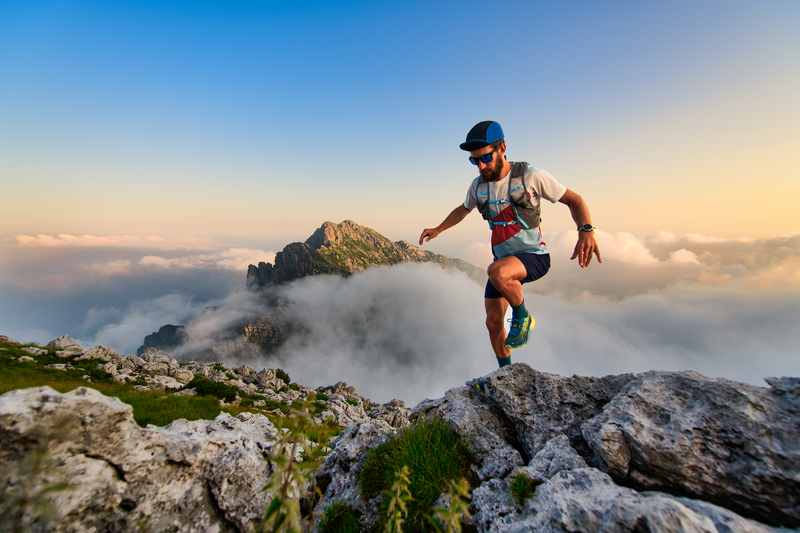
95% of researchers rate our articles as excellent or good
Learn more about the work of our research integrity team to safeguard the quality of each article we publish.
Find out more
REVIEW article
Front. Neurosci. , 27 April 2023
Sec. Gut-Brain Axis
Volume 17 - 2023 | https://doi.org/10.3389/fnins.2023.1130730
Being isolated from the peripheral system by the blood–brain barrier, the brain has long been considered a completely impervious tissue. However, recent findings show that the gut microbiome (GM) influences gastrointestinal and brain disorders such as Alzheimer’s disease (AD). Despite several hypotheses, such as neuroinflammation, tau hyperphosphorylation, amyloid plaques, neurofibrillary tangles, and oxidative stress, being proposed to explain the origin and progression of AD, the pathogenesis remains incompletely understood. Epigenetic, molecular, and pathological studies suggest that GM influences AD development and have endeavored to find predictive, sensitive, non-invasive, and accurate biomarkers for early disease diagnosis and monitoring of progression. Given the growing interest in the involvement of GM in AD, current research endeavors to identify prospective gut biomarkers for both preclinical and clinical diagnoses, as well as targeted therapy techniques. Here, we discuss the most recent findings on gut changes in AD, microbiome-based biomarkers, prospective clinical diagnostic uses, and targeted therapy approaches. Furthermore, we addressed herbal components, which could provide a new venue for AD diagnostic and therapy research.
Alzheimer’s disease (AD) is the most common neurodegenerative disease of cognitive decline in people over 65 years old, accounting for 60–70% of cases (Daniilidou et al., 2011; 2022 Alzheimer’s disease facts and figures, 2022; Dementia, 2022). AD is characterized by amyloid-β protein (Aβ) deposition, abnormal phosphorylation aggregation of the microtubule-associated protein tau, neuroinflammation, oxidative stress, and synaptic dysfunction, which are reflected by microglial reaction and increased cytokine production (Braak and Braak, 1991; Heneka et al., 2015; Lepeta et al., 2016; Butterfield and Halliwell, 2019; Bairamian et al., 2022; Paolicelli et al., 2022). The rapidly expanding effect of AD on socioeconomics and health care is becoming a global health issue. The global prevalence of dementia is expected to reach 78 million people by 2030 and is projected to reach 152 million by 2050 (ADI-Dementia Statistics, 2022). The gut microbiome (GM) comprises various microorganisms, including several species of viruses, bacteria, protozoa, and fungi (Cryan et al., 2019). GM displays vast diversity among different population groups and significantly influences human health, disease state, and overall well-being (Zmora et al., 2019). The microbiome-gut-brain axis (MGBA) links the GM and the brain via epigenetic, neural, and humoral pathways (Roy Sarkar and Banerjee, 2019; Liu et al., 2020; Nagu et al., 2021). Dysbiosis occurs when the GM is abnormally altered (Liu et al., 2020), leading to neurological disorders such as AD (Spielman et al., 2018; Sherwin et al., 2019; Zhu et al., 2021; Chidambaram et al., 2022).
This devastating and progressive disease’s neuropathological evaluation has evolved in preclinical and clinical fields (Zhang P.-F. et al., 2022). The diagnostic criteria for AD were refined in 2011 and 2018 while affirming the use of biomarkers and the new ability to describe the preclinical phase of the disease (Jack et al., 2018). Specific intestinal changes in pre-AD have been suggested to be involved in pre-AD pathology (Guo et al., 2021; Sheng et al., 2021). Therefore, gut-associated biomarkers may be a promising alternative or complementary tool for assessing disease conditions (O’Toole and Jeffery, 2015). Indeed, gut microbial-derived biomarkers have reported strong predictive and differential diagnostic power when used in studies such as psychological and neurodegenerative disorders (Qian et al., 2020; Lucidi et al., 2021). Furthermore, growing evidence demonstrates that MGBA is involved in the pathogenesis of AD. Hence, it might be modulated to regulate metabolite levels and remodel the gut barrier balance, improving cognition and providing a novel therapeutic approach. Consequently, it is imperative to explore in depth the critical role of the GM in the pathogenesis of AD and to evaluate further the value of gut biomarkers in early diagnosis, progression monitoring, and potential target therapy of the disease. Here, we review (1) gut involvement in peripheral and central nervous system (CNS) crosstalk in AD, (2) characteristic changes in the GM of AD and microbiome-based biomarkers, and (3) microbiome-potential targeted therapies to provide new ideas for early diagnosis of AD and directions for therapeutic drug development.
Epigenetics associated with AD include histone modifications and deoxyribonucleic acid (DNA) methylation, regulated by acetylases and methylases (Esposito and Sherr, 2019). Metabolites produced by the microbiome can inhibit histone deacetylases and other epigenetic marks (Maslowski and Mackay, 2011) and regulate inflammatory responses in the CNS (Bolduc et al., 2017). Moreover, they impact the acetylation and methylation of DNA and histones, allowing GM to trigger the expression of MHC class II molecules (Reid et al., 2017; Ye et al., 2017). Another aspect is microglia, myeloid innate immune cells in the CNS (Salter and Stevens, 2017). Recent preclinical, genetic, and bioinformatics data suggest that its reaction is accompanied by pathological changes in AD and is regulated by the microbiome (Colombo et al., 2021; Zhou et al., 2022). Downregulation of homeostatic genes in vivo and overexpression of recognized AD-associated genes, including apolipoprotein E and tyrosine protein tyrosine kinase binding protein, are linked to the transition to disease-associated microglia (Keren-Shaul et al., 2017; Leng and Edison, 2021). The identification of a correlation between AD and mutations in trigger receptor genes expressed on myeloid 2 (TREM2) and the myeloid cell surface antigen sialic acid binding Ig-like lectin 3 molecule supports for the first time the link between immune changes and AD pathogenesis (Bradshaw et al., 2013; Guerreiro et al., 2013; Jonsson et al., 2013). Functional TREM2 expression is downregulated in some cases of late-onset AD and may exacerbate intestinal microbial metabolites’ endotoxin-induced pro-inflammatory responses (Zhong et al., 2015), causing defective antibody clearance (Xiang et al., 2016).
Gut microbiome may lead to increased neuroinflammation and decreased synaptic plasticity, both of which are thought to contribute to the development and progression of AD (Jiang et al., 2021). Gut microbiomes can alter the composition of the immune cells in the brain, leading to an increase in neuroinflammation (Erny et al., 2015). For example, studies in animal models have shown that colonization with certain types of gut bacteria can lead to microglial activation. Deficiency of the gut microbiome first affects the microglial cell transcriptome, which primarily regulates the interconversion of microglial cell subpopulations, and transcriptome changes are primarily associated with AD (Huang et al., 2023). Studies have shown that changes in the gut microbiome can alter synaptic plasticity in the brain. For example, as recently reviewed, germ-free mice (raised without exposure to microorganisms) have impaired synaptic plasticity compared to conventionally-raised mice (Glinert et al., 2022).
Synaptic dysfunction and microglia may interact through the microbiome (Bairamian et al., 2022), and synaptic dysfunction may precede symptoms of cognitive impairment (Selkoe and Hardy, 2016), as evidenced by diminished long-term potentiation (LTP) and long-term depression, which are closely related to synaptic plasticity (Bairamian et al., 2022). In addition, animal model studies have identified gut microbiome metabolites that regulate LTP in physiological and AD-related pathologies mechanisms, including influencing the expression of pre-and postsynaptic neuronal membrane receptors and membrane genes that further influence ion channels and thus affect synaptic activity (Albuquerque et al., 2015; Tong et al., 2015). These findings suggest a complex relationship between the gut microbiome, neuroinflammation, synaptic plasticity, and AD development. While much more research is needed to fully understand these relationships, the emerging evidence suggests that targeting the gut microbiome may represent a promising avenue for developing new treatments for this devastating disease.
Inflammation in AD is not confined to neuroinflammation but includes the complex signals between microbial involvement in peripheral and central interaction. This crosstalk may comprise many neuronal, endocrine, immunological, and metabolic pathways. To be more precise, by exploiting the MGBA. This section concentrates on interacting with the brain through neurological and humoral pathways (Abdel-Haq et al., 2019). Figure 1 concisely summarizes the role that the gut microbiome plays in AD in terms of epigenetics as well as central and peripheral crosstalk.
Figure 1. Gut Microbiome epigenetic, peripheral, and central pathology of AD. Changes in gut microbial composition, abundance, and metabolite diversity are involved in AD through epigenetic regulation of CNS function. (1) through acetylases, methylases, and deacetylases, affecting acetylation and methylation of DNA and histones; and (2) through microglia upregulation of APOE and downregulation of TREM2 associated with AD pathogenesis. Abbreviations: AD: Alzheimer’s disease; CNS: central nervous system; DNA: deoxyribonucleic acid; APOE: apolipoprotein E; TREM2: trigger receptor genes expressed on myeloid 2.
The vagus nerve is a crucial neuronal pathway within the MGBA. Its functionality encompasses efferent and afferent modalities, facilitating communication between the gastrointestinal tract and the CNS. Specifically, sensory fibers within the vagus nerve transmit tonal information from vital organs such as the heart, lungs, stomach, and intestines to the CNS (Zhong et al., 2021). The main projection site for gut-associated vagal afferents is in the nucleus tractus solitarius (NTS); (Fülling et al., 2019). Neurons in the NTS that receive vagal sensory input can form synaptic connections with enteroendocrine cells (Kaelberer et al., 2018), and neurotransmitters and purinergic signaling transmit to the brain via specific ligand-receptor pairs to regulate microglia state and activity (Eyo and Wu, 2013). In addition, vagal efferent fibers transmit information to the intestine and release acetylcholine to reduce the inflammatory response (Pavlov and Tracey, 2005; Breit et al., 2018). A growing body of evidence indicates that microbiomes can significantly impact brain function through their interactions with the vagus nerve (Goehler et al., 2005; Bravo et al., 2011; Sgritta et al., 2019). A recent study indicated that Aβ or tau injected into the colon is transmitted to the brain via the vagus nerve, which, attenuated after vagotomy (Chen et al., 2021); stimulation of the vagus nerve can alter glutamate receptor levels involved in AD (Yesiltepe et al., 2022). Previous studies have found that stimulatory signals from the gut and vagus nerve activate the hypothalamic–pituitary–adrenal axis and promote corticosterone release (Zimomra et al., 2011), leading to changes in intestinal permeability (Moussaoui et al., 2014), dynamics (Gue et al., 1991), and mucus (Silva et al., 2014). In addition, recent studies have also revealed the importance of pathological changes in the gut-vagus-brain signaling pathway in AD (Das et al., 2022). These findings further suggest a critical role for vagal signaling as a bridge between peripheral and central crosstalk.
Humoral signals circulate in the blood, including microbial metabolites, hormones, cytokines, and immune cells, primarily across to CNS through the intestinal and blood–brain barrier (BBB); (Paouri and Georgopoulos, 2019). Changes in the intestinal microbiome promote the production of toxic metabolites and pro-inflammatory cytokines, decreasing beneficial substances such as short-chain fatty acids (SCFAs) and other anti-inflammatory factors. This increased intestinal permeability allows the entry of pathogenic, immunostimulatory, and neuroactive substances into the body’s circulation (Xie et al., 2022) and activates local and remote immune cells, resulting in BBB dysfunction and triggering a neuroinflammatory response, especially in the hippocampal region and cerebral cortex (Kempuraj et al., 2017; Welcome, 2019). Propionate acid can contribute to immune homeostasis by regulating cellular subpopulations (Dupraz et al., 2021). Butyric acid provides sufficient energy for intestinal epithelial cells and alternative substrates for brain metabolism and upregulates the expression of tight junction proteins to enhance the integrity of the BBB (Barichello et al., 2019; Silva et al., 2020; Chen et al., 2022). In addition, SCFAs clear protein aggregates in the brain by enhancing the function of microglia (Erny et al., 2015; Wenzel et al., 2020). Several studies in AD mice have confirmed butyrate’s important role in improving microglia function and reducing Aβ deposition (Jiang et al., 2021; Liu et al., 2021). Studies have shown that SCFAs activate G-protein-coupled receptors (GPR) 43, GPR 41, and GPR 109, receptors in the intestine and brain, which can protect the BBB from oxidative stress by modulating Recombinant Cluster of Differentiation 14 signaling and activating the nuclear factor (erythroid-derived 2)-like 2 (Tan et al., 2014; Hoyles et al., 2018). Conversely, lipopolysaccharide (LPS) induces the activation of macrophages and dendritic cells to produce pro-inflammatory cytokines (Jeong et al., 2019) and is capable of interacting Toll-like receptor-4 (TLR4); Park et al., 2009). Cani et al. found that LPS can stimulate the body’s immune response by destroying intestinal epithelial cells to enter the bloodstream (Cani et al., 2008). In addition, multiple lines of evidence suggest that endotoxin promotes Aβ42 fiber formation (Kahn et al., 2012; Asti and Gioglio, 2014) and that microglia can be activated by endotoxin, causing central inflammation (Shen et al., 2020).
