- Department of Applied Biology, Kyoto Institute of Technology, Kyoto, Japan
The circumventricular organs (CVOs) are located around the brain ventricles, lack a blood-brain barrier (BBB) and sense blood-derived molecules. This review discusses recent advances in the importance of CVO functions, especially glial cells transferring periphery inflammation signals to the brain. The CVOs show size-limited vascular permeability, allowing the passage of molecules with molecular weight <10,000. This indicates that the lack of an endothelial cell barrier does not mean the free movement of blood-derived molecules into the CVO parenchyma. Astrocytes and tanycytes constitute a dense barrier at the distal CVO subdivision, preventing the free diffusion of blood-derived molecules into neighboring brain regions. Tanycytes in the CVOs mediate communication between cerebrospinal fluid and brain parenchyma via transcytosis. Microglia and macrophages of the CVOs are essential for transmitting peripheral information to other brain regions via toll-like receptor 2 (TLR2). Inhibition of TLR2 signaling or depletion of microglia and macrophages in the brain eliminates TLR2-dependent inflammatory responses. In contrast to TLR2, astrocytes and tanycytes in the CVOs of the brain are crucial for initiating lipopolysaccharide (LPS)-induced inflammatory responses via TLR4. Depletion of microglia and macrophages augments LPS-induced fever and chronic sickness responses. Microglia and macrophages in the CVOs are continuously activated, even under normal physiological conditions, as they exhibit activated morphology and express the M1/M2 marker proteins. Moreover, the microglial proliferation occurs in various regions, such as the hypothalamus, medulla oblongata, and telencephalon, with a marked increase in the CVOs, due to low-dose LPS administration, and after high-dose LPS administration, proliferation is seen in most brain regions, except for the cerebral cortex and hippocampus. A transient increase in the microglial population is beneficial during LPS-induced inflammation for attenuating sickness response. Transient receptor potential receptor vanilloid 1 expressed in astrocytes and tanycytes of the CVOs is responsible for thermoregulation upon exposure to a warm environment less than 37°C. Alternatively, Nax expressed in astrocytes and tanycytes of the CVOs is crucial for maintaining body fluid homeostasis. Thus, recent findings indicate that glial cells in the brain CVOs are essential for initiating neuroinflammatory responses and maintaining body fluid and thermal homeostasis.
General introduction
The blood-brain barrier (BBB) was described more than 100 years ago with the observation that intravenous dye injection reached all organs but the brain (Ehrlich, 1885). The BBB is composed of a highly specialized brain endothelial cellular sheet that separates it from circulating blood and maintains brain homeostasis by tight and adherens junctions (Rubbin and Staddon, 1999; Tsukita et al., 2001; Hawkins and Davis, 2005; Zlokovic, 2011). The BBB does not preclude blood-to-brain communication as, via transporters and transcytosis, it permits the exchange of molecules such as peptides, proteins, vitamins, hormones, amino acids, nucleotides, fatty acids, and other compounds (Miller and Leslie, 1994; Barar et al., 2016; Yang et al., 2020).
The circumventricular organs (CVOs) are located around the brain ventricles and lack the BBB (Hofer, 1987; Johnson and Gross, 1993; Ganong, 2000; Fry et al., 2007; Sisó et al., 2010a,b; Mimee et al., 2013; Miyata, 2015, 2017), thereby allowing blood-derived molecules to be sensed, and the release of brain-synthesized neuropeptides into circulation (Miyata and Morita, 2011; Morita and Miyata, 2012; Morita et al., 2013, 2016; Miyata, 2015, 2017). Therefore, the CVOs are referred to as the “windows of the brain” (Gross and Weindl, 1987). The CVOs comprise the organum vasculosum of the lamina terminalis (OVLT), subfornical organ (SFO), median eminence (ME), area postrema (AP), neurohypophysis (NH), pineal gland, and choroid plexus shown in Figure 1 (Takagi et al., 2017). The CVOs are divided into two groups based on their primary role, sensory and secretory. The sensory CVOs include the OVLT, SFO, and AP, and they monitor ions, osmolality, pH, lipophobic amino acids, and neuropeptides in blood circulation via a wide variety of receptors (Sisó et al., 2010a,b; Ferguson, 2014). In addition, they integrate and transmit blood-derived information to neighboring brain regions to control inflammation and body fluid and thermal homeostasis (Roth et al., 2004; Sisó et al., 2010a,b; Miyata, 2015). The secretory CVOs, consisting of the ME, NH, and pineal glands release various neuropeptides or melatonin (Miyata and Hatton, 2002; Miyata, 2017). The innermost layer of the meninges forms vascularized invaginations, called choroid plexus, in some parts of the ventricles to produce the CSF. Our previous review describes detailed functions of the secretory CVOs (Miyata, 2017).
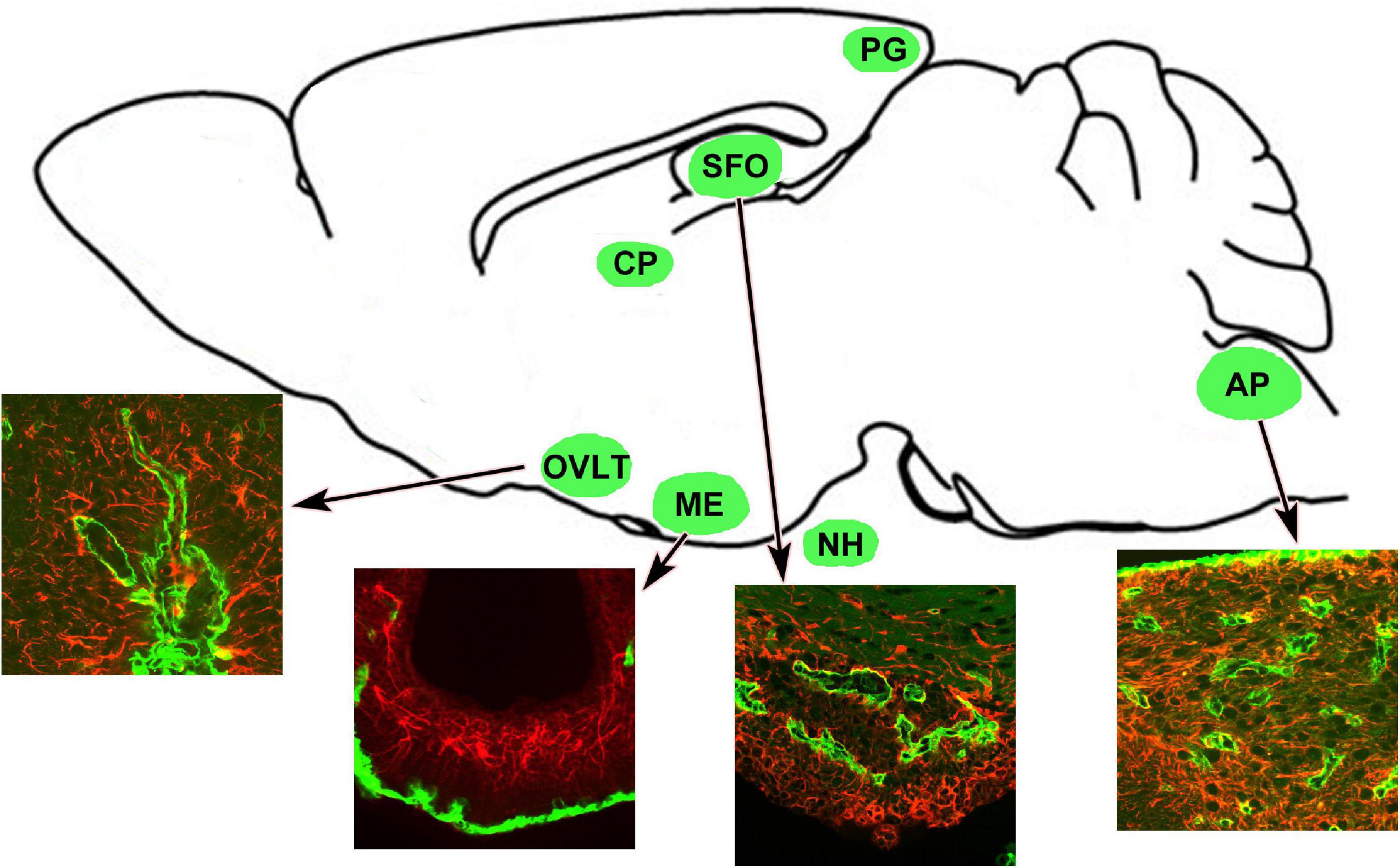
Figure 1. Schematic representation revealing CVOs locations in the adult rodent brain. Insets indicate that the CVOs are characterized by dense and thick laminin+ (green) capillaries and dense GFAP+ (red) astrocytic and tanycytic networks. Scale bar = 50 μm. AP, area postrema; CP, choroid plexus; GFAP, glial fibrillar acidic protein; ME, median eminence; NH, neurohypophysis; OVLT, organum vasculosum of the lamina terminalis; PG, pineal gland; SFO, subfornical organ. The illustration is rearranged with permission from Elsevier B.V. (Takagi et al., 2017).
The lack of the typical endothelial BBB does not mean that the fenestrate capillary in the CVOs allows free entry of blood-derived molecules to the parenchyma since only low blood-derived low-molecular-weight (LMW) molecules can pass through the fenestrate capillary (Morita and Miyata, 2012; Miyata, 2015; Morita et al., 2016). Moreover, blood-derived LMW molecules cannot diffuse into neighboring brain regions by dense walls constituted by astrocytes and tanycytes at distal CVO subdivisions. Tanycytes are specialized ependymal or glial cells found in the ventricular system and CVOs of the brain and possess long cellular processes extending deep into the brain parenchyma (Rodríguez et al., 2005, 2019). Tanycytes are classified as reminiscent radial glia owing to their highly polarized morphology and neural stem cell niche (Rodríguez et al., 2005; Furube et al., 2020).
The CVOs are essential brain regions for neuroinflammatory response initiation, for circulating pathogen-associated molecular patterns (PAMPs) and cytokines to induce faster transcriptional activation of genes encoding for pro-inflammatory molecules (Quan et al., 1997; Rummel et al., 2005; Yoshida et al., 2016). The CVOs are thought to function in pathological conditions such as sepsis, stress, the neuronal invasion of parasites, autoimmune encephalitis, and systemic amyloidosis (Sisó et al., 2010a,b; Kristensson et al., 2013), as they are involved in most autonomic and endocrine regulatory pathways and lack the BBB. The CVOs are also essential to the brain region for sensing thermal and osmotic signals and integrating their information to initiate proper physiological homeostatic responses (Sladek and Johnson, 2013; Noda and Hiyama, 2015; Park et al., 2020; Ilanges et al., 2022).
This review introduces advances in understanding the involvement of CVO glial cells in brain-to-periphery communication at the blood-brain interface. In this review, the following aspects of the CVOs are discussed: (1) Fundamental properties of fenestrated capillaries with size-limited permeability, glial barriers, and tanycytic transcytosis; (2) Significance of pattern recognition receptors such as toll-like receptors 2 (TLR2), TLR4, and glial cell inflammatory signal transduction from the periphery to the brain, and (3) Maintenance of body fluid and thermal homeostasis by glial Nax channel and transient receptor potential vanilloid 1 (TRPV1), respectively.
Size-limited vascular permeability at fenestrated capillaries
The BBB is important for normal brain physiology and protects neuronal tissues by limiting the ingress of blood-derived bioactive and toxic hydrophilic molecules. Disturbance of the BBB leads to the accumulation of these molecules within the parenchyma causing severe brain damage (Zlokovic, 2011; Obermeier et al., 2013). Mice deficient in tight junction proteins such as claudin-5 or ZO-1 die due to blood-derived toxic molecules accessing the brain (Morita et al., 1999; Furuse et al., 2001, 2002; Katsuno et al., 2008). However, CVO vasculature lacks the typical BBB, and possesses fenestrated features unlike those in other brain regions (Miyata and Morita, 2011; Morita and Miyata, 2012; Morita et al., 2013, 2016; Miyata, 2015, 2017). For example, levels of glutamate, an excitatory transmitter, in plasma and brain is in the range of 50–100 and 0.5–2 μmol/l, respectively (Hawkins, 2009). Even after oral ingestion of large quantities of glutamate, only small changes in glutamate plasma concentration occur (Stegink et al., 1982). The subcutaneous administration of high-dose monosodium glutamate increases fivefold increase of glutamate levels in the SFO and AP (Price et al., 1981). The administration of monosodium glutamate also induces neuronal loss in the ME and arcuate nucleus (Arc) of adult (Yulyaningsih et al., 2017; Heiss et al., 2022) and the CVOs of neonatal rodents (Lemkey-Johnston and Reynolds, 1974). Many studies demonstrate the free diffusion of LMW molecules into the CVOs parenchyma: fluorescein (MM = 332), fluorescein isothiocyanate (FITC; MM = 390), Evans blue (MM = 961), and Dextran 3,000 (MW = 3,000) (Willis et al., 2007; Morita and Miyata, 2012; Morita et al., 2013, 2016; Nishikawa et al., 2017). The molecular weight of these tracer molecules is within the range of bioactive neuropeptides, amino acids, or amines; e.g., adenohypophyseal hormone-releasing hormones such as thyrotropin-releasing hormone (minimum size at MW = 362.4) to growth-hormone-releasing hormone (maximum size at MW = 5040.4). In contrast to LMW molecules, blood-derived high-molecular-weight (HMW) molecules, more than MW 10,000, do not freely enter the CVOs parenchyma (Willis et al., 2007; Morita and Miyata, 2012; Morita et al., 2016). LMW soluble molecules (<3 nm molecular radius) are presumed to move passively through endothelial intercellular space in BBB-lacking capillaries (Mehta and Malik, 2006; Komarova and Malik, 2010). Thus, the CVOs endothelial intercellular space exhibits a size-limited vascular permeability lower than approximately MW 10,000 (Miyata, 2015, 2017). However, it is often misunderstood that the fenestrated capillaries of the CVOs allow molecules of any size to enter the parenchyma.
High-molecular-weight molecules cannot pass freely through fenestrate capillaries in the CVOs. HMW molecules such as hormones and cytokines reach the brain parenchyma beyond the BBB via transcytosis (Gonçalves and De Felice, 2021). Recently, more than a thousand proteins, including various cytokines and growth factors, readily permeated brain parenchyma with the transcytosis system by BBB-specific transcriptional programs (Yang et al., 2020). Autoradiographic and fluorescent microscopic techniques show heterogenous transcytosis of blood proteins within the brain, especially prominent at the basolateral hypothalamus, including the ME, Arc, and ventromedial hypothalamic nucleus (Yang et al., 2020). Blood-derived leptin activates leptin receptors in tanycytes of the ME and is transported along the tanycytic cellular process and secreted into the CSF (Balland et al., 2014). Blood-derived horseradish peroxidase extensively accumulates between the inner and outer BM in the NH and ME, but it also reaches the brain parenchyma, possibly via mannose receptor-mediated transcellular routes (Ellinger and Fuchs, 2010; Rodríguez et al., 2010).
Astrocytic and tanycytic barrier instead of endothelial blood-brain barrier
The basement membrane of fenestrated capillaries is permeable to blood-derived LMW molecules, which easily reach the CVOs parenchyma; however, they do not diffuse out of the CVO, as shown in Figures 2A–C (Morita et al., 2016). The capillaries of central subdivisions in the sensory CVOs possess a higher vascular permeability than those of distal subdivisions due to the lack of tight-junction protein expression in the endothelial cells (Miyata, 2015; Morita et al., 2016). Astrocytes in the distal regions of the sensory CVOs become hypertrophic and their density is remarkably high (Morita et al., 2016). Tanycytes in the OVLT and SFO, but not in the AP, extend cellular processes into the surrounding neuronal somata and fenestrated capillaries, connecting tightly (Noda and Hiyama, 2015; Prager-Khoutorsky and Bourque, 2015; Morita et al., 2016). Astrocytes and tanycytes often express several kinds of tight junction proteins (Figures 2D,E; Morita et al., 2016), and electron microscopy reveals junctions between intimately apposed astrocytic or tanycytic cellular membranes (Figure 2F; Morita et al., 2016). Astrocytes are reported to express ZO-1 and occludin in the periphery of infarct areas during the stroke recovery process to prevent ingress of neurotoxic molecules (Yang et al., 2007, 2013). Thus, the astrocytes and tanycytes, densely located at distal CVO subdivisions, are an alternative to the endothelial BBB and prevent blood-derived LMW molecules from diffusing into neighboring brain regions.
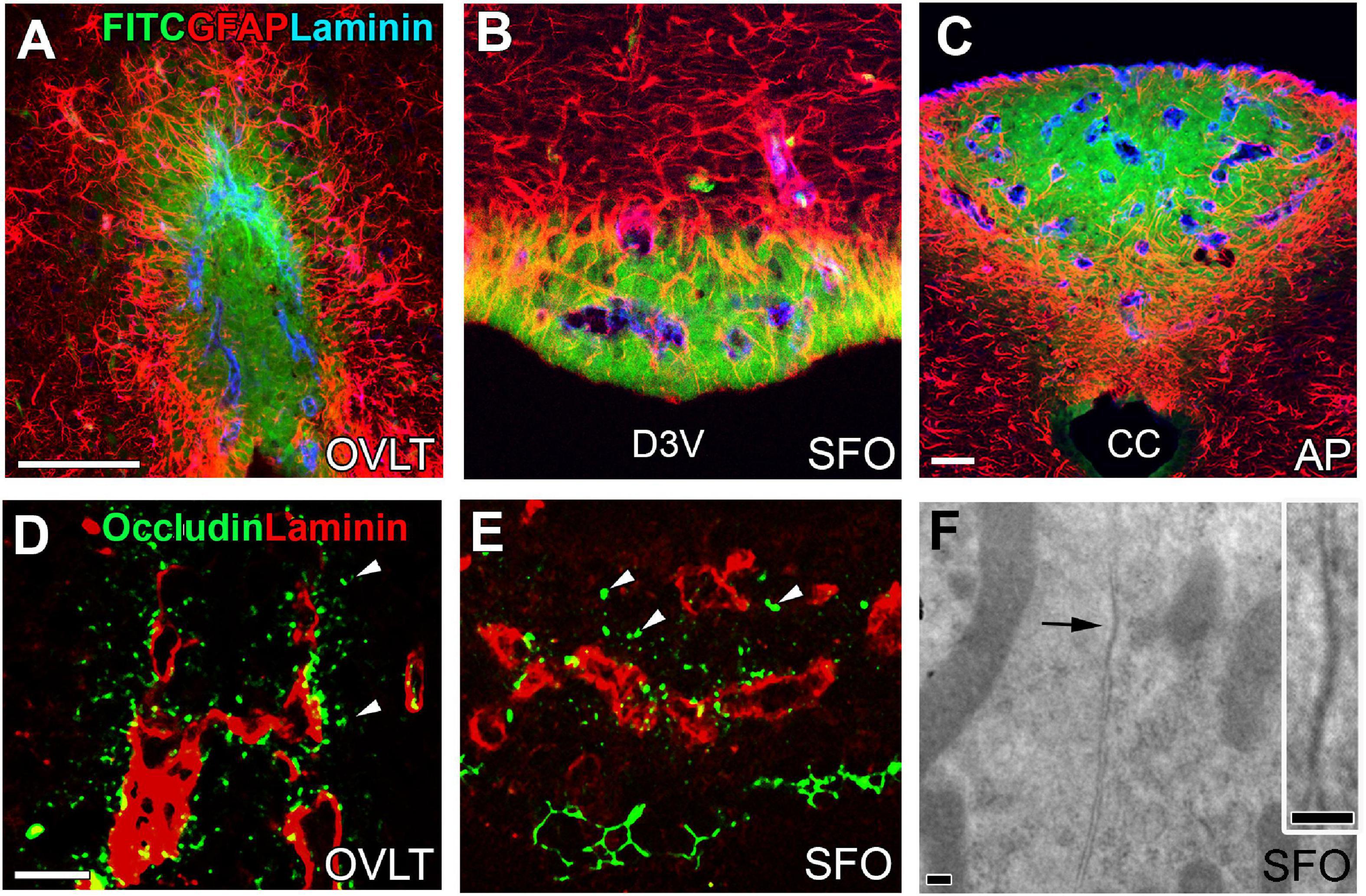
Figure 2. Vascular permeability and tight junctions in the CVOs of the adult mouse brain. Strong fluorescence of blood-derived fluorescein isothiocyanate is seen at the central subdivision of the sensory CVOs, but fluorescence is weak at distal subdivisions and no fluorescence is seen in the neighboring brain region beyond dense astrocytic and tanycytic barriers (A–C). Tight junction protein occludin is seen at parenchyma close to fenestrated capillaries and tanycytic layers (D,E). An electron microscopic image reveals the tight junction-like structure between the plasma membrane of neighboring cells making complete contact (F). Scale bar = 50 μm (A,C,D) and 100 nm (F). AP, area postrema; CC, central canal; D3V, dorsal 3rd ventricle; FITC, fluorescein isothiocyanate; GFAP, glial fibrillar acidic protein; OVLT, organum vasculosum of the lamina terminalis; SFO, subfornical organ. Photographs are rearranged with permission from Springer Nature (Morita et al., 2016).
