- 1Department of Neurosurgery, Maastricht University Medical Centre, Maastricht, Netherlands
- 2Department of Physiology, Faculty of Medicine, King Abdulaziz University, Rabigh, Saudi Arabia
- 3Netherlands Institute for Neuroscience, Royal Netherlands Academy of Arts and Sciences, Amsterdam, Netherlands
Deep brain stimulation (DBS) is among the most successful paradigms in both translational and reverse translational neuroscience. DBS has developed into a standard treatment for movement disorders such as Parkinson’s disease (PD) in recent decades, however, specific mechanisms behind DBS’s efficacy and side effects remain unrevealed. Several hypotheses have been proposed, including neuronal firing rate and pattern theories that emphasize the impact of DBS on local circuitry but detail distant electrophysiological readouts to a lesser extent. Furthermore, ample preclinical and clinical evidence indicates that DBS influences neurotransmitter dynamics in PD, particularly the effects of subthalamic nucleus (STN) DBS on striatal dopaminergic and glutamatergic systems; pallidum DBS on striatal dopaminergic and GABAergic systems; pedunculopontine nucleus DBS on cholinergic systems; and STN-DBS on locus coeruleus (LC) noradrenergic system. DBS has additionally been associated with mood-related side effects within brainstem serotoninergic systems in response to STN-DBS. Still, addressing the mechanisms of DBS on neurotransmitters’ dynamics is commonly overlooked due to its practical difficulties in monitoring real-time changes in remote areas. Given that electrical stimulation alters neurotransmitter release in local and remote regions, it eventually exhibits changes in specific neuronal functions. Consequently, such changes lead to further modulation, synthesis, and release of neurotransmitters. This narrative review discusses the main neurotransmitter dynamics in PD and their role in mediating DBS effects from preclinical and clinical data.
Introduction
Deep brain stimulation (DBS) involves a stereotaxic electrode implantation into a specific brain region (Benabid et al., 1993) and has been successful in managing symptoms in several movement disorders including Parkinson’s disease (PD) (Wichmann and DeLong, 2006). However, the exact mechanisms behind DBS effects and side effects in PD are not fully understood. Several theories work to explain underlying mechanisms of DBS, the most common being the rate and pattern theories. These theories propose DBS modulates an overactive basal ganglia target in PD patients by normalizing the firing rate at the electrophysiological single-cell level (Agnesi et al., 2013; Ashkan et al., 2017), interferes with pathological patterns such as subcortical beta oscillations (13–30 Hz), promotes cortical gamma activity (40–200 Hz), and modulates local field potential readouts (Eusebio and Brown, 2009; Cheney et al., 2013; Muthuraman et al., 2021). However, those theories only involve effects of DBS on local, and to a lesser extent, remote readouts. For instance, electrophysiological recordings of neuronal activity show that ventroanterior (VA) and ventrolateral (VL) thalamus-DBS (10 Hz) suppress the beta oscillation and increase the gamma power in the motor cortex in both in vitro and invivo animal models and improves motor function (Tucker et al., 2019). In addition, subthalamic nucleus (STN) DBS leads to a short-latency excitatory effect that tonically increases the firing rate in the globus pallidus internal/external (GPi/GPe) followed by post-stimulation suppression of the firing rate (Hashimoto et al., 2003). Another study showed STN-DBS only suppressed the neuronal activity in the GP and substantia nigra pars reticulata (SNr) in anesthetized rats (Benazzouz et al., 1995; Beurrier et al., 2001). Despite this discrepancy, another explanation could be from variations between DBS local effects vs. orthodromic activations from the STN to the GPe and GPi as well as the motor cortex. Furthermore, preclinical studies show that low-frequency stimulation (LFS) of the pedunculopontine nucleus (PPN) suppresses the firing rate in the STN and SNr in PD rat models (Alam et al., 2012; Park et al., 2014).
Neurotransmitters are largely neglected when discussing mechanisms behind DBS possibly due to technical challenges of recording real-time changes in remote areas distant from the stimulation zone. Both clinical and preclinical PD data show DBS modulates several neurotransmitter networks such as dopaminergic, glutamatergic, GABAergic, serotonergic, and cholinergic systems (Ogura et al., 2004; Boulet et al., 2006; Temel et al., 2007; He et al., 2014; Wen et al., 2015). For example, the effect of STN-DBS on motor function could be associated with various effects on striatal dopaminergic and glutamatergic neurotransmission (Boulet et al., 2006; He et al., 2014), while the effect of GPi-DBS could be associated with changes in the striatal dopaminergic and pallidum GABAergic systems (Ogura et al., 2004; Xiao et al., 2019). Likewise, the PPN may be investigated as a new candidate DBS target to assess its effects on the cholinergic system to reduce gait disturbances in PD (Wen et al., 2015). Additionally, STN-DBS has been associated with mood-related side effects possibly linked to brainstem serotoninergic systems (Temel et al., 2007). STN-DBS therapeutic effects showed to be diminished by severe degeneration of the locus coeruleus (LC) noradrenergic system (Guimarães et al., 2013; Faggiani et al., 2015). Furthermore, DBS has shown to modulate glia cells to induce glutamate release and modulate neurotransmission in nearby neurons in PD (McGeer and McGeer, 2004, 2008; Vedam-Mai et al., 2012).
Deep brain stimulation has also shown to exhibit long-term effects possibly due to neuroplasticity involving transmitter levels and neuronal communication (Ashkan et al., 2017). Understanding patterned changes at the transmitter level will contribute to a better understanding of the underlying mechanisms of DBS and lead to optimized targeting, improved symptom management, lesser side effects, and an improved quality of treatment. In this article, we discuss neurotransmitter dynamics in PD and briefly revise current theories on the mechanisms behind DBS within the context of neurotransmitter dynamics. We then address the extent of alterations in neurotransmitter systems before and after DBS in PD, the circuits involved, and the impacts such changes can have on PD symptoms.
Neurotransmitter systems’ dynamics in Parkinson’s diseases
Parkinson’s disease (PD) has been pathologically categorized as a disorder of the basal ganglia, a network consisting of three main regions, (1) the striatum (caudate and lentiform nuclei); the lentiform nucleus made up of putamen and dorsal pallidum; dorsal pallidum consists of GPi and GPe, (2) the nigra complex (SNc and SNr), and (3) the STN (Jahanshahi et al., 2020). The neocortex, thalamus and brainstem regions such as PPN are also heavily involved in PD as they are connected to the basal ganglia at multiple levels (Jahanshahi et al., 2020). The Albin et al. (1989)–DeLong (1990) model describes how the basal ganglia regulates movement execution or inhibition by two main pathways (Albin et al., 1989; DeLong, 1990); (1) The direct pathway where the striatum projects GABAergic inhibitory activity directly to the GPi/SNr that blocks inhibitory output to the thalamus; the thalamus projects excitatory glutamatergic activity to the cortex allowing for movement execution, and (2) the indirect pathway where the striatum projects GABAergic inhibitory activity to the GPe and STN activating the GABAergic inhibition of the GPi/SNr to the thalamus; thalamic glutamatergic activity is inhibited and ultimately suppresses cortical activity. Moreover, the striatum also receives dopaminergic projections as an output signal from the SNc that regulates both direct and indirect routes (Albin-Delong classic model). In addition, Nambu et al. (2002) described the “hyper-direct” pathway, a fast pathway forming a loop from the cortex that has a glutamatergic excitatory connection to the GPi via the STN and stimulates the GPi which then inhibits the thalamus and neocortex (Nambu et al., 2002).
The main pathological hallmark of PD is the neurodegeneration of SNc dopaminergic neurons (Yelnik, 2002). Dopaminergic neurons from the SNc that innervate the striatum are from the nigrostriatal pathway that regulates both the direct and indirect pathways. The nigrostriatal pathway connects to the thalamus and cortex via striatal interneurons and spinal projection neurons that have dopamine D1 or D2 receptors. Dopamine medications for PD reduce symptoms via their action on D1 receptors but have inhibitory effects on D2 receptors that could partially underlie side effects. This is one reason why medication such as Levodopa combined with D2 receptor antagonist increases the efficacy of the medication (Sarkar et al., 2016).
Degeneration of dopaminergic SNc neurons causes dysfunction of the direct and indirect pathways in the basal ganglia leading to less inhibition of the GPe and STN, increased activity of GPi, and decreased overall excitation input from the thalamus to the cortex. Furthermore, the STN also receives afferent glutamatergic innervations from the cortex and the parafascicular nucleus of the thalamus (Kitai and Deniau, 1981; Mouroux et al., 1995). Direct glutamatergic neuronal connections from the motor cortex (MC) to the STN investigated in 6-hyrdoxydopamine (6-OHDA) hemi-lesioned rats using anterograde tracing (Wang et al., 2018) showed MC-STN connectivity was impaired and glutamatergic terminals were reduced (Wang et al., 2018). In addition, the STN receives GABAergic input from the GPe, dopaminergic input from the SNc, and cholinergic/glutamatergic input from the PPN (Nauta and Cuenod, 1982; Campbell et al., 1985; Lavoie and Parent, 1994). As mentioned above, this dysfunctional connection of the MC-STN could lead to overactive STN glutamatergic neurons that may produce excitotoxins projecting to the SNc and GPi (Rodriguez et al., 1998; Przedborski, 2005; Dexter and Jenner, 2013). Glutamatergic excitotoxicity was found to be related in several validated animal models of PD, such as 6-OHDA and 1-methyl-4-phenyl-1,2,3,6-tetrahydropyridine (MPTP) (Dexter and Jenner, 2013). Furthermore, this excitotoxicity can be inhibited by a glutamate antagonist MK801 but not with the GABA antagonist bicuculline (Wu et al., 2012).
Role of neurotransmitters in mediating deep brain stimulation effects
Historically, surgical treatment using vascular or chemical lesions of the GP or thalamus were used to reduce PD symptoms before the discovery of Levodopa in 1967 (Quinn, 1999; Olanow, 2002). However, these treatments failed to suppress disease progression and lead to several adverse effects such as dyskinesia, motor fluctuations, and drug-resistance (Marsden and Parkes, 1977). The development of stereotactic surgery, neuroimaging, and intraoperative electrophysiology enabled more accurate brain region targeting with a higher therapeutic yield and less overall harm (Benabid et al., 1993; Benabid et al., 2001). STN-DBS was done in 1993 by Benabid then became the standard treatment especially for refractory PD patients (Benabid et al., 2001).
Despite progress in DBS as a treatment for movement disorders, its exact mechanisms of action have not been fully unraveled. Several theories have been proposed, the most common being the neuronal firing “rate-model” and the patterned “synchronized oscillations” hypotheses (Bergman et al., 1994; Levy et al., 2000; Brown et al., 2001; Breit et al., 2004; Lozano and Eltahawy, 2004; Benabid, 2007; Rosa et al., 2012; Ashkan et al., 2017). The rate model hypothesis postulates DBS blocks overactive basal ganglia neuronal firing rates in the STN and GPi caused by PD pathology. The blocking of the overactive basal ganglia was predicted by lesion effects of earlier neuromodulation techniques (Quinn, 1999). Interestingly, a post-mortem study on spinocerebellar ataxia disease found a consistent degeneration of SNc, although patients did not exhibit parkinsonian symptoms (Schöls et al., 2015). Further histological analysis of this post-mortem brain tissue showed a significant lesion in brain regions such as the thalamic ventral anterior and ventral lateral nuclei, pallidum, PPN, and STN.
Consequently, these findings suggest that lesion effects of these targets lead to a therapeutic output on PD symptoms. The pattern “synchronized oscillations” hypothesis has also been proposed as a mechanism of DBS, where pathological beta-band oscillatory activity (abnormal synchronized bursts of activity) in loops between the cortex, basal ganglia, thalamus, PPN, and cerebellum contribute to the genesis of PD motor symptoms (Bevan et al., 2006). DBS is thought to disrupt and suppress pathological beta-band oscillations and promote gamma power, thereby reducing bradykinesia and rigidity symptoms (Muthuraman et al., 2021).
In addition to local effects, DBS has shown to have downstream effects on remote areas. For instance, STN-DBS in PD has led to increased striatal dopamine release in PD rats (Meissner et al., 2001, 2002; He et al., 2014; Yamamoto et al., 2014). In addition, another study found a correlation between an increase in striatal monoamine (MA) levels and STN beta activity suppression via STN-DBS in a rat model of PD (hemi-PD 6-OHDA) (Yamamoto et al., 2014). GPi-DBS has also been shown to stimulate striatal dopamine release in rodents (Dostrovsky et al., 2000; Johnson and McIntyre, 2008). Moreover, preclinical research shows STN-DBS reduces dorsal raphe nucleus (DRN) serotonergic neuron firing rates in both PD and healthy control rat models (Temel et al., 2007) while PPN-DBS reduces acetylcholine (ACh) loss in the ventral thalamus (Wen et al., 2015).
