- 1Department of Neurology, China-Japan Union Hospital of Jilin University, Changchun, China
- 2Beijing Institute of Brain Disorders, Beijing Advanced Innovation Center for Big Data-Based Precision Medicine, Capital Medical University, Beijing, China
- 3Advanced Innovation Center for Big Data-Based Precision Medicine, School of Biological Science and Medical Engineering, Beihang University, Beijing, China
- 4Department of Neurosurgery, Xuanwu Hospital, Capital Medical University, Beijing, China
Ischemic stroke is associated with increasing morbidity and has become the main cause of death and disability worldwide. Cerebral edema is a serious complication arising from ischemic stroke. It causes an increase in intracranial pressure, rapid deterioration of neurological symptoms, and formation of cerebral hernia, and is an important risk factor for adverse outcomes after stroke. To date, the detailed mechanism of cerebral edema after stroke remains unclear. This limits advances in prevention and treatment strategies as well as drug development. This review discusses the classification and pathological characteristics of cerebral edema, the possible relationship of the development of cerebral edema after ischemic stroke with aquaporin 4, the SUR1-TRPM4 channel, matrix metalloproteinase 9, microRNA, cerebral venous reflux, inflammatory reactions, and cerebral ischemia/reperfusion injury. It also summarizes research on new therapeutic drugs for post-stroke cerebral edema. Thus, this review provides a reference for further studies and for clinical treatment of cerebral edema after ischemic stroke.
Introduction
Stroke places a heavy burden on society and families due to its high morbidity, associated disability, and mortality. Ischemic stroke accounts for nearly 76% of all stroke cases (Virani et al., 2021; Deng et al., 2022). Malignant brain edema (MBE) is a serious complication of stroke, with a mortality rate as high as 80% (Battey et al., 2014; Nawabi et al., 2019). Even in patients with non-life-threatening stroke, the severity of cerebral edema is a risk factor for poor prognosis. A recent study has shown that a midline shift greater than 3 mm can independently predict outcomes after ischemic stroke (McKeown et al., 2022). Cerebral edema after stroke is an important cause of the malignant progression of stroke, and is related to adverse outcomes. In this review, we discuss the classification and pathological characteristics of cerebral edema, the role of various molecules and underlying mechanisms, and new therapeutic drugs for management of cerebral edema that develops after stroke, to provide a basis for further studies and for clinical treatment of this condition.
Classification and pathological features of cerebral edema
Recent studies have focused on the scientific understanding of the malignant progression of stroke and on improvement of the long-term prognosis of stroke patients. The pathological mechanisms of cerebral edema after stroke are summarized as follows:
Edema after stroke is divided into three groups according to the molecular pathophysiology: cytotoxic edema, ionic edema, and vasogenic edema (Yao et al., 2020). Cytotoxic edema occurs rapidly after stroke, and is followed by ionic edema, vasogenic edema, and then mixed edema (Simard et al., 2007; Liebeskind et al., 2019). Cytotoxic edema and vasogenic edema are interdependent. The prolongation of cytotoxic edema induces vasogenic edema and vice versa (Jha et al., 2019).
The blood–brain barrier (BBB) is closely related to cerebral edema. It is a highly selective complex of cells located between the luminal substances of the blood vasculature and the brain interstitium (Stokum et al., 2016; Jiang et al., 2018). It is composed of continuous cerebral capillary endothelial cells, tight junctions between these cells, a complete basement membrane, pericytes, and a glial membrane surrounded by the end-feet of astrocytes (Yu et al., 2020; Ji et al., 2021; Figure 1). It contains transporters that provide nutrients to the central nervous system (CNS), ion transporters that participate in brain ion homeostasis, and efflux transporters that prevent compounds from entering the brain (Jiang et al., 2018). Cerebral ischemia can cause destruction of the BBB. Chemicals, liquids, and blood-borne cells enter the brain parenchyma through the damaged BBB, which destroy the water and ion homeostasis of the brain, resulting in cerebral edema (Keaney and Campbell, 2015).
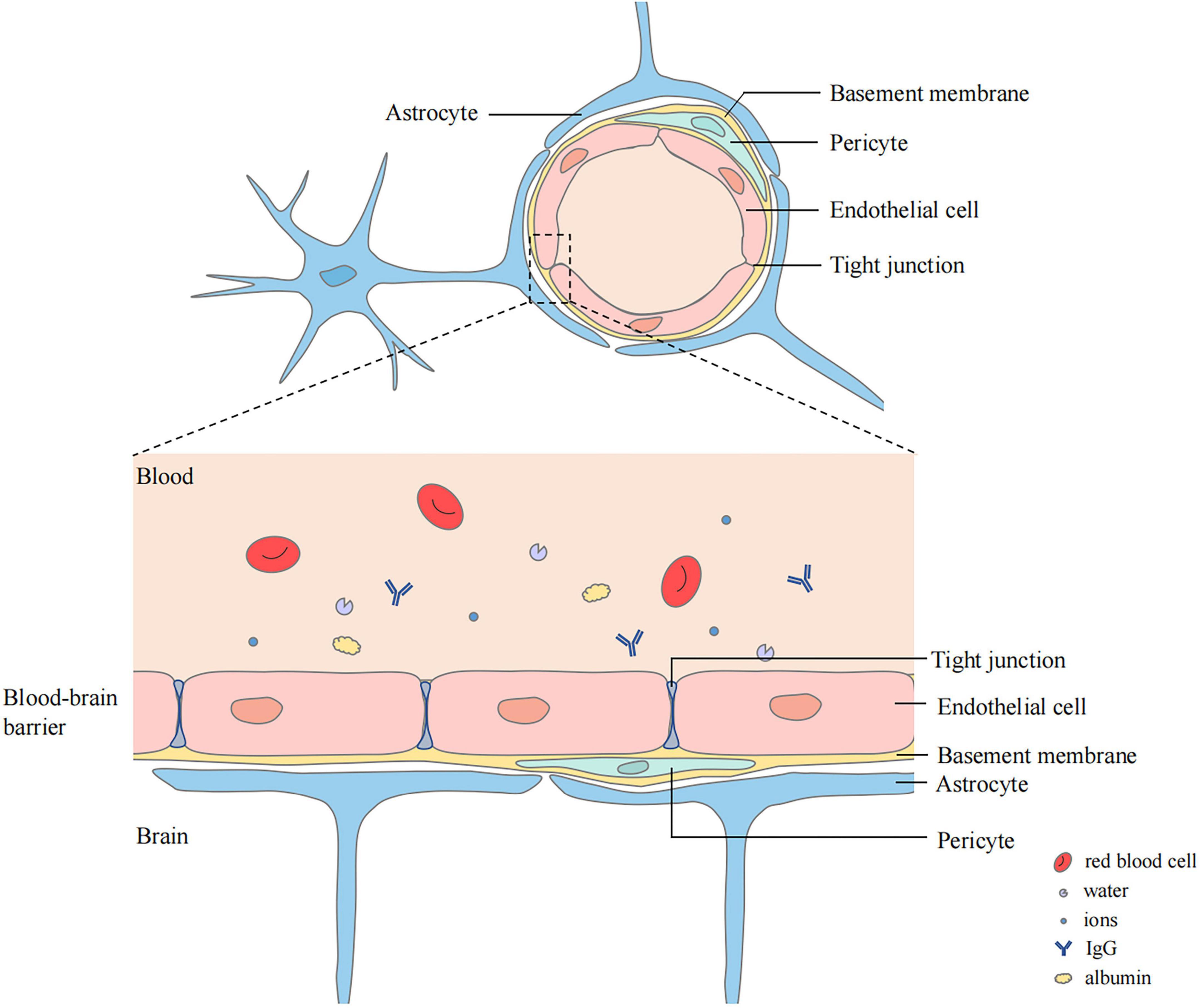
Figure 1. Structure of blood–brain barrier (BBB). BBB locates between the luminal substances of the blood vasculature and the brain interstitium. The capillary lumen is surrounded with endothelial cells connected by tight junctions. Pericytes and Endothelial cells are ensheathed by a basement membrane surrounded by the end-feet of astrocytes.
Cytotoxic edema
Cytotoxic edema is the initial step in the pathological process of cerebral edema. In the early stage of cerebral ischemia and hypoxia, Na+/K+-ATPase damage and ion osmotic-gradient changes cause the osmotically active molecules, mainly Na+, Cl–, and H2O, to transfer from outside to inside the cell, leading to cell swelling and providing a driving force for the formation of ionic edema and vasogenic edema (Stokum et al., 2016; Halstead and Geocadin, 2019; Figure 2). This pathological change is particularly prominent in astrocytes (Stokum et al., 2016).