Inability of blood-derived high-molecular-weight molecules to cross fenestrated capillaries into the parenchyma
In contrast to the high vascular permeability of blood-derived LMW molecules in the CVOs fenestrated capillaries, HMW molecules, MW > 10,000, can cross the outer basement membrane but do not diffuse into parenchyma past the inner basement membrane and accumulate between these membranes (Morita and Miyata, 2012; Morita et al., 2016; Nishikawa et al., 2017). Continuous capillaries in the typical BBB-containing brain vasculature possess a narrow perivascular space surrounded by inner and outer basement membrane generally fused, whereas fenestrate capillaries in the CVO have a large and complex perivascular space (Dellmann, 1998; Morita et al., 2016). Increased collagen type I expression is detected on the inner basement membrane compared with the outer basement membrane, whereas laminin expression is increased in the outer basement membrane compared with the inner (Morita et al., 2016; Nishikawa et al., 2017). These features are also seen in the kidney glomerulus, the glomerular basement membrane contributing to the size-limited kidney filtration barrier that separates proteins and LMW molecules such as glucose and urea (Chen and Miner, 2012; Miner, 2012). Mutations in either laminin or collagen type IV result in proteinuria, often leading to nephrotic syndrome and renal failure (Chen and Miner, 2012). Thus, like typical BBB-containing capillaries, fenestrated capillaries of the CVOs prevent parenchyma access to HMW molecules beyond the inner basement membrane. Blood-derived albumin in the brain parenchyma causes neurodegeneration (Mallmann et al., 2022), demonstrating the neurotoxicity of blood-derived HMW molecules. However, this evidence does not proscribe all HMW molecule access to the parenchyma, as recent studies found that active transport of blood-derived HMW molecules into the brain parenchyma occurs by ligand-specific receptor-mediated and non-specific caveolar transcytosis (Ellinger and Fuchs, 2010; Yang et al., 2020; Gonçalves and De Felice, 2021).
Tanycytic transcytosis mediated cerebrospinal fluid-brain communication in the circumventricular organs
Tanycytes are specialized ependymal cells interfacing with the CSF and have a long basal process that terminates at the capillary (Horstmann, 1954; Rodríguez et al., 2005). Ependymal cells in the Arc and ME are the best-known tanycytes and have a single, long cellular process projecting to discrete hypothalamic parenchyma and fenestrated capillaries (Rodríguez et al., 1979, 2005). Tanycytes also exist at the ventricular borders of the OVLT and SFO (Mullier et al., 2010; Langlet et al., 2013). A honeycomb distribution pattern of tight junction proteins is observed at tanycytes around the CVO proximal cell bodies, possibly to prevent the diffusion of blood-derived molecules into the CSF (Mullier et al., 2010; Langlet et al., 2013). Hypothalamic tanycytes also reduce the release into circulation of thyrotropin-releasing hormone by activating the tanycytic receptor for thyrotropin-releasing hormone (Müller-Fielitz et al., 2017). However, circulating leptin is recognized by leptin receptors on tanycytes in the ME, then extracellular signal-regulated kinase signaling of tanycytes promotes leptin transport, activating neurons in the mediobasal hypothalamus (Balland et al., 2014). Tanycytes participate in the release of gonadotropin-releasing hormone by the spatial relationship between the tanycytes and gonadotropin-releasing hormone-secreting terminals (Prevot et al., 2010; Bolborea and Dale, 2013). Hypothalamic tanycytes also reduce the release of thyrotropin-releasing hormone into the circulation by activating the tanycytic receptor for thyrotropin-releasing hormone (Müller-Fielitz et al., 2017). Active transcytosis occurs at tanycytes in the CVOs of the adult mouse brain, as in the Arc (Okamoto et al., 2022). The CVOs can, by tanycytic transcytosis, transport bioactive molecules between the CSF and CVO, suggesting that the long cellular processes of tanycytes act as communication routes between the CSF and brain parenchyma.
Circumventricular organ tanycytes are neural stem cells
Tanycytes in the ME and Arc are shown to be a neurogenic niche in adult mammals (Lee et al., 2012; Robins et al., 2013). Tanycytes in the spinal cord are NSCs that, remarkably, proliferate and differentiate into astrocytes and oligodendrocytes upon spinal injury (Meletis et al., 2008). Recently we have found two types of NSCs in the CVOs; astrocyte- and tanycyte-like NSCs (Hourai and Miyata, 2013; Furube et al., 2015, 2020; Nambu et al., 2021a,b). Radial glial cells in the ventricle zone are NSCs that, during cortical development, produce new neurons and glial cells (Ohtaka-Maruyama and Okado, 2015). Tanycytes in the adult mammalian brain are NSC precursors, ultimately derived from radial glia during development (Goodman and Hajihosseini, 2015). The CSF provides important functions to support brain homeostasis, morphogenesis, and proliferation during development (Johansson, 2014). In adults, the CSF actively synthesizes and secretes factors that promote the proliferation of quiescent and transit-amplifying NSCs in the subventricular zone (Silva-Vargas et al., 2016). Aging speed is controlled primarily by hypothalamic NSCs via levels of exosomal miRNAs in the CSF (Zhang et al., 2017). Moreover, continuous angiogenesis occurs in the CVOs of adult mice, possibly reconstructing neurons, glia, and fenestrated capillaries (Morita et al., 2013, 2015; Furube et al., 2014; Asami et al., 2020). Thus, tanycytes, throughout the adult mammalian brain, are accepted as NSCs, with their proliferation and differentiation potentially regulated by factors from the CSF and fenestrated capillaries, depending on body conditions.
The circumventricular organs are a possible communication route between the periphery and brain
Peripheral immune response transmits information to the brain to induce physiological and behavioral responses, but communication between the peripheral immune signal molecules or PAMPs and brain parenchyma is not well understood. Hypotheses proposed as to how peripheral inflammatory signals and PAMPs in circulation are transferred to the brain parenchyma to stimulate associated neural circuits include; 1) cytokine-dependent prostaglandin E2 (PGE2) synthesis and release at endothelial cells of brain vasculature, 2) activation of parenchymal cells in the CVOs by blood-derived molecules across fenestrated capillaries, 3) activation of peripheral nerves by cytokines and PGE2 (Blomqvist and Engblom, 2018). However, it is difficult to conclude that a single route transmits peripheral inflammatory information to the brain, as neuroinflammation is associated with various physiological changes such as body temperature, locomotor activity, food and water intake, and metabolism (Tesoriero et al., 2018). Therefore, several routes may work in conjunction to regulate complex physiological inflammation responses.
This section focuses on glial cells in CVOs with a specialized niche for the blood-brain interface to transport information from the periphery to the brain. The CVOs express a range of inflammation-associated receptors for cytokines, PAMPs, neuropeptides, and PGE2 (McKinley et al., 2003; Roth et al., 2004; Sisó et al., 2010a,b; Miyata, 2015). In comparison to other brain regions, peripheral inflammatory stimulation in the CVOs causes faster and stronger transcriptional activation of a vast number of proinflammatory molecules; such as nuclear factor-κ B (NF-κB; Quan et al., 1997; Laflamme et al., 1999; Chakravarty and Herkenham, 2005; Muneoka et al., 2019; Murayama et al., 2019), the signal transducer and activator of transcription factor 3 (STAT3; Rummel et al., 2004, 2005; Nakano et al., 2015; Yoshida et al., 2016), and Fos (Konsman and Dantzer, 1999; Muneoka et al., 2019; Murayama et al., 2019; Takagi et al., 2020). Microsomal prostaglandin E synthase-1, an inducible catalytic enzyme for converting PGH2 to PGE2, is highly expressed in autonomic relay structures such as the CVOs, preoptic area (POA), Arc, and paraventricular nucleus (Eskilsson et al., 2014). Importantly, microsomal prostaglandin E synthase-1 expression is observed in endothelial cells, pericytes, and neurons of the CVOs. Sickness responses induced by interleukin-1β (IL-1β) are not derived from cerebral parenchymal vasculature but depend on a relatively small number of fenestrated capillaries located in the CVOs (Knoll et al., 2017). Strong expression of receptors against cytokines such as IL-6, IL-1β, and tumor necrosis factor-α (TNF-α) is also demonstrated in the CVOs (Ericsson et al., 1995; Vallieres and Rivest, 1997; Nadeau and Rivest, 2000; Roth et al., 2009). IL-6-deficient mice do not exhibit fever generation in response to peripheral administration of lipopolysaccharide (LPS) in contrast to IL-1β and TNF-α-deficient animals (Chai et al., 1996). Also, remarkably high expression of TLR2 and TLR4 mRNA occurs in the CVOs (Laflamme et al., 2001; Laflamme and Rivest, 2001).
The AP forms the dorsal vagal complex with the solitary nucleus (Sol) and plays a role in the emetic reflex, immune responses, cardiovascular regulation, and energy homeostasis (Rogers et al., 1996; Price et al., 2008). The Arc and AP are the most critical brain regions for circulating factors involved in regulating food intake and energy homeostasis (McKinley et al., 2003; Price et al., 2008; Riediger, 2012). The AP is known to be implicated in nausea and vomiting to at least visceral stimulation (Miller and Leslie, 1994). Blood levels of growth/differentiation factor 15 are increased in sickness-associated events such as bacterial and viral infection, LPS, lithium chloride, and pregnancy (Tsai et al., 2018). Growth/differentiation factor 15 receptor-expressing excitatory neurons in the AP mediate aversion responses to visceral LPS and lithium chloride (Zhang C. et al., 2020). TNF-α stimulates neurons in the AP to elicit sympathetic excitation and blood pressure increases (Korim et al., 2019). Thus, the AP is chiefly concerned with inflammation-induced sickness responses, such as decreased food intake, nausea, and hypertension.
The OVLT is located close to the POA that regulates body temperature, and sizeable electrolytic lesion to the anteroventral 3rd ventricle (AV3V) region, including the OVLT and SFO, attenuates fever after peripheral administration of LPS (Blatteis et al., 1983; Stitt, 1985). The quantitative autoradiography of [3H] PGE2 shows the highest binding density in the AV3V wall surrounding the OVLT (Matsumura et al., 1990). Subsequent studies indicate that fever-generation abnormalities come from side effects due to body fluid deficiency, electrolyte, and cardiovascular homeostasis (Romanovsky et al., 2003). In contrast to the OVLT, an electrical lesion to the SFO prevents LPS-induced fever (Takahashi et al., 1997). Direct injection of IL-1β into the SFO increases body temperature and salt intake (Cerqueira et al., 2016).
When the SARS-CoV-2 virus reaches the brain, the subsequent neurological damage results in dysfunctions of long-term body condition (Graham et al., 2021). SARS-CoV-2 binds to angiotensin-converting enzyme 2 (ACE2) on brain endothelial cells and induces inflammatory responses and activation of microglia and astrocytes to disrupt the integrity of the BBB (Buzhdygan et al., 2020; Ong et al., 2022). Infection of SARS-CoV-2 to brain neurons also induces the release of inflammatory molecules and ROS and free radical formation (Sindona et al., 2021). ACE2 is highly expressed in the CVOs and brain regions connecting to the CVOs, such as paraventricular nucleus and Sol (Doobay et al., 2007). The CVOs are essential brain regions to control angiotensin-dependent regulation of osmotic thirst, the release of arginine-vasopressin (AVP) and oxytocin (OXT) release, and blood pressure (Ferguson, 2014). In addition to ACE2, SARS-CoV-2 virus interacts directly with TLR4 to cause an immune response and increase cell surface expression of ACE2 (Bhattacharya et al., 2020; Aboudounya and Heads, 2021; Zhao et al., 2021). TLR4 protein is highly expressed in astrocytes and tanycytes in the CVOs of adult mice (Nakano et al., 2015; Muneoka et al., 2019). These facts suggest that the CVOs are possibly the primary route of entry of SARS-CoV-2 virus into the brain.
In conjunction with viral and bacterial infection, the CVOs are also involved with parasitic infection. African trypanosomes first infect the ME fenestrated capillaries, then move, by rotating flagella and protease secretion, into adjacent hypothalamic regions such as the Arc and suprachiasmatic nucleus (Grab et al., 2009; Huet et al., 2009; Kristensson et al., 2013; Bargul et al., 2016; Bentivoglio et al., 2018).
Toll-like receptor 2 in microglia and macrophages of the circumventricular organs
Toll-like receptors recognize PAMPs, which play crucial roles in early innate recognition and host inflammatory responses against invading disease-causing microbes (Akira et al., 2001; Gay et al., 2014). Studies show that expression of TLR2 and TLR4 is remarkably higher in the CVOs of the adult brains than in other brain regions (Nakano et al., 2015; Muneoka et al., 2019; Murayama et al., 2019). Circulating LPS has limited access to the brain parenchyma in non-CVOs region due to the BBB, but it can reach the parenchymal in the CVOs possibly via the lipoprotein transport system (Vargas-Caraveo et al., 2017). Moreover, a TLR2 ligand zymosan also reaches the parenchymal in the CVOs, although the transport system is unclear (Takagi et al., 2020). These shreds of evidence suggest peripheral endotoxins can activate parenchymal TLR2 and TLR4 in the CVOs. Moreover, TLR2 and TLR4 have crucial functions in modulating inflammatory responses of the brain via endogenous ligands such as heat-shock proteins, high mobility group box 1, hyaluronic acids, and fibronectin (Wang et al., 2013; Li et al., 2021). However, the specialized spatial niches suggest that TLR2 and TLR4 ligands, derived from pathogens in the blood, activate TLR2 and TLR4 in the CVOs. This indicates that the CVOs can directly detect PAMPs, and integrate and relay information to deep brain regions, and initiate brain neuroinflammation.
Among TLR family members, TLR2 recognizes a variety of TLR2 molecules from viruses, fungi, bacteria, and parasites by forming a heterodimer with TLR1 or TLR6 (Zähringer et al., 2008; Oliveira-Nascimento et al., 2012). TLR2 heterodimers generally initiate a MyD88-dependent intracellular signaling pathway so that NF-κB is activated and translocated to the nucleus to modulate gene transcription of cytokines (Kawai and Akira, 2010). The activation of TLR2 in immune cells by lipoproteins is a significant factor in Gram-positive sepsis (Takeuchi et al., 2000; Hashimoto et al., 2006). In the CVOs, TLR2 mRNA expression was higher than in other brain regions (Laflamme et al., 2001), and TLR2-expressing cells are identified as microglia and macrophages in the CVOs (Figures 3A–D; Murayama et al., 2019). The systemic administration of macrophage-activating lipopeptide-2 induces the activation of STAT3 in a subpopulation of parenchyma cells in the CVOs, together with fever generation (Knorr et al., 2008). Peripheral administration of the LMW TLR2 agonist Pam3CSK4 caused NF-κB activation in microglia and macrophages and Fos expression in astrocytes, tanycytes, and neurons in the CVOs (Murayama et al., 2019). Peripheral and intracerebroventricular (i.c.v.) injection of a TLR2 ligand, Pam3CSK4, leads to sickness responses, including anorexia, hypoactivity, and fever, depending on NF-κB and PGE2 (Jin et al., 2016; Murayama et al., 2019). A robust and temporal elevation of the TLR2 mRNA expression occurs in the CVOs after the systemic injection of a TLR4 agonist LPS (Laflamme and Rivest, 2001; Rivest, 2003, 2009), indicating crosstalk between TLR4 and TLR2. Pam3CSK4-induced fever is augmented by pretreatment of LPS (Figure 3E; Murayama et al., 2019). The expression of TLR2 is reported in both perivascular macrophages and parenchyma microglia of the CVOs (Murayama et al., 2019; Takagi et al., 2020). The CVO perivascular structure is largely different from that of the general brain; Firstly, a broad perivascular space is present between the inner and outer basement membranes in the CVOs, whereas brain capillaries generally fuse, thus lacking perivascular space (Miyata, 2015, 2017). Secondly, blood-derived HMW molecules accumulate in the perivascular space (Morita et al., 2016; Miyata, 2017; Nishikawa et al., 2017). Perivascular macrophages possess crucial functions such as maintaining vascular permeability, pathogen phagocytosis, antigen representation, and limiting endothelial cell production of PGE2 to avoid unnecessary inflammation (Lapenna et al., 2018). IL-1β signaling in fenestrated capillaries of the CVOs is sufficient to trigger sickness responses in mice (Knoll et al., 2017).