Research suggests DBS has multimodal effects that are not simply due to an inhibition of local axons, but from effects that may pass through fiber, efferent, and afferent axons through orthodromic and antidromic pathways (McIntyre et al., 2004; Vachez and Creed, 2020). Moreover, DBS is thought to exhibit long-term effects, including tyrosine hydroxylase (TH) cell survival in the striatum as a possible form of neurogenesis and neuroplasticity (Khaindrava et al., 2011). Additionally, alleviating pathological oscillations by continuous DBS showed short-term and long-term synaptic plasticity in the SNr (Milosevic et al., 2018, 2019). These long-term effects may be activated via neurochemical changes displayed through neurotransmitters’ levels and neuronal communications. The following section will extensively discuss dynamic changes in main neurotransmitter systems involved in PD that mediate DBS effects (Table 1, Figure 1).
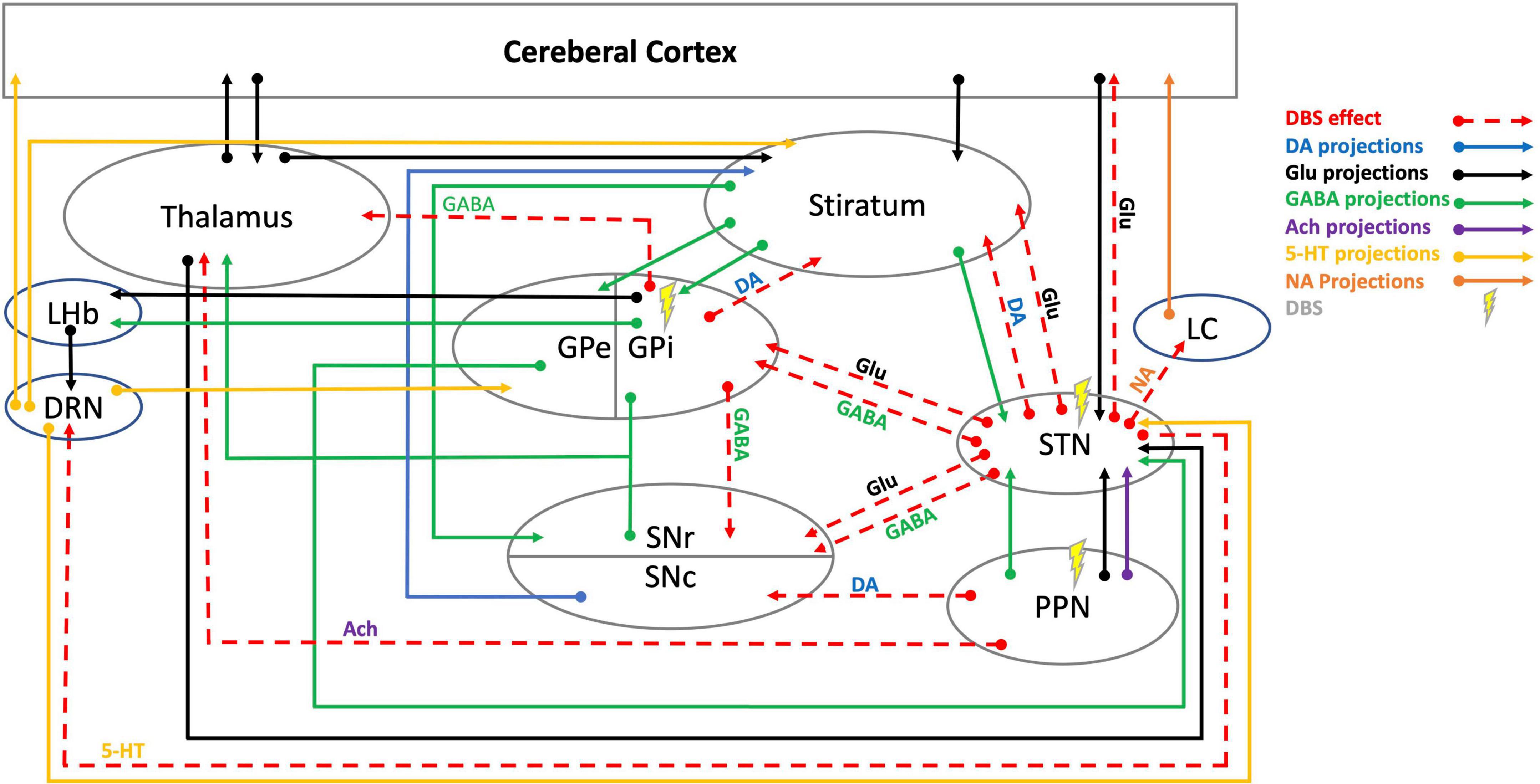
Figure 1. Schematic illustration of DBS neurotransmitter mediation in cortico-thalamic-basal ganglia-brainstem circuits. 5-HT, 5-hydroxytryptamine (serotonin); Ach, acetylcholine; DA, dopamine; DBS, deep brain stimulation; DRN, dorsal raphe nucleus; GABA, γ-aminobutyric acid; Glu, glutamate; GPe, globus pallidus external; GPi, globus pallidus internal; LC, locus coeruleus; LHb, lateral habenula; NA, noradrenaline; PPN, pedunculopontine nucleus; SNc, substantia nigra pars compacta; SNr, substantia nigra pars reticulata, STN, subthalamic nucleus. DBS effects data are cited in Table 1.
Dopaminergic system
Parkinson’s disease is principally defined as pathological degeneration of dopaminergic SNc neurons; newly diagnosed PD patients can show a 50% loss of SNc dopaminergic neurons (Marsden and Parkes, 1977). Moreover, the standard medical treatment for PD (drugs like Levodopa) mainly work on dopaminergic receptors, however, long-term use of medical treatments can exhibit unwanted side effects. Therefore, it has been proposed one mechanism of DBS for PD is its action on surviving striatal DA neurons (Khaindrava et al., 2011). Several early studies were based on this assumption, with some even attempting to directly stimulate the SNc (Preston et al., 1981; Vaccarino and Franklin, 1982; Lin and Yang, 1994). Overtime, STN-DBS showed to improve the motor symptoms of PD more effectively and reduce the need for Levodopa, eventually leading to fewer adverse drug effects (Karl et al., 2022).
Nigrostriatal dopaminergic neurons were assessed in several preclinical animal studies and showed that STN-DBS modulate SNc dopaminergic neurons. Sahai et al. (2020) showed that STN-DBS decreases the spiking activity in less than half (43%) of the SNc dopaminergic neurons in naïve rats group while increasing the spiking activity in the other 43% of the cells. However, the PD rat group showed a significant reduction in the spiking activity of 88% of dopaminergic cells (Sahai et al., 2020). Moreover, an in vivo microdialysis study on unilateral 6-OHDA rats showed short-term effects of stimulation and lesioning in the STN increased extracellular levels of dopamine in the striatum (Walker et al., 2009). In another microdialysis study in a freely moving rat model of PD (6-OHDA), STN-DBS increased striatal DA metabolites in awake, freely moving animals (Meissner et al., 2001, 2002). When in vivo real-time electrical and chemical detection of dopamine concentrations and neural firings in the caudate-putamen (CPU) of PD rats PD were assessed using fast-scan cyclic voltammetry (FSCV), dopamine concentrations increased and striatal neurons firing decreased following GPi-DBS (Xiao et al., 2019). Additionally, in vivo experiments in pigs using a Medtronic 3,389 device to perform FSCV combined with a carbon-fiber microelectrode (CFM) in the striatum to track dopamine release evoked by electrical stimulation showed STN-DBS elicited a stimulus-time-locked increase in striatal dopamine release that was both stimulus intensity- and frequency-dependent (Shon et al., 2010). Another experiment in monkeys showed STN-DBS induced phasic DA release in the striatum (Nakajima et al., 2017).
Further animal experimental studies have investigated the STN-DBS neurotherapeutic effects in improving motor symptoms caused by dopamine in non-human primates and in PD rodent models (He et al., 2014; Yamamoto et al., 2014). One study of MPTP rhesus monkeys treated with STN-DBS and microdialysis implanted bilaterally in the putamen and caudate nuclei showed improvement in motor symptoms and increased extracellular DA along with its metabolites in both brain regions (Zhao et al., 2009). Other researchers used multi-contact DBS electrode, fMRI and FSCV to identify optimum dopamine-recording sites in rhesus macaques and found dopamine release reduces or increases depending on the slight redirection of DBS electrode tip location in the STN while the highest evoked response was shown when the DBS electrode tip contact were in the dorsal part of STN (Min et al., 2016). Lastly, striatal dopaminergic receptors expression was assessed in intact and total nigrostriatal dopaminergic denervated rats after a 4-h unilateral STN-HFS using particular radioligands ([3H] SCH 23390, [125I] iodosulpride, and [125I] OH-PIPAT) and showed increased D1 receptor (D1R) binding in all areas of the striatum but decreased binding of both D2 and D3, leading some to suggest that D1R effects could explain the therapeutic effect of DBS while side effects could be more due to D2R and D3R (Carcenac et al., 2015; Melon et al., 2015).
Some evidence suggests that STN-DBS for PD has a long-term neuroprotective effect on the survival of SNc cells in animal models (Temel et al., 2006; Wallace et al., 2007; Spieles-Engemann et al., 2011). Khaindrava et al. (2011) investigated DBS of the STN and found no significant increase in cell proliferation, yet cell survival in dopaminergic neurons of the striatum was increased. In addition, lesser amounts of cell proliferation was observed in the hippocampus and olfactory bulb. Another study investigated the neuroprotective effects of STN-DBS on a unilateral 6-OHDA model in rats; post-stimulation effects revealed that nigral TH positive neurons and the protein phosphatase-2A (PP2A) were blocked inducing autophagy and dissociating the Bcl-2/Beclin1 complex showing a possible neuroprotective mechanism for PD (Du et al., 2018). Another STN-DBS study in 6-OHDA rat model of PD showed BDNF-mediated neuroprotection in the SNc by acute and long-term blocking of TrkB enzyme that decreased phosphorylation of Akt and ribosomal protein S6 (Fischer et al., 2017). Another study investigated the protective effect of DBS in the STN in unilateral 6-OHDA rats and found apoptosis significantly decreased in the DBS group.
Some clinical findings toward the effects of DBS on striatal dopaminergic neurons have shown to be contradictory. A PET study in PD patients assessed vesicular monoamine transporter 2 (VMAT2) and cerebral glucose metabolism before DBS surgery and 4–6 months after STN-DBS and showed improvement of motor and neuropsychiatric symptoms while VMAT2 had decreased in the caudate and putamen along with decreased cerebral glucose metabolism in the striatum (Smith et al., 2019). Another PET study showed synaptic dopamine concentrations increase in the putamen and caudate nucleus after STN-DBS alongside medication; Levodopa significantly reduced [11C] raclopride binding potential (RacloBP) in the putamen, while post-operatively the reduced RacloBP-binding reversed (Nimura et al., 2005) and the drug-induced increase in synaptic dopamine concentrations was also higher after stimulation.
A clinical study used SPECT to assess the dopamine D2 receptor and dopamine transporter (DAT) in PD patients pre-surgery and at 12 months post-surgery; the unified PD rating scale (UPDRS) scores post-surgery remarkably improved in those patients and titrated down their medication, but no changes in DAT availability or an increase in D2 receptor binding were detected (Hesse et al., 2008). Another clinical study investigated the occurrence of apathy and depression following 12-month STN-DBS in PD and control groups and found that PD exhibited more apathy and depression symptoms. Additionally, sections of each group received a PET-scan ([11C]-raclopride) that showed increased binding of D2/D3 receptor density. In sum, these findings reflect that decreased synaptic dopamine levels in the mesolimbic area, particularly the ventral tegmental area (VTA) are more substantial in PD patients, suggesting that apathy and depression occur post-surgery as a postponed dopamine withdrawal syndrome (Thobois et al., 2010).
Clinical PET scans in 6 PD patients receiving STN-DBS showed no difference in RACLO binding (ligand for dopamine D2/D3 receptor) between DBS on and off conditions along with no evidence of increased striatal dopamine concentration under effective STN-DBS (Hilker et al., 2003; Strafella et al., 2003). In another clinical trial, cerebrospinal fluid and plasma catecholamine levels in STN-DBS treated PD patients were measured after oral antiparkinsonian drug administration before surgery and an hour after medication while being on DBS; higher pre-operative catecholamine levels were linked to better STN-DBS outcomes (Yamamoto et al., 2015).