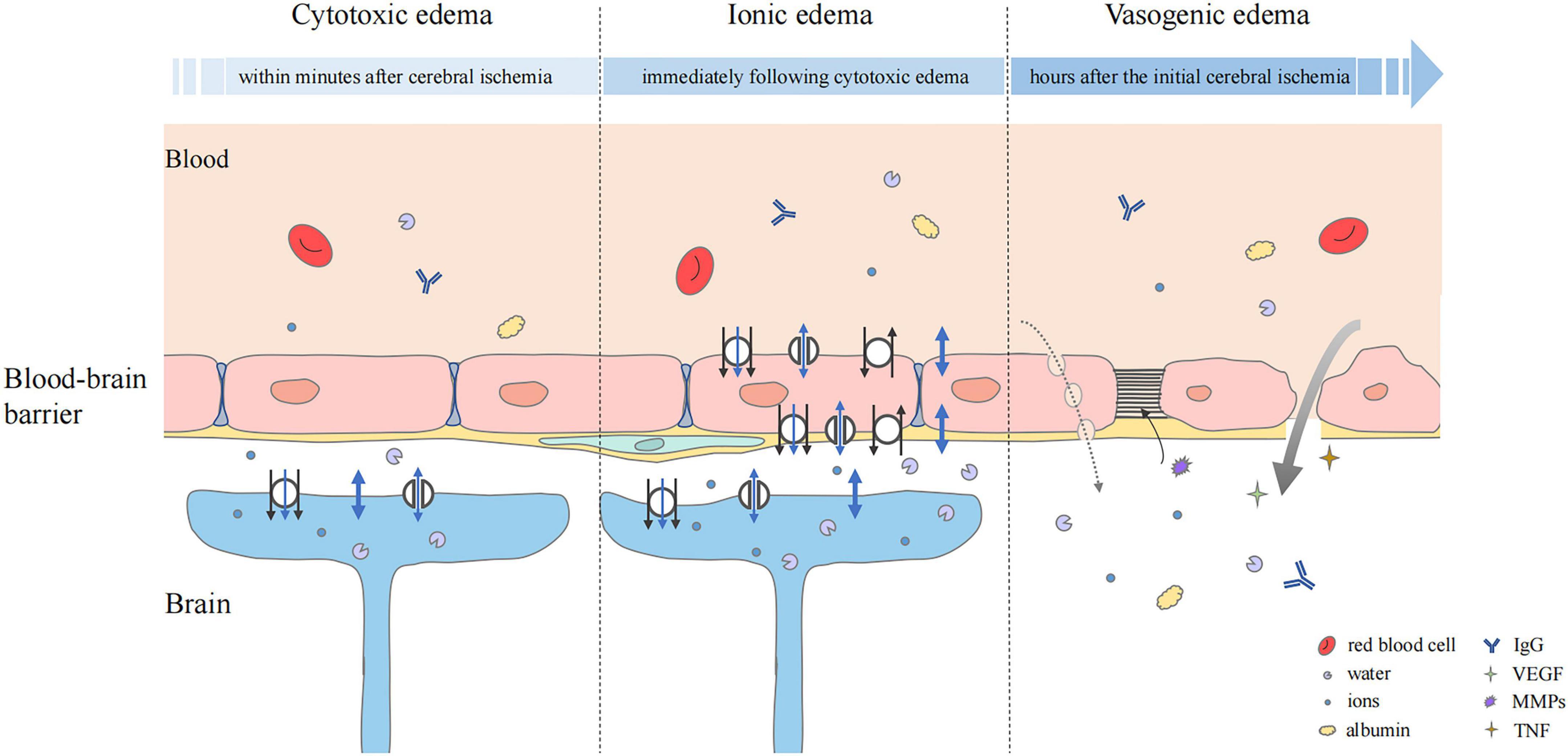
Figure 2. Status of the blood–brain barrier at three phases of cerebral edema. Cytotoxic edema is the initial step and particularly prominent in astrocytes. Cerebral ischemia and hypoxia induced the ion influx (black arrow), which leads to osmotic gradient changes. Water may flow into astrocytes in three ways, simple diffusion (thick blue double-headed arrows), passive transport through transmembrane channels (thin blue double-headed arrows), and water co-transport (blue single-headed arrows). In ionic edema, ion, and water influx are mediated by plasmalemma channels and transporters of endothelial cells. Upregulation of transporters and ion channels also occurs in astrocytes. Vasogenic edema is characterized by destruction of the BBB. The transport of ions, water, and serum proteins such as albumin and IgG may occur directly (thick gray arrow) or via pinocytic vesicles (dashed gray arrow). Multiple factors, including VEGF, MMPs, and pro-inflammatory cytokines such as TNF are involved. They mediate neuroinflammation and tight junction degradation, aggravating cerebral edema.
Water may flow into astrocytes in three ways (Stokum et al., 2016). Firstly, simple diffusion via the lipid bilayer can lead to the inflow of a large amount of water (MacAulay, 2021). Secondly, water flux is driven by osmotic gradients through transmembrane water channels, including the aquaporin (AQP) family and some astrocyte transporters, such as SGLT1, GLUT1, and GLUT2 (Zeuthen et al., 1997; MacAulay and Zeuthen, 2010). In addition, water can be translocated together with ion fluxes, which is driven by some ion transporters expressed by astrocytes. These transporters, such as NKCC1 and the glutamate transporter EAAT1, can regulate secondary water co-transport through transference of a fixed number of water molecules and ions per transport action (MacAulay and Zeuthen, 2010).
A study in an animal model of ischemia found that extracellular Na+ decreased from 141 mmol at baseline to 74 mmol after cerebral ischemia (Mori et al., 2002). When the plasma Na+ was 134 mmol, the cytotoxic edema produced a transendothelial Na+ concentration differential of about 60 mmol (Mori et al., 2002). The Na+ gradient produced by cytotoxic edema acts as a source of potential energy to drive the subsequent inflow of ionic edematous fluid (Stokum et al., 2016).
Ionic edema
Ionic edema occurs after cytotoxic edema and can develop in the early stage of endothelial dysfunction. Due to the ion concentration gradient formed by cytotoxic edema, Na+, Cl–, and water are first transported into endothelial cells through the luminal membrane, and then transported outside the lumen through the abluminal membrane of the cerebral capillary endothelial cells (Stokum et al., 2016; Figure 2). Na+, the main driver of ionic edema, propels the inflow of secondary participants, such as Cl– and water to balance the electrical and osmotic gradients.
There are three possible ways by which water is transported through the plasmalemma. Firstly, water can move through simple diffusion across the endothelial cell membrane (MacAulay, 2021). Secondly, secondary co-transport of water can be regulated by some common transport channels for water and ions, as well as some endothelial transporters, such as NKCC1, KCC, MCT1, and GAT-1 (Hamann et al., 2010; MacAulay and Zeuthen, 2010). Thirdly, cerebral endothelial cells express some membrane proteins that can regulate passive water transport (Stokum et al., 2016).
Vasogenic edema
Vasogenic edema occurs after ionic edema and is characterized by destruction of the BBB (Stokum et al., 2016; Chen et al., 2021). With the progress of edema, cross-endothelial permeability pores are formed, through which water and some plasma proteins can be extravasated into the cerebral interstitial compartment (Stokum et al., 2016; Zhang et al., 2020a; Figure 2). Protein and water can enter the interstitial fluid through reverse pinocytosis. Pinocytosis is a biological process in which blood solutes are folded and wrapped by the plasma membrane of endothelial cells to absorb and transport substances (Swanson and Watts, 1995). It is thought that vasogenic edema can also develop through paracellular transport of endothelial cells, which can be triggered by inflammation and cerebral ischemia to increase endothelial permeability (Garcia et al., 1986). There is evidence that the BBB disruption caused by endothelial cell contraction is not an adequate substitute for tight junction disruption (Moy et al., 1996). Endothelial cell contraction may help to enhance the formation of vasogenic edema rather than initiate it. VEGF signaling can generate paracellular permeability pores. When its expression is triggered by cerebral injury, tight junction protein expression is decreased, vascular permeability is increased, and edema formation is enhanced (Kovacs et al., 1996; Skold et al., 2005; Dore-Duffy et al., 2007). A previous study showed that early administration of recombinant VEGF after experimental rat stroke increased edema formation (Zhang et al., 2000).
The development of cerebral edema is a fatal risk factor for adverse outcomes after stroke. Quantifying the severity and evolution of cerebral edema after stroke plays an important but challenging role in clinical studies (Kumar et al., 2022). Midline shift is the most common measurement for cerebral edema and is defined as the maximum deviation of midline brain structures in the axial plane (Ropper, 1986; Vorasayan et al., 2019; Fonseca et al., 2021). It can be assessed by computed tomography (CT), magnetic resonance imaging (MRI), or bedside transcranial sonography (Gerriets et al., 2001; Walberer et al., 2007). However, it is a crude measurement that may be noticeable to substantial cerebral edema and less sensitive to smaller swelling changes. Relative hemispheric volume is defined as 3-dimensional volume ratio of the ischemic hemisphere to the contralateral hemisphere, and seems to be more accurate than midline shift in manifesting the clinical impact of post-stoke cerebral edema (Ostwaldt et al., 2018; Ng et al., 2022a). However, they both measure the mass effect but not water content (Ostwaldt et al., 2018). Net water uptake (NWU) is a CT densitometry-based method used to calculate water uptake in ischemic tissue (Minnerup et al., 2016; Cheng et al., 2022). It symbolizes the proportion of ischemic tissue composed of excess water, which is promising for quantifying the progression of cerebral edema (Broocks et al., 2020). However, the presence of hemorrhagic transformation within cerebral infarction and postangiographic iodine contrast staining might make the measurement of NWU inaccurate, compromising the value of NWU compared to volumetric edema biomarkers (Kumar et al., 2022; Ng et al., 2022b). The entry of water into the cerebral tissues can be visualized using MRI modalities (Obenaus and Jacobs, 2007). T2-weighted imaging (T2WI) and diffusion-weighted imaging (DWI) are two classical sequences used to evaluate edema processes (Obenaus and Badaut, 2022). The apparent diffusion coefficient (ADC), a quantitative parameter of DWI, can be used to evaluate water mobility within the cerebral cortex (Warach et al., 1996). Cytotoxic edema can be observed as early changes in DWI signal intensity. T2WI can visualize increased cerebral water content at later time points during the development of vasogenic edema (Obenaus and Badaut, 2022). However, these MRI techniques are expensive, time-consuming, and cannot monitor cerebral edema in real-time. Electrical impedance tomography (EIT) is a real-time functional imaging technique that allows imaging of electrical impedance changes in the volume of interest (Ke et al., 2022). Because the electrical impedance between cerebral edema tissue and normal cortical tissue is different, EIT could recognize different types of cerebral edema (Yang et al., 2019). However, the application of EIT is limited by factors such as the modeling accuracy and reconstruction algorithms (Ke et al., 2022). The emergence of artificial intelligence points to a new direction for evaluating cerebral edema, but minimal efforts have been made to detect cerebral edema (Obenaus and Badaut, 2022). The application of artificial intelligence in future extensive studies may facilitate the understanding and diagnosis of cerebral edema after stroke.