Escherichia coli-derived neurotoxins in Proteobacteria are associated with neuropathology in AD and increase the release of pro-inflammatory cytokines that induce systemic inflammation and exacerbate AD pathology (Kitazawa et al., 2005; Cattaneo et al., 2017). Many bacteria strains are capable of producing amyloid (Megur et al., 2020; Tran and Mohajeri, 2021), and the forms show similarity with the CNS amyloid (Hill and Lukiw, 2015), which can lead to an enhanced immune response and endogenous formation of neuronal amyloid in the brain (Friedland and Chapman, 2017). LPS and gram-negative E. coli fragments coexisted with amyloid plaques in AD brain tissue (Zhan et al., 2016). Aβ loading may initially occur in the gastrointestinal tract. Aβ1-42 migrates after being injected into the gastric wall, and Aβ deposition is present in the vagus nerve and brain and develops symptoms of cognitive impairment (CI; Sun Y. et al., 2020). Therefore, this could provide some basis for the movement of the GM to trigger the amyloid pathogenesis of AD. The microbiome is necessary for the normal development of hippocampal and microglial cell morphology (Luczynski et al., 2016). Recently, Liu et al. observed microbiome deficiency altered dendritic signaling integration in the Cornu Ammonis1 region of mice (Liu et al., 2022). The presence of activated microglia and reactive astrocytes in the vicinity of amyloid plaques is characteristic of AD neuroinflammation, mainly in the hippocampus (Crews and Masliah, 2010; Cattaneo et al., 2017). Moreover, in the elderly brain, microglia are dysfunctional and prone to chronic activation (Spittau, 2017). In the early pathological stages of AD, the overall microglial response can support neurons by phagocytosing Aβ fibers. However, as the disease progresses, the loss of the branching phenotype of microglia with excessive activation near plaques and aggregation of other immune cells triggers a neurotoxic environment, leading to neural network damage (Leng and Edison, 2021; Thu Thuy Nguyen and Endres, 2022). Other studies have also observed numerous roles for aberrantly regulated GM in triggering Aβ amyloidosis, neuroinflammation, and microglia regulation in AD mice (Dodiya et al., 2019).
The intestinal microbiome contains two major bacterial phylotypes, the Firmicutes, the Bacteroidetes, and to a lesser extent, the actinomycetes, fusobacteria, proteobacteria, and weberia (Grenham et al., 2011). Some studies have found less diverse Firmicutes and genera, but a higher prevalence of Proteobacteria in AD (Nagpal et al., 2019; Sheng et al., 2021; Hung et al., 2022). Intestinal α-diversity and β-diversity are altered in patients on the AD spectrum (Hung et al., 2022). Several studies have reported a significant decrease in α-diversity in AD patients (Vogt et al., 2017; Liu P. et al., 2019), but not obvious in mild cognitive impairment (MCI) patients, and there is a similar gradual decline trend from MCI to AD (Nagpal et al., 2019). Nevertheless, results vary regarding β-diversity studies, with one Austrian study showing that malnutrition and drug intake affect the abundance of the specific microbiome, SCFAs, and butyrate production, and statins may be one of the reasons affecting beta diversity (Stadlbauer et al., 2020). A recent systematic review and meta-analysis of 11 studies included 378 normal control (NC) and 427 AD patients to analyze the impact of different countries and clinical stages on GM abundance. GM diversity was significantly lower in AD patients than in NC but not in MCI patients. Compared to NC, the AD spectrum group had an increased abundance of Proteobacteria, Bifidobacterium, and Phascolarc to the Bacterium, and a decreased abundance of Firmicutes, Clostridiaceae, Lachnospiraceae, and Rikenellaceae (Hung et al., 2022). More importantly, the abundance distribution of the Alistipes and Bacteroides in the NC and AD differed by country (Hung et al., 2022).
Several studies have observed alterations in gut microbiology in patients with AD, but mostly with the influence of drugs and other interventions and varying disease duration. Although there is no direct evidence in clinical trials that the effects on MGBA of current medications for AD (acetylcholinesterase inhibitors or N-methyl-D-aspartate (NMDA) antagonists) have been studied (La Rosa et al., 2018), the latest study provides evidence that donepezil affects GM via amino acid pathways and sugar metabolism (Jo et al., 2022). In light of the results mentioned above, the researchers’ attention was drawn to MCI and subjective cognitive decline (SCD) patients’ gut changes. A study used 16S ribosomal ribonucleic acid (16SrRNA) sequencing to examine stool samples from 18 AD patients without treatment or intervention, 20 MCI patients, and 18 age-matched NC. The findings revealed that Prevotella levels were higher and Bacteroides, Lachnospira, and Ruminiclostridium_9 levels were lower in AD patients. Additionally, greater cognitive performance was favorably correlated with Bacteroides, Lachnospira, and Ruminiclostridium_9, although Prevotella had the opposite relationship (Guo et al., 2021). Based on reduced effects such as drugs, the above results likewise support the idea of a progressive worsening of the degree of intestinal dysbiosis from MCI to the disease stage of AD (Guo et al., 2021). Based on this, Sheng et al. further explored gut microbial composition changes in SCD, the earliest symptom of preclinical AD. The study included 38 NC, 53 patients with SCD, and 14 patients with CI and compared the gut microbial composition of the three groups and the relationship with cognition using 16SrRNA technology (Sheng et al., 2021). Findings revealed a decreasing trend in the abundance of the phylum Firmicutes, class Clostridia, order Clostridiales, family Ruminococcaceae, and genus Faecalibacterium from NC to SCD and CI (Sheng et al., 2021). In particular, the abundance of the anti-inflammatory genus Faecalibacterium was significantly lower in the SCD group than in the NC group. Notably, altered bacterial taxa were associated with cognitive performance and were validated in amyloid-positive SCD participants (Sheng et al., 2021). The results further suggest that the microbiome may influence the development of amyloid pathology.
Proteobacteria is a major phylum of gram-negative bacteria (Zhao and Lukiw, 2018). More gram-negative bacteria have also been reported in AD patients compared to NC (Vogt et al., 2017). In addition, the abundance of Proteobacteria increased with increasing memory dysfunction (Hossain et al., 2019; Jeong et al., 2019). Bacteroides have been shown to preserve the intestinal barrier and reverse leaky gut (Hsiao et al., 2013), indicating that they may be a microbiome protective factor. Bacteroides levels are reduced in AD and MCI patients (Cattaneo et al., 2017; Zhuang et al., 2018). Moreover, the relative abundance of the Actinomycete phylum was associated with diffusion tensor imaging of the thalamus, hypothalamus, and amygdala, as well as cognitive test scores (Fernandez-Real et al., 2015). Certain Lactobacillus and Bifidobacterium secrete gamma-aminobutyric acid, which may mediate cognitive function in AD (Barrett et al., 2012), and different levels of abundance of the Lactobacillus family have been observed at different stages of dementia (Stadlbauer et al., 2020). Vogt et al. compared the different bacterial classifications of stool samples from AD patients versus NC and observed levels of differentially abundant genera associated with cerebrospinal fluid (CSF) biomarkers of AD pathology (Vogt et al., 2017).
In addition to the intestinal bacteria noted by most researchers, some studies have also found specific alterations in MCI patients in the genus Fungi and a clear correlation with AD biomarkers (Nagpal et al., 2019). More importantly, the different microbiome and fungal biota composition in 3xTg-AD mice is similar to the microbial variation found in humans, and significantly different taxa may contribute to the pathogenic cues of AD being identified (D’Argenio et al., 2022). However, there are some differences in studies regarding oral microbiology. A Canadian study used 16SrRNA sequencing to analyze stool and oral samples from AD and NC comparatively. The study found that oral microbiota exhibited greater differences between patients and controls than GM but was not associated with cognitive function (Cirstea et al., 2022). Although some results support the possibility that oral bacteria or their components may themselves invade the brain (Laugisch et al., 2018; Jungbauer et al., 2022), analyses using publicly available genome-wide association studies on periodontitis and AD failed to reveal any genetically predicted association between AD and periodontitis risk (Sun Y.-Q. et al., 2020). However, preventing and treating periodontitis and its associated inflammation could reduce neuroinflammation and prevent and treat neurodegenerative diseases (Li et al., 2022).
The discovery of biomarkers over the past 20 years has allowed for the verification of Aβ and tau-related fluid biomarkers in clinical studies as well as their incorporation into the [AT(N)] framework for diagnosis (Mayeux, 2004; Jack et al., 2018). The [AT(N)] framework was proposed by the National Institute on Aging-Alzheimer’s Association in 2018 and focused on diagnosing AD with in vivo biomarkers. Biomarkers are classified as Aβ deposition, pathological tau, and neurodegeneration [AT(N)] (Jack et al., 2018). However, many pharmacological treatments targeting Aβ and tau pathological features have proven futile (Mahaman et al., 2022). This may be attributed to the difficulty of reversing the underlying neuropathological changes that have already occurred (Mahaman et al., 2022). Therefore, very early and reliable diagnostic biomarkers are urgently needed. Recently, a study found that alterations in the GM precede the development of key pathological features of AD (Chen et al., 2020; Bello-Medina et al., 2021). In addition, the apparent association between AD and specific bacterial strains has facilitated the exploration of highly promising biomarkers in GM. Gut bacterial metabolites, gut permeability, gut hormones, MGBA involvement in AD, and potential gut-based biomarkers and targeted herbal therapies are shown in Figure 2. More importantly, completely non-invasive biomarkers such as stool, saliva, or urine (Mahaman et al., 2022) may be a realistic option for large-scale population screening, early AD diagnosis, and disease progression monitoring at a low cost.
Figure 2. Biomarkers in the AD microbiome-gut-brain axis.Dysbiosis results in (1) a decrease in specific beneficial microbiome and metabolites and an increase in pro-inflammatory microbiome and harmful metabolites; (2) Increased intestinal permeability markers in serum and feces due to disruption of tight junctions; (3) Increased release of cytosolic inflammatory factors from microglia; (4) An increase or decrease in specific neurotransmitters involved in the development of AD. They are potential gut biomarkers in AD. Traditional Chinese Medicine ingredients can reduce AD pathology and improve cognitive function in a multi-targeted and multi-pathway manner by modulating intestinal microbes and metabolites, reshaping the intestinal barrier, and reducing neuroinflammation. Abbreviations: AD: Alzheimer’s disease; GM: gut microbiome; LPS: lipopolysaccharide; Aβ: amyloid-β protein; TCM: traditional Chinese.medicine.
Based on many characteristic changes in the GM detected in the pre-AD period, this finding may provide clues as a diagnostic biomarker in the pre-AD period (Guo et al., 2021; Sheng et al., 2021; Verhaar et al., 2022). Hence, numerous studies have attempted to use GM for early diagnostic identification of AD.
APP/PS1 mice combining 16SrRNA gene sequencing and extensively targeted metabolomics revealed that B. firmus, Rikenella, Clostridium sp. culture-27, and deoxyuridine might be important biomarkers of AD (Feng et al., 2022). Based on the GM differences in feces and blood established in the preliminary study, Li et al. built a random forest model based on 11 genera of differences in stools and blood from AD patients and NC. Using the random forest model’s cut-off values with all different stool input genera, 28 of 30 MCI patients could be accurately identified with a sensitivity of 93% (Li et al., 2019). In addition, 33 AD, 32 amnestic MCI (aMCI), and 32 NC were examined in cross-sectional research. According to the findings, AD patients had a reduced level of fecal microbial diversity compared to aMCI patients and NC. Further, as patients advanced from NC to aMCI and AD, Gammaproteobacteria, Enterobacteriales, and Enterobacteriaceae gradually enriched abundance. A strong association was also discovered between the clinical severity scores of AD patients and the abundance of changed microbiomes. Notably, the model based on the abundance of Enterobacteriaceae bacteria could distinguish AD from aMCI and NC (Liu P. et al., 2019). This initially validates the value of intestinal biomarkers in the early identification of patients with CI and affirms the great potential of gut biomarkers. To improve the robustness of gut biomarkers, a study in 2022 simultaneously combined the latest proposed [AT(N)] framework. The study collected 34 Aβ (−) cognitively normal (CN−), 32 Aβ (+) cognitively normal (CN+), and 22 (11 MCI, 11 AD) CI patients. According to the findings, the relative abundance of bacteria that produce SCFAs reduced from CN− to CN+ and CI. Moreover, all CN subjects had a negative correlation between total brain Aβ load, plasma Aβ42/Aβ40, and 3 particular types of bacteria. It was discovered that combining plasma Aβ markers, altered GM, and cognitive ability improved discrimination between CN+ and CN− (Sheng et al., 2022). The above promising findings suggest the possibility and accuracy of GM in diagnosing AD.
Patients with newly diagnosed AD or MCI have dysbiosis of the intestinal microbiome, including a decrease in the potentially protective microbiome and an increase in the pro-inflammatory microbiome (Chen et al., 2020; Bello-Medina et al., 2021). Significant changes in gut microbial composition are observed, especially in older people, and diet may be the most important driver of change (Kumar et al., 2016). The severity of human microbiome dysbiosis shows characteristic changes in different disease stages of AD (Stadlbauer et al., 2020); an assessment of disease progression and prognosis provides some basis, suggesting that these may be meaningful biomarkers for predicting AD development (Li et al., 2019). In addition, recent studies have reported that neuroimaging of the MGBA will further improve our understanding of GM by altering neural microstructure and function in time and space (Montoro et al., 2022).
Tight junctions are an important mechanism affecting the intestinal barrier and the BBB, with the interaction of intestinal bacteria with epithelial cells being a key factor in regulating epithelial permeability by modulating tight junctions (Allam-Ndoul et al., 2020). It has been found in the last decade to be associated with AD (Schoultz and Keita, 2020). Calprotectin, a marker of intestinal inflammation (Kowalski and Mulak, 2019), is significantly increased in the CSF and brain of AD patients (Wang et al., 2014). It implies that intestinal permeability could be associated with AD pathogenesis.
Human targeting studies-the sugar absorption assay (Ménard et al., 2010), identified plasma lipopolysaccharide-binding protein (LBP) as a promising biomarker of intestinal permeability in adults (Seethaler et al., 2021). However, the markers of intestinal barrier integrity LBP and intestinal fatty acid binding protein (IFABP), are not associated with independent AD dementia, MCI, cognition, and neuropathology (Voigt et al., 2021). It is worth noting that disruption of the apical junction complex was not evaluated, and the small sample size may have influenced the results. Another study indirectly illustrated the relationship between intestinal permeability and AD. The peripheral platelet alterations are involved in the pathological process of AD and are based on the fact that C-type lectin-like receptor 2 (CLEC-2) is an activated receptor on the platelet surface (Mukaetova-Ladinska et al., 2012; Akingbade et al., 2018). Wang et al. found elevated levels of CLEC-2 and zonulin in MCI and AD patients, with a progressive increase compared to NC. Furthermore, the study identified high levels of CLEC-2 and zonulin as significant factors for decreased Mini-Mental State Examination scores (Wang X. et al., 2020). An Austrian clinical study included CI and normal population controls with serum diamine oxidase (DAO) and fecal zona pellucida protein to detect intestinal permeability and found higher levels of DAO in patients with dementia. The results suggest a correlation between dementia and increased intestinal permeability biomarkers (Stadlbauer et al., 2020). In addition, GM disorders resulted in low expression of the major tight junction proteins of the BBB (zonula occludens-1, occluding, and claudin-5) in the frontal cortex, hippocampus, and perisylvian striatum of mice (Braniste et al., 2014). More importantly, these brain regions affect memory and cognitive functions.