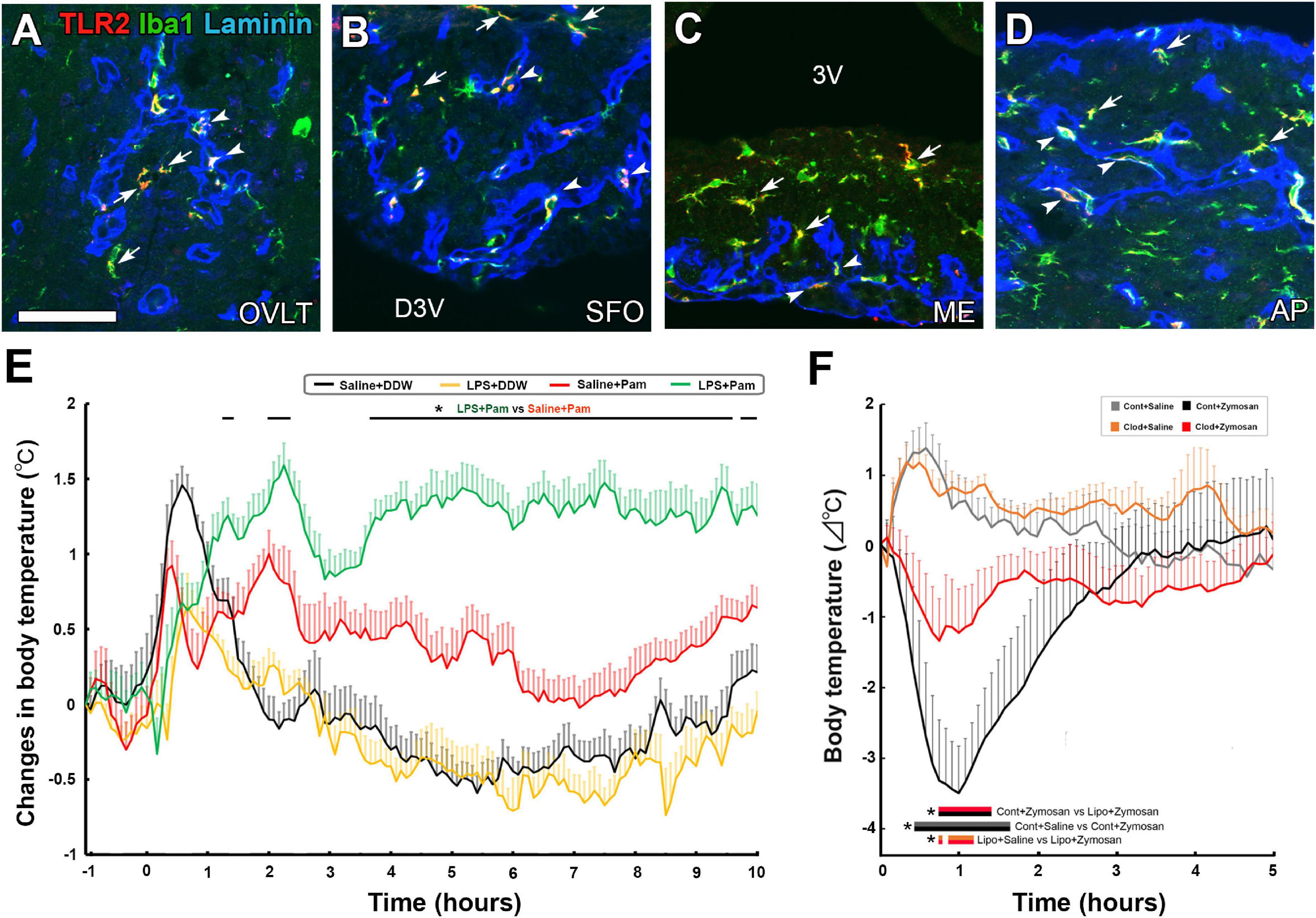
Figure 3. The expression of TLR2 at microglia and macrophages in the CVOs and TLR2 agonist-induced fever and hypothermia in the adult mouse. Triple labeled immunohistochemistry shows prominent TLR2 expression at Iba1+ microglia (arrows) in parenchyma and Iba1+ macrophages (arrowheads) in laminin+ capillary of the CVOs (A–D). LPS pretreatment promotes fever generation induced by a LMW TLR2 agonist Pam3CSK4 (E). Depletion of microglia and macrophages with clodronate liposomes attenuates hypothermia induced by the HMW TLR2 agonist zymosan (E). Scale bar = 50 μm. 3V, 3rd ventricle; AP, area postrema; D3V, dorsal 3rd ventricle; ME, median eminence; OVLT, organum vasculosum of the lamina terminalis; SFO, subfornical organ; TLR2, toll-like receptor 2. p < 0.05 by an ANOVA with Tukey’s post-hoc test. Photographs are rearranged with permission from Elsevier B.V. [(A–E) Murayama et al., 2019; (F) Takagi et al., 2020].
The depletion of microglia and macrophages with clodronate liposomes (Clod-Lips) has been shown to attenuate fever caused by the i.c.v. administration of Pam3CSK (Jin et al., 2016). I.c.v injection of Clod-Lips reduced zymosan-induced Fos expression in astrocytes, tanycytes, and neurons in the CVOs, while a Clod-Lips injection attenuated zymosan-induced hypothermia (Figure 3F; Takagi et al., 2020). Thus, microglia and macrophages are primary cells in initiating faster and stronger transcriptional activation of the CVOs, at least partially via direct activation of TLR2 and transmitting peripheral information to neurons in the CVOs to cause sickness responses in TLT2-dependent neuroinflammation.
TLR4 in astrocytes and tanycytes in the circumventricular organs
The expression of TLR4 mRNA is higher in the CVOs than in other brain regions (Laflamme and Rivest, 2001; Chakravarty and Herkenham, 2005). TLR4 protein is expressed in astrocytes and tanycytes in the CVOs of adult mice (Figure 4; Muneoka et al., 2019). The peripheral administration of LPS upregulates mRNA levels of CD14, which helps bind TLR4 to LPS-binding protein in the CVOs (Lacroix et al., 1998; Nadeau and Rivest, 2000). Peripheral administration of LPS activates STAT3 (Rummel et al., 2005; Yoshida et al., 2016) and NF-κB signaling (Laflamme et al., 1999; Muneoka et al., 2019) in astrocytes and tanycytes of the CVOs. The i.c.v. injection of LPS also causes STAT3 signaling activation in astrocytes and tanycytes of the CVOs (Nakano et al., 2015; Yoshida et al., 2016). There is no apparent difference in the activation of NF-κB and STAT3 between astrocytes and tanycytes after peripheral administration of LPS (Nakano et al., 2015; Muneoka et al., 2019). In TRM8 KO mice, (Shiraki et al., 2021; Kasuga et al., 2022) i.c.v. injection of an LPS antagonist LPS-RS attenuates NF-κB signaling in the astrocytes and tanycytes of the CVOs after peripheral administration of LPS (Muneoka et al., 2019). Thus, the cellular phenotype for faster and more robust activation of transcriptional activation is largely different between TLR2 and TLR4 in the CVOs; TLR2 in microglia and macrophages, and TLR4 in astrocytes and tanycytes. Thus, astrocytes are important in initiating neuroinflammation in the CVOs at least partially by TLR4 and transmitting peripheral information to neurons in the CVOs.
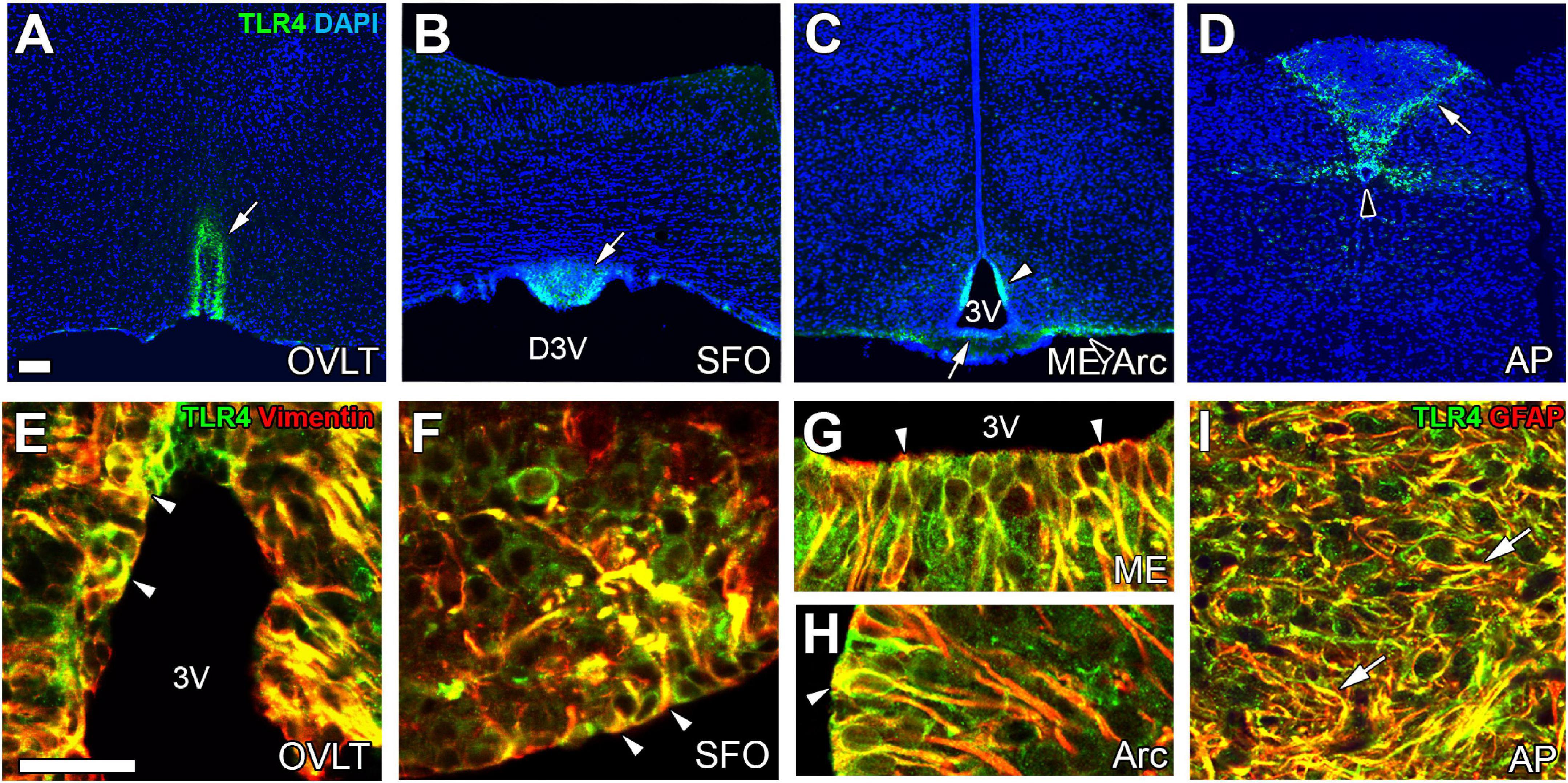
Figure 4. The expression of TLR4 at astrocytes/tanycytes of the adult mouse brain. Immunohistochemistry shows robust and specific TLR4 expression in the CVOs (open arrows), Arc (open arrowhead), and CC (solid arrowhead) (A–D). Double-labeling immunohistochemistry reveals that TLR4 is expressed at vimentin+ tanycytes (arrowheads) and GFAP+ astrocytes (arrows) (E–I). Scale bars = 50 μm. 3V, 3rd ventricle; AP, area postrema; Arc, arcuate nucleus; DAPI, 4′,6-diamidino-2-phenylindole; D3V, dorsal 3rd ventricle; GFAP, glial fibrillar acidic protein; ME, median eminence; OVLT, organum vasculosum of the lamina terminalis; SFO, subfornical organ; TLR4, toll-like receptor 4. Photographs are rearranged with permission from Elsevier B.V. (Muneoka et al., 2019).
The microglial and macrophage depletion by Clod-Lips enhanced the fever induced by peripheral administration of LPS (Serrats et al., 2010). The depletion of microglia and macrophages with the antagonist to the colony-stimulating factor-1 receptor and transgenic mouse fractalkine receptor does not attenuate LPS-induced sickness responses such as body-weight decrease, locomotor activity, and exacerbates voluntary wheel running (Vichaya et al., 2020). Moreover, it is shown that the depletion of microglia and macrophages does not prevent proinflammatory cytokine expression and exacerbates some cytokines in LPS-induced inflammation (Vichaya et al., 2020). Astrocytes have emerged as key contributors to the innate immune response of the brain to infections, neurodegenerative disorders, and injuries (Bsibsi et al., 2006; Farina et al., 2007; Li et al., 2021). These results suggest that TLR4-dependent sickness responses in acute inflammation are caused independently of microglia activation.
In contrast, we cannot deny the possibility that microglia participate in acute and chronic neuroinflammation during LPS-induced inflammation. For example, microglia inhibitor minocycline promotes recovery from sickness behaviors and reduces mRNA expression of proinflammatory cytokines upon LPS stimulation without changing plasma IL-1β levels (Henry et al., 2008). Minocycline treatment decreases STAT3 activation in astrocytes and tanycytes of the OVLT and AP, but not SFO, upon peripheral administration of LPS (Nakano et al., 2015). Central injection of TLR4 agonists induces morphological activation of microglia (Vargas-Caraveo et al., 2020). The treatment of LPS antagonist RS-LPS results in a markedly elongated morphology of microglia in the three CVOs, a shape generally seen in severe brain injury and infection (Vargas-Caraveo et al., 2020). Microglial proliferation in the brain is ubiquitous in chronic inflammation and exerts beneficial effects by attenuating sickness responses under LPS-induced chronic inflammation (Michels et al., 2019; Torii et al., 2022). Thus, the functional significance of microglia and macrophages in TLR4-dependent inflammation is largely different from TLR2, although they may work in conjunction with astrocytes during neuroinflammation.
Unique properties of microglia and macrophages in the circumventricular organs
Microglia are resident macrophage-like cells of mesoderm origin and are distinct from other types of neuroglia in the brain (Kettenmann et al., 2011). Although microglia are considered quiescent under normal physiological conditions, activated only upon an immune challenge or mechanical damage, recent evidence indicates that they control neuronal function and homeostasis without requiring these stimuli (Frick et al., 2013). For example, microglia are involved in synaptic pruning and the generation of neurons and oligodendrocytes (Paolicelli et al., 2011). Microglia exhibit a ramified morphology, a so-called “resting state” under physiologically normal conditions, but rapidly transform to amoeboid after infection of pathogens, toxins, mechanical injury, or radiation, together with dramatically increased proinflammatory cytokine gene expression and promotion of phagocytosis for dying cells or pathogens (Hanisch and Kettenmann, 2007; Colton, 2009). In the CVOs, surprisingly, microglia adopt the amoeboid form with fewer branched cellular processes even under normal conditions, whereas those in other brain regions exhibit the ramified form with well-branched and dendritic cellular processes (Figures 5A–E; Takagi et al., 2017). Moreover, the density of microglia and macrophages is more significant in the CVOs than in other brain regions (Figure 5F; Takagi et al., 2017).
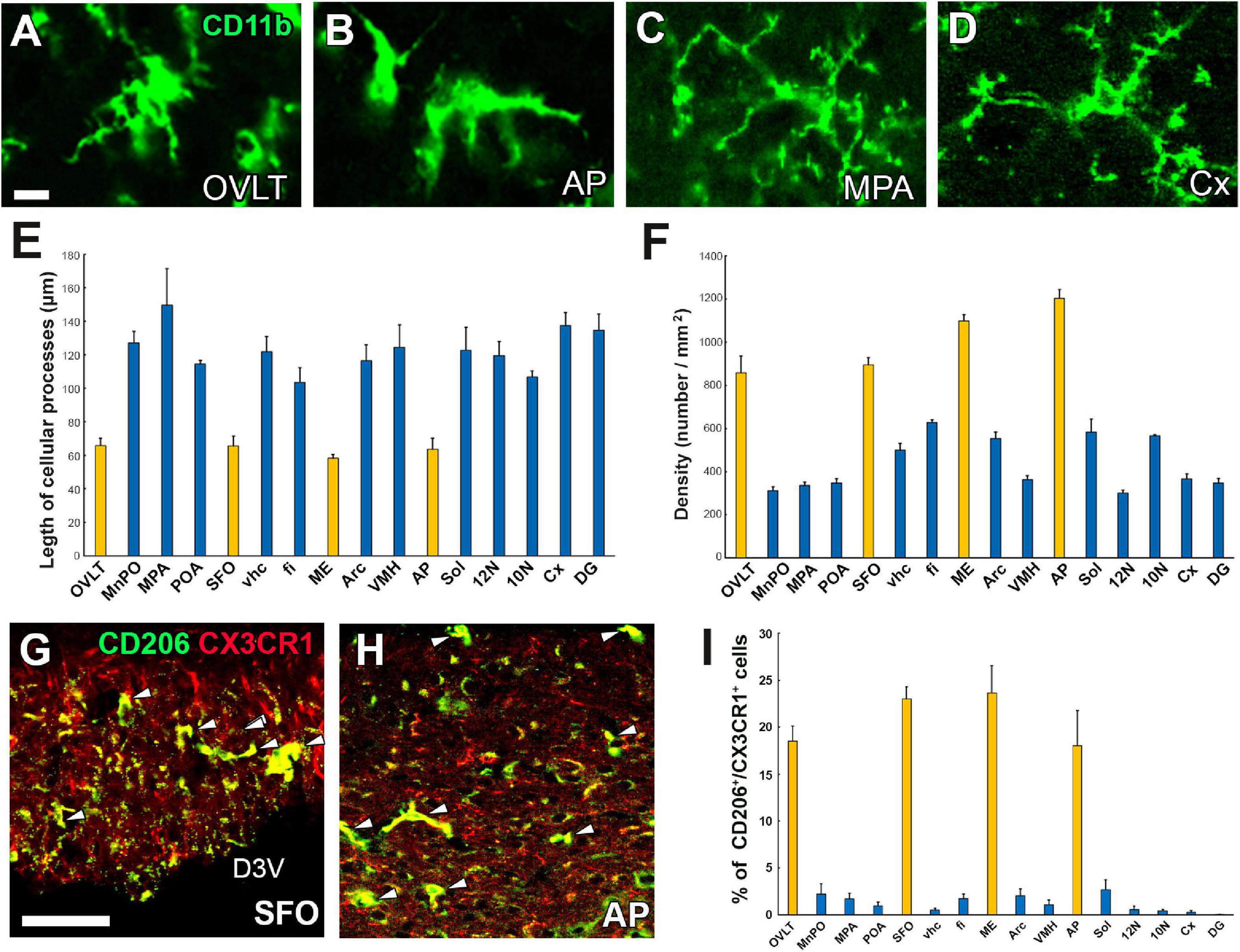
Figure 5. Activated state of microglia in the CVOs even under physiologically normal conditions. Microglia morphology in the CVOs, such as the OVLT and AP, is amoeboid, whereas in the medial preoptic area and cerebral cortex, it is dendritic (A–D). Quantitative analysis reveals shorter microglial cellular processes in the CVOs than other brain regions (E). The density of the CVOs microglia is significantly higher than that of other brain regions (F). Double-labeling immunohistochemistry shows that CX3CR1+ microglia express an M2 marker protein CD206 (arrowheads) in the SFO and AP (G,H). Quantitative analysis shows the percentage of CD206 expression in CX3CR1+ microglia is higher in the CVOs than that of other brain regions (I). 10N, dorsal motor nucleus of vagus nerve; 12N, hypoglossal nucleus; AP, area postrema; Cx, cerebral cortex; D3V, dorsal 3rd ventricle; DG, dentate gyrus; fi, fimbria; ME, median eminence; MnPO, median preoptic area; MPA, medial preoptic area; OVLT, organum vasculosum of the lamina terminalis; SFO, subfornical organ; Sol, solitary nucleus; vhc, ventral hippocampal commissure; VMH, ventromedial hypothalamic nucleus. Scale bars = 10 (A) and 50 μm (G). Scale bars = 50 μm. Yellow and blue bars indicate the CVOs and non-CVO regions, respectively. Photographs are rearranged with permission from Elsevier B.V. (Takagi et al., 2017).