Factors associated with the formation of cerebral edema
The mechanisms of cerebral edema are based on the principle formulated by Starling in the late 19th century (Starling, 1896). He established the basic model of transcapillary driving forces to promote edema formation, and proposed that two elements are needed during the formation of cerebral edema: the driving force, which “pushes” or “pulls” materials into or out of the brain; and the “permeability pores,” which regulate the flux of these materials over the capillary (Simard et al., 2007). There are many factors that can affect these process, and there are often intricate interactions among them (Figure 3). Therefore, the mechanisms underlying cerebral edema are very complex.
Below, we briefly summarize the factors that have been a focus of research in this context.
AQP4 and cerebral edema
Aquaporin, membrane proteins that allow bidirectional movement of water molecules across the phospholipid bilayer plasma membrane, contain 14 different members, although only AQP1, AQP4, AQP9, and AQP11 are expressed in the CNS (Gorelick et al., 2006; Vella et al., 2015; Stokum et al., 2018). AQP4, which is expressed by astrocytes, plays a bidirectional role in water transport and participates in the formation and elimination of cerebral edema (Stokum et al., 2015). The expression of AQP4 is mainly located in the following four positions in the cerebrum: the end-feet of the paravascular astrocytes, astrocyte processes under the glia limitans external membrane, the ependymal basolateral plasma membrane, and the astrocyte processes under the glia limitans external ependymal membrane (Papadopoulos and Verkman, 2013; Ji et al., 2021).
The end-feet of astrocytes and AQP4 play a vital role in clearance and regulation of cerebral edema (Stokum et al., 2016). AQP4 can also promote the flow of the glymphatic system to eliminate toxic substance deposition in the brain and regulate formation of cerebral edema (Liu et al., 2020). The significantly high expression of AQP4 after ischemic stroke may promote the formation of cerebral edema (Yu et al., 2015; Kitchen et al., 2020; Mestre et al., 2020; Ji et al., 2021). When AQP4 is knocked-out or inhibited, formation of cerebral edema after ischemic stroke is reduced. A study has shown that thyroid hormone therapy can weaken brain edema by inhibiting AQP4 and may have neuroprotective effects on post-stroke patients (Sadana et al., 2015). It has been reported that rat cerebral edema can be reduced by inhaling hydrogen sulfide, involving AQP4 inhibition (Wei et al., 2015). Cerebral edema was found to be alleviated by a combination therapy of AQP inhibitors and cerebrolysin in a permanent middle cerebral artery occlusion (MCAO) animal model (Catalin et al., 2018). After treatment with TGN-020, an AQP4 inhibitor, cerebral edema was reduced at 3 and 7 days in a rat MCAO model after ischemia (Pirici et al., 2017). AQP4 plays a complex dual role during the cerebral edema process after stroke, aggravating cerebral edema formation in the early stage and reducing edema in the later stage (Loh et al., 2019; Clement et al., 2020). However, the mechanisms of this function remain unclear. The use of AQP4 inhibitors after ischemic stroke has become a research hotspot. As the time limit for the formation of cytotoxic edema, ionic edema, and vasogenic edema remains unclear, the timing of initiation and termination of APQ4 inhibitor treatment remains an urgent unanswered question.
Sulfonylurea receptor 1-transient receptor potential melastatin 4 and cerebral edema
Sulfonylurea receptor 1 (SUR1) belongs to the adenosine 5′-triphosphate (ATP)-binding cassette superfamily encoded by ABCC8 and is a critical mediator of cell swelling in the CNS through the transient receptor potential melastatin 4 (TRPM4) channel (Aittoniemi et al., 2009; Jha et al., 2021; Alquisiras-Burgos et al., 2022). SUR1-TRPM4 is not expressed normally in the CNS, but expressed only after a CNS injury (Stokum et al., 2016; Gerzanich et al., 2019; Alquisiras-Burgos et al., 2020). In all central neurons, CNS injury triggers the activation of the hypoxia-inducible factor 1 transcription factor, which induces the binding of SUR1 to TRPM 4, increases its Ca2 + sensitivity, and makes the channel sensitive to ATP depletion (Woo et al., 2012, 2013; Mehta et al., 2013). The SUR1-TRPM4 channel contributes to the formation of ionic edema by regulating the Na+ inflow over the luminal membrane and the Na+ outflow over the abluminal membrane. In the case of severe CNS injury and ATP depletion, maladaptive cell swelling and cytotoxic edema can be caused by excessive Na+ inflow via the SUR1-TRPM4 channel (Stokum et al., 2016). Since the SUR1 regulatory mechanism depends on gene transcription and ATP, it plays a significant role in cerebral ischemia–reperfusion (Stokum et al., 2016). It has been proposed that SUR1-TRPM4 and AQP4 can form a novel heteromultimeric water/ion channel complex, which synergistically regulates rapid and high-volume water flow and astrocyte swelling (Stokum et al., 2018; Jha et al., 2021).
A large number of studies have shown that SUR1-TRPM4 expression is upregulated in ischemic stroke. In a rat model, TRPM4 was upregulated in cerebral endothelial cells 2 h after stroke reperfusion, and inhibition of TRPM4 by siRNA therapy reduced infarct volume and cerebral edema (Chen et al., 2019b). In addition, administration of a TRPM4-specific antibody could prevent the swelling of cells exposed to hypoxia, by inhibiting the channel current (Chen et al., 2019a). In view of the specific driver role of the SUR1-TRPM4 channel in cytotoxicity and ionic edema, pharmacological research on SUR1-TRPM4 has become a hotspot in stroke therapeutic research. Animal experimental studies have shown that glimepiride, a SUR1-TRPM4 channel inhibitor, can reduce stroke in mice and is as effective as glibenclamide in reducing cerebral edema in wild-type mice (Wang et al., 2020c). Currently, a SUR1-TRPM4 channel inhibitor is the only drug that has entered clinical trials for the treatment of cerebral edema after ischemic stroke (Yao et al., 2020). The results of phase II clinical trials have shown that intravenous glibenclamide can reduce cerebral edema and midline displacement (Sheth et al., 2016; Pergakis et al., 2019). Subsequent exploratory studies have shown that glibenclamide can alleviate water accumulation, abate the mass effect, improve the survival rate, reduce midline deviation, and weaken matrix metalloproteinases 9 (MMP9) expressions (Kimberly et al., 2018a; Sheth et al., 2018; Vorasayan et al., 2019). In a clinical study evaluating the efficacy of oral glibenclamide for the treatment of cerebral edema after ischemic stroke, the drug did not increase early death or hypoglycemia, and could prevent cerebral edema (Huang et al., 2019). These findings facilitated the large scale of the phase III clinical trials in large-scale cerebral infarction.
MMP9 and cerebral edema
Matrix metalloproteinases (MMPs) are a group of zinc endopeptidases that can degrade almost all types of extracellular membrane proteins (Kurzepa et al., 2014). There are over 23 different types of MMPs in the human body. According to their substrate specificity, they are divided into collagenase, gelatinase, matrix metalloelastase, enamel proteinase, and so on (Klein and Bischoff, 2011; Turner and Sharp, 2016). MMPs can mediate the destruction of basement membrane proteins, leading to increased permeability of the BBB, exudation of leukocytes, cerebral edema, and hemorrhagic transformation (Sifat et al., 2017). MMP expression levels are very low under normal conditions, but the levels of MMP2 and MMP9 increase significantly within hours of cerebral ischemia (Zhang et al., 2020d).
A meta-analysis showed that MMP9 levels were higher in patients who suffered a stroke with severe cerebral edema and hemorrhagic transformation (Wang et al., 2020b). The expression of MMPs, and particularly that of MMP9, increases after ischemia. This is strongly associated with extracellular matrix disruption and subsequent vascular permeability (Turner and Sharp, 2016; Beker et al., 2022). MMP9 increases rapidly after cerebral ischemia and hypoxia, destroying the integrity of the vascular wall by degrading tight junctions and the extracellular matrix to increase the BBB permeability, and further leading to neuronal death, cerebral edema, and hemorrhagic transformation (Yang and Rosenberg, 2011; Shi et al., 2016; Bernardo-Castro et al., 2020). Some studies found that MMP9 is involved in maintaining the structural integrity of the AQP4 water channel and participates in the regulation of cerebral edema (Wang et al., 2014; Datta et al., 2022). An in vitro study showed that MMP9 silencing downregulated the expression of AQP4 in astrocytes (Li et al., 2020). It has also been suggested that MMP9 is related to the inflammation induced by ischemic stroke and participates in the destruction of the BBB (Bellut et al., 2021; Kim et al., 2021). MMP inhibitors can reduce cerebral edema associated with ischemia by partially preventing the degradation of tight junction proteins (Turner and Sharp, 2016; Datta et al., 2022). Another study has shown that downregulation of MMP9 can reduce destruction of the BBB and cerebral edema in a murine middle cerebral artery occlusion–reperfusion model (Xiong et al., 2022). Some studies have reported that MMP9 may be one of the most promising biomarkers for assessing the BBB permeability and predicting hemorrhage transformation in ischemic stroke (Bernardo-Castro et al., 2020; Mechtouff et al., 2020). However, further research is needed in this regard.