The findings above support a link between intestinal barrier dysfunction and AD. Some bacteria, such as Lactobacillus plantarum, Escherichia coli, or Bifidobacterium infantis, can improve the intestinal wall barrier by boosting the expression of tight junction-related proteins (Hakansson and Molin, 2011; Kowalski and Mulak, 2019). Increasing butyrate-producing bacteria enhanced tight junction protein expression in mice’s frontal cortex and hippocampus (Marizzoni et al., 2020). These findings give more evidence for the role of intestinal permeability in AD and may have biomarkers potential. However, it is crucial to highlight that due to the difficulties of performing sugar absorption tests in people with dementia, blood, and stool indicators are typically employed clinically instead (Stadlbauer et al., 2020). Various biomarkers associated with epithelial damage, including citrulline, IFABP, and LBP, have been used as indirect indicators of the reduced intestinal barrier (Galipeau and Verdu, 2016). However, studies have shown that even before leakage occurs, when the Notch signaling pathway involved in regulating the BBB is altered, the BBB has been disrupted, and there is already an impact on synaptic function, triggering dementia (Calderon et al., 2022). Conceivably, this will provide insights into discovering additional early markers of barrier function. Nonetheless, the mechanistic pathways that connect the gut barrier with the BBB should be studied further.
In AD, the intestinal microbiome and permeability characteristics have led researchers to explore the potential of gut microbial metabolites as biomarkers. The Ruminococcaceae metabolite isoamylamine recognizes and binds the Recombinant S100 Calcium Binding Protein A8 promoter region and promotes neuronal cell death, leading to cognitive decline in mice (Teng et al., 2022). Moreover, memory deficit in APP/PS1 mice was correlated with metabolite status. Fasudil et al. found that glutamate, hypoxanthine, thymine, hexanoyl coenzyme A, and leukotriene metabolize nucleotides, lipids, and sugars as well as inflammatory pathways involved in AD mouse-related metabolism (Yan et al., 2021). These novel results provide a valuable reference for using gut microbial metabolites as diagnostic biomarkers and therapeutic targets in clinical studies of AD. Several bacterial metabolites have been used as fecal biomarkers to characterize patients with AD. Trimethylamine N-oxide (TMAO) is a microbial-dependent metabolite (Connell et al., 2022). The study found higher TMAO levels, enhanced oxidative stress, and intestinal barrier dysfunction in MCI and AD patients (Vogt et al., 2018; Yan et al., 2021). TMAO topped the list of 56 microbial metabolites considered biomarkers of AD in a study identifying associations between AD and microbial metabolites through a web-based algorithm, successfully predicting changes in memory and fluid cognition in aging individuals (Xu and Wang, 2016). A clinical study included AD (n = 40), MCI (n = 35), and NC (n = 335) results show TMAO was significantly and positively correlated with p-tau, p-tau/Aβ42, and t-tau, neurofilament light in the CSF of AD (Vogt et al., 2018). These results suggest that TMAO may be involved in AD pathology and neurodegeneration (Vogt et al., 2018; Arrona Cardoza et al., 2022). Changes in tryptophan, methionine, tyrosine, and purine metabolism were observed in CSF from AD patients, suggesting these may be risk factors for CI (Kaddurah-Daouk et al., 2013). Some derived molecules produced by GM in the body’s circulation are also considered potential biomarkers of AD. The outer membrane of gram-negative bacteria is primarily made up of LPS (Wu L. et al., 2021), which is present in the hippocampus and superior temporal lobe neocortex of AD brains and triggers neuroinflammatory properties (Zhao et al., 2017) that have important implications for CI (Sparkman et al., 2006). In aMCI and AD patients were found to have elevated serum LPS levels compared to NC patients (Wu L. et al., 2021). In another study, the metabolomic analysis revealed significant differences between AD and NC in tryptophan metabolites, SCFAs, and staphylococcal acid, and most were associated with the altered microbiome and CI. Notably, tryptophan disorders are already present in aMCI, and SCFAs show a decreasing trend from aMCI to AD. More importantly, indole-3-pyruvic acid, a tryptophan metabolite that accurately classifies aMCI and AD with NC, is a marker for identifying and predicting AD, and five SCFAs were identified as markers for the pre-onset and progression of AD (Wu L. et al., 2021).
Several studies have found that hormones synthesized by intestinal cells and gut microbiomes can affect AD through neural and humoral pathways (Welcome, 2018). Notably, the microbiome may also regulate hormone levels (Yan et al., 2016; Engevik et al., 2020), suggesting that gut hormones may also be potential biomarkers of AD. Leptin affects the cortex and hippocampus and reduces Aβ in the brains of AD mice (Fewlass et al., 2004; Farr et al., 2015). In addition, chronic lateral ventricular injection of leptin effectively alleviated Aβ1-42-induced spatial memory deficits and reversed Aβ1-42-induced hippocampal late-phase LTP inhibition in rats (Tong et al., 2015). Cholecystokinin (CCK) is a satiety hormone that binds directly to CCK receptors in the hypothalamus and hindbrain to regulate appetite and is highly expressed in the hippocampus, which is essential for protecting and enhancing memory (Blevins et al., 2000; Plagman et al., 2019). A study examined the CCK levels of CSF in 287 subjects with AD and found high levels of CCK were associated with higher total CSF tau and p-tau181, associated with better cognition and more gray matter volumes in the posterior cingulate gyrus, parahippocampal gyrus, and medial prefrontal cortex. These results suggest that CCK levels may reflect compensatory protective mechanisms during AD pathology (Plagman et al., 2019). Cortisol mediates cognition through two types of receptors: Mineralocorticoid Receptors (MRs) and Glucocorticoid Receptors (GRs) (Joëls, 2006; Daskalakis et al., 2013). MRs have a higher affinity, 6–10 times higher than GRs (de Kloet et al., 1999; Joëls, 2006). In animal models, Enterococcus faecalis was found to promote social activity and reduce Corticosterone (CORT) levels in mice after social stress (Wu W.-L. et al., 2021); GM imbalance causes elevated CORT in AD mice (Hendrickx et al., 2021) and remodeling of the GM was able to reduce CORT levels (Liu et al., 2021). In addition, Ghrelin was found to modulate the function of hippocampal neurons and synapses in mice, thereby enhancing memory (Li et al., 2013). The above results indicate that elevated levels of stress hormones may be a potential biomarker for pre-symptomatic AD (Hendrickx et al., 2021). In humans, Sami Ouanes et al. measured cortisol and Dehydroepiandrosterone sulfate (DHEAS) levels in CSF and found that cortisol and cortisol/DHEAS ratios were positively correlated with tau and p-tau CSF levels and negatively correlated with the amygdala and insula volumes at baseline. More importantly, higher CSF cortisol and DHEAS levels predicted a more pronounced cognitive decline and disease progression over 36 months (Ouanes et al., 2022a). The higher CSF cortisol may reflect or contribute to more severe neuropsychiatric symptoms at baseline unrelated to AD pathology and more pronounced deterioration over 3 years (Ouanes et al., 2022b). These findings have important implications for identifying AD. Other studies have found reduced levels of Ghrelin messenger ribonucleic acid (mRNA) in the temporal lobe of AD patients (Gahete et al., 2010). Ghrelin may be involved in changes in neuroinflammation and cognitive function in AD (Jeon et al., 2019). These promising results further demonstrate the potential of gut hormones as biomarkers of AD.
Dysbiosis may result in the degeneration of the CNS immune response and the significant release of inflammatory cytokines that activate macrophages (Wu and Wu, 2012; Belkaid and Hand, 2014). Animal models and clinical studies have demonstrated that GM contributes to AD’s pathogenesis by regulating microglia function to involve peripheral and central inflammation processes (Bradt et al., 2000; Wu M.-L. et al., 2021). Numerous studies have demonstrated a connection between the presence and metabolism of Aβ or tau protein and the release of pro-inflammatory cytokines such as Interleukin (IL)-1, IL-6, and IL-8 by activated microglia (Teixeira et al., 2008; Breer et al., 2012; Uslu et al., 2012). IL-6 has been found to have potential properties for detecting the severity of CI in AD patients (Lai et al., 2017). Two meta-analyses found that inflammatory biomarkers such as high-sensitivity c reactive protein, transforming growth factor-beta 1 (TGF-β1), IL-1β, IL-2, IL-6, IL-12, IL-18, monocyte chemotactic protein-1 (MCP-1), MCP-3, IL-8, and interferon-γ-inducible protein 10 were consistently elevated in AD patients (Decourt et al., 2017; Su et al., 2019). The results in AD, MCI, and controls supported the view that AD and MCI are accompanied by both peripheral and CSF inflammatory responses. In addition, AD patients have higher levels of soluble tumor necrosis factor (TNF) receptor (sTNFR)-1 and sTNFR-2, which previous studies have shown to exacerbate the major pathological changes in AD (Aβ and tau pathology) (Decourt et al., 2017; Shen et al., 2019; Varesi et al., 2022a). The significant differences in peripheral inflammatory factor concentrations found in the study suggest that these markers may be useful in monitoring disease progression.
Cattaneo et al. were the first to describe patients with cerebral amyloidosis-related CI. IL-1β, C-X-C motif ligand (CXCL)-2, and NOD-like receptor family pyrin domain containing (NLRP)-3 were all positively connected with E. coli/Shigella abundance, Eubacterium rectale abundance was negatively correlated with IL-1β, CXCL-2, and NLRP3 and positively correlated with IL-10. It was also confirmed that an increased abundance of E. coli/Shigella and a decreased abundance of anti-inflammatory Rectal fungi might be associated with peripheral inflammatory status in patients with CI in brain amyloidosis (Cattaneo et al., 2017). The above study improves the robustness of microbiome-based inflammatory factors as biomarkers. Several recent studies have validated the important role of microbiome-based inflammatory biomarkers in diagnosing and detecting disease development (Shen et al., 2020; Park J.-C. et al., 2020; Park et al., 2021; Xin et al., 2021). However, larger samples and the establishment of longitudinal studies to further narrow the range of inflammatory markers remain to be addressed.
Increasing the body of evidence further validated the important role of characteristic GM, intestinal permeability, bacterial metabolites, gut hormones, and various peripheral inflammatory biomarkers in diagnosing and monitoring disease progression. But some issues need to be further addressed. First, the gut microbial species are diverse and numerous, and we have mainly focused on bacterial species; although the influence of fungi and viruses is beginning to be noted, numerous impacts and mechanisms are unclear (Ye et al., 2022). Based on the current findings, further expansion of the scope and sample size is needed to further validate the feasibility and accuracy of intestinal biomarkers in AD (Sorboni et al., 2022). Second, due to the insufficient number of countries included in the existing studies and the limitations of some animal models to which the test results can be referred (Rahman et al., 2020; Hung et al., 2022). It is not easy to obtain consistent results across various dietary, environmental, genetic, and other confounding factors (David et al., 2014; Mullane and Williams, 2020). Consider that a single biomarker may not be sufficient to describe the pathophysiology of AD fully. Finally, combining multiple markers representing different stages of disease development may be a better option (Gu et al., 2021; Zhang X. et al., 2021). Therefore, based on these issues, using intestinal biomarkers for preclinical and clinical diagnosis is still some time away. However, previous studies have focused on serum, CSF, and tissue metabolomics of AD. Because fecal biomarkers are non-invasive, readily available, and found to have great potential as biomarkers for AD, their utility can be developed in the future, perhaps as an alternative or complementary tool. Combining imaging, plasma, and other biomarkers may improve early identification rates (Mahaman et al., 2022; Sheng et al., 2022). Numerous studies have used compound biomarkers to enable more sensitive detection and early disease diagnosis. For example, diet, GM, and microRNAs can detect MCI patients (Zhang X. et al., 2021). The combination of inflammatory factors (IL-6 and interferon-γ), phosphatidylcholine, and single-chain fatty acid-producing bacteria enables early diagnosis of AD (Gu et al., 2021). Hence, gut biomarkers may be a non-invasive and cost-effective diagnostic tool for early AD screening (Table 1) summarizes the latest potential human intestinal biomarkers.
Table 1. Microbiome-based biomarkers in humans (↑: increase; ↓: decrease; ↑↑: significant increase; ↓↓; significant).
With the rapid development of genomics, metabolomics, proteomics of blood, and other assays, the human microbiome is increasingly used as a diagnostic and therapeutic biomarkers (Wirbel et al., 2021). Given the high degree of disease heterogeneity in the population, the use of artificial intelligence (AI) and machine learning (ML) to generate predictive models allows for the more precise development of individualized treatment plans (Kaur et al., 2021). The latest large-scale meta-analysis on human gut metagenomics encompasses many diseases, different sequencing technologies, and analytical tools (Wirbel et al., 2021). The investigators developed SIAMCAT, a universal R toolbox for ML-based comparative metagenomics, and demonstrated its capability in a meta-analysis of fecal macro-genomic studies (10,803 samples). Some biomarkers were found to be shared in multiple contexts (Wirbel et al., 2021). Wang et al. constructed a context-sensitive network to prioritize and identify 8,094 potential microglia-microbial metabolite-gene-pathway-phenotype interactions in AD. The study comprehensively characterized a computational approach to complex gut-microbial metabolite-microglial-gene-pathway-phenotype-brain connections in AD by innovatively bringing together the large amount of publicly available data collected (Wang et al., 2021). By identifying gut microbial metabolites and understanding their function in AD, it may be possible to gain new knowledge about the underlying mechanisms underlying AD etiology and open new avenues for AD treatment and prevention (Wang et al., 2021). Kaur et al. proposed a framework for integrating multi-omics data by understanding the epigenetic regulation of the gut-brain axis, which could further precise detection and the development of treatments for disorders of the CNS (Kaur et al., 2021).
In proteomics, a recent study proposes a framework for microbiome computation, MetaProClust-mass spectrometry (MS) 1 (Simopoulos et al., 2022). The framework can evaluate and identify hippocampal proteomic changes in a mouse model of AD after small molecule treatment, validating that MetaProClust-MS1 can be used to screen microbiome and single species proteome and extended to any proteomics experiment (Simopoulos et al., 2022). The study suggests that MetaProClust-MS1 can be used for large-scale proteomic and clinical diagnostic screening (Simopoulos et al., 2022). In addition, numerous recent studies have begun experimenting with gut-based diagnostic models for identifying AD and obtained promising results (Jung et al., 2022; Kalecký et al., 2022; Sheng et al., 2022).