In addition to the amoeboid shape, microglia and macrophages in the CVOs express higher levels of M1 marker proteins CD16/32 and CD86 and M2 marker proteins CD206 and Ym1 than other brain regions (Figures 5G–I; Takagi et al., 2017). Peripheral macrophages are classified into two activated states; the M1 phenotype, which shows a classical proinflammatory response by the production of inflammatory cytokines and reactive oxygen species, antigen presentation, and the removal of pathogens (Murray, 2017), and the M2 phenotype that expresses mediators or receptors to down-regulate, repair, or protect the body from inflammation (Mantovani et al., 2004). M1/M2 classification of macrophages has also been applied to microglia in the brain, although the M1/M2 categories have limitations (Randsohoff, 2016). This demonstrates that microglia and macrophages in the CVOs are continuously activated, considering the amoeboid morphology and M1 and M2 protein expression.
The reason for continuous activation of microglia and macrophage is not well understood. Endothelial cells in the CVOs lack tight junction proteins, allowing blood-derived molecules into the parenchyma (Morita and Miyata, 2012, 2013; Morita et al., 2016). CD163-expressing macrophages in AP perivascular space sequester dextran 10 kDa (molecular weight 10,000) within 5 min of administration (Willis et al., 2007). Perivascular macrophages in the pineal gland express M1 marker, MHC II and display phagocytosis activity (Kaur et al., 1997; Møller et al., 2006). In considering the function of these M1 and M2 marker proteins, activated microglia and macrophages phagocytize neurotoxic blood-derived molecules or cells to maintain CVO parenchyma microenvironment (Wang et al., 2014). Another possible activated microglia role is in structural dynamics such as neurogenesis and angiogenesis. Continuous angiogenesis with proliferation and apoptosis of endothelial cells occurs in the CVOs of adult mice (Morita et al., 2013, 2015; Furube et al., 2014; Asami et al., 2020). Conditioned media of resting-state microglia inhibits brain endothelial cell proliferation, whereas that from the activated-state microglia facilitates proliferation (Welser et al., 2010). Activated microglia increase vascular branching density in the retina (Biswas et al., 2017). Thus, activated microglia may regulate endothelial cell proliferation or removal of apoptotic endothelial cells. Activated microglia in the subventricular zone of developing brains promote neurogenesis and oligodendrogenesis (Shigemoto-Mogami et al., 2014). Differentiation of NPCs with M1 microglia supernatant leads to neurogenesis, while exposure to M2 supernatants leads to oligodendrogenesis (Butovsky et al., 2006; Yuan et al., 2017). Activated microglia are likely involved in controlling of oligodendrogenesis and neurogenesis by acting on NSCs and/or their progenitor cells.
Inflammation-induced microglial proliferation in the brain
Microglia are long-lived cells in adult mammalian brains, and their density remains stable under normal physiological conditions, although their turnover occurs several times in a lifetime through the spatial and temporal coordination of proliferation and apoptosis (Askew et al., 2017). Robust microglial proliferation often occurs under severe pathological brain conditions such as stroke-induced brain injury (Hou et al., 2020), spinal cord injury (Bellver-Landete et al., 2019), traumatic brain injury (Henry et al., 2020), and Alzheimer’s disease (Olmos-Alonso et al., 2016). Recently, we revealed that LPS-induced microglial proliferation occurs in brain regions, such as the hypothalamus, medulla oblongata, and telencephalon, with marked increases in the CVOs, by LPS administration at a low dose of 100 μg/kg (Figures 6A–F; Furube et al., 2018). Moreover, the single peripheral administration of 1 mg/kg LPS induced robust microglial proliferation in most brain regions, except for the cerebral cortex and hippocampus, in the adult mouse (Fukushima et al., 2015; Furube et al., 2015, 2018). The CVOs are the blood-brain interface to initiate early stages of brain inflammation; prominent activation of STAT3 (Rummel et al., 2005; Yoshida et al., 2016) and NF-κB signaling (Muneoka et al., 2019), and increased expression of Fos (Brochu et al., 1999) and cytokine mRNA (Quan et al., 1997; Brochu et al., 1999). On the other hand, a proliferation of microglia does not occur in the cerebral cortex and hippocampus after peripheral LPS administration of 100 and 1 mg/kg. This indicates increased proliferation of microglia during LPS-induced inflammation in a region- and dose-dependent manner, demonstrating proliferation sensitivity variations of microglia. Microglial proliferation peaks 24–72 h after intraperitoneal LPS injection accompanied by increased microglial density, but the density returns to normal levels within 3 weeks (Figure 6G; Furube et al., 2018). Microglial proliferation is also observed during inflammation induced by the toll-like receptor 2 (TLR2) agonist zymosan and PGE2 (Torii et al., 2022). These results indicate that microglial proliferation is a general event of neuroinflammation rather than a specialized one in severe neurodegenerative diseases accompanied by neuronal death.
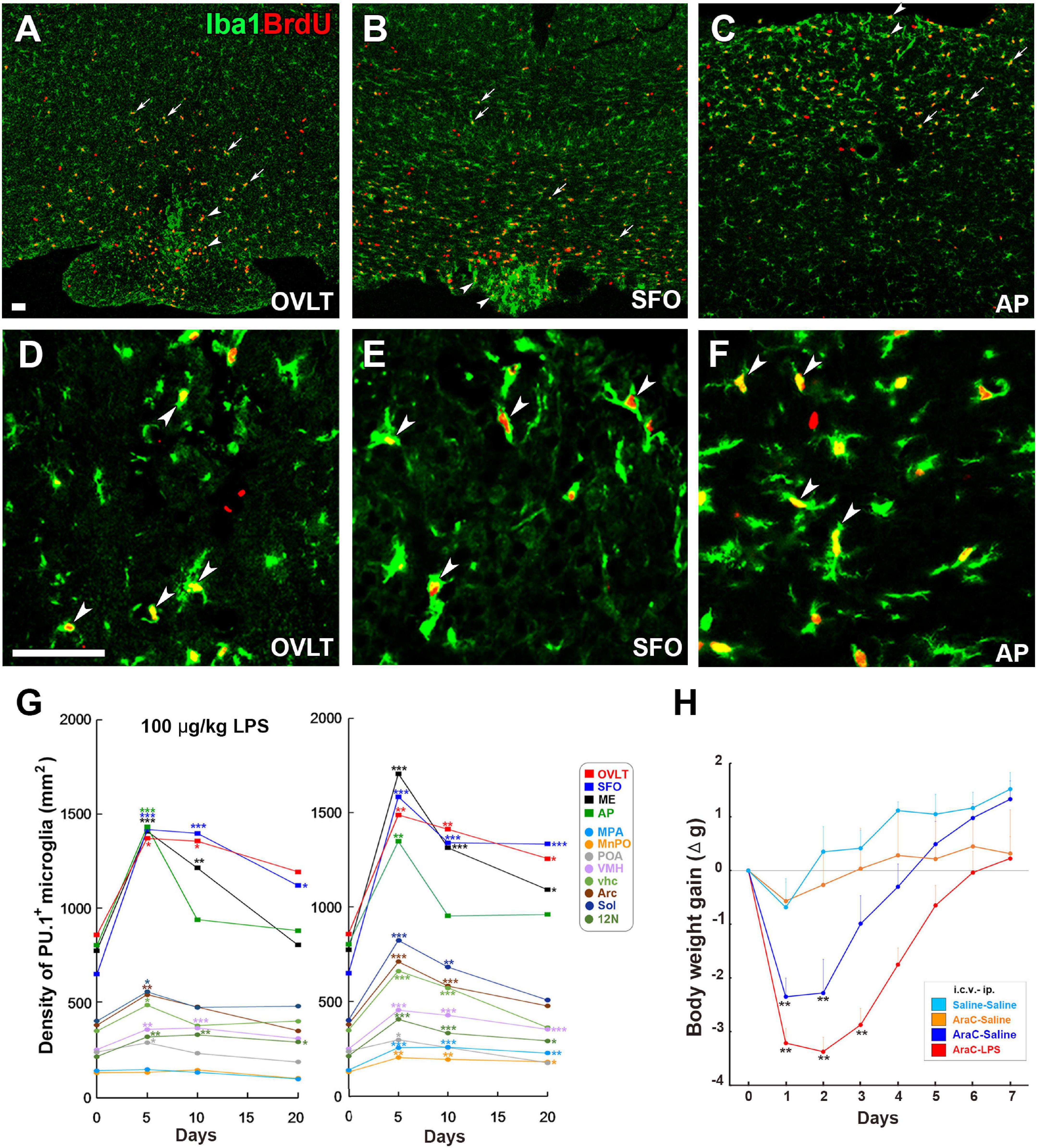
Figure 6. Remarkable increase in proliferation of microglia and macrophages in the CVOs and neighboring brain regions in adult mouse brain after LPS administration. Intraperitoneal administration of 100 μg/kg LPS induces a robust increase of Iba1+ BrdU+ proliferating microglia and macrophages in the OVLT (arrowheads) and medial preoptic area (MPA; arrows) (A), the SFO (arrowheads) and ventral hippocampal commissure (vhc; arrows) (B), and the AP (arrowheads) and solitary nucleus (Sol; arrows) (C). High magnification reveals many Iba1+ BrdU+ proliferating microglia and macrophages in the CVOs (D–F). Scale bars = 100 (A) and 50 μm (D). Photographs are rearranged with permission from Springer Nature (Furube et al., 2015, 2018). The density of PU.1+ microglia and macrophages is significantly elevated in the CVOs and neighboring brain regions on the 5th and 10th day after administration of 100 μg/kg and 1 mg/kg LPS, but returns to almost normal levels on the 20th day (G). The i.c.v. infusion of the mitotic inhibitor AraC significantly delays body weight recovery after 5 mg/kg administration (H). 10N, dorsal motor nucleus of vagus nerve; 12N, hypoglossal nucleus; AP, area postrema; fi, fimbria; MnPO, median preoptic area; MPA, medial preoptic area; OVLT, organum vasculosum of the lamina terminalis; SFO, subfornical organ; Sol, solitary nucleus; vhc, ventral hippocampal commissure; VMH, ventromedial hypothalamic nucleus. *p < 0.05, **p < 0.01, ***p 0.001 vs. the control by an ANOVA with Tukey’s post-hoc test. Graphs are rearranged with permission from Springer Nature [(A) Furube et al., 2018] and Elsevier B.V. [(B) Torii et al., 2022].
The inhibition of LPS-induced microglial proliferation with continuous i.c.v. infusion of AraC causes a persistent reduction in body weight and intake of food and water and prolongs LPS-induced sickness responses, such as lower locomotor activity and core body temperature (Figure 6H; Torii et al., 2022). Thus, a transient increase in the microglial population is beneficial during endotoxin-induced inflammation as it attenuates sickness response. The selective elimination of microglia with a colony-stimulating factor1 receptor (CSF1R) antagonist increased neuronal death in an acute stroke model (Hou et al., 2020) and spinal cord injury (Bellver-Landete et al., 2019). In contrast to the neuroprotective function in acute injury, the elimination of microglia with a CSF1R antagonist attenuates neurodegeneration during the chronic phase of traumatic brain injury (Henry et al., 2020) and Alzheimer’s (Olmos-Alonso et al., 2016). Therefore, microglia appear to exert beneficial or deleterious effects depending on brain disease, beneficial in acute inflammation and deleterious in chronic inflammation.
Fluid homeostasis by osmolality and Na+ sensitive sensor in the circumventricular organs
Animals with the electrolytic lesion of the OVLT and SFO, namely AV3V region, show a decreased response to dehydration or hypertonic saline ingestion, exhibiting reduced fluid intake, Fos expression, and AVP and OT secretion (Morris et al., 1994; Miyata et al., 1996; Rocha et al., 1999; Oliveira et al., 2004), indicating that AV3V region is crucial for the reception and integration of osmotic signals. TRPV1 is the first in the thermosensitive TRP family of proteins or non-selective cation channels (Caterina et al., 1997; Julius, 2013). TRPV1 is activated by noxious high temperatures (>42°C), pH, positive voltages, and chemicals such as capsaicin (Julius, 2013). Moreover, TRPV1 is involved in mechanical pain and high osmolality detection by responding to cellular shape alterations (Bourque, 2008). Although TRPV1 is known to be expressed at somatosensory neuron nerve endings (Romanovsky et al., 2009), it is also highly expressed in adult brain CVOs (Ciura and Bourque, 2006; Mannari et al., 2013; Sladek and Johnson, 2013). Moreover, prominent expression of TRPV1 is detected at astrocytic and tanycytic cellular processes, which contact closely with fenestrated capillaries and form dense networks (Figures 7A–C; Mannari et al., 2013). The TRPV1 deficiency of the OVLT results in decreased sensitivity to hyperosmolality (Ciura and Bourque, 2006). Full-length TRPV1 responds to hyperosmolality but is less sensitive to hypoosmolality (Nishihara et al., 2011). In contrast to these in vitro experiments, acute and chronic hyperosmolality produces a similar increase in the number of Fos-positive neurons in the OVLT, AVP secretion, and water intake in WT vs. TRPV1 KO mice (Taylor et al., 2008; Kinsman et al., 2014; Tucker and Stocker, 2016). These results indicate that TRPV1 is not responsible for in vivo body fluid homeostasis.
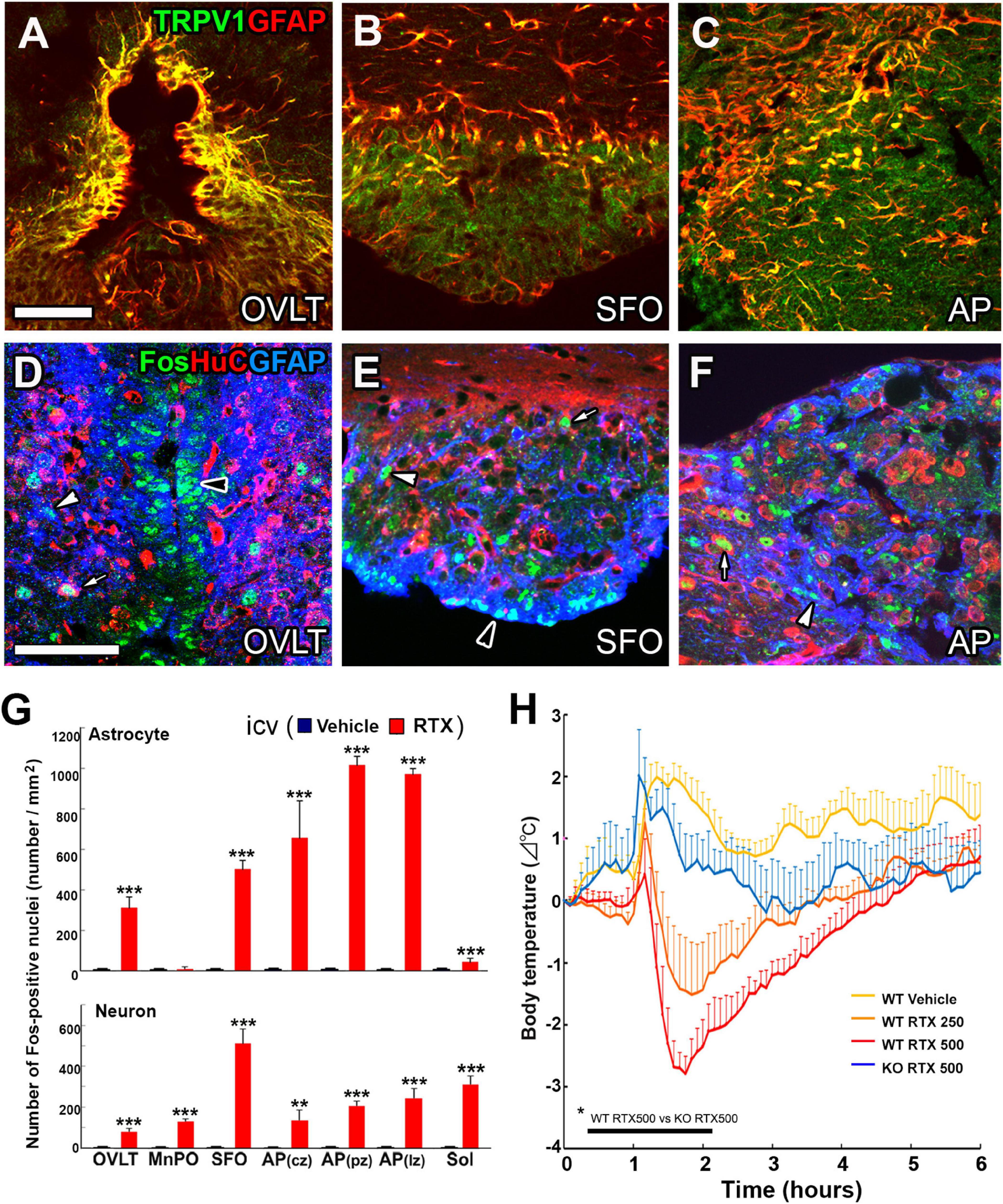
Figure 7. Prominent TRPV1 and TRPV1-dependent Fos expression at astrocytes in the CVOs of adult mouse brain and hypothermia by central activation of TRPV1. The expression of TRPV1 is prominent at GFAP+ astrocytes in the CVOs (A–C). The i.c.v. infusion of the TRPV1 agonist resiniferatoxin at 500 ng/kg causes prominent Fos expression at astrocytes rather than neurons in the CVOs (D–F). AP, area postrema; GFAP, glial fibrillar acidic protein; OVLT, organum vasculosum of the lamina terminalis; SFO, subfornical organ. Scale bars = 50 μm. Quantitative analysis shows central infusion of resiniferatoxin induces significant Fos expression at astrocytes preferentially in the CVOs and at neurons in the CVOs and neighboring brain regions (G). The i.c.v. infusion of the TRPV1 agonist resiniferatoxin induces hypothermia in a dose-dependent manner in WT mice, but not in TRPV1 KO mice (H). **p < 0.01, ***p0.001 between WT and TRPV1 KO mice with unpaired Student’s t-test (G) or an ANOVA with Tukey’s post-hoc-test (H). Photographs and graphs are rearranged with permission from John Wiley & Sons [(A–G) Mannari et al., 2013] and Springer Nature [(H) Yoshida et al., 2016].