MicroRNAs and cerebral edema
MicroRNAs (miRNAs), a class of non-coding single-stranded RNA molecules approximately 22 nucleotides in length, encoded by endogenous genes, can regulate gene expression at the transcriptional level and have become promising targets for disease treatments (Carleton et al., 2007; Li et al., 2018). The expression of MiR-1 has been shown to be related to ischemic injury and apoptosis (Chen et al., 2006). The volume of cerebral infarction can be decreased after anti-MiR-1 treatment (Selvamani et al., 2012). The application of a MiR-1 antagonist significantly reduced cerebral edema and BBB damage (Talebi et al., 2019). Notably, studies have shown that other miRNAs, such as miRNA-132 and miRNA-1906, are potential targets for the treatment of cerebral edema (Zuo et al., 2019; Yu and Li, 2020). However, the mechanisms remain unclear and further research is required.
Cerebral veins and cerebral edema
The intracranial venous system comprises 70–80% of the intracranial circulating blood volume and is the main blood storage and reflux system (Pang, 2001). In recent years, studies of intracranial veins have attracted increasing attention. An increasing number of studies have shown that, during acute ischemic stroke (AIS), the functional reflux of the cerebral vein may be as important as the arterial flow, and that the status of cerebral venous reflux may provide additional information about patients, such as prognosis prediction.
A clinical study showed that hypoplasia or occlusion of the transverse sinus is related to prolongation of intracranial circulation time and impairment of brain autonomous regulation and is positively associated with severe cerebral edema after massive middle cerebral artery infarction (Yu et al., 2009). A clinical study showed that, regardless of the status of the collateral vessels on CT angiography (CTA) after treatment of AIS, the venous outflow was associated with cerebral edema formation. A good venous outflow was related to a reduction of the NWU and good functional outcomes (Faizy et al., 2021b). The team also showed that good venous outflow before treatment was associated with successful reperfusion, favorable tissue-level collaterals, and a good prognosis in AIS patients who received intravascular treatment (Faizy et al., 2021a,c). A previous study has shown that the ipsilateral medullary vein can be an important predictor of adverse clinical outcomes after AIS and is related to hypoperfusion (Yu et al., 2016). In patients with acute large artery occlusion, the absence of the ipsilateral cortical vein as a specific imaging marker of cerebral midline shift contributes to the estimation of the baseline core volume of ischemic stroke in the prediction of a midline shift (Zhang et al., 2020c). A midline shift is more likely to develop in patients with dural sinus hypoplasia (Volny et al., 2016). However, to date, most of these have been clinical studies, and the underlying mechanisms remain unclear.
Inflammatory response and cerebral edema
Recently, the role of inflammatory response in the BBB destruction in ischemic stroke has been increasingly recognized. Cerebral ischemia manifests as decreased inflammation inhibitory signals and increased alert signals from dead or necrotic neurons cells and glial cells, which may activate quiescent brain immune cells (Liesz et al., 2015; He et al., 2019). Cerebral immune cell activation further upregulates pro-inflammatory factor and chemokine expressions, activates MMPs to damage the integrity of the BBB, enlists peripheral immune cells to the damaged site, promotes the development of irreversible injury in the infarct core area, and results in secondary BBB injury (Malone et al., 2019; Mulder et al., 2021; Qiu et al., 2021). Immune cells, including cerebral immune cells (CICs) and peripheral immune cells (PICs) play a vital role.
After ischemic stroke, PICs, including neutrophils, monocytes, and T lymphocytes, lead to microvascular diseases and the secretion of inflammatory molecules, thereby increasing BBB permeability. In the late stage of ischemic stroke, these contribute to BBB repair and angiogenesis (Qiu et al., 2021). Specifically blocking the adhesion of neutrophils to endothelial cells in venules in the mouse MCAO model was found to significantly reduce the volume of cerebral infarction and neurological deficit and improve both short-term and long-term functional outcomes (Dhanesha et al., 2020). Other studies have shown that neutrophils may express anti-inflammatory phenotypes; the N2 polarization of neutrophils contributes to the phagocytosis of neutrophils by microglia/macrophages, resulting in a reduction of cerebral edema and infarct volume (Garcia-Culebras et al., 2019; Hou et al., 2019). Therefore, neutrophils may play a dual role in the evolution of ischemic stroke.
Similarly, CICs, including microglia, astrocytes, and pericytes of the BBB, play a profound immunomodulatory role in ischemic stroke. Microglia and astrocytes are activated within minutes after cerebral ischemia and release some pro-inflammatory factors, such as TNF-α, NF-κB, IL-1β, and IL-6 (Bonaventura et al., 2016; Kim and Cho, 2016). They promote the inflammatory response, destruction of the BBB structure, increase of the BBB permeability, and uncontrolled MBE or symptomatic intracerebral hemorrhage (Kanazawa et al., 2017). In a rat permanent MCAO model, preconditioning with a TNF-α receptor inhibitor had a protective effect against neurological impairment, cerebral infarction, and edema after stroke (Lin et al., 2021). NF-κB, generally considered as the “central link” of inflammation in vivo, is an important mediator in the process of ischemia–reperfusion injury. Inhibition of NF-κB expression can reduce the inflammatory cascade and protect the structure and function of the BBB (Wu et al., 2018; Howell and Bidwell, 2020). After cerebral ischemia, pericytes express an inflammatory phenotype (CD11b-positive), upregulate pro-inflammatory cytokine expression, and promote increased BBB permeability (Zhou et al., 2018). It is thought that the phagocytosis of pericytes may contribute to the regression of inflammation and repair of the BBB in ischemic stroke (Qiu et al., 2021). Therefore, pericytes may play a bidirectional role in ischemic stroke, which needs to be confirmed by further studies.
CICs and PICs are interwoven in a subtle and complex network (Qiu et al., 2021). Moreover, the phenotypes of inflammatory response involved by immune cells are different in the initiation, progression, and regression stages of cerebral ischemia (Mulder et al., 2021). Treatments that target only one kind of immune cell may affect another kind of immune cell and even lead to poor outcomes in ischemic stroke. Therefore, more studies are needed to explore the mechanisms of inflammatory response of cerebral edema after ischemic stroke.
Cerebral ischemia/reperfusion and cerebral edema
The most effective treatment for AIS is to achieve the recanalization and reperfusion of ischemic brain tissue. Intravenous thrombolysis and mechanical embolectomy are two widely recognized treatment strategies (Rabinstein, 2017; Feng et al., 2019). It is true that many patients benefit from the above treatments, but some patients who receive the above treatments have a poor prognosis. Scholars gradually put forward the concept of cerebral ischemia/reperfusion injury. It is caused by a series of pathological cascade reactions triggered by the recovery of oxygenated blood flow into the ischemic brain tissue (Eltzschig and Eckle, 2011; Fisher and Savitz, 2022; Wang et al., 2022). At present, the relationship between reperfusion and cerebral edema is still not clear, as the views on the effect of reperfusion on cerebral edema are different.
In secondary analysis of a multicenter prospective clinical trial of endovascular treatment for patients with AIS, Kimberly et al. (2018b) showed that successful reperfusion was linked to a reduced midline shift and that reperfusion therapy reduced cerebral edema. However, this analysis was performed in patients using a baseline non-contrast CT and CTA, a follow-up CTA, or magnetic resonance angiogram only, and no perfusion imaging was used to assess core infarct volumes. A meta-analysis of seven randomized controlled trials for AIS reperfusion therapy in 2021 showed that reperfusion therapy was associated with maximal midline shift as a measure of space-occupying cerebral edema in patients with a large baseline core infarct volume (> 130 mL) (Ng et al., 2021b). Ng et al. (2021a) performed a post hoc analysis of randomized trials of endovascular therapy for patients with anterior circulation strokes and explored the relationships between pretreatment core and mismatch volume, reperfusion, and cerebral edema after stroke. The patients underwent baseline CT perfusion imaging. Cerebral edema was measured in the midline shift on a 24-h follow-up CT or MRI. Most patients had a small to moderate core volume. This study showed that successful reperfusion was associated with a reduced cerebral edema in patients with small to medium cerebral infarction. Ng et al. (2021a) also found that a large core volume and small mismatch volume were associated with increased cerebral edema after reperfusion, indicating that the effect of reperfusion on post-stroke cerebral edema may vary. To assess the role of ischemic lesion volume after reperfusion treatment in cerebral edema, Ng et al. carried out further research to overcome the limitations of imaging technology and midline shift. They used midline shift and relative hemispheric volume to measure the cerebral edema. The patients underwent 24-h follow-up MRI with dynamic susceptibility contrast-enhanced perfusion-weighted imaging (Ng et al., 2022a). Ng et al. (2022a) found that continuous hypoperfusion for 24 h after reperfusion treatment was related to worse cerebral edema, even though some patients successfully achieved reperfusion. Their series of studies showed that the development of cerebral edema in response to reperfusion may depend on the physiological state of brain tissue, while the irretrievable infarcted tissue swells significantly after reperfusion and the salvable tissue does not swell nor has a low risk of swelling after reperfusion.
In animal experiments, the transient MCAO model showed that there was significant cerebral edema after reperfusion, suggesting that reperfusion injury promoted the formation of cerebral edema (Pillai et al., 2009; Winkler et al., 2021). This may be related to the oxidative/nitrosative stress reaction after reperfusion, in which free radicals play an important role (Sun et al., 2018). An animal experiment in 2022 showed that preventive inhibition of free radical production could reduce cerebral edema in reperfused MCAO rats (Xing et al., 2022). Excessive nitric oxide levels after reperfusion can affect activation of the MMP pathway and the distribution of tight junctions, leading to destruction of the BBB and cerebral edema aggravation (Sun et al., 2018). Oxidative stress promotes the release of pro-inflammatory cytokines, leading to a neuroinflammatory response (as described above), directly or indirectly resulting in destruction of the BBB and cerebral edema (L et al., 2016; Jurcau and Simion, 2021). The mechanism is complex and requires further investigation.