Indeed, based on the solid evidence that the MGBA mediates AD pathology, GM is emerging as a potential target for treating AD (Seo et al., 2019). Recently, researchers have been targeting therapies to regulate GM in various ways, such as diet (Ghosh et al., 2020; Moreno-Arribas et al., 2020). In a review by Varesi et al., numerous promising dietary therapies were systematically reviewed, including the Mediterranean diet, Dietary Approaches to Stop Hypertension (DASH), the Mediterranean-DASH Neurodegenerative Delay Intervention diet, and the ketogenic diet, and intermitting fasting might be a promising protective dietary strategy for dementia (Park S. et al., 2020; Varesi et al., 2022b). In addition, fecal microbiota transplantation (FMT) is a method of repairing dysbiotic gut by re-cloning the normal microbiota into the “diseased” intestine (Gupta et al., 2016; Matheson and Holsinger, 2023). Although promising results such as restoration of microbiota composition, improved cognitive performance, and reduction in amyloid accumulation and tau expression have been observed in mice in several studies that have been reported (Dodiya et al., 2019; Sun et al., 2019), current studies in humans remain in the single digits. Therefore, more human studies are needed before pointing to FMT as a complementary therapy for AD. In the meantime, the researchers have tried other treatments, like probiotics (Athari Nik Azm et al., 2018; Bonfili et al., 2018), prebiotics (Nishikawa et al., 2021), nanotechnology (Li et al., 2021; Qu et al., 2022; Yang et al., 2022) and neurotherapy (He et al., 2021).
Currently, researchers are working to develop new drugs based on gut regulatory mechanisms. Sodium mannitrate (GV-971), a mixture of acidic linear oligosaccharides that inhibits intestinal microbiome dysbiosis and associated phenylalanine/isoleucine accumulation, treats neuroinflammation, and reverses CI, was approved for the first time in China for treatment of mild to moderate AD in 2019 and approved by the Food and Drug Administration (FDA) to carry out a phase III clinical study in 2020 (Syed, 2020). The approved anti-AD drugs by the FDA are relatively specific to a single target, like anti-amyloid drugs, acetylcholinesterase inhibitors, and NMDA antagonists. The difference is that traditional Chinese medicine (TCM) has multi-component, multi-target, and multi-pathway characteristics (Maimaiti et al., 2021). Some herbal medicines improve AD by modulating the GM and endogenous metabolites (Lu et al., 2019; Liu Y. et al., 2019), such as GuanXinNing ingredients, which exert an anti-AD effect by regulating Akkermansia and the dgA-11_gut_group and ameliorating GM dysbiosis (Zhang F. et al., 2021). Ginseng Radix Et Rhizoma and Poria were one of the main medication combinations used to treat dementia, according to earlier Chinese medical literature (Wang et al., 2018), and the combination with donepezil may increase the efficacy of improving cognitive levels (Lin and Chen, 2018). Danshen, Chuanxiong, and their active ingredients have neuroprotective effects (Zhou et al., 2016), respectively salvianolic acid A and salvianolic acid B, as well as ferulic acid, act by regulating the host metabolites (Li et al., 2020; Song et al., 2020). Moreover, G. elata contains gastrodin mechanisms of action, including modulation of neurotransmitters, exerting antioxidant and anti-inflammatory effects, inhibiting microglia activation, regulating mitochondrial cascades, and upregulating neurotrophic factors (Liu et al., 2018). Lanolin inhibits the cAMP-PKA-CREB-HDAC3 pathway in AD microglia and exerts anti-inflammatory effects (Zhang S. et al., 2022). This shows that some TCM has recently been gradually tried in treating AD, and their positive efficacy in animal models has been verified. We summarize recent microbial-based targeted therapies, focusing on traditional Chinese herbal formulas containing various natural ingredients (Table 2). However, it is undeniable that herbal attempts are still stuck in animal models, and the safety, as well as tolerability of the drugs, still need to be tested over a long period. Nonetheless, it still gives us a new direction for drug development to overcome this devastating disease.
GM exerts a significant role in AD pathogenesis by affecting brain function directly or indirectly. In this review, we address dysbiosis and identify the important roles of GM, permeability alterations, bacterial metabolites, gut hormones, and inflammatory factors in AD as potential preclinical and clinical biomarkers and potential target treatment effects. A single biomarker may not be enough to comprehend AD’s pathophysiology. The early and precise identification of preclinical AD and monitoring of disease progression at different stages, using the combination of different sensitive intestinal biomarkers and composite biomarkers of plasma, neuroimages, saliva, and other binding substances, may be one of the tools for a more precise diagnosis. However, the reproducibility and accuracy of gut biomarker applications remain to be addressed and need to be further validated on a larger scale and with larger sample sizes. Nevertheless, the potentially reliable intestinal biomarkers in human AD clinical studies will provide insight into the future of early, accurate, non-invasive diagnosis. Notably, applying AI and ML makes applying gut biomarkers a vast prospect. Identifying possible targeted therapeutic efficacy in herbs that modify the balance of the intestinal environment to alleviate AD pathology and enhance cognitive-behavioral symptoms provides us with optimism in the battle against AD. Future attempts at combining gut modulation with other drugs for AD pathogenesis may yield new and surprising efficacy and provide new ideas for future AD treatment.
YZ and MA-N conception and literature review. CoD writing–original draft and illustrated paintings. LZ and ChD supervised and edited the manuscript. All authors contributed to the article and approved the submitted version.
The authors declare that the research was conducted in the absence of any commercial or financial relationships that could be construed as a potential conflict of interest.
All claims expressed in this article are solely those of the authors and do not necessarily represent those of their affiliated organizations, or those of the publisher, the editors and the reviewers. Any product that may be evaluated in this article, or claim that may be made by its manufacturer, is not guaranteed or endorsed by the publisher.
The Supplementary material for this article can be found online at: https://www.frontiersin.org/articles/10.3389/fnins.2023.1130730/full#supplementary-material
2022 Alzheimer’s disease facts and figures (2022). 2022 Alzheimer’s disease facts and figures. Alzheimers Dement 18, 700–789. doi: 10.1002/alz.12638
Abdel-Haq, R., Schlachetzki, J. C. M., Glass, C. K., and Mazmanian, S. K. (2019). Microbiome–microglia connections via the gut–brain axis. J. Exp. Med. 216, 41–59. doi: 10.1084/jem.20180794
ADI-Dementia Statistics (2022). Alzheimer’s disease international. Available at: https://www.alzint.org/about/dementia-facts-figures/dementia-statistics/
Akingbade, O. E. S., Gibson, C., Kalaria, R. N., and Mukaetova-Ladinska, E. B. (2018). Platelets: peripheral biomarkers of dementia? J. Alzheimers Dis. 63, 1235–1259. doi: 10.3233/JAD-180181
Albuquerque, M. S., Mahar, I., Davoli, M. A., Chabot, J.-G., Mechawar, N., Quirion, R., et al. (2015). Regional and sub-regional differences in hippocampal GABAergic neuronal vulnerability in the TgCRND8 mouse model of Alzheimer’s disease. Front. Aging Neurosci. 7:30. doi: 10.3389/fnagi.2015.00030
Allam-Ndoul, B., Castonguay-Paradis, S., and Veilleux, A. (2020). Gut microbiota and intestinal trans-epithelial permeability. Int. J. Mol. Sci. 21:6402. doi: 10.3390/ijms21176402
Arrona Cardoza, P., Spillane, M. B., and Morales Marroquin, E. (2022). Alzheimer’s disease and gut microbiota: does trimethylamine N-oxide (TMAO) play a role? Nutr. Rev. 80, 271–281. doi: 10.1093/nutrit/nuab022
Asti, A., and Gioglio, L. (2014). Can a bacterial endotoxin be a key factor in the kinetics of amyloid fibril formation? J. Alzheimers Dis. 39, 169–179. doi: 10.3233/JAD-131394
Athari Nik Azm, S., Djazayeri, A., Safa, M., Azami, K., Ahmadvand, B., Sabbaghziarani, F., et al. (2018). Lactobacilli and bifidobacteria ameliorate memory and learning deficits and oxidative stress in β-amyloid (1–42) injected rats. Appl. Physiol. Nutr. Metab. 43, 718–726. doi: 10.1139/apnm-2017-0648
Bairamian, D., Sha, S., Rolhion, N., Sokol, H., Dorothée, G., Lemere, C. A., et al. (2022). Microbiota in neuroinflammation and synaptic dysfunction: a focus on Alzheimer’s disease. Mol. Neurodegener. 17:19. doi: 10.1186/s13024-022-00522-2
Barichello, T., Giridharan, V. V., and Dal-Pizzol, F. (2019). A cerebrospinal fluid biosignature for the diagnosis of Alzheimer’s disease. Braz. J. Psychiatry 41, 467–468. doi: 10.1590/1516-4446-2019-0629
Barrett, E., Ross, R. P., O’Toole, P. W., Fitzgerald, G. F., and Stanton, C. (2012). γ-Aminobutyric acid production by culturable bacteria from the human intestine. J. Appl. Microbiol. 113, 411–417. doi: 10.1111/j.1365-2672.2012.05344.x
Belkaid, Y., and Hand, T. (2014). Role of the microbiota in immunity and inflammation. Cells 157, 121–141. doi: 10.1016/j.cell.2014.03.011
Bello-Medina, P. C., Hernández-Quiroz, F., Pérez-Morales, M., González-Franco, D. A., Cruz-Pauseno, G., García-Mena, J., et al. (2021). Spatial memory and gut microbiota alterations are already present in early adulthood in a pre-clinical transgenic model of Alzheimer’s disease. Front. Neurosci. 15:595583. doi: 10.3389/fnins.2021.595583
Blevins, J. E., Stanley, B. G., and Reidelberger, R. D. (2000). Brain regions where cholecystokinin suppresses feeding in rats. Brain Res. 860, 1–10. doi: 10.1016/S0006-8993(99)02477-4
Bolduc, J.-F., Hany, L., Barat, C., Ouellet, M., and Tremblay, M. J. (2017). Epigenetic metabolite acetate inhibits class I/II histone Deacetylases, promotes histone acetylation, and increases HIV-1 integration in CD4+ T cells. J. Virol. 91, e01943–e01916. doi: 10.1128/JVI.01943-16
Bonfili, L., Cecarini, V., Cuccioloni, M., Angeletti, M., Berardi, S., Scarpona, S., et al. (2018). SLAB51 probiotic formulation activates SIRT1 pathway promoting antioxidant and Neuroprotective effects in an AD mouse model. Mol. Neurobiol. 55, 7987–8000. doi: 10.1007/s12035-018-0973-4
Braak, H., and Braak, E. (1991). Neuropathological stageing of Alzheimer-related changes. Acta Neuropathol. 82, 239–259. doi: 10.1007/BF00308809
Bradshaw, E. M., Chibnik, L. B., Keenan, B. T., Ottoboni, L., Raj, T., Tang, A., et al. (2013). CD33 Alzheimer’s disease locus: altered monocyte function and amyloid biology. Nat. Neurosci. 16, 848–850. doi: 10.1038/nn.3435
Bradt, B., Bauer, J., Cole, G. M., Cooper, N. R., Eikelenboom, P., Emmerling, M., et al. (2000). Inflammation and Alzheimer’s disease inflammation and Alzheimer’s disease. Neurobiol. Aging 39, 383–421. doi: 10.1016/s0197-4580(00)00124-x
Braniste, V., Al-Asmakh, M., Kowal, C., Anuar, F., Abbaspour, A., Tóth, M., et al. (2014). The gut microbiota influences blood-brain barrier permeability in mice. Sci. Transl. Med. 6:263ra158. doi: 10.1126/scitranslmed.3009759
Bravo, J. A., Forsythe, P., Chew, M. V., Escaravage, E., Savignac, H. M., Dinan, T. G., et al. (2011). Ingestion of lactobacillus strain regulates emotional behavior and central GABA receptor expression in a mouse via the vagus nerve. Proc. Natl. Acad. Sci. U. S. A. 108, 16050–16055. doi: 10.1073/pnas.1102999108
Breer, H., Eberle, J., Frick, C., Haid, D., and Widmayer, P. (2012). Gastrointestinal chemosensation: chemosensory cells in the alimentary tract. Histochem. Cell Biol. 138, 13–24. doi: 10.1007/s00418-012-0954-z
Breit, S., Kupferberg, A., Rogler, G., and Hasler, G. (2018). Vagus nerve as modulator of the brain–gut Axis in psychiatric and inflammatory disorders. Front. Psych. 9:44. doi: 10.3389/fpsyt.2018.00044
Butterfield, D. A., and Halliwell, B. (2019). Oxidative stress, dysfunctional glucose metabolism, and Alzheimer disease. Nat. Rev. Neurosci. 20, 148–160. doi: 10.1038/s41583-019-0132-6
Calderon, M. R., Mori, M., Kauwe, G., Farnsworth, J., Ulian-Benitez, S., Maksoud, E., et al. (2022). Delta/notch signaling in glia maintains motor nerve barrier function and synaptic transmission by controlling matrix metalloproteinase expression. Proc. Natl. Acad. Sci. U. S. A. 119:e2110097119. doi: 10.1073/pnas.2110097119
Cani, P. D., Bibiloni, R., Knauf, C., Waget, A., Neyrinck, A. M., Delzenne, N. M., et al. (2008). Changes in gut microbiota control metabolic Endotoxemia-induced inflammation in high-fat diet–induced obesity and diabetes in mice. Diabetes 57, 1470–1481. doi: 10.2337/db07-1403
Cattaneo, A., Cattane, N., Galluzzi, S., Provasi, S., Lopizzo, N., Festari, C., et al. (2017). Association of brain amyloidosis with pro-inflammatory gut bacterial taxa and peripheral inflammation markers in cognitively impaired elderly. Neurobiol. Aging 49, 60–68. doi: 10.1016/j.neurobiolaging.2016.08.019
Chen, Y., Fang, L., Chen, S., Zhou, H., Fan, Y., Lin, L., et al. (2020). Gut microbiome alterations precede cerebral amyloidosis and microglial pathology in a mouse model of Alzheimer’s disease. Biomed. Res. Int. 2020:8456596. doi: 10.1155/2020/8456596
Chen, H., Meng, L., and Shen, L. (2022). Multiple roles of short-chain fatty acids in Alzheimer disease. Nutrition 93:111499. doi: 10.1016/j.nut.2021.111499
Chen, C., Zhou, Y., Wang, H., Alam, A., Kang, S. S., Ahn, E. H., et al. (2021). Gut inflammation triggers C/EBPβ/δ-secretase-dependent gut-to-brain propagation of Aβ and tau fibrils in Alzheimer’s disease. EMBO J. 40:e106320. doi: 10.15252/embj.2020106320
Chidambaram, S. B., Essa, M. M., Rathipriya, A. G., Bishir, M., Ray, B., Mahalakshmi, A. M., et al. (2022). Gut dysbiosis, defective autophagy and altered immune responses in neurodegenerative diseases: Tales of a vicious cycle. Pharmacol. Ther. 231:107988. doi: 10.1016/j.pharmthera.2021.107988
Cirstea, M. S., Kliger, D., Mac Lellan, A. D., Yu, A. C., Langlois, J., Fan, M., et al. (2022). The Oral and fecal microbiota in a Canadian cohort of Alzheimer’s disease. J. Alzheimers Dis. 87, 247–258. doi: 10.3233/JAD-215520
Colombo, A. V., Sadler, R. K., Llovera, G., Singh, V., Roth, S., Heindl, S., et al. (2021). Microbiota-derived short chain fatty acids modulate microglia and promote Aβ plaque deposition. eLife 10:e59826. doi: 10.7554/eLife.59826
Connell, E., Le Gall, G., Pontifex, M. G., Sami, S., Cryan, J. F., Clarke, G., et al. (2022). Microbial-derived metabolites as a risk factor of age-related cognitive decline and dementia. Mol. Neurodegener. 17:43. doi: 10.1186/s13024-022-00548-6
Crews, L., and Masliah, E. (2010). Molecular mechanisms of neurodegeneration in Alzheimer’s disease. Hum. Mol. Genet. 19, R12–R20. doi: 10.1093/hmg/ddq160
Cryan, J. F., O’Riordan, K. J., Cowan, C. S. M., Sandhu, K. V., Bastiaanssen, T. F. S., Boehme, M., et al. (2019). The microbiota-gut-brain Axis. Physiol. Rev. 99, 1877–2013. doi: 10.1152/physrev.00018.2018
de Kloet, E. R., Oitzl, M. S., and Joëls, M. (1999). Stress and cognition: are corticosteroids good or bad guys?. Trends in Neurosciences. 22: 422–426. doi: 10.1016/S0166-2236(99)01438-1
D’Argenio, V., Veneruso, I., Gong, C., Cecarini, V., Bonfili, L., and Eleuteri, A. M. (2022). Gut microbiome and Mycobiome alterations in an in vivo model of Alzheimer’s disease. Genes 13:1564. doi: 10.3390/genes13091564
Daniilidou, M., Koutroumani, M., and Tsolaki, M. (2011). Epigenetic mechanisms in Alzheimer’s disease. Curr. Med. Chem. 18, 1751–1756. doi: 10.2174/092986711795496872
Das, T. K., Blasco-Conesa, M. P., Korf, J., Honarpisheh, P., Chapman, M. R., and Ganesh, B. P. (2022). Bacterial amyloid Curli associated gut epithelial neuroendocrine activation predominantly observed in Alzheimer’s disease mice with central amyloid-β pathology. J. Alzheimers Dis. 88, 191–205. doi: 10.3233/JAD-220106
Daskalakis, N. P., Lehrner, A., and Yehuda, R. (2013). Endocrine aspects of post-traumatic stress disorder and implications for diagnosis and treatment. Endocrinol. Metab. Clin. N. Am. 42, 503–513. doi: 10.1016/j.ecl.2013.05.004
David, L. A., Maurice, C. F., Carmody, R. N., Gootenberg, D. B., Button, J. E., Wolfe, B. E., et al. (2014). Diet rapidly and reproducibly alters the human gut microbiome. Nature 505, 559–563. doi: 10.1038/nature12820
Decourt, B., Lahiri, D. K., and Sabbagh, M. N. (2017). Targeting tumor necrosis factor alpha for Alzheimer’s disease. Curr. Alzheimer Res. 14, 412–425. doi: 10.2174/1567205013666160930110551
Dementia (2022). World Health Organization. Available at: https://www.who.int/news-room/fact-sheets/detail/dementia.