An alternative candidate for in vivo fluid homeostasis control is the Nax channel, which detects Na+ levels (Noda, 2006, 2007; Noda and Hiyama, 2015). The expression of the Nax channel occurs specifically at astrocytes and tanycytes in the CVOs such as the OVLT, SFO, ME, and NH (Watanabe et al., 2000, 2006). The Nax channels are activated depending on Na+ concentration, but not osmolality, with a threshold of about 150 mM (Hiyama et al., 2002). WT mice show extensive water intake and aversion to saline intake, whereas Nax KO animals did not exhibit such behaviors (Hiyama et al., 2004). Abnormal behaviors, such as water intake and aversion to saline in Nax KO mice, are recovered by a site-directed infection of an adenoviral vector with the Nax gene into the SFO, indicating that the SFO is the primary brain center regulating water and salt intake behaviors (Hiyama et al., 2004).
Warm-sensitive transient receptor potential vanilloid 1-expressing glial cells in the circumventricular organs
Body thermoregulation is closely connected to body water homeostasis, and the CVOs act as integrators of thermal and osmotic signals in the brain (Sladek and Johnson, 2013). The physiological benefits of this are; first, the CVOs lack the typical BBB to protect from blood-derived molecules such as ions and low and high molecular hydrophilic molecules (Morita and Miyata, 2012; Miyata, 2015), but they directly and rapidly detect ions, osmolality, and blood temperature. Second, the capillary density is higher in the CVOs than in other brain regions, enabling efficient detection of blood-derived signal. The peripheral and central administration of the TRPV1 agonist resiniferatoxin, induced Fos expression preferentially in the CVO astrocytes and tanycytes and neurons of the median preoptic nuclei and Sol (Figures 7D–F; Mannari et al., 2013). Moreover, the peripheral and central administration of resiniferatoxin robustly induces STAT3 signaling in astrocytes of the CVOs and POA, but less in neurons (Figure 7G; Mannari et al., 2013). A similar response to the peripheral and central administration of LPS (Rummel et al., 2004, 2005; Nakano et al., 2015). IL-6 activates STAT3 signaling in the brain (Akira, 1997) and is necessary to induce fever (Chai et al., 1996; Nilsberth et al., 2009). The activation of TRPV1 stimulates c-Jun and p38 mitogen-activated protein kinases, releasing cytokines IL-6 and -8 in CD4+ cells (Bertin et al., 2014). The central administration of RTX causes a strong dose-dependent decrease in body temperature (Figure 7H; Yoshida et al., 2016). Peripheral administration of LPS induces less fever response in TRPV1 KO mice compared with WT animals (Iida et al., 2005). Thus, TRPV1 is necessary to maintain LPS-induced fever, as LPS sensitizes TRPV1 through TLR4 activation in the trigeminal sensory neurons (Diogenes et al., 2011).
The deletion of TRPV1-expressing somatosensory neurons eliminates heat avoidance behavior in two-plate preference tests (Pogorzala et al., 2013). However, TRPV1 KO mice are dispensable for typical heat avoidance between 40 and 50°C by two-plate preference tests (Pogorzala et al., 2013) and normal warm sensation between 32 and 42°C by goal-directed thermal perception task (Paricio-Montesinos et al., 2020). No significant difference is detected in colonic temperature between WT and TRPV1 KO mice when exposed to 37.0°C (Szelenyi et al., 2004), 39.0°C (Garami et al., 2011), or 40°C (Figure 8A; Park et al., 2020). This raises the question as to the function of TRPV1 upon acute ambient temperature increase. Recently, our research suggests that TRPV1 is crucial for thermoregulation by initiating heat loss behaviors under warm ambient temperatures less than 37°C (Figure 8B; Park et al., 2020). TRPV1 KO mice showed significantly shorter durations for heat loss behaviors such as sleeping and body licking than WT mice upon exposure conditions between 30.0 and 35.0°C (Park et al., 2020). On the other hand, no significant difference in heat loss behavior time is observed between WT and TRPV1 KO mice upon exposure to 40°C (Park et al., 2020), indicating that exposure to heat greater than body temperature is beyond the cooling capacity of these heat loss behaviors. Thus, TRPV1 is necessary for thermoregulation under moderate warm ambient temperatures to control heat loss behaviors. The oral gavage of the TRPV1 agonist capsaicin quickly decreases core body temperature together with increased tail surface temperature in WT mice via activation of the CVOs and POA, whereas TRPV1 KO animals do not exhibit such changes (Inagaki et al., 2019). Moreover, oral gavage of capsaicin induces a long-lasting increase in locomotor activity in a TRPV1-independent manner, as increased locomotor activity was seen in both WT and TRPV1 KO animals (Inagaki et al., 2019). Capsaicin dilates blood vessels in the skin, and increases heat exchange and metabolic activity in humans (Luo et al., 2011; Reyes-Escogido Mde et al., 2011; Adaszek et al., 2019).
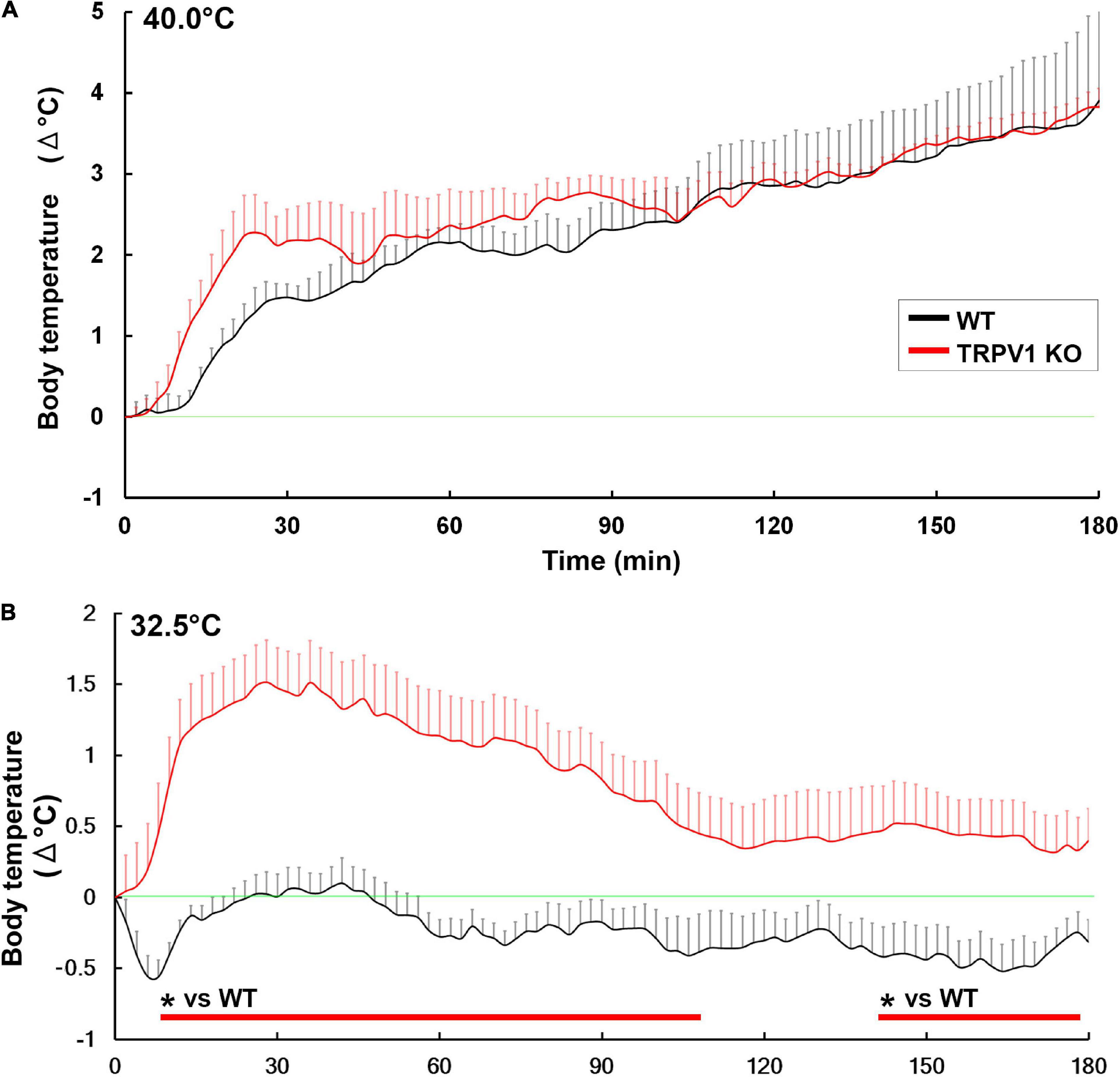
Figure 8. Abnormal hyperthermia in TRPV1 KO mice upon exposure to moderately warm ambient temperature. Both WT and TRPV1 KO mice showed similar increases in core body temperature upon exposure to 40°C (A). Upon exposure to 32.5°C, TRPV1 KO mice exhibited hyperthermia, but hyperthermia was not seen in WT mice (B). *p < 0.05 between WT and TRPV1 KO mice with unpaired Student’s t-test. Graphs are rearranged with permission from Springer Nature (Park et al., 2020).
Transient receptor potential vanilloid 1 is expressed in astrocytes and tanycytes of the CVOs, as previously mentioned (Mannari et al., 2013; Sladek and Johnson, 2013). Central and peripheral administration of RTX causes STAT3 signaling activation and Fos expression at astrocytes and tanycytes rather than neurons in the CVOs (Mannari et al., 2013). In the CVOs, the fenestrated capillaries are surrounded by dense networks of astrocytic and tanycytic cellular processes. Neuronal somata are generally located at a moderate distance from fenestrated capillaries and are covered with glial cellular processes (Miyata, 2015; Morita et al., 2016). This unique spatial niche is necessary to protect neurons from molecules and ions that diffuse beyond the fenestrated capillaries. Hypoosmolality results in an influx of water into the brain parenchyma via glial cells, then brain water is shunted into astrocytes, which preserves neuronal cell size and prevents brain damage (Ayus et al., 2008). These results indicate that TRPV1-expressing astrocytes and tanycytes are “warm-sensitive glial cells.”
Glia-neuronal communication in the circumventricular organs and transmission to other brain regions
Bidirectional communications between glial cells and neurons are essential for axonal conduction and synaptic transmission (Fields and Stevens-Graham, 2002). A transient increase in astrocytic Ca2+ levels results in a gliotransmitter release, which acts on neurons and vascular smooth muscle (Bazargani and Attwell, 2016). Raising the question of how the fluid or thermal information sensed by astrocytes and tanycytes is then transferred to neurons. Coordinated activation of Nax and Na+/K+ ATPase enhances glucose uptake and extensive lactate production in astrocytes and tanycytes in the SFO in response to elevated Na+ levels (Shimizu et al., 2007). Moreover, the Na+-dependent release of the glial transmitter lactate from astrocytes and tanycytes stimulates GABAergic neurons in the SFO to induce water intake behavior (Shimizu et al., 2007). Endothelin-3 decreases the Nax sensitivity threshold of Na+ from ∼ 150 to 135–145 mM and increases lactate release in the SFO (Hiyama et al., 2013). Thus, thermal information detected by TRPV1 in astrocytes and tanycytes is also transferred to neurons by glial transmitters.
Peripheral administration of a TRPV1 agonist induces hypothermia (Caterina et al., 1997), but a TRPV1 antagonist causes fever (Szelenyi et al., 2004). While central administration of TRPV1 agonists induced hypothermia, TRPV1 antagonists had no effect (Yoshida et al., 2016; Park et al., 2020). These results indicate that peripheral TRPV1 is always activated, but central TRPV1 is inactivated. The glial-neuronal pathways of activated TRPV1 in the CVOs are well understood; however, the hypothermic brain center was recently reported. Opsin 5-, adcyap1-, or QRFP-expressing neurons in the lateral hypothalamic area around the third ventricle of the anterior part of the hypothalamus are shown to be hypothermic and hypometabolic neurons (Hrvatin et al., 2020; Takahashi et al., 2020; Zhang K. X. et al., 2020). These hypothalamic neurons are closely located to the OVLT, indicating that glial TRPV1 activation information is transferred to these neurons, thereby causing hypothermia.
Thermal and osmotic information monitoring and integration in the OVLT and SFO are sent to the median preoptic area (MnPO) that functions as osmoregulation, thermoregulation, and sleep homeostasis (Sladek and Johnson, 2013). In addition, the OVLT and SFO project to the supraoptic and paraventricular nuclei, which are the autonomic center, oxytocin, and vasopressin-synthesizing regions (Miyata and Hatton, 2002; Sladek and Johnson, 2013). The SFO and OVLT respond to blood angiotensin II, relaxin, and hyperosmolarity to drive thirst-related neural pathways. However, amylin and leptin act at the AP to influence neural pathways inhibiting food intake (McKinley et al., 2019). Activation of ADCYAP1-expressing neurons in the AP and Sol induces LPS-induced sickness responses such as reduced food and water intake, locomotor activity, and body temperature using the TRAP2 system. In contrast, inhibition of these neurons significantly weakens all responses except for effect on body temperature (Ilanges et al., 2022). Moreover, the activation of PHOX2B-expressing neurons in nearly all AP cells and some NTS cells and DBH-expressing neurons in LPS-activated AP neurons decreases food and water intake and locomotor activity (Ilanges et al., 2022). These data indicate that ADCYAP1-expressing neurons in the AP and Sol are essential for endotoxin-induced sickness behaviors.
Author contributions
The author confirms being the sole contributor of this work and has approved it for publication.
Funding
This work was supported in part by the Scientific Research Grants from The Japan Society for the Promotion of Science (Nos. 19K06921 and 22K06456).
Acknowledgments
The author grateful to E. Furube, H. Mannari, S. Morita, S. Muneoka, S. Murayama, Y. Park, S. Takagi, K. Torii, and A. Yoshida for collaboration and discussion on the early stages of work. The author would also like to show our gratitude to the Elsevier B.V., John Wiley & Sons, and Springer Nature for permission to reuse figures.
Conflict of interest
The author declares that the research was conducted in the absence of any commercial or financial relationships that could be construed as a potential conflict of interest.
Publisher’s note
All claims expressed in this article are solely those of the authors and do not necessarily represent those of their affiliated organizations, or those of the publisher, the editors and the reviewers. Any product that may be evaluated in this article, or claim that may be made by its manufacturer, is not guaranteed or endorsed by the publisher.