It is apparent that there are inconsistencies between the results of clinical studies and animal experiments. Moreover, some conclusions from animal experiments have not been verified in clinical practice. This may be because the transient MCAO model used in animal experiments rapidly forms a large cerebral infarction in the middle cerebral artery distribution area, demonstrating the adverse effects of reperfusion on cerebral edema. However, in clinical studies, the volume of the core infarction in many patients is relatively small, which reflects the different effects of reperfusion on cerebral edema (Ng et al., 2021b). It is crucial to study the relationship between reperfusion and cerebral edema, clarify the influence of reperfusion on cerebral edema, and explore effective treatment.
Treatment of cerebral edema
At present, osmotic diuretics, particularly mannitol and hypertonic saline, remain the main drugs used clinically to reduce cerebral edema. The main mechanism involves establishment of an intravascular osmotic gradient, resulting in water flowing from the intercellular space to the intravascular space. The main function of this intervention is to reduce intracranial hypertension and alleviate the mass effect, but it may lead to serious complications, such as kidney injury and water and electrolyte disorder (Zhang et al., 2019; Stokum et al., 2020). Decompressive craniectomy is an effective method for the treatment of MBE, which can reduce mortality and improve prognosis, but most surviving patients are left with severe disability (Shah et al., 2019). Moreover, these interventions can only be applied after complete development of destructive edema, and are high-risk and low-efficacy approaches (Torre-Healy et al., 2012; Gopalakrishnan et al., 2018). Treatments targeting multiple pathways involved in the formation of edema in the CNS, with a view to prevention, may be more valuable than those that eliminate edema once it has developed (Jha et al., 2019).
It is currently a research hotspot to select targets and study new drugs to prevent and treat cerebral edema, based on the underlying molecular mechanisms. A large number of studies have been conducted to investigate these targets. These studies included treatment with SUR1-TRPM4 channel inhibitors (Vorasayan et al., 2019; Wang et al., 2020c), AQP4 blockers (Darabi and Mohammadi, 2017; Catalin et al., 2018), MMP9 inhibitors (Cui et al., 2012; Kimberly et al., 2018a), ion channel blockers, such as NKCC1 and NHE (Spasov et al., 2016; Zhang et al., 2020b), VEGF-related drugs (Yang et al., 2018; Wang et al., 2019), miRNAs (Talebi et al., 2019; Zuo et al., 2019), and some other protective agents, such as edaravone, calcitriol, and 3-aminobenzamide (Liu et al., 2019; Sadeghian et al., 2019; Wang et al., 2020a). However, most of these studies involved animal experiments and few involved clinical trials. Glibenclamide, a potent SUR1-TRPM4 channel inhibitor, is known to enter clinical trials for the treatment of post-stroke cerebral edema. GAMES-Pilot (NCT01268683) is a phase II trial that used intravenous glibenclamide in patients with anterior circulation stroke. A post-exploratory analysis of this trial suggested that glibenclamide was related to decreased water diffusivity and MMP-9 levels, indicating vasogenic edema reduction (Sheth et al., 2014). GAMES-RP (NCT01794182) was a phase II trial that used intravenous glibenclamide to verify the efficacy of intravenous glibenclamide compared with placebo in patients with large anterior hemisphere infarctions at risk of MBE. Post-exploratory analyses of this trial showed that intravenous glibenclamide reduced the midline shift, NWU, and MMP9 expression (Kimberly et al., 2018a; Sheth et al., 2018; Vorasayan et al., 2019). CHARM (NCT02864953), a phase III trial to assess the efficacy and safety of glibenclamide in large hemispheric infarctions for cerebral edema, is currently underway. A phase I trial of AER-271, an aquaporin inhibitor, is currently in progress for its eventual use in patients with AIS (NCT03804476). No specific new drugs have been approved for clinical application.
Conclusion and perspective
Cerebral edema after ischemic stroke has a high morbidity, mortality, and disability, and is increasingly focused on in research. At present, the treatment of cerebral edema is mainly based on clinical experience, and mostly involves symptomatic treatment after cerebral edema has developed. Such treatment cannot curb the occurrence and development of malignant edema from its basis. Therefore, it is essential to clarify the mechanisms underlying cerebral edema development after ischemic stroke, in order to identify patients prone to MBE early, to find effective therapeutic targets, to determine more effective forms of diagnosis and treatment, and to carry out effective prevention and treatment in time.
Author contributions
YG designed and wrote the manuscript. CZ contributed to the conception and carried out logic examination of the manuscript. ZP and HY participated in the review of medical professional knowledge of the manuscript. HJ, HW, and YZ helped with proofreading and revision. GN and XJ contributed to the conception and critically revised the manuscript. All authors contributed to the article and approved the final version.
Funding
This work was supported by the Cheung Kong (Changjiang) Scholars Program (T2014251) and the Pharmaceutical Collaboration Project of Beijing Science and Technology Commission (Z181100001918026).
Conflict of interest
The authors declare that the research was conducted in the absence of any commercial or financial relationships that could be construed as a potential conflict of interest.
Publisher’s note
All claims expressed in this article are solely those of the authors and do not necessarily represent those of their affiliated organizations, or those of the publisher, the editors and the reviewers. Any product that may be evaluated in this article, or claim that may be made by its manufacturer, is not guaranteed or endorsed by the publisher.
References
Aittoniemi, J., Fotinou, C., Craig, T. J., de Wet, H., Proks, P., and Ashcroft, F. M. (2009). Review. SUR1: A unique ATP-binding cassette protein that functions as an ion channel regulator. Philos. Trans. R. Soc. Lond. B Biol. Sci. 364, 257–267. doi: 10.1098/rstb.2008.0142
Alquisiras-Burgos, I., Franco-Perez, J., Rubio-Osornio, M., and Aguilera, P. (2022). The short form of the SUR1 and its functional implications in the damaged brain. Neural Regen. Res. 17, 488–496. doi: 10.4103/1673-5374.320967
Alquisiras-Burgos, I., Ortiz-Plata, A., Franco-Perez, J., Millan, A., and Aguilera, P. (2020). Resveratrol reduces cerebral edema through inhibition of de novo SUR1 expression induced after focal ischemia. Exp. Neurol. 330:113353. doi: 10.1016/j.expneurol.2020.113353
Battey, T. W., Karki, M., Singhal, A. B., Wu, O., Sadaghiani, S., Campbell, B. C., et al. (2014). Brain edema predicts outcome after nonlacunar ischemic stroke. Stroke 45, 3643–3648. doi: 10.1161/STROKEAHA.114.006884
Beker, M. C., Caglayan, A. B., Altunay, S., Ozbay, E., Ates, N., Kelestemur, T., et al. (2022). Phosphodiesterase 10A is a critical target for neuroprotection in a mouse model of ischemic stroke. Mol. Neurobiol. 59, 574–589. doi: 10.1007/s12035-021-02621-5
Bellut, M., Papp, L., Bieber, M., Kraft, P., Stoll, G., and Schuhmann, M. K. (2021). NLPR3 inflammasome inhibition alleviates hypoxic endothelial cell death in vitro and protects blood-brain barrier integrity in murine stroke. Cell Death Dis. 13:20. doi: 10.1038/s41419-021-04379-z
Bernardo-Castro, S., Sousa, J. A., Bras, A., Cecilia, C., Rodrigues, B., Almendra, L., et al. (2020). Pathophysiology of blood-brain barrier permeability throughout the different stages of ischemic stroke and its implication on hemorrhagic transformation and recovery. Front. Neurol. 11:594672. doi: 10.3389/fneur.2020.594672
Bonaventura, A., Liberale, L., Vecchie, A., Casula, M., Carbone, F., Dallegri, F., et al. (2016). Update on inflammatory biomarkers and treatments in ischemic stroke. Int J Mol Sci 17:1967. doi: 10.3390/ijms17121967
Broocks, G., Hanning, U., Faizy, T. D., Scheibel, A., Nawabi, J., Schon, G., et al. (2020). Ischemic lesion growth in acute stroke: Water uptake quantification distinguishes between edema and tissue infarct. J. Cereb. Blood Flow Metab. 40, 823–832. doi: 10.1177/0271678X19848505