Dodiya, H. B., Kuntz, T., Shaik, S. M., Baufeld, C., Leibowitz, J., Zhang, X., et al. (2019). Sex-specific effects of microbiome perturbations on cerebral Aβ amyloidosis and microglia phenotypes. J. Exp. Med. 216, 1542–1560. doi: 10.1084/jem.20182386
Dupraz, L., Magniez, A., Rolhion, N., Richard, M. L., Da Costa, G., Touch, S., et al. (2021). Gut microbiota-derived short-chain fatty acids regulate IL-17 production by mouse and human intestinal γδ T cells. Cell Rep. 36:109332. doi: 10.1016/j.celrep.2021.109332
Engevik, M. A., Luck, B., Visuthranukul, C., Ihekweazu, F. D., Engevik, A. C., Shi, Z., et al. (2020). Human-derived Bifidobacterium dentium modulates the mammalian serotonergic system and gut–brain Axis. Cell. Mol. Gastroenterol. Hepatol. 11, 221–248. doi: 10.1016/j.jcmgh.2020.08.002
Erny, D., Hrabě de Angelis, A. L., Jaitin, D., Wieghofer, P., Staszewski, O., David, E., et al. (2015). Host microbiota constantly control maturation and function of microglia in the CNS. Nat. Neurosci. 18, 965–977. doi: 10.1038/nn.4030
Esposito, M., and Sherr, G. L. (2019). Epigenetic modifications in Alzheimer’s neuropathology and therapeutics. Front. Neurosci. 13:476. doi: 10.3389/fnins.2019.00476
Eyo, U. B., and Wu, L.-J. (2013). Bidirectional microglia-neuron communication in the healthy brain. Neural Plast. 2013, 1–10. doi: 10.1155/2013/456857
Farr, O. M., Tsoukas, M. A., and Mantzoros, C. S. (2015). Leptin and the brain: influences on brain development, cognitive functioning and psychiatric disorders. Metabolism 64, 114–130. doi: 10.1016/j.metabol.2014.07.004
Fasina, O. B., Wang, J., Mo, J., Osada, H., Ohno, H., Pan, W., et al. (2022). Gastrodin from Gastrodia elata enhances cognitive function and Neuroprotection of AD mice via the regulation of gut microbiota composition and inhibition of neuron inflammation. Front. Pharmacol. 13:814271. doi: 10.3389/fphar.2022.814271
Feng, M., Hou, T., Zhou, M., Cen, Q., Yi, T., Bai, J., et al. (2022). Gut microbiota may be involved in Alzheimer’s disease pathology by dysregulating pyrimidine metabolism in APP/PS1 mice. Front. Aging Neurosci. 14:967747. doi: 10.3389/fnagi.2022.967747
Fernandez-Real, J.-M., Serino, M., Blasco, G., Puig, J., Daunis-i-Estadella, J., Ricart, W., et al. (2015). Gut microbiota interacts with brain microstructure and function. J. Clin. Endocrinol. Metabol. 100, 4505–4513. doi: 10.1210/jc.2015-3076
Fewlass, D. C., Noboa, K., Pi-Sunyer, F. X., Johnston, J. M., Yan, S. D., and Tezapsidis, N. (2004). Obesity-related leptin regulates Alzheimer’s Aβ. FASEB J. 18, 1870–1878. doi: 10.1096/fj.04-2572com
Friedland, R. P., and Chapman, M. R. (2017). The role of microbial amyloid in neurodegeneration. PLoS Pathog. 13:e1006654. doi: 10.1371/journal.ppat.1006654
Fülling, C., Dinan, T. G., and Cryan, J. F. (2019). Gut microbe to brain signaling: what happens in Vagus…. Neuron 101, 998–1002. doi: 10.1016/j.neuron.2019.02.008
Gahete, M. D., Rubio, A., Córdoba-Chacón, J., Gracia-Navarro, F., Kineman, R. D., Avila, J., et al. (2010). Expression of the ghrelin and Neurotensin systems is altered in the temporal lobe of Alzheimer’s disease patients. J. Alzheimers Dis. 22, 819–828. doi: 10.3233/JAD-2010-100873
Galipeau, H. J., and Verdu, E. F. (2016). The complex task of measuring intestinal permeability in basic and clinical science. Neurogastroenterol. Motil. 28:9. doi: 10.1111/nmo.12871
Ghosh, T. S., Rampelli, S., Jeffery, I. B., Santoro, A., Neto, M., Capri, M., et al. (2020). Mediterranean diet intervention alters the gut microbiome in older people reducing frailty and improving health status: the NU-AGE 1-year dietary intervention across five European countries. Gut 69, 1218–1228. doi: 10.1136/gutjnl-2019-319654
Glinert, A., Turjeman, S., Elliott, E., and Koren, O. (2022). Microbes, metabolites and (synaptic) malleability, oh my! The effect of the microbiome on synaptic plasticity. Biol. Rev. Camb. Philos. Soc. 97, 582–599. doi: 10.1111/brv.12812
Goehler, L. E., Gaykema, R. P. A., Opitz, N., Reddaway, R., Badr, N., and Lyte, M. (2005). Activation in vagal afferents and central autonomic pathways: early responses to intestinal infection with campylobacter jejuni. Brain Behav. Immun. 19, 334–344. doi: 10.1016/j.bbi.2004.09.002
Grenham, S., Clarke, G., Cryan, J. F., and Dinan, T. G. (2011). Brain? Gut? Microbe communication in health and disease. Front. Physio. 2:94. doi: 10.3389/fphys.2011.00094
Gu, X., Zhou, J., Zhou, Y., Wang, H., Si, N., Ren, W., et al. (2021). Huanglian Jiedu decoction remodels the periphery microenvironment to inhibit Alzheimer’s disease progression based on the brain-gut axis through multiple integrated omics. Alz. Res. Therapy 13:44. doi: 10.1186/s13195-021-00779-7
Gue, M., Junien, J. L., and Bueno, L. (1991). Conditioned emotional response in rats enhances colonic motility through the central release of corticotropin-releasing factor. Gastroenterology 100, 964–970. doi: 10.1016/0016-5085(91)90270-U
Guerreiro, R., Wojtas, A., Bras, J., Carrasquillo, M., Rogaeva, E., Majounie, E., et al. (2013). TREM2 variants in Alzheimer’s disease. N. Engl. J. Med. 368, 117–127. doi: 10.1056/NEJMoa1211851
Guo, M., Peng, J., Huang, X., Xiao, L., Huang, F., and Zuo, Z. (2021). Gut microbiome features of Chinese patients newly diagnosed with Alzheimer’s disease or mild cognitive impairment. J. Alzheimers Dis. 80, 299–310. doi: 10.3233/JAD-201040
Gupta, S., Allen-Vercoe, E., and Petrof, E. O. (2016). Fecal microbiota transplantation: in perspective. Ther. Adv. Gastroenterol. 9, 229–239. doi: 10.1177/1756283X15607414
Hakansson, A., and Molin, G. (2011). Gut microbiota and inflammation. Nutrients 3, 637–682. doi: 10.3390/nu3060637
He, C., Huang, Z.-S., Yu, C.-C., Wang, X.-S., Jiang, T., Wu, M., et al. (2021). Preventive electroacupuncture ameliorates D-galactose-induced Alzheimer’s disease-like inflammation and memory deficits, probably via modulating the microbiota–gut–brain axis. Iran. J. Basic Med. Sci. 24, 341–348. doi: 10.22038/ijbms.2021.49147.11256
Hendrickx, J. O., De Moudt, S., Calus, E., Martinet, W., Guns, P.-J. D. F., Roth, L., et al. (2021). Serum Corticosterone and insulin resistance as early biomarkers in the hAPP23 overexpressing mouse model of Alzheimer’s disease. Int. J. Mol. Sci. 22:6656. doi: 10.3390/ijms22136656
Heneka, M. T., Carson, M. J., El Khoury, J., Landreth, G. E., Brosseron, F., Feinstein, D. L., et al. (2015). Neuroinflammation in Alzheimer’s disease. Lancet Neurol. 14, 388–405. doi: 10.1016/S1474-4422(15)70016-5
Hill, J. M., and Lukiw, W. J. (2015). Microbial-generated amyloids and Alzheimer’s disease (AD). Front. Aging Neurosci. 7:9. doi: 10.3389/fnagi.2015.00009
Hossain, S., Beydoun, M. A., Kuczmarski, M. F., Tajuddin, S., Evans, M. K., and Zonderman, A. B. (2019). The interplay of diet quality and Alzheimer’s disease genetic risk score in relation to cognitive performance among urban African Americans. Nutrients 11:2181. doi: 10.3390/nu11092181
Hoyles, L., Snelling, T., Umlai, U.-K., Nicholson, J. K., Carding, S. R., Glen, R. C., et al. (2018). Microbiome–host systems interactions: protective effects of propionate upon the blood–brain barrier. Microbiome 6:55. doi: 10.1186/s40168-018-0439-y
Hsiao, E. Y., McBride, S. W., Hsien, S., Sharon, G., Hyde, E. R., McCue, T., et al. (2013). Microbiota modulate behavioral and physiological abnormalities associated with neurodevelopmental disorders. Cells 155, 1451–1463. doi: 10.1016/j.cell.2013.11.024
Huang, Y., Wu, J., Zhang, H., Li, Y., Wen, L., Tan, X., et al. (2023). The gut microbiome modulates the transformation of microglial subtypes. Mol. Psychiatry. doi: 10.1038/s41380-023-02017-y [Online ahead of print].