Stereotactic microdialysis is feasible in PD patients during STN-DBS surgery (Kilpatrick et al., 2010; Zsigmond et al., 2012). Within 30 min of DBS electrode placement in the STN and a microdialysis probe in the STN or SN researchers can get a steady-state baseline level of glutamate, dopamine, and GABA (Kilpatrick et al., 2010). A microdialysis study in the GPi during GPi-DBS surgery investigated dopamine levels before and after DBS and found that 4 out of 5 patients had significantly increased pallidal DA after stimulation (Martinez et al., 2013). However, there was no association between the improvement in rigidity and pallidal DA increase, suggesting other mechanisms might be involved in these clinical effects of GPi-DBS (Martinez et al., 2013).
In conclusion, the dopaminergic system is essential in PD as is clearly mediated with pharmaceutical and DBS therapies. DBS has shown to treat motor symptoms of PD and decrease the need for DA medication, suggesting DBS has a synergetic effect on DA. Preclinical animal data suggests that STN DBS influences both local and remote dopaminergic systems, while clinical microdialysis data in human subjects point more toward a local effect. Remote effects are less consistent in imaging studies, as inconsistencies stem from limitations innate to PET and SPECT neuroimaging techniques.
Glutamatergic system
Glutamatergic neurons are distributed throughout the central nervous system (Greenamyre, 2001). Several theories suggest overactive glutamate in the basal ganglia induces excitotoxin production in SNc neurons contributing to the pathogenesis of PD (Olney et al., 1975; Piallat et al., 1996; Saji et al., 1996; Smith et al., 1996; Rodriguez et al., 1998; Nakao et al., 1999). An experimental stereotactic injection of glutamate receptors antagonist (NMDA blocker) in the striatum of rodents has shown to alleviate parkinsonian symptoms (Carroll et al., 1995; Schmidt, 1995). It then appears that DBS could influence the glutamatergic system in a net positive way as neurons within the striatum act as intermediate excitatory neurons between basal ganglia-thalamocortical circuits (Greenamyre, 2001).
Several pieces of evidence suggest that DBS effects on glutamate is solely a local effect that only lasts during stimulation. In an experimental study of anesthetized rats, a dual enzyme-based electrochemical sensor measured extracellular glutamate concentrations in the STN and found that glutamate concentrations increased rapidly during DBS and were sustained during stimulation. After cessation of stimulation, elevated glutamate levels slowly fell toward baseline (Lee et al., 2007). Another study assessed the dyskinesia side-effects of STN-DBS in unilateral 6-OHDA rats using microdialysis in the SNr; STN-DBS increased glutamate and induced dyskinesia in both intact and hemiparkinsonian rats (Boulet et al., 2006). When the stimulation frequency was lowered, glutamate levels were unaffected suggesting dyskinesia blocks glutamate receptor antagonists and facilitates agonists. In another study, STN-DBS was performed in rats to determine if neurochemical changes in the GP and SNr were frequency-dependent and found that glutamate concentrations were significantly increased at a high frequency (60 and 130 Hz) in the GP and SNr but did not show significant change at LFS (10 Hz) (Windels et al., 2000, 2003). It should be noted that the measured glutamate levels in the GP refers only to the GPe, and is not a basal ganglia’s output structure unlike the SNr. The entopeduncular (EP) nucleus is homologous to the primate GPi in rats (Karain et al., 2015).
Chassain et al. (2016) studied glutamate metabolic, synaptic, and behavioral changes in hemiparkinsonian rats after 5 weeks of chronic STN-DBS using proton magnetic resonance spectroscopy (11.7T) and found chronic STN-DBS corrects the glutamate metabolites levels associated with neurotransmission in the striatum and SNr, restores corticostriatal synaptic plasticity, and restores motor skills progressively in the staircase test. Consequently, these findings suggest chronic STN-DBS not only has a local effect but also a remote effect in the basal ganglia. Furthermore, STN-DBS showed a reduction in sensitivity toward glutamate neurotransmission via downregulating calcium/calmodulin-dependent kinase IIa (CaMKIIa) and Homer1 genes in the STN as these genes are associated with glutamate neurotransmission (Henning et al., 2007). These findings suggest STN-DBS could have a neurotherapeutic effect by alleviating overactive glutamatergic neurons in the STN. To validate these findings in humans, clinical studies were performed to assess glutamate levels in PD patients and controls using magnetic resonance spectroscopy. Pontine glutamate levels were shown to be lower in PD patients while glutamate levels also emerged as a significant predictor of outcome, further implementing glutamatergic neurotransmission within the mechanisms of DBS (Tuura et al., 2018).
In sum, the glutamatergic system has shown to be involved in PD pathology. STN-DBS modulates glutamatergic neurons and glutamate neurotransmission and exhibits neurotherapeutic effects by improving motor and adverse effects. Acute effects of STN-DBS on the glutamatergic system were thought to be merely local and stimulation-dependent, however, chronic effects of STN-DBS show increases in glutamate in the striatum and SNr and further demonstrate to improve motor symptoms of PD in preclinical studies.
GABAergic system
GABAergic neurons are distributed widely through the entire central nervous system (Olsen and Li, 2012) and act mainly as interneurons, having an essential role in regulating cortical and subcortical circuits including the cortico-thalamic-basal ganglia circuits (Zhang et al., 2021). GABAergic neurons function harmoniously with glutamatergic and dopaminergic neurons in the cortical-thalamic-basal ganglia circuit to control movement. As previously described, glutamatergic neurons have an excitatory effect while GABAergic neurons have inhibitory projections in the basal ganglia. Dopaminergic neurons also have both excitatory and/or inhibitory (depending on momentum) after-effects on glutamatergic and GABAergic modulation balance (Zhang et al., 2021).
Subthalamic nucleus-DBS has shown to increase the neuronal firing rate in the SNr of PD rats (Benazzouz and Hallett, 2000). Moreover, preclinical studies show an increased selective extracellular GABA release in the SNr after STN-DBS in rats (Windels et al., 2000; Savasta et al., 2002). This increase of GABA release in the SNr was shown within a stimulation frequency-dependent range of 60 to 350 Hz, while glutamate release only increased until 130 Hz (Windels et al., 2003). STN-DBS could then have both local and cumulative effects on GABA release in the SNr that pass from the STN to the SNr, independent of the STN-DBS effect on glutamate release in the GP (McIntyre et al., 2004). Additionally, in situ hybridization of glutamate decarboxylase 67 kDa isoform (GAD67) study also showed an increase in GABA in the GP after STN-DBS in unilateral 6-OHDA rats (Salin et al., 2002).
Clinical studies demonstrate GPi-DBS to improve bradykinesia and Levodopa-induced dyskinesia (LID) in PD patients through its effects on pallidum GABAergic neurons (Obeso et al., 2000; Calon and Di Paolo, 2002). Moreover, depending on the specific stimulation site within the GPi it was found that DBS of the ventral GPi reduced LID while stimulation of the dorsal part showed to improve bradykinesia (Krack et al., 1998; Vitek et al., 2004).
Using microdialysis in the GPi and ventral anterior (VA) thalamus during the first delivery of STN-DBS or Levodopa, researchers found both treatments reduced GABA levels in the VA thalamus, while STN-DBS also increased cyclic guanosine monophosphate (cGMP) levels in the GPi (Stefani, 2011; Stefani et al., 2011). A clinical imaging study assessing GABA levels of PD patients and controls using magnetic resonance spectroscopy showed basal ganglia GABA levels to be higher in PD patients (Tuura et al., 2018). In another clinical study on neurotransmitter levels in CSF of PD patients with GPi-DBS, CSF was collected a day after surgery 1 h before and 1 h after GPi stimulation. GABA levels increased during stimulation but no differences were seen in levels of dopamine, noradrenaline, or homovanillic acid (Ogura et al., 2004).
In conclusion, it is clear that GABAergic interneurons play a critical role in movement regulation and within the dynamics of STN- and GPI-DBS. However, GPi-DBS effects depend on whether the ventral or dorsal part of the GPi is stimulated (Krack et al., 1998; Obeso et al., 2000; Calon and Di Paolo, 2002; Vitek et al., 2004). Furthermore, local impacts of STN-DBS show an increase in GABA in the GP while remote effects connected to the SNr are low to high frequency stimulation-dependent and differ from glutamate responses (Windels et al., 2000; Salin et al., 2002; Savasta et al., 2002).
Serotoninergic system
The serotonergic system is well known to be involved in mood and anxiety (Smith et al., 1997), while patients who undergo STN-DBS have shown side effects related to serotoninergic and dopaminergic systems such as depression, suicide ideation, and impulsivity (Voon et al., 2008; Temel et al., 2009; Thobois et al., 2010; Tan et al., 2011a). Several lines of evidence show that STN-DBS inhibits the serotoninergic system, and while the STN has no direct connection to the DRN, its relay station via the lateral habenula (LHb) suggests an indirect serotonin-modulating mechanism (Tan et al., 2011b).
Studies have shown that bilateral STN-DBS reduces the firing rate of DRN serotonergic neurons and induces depressive-like behavior in both PD and control rat models (Temel et al., 2007). Furthermore, the firing rate of DRN neurons that were inhibited by STN-DBS did not quickly recover to their pre-stimulus firing rates; many of these neurons remained to show reduced activity throughout the 5-min post-stimulus recording period. This suggests STN-DBS elicits mechanisms that may cause sustained suppression of the serotonergic system (Hartung et al., 2011). Moreover, STN-DBS inhibited 5-HT release in forebrain regions such as the prefrontal cortex (PFC), hippocampus (HIPP), and striatum (Navailles et al., 2010; Tan et al., 2012). Recent research shows that long-term (10 weeks) STN-DBS inhibits the serotoninergic neuronal activity during stimulation measured by calcium transients photometry and leads to a loss of serotoninergic cell phenotypes inducing depressive-like behavior in the MPTP mouse model of PD (Alosaimi et al., 2022). Animal studies also show that presence of severe serotoninergic dysfunction reduces STN-DBS therapeutic efficiency (Faggiani et al., 2015). In addition, the 5-HT system has been implicated in dyskinesia (Sandler, 1996; Lauterbach and Shillcutt, 1997; Caley, 1998; Kapur et al., 1999; Creed-Carson et al., 2011). However, it can be speculated that a sustained suppression of the 5-HT system via loss of 5-HT cell phenotype could contribute to the lower incidence of dyskinesia following STN-DBS. In the other words, reduced basal ganglia 5-HT function supports the therapeutic effects of DBS. Future research is needed to explore the exact trajectory connections between the STN and the DRN that engender DBS targeting to display fewer adverse effects.
Cholinergic system
Neurons of the cholinergic system are predominantly located in the nucleus basalis of Meynert, the PPN, and striatum (Mesulam and Geula, 1988; Heckers et al., 1992; Mesulam et al., 1992). Recent data suggests the degeneration of cholinergic neurons could be involved in the pathogenesis of early stage PD and linked to its axial and non-motor symptoms, including cognitive decline and mood disorder (Mayeux et al., 1981; Green et al., 2002; Braak et al., 2003; Aarsland and Kurz, 2010; Wilkins et al., 2020). Moreover, striatal cholinergic interneurons regulate basal ganglia circuits and could modulate effects of dopaminergic and glutamatergic systems (Liu, 2020).
Animal studies have investigated tonically active neurons (TANs) and putative cholinergic interneurons in the striatum during DBS; STN-DBS produced a more extensive suppression of TAN spiking rates compared to GPi-DBS in healthy monkeys, additionally, a local DA antagonist infusion in the striatum only reduced the spike rate after STN-DBS, but not in the GPi-DBS group (Nakajima et al., 2017). This suggests a more apparent increase of DA after STN-DBS antagonized by the local DA infusion and counterbalanced by the putative cholinergic interneurons.
Pedunculopontine nucleus-DBS has been proposed to treat axial and gait symptoms of PD. PPN-LFS (25 Hz) in unilateral 6-OHA rats improved gait symptoms including base of support and maximum contact area in the catwalk test assessing locomotion and gait function (Wen et al., 2015). Furthermore, PPN-LFS also mildly reversed acetylcholine loss in the ventrolateral thalamic nucleus in rodents (Wen et al., 2015). Sharma et al. (2020) investigated the stimulation effects of designer receptors exclusively activated by designer drugs in the PPN using a PET scan to assess nigrostriatal dopaminergic neurons in a chemogenetic PD model and found that residual nigrostriatal dopaminergic neurons reduced motor symptoms (Sharma et al., 2020). PPN-HFS has also shown to reduce postural instability of PD-rats (6-OHDA), however, rats stimulated in the PPN showed complex behavior effects (Rauch et al., 2010). In sum, the cholinergic system regulates motor functions in the basal ganglia and is associated with axial and gait symptoms in PD. Preclinical animal studies of PPN-DBS exhibits an improvement in axial and gait symptoms however the data is mixed. It remains to be determined whether the PPN is a suitable new stimulation target for patients with severe axial and gait dysfunction.