Carleton, M., Cleary, M. A., and Linsley, P. S. (2007). MicroRNAs and cell cycle regulation. Cell Cycle 6, 2127–2132. doi: 10.4161/cc.6.17.4641
Catalin, B., Rogoveanu, O. C., Pirici, I., Balseanu, T. A., Stan, A., Tudorica, V., et al. (2018). Cerebrolysin and aquaporin 4 inhibition improve pathological and motor recovery after ischemic stroke. CNS Neurol. Disord. Drug Targets 17, 299–308. doi: 10.2174/1871527317666180425124340
Chen, B., Gao, Y., Wei, S., Low, S. W., Ng, G., Yu, D., et al. (2019a). TRPM4-specific blocking antibody attenuates reperfusion injury in a rat model of stroke. Pflugers Arch. 471, 1455–1466. doi: 10.1007/s00424-019-02326-8
Chen, B., Ng, G., Gao, Y., Low, S. W., Sandanaraj, E., Ramasamy, B., et al. (2019b). Non-invasive multimodality imaging directly shows TRPM4 inhibition ameliorates stroke reperfusion injury. Transl. Stroke Res. 10, 91–103. doi: 10.1007/s12975-018-0621-3
Chen, J. F., Mandel, E. M., Thomson, J. M., Wu, Q., Callis, T. E., Hammond, S. M., et al. (2006). The role of microRNA-1 and microRNA-133 in skeletal muscle proliferation and differentiation. Nat. Genet. 38, 228–233. doi: 10.1038/ng1725
Chen, S., Shao, L., and Ma, L. (2021). Cerebral edema formation after stroke: Emphasis on blood-brain barrier and the lymphatic drainage system of the brain. Front. Cell Neurosci. 15:716825. doi: 10.3389/fncel.2021.716825
Cheng, X., Shi, J., Wu, H., Zhu, W., and Lu, G. (2022). Review of net water uptake in the management of acute ischemic stroke. Eur. Radiol. 32, 5517–5524. doi: 10.1007/s00330-022-08658-x
Clement, T., Rodriguez-Grande, B., and Badaut, J. (2020). Aquaporins in brain edema. J. Neurosci. Res. 98, 9–18. doi: 10.1002/jnr.24354
Cui, J., Chen, S., Zhang, C., Meng, F., Wu, W., Hu, R., et al. (2012). Inhibition of MMP-9 by a selective gelatinase inhibitor protects neurovasculature from embolic focal cerebral ischemia. Mol. Neurodegener. 7:21. doi: 10.1186/1750-1326-7-21
Darabi, S., and Mohammadi, M. T. (2017). Fullerenol nanoparticles decrease ischaemia-induced brain injury and oedema through inhibition of oxidative damage and aquaporin-1 expression in ischaemic stroke. Brain Inj. 31, 1142–1150. doi: 10.1080/02699052.2017.1300835
Datta, A., Sarmah, D., Kaur, H., Chaudhary, A., Mounica, K. L., Kalia, K., et al. (2022). Post-stroke impairment of the blood-brain barrier and perifocal vasogenic edema is alleviated by endovascular mesenchymal stem cell administration: Modulation of the PKCdelta/MMP9/AQP4-mediated pathway. Mol. Neurobiol. 59, 2758–2775. doi: 10.1007/s12035-022-02761-2
Deng, L. D., Qi, L., Suo, Q., Wu, S. J., Mamtilahun, M., Shi, R. B., et al. (2022). Transcranial focused ultrasound stimulation reduces vasogenic edema after middle cerebral artery occlusion in mice. Neural Regen. Res. 17, 2058–2063. doi: 10.4103/1673-5374.335158
Dhanesha, N., Jain, M., Tripathi, A. K., Doddapattar, P., Chorawala, M., Bathla, G., et al. (2020). Targeting myeloid-specific integrin alpha9beta1 improves short- and long-term stroke outcomes in murine models with preexisting comorbidities by limiting thrombosis and inflammation. Circ. Res. 126, 1779–1794. doi: 10.1161/CIRCRESAHA.120.316659
Dore-Duffy, P., Wang, X., Mehedi, A., Kreipke, C. W., and Rafols, J. A. (2007). Differential expression of capillary VEGF isoforms following traumatic brain injury. Neurol Res. 29, 395–403. doi: 10.1179/016164107X204729
Eltzschig, H. K., and Eckle, T. (2011). Ischemia and reperfusion–from mechanism to translation. Nat. Med. 17, 1391–1401. doi: 10.1038/nm.2507
Faizy, T. D., Kabiri, R., Christensen, S., Mlynash, M., Kuraitis, G., Mader, M. M., et al. (2021a). Association of venous outflow profiles and successful vessel reperfusion after thrombectomy. Neurology 96, e2903–e2911. doi: 10.1212/WNL.0000000000012106
Faizy, T. D., Kabiri, R., Christensen, S., Mlynash, M., Kuraitis, G., Meyer, L., et al. (2021b). Venous outflow profiles are linked to cerebral edema formation at noncontrast head ct after treatment in acute ischemic stroke regardless of collateral vessel status at CT angiography. Radiology 299, 682–690. doi: 10.1148/radiol.2021203651
Faizy, T. D., Kabiri, R., Christensen, S., Mlynash, M., Kuraitis, G. M., Broocks, G., et al. (2021c). Favorable venous outflow profiles correlate with favorable tissue-level collaterals and clinical outcome. Stroke 52, 1761–1767. doi: 10.1161/STROKEAHA.120.032242
Feng, Z., Sun, Q., Chen, W., Bai, Y., Hu, D., and Xie, X. (2019). The neuroprotective mechanisms of ginkgolides and bilobalide in cerebral ischemic injury: A literature review. Mol. Med. 25:57. doi: 10.1186/s10020-019-0125-y
Fisher, M., and Savitz, S. I. (2022). Pharmacological brain cytoprotection in acute ischaemic stroke - renewed hope in the reperfusion era. Nat. Rev. Neurol. 18, 193–202. doi: 10.1038/s41582-021-00605-6
Fonseca, S., Costa, F., Seabra, M., Dias, R., Soares, A., Dias, C., et al. (2021). Systemic inflammation status at admission affects the outcome of intracerebral hemorrhage by increasing perihematomal edema but not the hematoma growth. Acta Neurol. Belg. 121, 649–659. doi: 10.1007/s13760-019-01269-2
Garcia, J. G., Siflinger-Birnboim, A., Bizios, R., Del Vecchio, P. J., Fenton, J. W. II, and Malik, A. B. (1986). Thrombin-induced increase in albumin permeability across the endothelium. J. Cell Physiol. 128, 96–104. doi: 10.1002/jcp.1041280115
Garcia-Culebras, A., Duran-Laforet, V., Pena-Martinez, C., Moraga, A., Ballesteros, I., Cuartero, M. I., et al. (2019). Role of TLR4 (Toll-Like Receptor 4) in N1/N2 neutrophil programming after stroke. Stroke 50, 2922–2932. doi: 10.1161/STROKEAHA.119.025085
Gerriets, T., Stolz, E., Konig, S., Babacan, S., Fiss, I., Jauss, M., et al. (2001). Sonographic monitoring of midline shift in space-occupying stroke: An early outcome predictor. Stroke 32, 442–447. doi: 10.1161/01.str.32.2.442
Gerzanich, V., Stokum, J. A., Ivanova, S., Woo, S. K., Tsymbalyuk, O., Sharma, A., et al. (2019). Sulfonylurea receptor 1, transient receptor potential cation channel subfamily M member 4, and KIR6.2:Role in hemorrhagic progression of contusion. J. Neurotrauma 36, 1060–1079. doi: 10.1089/neu.2018.5986
Gopalakrishnan, M. S., Shanbhag, N. C., Shukla, D. P., Konar, S. K., Bhat, D. I., and Devi, B. I. (2018). Complications of decompressive craniectomy. Front. Neurol. 9:977. doi: 10.3389/fneur.2018.00977
Gorelick, D. A., Praetorius, J., Tsunenari, T., Nielsen, S., and Agre, P. (2006). Aquaporin-11: A channel protein lacking apparent transport function expressed in brain. BMC Biochem. 7:14. doi: 10.1186/1471-2091-7-14
Halstead, M. R., and Geocadin, R. G. (2019). The medical management of cerebral edema: Past, present, and future therapies. Neurotherapeutics 16, 1133–1148. doi: 10.1007/s13311-019-00779-4
Hamann, S., Herrera-Perez, J. J., Zeuthen, T., and Alvarez-Leefmans, F. J. (2010). Cotransport of water by the Na+-K+-2Cl(-) cotransporter NKCC1 in mammalian epithelial cells. J. Physiol. 588(Pt 21), 4089–4101. doi: 10.1113/jphysiol.2010.194738
He, H. Y., Ren, L., Guo, T., and Deng, Y. H. (2019). Neuronal autophagy aggravates microglial inflammatory injury by downregulating CX3CL1/fractalkine after ischemic stroke. Neural Regen. Res. 14, 280–288. doi: 10.4103/1673-5374.244793
Hou, Y., Yang, D., Xiang, R., Wang, H., Wang, X., Zhang, H., et al. (2019). N2 neutrophils may participate in spontaneous recovery after transient cerebral ischemia by inhibiting ischemic neuron injury in rats. Int. Immunopharmacol. 77:105970. doi: 10.1016/j.intimp.2019.105970
Howell, J. A., and Bidwell, G. L. III (2020). Targeting the NF-kappaB pathway for therapy of ischemic stroke. Ther. Deliv. 11, 113–123. doi: 10.4155/tde-2019-0075
Huang, K., Hu, Y., Wu, Y., Ji, Z., Wang, S., Lin, Z., et al. (2019). Exploratory analysis of oral glibenclamide in acute ischemic stroke. Acta Neurol. Scand. 140, 212–218. doi: 10.1111/ane.13134
Jha, R. M., Kochanek, P. M., and Simard, J. M. (2019). Pathophysiology and treatment of cerebral edema in traumatic brain injury. Neuropharmacology 145, 230–246. doi: 10.1016/j.neuropharm.2018.08.004
Jha, R. M., Rani, A., Desai, S. M., Raikwar, S., Mihaljevic, S., Munoz-Casabella, A., et al. (2021). Sulfonylurea receptor 1 in central nervous system injury: An updated review. Int. J. Mol Sci 22:11899. doi: 10.3390/ijms222111899