References
Aboudounya, M. A., and Heads, R. J. (2021). COVID-19 and Toll-like receptor 4 (TLR4): SARS-CoV-2 may bind and activate TLR4 to increase ACE2 expression, facilitating entry and causing hyperinflammation. Mediators Inflamm. 2021:8874339. doi: 10.1155/2021/8874339
Adaszek, Ł, Gadomska, D., Mazurek, Ł, Łyp, P., Madany, J., and Winiarczyk, S. (2019). Properties of capsaicin and its utility in veterinary and human medicine. Res. Vet. Sci. 123, 14–19. doi: 10.1016/j.rvsc.2018.12.002
Akira, S. (1997). IL-6-regulated transcription factors. Int. J. Biochem. Cell Biol. 29, 1401–1418. doi: 10.1016/s1357-2725(97)00063-0
Akira, S., Takeda, K., and Kaisho, T. (2001). Toll-like receptors: Critical proteins linking innate and acquired immunity. Nat. Immunol. 2, 675–680. doi: 10.1038/90609
Asami, A., Kurganov, E., and Miyata, S. (2020). Proliferation of endothelial cells in the choroid plexus of normal and hydrocephalic mice. J. Chem. Neuroanat. 106:101796. doi: 10.1016/j.jchemneu.2020.101796
Askew, K., Li, K., Olmos-Alonsom, A., Garcia-Morenom, F., Liangm, Y., Richardsonm, P., et al. (2017). Coupled proliferation and apoptosis maintain the rapid turnover of microglia in the adult brain. Cell Rep. 18, 391–405. doi: 10.1016/j.celrep.2016.12.041
Ayus, J. C., Achinger, S. G., and Arieff, A. (2008). Brain cell volume regulation in hyponatremia: Role of sex, age, vasopressin and hypoxia. Am. J. Physiol. 295, F619–F624. doi: 10.1152/ajprenal.00502.2007
Balland, E., Dam, J., Langlet, F., Caron, E., Steculorum, S., Messina, A., et al. (2014). Hypothalamic tanycytes are an ERK-gated conduit for leptin into the brain. Cell Metabol. 19, 293–301. doi: 10.1016/j.cmet.2013.12.015
Barar, J., Rafi, M. A., Pourseif, M. M., and Omidi, Y. (2016). Blood-brain barrier transport machineries and targeted therapy of brain diseases. Bioimpacts 6, 225–248. doi: 10.15171/bi.2016.30
Bargul, J. L., Jung, J., McOdimba, F. A., Omogo, C. O., Adung’a, V. O., and Krüger, T. (2016). Species-specific adaptations of trypanosome morphology and motility to the mammalian host. PLoS Pathog. 12:e1005448. doi: 10.1371/journal.ppat.1005448
Bazargani, N., and Attwell, D. (2016). Astrocyte calcium signaling: The third wave. Nat. Neurosci. 19, 182–189. doi: 10.1038/nn.4201
Bellver-Landete, V., Bretheau, F., Mailhot, B., Vallieres, N., Lessard, M., Janelle, M. E., et al. (2019). Microglia are an essential component of the neuroprotective scar that forms after spinal cord injury. Nat. Commun. 10:518. doi: 10.1038/s41467-019-08446-0
Bentivoglio, M., Kristensson, K., and Rottenberg, M. E. (2018). Circumventricular organs and parasite neurotropism: Neglected gates to the brain? Front. Immunol. 9:2877. doi: 10.3389/fimmu.2018.02877
Bertin, S., Aoki-Nonaka, Y., Rudolf de Jong, P., Nohara, L., Xu, H., and Stanwood, S. R. (2014). The ion channel TRPV1 regulates the activation and proinflammatory properties of CD4? T cells. Nat. Immunol. 15, 1055–1063. doi: 10.1038/ni.3009
Bhattacharya, M., Sharma, A. R., Mallick, B., Sharma, G., Lee, S. S., and Chakraborty, C. (2020). Immunoinformatics approach to understand molecular interaction between multi-epitopic regions of SARS-CoV-2 spike-protein with TLR4/MD-2 complex. Infect. Genet. Evol. 85:104587. doi: 10.1016/j.meegid.2020.104587
Biswas, S., Bachay, G., Chu, J., Hunter, D. D., and Brunken, W. J. (2017). Laminin-dependent interaction between astrocytes and microglia: A role in retinal angiogenesis. Am. J. Pathol. 187, 2112–2127. doi: 10.1016/j.ajpath.2017.05.016
Blatteis, C. M., Bealer, S. L., Hunter, W. S., Llanos-Q, J., Ahokas, R. A., and Mashburn, T. A. Jr. (1983). Suppression of fever after lesions of the anteroventral third ventricle in guinea pigs. Brain Res. Bull. 11, 519–526. doi: 10.1016/0361-9230(83)90124-7
Blomqvist, A., and Engblom, D. (2018). Neural mechanisms of inflammation-induced fever. Neuroscientist 24, 381–399. doi: 10.1177/1073858418760481
Bolborea, M., and Dale, N. (2013). Hypothalamic tanycytes: Potential roles in the control of feeding and energy balance. Trends Neurosci. 36, 91–100. doi: 10.1016/j.tins.2012.12.008
Bourque, C. W. (2008). Central mechanisms of osmosensation and systemic osmoregulation. Nat. Rev. Neurosci. 9, 519–531. doi: 10.1038/nrn2400
Brochu, S., Olivier, M., and Rivest, S. (1999). Neuronal activity and transcription of proinflammatory cytokines, IkappaBalpha, and iNOS in the mouse brain during acute endotoxemia and chronic infection with Trypanosoma brucei brucei. J. Neurosci. Res. 57, 801–816. doi: 10.1002/(SICI)1097-4547(19990915)57:6<801::AID-JNR5<3.0.CO;2-B
Bsibsi, M., Persoon-Deen, C., Verwer, R. W. H., Meeuwsen, S., Ravid, R., and Van Noort, J. M. (2006). Toll-like receptor 3 on adult human astrocytes triggers production of neuroprotective mediators. Glia 53, 688–695. doi: 10.1002/glia.20328
Butovsky, O., Ziv, Y., Schwartz, A., Landa, G., Talpalar, A. E., Pluchino, S., et al. (2006). Microglia activated by IL-4 or IFN-gamma differentially induce neurogenesis and oligodendrogenesis from adult stem/progenitor cells. Mol. Cell. Neurosci. 31, 149–160. doi: 10.1016/j.mcn.2005.10.006
Buzhdygan, T. P., DeOre, B. J., Baldwin-Leclair, A., Bullock, T. A., McGary, H. M., Khan, J. A., et al. (2020). The SARS-CoV-2 spike protein alters barrier function in 2D static and 3D microfluidic in-vitro models of the human blood-brain barrier. Neurobiol. Dis. 146:105131. doi: 10.1016/j.nbd.2020.105131
Caterina, M. J., Schumacher, M. A., Tominaga, M., Rosen, T. A., Levine, J. D., and Julius, D. (1997). The capsaicin receptor: A heat-activated ion channel in the pain pathway. Nature 389, 816–824. doi: 10.1038/39807
Cerqueira, D. R., Ferreira, H. S., Moiteiro, A. L. B. B., and Fregoneze, J. B. (2016). Effects of interleukin-1 beta injections into the subfornical organ and median preoptic nucleus on sodium appetite, blood pressure and body temperature of sodium-depleted rats. Physiol. Behav. 163, 149–160. doi: 10.1016/j.physbeh.2016.05.003
Chai, Z., Gatti, S., Toniatti, C., Poli, V., and Bartfai, T. (1996). Interleukin (IL)-6 gene expression in the central nervous system is necessary for fever response to lipopolysaccharide or IL-1β: A study on IL-6-deficient mice. J. Exp. Med. 183, 311–316. doi: 10.1084/jem.183.1.311
Chakravarty, S., and Herkenham, M. (2005). Toll-like receptor 4 on nonhematopoietic cells sustains CNS inflammation during endotoxemia, independent of systemic cytokines. J. Neurosci. 25, 1788–1796. doi: 10.1523/JNEUROSCI.4268-04.2005
Chen, Y. M., and Miner, J. H. (2012). Glomerular basement membrane and related glomerular disease. Trans. Res. 160, 291–297. doi: 10.1016/j.trsl.2012.03.004
Ciura, S., and Bourque, C. W. (2006). Transient receptor potential vanilloid 1 is required for intrinsic osmoreception in organum vasculosum lamina terminalis neurons and the normal thirst responses to systemic hyperosmolality. J. Neurosci. 26, 9069–9075. doi: 10.1523/JNEUROSCI.0877-06.2006
Colton, C. A. (2009). Heterogeneity of microglial activation in the innate immune response in the brain. J. NeuroImmune Pharmacol. 4, 399–418. doi: 10.1007/s11481-009-9164-4
Dellmann, H. D. (1998). Structure of the subfornical organ: A review. Microsc. Res. Tech. 41, 85–97. doi: 10.1002/(SICI)1097-0029(19980415)41:2<85::AID-JEMT1<3.0.CO;2-P
Diogenes, A., Ferraz, C. C. R., Akopian, A. N., Henry, M. A., and Hargreaves, K. M. (2011). LPS sensitizes TRPV1 via activation of TLR4 in trigeminal sensory neurons. J. Dental. Res. 90, 759–764. doi: 10.1177/0022034511400225
Doobay, M. F., Talman, L. S., Obr, T. D., Tian, X., Davisson, R. D., and Lazartigues, E. (2007). Differential expression of neuronal ACE2 in transgenic mice with overexpression of the brain renin-angiotensin system. Am. J. Physiol. 292, R373–R381. doi: 10.1152/ajpregu.00292.2006
Ehrlich, R. H. (1885). Das Sauerstoff-Bedurfinis dews Oraganisms. Eine Farenanalytisch Studie. Ph.D. Thesis. Berlin: Herschwarld, 69–72.
Ellinger, I., and Fuchs, R. (2010). Receptor-mediated and fluid-phase transcytosis of horseradish peroxidase across rat hepatocytes. J. Biomed. Biotechnol. 2010:850320. doi: 10.1155/2010/850320
Ericsson, A., Liu, C., Hart, R. P., and Sawchenko, P. E. (1995). Type 1 interleukin-1 receptor in the rat brain: Distribution, regulation, and relationship to sites of IL-1-induced cellular activation. J. Comp. Neurol. 361, 681–698. doi: 10.1002/cne.903610410
Eskilsson, A., Tachikawa, M., Hosoya, K., and Blomqvist, A. (2014). Distribution of microsomal prostaglandin E synthase-1 in the mouse brain. J. Comp. Neurol. 522, 3229–3244. doi: 10.1002/cne.23593
Farina, C., Aloisi, F., and Meinl, E. (2007). Astrocytes are active players in cerebral innate immunity. Trends Immunol. 28, 138–145. doi: 10.1016/j.it.2007.01.005
Ferguson, A. V. (2014). “Circumventricular organs; integrators of circulating signals controlling hydration, energy balance, and immune function,” in Neurobiology of Body Fluid Homeostasis: Transduction and Integration, eds L. A. De Luca Jr., J. V. Menani, and A. K. Johnson (Boca Raton, FL: CRC Press).
Fields, R. D., and Stevens-Graham, B. (2002). New insights into neuron-glia communication. Science 298, 556–562. doi: 10.1126/science.298.5593.556
Frick, L. R., Williams, K., and Pittenger, C. (2013). Microglial dysregulation in psychiatric disease. Clin. Dev. Immunol. 2013:608654. doi: 10.1155/2013/608654
Fry, M., Hoyda, T. D., and Ferguson, A. V. (2007). Making sense of it: Roles of the sensory circumventricular organs in feeding and regulation of energy homeostasis. Exp. Biol. Med. 232, 14–26.
Fukushima, S., Furube, E., Itoh, M., Nakashima, T., and Miyata, S. (2015). Robust increase of microglia proliferation in the fornix of hippocampal axonal pathway after a single LPS stimulation. J. Neuroimmunol. 285, 31–40. doi: 10.1016/j.jneuroim.2015.05.014
Furube, E., Ishi, H., Nambu, Y., Kurganov, E., Nagaoka, S., Morita, M., et al. (2020). Neural stem cell phenotype of tanycyte-like ependymal cells in the circumventricular organs and central canal of adult mouse. Sci. Rep. 10:2826. doi: 10.1038/s41598-020-59629-5
Furube, E., Kawai, S., Inagaki, H., Takagi, S., and Miyata, S. (2018). Brain region-dependent heterogeneity and dose-dependent difference in transient microglia population increase during lipopolysaccharide-induced inflammation. Sci. Rep. 8:2203. doi: 10.1038/s41598-018-20643-3
Furube, E., Mannari, T., Morita, S., Nishikawa, K., Yoshida, A., Itoh, M., et al. (2014). VEGF-dependent and PDGF-dependent dynamic neurovascular reconstruction in the neurohypophysis of adult mice. J. Endocrinol. 222, 161–179. doi: 10.1530/JOE-14-0075
Furube, E., Morita, M., and Miyata, S. (2015). Characterization of neural stem cells and their progeny in the sensory circumventricular organs of adult mouse. Cell. Tissue Res. 362, 347–365. doi: 10.1007/s00441-015-2201-0
Furuse, M., Furuse, K., Sasaki, H., and Tsukita, S. (2001). Conversion of zonulae occludentes from tight to leaky strand type by introducing claudin-2 into Madin-Darby canine kidney I cells. J. Cell Biol. 153, 263–272. doi: 10.1083/jcb.153.2.263
Furuse, M., Hata, M., Furuse, K., Yoshida, Y., Haratake, A., Sugitani, Y., et al. (2002). Claudin-based tight junctions are crucial for the mammalian epidermal barrier: A lesson from claudin-1-deficient mice. J. Cell Biol. 156, 1099–1111. doi: 10.1083/jcb.200110122
Ganong, W. F. (2000). Circumventricular organs: Definition and role in the regulation of endocrine and autonomic function. Clin. Exp. Pharmacol. Physiol. 27, 422–427. doi: 10.1046/j.1440-1681.2000.03259.x
Garami, A., Pakai, E., Oliveira, D. L., Steiner, A. A., Wanner, S. P., Almeida, M. C., et al. (2011). Thermoregulatory phenotype of the Trpv1 knockout mouse: Thermoeffector dysbalance with hyperkinesis. J. Neurosci. 31, 1721–1733. doi: 10.1523/JNEUROSCI.4671-10.2011
Gay, N. J., Symmons, M. F., Gangloff, M., and Bryant, C. E. (2014). Assembly and localization of Toll-like receptor signalling complexes. Nat. Rev. Immunol. 14, 546–558. doi: 10.1038/nri3713
Gonçalves, R. A., and De Felice, F. G. (2021). The crosstalk between brain and periphery: Implications for brain health and disease. Neuropharmacol. 197:108728. doi: 10.1016/j.neuropharm.2021.108728
Goodman, T., and Hajihosseini, M. K. (2015). Hypothalamic tanycytes—masters and servants of metabolic, neuroendocrine, and neurogenic functions. Front. Neurosci. 9:387. doi: 10.3389/fnins.2015.00387
Grab, D. J., Garcia-Garcia, J. C., Nikolskaia, O. V., Kim, Y. V., Brown, A., Pardo, C. A., et al. (2009). Protease activated receptor signaling is required for African trypanosome traversal of human brain microvascular endothelial cells. PLoS Negl. Trop. Dis. 3:e479. doi: 10.1371/journal.pntd.0000479
Graham, E. L., Clark, J. R., Orban, Z. S., Lim, P. H., Szymanski, A. L., Taylor, C., et al. (2021). Persistent neurologic symptoms and cognitive dysfunction in non-hospitalized Covid-19 “long haulers”. Ann. Clin. Transl. Neurol. 8, 1073–1085. doi: 10.1002/acn3.51350
Gross, P. M., and Weindl, A. (1987). Peering through the windows of the brain. J. Cereb. Blood Flow Metabol. 7, 662–671. doi: 10.1038/jcbfm.1987.120
Hanisch, U. K., and Kettenmann, H. (2007). Microglia: Active sensor and versatile effector cells in the normal and pathologic brain. Nat. Neurosci. 10, 1387–1394. doi: 10.1038/nn1997
Hashimoto, M., Tawaratsumida, K., Kariya, H., Kiyohara, A., Suda, Y., Krikae, F., et al. (2006). Not lipoteichoic acid but lipoproteins appear to be the dominant immunobiologically active compounds in Staphylococcus aureus. J. Immunol. 177, 3162–3169. doi: 10.4049/jimmunol.177.5.3162
Hawkins, B. T., and Davis, T. P. (2005). The blood-brain barrier/neurovascular unit in health and disease. Pharmacol. Rev. 57, 173–185. doi: 10.1124/pr.57.2.4
Hawkins, R. A. (2009). The blood-brain barrier and glutamate. Am. J. Clin. Nutr. 90, 867S–874S. doi: 10.3945/ajcn.2009.27462BB
Heiss, C. N., Gravert, E., Hultén, M., and Olofsson, L. E. (2022). MyD88 deficiency, but not gut microbiota depletion, is sufficient to modulate the blood–brain barrier function in the mediobasal hypothalamus. Mol. Neurobiol. 59, 3755–3766. doi: 10.1007/s12035-022-02802-w
Henry, C. J., Huang, Y., Wynne, A., Hanke, M., Himler, J., Bailey, M. T., et al. (2008). Minocycline attenuates lipopolysaccharide (LPS)-induced neuroinflammation, sickness behavior, and anhedonia. J. Neuroinflamm. 5:15. doi: 10.1186/1742-2094-5-15
Henry, R. J., Ritzel, R. M., Barrett, J. P., Doran, S. J., Jiao, Y., Leach, J. B., et al. (2020). Microglial depletion with CSF1R Inhibitor during chronic phase of experimental traumatic brain injury reduces neurodegeneration and neurological deficits. J. Neurosci. 40, 2960–2974. doi: 10.1523/JNEUROSCI.2402-19.2020
Hiyama, T.-H., Yoshida, M., Matsumoto, M., Suzuki, R., Matsuda, T., Watanabe, E., et al. (2013). Endotheli-3 expression in the subfornical organ enhances the sensitivity of Nax, the brain sodium-level sensor, to suppress salt intake. Cell Metabol. 17, 507–519. doi: 10.1016/j.cmet.2013.02.018
Hiyama, T.-Y., Watanabe, E., Okado, H., and Noda, M. (2004). The subfornical organ is the primary locus of sodium-level sensing by Na(x) sodium channels for the control of salt-intake behavior. J. Neurosci. 24, 9276–9281. doi: 10.1523/JNEUROSCI.2795-04.2004
Hiyama, T.-Y., Watanabe, E., Ono, K., Inenaga, K., Tamkun, M. M., Yoshida, S., et al. (2002). Nax channel in CNS sodim-level sensing. Nat. Neurosci. 5, 511–512. doi: 10.1038/nn0602-856
Hofer, H. O. (1987). The terminal organ of the subcommissural complex of chordates: Definition and perspectives. Gegenbaurs Morphol. Jahrb. 133, 217–226.