Noradrenergic system
Noradrenaline (NA) is predominantly produced by the LC located in the pons and projects to cortical, subcortical, and spinal structures (Tubbs et al., 2011). Noradrenergic LC neurons have shown to be involved in a wide range of sensory-motor, behavioral, and cognitive functions (Foote et al., 1980, 1983; Aston-Jones, 2005; Benarroch, 2009; Berridge et al., 2012; Uematsu et al., 2015; Feinstein et al., 2016; Breton-Provencher et al., 2021). Evidence suggests the loss of NA neurons occurs several years prior to presentation of PD symptoms and more extensively than in SNc dopaminergic neurons (Zarow et al., 2003; Rommelfanger and Weinshenker, 2007; McMillan et al., 2011; Espay et al., 2014). Additionally, PD exhibits a neuropathological vulnerability to neuromelanin loss that is shown to affect both DA in the SNc and NA in the LC (Hirsch et al., 1988; Sasaki et al., 2006; Ohtsuka et al., 2013; Martin-Bastida et al., 2017; Singh, 2020).
In recent years, the effects of DBS on LC noradrenergic system have gained considerable attention. A preclinical animal study showed that severe noradrenergic dysfunction reduces STN-DBS therapeutic efficiency within a PD rat model (Faggiani et al., 2015). Furthermore, Guimarães et al. (2013) investigated the involvement of the LC noradrenergic system on weight loss in a PD rat model and showed STN-DBS abolished weight loss in bilateral LC and striatum 6-OHDA lesioned rat without any observed changes in their food or other metabolic parameters. Additionally, the degeneration of the LC was not accompanied by significant changes in motor behavior but led to an extra decrease in striatal monoamine levels reflected by the decrease in the DA/L-3,4-dihydroxyphenylalanine (L-DOPA) ratio (Guimarães et al., 2013).
A clinical study suggests a role for the LC noradrenergic system in STN-DBS. When stimulation is combined with administration of clonidine, a selective alpha2 adrenergic agonist, STN-DBS related benefits on akinesia are diminished (Albares et al., 2015). On the other hand, one clinical study showed that metoprolol, a beta1-adrenergic antagonist, suppressed STN bursting activities marking a brief decrease in rigidity pre-STN-DBS surgery (Coenen et al., 2008). Retrospective clinical data showed STN-DBS led to weight regain in PD after DBS surgery (Tuite et al., 2005; Strowd et al., 2010) and this was suggested to be related to a STN-DBS effect on the LC noradrenergic system (Guimarães et al., 2012).
In conclusion, LC noradrenergic dysfunction occurs years before clinical diagnosis and shows a similar pathological root to PD comparable to SNc dopaminergic systems. Furthermore, severe dysfunction of the noradrenergic system diminishes positive effects of STN-DBS, as STN-DBS has shown to improve weight loss in PD possibly through its actions on the LC noradrenergic system. Pharmacological manipulations of the noradrenergic system further impact PD symptoms even alongside STN-DBS and this underlines the need for research combining pharmacological and DBS treatments.
The role of glia cells in neurotransmitter homeostasis
Glia cells, including astrocytes and microglia, are involved in inflammatory processes and contribute to neurodegeneration of the SNc in PD, as previously reviewed by McGeer and McGeer (2004, 2008). In addition, astrocytes have a role in neural communication and exhibit effects on neuronal activities (Mennerick and Zorumski, 1994; Pfrieger and Barres, 1997). For instance, astrocytes can store and release glutamate stimulating pre- and post-synaptic receptors of surrounding neurons (Norenberg, 1979). Consequently, inflammatory processes activate astrocytes in the SNc to release glutamate which project to the STN and can lead to glutamatergic neuron overactivity. This glutamatergic overactivity has also been shown to promote microglia and pro-inflammatory cytokines release that can contribute further to SNc neuronal damage (Brochard et al., 2008). Additionally, inflammatory processes can enhance α-synuclein release linked to glutamatergic excitotoxicity in the SNc (Gu et al., 2010; Sarafian et al., 2017).
Deep brain stimulation has shown to affect glia cells even starting from an initial reaction to the implantation of a DBS electrode (DiLorenzo et al., 2010). Although the glia cells cannot generate action potentials, their cellular properties allow sensitivity to voltage changes possibly including external electrical stimulation (Marrero et al., 1989; Barres et al., 1990). This electrical stimulation mainly activates astrocytes via intracellular calcium released locally on the stimulation site and to surrounding cells (Cornell-Bell and Finkbeiner, 1991; Pasti et al., 1997; Wang et al., 2006; Shigetomi et al., 2008). Moreover, calcium propagation to astrocytes could induce glutamate neurotransmission (Cornell-Bell et al., 1990; Angulo et al., 2004). DBS has been further indicated to modulate different subtypes of glia cells, including astrocytes, microglia, and macrophages that can change glial phenotypes and their functions contributing to therapeutic effects (Vedam-Mai et al., 2012). A recent experimental animal study showed STN-DBS inhibits neuroinflammatory processes in PD by modulating glia cells including astrocytes in the GP, shown in both in vivo rat models and in vitro cell cultures (Campos et al., 2020). Another experimental study showed STN-DBS inhibits microglia and normalizes neuroinflammatory cytokine levels in the SN of a rat PD model (Chen et al., 2020). As a consequence, glia cells involved in both PD and STN-DBS modulate neuroinflammatory processes, while a greater understanding of the underlying mechanisms could improve therapeutic outcomes involving the survival of SNc dopaminergic and STN glutamatergic neurons (Collier and Sortwell, 1999).
Conclusion
It has been shown that multiple neurotransmitters are involved in the neuropathology and pathophysiology of PD, including the SNc dopaminergic, LC noradrenergic, pallidal GABAergic, PPN cholinergic, DRN serotoninergic, and STN glutamatergic systems. Growing evidence supports glia cells, especially astrocytes, to be involved in PD as well, while DBS modulates these cells by mainly effecting glutamatergic neurons (Brochard et al., 2008). Although DBS improves motor symptoms in PD and continued evidence shows underlying neurochemical alterations involved in PD can be mediated by DBS, the exact mechanisms of action underlying DBS effects on neurotransmitters are still not fully understood.
Deep brain stimulation has demonstrated alleviation of motor symptoms of PD while decreasing the need for pharmaceutical dopamine treatment, indicating DBS impacts dopaminergic system. In preclinical research, STN-DBS has both local and distant effects on dopaminergic systems (Meissner et al., 2002; Walker et al., 2009; He et al., 2014; Yamamoto et al., 2014; Carcenac et al., 2015; Melon et al., 2015; Min et al., 2016; Nakajima et al., 2017; Du et al., 2018; Sahai et al., 2020). In contrast, clinical results demonstrate local impacts from STN-DBS using electrophysiological and microdialysis, but such data shows less consistency compared to distant effect from neuroimaging studies (Hilker et al., 2003; Strafella et al., 2003; Nimura et al., 2005; Hesse et al., 2008; Thobois et al., 2010; Yamamoto et al., 2015). Moreover, STN- and GPi-DBS affects glutamatergic neurons and glutamate neurotransmitter release leading to side effects, mainly dyskinesia (Boulet et al., 2006). The acute effects of DBS on the glutamatergic system were once assumed to be local and stimulus-dependent, but preclinical investigations of prolonged DBS have shown to increase glutamate in distant areas including the striatum and the SNr (Windels et al., 2000, 2003). DBS effects on the GABAergic system also differ based on specific DBS target; STN-DBS has local influence on GABA in the GPi and a distant effect on the SNr where it is more stimulation-dependent (Benazzouz and Hallett, 2000; Johnson and McIntyre, 2008). GPi-DBS also has local effects on GABA release in the GPi and remote effects in the SNr, however, ventral GPi-DBS shows alleviation in LID whereas dorsal GPi-DBS reduces bradykinesia symptoms (Krack et al., 1998; Vitek et al., 2004). STN-DBS has demonstrated superiority in treating bradykinesia and reducing Levodopa requirements (Williams et al., 2014).
The frequent occurrence of depression among PD patients suggests a role for the DRN serotoninergic system. This is stressed by STN-DBS induced inhibition in serotoninergic neurons in PD. From preclinical data, PPN-DBS has shown to improve axial symptoms in PD, although further research is needed before clinical translation can move forward (Wen et al., 2015; Sharma et al., 2020). Lastly, noradrenergic systems have recently driven attention there way as the degeneration of the LC noradrenergic system has shown to lead to DBS therapy resistance (Faggiani et al., 2015). Combined pharmacological-DBS treatments should continue be a focus in future research to investigate if other neurotransmitters including the cannabinoid and opioidergic systems may also prove to be involved in mechanistic symptom mediation (Turner et al., 2017; Han et al., 2018).
One reason neurochemical changes from DBS are not extensively covered within the literature could be from technical limitations innate to detecting transmitter release and transmitter-related charges in remote neuronal areas. Optogenetic studies investigating specific effects of neuromodulation on neurotransmitter release are warranted as they would further help assess DBS cumulative and chronic effects on local and remote neural elements. Further understanding dynamic changes in neurotransmitters will work to improve DBS effectiveness, provide more precise targeting, reduce adverse effects, and provide more appropriate pharmacological intervention options to improve overall quality of PD treatment.
Author contributions
FA: writing–original draft preparation, editing, and visualization. JB: writing–review, editing, proofreading, and visualization. ST: writing–review and editing. YT: writing–review. AJ: conceptualization, writing–review, and editing. All authors contributed to the article and approved the submitted version.
Funding
This work was supported by a VENI grant to AJ by the Nederlandse Organisatie voor Wetenschappelijk Onderzoek (NWO) with grant no: 91616043.
Acknowledgments
FA thanks King Abdulaziz University for his Ph.D. scholarship.
Conflict of interest
The authors declare that the research was conducted in the absence of any commercial or financial relationships that could be construed as a potential conflict of interest.
Publisher’s note
All claims expressed in this article are solely those of the authors and do not necessarily represent those of their affiliated organizations, or those of the publisher, the editors and the reviewers. Any product that may be evaluated in this article, or claim that may be made by its manufacturer, is not guaranteed or endorsed by the publisher.
References
Aarsland, D., and Kurz, M. W. (2010). The epidemiology of dementia associated with Parkinson disease. J. Neurol. Sci. 289, 18–22. doi: 10.1016/j.jns.2009.08.034
Agnesi, F., Johnson, M. D., and Vitek, J. L. (2013). Deep brain stimulation: How does it work? Handb. Clin. Neurol. 116, 39–54. doi: 10.1016/B978-0-444-53497-2.00004-8
Alam, M., Heissler, H. E., Schwabe, K., and Krauss, J. K. (2012). Deep brain stimulation of the pedunculopontine tegmental nucleus modulates neuronal hyperactivity and enhanced beta oscillatory activity of the subthalamic nucleus in the rat 6-hydroxydopamine model. Exp. Neurol. 233, 233–242. doi: 10.1016/j.expneurol.2011.10.006
Albares, M., Thobois, S., Favre, E., Broussolle, E., Polo, G., Domenech, P., et al. (2015). Interaction of noradrenergic pharmacological manipulation and subthalamic stimulation on movement initiation control in Parkinson’s disease. Brain Stimul. 8, 27–35. doi: 10.1016/j.brs.2014.09.002
Albin, R. L., Young, A. B., and Penney, J. B. (1989). The functional anatomy of basal ganglia disorders. Trends Neurosci. 12, 366–375. doi: 10.1016/0166-2236(89)90074-X
Alosaimi, F., Temel, Y., Hescham, S., Witzig, V. S., Almasabi, F., Tan, S. K. H., et al. (2022). High-frequency stimulation of the subthalamic nucleus induces a sustained inhibition of serotonergic system via loss of cell phenotype. Sci. Rep. 12:14011. doi: 10.1038/s41598-022-18294-6
Angulo, M. C., Kozlov, A. S., Charpak, S., and Audinat, E. (2004). Glutamate released from glial cells synchronizes neuronal activity in the hippocampus. J. Neurosci. 24, 6920–6927. doi: 10.1523/JNEUROSCI.0473-04.2004
Ashkan, K., Rogers, P., Bergman, H., and Ughratdar, I. (2017). Insights into the mechanisms of deep brain stimulation. Nat. Rev. Neurol. 13, 548–554. doi: 10.1038/nrneurol.2017.105
Aston-Jones, G. (2005). Brain structures and receptors involved in alertness. Sleep Med. 6, S3–S7. doi: 10.1016/S1389-9457(05)80002-4
Barres, B. A., Koroshetz, W. J., Chun, L. L., and Corey, D. P. (1990). Ion channel expression by white matter glia: The type-1 astrocyte. Neuron 5, 527–544. doi: 10.1016/0896-6273(90)90091-S
Benabid, A. L. (2007). What the future holds for deep brain stimulation. Expert Rev. Med. Devices 4, 895–903. doi: 10.1586/17434440.4.6.895
Benabid, A. L., Koudsié, A., Benazzouz, A., Vercueil, L., Fraix, V., Chabardes, S., et al. (2001). Deep brain stimulation of the corpus luysi (subthalamic nucleus) and other targets in Parkinson’s disease. Extension to new indications such as dystonia and epilepsy. J. Neurol. 248, 37–47. doi: 10.1007/PL00007825
Benabid, A., Pollak, P., Seigneuret, E., Hoffmann, D., Gay, E., and Perret, J. (1993). Chronic VIM thalamic stimulation in Parkinson’s disease, essential tremor and extra-pyramidal dyskinesias. Adv. Stereotact. Funct. Neurosurg. 10, 39–44. doi: 10.1007/978-3-7091-9297-9_8
Benarroch, E. E. (2009). The locus ceruleus norepinephrine system: Functional organization and potential clinical significance. Neurology 73, 1699–1704. doi: 10.1212/WNL.0b013e3181c2937c
Benazzouz, A., and Hallett, M. (2000). Mechanism of action of deep brain stimulation. Neurology 55, S13–S16.