Ji, C., Yu, X., Xu, W., Lenahan, C., Tu, S., and Shao, A. (2021). The role of glymphatic system in the cerebral edema formation after ischemic stroke. Exp. Neurol. 340:113685. doi: 10.1016/j.expneurol.2021.113685
Jiang, X., Andjelkovic, A. V., Zhu, L., Yang, T., Bennett, M. V. L., Chen, J., et al. (2018). Blood-brain barrier dysfunction and recovery after ischemic stroke. Prog. Neurobiol. 16, 144–171. doi: 10.1016/j.pneurobio.2017.10.001
Jurcau, A., and Simion, A. (2021). Neuroinflammation in cerebral ischemia and ischemia/reperfusion injuries: From pathophysiology to therapeutic strategies. Int. J. Mol. Sci 23:14. doi: 10.3390/ijms23010014
Kanazawa, M., Takahashi, T., Nishizawa, M., and Shimohata, T. (2017). Therapeutic strategies to attenuate hemorrhagic transformation after tissue plasminogen activator treatment for acute ischemic stroke. J. Atheroscler. Thromb. 24, 240–253. doi: 10.5551/jat.RV16006
Ke, X. Y., Hou, W., Huang, Q., Hou, X., Bao, X. Y., Kong, W. X., et al. (2022). Advances in electrical impedance tomography-based brain imaging. Mil. Med. Res. 9:10. doi: 10.1186/s40779-022-00370-7
Keaney, J., and Campbell, M. (2015). The dynamic blood-brain barrier. FEBS J. 282, 4067–4079. doi: 10.1111/febs.13412
Kim, E. H., Kim, E. S., Shin, D., Kim, D., Choi, S., Shin, Y. J., et al. (2021). Carnosine protects against cerebral ischemic injury by inhibiting matrix-metalloproteinases. Int. J. Mol. Sci. 22:7495. doi: 10.3390/ijms22147495
Kim, E., and Cho, S. (2016). Microglia and monocyte-derived macrophages in stroke. Neurotherapeutics 13, 702–718. doi: 10.1007/s13311-016-0463-1
Kimberly, W. T., Bevers, M. B., von Kummer, R., Demchuk, A. M., Romero, J. M., Elm, J. J., et al. (2018a). Effect of IV glyburide on adjudicated edema endpoints in the GAMES-RP Trial. Neurology 91, e2163–e2169. doi: 10.1212/WNL.0000000000006618
Kimberly, W. T., Dutra, B. G., Boers, A. M. M., Alves, H., Berkhemer, O. A., van den Berg, L., et al. (2018b). Association of reperfusion with brain edema in patients with acute ischemic stroke: A secondary analysis of the MR CLEAN trial. JAMA Neurol. 75, 453–461. doi: 10.1001/jamaneurol.2017.5162
Kitchen, P., Salman, M. M., Halsey, A. M., Clarke-Bland, C., MacDonald, J. A., Ishida, H., et al. (2020). Targeting aquaporin-4 subcellular localization to treat central nervous system edema. Cell 181, 784–799e719. doi: 10.1016/j.cell.2020.03.037
Klein, T., and Bischoff, R. (2011). Physiology and pathophysiology of matrix metalloproteases. Amino Acids 41, 271–290. doi: 10.1007/s00726-010-0689-x
Kovacs, Z., Ikezaki, K., Samoto, K., Inamura, T., and Fukui, M. (1996). VEGF and flt. Expression time kinetics in rat brain infarct. Stroke 27, 1865–1872. doi: 10.1161/01.str.27.10.1865
Kumar, A., Chen, Y., Corbin, A., Hamzehloo, A., Abedini, A., Vardar, Z., et al. (2022). Automated measurement of net water uptake from baseline and follow-up CTs in patients with large vessel occlusion stroke. Front. Neurol. 13:898728. doi: 10.3389/fneur.2022.898728
Kurzepa, J., Kurzepa, J., Golab, P., Czerska, S., and Bielewicz, J. (2014). The significance of matrix metalloproteinase (MMP)-2 and MMP-9 in the ischemic stroke. Int. J. Neurosci. 124, 707–716. doi: 10.3109/00207454.2013.872102
L, L., X, W., and Z, Y. (2016). Ischemia-reperfusion injury in the brain: Mechanisms and potential therapeutic strategies. Biochem. Pharmacol. (Los Angel) 5:213. doi: 10.4172/2167-0501.1000213
Li, G., Morris-Blanco, K. C., Lopez, M. S., Yang, T., Zhao, H., Vemuganti, R., et al. (2018). Impact of microRNAs on ischemic stroke: From pre- to post-disease. Prog. Neurobiol. 16, 59–78. doi: 10.1016/j.pneurobio.2017.08.002
Li, S., Li, Y., Huang, S., Fan, B., Wei, J., Ouyang, L., et al. (2020). Silencing matrix metalloproteinase 9 exerts a protective effect on astrocytes after oxygen-glucose deprivation and is correlated with suppression of aquaporin-4. Neurosci. Lett. 731:135047. doi: 10.1016/j.neulet.2020.135047
Liebeskind, D. S., Juttler, E., Shapovalov, Y., Yegin, A., Landen, J., and Jauch, E. C. (2019). Cerebral edema associated with large hemispheric infarction. Stroke 50, 2619–2625. doi: 10.1161/STROKEAHA.118.024766
Liesz, A., Dalpke, A., Mracsko, E., Antoine, D. J., Roth, S., Zhou, W., et al. (2015). DAMP signaling is a key pathway inducing immune modulation after brain injury. J. Neurosci. 35, 583–598. doi: 10.1523/JNEUROSCI.2439-14.2015
Lin, S. Y., Wang, Y. Y., Chang, C. Y., Wu, C. C., Chen, W. Y., Liao, S. L., et al. (2021). TNF-alpha receptor inhibitor alleviates metabolic and inflammatory changes in a rat model of ischemic stroke. Antioxidants (Basel) 10:851. doi: 10.3390/antiox10060851
Liu, E., Sun, L., Zhang, Y., Wang, A., and Yan, J. (2020). Aquaporin4 knockout aggravates early brain injury following subarachnoid hemorrhage through impairment of the glymphatic system in rat brain. Acta Neurochir. Suppl. 127, 59–64. doi: 10.1007/978-3-030-04615-6_10
Liu, J., Jiang, Y., Zhang, G., Lin, Z., and Du, S. (2019). Protective effect of edaravone on blood-brain barrier by affecting NRF-2/HO-1 signaling pathway. Exp. Ther. Med. 18, 2437–2442. doi: 10.3892/etm.2019.7859
Loh, K. Y., Wang, Z., and Liao, P. (2019). Oncotic Cell Death in Stroke. Rev. Physiol. Biochem. Pharmacol. 176, 37–64. doi: 10.1007/112_2018_13
MacAulay, N. (2021). Molecular mechanisms of brain water transport. Nat. Rev. Neurosci. 22, 326–344. doi: 10.1038/s41583-021-00454-8
MacAulay, N., and Zeuthen, T. (2010). Water transport between CNS compartments: Contributions of aquaporins and cotransporters. Neuroscience 168, 941–956. doi: 10.1016/j.neuroscience.2009.09.016
Malone, K., Amu, S., Moore, A. C., and Waeber, C. (2019). The immune system and stroke: From current targets to future therapy. Immunol. Cell Biol. 97, 5–16. doi: 10.1111/imcb.12191
McKeown, M. E., Prasad, A., Kobsa, J., Top, I., Snider, S. B., Kidwell, C., et al. (2022). Midline shift greater than 3 mm independently predicts outcome after ischemic stroke. Neurocrit. Care 36, 46–51. doi: 10.1007/s12028-021-01341-x
Mechtouff, L., Bochaton, T., Paccalet, A., Crola Da Silva, C., Buisson, M., Amaz, C., et al. (2020). Matrix metalloproteinase-9 relationship with infarct growth and hemorrhagic transformation in the era of thrombectomy. Front. Neurol. 11:473. doi: 10.3389/fneur.2020.00473
Mehta, R. I., Ivanova, S., Tosun, C., Castellani, R. J., Gerzanich, V., and Simard, J. M. (2013). Sulfonylurea receptor 1 expression in human cerebral infarcts. J. Neuropathol. Exp. Neurol. 72, 871–883. doi: 10.1097/NEN.0b013e3182a32e40
Mestre, H., Du, T., Sweeney, A. M., Liu, G., Samson, A. J., Peng, W., et al. (2020). Cerebrospinal fluid influx drives acute ischemic tissue swelling. Science 367:eaax7171. doi: 10.1126/science.aax7171
Minnerup, J., Broocks, G., Kalkoffen, J., Langner, S., Knauth, M., Psychogios, M. N., et al. (2016). Computed tomography-based quantification of lesion water uptake identifies patients within 4.5 hours of stroke onset: A multicenter observational study. Ann. Neurol. 80, 924–934. doi: 10.1002/ana.24818
Mori, K., Miyazaki, M., Iwase, H., and Maeda, M. (2002). Temporal profile of changes in brain tissue extracellular space and extracellular ion (Na(+), K(+)) concentrations after cerebral ischemia and the effects of mild cerebral hypothermia. J. Neurotrauma 19, 1261–1270. doi: 10.1089/08977150260338047
Moy, A. B., Van Engelenhoven, J., Bodmer, J., Kamath, J., Keese, C., Giaever, I., et al. (1996). Histamine and thrombin modulate endothelial focal adhesion through centripetal and centrifugal forces. J. Clin. Invest. 97, 1020–1027. doi: 10.1172/JCI118493
Mulder, I. A., van Bavel, E. T., de Vries, H. E., and Coutinho, J. M. (2021). Adjunctive cytoprotective therapies in acute ischemic stroke: A systematic review. Fluids Barriers CNS 18:46. doi: 10.1186/s12987-021-00280-1
Nawabi, J., Flottmann, F., Hanning, U., Bechstein, M., Schon, G., Kemmling, A., et al. (2019). Futile recanalization with poor clinical outcome is associated with increased edema volume after ischemic stroke. Invest. Radiol. 54, 282–287. doi: 10.1097/RLI.0000000000000539
Ng, F. C., Churilov, L., Yassi, N., Kleinig, T. J., Thijs, V., Wu, T. Y., et al. (2021a). Association between pre-treatment perfusion profile and cerebral edema after reperfusion therapies in ischemic stroke. J. Cereb. Blood Flow Metab. 41, 2887–2896. doi: 10.1177/0271678X211017696