Hung, C.-C., Chang, C.-C., Huang, C.-W., Nouchi, R., and Cheng, C.-H. (2022). Gut microbiota in patients with Alzheimer’s disease spectrum: a systematic review and meta-analysis. Aging (Albany NY) 14, 477–496. doi: 10.18632/aging.203826
Jack, C. R., Bennett, D. A., Blennow, K., Carrillo, M. C., Dunn, B., Haeberlein, S. B., et al. (2018). NIA-AA research framework: toward a biological definition of Alzheimer’s disease. Alzheimer’s Dementia 14, 535–562. doi: 10.1016/j.jalz.2018.02.018
Jeon, S. G., Hong, S. B., Nam, Y., Tae, J., Yoo, A., Song, E. J., et al. (2019). Ghrelin in Alzheimer’s disease: pathologic roles and therapeutic implications. Ageing Res. Rev. 55:100945. doi: 10.1016/j.arr.2019.100945
Jeong, M.-Y., Jang, H.-M., and Kim, D.-H. (2019). High-fat diet causes psychiatric disorders in mice by increasing Proteobacteria population. Neurosci. Lett. 698, 51–57. doi: 10.1016/j.neulet.2019.01.006
Jiang, Y., Li, K., Li, X., Xu, L., and Yang, Z. (2021). Sodium butyrate ameliorates the impairment of synaptic plasticity by inhibiting the neuroinflammation in 5XFAD mice. Chem. Biol. Interact. 341:109452. doi: 10.1016/j.cbi.2021.109452
Jo, J.-K., Lee, G., Nguyen, C. D., Park, S.-E., Kim, E.-J., Kim, H.-W., et al. (2022). Effects of donepezil treatment on brain metabolites, gut microbiota, and gut metabolites in an amyloid Beta-induced cognitive impairment mouse pilot model. Molecules 27:6591. doi: 10.3390/molecules27196591
Joëls, M. (2006). Corticosteroid effects in the brain: U-shape it. Trends Pharmacol. Sci. 27, 244–250. doi: 10.1016/j.tips.2006.03.007
Jonsson, T., Stefansson, H., Steinberg, S., Jonsdottir, I., Jonsson, P. V., Snaedal, J., et al. (2013). Variant of TREM2 associated with the risk of Alzheimer’s disease. N. Engl. J. Med. 368, 107–116. doi: 10.1056/NEJMoa1211103
Jung, J. H., Kim, G., Byun, M. S., Lee, J. H., Yi, D., Park, H., et al. (2022). Gut microbiome alterations in preclinical Alzheimer’s disease. PLoS One 17:e0278276. doi: 10.1371/journal.pone.0278276
Jungbauer, G., Stähli, A., Zhu, X., Auber Alberi, L., Sculean, A., and Eick, S. (2022). Periodontal microorganisms and Alzheimer disease – a causative relationship? Periodontology 2000, 59–82. doi: 10.1111/prd.12429
Kaddurah-Daouk, R., Zhu, H., Sharma, S., Bogdanov, M., Rozen, S. G., Matson, W., et al. (2013). Alterations in metabolic pathways and networks in Alzheimer’s disease. Transl. Psychiatry 3:e244. doi: 10.1038/tp.2013.18
Kaelberer, M. M., Buchanan, K. L., Klein, M. E., Barth, B. B., Montoya, M. M., Shen, X., et al. (2018). A gut-brain neural circuit for nutrient sensory transduction. Science 361:eaat5236. doi: 10.1126/science.aat5236
Kahn, M. S., Kranjac, D., Alonzo, C. A., Haase, J. H., Cedillos, R. O., McLinden, K. A., et al. (2012). Prolonged elevation in hippocampal Aβ and cognitive deficits following repeated endotoxin exposure in the mouse. Behav. Brain Res. 229, 176–184. doi: 10.1016/j.bbr.2012.01.010
Kalecký, K., German, D. C., Montillo, A. A., and Bottiglieri, T. (2022). Targeted Metabolomic analysis in Alzheimer’s disease plasma and brain tissue in non-Hispanic whites. J. Alzheimers Dis. 86, 1875–1895. doi: 10.3233/JAD-215448
Kaur, H., Singh, Y., Singh, S., and Singh, R. B. (2021). Gut microbiome-mediated epigenetic regulation of brain disorder and application of machine learning for multi-omics data analysis. Genome 64, 355–371. doi: 10.1139/gen-2020-0136
Kempuraj, D., Thangavel, R., Selvakumar, G. P., Zaheer, S., Ahmed, M. E., Raikwar, S. P., et al. (2017). Brain and peripheral atypical inflammatory mediators potentiate Neuroinflammation and Neurodegeneration. Front. Cell. Neurosci. 11:216. doi: 10.3389/fncel.2017.00216
Keren-Shaul, H., Spinrad, A., Weiner, A., Matcovitch-Natan, O., Dvir-Szternfeld, R., Ulland, T. K., et al. (2017). A unique microglia type associated with restricting development of Alzheimer’s disease. Cells 169, 1276–1290.e17. doi: 10.1016/j.cell.2017.05.018
Kitazawa, M., Oddo, S., Yamasaki, T. R., Green, K. N., and LaFerla, F. M. (2005). Lipopolysaccharide-induced inflammation exacerbates tau pathology by a Cyclin-dependent kinase 5-mediated pathway in a transgenic model of Alzheimer’s disease. J. Neurosci. 25, 8843–8853. doi: 10.1523/JNEUROSCI.2868-05.2005
Kowalski, K., and Mulak, A. (2019). Brain-gut-microbiota Axis in Alzheimer’s disease. J. Neurogastroenterol. Motil. 25, 48–60. doi: 10.5056/jnm18087
Kumar, M., Babaei, P., Ji, B., and Nielsen, J. (2016). Human gut microbiota and healthy aging: recent developments and future prospective. Nutr. Healthy Aging 4, 3–16. doi: 10.3233/NHA-150002
La Rosa, F., Clerici, M., Ratto, D., Occhinegro, A., Licito, A., Romeo, M., et al. (2018). The gut-brain Axis in Alzheimer’s disease and Omega-3. A critical overview of clinical trials. Nutrients 10:1267. doi: 10.3390/nu10091267
Lai, K. S. P., Liu, C. S., Rau, A., Lanctôt, K. L., Köhler, C. A., Pakosh, M., et al. (2017). Peripheral inflammatory markers in Alzheimer’s disease: a systematic review and meta-analysis of 175 studies. J. Neurol. Neurosurg. Psychiatry 88, 876–882. doi: 10.1136/jnnp-2017-316201
Laugisch, O., Johnen, A., Maldonado, A., Ehmke, B., Bürgin, W., Olsen, I., et al. (2018). Periodontal pathogens and associated Intrathecal antibodies in early stages of Alzheimer’s disease. J. Alzheimers Dis. 66, 105–114. doi: 10.3233/JAD-180620
Leng, F., and Edison, P. (2021). Neuroinflammation and microglial activation in Alzheimer disease: where do we go from here? Nat. Rev. Neurol. 17, 157–172. doi: 10.1038/s41582-020-00435-y
Lepeta, K., Lourenco, M. V., Schweitzer, B. C., Martino Adami, P. V., Banerjee, P., Catuara-Solarz, S., et al. (2016). Synaptopathies: synaptic dysfunction in neurological disorders – a review from students to students. J. Neurochem. 138, 785–805. doi: 10.1111/jnc.13713
Li, E., Chung, H., Kim, Y., Kim, D. H., Ryu, J. H., Sato, T., et al. (2013). Ghrelin directly stimulates adult hippocampal neurogenesis: implications for learning and memory. Endocr. J. 60, 781–789. doi: 10.1507/endocrj.EJ13-0008
Li, B., He, Y., Ma, J., Huang, P., Du, J., Cao, L., et al. (2019). Mild cognitive impairment has similar alterations as Alzheimer’s disease in gut microbiota. Alzheimers Dement. 15, 1357–1366. doi: 10.1016/j.jalz.2019.07.002
Li, X., Kiprowska, M., Kansara, T., Kansara, P., and Li, P. (2022). Neuroinflammation: a distal consequence of periodontitis. J. Dent. Res. 101, 1441–1449. doi: 10.1177/00220345221102084
Li, L., Li, R., Zhu, R., Chen, B., Tian, Y., Zhang, H., et al. (2020). Salvianolic acid B prevents body weight gain and regulates gut microbiota and LPS/TLR4 signaling pathway in high-fat diet-induced obese mice. Food Funct. 11, 8743–8756. doi: 10.1039/D0FO01116A
Li, C., Wang, N., Zheng, G., and Yang, L. (2021). Oral Administration of Resveratrol-Selenium-Peptide Nanocomposites Alleviates Alzheimer’s disease-like pathogenesis by inhibiting Aβ aggregation and regulating gut microbiota. ACS Appl. Mater. Interfaces 13, 46406–46420. doi: 10.1021/acsami.1c14818
Lin, D., and Chen, Z. (2018). A clinical study on treating AD with Kaixin san plus donepezil hydrochlo tablets (in Chinese). Clin. J. Chin. Med. 10, 73–75.
Ling, Z., Zhu, M., Yan, X., Cheng, Y., Shao, L., Liu, X., et al. (2021). Structural and functional Dysbiosis of fecal microbiota in Chinese patients with Alzheimer’s disease. Front. Cell Dev. Biol. 8:634069. doi: 10.3389/fcell.2020.634069
Liu, Y., Du, T., Zhang, W., Lu, W., Peng, Z., Huang, S., et al. (2019). Modified Huang-Lian-Jie-Du decoction ameliorates Aβ Synaptotoxicity in a murine model of Alzheimer’s disease. Oxid. Med. Cell. Longev. 2019:8340192. doi: 10.1155/2019/8340192
Liu, Y., Gao, J., Peng, M., Meng, H., Ma, H., Cai, P., et al. (2018). A review on central nervous system effects of Gastrodin. Front. Pharmacol. 9:24. doi: 10.3389/fphar.2018.00024
Liu, S., Gao, J., Zhu, M., Liu, K., and Zhang, H.-L. (2020). Gut microbiota and Dysbiosis in Alzheimer’s disease: implications for pathogenesis and treatment. Mol. Neurobiol. 57, 5026–5043. doi: 10.1007/s12035-020-02073-3
Liu, P., Wu, L., Peng, G., Han, Y., Tang, R., Ge, J., et al. (2019). Altered microbiomes distinguish Alzheimer’s disease from amnestic mild cognitive impairment and health in a Chinese cohort. Brain Behav. Immun. 80, 633–643. doi: 10.1016/j.bbi.2019.05.008
Liu, Q., Xi, Y., Wang, Q., Liu, J., Li, P., Meng, X., et al. (2021). Mannan oligosaccharide attenuates cognitive and behavioral disorders in the 5xFAD Alzheimer’s disease mouse model via regulating the gut microbiota-brain axis. Brain Behav. Immun. 95, 330–343. doi: 10.1016/j.bbi.2021.04.005
Liu, G., Yu, Q., Tan, B., Ke, X., Zhang, C., Li, H., et al. (2022). Gut dysbiosis impairs hippocampal plasticity and behaviors by remodeling serum metabolome. Gut Microbes 14:2104089. doi: 10.1080/19490976.2022.2104089
Lu, J., Guo, P., Liu, X., Zhang, Y., Guo, X., Gao, X., et al. (2019). Herbal formula Fo Shou san attenuates Alzheimer’s disease-related pathologies via the gut-liver-brain Axis in APP/PS1 mouse model of Alzheimer’s disease. Evid. Based Complement. Alternat. Med. 2019, 1–14. doi: 10.1155/2019/8302950
Lucidi, L., Pettorruso, M., Vellante, F., Di Carlo, F., Ceci, F., Santovito, M. C., et al. (2021). Gut microbiota and bipolar disorder: an overview on a novel biomarker for diagnosis and treatment. Int. J. Mol. Sci. 22:3723. doi: 10.3390/ijms22073723
Luczynski, P., Neufeld, K.-A. M., Oriach, C. S., and Clarke, G. (2016). Growing up in a bubble: using germ-free animals to assess the influence of the gut microbiota on brain and behaviour. Int. J. Neuropsychopharmacol. 19:57. doi: 10.1093/ijnp/pyw020
Mahaman, Y. A. R., Embaye, K. S., Huang, F., Li, L., Zhu, F., Wang, J.-Z., et al. (2022). Biomarkers used in Alzheimer’s disease diagnosis, treatment, and prevention. Ageing Res. Rev. 74:101544. doi: 10.1016/j.arr.2021.101544
Maimaiti, A., Tao, Y., Minmin, W., Weiwei, M., Wenhui, S., Aikemu, A., et al. (2021). Improvement of Total flavonoids from Dracocephalum moldavica L. in rats with Chronic Mountain sickness through 1H-NMR Metabonomics. Evid. Based Complement. Alternat. Med. 2021, 1–13. doi: 10.1155/2021/6695346
Marizzoni, M., Cattaneo, A., Mirabelli, P., Festari, C., Lopizzo, N., Nicolosi, V., et al. (2020). Short-chain fatty acids and lipopolysaccharide as mediators between gut Dysbiosis and amyloid pathology in Alzheimer’s disease. J. Alzheimers Dis. 78, 683–697. doi: 10.3233/JAD-200306
Maslowski, K. M., and Mackay, C. R. (2011). Diet, gut microbiota and immune responses. Nat. Immunol. 12, 5–9. doi: 10.1038/ni0111-5
Matheson, J.-A. T., and Holsinger, R. M. D. (2023). The role of fecal microbiota transplantation in the treatment of neurodegenerative diseases: a review. Int. J. Mol. Sci. 24:1001. doi: 10.3390/ijms24021001
Mayeux, R. (2004). Biomarkers: potential uses and limitations. NeuroRx 1, 182–188. doi: 10.1602/neurorx.1.2.182
Megur, A., Baltriukienė, D., Bukelskienė, V., and Burokas, A. (2020). The microbiota–gut–brain Axis and Alzheimer’s disease: neuroinflammation is to blame? Nutrients 13:37. doi: 10.3390/nu13010037
Ménard, S., Cerf-Bensussan, N., and Heyman, M. (2010). Multiple facets of intestinal permeability and epithelial handling of dietary antigens. Mucosal Immunol. 3, 247–259. doi: 10.1038/mi.2010.5
Montoro, R. A., Singh, A. P., and Yu, J.-P. J. (2022). Structural and functional neuroimaging of the effects of the gut microbiome. Eur. Radiol. 32, 3683–3692. doi: 10.1007/s00330-021-08486-5
Moreno-Arribas, M. V., Bartolomé, B., Peñalvo, J. L., Pérez-Matute, P., and Motilva, M. J. (2020). Relationship between wine consumption, diet and microbiome modulation in Alzheimer’s disease. Nutrients 12:3082. doi: 10.3390/nu12103082
Moussaoui, N., Braniste, V., Ait-Belgnaoui, A., Gabanou, M., Sekkal, S., Olier, M., et al. (2014). Changes in intestinal glucocorticoid sensitivity in early life shape the risk of epithelial barrier defect in maternal-deprived rats. PLoS One 9:e88382. doi: 10.1371/journal.pone.0088382
Mukaetova-Ladinska, E. B., Abdel-All, Z., Dodds, S., Andrade, J., Alves da Silva, J., Kalaria, R. N., et al. (2012). Platelet immunoglobulin and amyloid precursor protein as potential peripheral biomarkers for Alzheimer’s disease: findings from a pilot study. Age Ageing 41, 408–412. doi: 10.1093/ageing/afr171
Mullane, K., and Williams, M. (2020). Alzheimer’s disease beyond amyloid: can the repetitive failures of amyloid-targeted therapeutics inform future approaches to dementia drug discovery? Biochem. Pharmacol. 177:113945. doi: 10.1016/j.bcp.2020.113945