Benazzouz, A., Piallat, B., Pollak, P., and Benabid, A. L. (1995). Responses of substantia nigra pars reticulata and globus pallidus complex to high frequency stimulation of the subthalamic nucleus in rats: Electrophysiological data. Neurosci. Lett. 189, 77–80. doi: 10.1016/0304-3940(95)11455-6
Bergman, H., Wichmann, T., Karmon, B., and DeLong, M. (1994). The primate subthalamic nucleus. II. Neuronal activity in the MPTP model of parkinsonism. J. Neurophysiol. 72, 507–520. doi: 10.1152/jn.1994.72.2.507
Berridge, C. W., Schmeichel, B. E., and España, R. A. (2012). Noradrenergic modulation of wakefulness/arousal. Sleep Med. Rev. 16, 187–197. doi: 10.1016/j.smrv.2011.12.003
Beurrier, C., Bioulac, B., Audin, J., and Hammond, C. (2001). High-frequency stimulation produces a transient blockade of voltage-gated currents in subthalamic neurons. J. Neurophysiol. 85, 1351–1356. doi: 10.1152/jn.2001.85.4.1351
Bevan, M. D., Atherton, J. F., and Baufreton, J. (2006). Cellular principles underlying normal and pathological activity in the subthalamic nucleus. Curr. Opin. Neurobiol. 16, 621–628. doi: 10.1016/j.conb.2006.10.003
Boulet, S., Lacombe, E., Carcenac, C., Feuerstein, C., Sgambato-Faure, V., Poupard, A., et al. (2006). Subthalamic stimulation-induced forelimb dyskinesias are linked to an increase in glutamate levels in the substantia nigra pars reticulata. J. Neurosci. 26, 10768–10776. doi: 10.1523/JNEUROSCI.3065-06.2006
Braak, H., Del Tredici, K., Rüb, U., De Vos, R. A., Steur, E. N. J., and Braak, E. (2003). Staging of brain pathology related to sporadic Parkinson’s disease. Neurobiol. Aging 24, 197–211. doi: 10.1016/S0197-4580(02)00065-9
Breit, S., Schulz, J. B., and Benabid, A. L. (2004). Deep brain stimulation. Cell Tissue Res. 318, 275–288. doi: 10.1007/s00441-004-0936-0
Breton-Provencher, V., Drummond, G. T., and Sur, M. (2021). Locus coeruleus norepinephrine in learned behavior: Anatomical modularity and spatiotemporal integration in targets. Front. Neural Circuits 15:638007. doi: 10.3389/fncir.2021.638007
Brochard, V., Combadière, B., Prigent, A., Laouar, Y., Perrin, A., Beray-Berthat, V., et al. (2008). Infiltration of CD4+ lymphocytes into the brain contributes to neurodegeneration in a mouse model of Parkinson disease. J. Clin. Investig. 119, 182–192. doi: 10.1172/JCI36470
Brown, P., Oliviero, A., Mazzone, P., Insola, A., Tonali, P., and Di Lazzaro, V. (2001). Dopamine dependency of oscillations between subthalamic nucleus and pallidum in Parkinson’s disease. J. Neurosci. 21, 1033–1038. doi: 10.1523/JNEUROSCI.21-03-01033.2001
Caley, C. F. (1998). Extrapyramidal reactions from concurrent SSRI and atypical antipsychotic use. Can. J. Psychiatry 43, 307–308. doi: 10.1017/S0790966700003669
Calon, F., and Di Paolo, T. (2002). Levodopa response motor complications—GABA receptors and preproenkephalin expression in human brain. Parkinsonism Related Disord. 8, 449–454. doi: 10.1016/s1353-8020(02)00029-9
Campbell, G. A., Eckardt, M. J., and Weight, F. F. (1985). Dopaminergic mechanisms in subthalamic nucleus of rat: Analysis using horseradish peroxidase and microiontophoresis. Brain Res. 333, 261–270. doi: 10.1016/0006-8993(85)91580-x
Campos, A. C. P., Kikuchi, D. S., Paschoa, A. F. N., Kuroki, M. A., Fonoff, E. T., Hamani, C., et al. (2020). Unraveling the role of astrocytes in subthalamic nucleus deep brain stimulation in a Parkinson’s disease rat model. Cell. Mol. Neurobiol. 40, 939–954. doi: 10.1007/s10571-019-00784-3
Carcenac, C., Favier, M., Vachez, Y., Lacombe, E., Carnicella, S., Savasta, M., et al. (2015). Subthalamic deep brain stimulation differently alters striatal dopaminergic receptor levels in rats. Mov. Disord. 30, 1739–1749. doi: 10.1002/mds.26146
Carroll, C., Holloway, V., Brotchie, J., and Mitchell, I. (1995). Neurochemical and behavioural investigations of the NMDA receptor-associated glycine site in the rat striatum: Functional implications for treatment of parkinsonian symptoms. Psychopharmacology 119, 55–65. doi: 10.1007/BF02246054
Chassain, C., Melon, C., Salin, P., Vitale, F., Couraud, S., Durif, F., et al. (2016). Metabolic, synaptic and behavioral impact of 5-week chronic deep brain stimulation in hemiparkinsonian rats. J. Neurochem. 136, 1004–1016. doi: 10.1111/jnc.13438
Chen, Y., Zhu, G., Liu, D., Zhang, X., Liu, Y., Yuan, T., et al. (2020). Subthalamic nucleus deep brain stimulation suppresses neuroinflammation by Fractalkine pathway in Parkinson’s disease rat model. Brain Behav. Immun. 90, 16–25. doi: 10.1016/j.bbi.2020.07.035
Cheney, P., Griffin, D., and Van Acker, G. III (2013). Neural hijacking: Action of high-frequency electrical stimulation on cortical circuits. Neuroscientist 19, 434–441. doi: 10.1177/1073858412458368
Coenen, V., Gielen, F., Castro-Prado, F., Abdel Rahman, A., and Honey, C. (2008). Noradrenergic modulation of subthalamic nucleus activity in human: Metoprolol reduces spiking activity in microelectrode recordings during deep brain stimulation surgery for Parkinson’s disease. Acta Neurochir. 150, 757–762. doi: 10.1007/s00701-008-1619-5
Collier, T. J., and Sortwell, C. E. (1999). Therapeutic potential of nerve growth factors in Parkinson’s disease. Drugs Aging 14, 261–287. doi: 10.2165/00002512-199914040-00003
Cornell-Bell, A. H., Finkbeiner, S. M., Cooper, M. S., and Smith, S. J. (1990). Glutamate induces calcium waves in cultured astrocytes: Long-range glial signaling. Science 247, 470–473. doi: 10.1126/science.1967852
Cornell-Bell, A., and Finkbeiner, S. (1991). Ca2+ waves in astrocytes. Cell Calcium 12, 185–204. doi: 10.1016/0143-4160(91)90020-F
Creed-Carson, M., Oraha, A., and Nobrega, J. N. (2011). Effects of 5-HT(2A) and 5-HT(2C) receptor antagonists on acute and chronic dyskinetic effects induced by haloperidol in rats. Behav. Brain Res. 219, 273–279. doi: 10.1016/j.bbr.2011.01.025
DeLong, M. R. (1990). Primate models of movement disorders of basal ganglia origin. Trends Neurosci. 13, 281–285. doi: 10.1016/0166-2236(90)90110-V
Dexter, D. T., and Jenner, P. (2013). Parkinson disease: From pathology to molecular disease mechanisms. Free Radic. Biol. Med. 62, 132–144. doi: 10.1016/j.freeradbiomed.2013.01.018
DiLorenzo, D. J., Jankovic, J., Simpson, R. K., Takei, H., and Powell, S. Z. (2010). Long-term deep brain stimulation for essential tremor: 12-year clinicopathologic follow-up. Mov. Disord. 25, 232–238. doi: 10.1002/mds.22935
Dostrovsky, J., Levy, R., Wu, J., Hutchison, W., Tasker, R., and Lozano, A. (2000). Microstimulation-induced inhibition of neuronal firing in human globus pallidus. J. Neurophysiol. 84, 570–574. doi: 10.1152/jn.2000.84.1.570
Du, T. T., Chen, Y. C., Lu, Y. Q., Meng, F. G., Yang, H., and Zhang, J. G. (2018). Subthalamic nucleus deep brain stimulation protects neurons by activating autophagy via PP2A inactivation in a rat model of Parkinson’s disease. Exp. Neurol. 306, 232–242. doi: 10.1016/j.expneurol.2018.05.017
Espay, A. J., LeWitt, P. A., and Kaufmann, H. (2014). Norepinephrine deficiency in Parkinson’s disease: The case for noradrenergic enhancement. Mov. Disord. 29, 1710–1719. doi: 10.1002/mds.26048
Eusebio, A., and Brown, P. (2009). Synchronisation in the beta frequency-band—the bad boy of parkinsonism or an innocent bystander? Exp. Neurol. 217, 1–3. doi: 10.1016/j.expneurol.2009.02.003
Faggiani, E., Delaville, C., and Benazzouz, A. (2015). The combined depletion of monoamines alters the effectiveness of subthalamic deep brain stimulation. Neurobiol. Dis. 82, 342–348. doi: 10.1016/j.nbd.2015.07.010
Feinstein, D. L., Kalinin, S., and Braun, D. (2016). Causes, consequences, and cures for neuroinflammation mediated via the locus coeruleus: Noradrenergic signaling system. J. Neurochem. 139, 154–178. doi: 10.1111/jnc.13447
Fischer, D. L., Kemp, C. J., Cole-Strauss, A., Polinski, N. K., Paumier, K. L., Lipton, J. W., et al. (2017). Subthalamic nucleus deep brain stimulation employs trkB signaling for neuroprotection and functional restoration. J. Neurosci. 37, 6786–6796. doi: 10.1523/JNEUROSCI.2060-16.2017
Foote, S. L., Bloom, F. E., and Aston-Jones, G. (1983). Nucleus locus ceruleus: New evidence of anatomical and physiological specificity. Physiol. Rev. 63, 844–914. doi: 10.1152/physrev.1983.63.3.844
Foote, S., Aston-Jones, G., and Bloom, F. (1980). Impulse activity of locus coeruleus neurons in awake rats and monkeys is a function of sensory stimulation and arousal. Proc. Natl. Acad. Sci. U. S. A. 77, 3033–3037. doi: 10.1073/pnas.77.5.3033
Green, J., McDonald, W., Vitek, J., Evatt, M., Freeman, A., Haber, M., et al. (2002). Cognitive impairments in advanced PD without dementia. Neurology 59, 1320–1324. doi: 10.1212/01.WNL.0000031426.21683.E2
Greenamyre, J. T. (2001). Glutamatergic influences on the basal ganglia. Clin. Neuropharmacol. 24, 65–70. doi: 10.1097/00002826-200103000-00001
Gu, X. L., Long, C. X., Sun, L., Xie, C., Lin, X., and Cai, H. (2010). Astrocytic expression of Parkinson’s disease-related A53T α-synuclein causes neurodegeneration in mice. Mol. Brain 3, 1–16. doi: 10.1186/1756-6606-3-12
Guimarães, J., Moura, E., Silva, E., Aguiar, P., Garrett, C., and Vieira-Coelho, M. A. (2013). Locus coeruleus is involved in weight loss in a rat model of Parkinson’s disease: An effect reversed by deep brain stimulation. Brain Stimul. 6, 845–855. doi: 10.1016/j.brs.2013.06.002
Guimarães, J., Moura, E., Vieira-Coelho, M. A., and Garrett, C. (2012). Weight variation before and after surgery in Parkinson’s disease: A noradrenergic modulation? Mov. Disord. 27, 1078–1082. doi: 10.1002/mds.25063
Han, D. J., He, Z. G., and Yang, H. (2018). Melanocortin-4 receptor in subthalamic nucleus is involved in the modulation of nociception. Am. J. Clin. Exp. Immunol. 7:76.