Hou, B., Jiang, C., Wang, D., Wang, G., Wang, Z., and Zhu, M. (2020). Pharmacological targeting of CSF1R inhibits microglial proliferation and aggravates the progression of cerebral ischemic pathology. Front. Cell Neurosci. 14:267. doi: 10.3389/fncel.2020.00267
Hourai, A., and Miyata, S. (2013). Neurogenesis in the circumventricular organs of adult mouse brains. J. Neurosci. Res. 91, 757–770. doi: 10.1002/jnr.23206
Hrvatin, S., Sun, S., Wilcox, O. F., Yao, H., Lavin-Peter, A. J., Cicconet, M., et al. (2020). Neurons that regulate mouse torpor. Nature 583, 115–121. doi: 10.1038/s41586-020-2387-5
Huet, G., Richet, C., Demeyer, D., Bisiau, H., Soudan, B., Tetaert, D., et al. (2009). Characterization of different proteolytic activities in Trypanosoma brucei brucei. Biochim. Biophys. Acta. 1138, 213–221. doi: 10.1016/0925-4439(92)90040-t
Iida, T., Shimizu, I., Nealen, M. L., Campbell, A., and Caterina, M. (2005). Attenuated fever response in mice lacking TRPV1. Neurosci. Lett. 378, 28–33. doi: 10.1016/j.neulet.2004.12.007
Ilanges, A., Shiao, R., Shaked, J., Luo, J. D., Yu, X., and Friedman, J. M. (2022). Brainstem ADCYAP1+ neurons control multiple aspects of sickness behaviour. Nature 609, 761–771. doi: 10.1038/s41586-022-05161-7
Inagaki, H., Kurganov, E., Park, Y., Furube, E., and Miyata, S. (2019). Oral gavage of capsaicin causes TRPV1-dependent acute hypothermia and TRPV1-independent long-lasting increase of locomotor activity in the mouse. Physiol. Behav. 206, 213–224. doi: 10.1016/j.physbeh.2019.04.015
Jin, S., Kim, J. G., Park, J. W., Koch, M., Horvath, T. L., Lee, B. J., et al. (2016). Hypothalamic TLR2 triggers sickness behavior via a microglia-neuronal axis. Sci. Rep. 6:29424. doi: 10.1038/srep29424
Johansson, P. A. (2014). The choroid plexuses and their impact on developmental neurogenesis. Front. Neurosci. 8:340. doi: 10.3389/fnins.2014.00340
Johnson, A. K., and Gross, P. M. (1993). Sensory circumventricular organs and brain homeostatic pathways. FASEB J. 7, 678–686. doi: 10.1096/fasebj.7.8.8500693
Julius, D. (2013). TRP channels and pain. Annu. Rev. Cell Dev. Biol. 29, 355–384. doi: 10.1146/annurev-cellbio-101011-155833
Kasuga, R., Shiraki, C., Horikawa, R., Yoshimura, R., Kurganov, E., and Miyata, S. (2022). Role of TRPM8 in cold avoidance behaviors and brain activation during innocuous and nocuous cold stimuli. Physiol. Behav. 248:113729. doi: 10.1016/j.physbeh.2022.113729
Katsuno, T., Umeda, K., Matsui, T., Hata, M., Tamura, A., Itoh, M., et al. (2008). Deficiency of zonula occludens-1 causes embryonic lethal phenotype associated with defected yolk sac angiogenesis and apoptosis of embryonic cells. Mol. Biol. Cell 19, 2465–2475. doi: 10.1091/mbc.e07-12-1215
Kaur, C., Singh, J., Lim, M. K., Ng, B. L., and Ling, E. A. (1997). Macrophages/microglia as ‘sensors’ of injury in the pineal gland of rats following a non-penetrative blast. Neurosci. Res. 27, 317–322. doi: 10.1016/s0168-0102(97)01164-4
Kawai, T., and Akira, S. (2010). The role of pattern-recognition receptors in innate immunity: Update on Toll-like receptors. Nat. Immunol. 11, 373–384. doi: 10.1038/ni.1863
Kettenmann, H., Hanisch, U. K., Noda, M., and Verkhratsky, A. (2011). Physiology of microglia. Physiol. Rev. 91, 461–553. doi: 10.1152/physrev.00011.2010
Kinsman, B., Cowles, J., Lay, J., Simmonds, S. S., Browning, K. N., and Stocker, S. D. (2014). Osmoregulatory thirst in mice lacking the transient receptor potential vanilloid type 1 (TRPV1) and/or type 4 (TRPV4) receptor. Am. J. Physiol. 307, R1092–R1100. doi: 10.1152/ajpregu.00102.2014
Knoll, J. G., Krasnow, S. M., and Marks, D. L. (2017). Interleukin-1B signaling in fenestrated capillaries is sufficient to trigger sickness responses in mice. J. Neuroinflamm. 14:219. doi: 10.1186/s12974-017-0990-7
Knorr, C., Hübschle, T., Murgott, J., Mühlradt, P., Gerstberger, R., and Roth, J. (2008). Macrophage-activating lipopeptide-2 (MALP-2) induces a localized inflammatory response in rats resulting in activation of brain sites implicated in fever. Brain Res. 1205, 36–46. doi: 10.1016/j.brainres.2008.02.021
Komarova, Y., and Malik, A. B. (2010). Regulation of endothelial permeability via paracellular and transcellular transport pathways. Annu. Rev. Physiol. 72, 463–493. doi: 10.1146/annurev-physiol-021909-135833
Konsman, J. P., and Dantzer, R. (1999). Temporal and spatial relationships between lipopolysaccharide-induced expression of fos, interleukin-1 β and inducible nitric oxide synthase in rat brain. Neuroscience 89, 535–548. doi: 10.1016/s0306-4522(98)00368-6
Korim, K. S., Elsaafien, K., Basser, J. R., Setiadi, A., May, C. N., and Yao, S. T. (2019). In renovascular hypertension, TNF-α type-1 receptors in the area postrema mediate increases in cardiac and renal sympathetic nerve activity and blood pressure. Cardiovasc. Res. 115, 1092–1101. doi: 10.1093/cvr/cvy268
Kristensson, K., Masocha, W., and Bentivoglio, M. (2013). Mechanisms of CNS invasion and damage by parasites. Handb. Clin. Neurol. 114, 11–22. doi: 10.1016/B978-0-444-53490-3.00002-9
Lacroix, S., Feinstein, D., and Rivest, S. (1998). The bacteria endotoxin lipopolysaccharide has the ability to target the brain in upregulating its membrane CD14 receptor within specific cellular populations. Brain Pathol. 8, 625–640. doi: 10.1111/j.1750-3639.1998.tb00189.x
Laflamme, N., and Rivest, S. (2001). Toll-like receptor 4: The missing link of the cerebral innate immune response triggered by circulating gram-negative bacterial cell wall components. FASEB J. 15, 155–163. doi: 10.1096/fj.00-0339com
Laflamme, N., Lacroix, S., and Rivest, S. (1999). An essential role of interleukin-1b in mediating NF-κB activity and COX-2 transcription in cells of the blood–brain barrier in response to a systemic and localized inflammation but not during endotoxemia. J. Neurosci. 19, 10923–10930. doi: 10.1523/JNEUROSCI.19-24-10923.1999
Laflamme, N., Soucy, G., and Rivest, S. (2001). Circulating cell wall components derived from gram-negative, not gram-positive, bacteria cause a profound induction of the gene-encoding Toll-like receptor 2 in the CNS. J. Neurochem. 79, 648–657. doi: 10.1046/j.1471-4159.2001.00603.x
Langlet, F., Mullier, A., Bouret, S. G., Prevot, V., and Dehouck, B. (2013). Tanycyte-like cells form a blood-cerebrospinal fluid barrier in the circumventricular organs of the mouse brain. J. Comp. Neurol. 521, 3389–3405. doi: 10.1002/cne.23355
Lapenna, A., De Palma, M., and Lewis, C. E. (2018). Perivascular macrophages in health and disease. Nat. Rev. Immunol. 18, 689–702. doi: 10.1038/s41577-018-0056-9
Lee, D. A., Bedont, J. L., Pak, T., Wang, H., Song, J., and Miranda-Angulo, A. (2012). Tanycytes of the hypothalamic median eminence form a diet-responsive neurogenic niche. Nat. Neurosci. 15, 700–702. doi: 10.1038/nn.3079
Lemkey-Johnston, N., and Reynolds, W. A. (1974). Nature and extent of brain lesions in mice related to ingestion of monosodium glutamate. J. Neuropath. Exp. Neurol. 33, 74–97. doi: 10.1097/00005072-197401000-00006
Li, L., Acioglu, C., Heary, R. F., and Elkabes, S. (2021). Role of astroglial toll-like receptors (TLRs) in central nervous system infections, injury and neurodegenerative diseases. Brain Behav. Immun. 91, 740–755. doi: 10.1016/j.bbi.2020.10.007
Luo, X. J., Peng, J., and Li, Y. J. (2011). Recent advances in the study on capsaicinoids and capsinoids. Eur. J. Pharmacol. 650, 1–7. doi: 10.1016/j.ejphar.2010.09.074
Mallmann, M. P., Mello, F. K., Neuberger, B., da Costa Sobral, K. G., Fighera, M. R., Royes, L. F. F., et al. (2022). Beta-caryophyllene attenuates short-term recurrent seizure activity and blood-brain-barrier breakdown after pilocarpine-induced status epilepticus in rats. Brain Res. 1784:147883. doi: 10.1016/j.brainres.2022.147883
Mannari, T., Morita, S., Furube, E., Tominaga, M., and Miyata, S. (2013). Astrocytic TRPV1 ion channels detect blood-borne signals in the sensory circumventricular organs of adult mouse brains. Glia 61, 957–971. doi: 10.1002/glia.22488
Mantovani, A., Sica, A., Sozzani, S., Allavena, P., Vecchi, A., Locati, M., et al. (2004). The chemokine system in diverse forms of macrophage activation and polarization. Trends Immunol. 25, 677–686. doi: 10.1016/j.it.2004.09.015
Matsumura, K., Watanabe, Y., Onoe, H., Watanabe, Y., and Hayaishi, O. (1990). High density of prostaglandin E2 binding sites in the anterior wall of the 3rd ventricle: A possible site of its hyperthermic action. Brain Res. 533, 147–151. doi: 10.1016/0006-8993(90)91808-t
McKinley, M. J., Denton, D. A., Ryan, P. J., Yao, S. T., Stefanidis, A., and Oldfield, B. J. (2019). From sensory circumventricular organs to cerebral cortex: Neural pathways controlling thirst and hunger. J. Neuroendocrinol. 31:e12689. doi: 10.1111/jne.12689
McKinley, M. J., McAllen, R. M., Davern, P., Giles, M. E., Penschow, J., Sunn, N., et al. (2003). The sensory circumventricular organs of the mammalian brain. Adv. Anat. Embryol. Cell Biol. 172, III–XII, 1–122. doi: 10.1007/978-3-642-55532-9
Mehta, D., and Malik, A. B. (2006). Signaling mechanisms regulating endothelial permeability. Physiol. Rev. 86, 279–367. doi: 10.1152/physrev.00012.2005
Meletis, K., Barnabé-Heider, F., Carlén, M., Evergren, E., Tomilin, N., Shupliakov, O., et al. (2008). Spinal cord injury reveals multilineage differentiation of ependymal cells. PLoS Biol. 6:e182. doi: 10.1371/journal.pbio.0060182
Michels, M., Ávila, P., Pescador, B., Vieira, A., Abatti, M., Cucker, L., et al. (2019). Microglial cells depletion increases inflammation and modifies microglial phenotypes in an animal model of severe sepsis. Mol. Neurobiol. 56, 7296–7304. doi: 10.1007/s12035-019-1606-2
Miller, A. D., and Leslie, R. A. (1994). The area postrema and vomiting. Front. Neuroendocrinol. 15:301–320. doi: 10.1006/frne.1994.1012
Mimee, A., Smith, P. M., and Ferguson, A. V. (2013). Circumventricular organs: Targets for integration of circulating fluid and energy balance signals? Physiol. Behav. 121, 96–102. doi: 10.1016/j.physbeh.2013.02.012
Miner, J. H. (2012). The glomerular basement membrane. Exp. Cell Res. 318, 973–978. doi: 10.1016/j.yexcr.2012.02.031
Miyata, S. (2015). New aspects in fenestrated capillary and tissue dynamics in the sensory circumventricular organs of adult brains. Front. Neurosci. 9:390. doi: 10.3389/fnins.2015.00390
Miyata, S. (2017). Advances in understanding of structural reorganization in the hypothalamic neurosecretory system. Front. Endocrinol. 8:275. doi: 10.3389/fendo.2017.00275
Miyata, S., and Hatton, G. I. (2002). Activity-related, dynamic neuron-glial interactions in the hypothalamo-neurohypophysial system. Microsc. Res. Tech. 56, 143–157. doi: 10.1002/jemt.10012
Miyata, S., and Morita, S. (2011). A new method for visualization of endothelial cells and extravascular leakage in adult mouse brain using fluorescein isothiocyanate. J. Neurosci. Methods 202, 9–16. doi: 10.1016/j.jneumeth.2011.08.002
Miyata, S., Matsunaga, W., Mondoh, H., Nakashima, T., and Kiyohara, T. (1996). Effect of AV3V lesions on Fos expression and cell size increases in magnocellular neurons of the rat hypothalamus during chronic dehydration. Neurosci. Res. 26, 149–156. doi: 10.1016/s0168-0102(96)01099-1
Møller, M., Rath, M. F., and Klein, D. C. (2006). The perivascular phagocyte of the mouse pineal gland: An antigen-presenting cell. Chronobiol. Int. 23, 393–401. doi: 10.1080/07420520500521855
Morita, K., Sasaki, H., Furuse, M., and Tsukita, S. (1999). Endothelial claudin: Claudin-5/TMVCF constitutes tight junction strands in endothelial cells. J. Cell Biol. 147, 185–194. doi: 10.1083/jcb.147.1.185
Morita, S., and Miyata, S. (2012). Different vascular permeability between the sensory and secretory circumventricular organs of adult mouse brain. Cell Tissue Res. 349, 589–603. doi: 10.1007/s00441-012-1421-9
Morita, S., and Miyata, S. (2013). Accessibility of low-molecular-mass molecules to the median eminence and arcuate hypothalamic nucleus of adult mouse. Cell Biochem. Funct. 31, 668–677. doi: 10.1002/cbf.2953
Morita, S., Furube, E., Mannari, T., Okuda, H., Tatsumi, K., Wanaka, A., et al. (2015). Vascular endothelial growth factor-dependent angiogenesis and dynamic vascular plasticity in the sensory circumventricular organs of adult mouse brain. Cell Tissue Res. 359, 865–884. doi: 10.1007/s00441-014-2080-9
Morita, S., Furube, E., Mannari, T., Okuda, H., Tatsumi, K., Wanaka, A., et al. (2016). Heterogeneous vascular permeability and alternative diffusion barrier in sensory circumventricular organs of adult mouse brain. Cell Tissue Res. 363, 497–511. doi: 10.1007/s00441-015-2207-7
Morita, S., Ukai, S., and Miyata, S. (2013). VEGF-dependent continuous angiogenesis in the median eminence of adult mice. Eur. J. Neurosci. 37, 508–518. doi: 10.1111/ejn.12047
Morris, M. J., Rocha, L. J., Sim, A. K., and Johnson, M. F. (1994). Callahan dissociation between vasopressin and oxytocin mRNA and peptide secretion after AV3V lesions. Am. J. Physiol. 267, R1640–R1645. doi: 10.1152/ajpregu.1994.267.6.R1640
Müller-Fielitz, H., Stahr, M., Bernau, M., Richter, M., Abele, S., Krajka, V., et al. (2017). Tanycytes control the hormonal output of the hypothalamic-pituitary-thyroid axis. Nat. Commun. 8:484. doi: 10.1038/s41467-017-00604-6
Mullier, A., Bouret, S. G., Prevot, V., and Dehouck, B. (2010). Differential distribution of tight junction proteins suggests a role for tanycytes in blood-hypothalamus barrier regulation in the adult mouse brain. J. Comp. Neurol. 518, 943–962. doi: 10.1002/cne.22273
Muneoka, S., Murayama, S., Nakano, Y., and Miyata, S. (2019). TLR4 in circumventricular neural stem cells is a negative regulator for thermogenic pathways in the mouse brain. J. Neuroimmunol. 331, 58–73. doi: 10.1016/j.jneuroim.2018.04.017
Murayama, S., Kurganov, E., and Miyata, S. (2019). Activation of microglia and macrophages in the circumventricular organs of the mouse brain during TLR2-induced fever and sickness responses. J. Neuroimmunol. 334:576973. doi: 10.1016/j.jneuroim.2019.576973
Murray, P. J. (2017). Macrophage Polarization. Ann. Rev. Physiol. 79, 541–566. doi: 10.1146/annurev-physiol-022516-034339
Nadeau, S., and Rivest, S. (2000). Role of microglial-derived tumor necrosis factor in mediating CD14 transcription and nuclear factor k B activity in the brain during endotoxemia. J. Neurosci. 20, 3456–3468. doi: 10.1523/JNEUROSCI.20-09-03456.2000
Nakano, Y., Furube, E., Morita, S., Wanaka, A., Nakashima, T., and Miyata, S. (2015). Astrocytic TLR4 expression and LPS-induced nuclear translocation of STAT3 in the sensory circumventricular organs of adult mouse brain. J. Neuroimmunol. 278, 144–158. doi: 10.1016/j.jneuroim.2014.12.013
Nambu, Y., Horie, K., Kurganov, E., and Miyata, S. (2021a). Chronic running and a corticosterone treatment attenuate astrocyte-like neural stem cell proliferation in the area postrema of the adult mouse brain. Neurosci. Lett. 748:135732. doi: 10.1016/j.neulet.2021.135732
Nambu, Y., Ohira, K., Morita, M., Yasumoto, H., Kurganov, E., and Miyata, S. (2021b). Effects of leptin on proliferation of astrocyte- and tanycyte-like neural stem cells in the adult mouse medulla oblongata. Neurosci. Res. 173, 44–53. doi: 10.1016/j.neures.2021.05.012
Nilsberth, C., Elander, L., Hamzic, N., Norell, M., Lönn, J., Engström, L., et al. (2009). The role of interleukin-6 in lipopolysaccharide-induced fever by mechanisms independent of prostaglandin E2. Endocrinology 150, 1850–1860. doi: 10.1210/en.2008-0806
Nishihara, E., Hiyama, T.-Y., and Noda, M. (2011). Osmosensitivity of transient receptor potential vanilloid 1 is synergistically enhanced by distinct activating stimuli such as temperature and protons. PLoS One 6:e22246. doi: 10.1371/journal.pone.0022246
Nishikawa, K., Furube, E., Morita, S., Horii-Hayashi, N., Nishi, M., and Miyata, S. (2017). Structural reconstruction of the perivascular space in the adult mouse neurohypophysis during an osmotic stimulation. J. Neuroendocrinol. 29:2. doi: 10.1111/jne.12456
Noda, M. (2006). The subfornical organ, a specialized sodium channel, and the sensing of sodium levels in the brain. Neuroscientist 12, 80–91. doi: 10.1177/1073858405279683
Noda, M. (2007). Hydromineral neuroendocrinology: Mechanism of sensing sodium levels in the mammalian brain. Exp. Physiol. 92, 513–522. doi: 10.1113/expphysiol.2006.035659
Noda, M., and Hiyama, T. (2015). Sodium sensing in the brain. Eur. J. Physiol. 467, 465–474. doi: 10.1007/s00424-014-1662-4
Obermeier, B., Daneman, R., and Ransohoff, R. M. (2013). Development, maintenance and disruption of the blood-brain barrier. Nat. Med. 19, 1584–1596. doi: 10.1038/nm.3407
Ohtaka-Maruyama, C., and Okado, H. (2015). Molecular pathways underlying projection neuron production and migration during cerebral cortical development. Front. Neurosci. 9:447. doi: 10.3389/fnins.2015.00447
Okamoto, A., Fujii, R., Yoshimura, R., and Miyata, S. (2022). Transcytosis of tanycytes in the circumventricular organs of adult mouse. Neurosci. Lett. 779:136633. doi: 10.1016/j.neulet.2022.136633
Oliveira, G. R., Franci, C. R., Rodovalho, G. V., Franci, J. A., Morris, M., Rocha, M. J., et al. (2004). Alterations in the central vasopressin and oxytocin axis after lesion of a brain osmotic sensory region. Brain Res. Bull. 63, 515–520. doi: 10.1016/j.brainresbull.2004.04.009
Oliveira-Nascimento, L., Massari, P., and Wetzler, L. M. (2012). The role of TLR2 in infection and immunity. Front. Immunol. 3:79. doi: 10.3389/fimmu.2012.00079
Olmos-Alonso, A., Schetters, S. T., Sri, S., Askew, K., Mancuso, R., Vargas-Caballero, M., et al. (2016). Pharmacological targeting of CSF1R inhibits microglial proliferation and prevents the progression of Alzheimer’s-like pathology. Brain 139, 891–907. doi: 10.1093/brain/awv379
Ong, W. Y., Satish, R. L., and Herr, D. R. (2022). ACE2, circumventricular oragns and the hypothalamus, and COVID-19. NeuroMolecular Med. 22, 1–11. doi: 10.1007/s12017-022-08706-1
Paolicelli, R., Bolasco, G., Pagani, F., Maggi, L., Scianni, M., Panzanelli, M., et al. (2011). Synaptic pruning by microglia is necessary for normal brain development. Science 333, 1456–1458. doi: 10.1126/science.1202529
Paricio-Montesinos, R., Schwaller, F., Udhayachandran, A., Rau, F., Walcher, J., Evangelista, R., et al. (2020). The sensory coding of warm perception. Neuron 106, 830–841. doi: 10.1016/j.neuron.2020.02.035
Park, Y., Miyata, S., and Kurganov, E. (2020). TRPV1 is crucial for thermal homeostasis in the mouse by heat loss behaviors under warm ambient temperature. Sci. Rep. 10:8799. doi: 10.1038/s41598-020-65703-9
Pogorzala, L. A., Mishra, S. K., and Hoon, M. A. (2013). The cellular code for mammalian thermosensation. J. Neurosci. 33, 5533–5541. doi: 10.1523/JNEUROSCI.5788-12.2013
Prager-Khoutorsky, M., and Bourque, C. W. (2015). Anatomical organization of the rat organum vasculosum laminae terminalis. Am. J. Physiol. 309, R324–R337. doi: 10.1152/ajpregu.00134.2015
Prevot, V., Bellefontaine, N., Baroncini, M., Sharif, A., Hanchate, N. K., Parkash, J., et al. (2010). Gonadotrophin-releasing hormone nerve terminals, tanycytes and neurohaemal junction remodelling in the adult median eminence: Functional consequences for reproduction and dynamic role of vascular endothelial cells. J. Neuroendocrinol. 22, 639–649. doi: 10.1111/j.1365-2826.2010.02033.x
Price, C. J., Hoyda, T. D., and Ferguson, A. V. (2008). The area postrema: A brain monitor and integrator of systemic autonomic state. Neuroscientist 14, 182–194. doi: 10.1177/1073858407311100
Price, M. T., Olney, J. W., Lowry, O. H., and Buchsbaum, S. (1981). Uptake of exogenous glutamate and aspartate by circumventricular organs but not other regions of brain. J. Neurochem. 36, 1774–1780. doi: 10.1111/j.1471-4159.1981.tb00430.x
Quan, N., Whiteside, M., Kim, L., and Herkenham, M. (1997). Induction of inhibitory factor kappa B alpha mRNA in the central nervous system after peripheral lipopolysaccharide administration: An in situ hybridization histochemistry study in the rat. Proc. Natl. Acad. Sci. U.S.A. 94, 10985–10990. doi: 10.1073/pnas.94.20.10985
Randsohoff, R. M. (2016). A polarizing question: Do M1 and M2 microglia exist? Nat. Neurosci. 19, 987–991. doi: 10.1038/nn.4338
Reyes-Escogido Mde, L., Gonzalez-Mondragon, E. G., and Vazquez-Tzompantzi, E. (2011). Chemical and pharmacological aspects of capsaicin. Molecules 16, 1253–1270. doi: 10.3390/molecules16021253
Riediger, T. (2012). The receptive function of hypothalamic and brainstem centres to hormonal and nutrient signals affecting energy balance. Proc. Nutr. Soc. 71, 463–477. doi: 10.1017/S0029665112000778
Rivest, S. (2009). Regulation of innate immune responses in the brain. Nat. Rev. Immunol. 9, 429–439. doi: 10.1038/nri2565
Rivest, V. (2003). Molecular insights on the cerebral innate immune system. Brain Behav. Immun. 17, 13–19. doi: 10.1016/s0889-1591(02)00055-7
Robins, S. C., Stewart, I., McNay, D. E., Taylor, V., Giachino, C., and Goetz, M. (2013). α-Tanycytes of the adult hypothalamic third ventricle include distinct populations of FGF-responsive neural progenitors. Nat. Commun. 4:2049. doi: 10.1038/ncomms3049
Rocha, M. J., Beltz, T. G., Dornelles, R. C., Johnson, A. K., and Franci, C. R. (1999). Anteroventral third ventricle (AV3V) lesions alter c-fos expression induced by salt loading. Brain Res. 829, 197–200. doi: 10.1016/s0006-8993(99)01366-9
Rodríguez, E. M., Blázquez, J. L., and Guerraa, M. (2010). The design of barriers in the hypothalamus allows the median eminence and the arcuate nucleus to enjoy private milieus: The former opens to the portal blood and the latter to the cerebrospinal fluid. Peptides 31, 757–776. doi: 10.1016/j.peptides.2010.01.003
Rodríguez, E. M., Blázquez, J. L., Pastor, F. E., Peláez, B., Peña, P., Peruzzo, B., et al. (2005). Hypothalamic tanycytes: A key component of brain-endocrine interaction. Int. Rev. Cytol. 247, 89–164.