Nagpal, R., Neth, B. J., Wang, S., Craft, S., and Yadav, H. (2019). Modified Mediterranean-ketogenic diet modulates gut microbiome and short-chain fatty acids in association with Alzheimer’s disease markers in subjects with mild cognitive impairment. EBioMedicine 47, 529–542. doi: 10.1016/j.ebiom.2019.08.032
Nagpal, R., Neth, B. J., Wang, S., Mishra, S. P., Craft, S., and Yadav, H. (2020). Gut mycobiome and its interaction with diet, gut bacteria and alzheimer’s disease markers in subjects with mild cognitive impairment: a pilot study. EBioMedicine 59:102950. doi: 10.1016/j.ebiom.2020.102950
Nagu, P., Parashar, A., Behl, T., and Mehta, V. (2021). Gut microbiota composition and epigenetic molecular changes connected to the pathogenesis of Alzheimer’s disease. J. Mol. Neurosci. 71, 1436–1455. doi: 10.1007/s12031-021-01829-3
Nho, K., Kueider-Paisley, A., MahmoudianDehkordi, S., Arnold, M., Risacher, S. L., Louie, G., et al. (2019). Altered bile acid profile in mild cognitive impairment and Alzheimer’s disease: relationship to neuroimaging and CSF biomarkers. Alzheimer’s Dementia 15, 232–244. doi: 10.1016/j.jalz.2018.08.012
Nishikawa, M., Brickman, A. M., Manly, J. J., Schupf, N., Mayeux, R. P., and Gu, Y. (2021). Dietary prebiotic consumption is associated with reduced risk of Alzheimer’s disease in a multiethnic population. Curr. Alzheimer Res. 18, 984–992. doi: 10.2174/1567205019666211222115142
O’Toole, P. W., and Jeffery, I. B. (2015). Gut microbiota and aging. Science 350, 1214–1215. doi: 10.1126/science.aac8469
Ouanes, S., Clark, C., Richiardi, J., Maréchal, B., Lewczuk, P., Kornhuber, J., et al. (2022a). Cerebrospinal fluid cortisol and Dehydroepiandrosterone sulfate, Alzheimer’s disease pathology, and cognitive decline. Front. Aging Neurosci. 14:892754. doi: 10.3389/fnagi.2022.892754
Ouanes, S., Rabl, M., Clark, C., Kirschbaum, C., and Popp, J. (2022b). Persisting neuropsychiatric symptoms, Alzheimer’s disease, and cerebrospinal fluid cortisol and dehydroepiandrosterone sulfate. Alzheimers Res. Ther. 14:190. doi: 10.1186/s13195-022-01139-9
Paolicelli, R. C., Sierra, A., Stevens, B., Tremblay, M.-E., Aguzzi, A., Ajami, B., et al. (2022). Microglia states and nomenclature: a field at its crossroads. Neuron 110, 3458–3483. doi: 10.1016/j.neuron.2022.10.020
Paouri, E., and Georgopoulos, S. (2019). Systemic and CNS inflammation crosstalk: implications for Alzheimer’s disease. Curr. Alzheimer Res. 16, 559–574. doi: 10.2174/1567205016666190321154618
Park, J.-C., Han, S.-H., and Mook-Jung, I. (2020). Peripheral inflammatory biomarkers in Alzheimer’s disease: a brief review. BMB Rep. 53, 10–19. doi: 10.5483/BMBRep.2020.53.1.309
Park, J. K., Lee, K. J., Kim, J. Y., and Kim, H. (2021). The Association of Blood-Based Inflammatory Factors IL-1β, TGF-β and CRP with cognitive function in Alzheimer’s disease and mild cognitive impairment. Psychiatry Investig. 18, 11–18. doi: 10.30773/pi.2020.0205
Park, B. S., Song, D. H., Kim, H. M., Choi, B. S., Lee, H., and Lee, J. O. (2009). The structural basis of lipopolysaccharide recognition by the TLR4-MD-2 complex. Nature 458, 1191–1195. doi: 10.1038/nature07830
Park, S., Zhang, T., Wu, X., and Yi Qiu, J. (2020). Ketone production by ketogenic diet and by intermittent fasting has different effects on the gut microbiota and disease progression in an Alzheimer’s disease rat model. J. Clin. Biochem. Nutr. 67, 188–198. doi: 10.3164/jcbn.19-87
Pavlov, V. A., and Tracey, K. J. (2005). The cholinergic anti-inflammatory pathway. Brain Behav. Immun. 19, 493–499. doi: 10.1016/j.bbi.2005.03.015
Plagman, A., Hoscheidt, S., McLimans, K. E., Klinedinst, B., Pappas, C., Anantharam, V., et al. (2019). Cholecystokinin and Alzheimer’s disease: a biomarker of metabolic function, neural integrity, and cognitive performance. Neurobiol. Aging 76, 201–207. doi: 10.1016/j.neurobiolaging.2019.01.002
Qian, Y., Yang, X., Xu, S., Huang, P., Li, B., Du, J., et al. (2020). Gut metagenomics-derived genes as potential biomarkers of Parkinson’s disease. Brain 143, 2474–2489. doi: 10.1093/brain/awaa201
Qu, C., Li, Q.-P., Su, Z.-R., Ip, S.-P., Yuan, Q.-J., Xie, Y.-L., et al. (2022). Nano-Honokiol ameliorates the cognitive deficits in TgCRND8 mice of Alzheimer’s disease via inhibiting neuropathology and modulating gut microbiota. J. Adv. Res. 35, 231–243. doi: 10.1016/j.jare.2021.03.012
Rahman, M. H., Akter, R., Bhattacharya, T., Abdel-Daim, M. M., Alkahtani, S., Arafah, M. W., et al. (2020). Resveratrol and Neuroprotection: impact and its therapeutic potential in Alzheimer’s disease. Front. Pharmacol. 11:619024. doi: 10.3389/fphar.2020.619024
Reid, M. A., Dai, Z., and Locasale, J. W. (2017). The impact of cellular metabolism on chromatin dynamics and epigenetics. Nat. Cell Biol. 19, 1298–1306. doi: 10.1038/ncb3629
Roy Sarkar, S., and Banerjee, S. (2019). Gut microbiota in neurodegenerative disorders. J. Neuroimmunol. 328, 98–104. doi: 10.1016/j.jneuroim.2019.01.004
Salter, M. W., and Stevens, B. (2017). Microglia emerge as central players in brain disease. Nat. Med. 23, 1018–1027. doi: 10.1038/nm.4397
Schoultz, I., and Keita, Å. V. (2020). The intestinal barrier and current techniques for the assessment of gut permeability. Cells 9:1909. doi: 10.3390/cells9081909
Seethaler, B., Basrai, M., Neyrinck, A. M., Nazare, J.-A., Walter, J., Delzenne, N. M., et al. (2021). Biomarkers for assessment of intestinal permeability in clinical practice. Am. J. Physiol.-Gastrointest. Liver Physiol. 321, G11–G17. doi: 10.1152/ajpgi.00113.2021
Selkoe, D. J., and Hardy, J. (2016). The amyloid hypothesis of Alzheimer’s disease at 25 years. EMBO Mol. Med. 8, 595–608. doi: 10.15252/emmm.201606210
Seo, D., Boros, B. D., and Holtzman, D. M. (2019). The microbiome: a target for Alzheimer disease? Cell Res. 29, 779–780. doi: 10.1038/s41422-019-0227-7
Sgritta, M., Dooling, S. W., Buffington, S. A., Momin, E. N., Francis, M. B., Britton, R. A., et al. (2019). Mechanisms underlying microbial-mediated changes in social behavior in mouse models of autism Spectrum disorder. Neuron 101, 246–259.e6. doi: 10.1016/j.neuron.2018.11.018
Shen, H., Guan, Q., Zhang, X., Yuan, C., Tan, Z., Zhai, L., et al. (2020). New mechanism of neuroinflammation in Alzheimer’s disease: the activation of NLRP3 inflammasome mediated by gut microbiota. Prog. Neuro-Psychopharmacol. Biol. Psychiatry 100:109884. doi: 10.1016/j.pnpbp.2020.109884
Shen, X.-N., Niu, L.-D., Wang, Y.-J., Cao, X.-P., Liu, Q., Tan, L., et al. (2019). Inflammatory markers in Alzheimer’s disease and mild cognitive impairment: a meta-analysis and systematic review of 170 studies. J. Neurol. Neurosurg. Psychiatry 90, 590–598. doi: 10.1136/jnnp-2018-319148
Sheng, C., Lin, L., Lin, H., Wang, X., Han, Y., and Liu, S.-L. (2021). Altered gut microbiota in adults with subjective cognitive decline: the SILCODE study. J. Alzheimers Dis. 82, 513–526. doi: 10.3233/JAD-210259
Sheng, C., Yang, K., He, B., Du, W., Cai, Y., and Han, Y. (2022). Combination of gut microbiota and plasma amyloid-β as a potential index for identifying preclinical Alzheimer’s disease: a cross-sectional analysis from the SILCODE study. Alz. Res. Therapy 14:35. doi: 10.1186/s13195-022-00977-x
Sherwin, E., Bordenstein, S. R., Quinn, J. L., Dinan, T. G., and Cryan, J. F. (2019). Microbiota and the social brain. Science 366:eaar2016. doi: 10.1126/science.aar2016
Silva, Y. P., Bernardi, A., and Frozza, R. L. (2020). The role of short-chain fatty acids from gut microbiota in gut-brain communication. Front. Endocrinol. (Lausanne) 11:25. doi: 10.3389/fendo.2020.00025
Silva, S. D., Robbe-Masselot, C., Ait-Belgnaoui, A., Mancuso, A., Mercade-Loubière, M., Salvador-Cartier, C., et al. (2014). Stress disrupts intestinal mucus barrier in rats via mucin O-glycosylation shift: prevention by a probiotic treatment. Am. J. Physiol.-Gastrointes Liver Physiol. 307, G420–G429. doi: 10.1152/ajpgi.00290.2013
Simopoulos, C. M. A., Ning, Z., Li, L., Khamis, M. M., Zhang, X., Lavallée-Adam, M., et al. (2022). MetaProClust-MS1: an MS1 profiling approach for large-scale microbiome screening. mSystems 7, e00381–e00322. doi: 10.1128/msystems.00381-22
Song, Y., Wu, M., Tao, G., Lu, M., Lin, J., and Huang, J. (2020). Feruloylated oligosaccharides and ferulic acid alter gut microbiome to alleviate diabetic syndrome. Food Res. Int. 137:109410. doi: 10.1016/j.foodres.2020.109410
Sorboni, S. G., Moghaddam, H. S., Jafarzadeh-Esfehani, R., and Soleimanpour, S. (2022). A comprehensive review on the role of the gut microbiome in human neurological disorders. Clin. Microbiol. Rev. 35:e0033820. doi: 10.1128/CMR.00338-20
Sparkman, N. L., Buchanan, J. B., Heyen, J. R. R., Chen, J., Beverly, J. L., and Johnson, R. W. (2006). Interleukin-6 facilitates lipopolysaccharide-induced disruption in working memory and expression of other Proinflammatory cytokines in hippocampal neuronal cell layers. J. Neurosci. 26, 10709–10716. doi: 10.1523/JNEUROSCI.3376-06.2006
Spielman, L. J., Gibson, D. L., and Klegeris, A. (2018). Unhealthy gut, unhealthy brain: the role of the intestinal microbiota in neurodegenerative diseases. Neurochem. Int. 120, 149–163. doi: 10.1016/j.neuint.2018.08.005
Spittau, B. (2017). Aging microglia—phenotypes, functions and implications for age-related neurodegenerative diseases. Front. Aging Neurosci. 9:194. doi: 10.3389/fnagi.2017.00194
Stadlbauer, V., Engertsberger, L., Komarova, I., Feldbacher, N., Leber, B., Pichler, G., et al. (2020). Dysbiosis, gut barrier dysfunction and inflammation in dementia: a pilot study. BMC Geriatr. 20:248. doi: 10.1186/s12877-020-01644-2
Su, C., Zhao, K., Xia, H., and Xu, Y. (2019). Peripheral inflammatory biomarkers in Alzheimer’s disease and mild cognitive impairment: a systematic review and meta-analysis: a systematic review and meta-analysis. Psychogeriatrics 19, 300–309. doi: 10.1111/psyg.12403
Sun, Y.-Q., Richmond, R. C., Chen, Y., and Mai, X.-M. (2020). Mixed evidence for the relationship between periodontitis and Alzheimer’s disease: a bidirectional Mendelian randomization study. PLoS One 15:e0228206. doi: 10.1371/journal.pone.0228206
Sun, Y., Sommerville, N. R., Liu, J. Y. H., Ngan, M. P., Poon, D., Ponomarev, E. D., et al. (2020). Intra-gastrointestinal amyloid-β1–42 oligomers perturb enteric function and induce Alzheimer’s disease pathology. J. Physiol. 598, 4209–4223. doi: 10.1113/JP279919
Sun, J., Xu, J., Ling, Y., Wang, F., Gong, T., Yang, C., et al. (2019). Fecal microbiota transplantation alleviated Alzheimer’s disease-like pathogenesis in APP/PS1 transgenic mice. Transl. Psychiatry 9:189. doi: 10.1038/s41398-019-0525-3
Syed, Y. Y. (2020). Sodium Oligomannate: first approval. Drugs 80, 441–444. doi: 10.1007/s40265-020-01268-1
Tan, J., McKenzie, C., Potamitis, M., Thorburn, A. N., Mackay, C. R., and Macia, L. (2014). The role of short-chain fatty acids in health and disease. Adv. Immunol. 121, 91–119. doi: 10.1016/B978-0-12-800100-4.00003-9
Teixeira, A. L., Reis, H. J., Coelho, F. M., Carneiro, D. S., Teixeira, M. M., Vieira, L. B., et al. (2008). All-or-nothing type biphasic cytokine production of human lymphocytes after exposure to Alzheimer’s β-amyloid peptide. Biol. Psychiatry 64, 891–895. doi: 10.1016/j.biopsych.2008.07.019
Teng, Y., Mu, J., Xu, F., Zhang, X., Sriwastva, M. K., Liu, Q. M., et al. (2022). Gut bacterial isoamylamine promotes age-related cognitive dysfunction by promoting microglial cell death. Cell Host Microbe 30, 944–960.e8. doi: 10.1016/j.chom.2022.05.005
Thu Thuy Nguyen, V., and Endres, K. (2022). Targeting gut microbiota to alleviate neuroinflammation in Alzheimer’s disease. Adv. Drug Deliv. Rev. 188:114418. doi: 10.1016/j.addr.2022.114418
Tong, J., Zhang, J., Hao, M., Yang, J., Han, Y., Liu, X., et al. (2015). Leptin attenuates the detrimental effects of β-amyloid on spatial memory and hippocampal later-phase long term potentiation in rats. Horm. Behav. 73, 125–130. doi: 10.1016/j.yhbeh.2015.06.013
Tran, S. M.-S., and Mohajeri, M. H. (2021). The role of gut bacterial metabolites in brain development, aging and disease. Nutrients 13:732. doi: 10.3390/nu13030732
Uslu, S., Akarkarasu, Z. E., Ozbabalik, D., Ozkan, S., Çolak, O., Demirkan, E. S., et al. (2012). Levels of amyloid Beta-42, Interleukin-6 and tumor necrosis factor-alpha in Alzheimer’s disease and vascular dementia. Neurochem. Res. 37, 1554–1559. doi: 10.1007/s11064-012-0750-0
Varesi, A., Carrara, A., Pires, V. G., Floris, V., Pierella, E., Savioli, G., et al. (2022a). Blood-based biomarkers for Alzheimer’s disease diagnosis and progression: an overview. Cells 11:1367. doi: 10.3390/cells11081367