Hartung, H., Tan, S., Steinbusch, H., Temel, Y., and Sharp, T. (2011). High-frequency stimulation of the subthalamic nucleus inhibits the firing of juxtacellular labelled 5-HT-containing neurones. Neuroscience 186, 135–145. doi: 10.1016/j.neuroscience.2011.04.004
Hashimoto, T., Elder, C. M., Okun, M. S., Patrick, S. K., and Vitek, J. L. (2003). Stimulation of the subthalamic nucleus changes the firing pattern of pallidal neurons. J. Neurosci. 23, 1916–1923. doi: 10.1523/JNEUROSCI.23-05-01916.2003
He, Z., Jiang, Y., Xu, H., Jiang, H., Jia, W., Sun, P., et al. (2014). High frequency stimulation of subthalamic nucleus results in behavioral recovery by increasing striatal dopamine release in 6-hydroxydopamine lesioned rat. Behav. Brain Res. 263, 108–114. doi: 10.1016/j.bbr.2014.01.014
Heckers, S., Geula, C., and Mesulam, M. M. (1992). Cholinergic innervation of the human thalamus: Dual origin and differential nuclear distribution. J. Comp. Neurol. 325, 68–82. doi: 10.1002/cne.903250107
Henning, J., Koczan, D., Glass, A., Karopka, T., Pahnke, J., Rolfs, A., et al. (2007). Deep brain stimulation in a rat model modulates TH, CaMKIIa and Homer1 gene expression. Eur. J. Neurosci. 25, 239–250. doi: 10.1111/j.1460-9568.2006.05264.x
Hesse, S., Strecker, K., Winkler, D., Luthardt, J., Scherfler, C., Reupert, A., et al. (2008). Effects of subthalamic nucleus stimulation on striatal dopaminergic transmission in patients with Parkinson’s disease within one-year follow-up. J. Neurol. 255, 1059–1066. doi: 10.1007/s00415-008-0849-z
Hilker, R., Voges, J., Ghaemi, M., Lehrke, R., Rudolf, J., Koulousakis, A., et al. (2003). Deep brain stimulation of the subthalamic nucleus does not increase the striatal dopamine concentration in parkinsonian humans. Mov. Disord. 18, 41–48. doi: 10.1002/mds.10297
Hirsch, E., Graybiel, A. M., and Agid, Y. A. (1988). Melanized dopaminergic neurons are differentially susceptible to degeneration in Parkinson’s disease. Nature 334, 345–348. doi: 10.1038/334345a0
Jahanshahi, A., Mai, J. K., and Temel, Y. (2020). “Anatomy of Targets for Deep Brain Stimulation,” in Fundamentals and Clinics of Deep Brain Stimulation, eds Y. Temel, A. F. G. Leentjens, R. M. A. de Bie, S. Chabardes, and A. Fasano (Cham: Springer), 15–28. doi: 10.1007/978-3-030-36346-8_2
Johnson, M. D., and McIntyre, C. C. (2008). Quantifying the neural elements activated and inhibited by globus pallidus deep brain stimulation. J. Neurophysiol. 100, 2549–2563. doi: 10.1152/jn.90372.2008
Kapur, S., Zipursky, R. B., and Remington, G. (1999). Clinical and theoretical implications of 5-HT2 and D2 receptor occupancy of clozapine, risperidone, and olanzapine in schizophrenia. Am. J. Psychiatry 156, 286–293. doi: 10.1176/ajp.156.2.286
Karain, B., Xu, D., Bellone, J. A., Hartman, R. E., and Shi, W. X. (2015). Rat globus pallidus neurons: Functional classification and effects of dopamine depletion. Synapse 69, 41–51. doi: 10.1002/syn.21783
Karl, J. A., Joyce, J., Ouyang, B., and Verhagen Metman, L. (2022). Long-Term Clinical Experience with Directional Deep Brain Stimulation Programming: A Retrospective Review. Neurol. Ther. 11, 1309–1318. doi: 10.1007/s40120-022-00381-5
Khaindrava, V., Salin, P., Melon, C., Ugrumov, M., Kerkerian-Le-Goff, L., and Daszuta, A. (2011). High frequency stimulation of the subthalamic nucleus impacts adult neurogenesis in a rat model of Parkinson’s disease. Neurobiol. Dis. 42, 284–291. doi: 10.1016/j.nbd.2011.01.018
Kilpatrick, M., Church, E., Danish, S., Stiefel, M., Jaggi, J., Halpern, C., et al. (2010). Intracerebral microdialysis during deep brain stimulation surgery. J. Neurosci. Methods 190, 106–111. doi: 10.1016/j.jneumeth.2010.04.013
Kitai, S., and Deniau, J. (1981). Cortical inputs to the subthalamus: Intracellular analysis. Brain Res. 214, 411–415. doi: 10.1016/0006-8993(81)91204-x
Krack, P., Pollak, P., Limousin, P., Hoffmann, D., Benazzouz, A., Le Bas, J., et al. (1998). Opposite motor effects of pallidal stimulation in Parkinson’s disease. Ann. Neurol. 43, 180–192. doi: 10.1002/ana.410430208
Lauterbach, E. C., and Shillcutt, S. D. (1997). Dyskinesia with fluoxetine: Tardive or late-onset persistent, acute norfluoxetine dyskinesia? J. Clin. Psychiatry 58, 85–86. doi: 10.4088/JCP.v58n0206b
Lavoie, B., and Parent, A. (1994). Pedunculopontine nucleus in the squirrel monkey: Projections to the basal ganglia as revealed by anterograde tract-tracing methods. J. Comp. Neurol. 344, 210–231. doi: 10.1002/cne.903440204
Lee, K. H., Kristic, K., van Hoff, R., Hitti, F. L., Blaha, C., Harris, B., et al. (2007). High-frequency stimulation of the subthalamic nucleus increases glutamate in the subthalamic nucleus of rats as demonstrated by in vivo enzyme-linked glutamate sensor. Brain Res. 1162, 121–129. doi: 10.1016/j.brainres.2007.06.021
Levy, R., Hutchison, W. D., Lozano, A. M., and Dostrovsky, J. O. (2000). High-frequency synchronization of neuronal activity in the subthalamic nucleus of parkinsonian patients with limb tremor. J. Neurosci. 20, 7766–7775. doi: 10.1523/JNEUROSCI.20-20-07766.2000
Lin, M., and Yang, J. (1994). Stimulation of the nigrostriatal dopamine system produces hypertension and tachycardia in rats. Am. J. Physiol. Heart Circ. Physiol. 266, H2489–H2496. doi: 10.1152/ajpheart.1994.266.6.H2489
Liu, C. (2020). Targeting the cholinergic system in Parkinson’s disease. Acta Pharmacol. Sin. 41, 453–463. doi: 10.1038/s41401-020-0380-z
Lozano, A. M., and Eltahawy, H. (2004). How does DBS work?. Suppl. Clin. Neurophysiol. 57, 733–736. doi: 10.1016/S1567-424X(09)70414-3
Marrero, H., Astion, M. L., Coles, J. A., and Orkand, R. K. (1989). Facilitation of voltage-gated ion channels in frog neuroglia by nerve impulses. Nature 339, 378–380. doi: 10.1038/339378a0
Marsden, C., and Parkes, J. (1977). Success and problems of long-term levodopa therapy in Parkinson’s disease. Lancet 309, 345–349. doi: 10.1016/S0140-6736(77)91146-1
Martin-Bastida, A., Pietracupa, S., and Piccini, P. (2017). Neuromelanin in parkinsonian disorders: An update. Int. J. Neurosci. 127, 1116–1123. doi: 10.1080/00207454.2017.1325883
Martinez, R. C., Hamani, C., de Carvalho, M. C., de Oliveira, A. R., Alho, E., Navarro, J., et al. (2013). Intraoperative dopamine release during globus pallidus internus stimulation in Parkinson’s disease. Mov. Disord. 28, 2027–2032. doi: 10.1002/mds.25691
Mayeux, R., Stern, Y., Rosen, J., and Leventhal, J. (1981). Depression, intellectual impairment, and Parkinson disease. Neurology 31, 645–645. doi: 10.1212/WNL.31.6.645
McGeer, P. L., and McGeer, E. G. (2004). Inflammation and neurodegeneration in Parkinson’s disease. Parkinsonism Related Disord. 10, S3–S7. doi: 10.1016/j.parkreldis.2004.01.005
McGeer, P. L., and McGeer, E. G. (2008). Glial reactions in Parkinson’s disease. Mov. Disord. 23, 474–483. doi: 10.1002/mds.21751
McIntyre, C. C., Savasta, M., Kerkerian-Le Goff, L., and Vitek, J. L. (2004). Uncovering the mechanism (s) of action of deep brain stimulation: Activation, inhibition, or both. Clin. Neurophysiol. 115, 1239–1248. doi: 10.1016/j.clinph.2003.12.024
McMillan, P. J., White, S. S., Franklin, A., Greenup, J. L., Leverenz, J. B., Raskind, M. A., et al. (2011). Differential response of the central noradrenergic nervous system to the loss of locus coeruleus neurons in Parkinson’s disease and Alzheimer’s disease. Brain Res. 1373, 240–252. doi: 10.1016/j.brainres.2010.12.015
Meissner, W., Harnack, D., Paul, G., Reum, T., Sohr, R., Morgenstern, R., et al. (2002). Deep brain stimulation of subthalamic neurons increases striatal dopamine metabolism and induces contralateral circling in freely moving 6-hydroxydopamine-lesioned rats. Neurosci. Lett. 328, 105–108. doi: 10.1016/s0304-3940(02)00463-9
Meissner, W., Reum, T., Paul, G., Harnack, D., Sohr, R., Morgenstern, R., et al. (2001). Striatal dopaminergic metabolism is increased by deep brain stimulation of the subthalamic nucleus in 6-hydroxydopamine lesioned rats. Neurosci. Lett. 303, 165–168. doi: 10.1016/S0304-3940(01)01758-X
Melon, C., Chassain, C., Bielicki, G., Renou, J. P., Kerkerian-Le Goff, L., Salin, P., et al. (2015). Progressive brain metabolic changes under deep brain stimulation of subthalamic nucleus in parkinsonian rats. J. Neurochem. 132, 703–712. doi: 10.1111/jnc.13015
Mennerick, S., and Zorumski, C. F. (1994). Glial contributions to excitatory neurotransmission in cultured hippocampal cells. Nature 368, 59–62. doi: 10.1038/368059a0
Mesulam, M. M., and Geula, C. (1988). Nucleus basalis (Ch4) and cortical cholinergic innervation in the human brain: Observations based on the distribution of acetylcholinesterase and choline acetyltransferase. J. Comp. Neurol. 275, 216–240. doi: 10.1002/cne.902750205
Mesulam, M. M., Mash, D., Hersh, L., Bothwell, M., and Geula, C. (1992). Cholinergic innervation of the human striatum, globus pallidus, subthalamic nucleus, substantia nigra, and red nucleus. J. Comp. Neurol. 323, 252–268. doi: 10.1002/cne.903230209
Milosevic, L., Gramer, R., Kim, T. H., Algarni, M., Fasano, A., Kalia, S. K., et al. (2019). Modulation of inhibitory plasticity in basal ganglia output nuclei of patients with Parkinson’s disease. Neurobiol. Dis. 124, 46–56. doi: 10.1016/j.nbd.2018.10.020
Milosevic, L., Kalia, S. K., Hodaie, M., Lozano, A. M., Fasano, A., Popovic, M. R., et al. (2018). Neuronal inhibition and synaptic plasticity of basal ganglia neurons in Parkinson’s disease. Brain 141, 177–190. doi: 10.1093/brain/awx296
Min, H. K., Ross, E. K., Jo, H. J., Cho, S., Settell, M. L., Jeong, J. H., et al. (2016). Dopamine release in the nonhuman primate caudate and putamen depends upon site of stimulation in the subthalamic nucleus. J. Neurosci. 36, 6022–6029. doi: 10.1523/JNEUROSCI.0403-16.2016
Mouroux, M., Hassani, O. K., and Feger, J. (1995). Electrophysiological study of the excitatory parafascicular projection to the subthalamic nucleus and evidence for ipsi-and contralateral controls. Neuroscience 67, 399–407. doi: 10.1016/0306-4522(95)00032-e
Muthuraman, M., Palotai, M., ávor-Duray, B. J., Kelemen, A., Koirala, N., Halász, L., et al. (2021). Frequency-specific network activity predicts bradykinesia severity in Parkinson’s disease. NeuroImage 32:102857. doi: 10.1016/j.nicl.2021.102857
Nakajima, A., Shimo, Y., Uka, T., and Hattori, N. (2017). Subthalamic nucleus and globus pallidus interna influence firing of tonically active neurons in the primate striatum through different mechanisms. Eur. J. Neurosci. 46, 2662–2673. doi: 10.1111/ejn.13726
Nakao, N., Nakai, E., Nakai, K., and Itakura, T. (1999). Ablation of the subthalamic nucleus supports the survival of nigral dopaminergic neurons after nigrostriatal lesions induced by the mitochondrial toxin 3-nitropropionic acid. Ann. Neurol. 45, 640–651. doi: 10.1002/1531-8249(199905)45:5<640::AID-ANA13<3.0.CO;2-U
Nambu, A., Tokuno, H., and Takada, M. (2002). Functional significance of the cortico-subthalamo-pallidal ‘hyperdirect’ pathway. Neurosci. Res. 43, 111–117. doi: 10.1016/S0168-0102(02)00027-5
Nauta, H., and Cuenod, M. (1982). Perikaryal cell labeling in the subthalamic nucleus following the injection of 3H-γ-aminobutyric acid into the pallidal complex: An autoradiographic study in cat. Neuroscience 7, 2725–2734. doi: 10.1016/0306-4522(82)90096-3
Navailles, S., Benazzouz, A., Bioulac, B., Gross, C., and De Deurwaerdere, P. (2010). High-frequency stimulation of the subthalamic nucleus and L-3, 4-dihydroxyphenylalanine inhibit in vivo serotonin release in the prefrontal cortex and hippocampus in a rat model of Parkinson’s disease. J. Neurosci. 30, 2356–2364. doi: 10.1523/JNEUROSCI.5031-09.2010
Nimura, T., Yamaguchi, K., Ando, T., Shibuya, S., Oikawa, T., Nakagawa, A., et al. (2005). Attenuation of fluctuating striatal synaptic dopamine levels in patients with Parkinson disease in response to subthalamic nucleus stimulation: A positron emission tomography study. J. Neurosurg. 103, 968–973. doi: 10.3171/jns.2005.103.6.0968
Norenberg, M. D. (1979). Distribution of glutamine synthetase in the rat central nervous system. J. Histochem. Cytochem. 27, 756–762. doi: 10.1177/27.3.39099
Obeso, J. A., Rodriguez-Oroz, M. C., Rodriguez, M., DeLong, M. R., and Olanow, C. W. (2000). Pathophysiology of levodopa-induced dyskinesias in Parkinson’s disease: Problems with the current model. Ann. Neurol. 47, S22–S32.