Rodríguez, E. M., González, C. B., and Delannoy, L. (1979). Cellular organization of the lateral and postinfundibular regions of the median eminence in the rat. Cell Tissue Res. 201, 377–408. doi: 10.1007/BF00236998
Rodríguez, E. M., Guerra, M., Peruzzo, B., and Blázquez, J. L. (2019). Tanycytes: A rich morphological history to underpin future molecular and physiological investigations. J. Neuroendocrinol. 2019:e12690. doi: 10.1111/jne.12690
Rogers, R. C., McTigue, D. M., and Hermann, G. E. (1996). Vagal control of digestion: Modulation by central neural and peripheral endocrine factors. Neurosci. Biobehav. Rev. 20, 57–66. doi: 10.1016/0149-7634(95)00040-l
Romanovsky, A. A., Almeida, M. C., Garami, A., Steiner, A. A., Norman, M. H., Morrison, S. F., et al. (2009). The transient receptor potential vanilloid-1 channel in thermoregulation: A thermosensor it is not. Pharmacol. Rev. 61, 228–261. doi: 10.1124/pr.109.001263
Romanovsky, A. J., Sugimoto, N., Simons, C. T., Hunter, W. S., et al. (2003). The organum vasculosum laminae terminalis in immune-to-brain febrigenic signaling: A reappraisal of lesion experiments. Am. J. Physiol. 285, R420–R428. doi: 10.1152/ajpregu.00757.2002
Roth, J., Harré, E.-M., Rummel, C., Gerstberger, R., and Hübschle, T. (2004). Signaling the brain in systemic inflammation: Role of sensory circumventricular organs. Front. Biosci. 9:290–300. doi: 10.2741/1241
Roth, J., Rummel, C., Barth, S. W., Gerstberger, R., and Hübschle, T. (2009). Molecular aspects of fever and hyperthermia. Immunol. Allergy Clin. North Am. 29, 229–245. doi: 10.1016/j.iac.2009.02.005
Rubbin, L. L., and Staddon, J. M. (1999). The cell biology of the blood-brain barrier. Annu. Rev. Neurosci. 22, 11–28. doi: 10.1146/annurev.neuro.22.1.11
Rummel, C., Hübschle, T., Gerstberger, R., and Roth, J. (2004). Nuclear translocation of the transcription factor STAT3 in the guinea pig brain during systemic or localized inflammation. J. Physiol. 557, 671–687. doi: 10.1113/jphysiol.2003.058834
Rummel, C., Voss, T., Matsumura, K., Korte, S., Gerstberger, R., Roth, J., et al. (2005). Nuclear STAT3 translocation in guinea pig and rat brain endothelium during systemic challenge with lipopolysaccharide and interleukin-6. J. Comp. Neurol. 491, 1–14. doi: 10.1002/cne.20653
Serrats, J., Schiltz, J. C., Garcia-Bueno, B., van Rooijen, N., Reyes, T. M., and Sawchenko, P. E. (2010). Dual roles for perivascular macrophages in immune-to-brain signaling. Neuron 65, 94–106. doi: 10.1016/j.neuron.2009.11.032
Shigemoto-Mogami, Y., Hoshikawa, K., Goldman, J. E., Sekino, Y., and Sato, K. (2014). Microglia enhance neurogenesis and oligodendrogenesis in the early postnatal subventricular zone. J. Neurosci. 34, 2231–2243. doi: 10.1523/JNEUROSCI.1619-13.2014
Shimizu, H., Watanabe, E., Hiyama, T.-Y., Nagakura, A., Fujikawa, A., Okado, H., et al. (2007). Glial Nax channels control lactate signaling to neurons for brain [Na+] sensing. Neuron 54, 59–72. doi: 10.1016/j.neuron.2007.03.014
Shiraki, C., Horikawa, R., Oe, Y., Fujimoto, M., Okamoto, K., Kurganov, E., et al. (2021). Role of TRPM8 in switching between fever and hypothermia in adult mice during endotoxin-induced inflammation. Brain Behav. Immun. Health 16:e100291. doi: 10.1016/j.bbih.2021.100291
Silva-Vargas, V., Maldonado-Soto, A. R., Mizrak, D., Codega, P., and Doetsch, F. (2016). Age-dependent niche signals from the choroid plexus regulate adult neural stem cells. Cell Stem Cell 19, 643–652. doi: 10.1016/j.stem.2016.06.013
Sindona, C., Schepici, G., Contestabile, V., Bramanti, P., and Mazzon, E. (2021). NOX2 activation in COVID-19: Possible implications for neurodegenerative diseases. Medicina 57:604. doi: 10.3390/medicina57060604
Sisó, S., Jeffrey, M., and González, L. (2010a). Sensory circumventricular organs in health and disease. Acta. Neuropathol. 120, 689–705. doi: 10.1007/s00401-010-0743-5
Sisó, S., González, L., and Jeffrey, M. (2010b). Neuroinvasion in prion diseases: The role of ascending neural infection and blood dissemination. Interdiscip. Perspect. Infect. Dis. 2010:747892. doi: 10.1155/2010/747892
Sladek, C. D., and Johnson, A. K. (2013). Integration of thermal and osmotic regulation of water homeostasis: The role of TRPV channels. Am. J. Physiol. 305, R669–R678. doi: 10.1152/ajpregu.00270.2013
Stegink, L. D., Filer, L. J. Jr., and Baker, G. L. (1982). Plasma and erythrocyte amino acid levels in normal adult subjects fed a high protein meal with and without added monosodium glutamate. J. Nutr. 112, 1953–1960. doi: 10.1093/jn/112.10.1953
Stitt, J. T. (1985). Evidence for the involvement of the organum vasculosum laminae terminalis in the febrile response of rabbits and rats. J. Physiol. 368, 501–511. doi: 10.1113/jphysiol.1985.sp015872
Szelenyi, Z., Hummel, Z., Szolcsanyi, J., and Davis, J. B. (2004). Daily body temperature rhythm and heat tolerance in TRPV1 knockout and capsaicin pretreated mice. Eur. J. Neurosci. 19, 1421–1424. doi: 10.1111/j.1460-9568.2004.03221.x
Takagi, S., Furube, E., Nakano, Y., Morita, M., and Miyata, S. (2017). Microglia are continuously activated in the circumventricular organs of mouse brain. J. Neuroimmunol. 331, 74–86. doi: 10.1016/j.jneuroim.2017.10.008
Takagi, S., Murayama, S., Torii, K., Takemura-Morita, S., Kurganov, E., Nagaoka, S., et al. (2020). Depletion of microglia and macrophages with clodronate liposomes attenuates zymosan-induced Fos expression and hypothermia in the adult mouse. J. Neuroimmunol. 344:577244. doi: 10.1016/j.jneuroim.2020.577244
Takahashi, T.-M., Sunagawa, G.-A., Soya, S., Abe, M., Sakurai, K., Ishikawa, K., et al. (2020). A discrete neuroal circuit induces a hibernation-like state in rodents. Nature 583, 109–114. doi: 10.1038/s41586-020-2163-6
Takahashi, Y., Smith, P., Ferguson, A., and Pittman, Q. J. (1997). Circumventricular organs and fever. Am. J. Physiol. 273, R1690–R1695. doi: 10.1152/ajpregu.1997.273.5.R1690
Takeuchi, O., Hoshino, K., and Akira, S. (2000). Cutting edge: TLR2-deficient and MyD88-deficient mice are highly susceptible to Staphylococcus aureus infection. J. Immunol. 165, 5392–5396. doi: 10.4049/jimmunol.165.10.5392
Taylor, A. C., McCarthy, J. J., and Stocker, S. D. (2008). Mice lacking the transient receptor vanilloid potential 1 channel display normal thirst responses and central Fos activation to hypernatremia. Am. J. Physiol. 294, R1285–R1293. doi: 10.1152/ajpregu.00003.2008
Tesoriero, C., Del Gallo, F., and Bentivoglio, M. (2018). Sleep and brain infections. Brain Res. Bull. 145, 59–74. doi: 10.1016/j.brainresbull.2018.07.002
Torii, K., Takagi, S., Yoshimura, R., and Miyata, S. (2022). Microglial proliferation attenuates sickness responses in adult mice during endotoxin-induced inflammation. J. Neuroimmunol. 365:577832. doi: 10.1016/j.jneuroim.2022.577832
Tsai, V. W. W., Husainim, Y., Sainsbury, A., Brown, D. A., and Breit, S. N. (2018). The MIC-1/GDF15-GFRAL pathway in energy homeostasis: Implications for obesity, cachexia, and other associated diseases. Cell Metab. 28, 353–368. doi: 10.1016/j.cmet.2018.07.018
Tsukita, S., Furuse, M., and Itoh, M. (2001). Multifunctional strands in tight junctions. Nat. Rev. Mol. Cell Biol. 2, 285–293. doi: 10.1038/35067088
Tucker, A. B., and Stocker, S. D. (2016). Hypernatremia-induced vasopressin secretion is not altered in TRPV1-/- rats. Am. J. Physiol. 311, R451–R456. doi: 10.1152/ajpregu.00483.2015
Vallieres, L., and Rivest, S. (1997). Regulation of the genes encoding interleukin-6, its receptor, and gp130 in the rat brain in response to the immune activator lipopolysaccharide and the proinflammatory cytokine interleukin-1beta. J. Neurochem. 69, 1668–1683. doi: 10.1046/j.1471-4159.1997.69041668.x
Vargas-Caraveo, A., Sayd, A., Maus, S. R., Caso, J. R., Madrigal, J. L. M., and García-Bueno, B. (2017). Lipopolysaccharide enters the rat brain by a lipoprotein-mediated transport mechanism in physiological conditions. Sci. Rep. 7:13113. doi: 10.1038/s41598-017-13302-6
Vargas-Caraveo, A., Sayd, A., Robledo-Montaña, J., Caso, J. R., Madrigal, J. L. M., García-Bueno, B., et al. (2020). Toll-like receptor 4 agonist and antagonist lipopolysaccharides modify innate immune response in rat brain circumventricular organs. J. Neuroinflam. 17:6. doi: 10.1186/s12974-019-1690-2
Vichaya, E. G., Malik, S., Sominsky, L., Ford, B. G., Spencer, S. J., and Dantzer, R. (2020). Microglia depleation fails to abrogate inflammation-induced sickness in mice and rats. J. Neuroinflamm. 17:172. doi: 10.1186/s12974-020-01832-2
Wang, N., Liang, H., and Zen, K. (2014). Molecular mechanisms that influence the macrophage m1-m2 polarization balance. Front. Immunol. 5:614. doi: 10.3389/fimmu.2014.00614
Wang, Y., Ge, P., and Zhu, Y. (2013). TLR2 and TLR4 in the brain injury caused by cerebral ischemia and reperfusion. Mediators Inflamm. 2013:124614. doi: 10.1155/2013/124614
Watanabe, E., Fujikawa, A., Matsunaga, H., Yasoshima, Y., Sako, N., Yamamoto, N., et al. (2000). Nav2/NaG channel is involved in control of salt-intake behavior in the CNS. J. Neurosci. 20, 7743–7751. doi: 10.1523/JNEUROSCI.20-20-07743.2000
Watanabe, E., Hiyama, T.-Y., Shimizu, H., Kodama, R., Hayashi, N., Miyata, S., et al. (2006). Sodium-level-sensitive sodium channel Nax is expressed in glial laminate processes in the sensory circumventricular organs. Am. J. Physiol. 290, R568–R576. doi: 10.1152/ajpregu.00618.2005
Welser, J. V., Li, L., and Milner, R. (2010). Microglial activation state exerts a biphasic influence on brain endothelial cell proliferation by regulating the balance of TNF and TGF-β1. J. Neuroinflamm. 7:89. doi: 10.1186/1742-2094-7-89
Willis, C. L., Garwood, C. J., and Ray, D. E. (2007). A size selective vascular barrier in the rat area postrema formed by perivascular macrophages and the extracellular matrix. Neuroscience 150, 498–509. doi: 10.1016/j.neuroscience.2007.09.023
Yang, A. C., Stevens, M. Y., Chen, M. B., Lee, D. P., Stähli, D., Gate, D., et al. (2020). Physiological blood-brain transport is impaired with age by a shift in transcytosis. Nature 583, 425–430. doi: 10.1038/s41586-020-2453-z
Yang, Y., Estrada, E. Y., Thompson, J. F., Liu, W., and Rosenberg, G. A. (2007). Matrix metalloproteinase-mediated disruption of tight junction proteins in cerebral vessels is reversed by synthetic matrix metalloproteinase inhibitor in focal ischemia in rat. J. Cereb. Blood Flow Metab. 27, 697–709. doi: 10.1038/sj.jcbfm.9600375
Yang, Y., Thompson, J. F., Taheri, S., Salayandia, V. M., McAvoy, T. A., Hill, J. W., et al. (2013). Early inhibition of MMP activity in ischemic rat brain promotes expression of tight junction proteins and angiogenesis during recovery. J. Cereb. Blood Flow Metab. 33, 1104–1114. doi: 10.1038/jcbfm.2013.56
Yoshida, A., Furube, E., Mannari, T., Takayama, Y., Kittaka, H., Tominaga, M., et al. (2016). TRPV1 is crucial for proinflammatory STAT3 signaling and thermoregulation-associated pathways in the brain during inflammation. Sci. Rep. 6:26088. doi: 10.1038/srep26088
Yuan, J., Ge, H., Liu, W., Zhu, H., Chen, Y., Zhang, X., et al. (2017). M2 microglia promotes neurogenesis and oligodendrogenesis from neural stem/progenitor cells via the PPARγ signaling pathway. Oncotarget. 8, 19855–19865. doi: 10.18632/oncotarget.15774
Yulyaningsih, E., Rudenko, I. A., Valdearcos, M., Dahlen, E., Vagena, E., Chan, A., et al. (2017). Acute lesioning and rapid repair of hypothalamic neurons outside the blood-brain barrier. Cell Rep. 19, 2257–2271. doi: 10.1016/j.celrep.2017.05.060
Zähringer, U., Lindner, B., Inamura, S., Heine, H., and Alexander, C. (2008). TLR2 - promiscuous or specific? A critical re-evaluation of a receptor expressing apparent broad specificity. Immunobiology 213, 205–224. doi: 10.1016/j.imbio.2008.02.005
Zhang, C., Kaye, J. A., Cai, Z., Wang, Y., Prescott, S. L., Liberles, S. D., et al. (2020). Area postrema cell types that mediates nausea-associated behaviors. Neuron 109, 461–472. doi: 10.1016/j.neuron.2020.11.010
Zhang, K. X., D’Souza, S., Upton, B. A., Kernodle, S., Vemaraju, S., Nayak, G., et al. (2020). Violet-light suppression of thermogenesisby opsin 5 hypothalamic neurons. Nature 585, 420–425. doi: 10.1038/s41586-020-2683-0
Zhang, Y., Kim, M. S., Jia, B., Yan, J., Zuniga-Hertz, J. P., Han, C., et al. (2017). Hypothalamic stem cells control aging speed partly through exosomal miRNAs. Nature 548, 52–57. doi: 10.1038/nature23282
Zhao, Y., Kuang, M., Li, J., Zhu, L., Jia, Z., Guo, X., et al. (2021). SARS-CoV-2 spike protein interacts with and activates TLR4. Cell Res. 31, 818–820. doi: 10.1038/s41422-021-00495-9
Keywords: brain, inflammation, microglia, macrophage, astrocyte, tanycyte, fluid homeostasis, body temperature
Citation: Miyata S (2022) Glial functions in the blood-brain communication at the circumventricular organs. Front. Neurosci. 16:991779. doi: 10.3389/fnins.2022.991779
Received: 12 July 2022; Accepted: 20 September 2022;
Published: 06 October 2022.
Edited by:
Olfa Masmoudi-Kouki, University Tunis El Manar, TunisiaReviewed by:
Malgorzata Burek, Julius Maximilian University of Würzburg, GermanyÁkos Menyhárt, University of Szeged, Hungary
Eszter Farkas, University of Szeged, Hungary
Copyright © 2022 Miyata. This is an open-access article distributed under the terms of the Creative Commons Attribution License (CC BY). The use, distribution or reproduction in other forums is permitted, provided the original author(s) and the copyright owner(s) are credited and that the original publication in this journal is cited, in accordance with accepted academic practice. No use, distribution or reproduction is permitted which does not comply with these terms.
*Correspondence: Seiji Miyata, smiyata@kit.ac.jp