Varesi, A., Pierella, E., Romeo, M., Piccini, G. B., Alfano, C., Bjørklund, G., et al. (2022b). The potential role of gut microbiota in Alzheimer’s disease: from diagnosis to treatment. Nutrients 14:668. doi: 10.3390/nu14030668
Verhaar, B. J. H., Hendriksen, H. M. A., de Leeuw, F. A., Doorduijn, A. S., van Leeuwenstijn, M., Teunissen, C. E., et al. (2022). Gut microbiota composition is related to AD pathology. Front. Immunol. 12:794519. doi: 10.3389/fimmu.2021.794519
Vogt, N. M., Kerby, R. L., Dill-McFarland, K. A., Harding, S. J., Merluzzi, A. P., Johnson, S. C., et al. (2017). Gut microbiome alterations in Alzheimer’s disease. Sci. Rep. 7:13537. doi: 10.1038/s41598-017-13601-y
Vogt, N. M., Romano, K. A., Darst, B. F., Engelman, C. D., Johnson, S. C., Carlsson, C. M., et al. (2018). The gut microbiota-derived metabolite trimethylamine N-oxide is elevated in Alzheimer’s disease. Alz. Res. Therapy 10:124. doi: 10.1186/s13195-018-0451-2
Voigt, R. M., Raeisi, S., Yang, J., Leurgans, S., Forsyth, C. B., Buchman, A. S., et al. (2021). Systemic brain derived neurotrophic factor but not intestinal barrier integrity is associated with cognitive decline and incident Alzheimer’s disease. PLoS One 16:e0240342. doi: 10.1371/journal.pone.0240342
Wang, Q., Davis, P. B., Qi, X., Chen, S. G., Gurney, M. E., Perry, G., et al. (2021). Gut–microbiota–microglia–brain interactions in Alzheimer’s disease: knowledge-based, multi-dimensional characterization. Alzheimers Res. Ther. 13:177. doi: 10.1186/s13195-021-00917-1
Wang, C., Klechikov, A. G., Gharibyan, A. L., Wärmländer, S. K. T. S., Jarvet, J., Zhao, L., et al. (2014). The role of pro-inflammatory S100A9 in Alzheimer’s disease amyloid-neuroinflammatory cascade. Acta Neuropathol. 127, 507–522. doi: 10.1007/s00401-013-1208-4
Wang, T., Kuang, W., Chen, W., Xu, W., Zhang, L., Li, Y., et al. (2020). A phase II randomized trial of sodium oligomannate in Alzheimer’s dementia. Alz. Res. Therapy 12:110. doi: 10.1186/s13195-020-00678-3
Wang, J., Lei, X., Xie, Z., Zhang, X., Cheng, X., Zhou, W., et al. (2019). CA-30, an oligosaccharide fraction derived from Liuwei Dihuang decoction, ameliorates cognitive deterioration via the intestinal microbiome in the senescence-accelerated mouse prone 8 strain. Aging (Albany NY) 40, 3463–3486. doi: 10.18632/aging.101990
Wang, X., Li, R., and Liu, Y. (2018). Based on ‘ancient and modern medical case cloud platform (V 1.3)’, this paper discusses the medication rules of TCM in the treatment of dementia. Hebei J. Tradit. Chin. Med. 40, 1252–1255. (in Chinese)
Wang, X., Liu, G., Gao, Q., Li, N., and Wang, R. (2020). C-type lectin-like receptor 2 and zonulin are associated with mild cognitive impairment and Alzheimer’s disease. Acta Neurol. Scand. 141, 250–255. doi: 10.1111/ane.13196
Welcome, M. O. (2019). Gut microbiota disorder, gut epithelial and blood–brain barrier dysfunctions in Etiopathogenesis of dementia: molecular mechanisms and signaling pathways. NeuroMolecular Med. 21, 205–226. doi: 10.1007/s12017-019-08547-5
Wenzel, T. J., Gates, E. J., Ranger, A. L., and Klegeris, A. (2020). Short-chain fatty acids (SCFAs) alone or in combination regulate select immune functions of microglia-like cells. Mol. Cell. Neurosci. 105:103493. doi: 10.1016/j.mcn.2020.103493
Wirbel, J., Zych, K., Essex, M., Karcher, N., Kartal, E., Salazar, G., et al. (2021). Microbiome meta-analysis and cross-disease comparison enabled by the SIAMCAT machine learning toolbox. Genome Biol. 22:93. doi: 10.1186/s13059-021-02306-1
Wu, W.-L., Adame, M. D., Liou, C.-W., Barlow, J. T., Lai, T.-T., Sharon, G., et al. (2021). Microbiota regulate social behaviour via stress response neurons in the brain. Nature 595, 409–414. doi: 10.1038/s41586-021-03669-y
Wu, L., Han, Y., Zheng, Z., Peng, G., Liu, P., Yue, S., et al. (2021). Altered gut microbial metabolites in amnestic mild cognitive impairment and Alzheimer’s disease: signals in host–microbe interplay. Nutrients 13:228. doi: 10.3390/nu13010228
Wu, H.-J., and Wu, E. (2012). The role of gut microbiota in immune homeostasis and autoimmunity. Gut Microbes 3, 4–14. doi: 10.4161/gmic.19320
Wu, M.-L., Yang, X.-Q., Xue, L., Duan, W., and Du, J.-R. (2021). Age-related cognitive decline is associated with microbiota-gut-brain axis disorders and neuroinflammation in mice. Behav. Brain Res. 402:113125. doi: 10.1016/j.bbr.2021.113125
Xiang, X., Werner, G., Bohrmann, B., Liesz, A., Mazaheri, F., Capell, A., et al. (2016). TREM2 deficiency reduces the efficacy of immunotherapeutic amyloid clearance. EMBO Mol. Med. 8, 992–1004. doi: 10.15252/emmm.201606370
Xiao, S., Chan, P., Wang, T., Hong, Z., Wang, S., Kuang, W., et al. (2021). A 36-week multicenter, randomized, double-blind, placebo-controlled, parallel-group, phase 3 clinical trial of sodium oligomannate for mild-to-moderate Alzheimer’s dementia. Alz. Res. Therapy 13:62. doi: 10.1186/s13195-021-00795-7
Xie, J., Van Hoecke, L., and Vandenbroucke, R. E. (2022). The impact of systemic inflammation on Alzheimer’s disease pathology. Front. Immunol. 12:796867. doi: 10.3389/fimmu.2021.796867
Xin, Y., Zhang, L., Hu, J., Gao, H., and Zhang, B. (2021). Correlation of early cognitive dysfunction with inflammatory factors and metabolic indicators in patients with Alzheimer's disease. Am. J. Transl. Res. 13, 9208–9215.
Xiong, W., Zhao, X., Xu, Q., Wei, G., Zhang, L., Fan, Y., et al. (2022). Qisheng wan formula ameliorates cognitive impairment of Alzheimer’s disease rat via inflammation inhibition and intestinal microbiota regulation. J. Ethnopharmacol. 282:114598. doi: 10.1016/j.jep.2021.114598
Xu, R., and Wang, Q. (2016). Towards understanding brain-gut-microbiome connections in Alzheimer’s disease. BMC Syst. Biol. 10:63. doi: 10.1186/s12918-016-0307-y
Yan, Y., Gao, Y., Fang, Q., Zhang, N., Kumar, G., Yan, H., et al. (2021). Inhibition of rho kinase by Fasudil ameliorates cognition impairment in APP/PS1 transgenic mice via modulation of gut microbiota and metabolites. Front. Aging Neurosci. 13:755164. doi: 10.3389/fnagi.2021.755164
Yan, J., Herzog, J. W., Tsang, K., Brennan, C. A., Bower, M. A., Garrett, W. S., et al. (2016). Gut microbiota induce IGF-1 and promote bone formation and growth. Proc. Natl. Acad. Sci. U. S. A. 113, E7554–E7563. doi: 10.1073/pnas.1607235113
Yang, L., Cui, Y., Liang, H., Li, Z., Wang, N., Wang, Y., et al. (2022). Multifunctional selenium nanoparticles with different surface modifications ameliorate Neuroinflammation through the gut microbiota-NLRP3 Inflammasome-brain Axis in APP/PS1 mice. ACS Appl. Mater. Interfaces 14, 30557–30570. doi: 10.1021/acsami.2c06283
Ye, J., Wu, W., Li, Y., and Li, L. (2017). Influences of the gut microbiota on DNA methylation and histone modification. Dig. Dis. Sci. 62, 1155–1164. doi: 10.1007/s10620-017-4538-6
Ye, T., Yuan, S., Kong, Y., Yang, H., Wei, H., Zhang, Y., et al. (2022). Effect of probiotic fungi against cognitive impairment in mice via regulation of the fungal microbiota–gut–brain Axis. J. Agric. Food Chem. 70, 9026–9038. doi: 10.1021/acs.jafc.2c03142
Yesiltepe, M., Cimen, B., and Sara, Y. (2022). Effects of chronic vagal nerve stimulation in the treatment of β-amyloid-induced neuropsychiatric symptoms. Eur. J. Pharmacol. 931:175179. doi: 10.1016/j.ejphar.2022.175179
Zhan, X., Stamova, B., Jin, L.-W., DeCarli, C., Phinney, B., and Sharp, F. R. (2016). Gram-negative bacterial molecules associate with Alzheimer disease pathology. Neurology 87, 2324–2332. doi: 10.1212/WNL.0000000000003391
Zhang, P.-F., Wang, Z.-T., Liu, Y., Hu, H., Sun, Y., Hu, H.-Y., et al. (2022). Peripheral immune cells and cerebrospinal fluid biomarkers of Alzheimer’s disease pathology in cognitively intact older adults: the CABLE study. J. Alzheimers Dis. 87, 721–730. doi: 10.3233/JAD-220057
Zhang, X., Wang, Y., Liu, W., Wang, T., Wang, L., Hao, L., et al. (2021). Diet quality, gut microbiota, and microRNAs associated with mild cognitive impairment in middle-aged and elderly Chinese population. Am. J. Clin. Nutr. 114, 429–440. doi: 10.1093/ajcn/nqab078
Zhang, S., Wei, D., Lv, S., Wang, L., An, H., Shao, W., et al. (2022). Scutellarin modulates the microbiota-gut-brain Axis and improves cognitive impairment in APP/PS1 mice. J. Alzheimers Dis. 89, 955–975. doi: 10.3233/JAD-220532
Zhang, F., Xu, Y., Shen, L., Huang, J., Xu, S., Li, J., et al. (2021). GuanXinNing tablet attenuates Alzheimer’s disease via improving gut microbiota, host metabolites, and neuronal apoptosis in rabbits. Evid. Based Complement. Alternat. Med. 2021:9253281. doi: 10.1155/2021/9253281
Zhao, Y., Jaber, V., and Lukiw, W. J. (2017). Secretory products of the human GI tract microbiome and their potential impact on Alzheimer’s disease (AD): detection of lipopolysaccharide (LPS) in AD hippocampus. Front. Cell. Infect. Microbiol. 7:318. doi: 10.3389/fcimb.2017.00318
Zhao, Y., and Lukiw, W. J. (2018). Bacteroidetes neurotoxins and inflammatory Neurodegeneration. Mol. Neurobiol. 55, 9100–9107. doi: 10.1007/s12035-018-1015-y
Zhong, L., Chen, X.-F., Zhang, Z.-L., Wang, Z., Shi, X.-Z., Xu, K., et al. (2015). DAP12 stabilizes the C-terminal fragment of the triggering receptor expressed on myeloid Cells-2 (TREM2) and protects against LPS-induced pro-inflammatory response. J. Biol. Chem. 290, 15866–15877. doi: 10.1074/jbc.M115.645986
Zhong, S.-R., Kuang, Q., Zhang, F., Chen, B., and Zhong, Z.-G. (2021). Functional roles of the microbiota-gut-brain axis in Alzheimer’s disease: implications of gut microbiota-targeted therapy. Transl. Neurosci. 12, 581–600. doi: 10.1515/tnsci-2020-0206
Zhou, X., Cui, G., Tseng, H. H. L., Lee, S. M.-Y., Leung, G. P. H., Chan, S. W., et al. (2016). Vascular contributions to cognitive impairment and treatments with traditional Chinese medicine. Evid. Based Complement. Alternat. Med. 2016, 1–12. doi: 10.1155/2016/9627258
Zhou, R., Qian, S., Cho, W. C. S., Zhou, J., Jin, C., Zhong, Y., et al. (2022). Microbiota-microglia connections in age-related cognition decline. Aging Cell 21:e13599. doi: 10.1111/acel.13599
Zhu, X., Li, B., Lou, P., Dai, T., Chen, Y., Zhuge, A., et al. (2021). The relationship between the gut microbiome and neurodegenerative diseases. Neurosci. Bull. 37, 1510–1522. doi: 10.1007/s12264-021-00730-8
Zhuang, Z.-Q., Shen, L.-L., Li, W.-W., Fu, X., Zeng, F., Gui, L., et al. (2018). Gut microbiota is altered in patients with Alzheimer’s disease. J. Alzheimers Dis. 63, 1337–1346. doi: 10.3233/JAD-180176
Zimomra, Z. R., Porterfield, V. M., Camp, R. M., and Johnson, J. D. (2011). Time-dependent mediators of HPA axis activation following live Escherichia coli. Am. J. Phys. Regul. Integr. Comp. Phys. 301, R1648–R1657. doi: 10.1152/ajpregu.00301.2011
Keywords: Alzheimer’s disease, microbiome-gut-brain axis, biomarkers, traditional Chinese medicine, gut microbiome
Citation: Zhan Y, Al-Nusaif M, Ding C, Zhao L and Dong C (2023) The potential of the gut microbiome for identifying Alzheimer’s disease diagnostic biomarkers and future therapies. Front. Neurosci. 17:1130730. doi: 10.3389/fnins.2023.1130730
Received: 23 December 2022; Accepted: 05 April 2023;
Published: 27 April 2023.
Edited by:
Weigang Gong, Shandong University, ChinaReviewed by:
Govindaraju T, Jawaharlal Nehru Centre for Advanced Scientific Research, IndiaCopyright © 2023 Zhan, Al-Nusaif, Ding, Zhao and Dong. This is an open-access article distributed under the terms of the Creative Commons Attribution License (CC BY). The use, distribution or reproduction in other forums is permitted, provided the original author(s) and the copyright owner(s) are credited and that the original publication in this journal is cited, in accordance with accepted academic practice. No use, distribution or reproduction is permitted which does not comply with these terms.
*Correspondence: Li Zhao, ZGx6aGFvbGkwMjA5QDEyNi5jb20=; Chunbo Dong, ZGNiMTAxZGFsaWFuQDE2My5jb20=
†These authors have contributed equally to this work and share first authorship
Disclaimer: All claims expressed in this article are solely those of the authors and do not necessarily represent those of their affiliated organizations, or those of the publisher, the editors and the reviewers. Any product that may be evaluated in this article or claim that may be made by its manufacturer is not guaranteed or endorsed by the publisher.
Research integrity at Frontiers
Learn more about the work of our research integrity team to safeguard the quality of each article we publish.