Ogura, M., Nakao, N., Nakai, E., Uematsu, Y., and Itakura, T. (2004). The mechanism and effect of chronic electrical stimulation of the globus pallidus for treatment of Parkinson disease. J. Neurosurg. 100, 997–1001. doi: 10.3171/jns.2004.100.6.0997
Ohtsuka, C., Sasaki, M., Konno, K., Koide, M., Kato, K., Takahashi, J., et al. (2013). Changes in substantia nigra and locus coeruleus in patients with early-stage Parkinson’s disease using neuromelanin-sensitive MR imaging. Neurosci. Lett. 541, 93–98. doi: 10.1016/j.neulet.2013.02.012
Olanow, C. (2002). Surgical therapy for Parkinson’s disease. Eur. J. Neurol. 9, 31–39. doi: 10.1046/j.1468-1331.9.s3.4.x
Olney, J. W., Misra, C. H., and de Gubareff, T. (1975). Cysteine-S-sulfate: Brain damaging metabolite in sulfite oxidase deficiency. J. Neuropathol. Exp. Neurol. 34, 167–177. doi: 10.1097/00005072-197503000-00005
Olsen, R. W., and Li, G. D. (2012). “Gaba,” in Basic neurochemistry, eds S. Brady, G. Siegel, R. W. Albers, and D. L. Price (Amsterdam: Elsevier), 367–376. doi: 10.1016/B978-0-12-374947-5.00018-3
Park, E., Song, I., Jang, D. P., and Kim, I. Y. (2014). The effect of low frequency stimulation of the pedunculopontine tegmental nucleus on basal ganglia in a rat model of Parkinson’s disease. Neurosci. Lett. 577, 16–21. doi: 10.1016/j.neulet.2014.05.062
Pasti, L., Volterra, A., Pozzan, T., and Carmignoto, G. (1997). Intracellular calcium oscillations in astrocytes: A highly plastic, bidirectional form of communication between neurons and astrocytes in situ. J. Neurosci. 17, 7817–7830. doi: 10.1523/JNEUROSCI.17-20-07817.1997
Pfrieger, F. W., and Barres, B. A. (1997). Synaptic efficacy enhanced by glial cells in vitro. Science 277, 1684–1687. doi: 10.1126/science.277.5332.1684
Piallat, B., Benazzouz, A., and Benabid, A. L. (1996). Subthalamic nucleus lesion in rats prevents dopaminergic nigral neuron degeneration after striatal 6-OHDA injection: Behavioural and immunohistochemical studies. Eur. J. Neurosci. 8, 1408–1414. doi: 10.1111/j.1460-9568.1996.tb01603.x
Preston, R., McCrea, R., Chang, H., and Kitai, S. (1981). Anatomy and physiology of substantia nigra and retrorubral neurons studied by extra-and intracellular recording and by horseradish peroxidase labeling. Neuroscience 6, 331–344. doi: 10.1016/0306-4522(81)90127-5
Przedborski, S. (2005). Pathogenesis of nigral cell death in Parkinson’s disease. Parkinsonism Related Disord. 11, S3–S7. doi: 10.1016/j.parkreldis.2004.10.012
Quinn, N. (1999). Progress in functional neurosurgery for Parkinson’s disease. Lancet 354, 1658–1659. doi: 10.1016/S0140-6736(99)00292-5
Rauch, F., Schwabe, K., and Krauss, J. K. (2010). Effect of deep brain stimulation in the pedunculopontine nucleus on motor function in the rat 6-hydroxydopamine Parkinson model. Behav. Brain Res. 210, 46–53. doi: 10.1016/j.bbr.2010.02.003
Rodriguez, M., Obeso, J., and Olanow, C. W. (1998). Subthalamic nucleus-mediated excitotoxicity in Parkinson’s disease: A target for neuroprotection. Ann. Neurol. 44, S175–S188. doi: 10.1002/ana.410440726
Rommelfanger, K., and Weinshenker, D. (2007). Norepinephrine: The redheaded stepchild of Parkinson’s disease. Biochem. Pharmacol. 74, 177–190. doi: 10.1016/j.bcp.2007.01.036
Rosa, M., Giannicola, G., Marceglia, S., Fumagalli, M., Barbieri, S., and Priori, A. (2012). Neurophysiology of deep brain stimulation. Int. Rev. Neurobiol. 107, 23–55. doi: 10.1016/B978-0-12-404706-8.00004-8
Sahai, S., Effendi, E. T., Mahoney, E. C., Tucker, H. R., Moolick, B. J., Mamone, G., et al. (2020). Effects of subthalamic nucleus deep brain stimulation on neuronal spiking activity in the substantia nigra pars compacta in a rat model of Parkinson’s disease. Neurosci. Lett. 739:135443. doi: 10.1016/j.neulet.2020.135443
Saji, M., Blau, A. D., and Volpe, B. T. (1996). Prevention of transneuronal degeneration of neurons in the substantia nigra reticulata by ablation of the subthalamic nucleus. Exp. Neurol. 141, 120–129. doi: 10.1006/exnr.1996.0145
Salin, P., Manrique, C., Forni, C., and Kerkerian-Le Goff, L. (2002). High-frequency stimulation of the subthalamic nucleus selectively reverses dopamine denervation-induced cellular defects in the output structures of the basal ganglia in the rat. J. Neurosci. 22, 5137–5148. doi: 10.1523/JNEUROSCI.22-12-05137.2002
Sarafian, T. A., Littlejohn, K., Yuan, S., Fernandez, C., Cilluffo, M., Koo, B. K., et al. (2017). Stimulation of synaptoneurosome glutamate release by monomeric and fibrillated α−synuclein. J. Neurosci. Res. 95, 1871–1887. doi: 10.1002/jnr.24024
Sarkar, S., Raymick, J., and Imam, S. (2016). Neuroprotective and therapeutic strategies against Parkinson’s disease: Recent perspectives. Int. J. Mol. Sci. 17:904. doi: 10.3390/ijms17060904
Sasaki, M., Shibata, E., Tohyama, K., Takahashi, J., Otsuka, K., Tsuchiya, K., et al. (2006). Neuromelanin magnetic resonance imaging of locus ceruleus and substantia nigra in Parkinson’s disease. Neuroreport 17, 1215–1218. doi: 10.1097/01.wnr.0000227984.84927.a7
Savasta, M., Windels, F., Bruet, N., Bertrand, A., and Poupard, A. (2002). “Neurochemical modifications induced by high frequency stimulation of the subthalamic nucleus in rats,” in The Basal Ganglia VII. Advances in Behavioral Biology, eds L. F. B. Nicholson and R. L. M. Faull (Boston, MA: Springer), 581–590. doi: 10.1007/978-1-4615-0715-4_58
Schmidt, W. (1995). Balance of transmitter activities in the basal ganglia loops. J. Neural Transm. Suppl. 46, 67–76.
Schöls, L., Reimold, M., Seidel, K., Globas, C., Brockmann, K., Karsten Hauser, T., et al. (2015). No parkinsonism in SCA2 and SCA3 despite severe neurodegeneration of the dopaminergic substantia nigra. Brain 138, 3316–3326. doi: 10.1093/brain/awv255
Sharma, P. K., Wells, L., Rizzo, G., Elson, J. L., Passchier, J., Rabiner, E. A., et al. (2020). DREADD activation of pedunculopontine cholinergic neurons reverses motor deficits and restores striatal dopamine signaling in parkinsonian rats. Neurotherapeutics 17, 1120–1141. doi: 10.1007/s13311-019-00830-4
Shigetomi, E., Bowser, D. N., Sofroniew, M. V., and Khakh, B. S. (2008). Two forms of astrocyte calcium excitability have distinct effects on NMDA receptor-mediated slow inward currents in pyramidal neurons. J. Neurosci. 28, 6659–6663. doi: 10.1523/JNEUROSCI.1717-08.2008
Shon, Y. M., Lee, K. H., Goerss, S. J. I, Kim, Y., Kimble, C., Van Gompel, J. J., et al. (2010). High frequency stimulation of the subthalamic nucleus evokes striatal dopamine release in a large animal model of human DBS neurosurgery. Neurosci. Lett. 475, 136–140. doi: 10.1016/j.neulet.2010.03.060
Singh, S. (2020). Noradrenergic pathways of locus coeruleus in Parkinson’s and Alzheimer’s pathology. Int. J. Neurosci. 130, 251–261. doi: 10.1080/00207454.2019.1667799
Smith, G. S., Mills, K. A., Pontone, G. M., Anderson, W. S., Perepezko, K. M., Brasic, J., et al. (2019). Effect of STN DBS on vesicular monoamine transporter 2 and glucose metabolism in Parkinson’s disease. Parkinsonism Related Disord. 64, 235–241. doi: 10.1016/j.parkreldis.2019.04.006
Smith, K., Fairburn, C., and Cowen, P. (1997). Relapse of depression after rapid depletion of tryptophan. Lancet 349, 915–919.
Smith, Y., Charara, A., and Parent, A. (1996). Synaptic innervation of midbrain dopaminergic neurons by glutamate-enriched terminals in the squirrel monkey. J. Comp. Neurol. 364, 231–253. doi: 10.1002/(SICI)1096-9861(19960108)364:2<231::AID-CNE4<3.0.CO;2-6
Spieles-Engemann, A. L., Steece-Collier, K., Behbehani, M. M., Collier, T. J., Wohlgenant, S. L., Kemp, C. J., et al. (2011). Subthalamic nucleus stimulation increases brain derived neurotrophic factor in the nigrostriatal system and primary motor cortex. J. Parkinsons Dis. 1, 123–136. doi: 10.3233/JPD-2011-11008
Stefani, A. (2011). Reduced GABA content in the motor thalamus during effective deep brain stimulation of the subthalamic nucleus. Front. Syst. Neurosci. 5:17. doi: 10.3389/fnsys.2011.00017
Stefani, A., Fedele, E., Vitek, J., Pierantozzi, M., Galati, S., Marzetti, F., et al. (2011). The clinical efficacy of L-DOPA and STN-DBS share a common marker: Reduced GABA content in the motor thalamus. Cell Death Dis. 2:e154. doi: 10.1038/cddis.2011.35
Strafella, A. P., Sadikot, A. F., and Dagher, A. (2003). Subthalamic deep brain stimulation does not induce striatal dopamine release in Parkinson’s disease. Neuroreport 14, 1287–1289. doi: 10.1097/00001756-200307010-00020
Strowd, R. E., Cartwright, M. S., Passmore, L. V., Ellis, T. L., Tatter, S. B., and Siddiqui, M. S. (2010). Weight change following deep brain stimulation for movement disorders. J. Neurol. 257, 1293–1297. doi: 10.1007/s00415-010-5509-4
Tan, S. K., Hartung, H., Sharp, T., and Temel, Y. (2011a). Serotonin-dependent depression in Parkinson’s disease: A role for the subthalamic nucleus? Neuropharmacology 61, 387–399. doi: 10.1016/j.neuropharm.2011.01.006
Tan, S. K., Hartung, H., Visser-Vandewalle, V., Steinbusch, H. W., Temel, Y., and Sharp, T. (2012). A combined in vivo neurochemical and electrophysiological analysis of the effect of high-frequency stimulation of the subthalamic nucleus on 5-HT transmission. Exp. Neurol. 233, 145–153. doi: 10.1016/j.expneurol.2011.08.027
Tan, S. K., Janssen, M. L., Jahanshahi, A., Chouliaras, L., Visser-Vandewalle, V., Lim, L. W., et al. (2011b). High frequency stimulation of the subthalamic nucleus increases c-fos immunoreactivity in the dorsal raphe nucleus and afferent brain regions. J. Psychiatr. Res. 45, 1307–1315.
Temel, Y., Boothman, L. J., Blokland, A., Magill, P. J., Steinbusch, H. W., Visser-Vandewalle, V., et al. (2007). Inhibition of 5-HT neuron activity and induction of depressive-like behavior by high-frequency stimulation of the subthalamic nucleus. Proc. Natl. Acad. Sci. U. S. A. 104, 17087–17092. doi: 10.1073/pnas.0704144104
Temel, Y., Tan, S., Visser-Vandewalle, V., and Sharp, T. (2009). Parkinson’s disease, DBS and suicide: A role for serotonin? Brain 132:e126. doi: 10.1093/brain/awp150
Temel, Y., Visser-Vandewalle, V., Kaplan, S., Kozan, R., Daemen, M. A., Blokland, A., et al. (2006). Protection of nigral cell death by bilateral subthalamic nucleus stimulation. Brain Res. 1120, 100–105. doi: 10.1016/j.brainres.2006.08.082
Thobois, S., Ardouin, C., Lhommée, E., Klinger, H., Lagrange, C., Xie, J., et al. (2010). Non-motor dopamine withdrawal syndrome after surgery for Parkinson’s disease: Predictors and underlying mesolimbic denervation. Brain 133, 1111–1127. doi: 10.1093/brain/awq032
Tubbs, R. S., Loukas, M., Shoja, M. M., Mortazavi, M. M., and Cohen-Gadol, A. A. (2011). Félix Vicq d’Azyr (1746–1794): Early founder of neuroanatomy and royal French physician. Childs Nervous Syst. 27, 1031–1034. doi: 10.1007/s00381-011-1424-y
Tucker, H. R., Mahoney, E., Chhetri, A., Unger, K., Mamone, G., Kim, G., et al. (2019). Deep brain stimulation of the ventroanterior and ventrolateral thalamus improves motor function in a rat model of Parkinson’s disease. Exp. Neurol. 317, 155–167. doi: 10.1016/j.expneurol.2019.03.008
Tuite, P. J., Maxwell, R. E., Ikramuddin, S., Kotzd, C. M., Billingtond, C. J., Laseski, M. A., et al. (2005). Weight and body mass index in Parkinson’s disease patients after deep brain stimulation surgery. Parkinsonism Related Disord. 11, 247–252. doi: 10.1016/j.parkreldis.2005.01.006
Turner, H., Chueh, D., Ortiz, T., Stokes, A. J., and Small-Howard, A. L. (2017). Cannabinoid therapeutics in Parkinson’s disease: Promise and paradox. J. Herbs Spices Med. Plants 23, 231–248. doi: 10.1080/10496475.2017.1312724
Tuura, R. L. G., Baumann, C. R., and Baumann-Vogel, H. (2018). Neurotransmitter activity is linked to outcome following subthalamic deep brain stimulation in Parkinson’s disease. Parkinsonism Related Disord. 50, 54–60. doi: 10.1016/j.parkreldis.2018.02.014
Uematsu, A., Tan, B. Z., and Johansen, J. P. (2015). Projection specificity in heterogeneous locus coeruleus cell populations: Implications for learning and memory. Learn. Mem. 22, 444–451. doi: 10.1101/lm.037283.114
Vaccarino, F. J., and Franklin, K. (1982). Dopamine mediates ipsi-and contraversive circling elicited from the substantia nigra. Pharmacol. Biochem. Behav. 17, 431–434. doi: 10.1016/0091-3057(82)90300-8
Vachez, Y. M., and Creed, M. C. (2020). Deep Brain Stimulation of the Subthalamic Nucleus Modulates Reward-Related Behavior: A Systematic Review. Front. Hum. Neurosci. 14:578564. doi: 10.3389/fnhum.2020.578564
Vedam-Mai, V., Van Battum, E., Kamphuis, W., Feenstra, M., Denys, D., Reynolds, B., et al. (2012). Deep brain stimulation and the role of astrocytes. Mol. Psychiatry 17, 124–131. doi: 10.1038/mp.2011.61
Vitek, J. L., Hashimoto, T., Peoples, J., DeLong, M. R., and Bakay, R. A. (2004). Acute stimulation in the external segment of the globus pallidus improves parkinsonian motor signs. Mov. Disord. 19, 907–915. doi: 10.1002/mds.20137
Voon, V., Krack, P., Lang, A. E., Lozano, A. M., Dujardin, K., Schüpbach, M., et al. (2008). A multicentre study on suicide outcomes following subthalamic stimulation for Parkinson’s disease. Brain 131, 2720–2728. doi: 10.1093/brain/awn214
Walker, R. H., Koch, R. J., Moore, C., and Meshul, C. K. (2009). Subthalamic nucleus stimulation and lesioning have distinct state-dependent effects upon striatal dopamine metabolism. Synapse 63, 136–146. doi: 10.1002/syn.20592
Wallace, B. A., Ashkan, K., Heise, C. E., Foote, K. D., Torres, N., Mitrofanis, J., et al. (2007). Survival of midbrain dopaminergic cells after lesion or deep brain stimulation of the subthalamic nucleus in MPTP-treated monkeys. Brain 130, 2129–2145. doi: 10.1093/brain/awm137
Wang, X., Lou, N., Xu, Q., Tian, G.-F., Peng, W. G., Han, X., et al. (2006). Astrocytic Ca2+ signaling evoked by sensory stimulation in vivo. Nat. Neurosci. 9, 816–823. doi: 10.1038/nn1703
Wang, Y. Y., Wang, Y., Jiang, H. F., Liu, J. H., Jia, J., Wang, K., et al. (2018). Impaired glutamatergic projection from the motor cortex to the subthalamic nucleus in 6-hydroxydopamine-lesioned hemi-parkinsonian rats. Exp. Neurol. 300, 135–148. doi: 10.1016/j.expneurol.2017.11.006
Wen, P., Li, M., Xiao, H., Ding, R., Chen, H., Chang, J., et al. (2015). Low-frequency stimulation of the pedunculopontine nucleus affects gait and the neurotransmitter level in the ventrolateral thalamic nucleus in 6-OHDA Parkinsonian rats. Neurosci. Lett. 600, 62–68. doi: 10.1016/j.neulet.2015.06.006
Wichmann, T., and DeLong, M. R. (2006). Deep brain stimulation for neurologic and neuropsychiatric disorders. Neuron 52, 197–204. doi: 10.1016/j.neuron.2006.09.022
Wilkins, K. B., Parker, J. E., and Bronte-Stewart, H. M. (2020). Gait variability is linked to the atrophy of the Nucleus Basalis of Meynert and is resistant to STN DBS in Parkinson’s disease. Neurobiol. Dis. 146:105134. doi: 10.1016/j.nbd.2020.105134
Williams, N. R., Foote, K. D., and Okun, M. S. (2014). Subthalamic Nucleus Versus Globus Pallidus I nternus Deep Brain Stimulation: Translating the Rematch Into Clinical Practice. Mov. Disord. Clin. Pract. 1, 24–35. doi: 10.1002/mdc3.12004
Windels, F., Bruet, N., Poupard, A., Feuerstein, C., Bertrand, A., and Savasta, M. (2003). Influence of the frequency parameter on extracellular glutamate and γ−aminobutyric acid in substantia nigra and globus pallidus during electrical stimulation of subthalamic nucleus in rats. J. Neurosci. Res. 72, 259–267. doi: 10.1002/jnr.10577
Windels, F., Bruet, N., Poupard, A., Urbain, N., Chouvet, G., Feuerstein, C., et al. (2000). Effects of high frequency stimulation of subthalamic nucleus on extracellular glutamate and GABA in substantia nigra and globus pallidus in the normal rat. Eur. J. Neurosci. 12, 4141–4146. doi: 10.1046/j.1460-9568.2000.00296.x
Wu, S. T., Yu, M., Zhang, K., and Zhang, J. G. (2012). Effect of deep brain stimulation on substantia nigra neurons in a rat model of Parkinson’s disease. Chin. Med. J. 125, 4072–4075.
Xiao, G., Song, Y., Zhang, Y., Xing, Y., Zhao, H., Xie, J., et al. (2019). Microelectrode arrays modified with nanocomposites for monitoring dopamine and spike firings under deep brain stimulation in rat models of parkinson’s disease. ACS Sens. 4, 1992–2000. doi: 10.1021/acssensors.9b00182
Yamamoto, T., Uchiyama, T., Higuchi, Y., Asahina, M., Hirano, S., Yamanaka, Y., et al. (2015). Subthalamic nucleus deep brain stimulation modulate catecholamine levels with significant relations to clinical outcome after surgery in patients with Parkinson’s disease. PLoS One 10:e0138462. doi: 10.1371/journal.pone.0138462
Yamamoto, T., Uchiyama, T., Sakakibara, R., Taniguchi, J., and Kuwabara, S. (2014). The subthalamic activity and striatal monoamine are modulated by subthalamic stimulation. Neuroscience 259, 43–52. doi: 10.1016/j.neuroscience.2013.11.034
Yelnik, J. (2002). Functional anatomy of the basal ganglia. Mov. Disord. 17, S15–S21. doi: 10.1002/mds.10138
Zarow, C., Lyness, S. A., Mortimer, J. A., and Chui, H. C. (2003). Neuronal loss is greater in the locus coeruleus than nucleus basalis and substantia nigra in Alzheimer and Parkinson diseases. Arch. Neurol. 60, 337–341. doi: 10.1001/archneur.60.3.337
Zhang, W., Xiong, B. R., Zhang, L. Q., Huang, X., Yuan, X., Tian, Y. K., et al. (2021). The role of the GABAergic system in diseases of the central nervous system. Neuroscience 470, 88–99. doi: 10.1016/j.neuroscience.2021.06.037
Zhao, X. D., Cao, Y. Q., Liu, H. H., Li, F. Q., You, B. M., and Zhou, X. P. (2009). Long term high frequency stimulation of STN increases dopamine in the corpus striatum of hemiparkinsonian rhesus monkey. Brain Res. 1286, 230–238. doi: 10.1016/j.brainres.2009.06.069
Keywords: deep brain stimulation, DBS, neurotransmitters, Parkinson’s disease, PD
Citation: Alosaimi F, Boonstra JT, Tan S, Temel Y and Jahanshahi A (2022) The role of neurotransmitter systems in mediating deep brain stimulation effects in Parkinson’s disease. Front. Neurosci. 16:998932. doi: 10.3389/fnins.2022.998932
Received: 20 July 2022; Accepted: 14 September 2022;
Published: 05 October 2022.
Edited by:
Wael M. Y. Mohamed, International Islamic University Malaysia, MalaysiaReviewed by:
Dumitru Ciolac, Nicolae Testemiñanu State University of Medicine and Pharmacy, MoldovaManoj P. Dandekar, National Institute of Pharmaceutical Education and Research, India
Gertrud Tamas, Semmelweis University, Hungary
Copyright © 2022 Alosaimi, Boonstra, Tan, Temel and Jahanshahi. This is an open-access article distributed under the terms of the Creative Commons Attribution License (CC BY). The use, distribution or reproduction in other forums is permitted, provided the original author(s) and the copyright owner(s) are credited and that the original publication in this journal is cited, in accordance with accepted academic practice. No use, distribution or reproduction is permitted which does not comply with these terms.
*Correspondence: Faisal Alosaimi, Zi5hbG9zYWltaUBtYWFzdHJpY2h0dW5pdmVyc2l0eS5ubA==; Ali Jahanshahi, YS5qYWhhbnNoYWhpQG1hYXN0cmljaHR1bml2ZXJzaXR5Lm5s