Ng, F. C., Churilov, L., Yassi, N., Kleinig, T. J., Thijs, V., Wu, T. Y., et al. (2022a). Microvascular dysfunction in blood-brain barrier disruption and hypoperfusion within the infarct posttreatment are associated with cerebral edema. Stroke 53, 1597–1605. doi: 10.1161/STROKEAHA.121.036104
Ng, F. C., Yassi, N., Sharma, G., Brown, S. B., Goyal, M., Majoie, C., et al. (2021b). Cerebral edema in patients with large hemispheric infarct undergoing reperfusion treatment: A HERMES meta-analysis. Stroke 52, 3450–3458. doi: 10.1161/STROKEAHA.120.033246
Ng, F. C., Yassi, N., Sharma, G., Brown, S. B., Goyal, M., Majoie, C., et al. (2022b). Correlation between computed tomography-based tissue net water uptake and volumetric measures of cerebral edema after reperfusion therapy. Stroke 53, 2628–2636. doi: 10.1161/STROKEAHA.121.037073
Obenaus, A., and Badaut, J. (2022). Role of the non-invasive imaging techniques in monitoring and understanding the evolution of brain edema. J. Neurosci. Res. 100, 1191–1200. doi: 10.1002/jnr.24837
Obenaus, A., and Jacobs, R. E. (2007). Magnetic resonance imaging of functional anatomy: Use for small animal epilepsy models. Epilepsia 48(Suppl. 4), 11–17. doi: 10.1111/j.1528-1167.2007.01237.x
Ostwaldt, A. C., Battey, T. W. K., Irvine, H. J., Campbell, B. C. V., Davis, S. M., Donnan, G. A., et al. (2018). Comparative analysis of markers of mass effect after ischemic stroke. J. Neuroimaging 28, 530–534. doi: 10.1111/jon.12525
Pang, C. C. (2001). Autonomic control of the venous system in health and disease: Effects of drugs. Pharmacol. Ther. 90, 179–230. doi: 10.1016/s0163-7258(01)00138-3
Papadopoulos, M. C., and Verkman, A. S. (2013). Aquaporin water channels in the nervous system. Nat. Rev. Neurosci. 14, 265–277. doi: 10.1038/nrn3468
Pergakis, M., Badjatia, N., Chaturvedi, S., Cronin, C. A., Kimberly, W. T., Sheth, K. N., et al. (2019). BIIB093 (IV glibenclamide): An investigational compound for the prevention and treatment of severe cerebral edema. Expert Opin. Investig. Drugs 28, 1031–1040. doi: 10.1080/13543784.2019.1681967
Pillai, D. R., Dittmar, M. S., Baldaranov, D., Heidemann, R. M., Henning, E. C., Schuierer, G., et al. (2009). Cerebral ischemia-reperfusion injury in rats–a 3 T MRI study on biphasic blood-brain barrier opening and the dynamics of edema formation. J. Cereb. Blood Flow Metab. 29, 1846–1855. doi: 10.1038/jcbfm.2009.106
Pirici, I., Balsanu, T. A., Bogdan, C., Margaritescu, C., Divan, T., Vitalie, V., et al. (2017). Inhibition of aquaporin-4 improves the outcome of ischaemic stroke and modulates brain paravascular drainage pathways. Int. J. Mol. Sci. 19:46. doi: 10.3390/ijms19010046
Qiu, Y. M., Zhang, C. L., Chen, A. Q., Wang, H. L., Zhou, Y. F., Li, Y. N., et al. (2021). Immune cells in the BBB disruption after acute ischemic stroke: Targets for immune therapy? Front. Immunol. 12:678744. doi: 10.3389/fimmu.2021.678744
Rabinstein, A. A. (2017). Treatment of acute ischemic stroke. Continuum (Minneap Minn) 23, 62–81. doi: 10.1212/CON.0000000000000420
Ropper, A. H. (1986). Lateral displacement of the brain and level of consciousness in patients with an acute hemispheral mass. N. Engl. J. Med. 314, 953–958. doi: 10.1056/NEJM198604103141504
Sadana, P., Coughlin, L., Burke, J., Woods, R., and Mdzinarishvili, A. (2015). Anti-edema action of thyroid hormone in MCAO model of ischemic brain stroke: Possible association with AQP4 modulation. J. Neurol. Sci. 354, 37–45. doi: 10.1016/j.jns.2015.04.042
Sadeghian, N., Shadman, J., Moradi, A., Ghasem Golmohammadi, M., and Panahpour, H. (2019). Calcitriol protects the Blood-Brain Barrier integrity against ischemic stroke and reduces vasogenic brain edema via antioxidant and antiapoptotic actions in rats. Brain Res. Bull. 150, 281–289. doi: 10.1016/j.brainresbull.2019.06.010
Selvamani, A., Sathyan, P., Miranda, R. C., and Sohrabji, F. (2012). An antagomir to microRNA Let7f promotes neuroprotection in an ischemic stroke model. PLoS One 7:e32662. doi: 10.1371/journal.pone.0032662
Shah, A., Almenawer, S., and Hawryluk, G. (2019). Timing of decompressive craniectomy for ischemic stroke and traumatic brain injury: A review. Front. Neurol. 10:11. doi: 10.3389/fneur.2019.00011
Sheth, K. N., Elm, J. J., Molyneaux, B. J., Hinson, H., Beslow, L. A., Sze, G. K., et al. (2016). Safety and efficacy of intravenous glyburide on brain swelling after large hemispheric infarction (GAMES-RP): A randomised, double-blind, placebo-controlled phase 2 trial. Lancet Neurol. 15, 1160–1169. doi: 10.1016/S1474-4422(16)30196-X
Sheth, K. N., Kimberly, W. T., Elm, J. J., Kent, T. A., Yoo, A. J., Thomalla, G., et al. (2014). Exploratory analysis of glyburide as a novel therapy for preventing brain swelling. Neurocrit. Care 21, 43–51. doi: 10.1007/s12028-014-9970-2
Sheth, K. N., Petersen, N. H., Cheung, K., Elm, J. J., Hinson, H. E., Molyneaux, B. J., et al. (2018). Long-term outcomes in patients aged </=70 years with intravenous glyburide from the phase II GAMES-RP study of large hemispheric infarction: An exploratory analysis. Stroke 49, 1457–1463. doi: 10.1161/STROKEAHA.117.020365
Shi, Y., Zhang, L., Pu, H., Mao, L., Hu, X., Jiang, X., et al. (2016). Rapid endothelial cytoskeletal reorganization enables early blood-brain barrier disruption and long-term ischaemic reperfusion brain injury. Nat. Commun. 7:10523. doi: 10.1038/ncomms10523
Sifat, A. E., Vaidya, B., and Abbruscato, T. J. (2017). Blood-brain barrier protection as a therapeutic strategy for acute ischemic stroke. AAPS J. 19, 957–972. doi: 10.1208/s12248-017-0091-7
Simard, J. M., Kent, T. A., Chen, M., Tarasov, K. V., and Gerzanich, V. (2007). Brain oedema in focal ischaemia: Molecular pathophysiology and theoretical implications. Lancet Neurol. 6, 258–268. doi: 10.1016/S1474-4422(07)70055-8
Skold, M. K., von Gertten, C., Sandberg-Nordqvist, A. C., Mathiesen, T., and Holmin, S. (2005). VEGF and VEGF receptor expression after experimental brain contusion in rat. J. Neurotrauma 22, 353–367. doi: 10.1089/neu.2005.22.353
Spasov, A. A., Murav’eva, V. U., Gurova, N. A., Cheplyaeva, N. I., Reznikov, E. V., and Anisimova, V. A. (2016). Neuroprotective properties of a new inhibitor of Na+/H+ Exchanger (Compound Ru-1355) on the Model of Focal Ischemia in Rats. Eksp. Klin. Farmakol. 79, 3–7.
Starling, E. H. (1896). On the absorption of fluids from the connective tissue spaces. J. Physiol. 19, 312–326. doi: 10.1113/jphysiol.1896.sp000596
Stokum, J. A., Gerzanich, V., and Simard, J. M. (2016). Molecular pathophysiology of cerebral edema. J. Cereb. Blood Flow Metab. 36, 513–538. doi: 10.1177/0271678X15617172
Stokum, J. A., Gerzanich, V., Sheth, K. N., Kimberly, W. T., and Simard, J. M. (2020). Emerging Pharmacological treatments for cerebral edema: Evidence from clinical studies. Ann. Rev. Pharmacol. Toxicol. 60, 291–309. doi: 10.1146/annurev-pharmtox-010919-023429
Stokum, J. A., Kurland, D. B., Gerzanich, V., and Simard, J. M. (2015). Mechanisms of astrocyte-mediated cerebral edema. Neurochem. Res. 40, 317–328. doi: 10.1007/s11064-014-1374-3
Stokum, J. A., Kwon, M. S., Woo, S. K., Tsymbalyuk, O., Vennekens, R., Gerzanich, V., et al. (2018). SUR1-TRPM4 and AQP4 form a heteromultimeric complex that amplifies ion/water osmotic coupling and drives astrocyte swelling. Glia 66, 108–125. doi: 10.1002/glia.23231
Sun, M. S., Jin, H., Sun, X., Huang, S., Zhang, F. L., Guo, Z. N., et al. (2018). Free radical damage in ischemia-reperfusion injury: An obstacle in acute ischemic stroke after revascularization therapy. Oxid Med. Cell Longev 2018:3804979. doi: 10.1155/2018/3804979
Swanson, J. A., and Watts, C. (1995). Macropinocytosis. Trends Cell Biol. 5, 424–428. doi: 10.1016/s0962-8924(00)89101-1
Talebi, A., Rahnema, M., and Bigdeli, M. R. (2019). Effect of intravenous injection of antagomiR-1 on brain ischemia. Mol. Biol. Rep. 46, 1149–1155. doi: 10.1007/s11033-018-04580-y
Torre-Healy, A., Marko, N. F., and Weil, R. J. (2012). Hyperosmolar therapy for intracranial hypertension. Neurocrit. Care 17, 117–130. doi: 10.1007/s12028-011-9649-x
Turner, R. J., and Sharp, F. R. (2016). Implications of MMP9 for blood brain barrier disruption and hemorrhagic transformation following ischemic stroke. Front. Cell Neurosci. 10:56. doi: 10.3389/fncel.2016.00056
Vella, J., Zammit, C., Di Giovanni, G., Muscat, R., and Valentino, M. (2015). The central role of aquaporins in the pathophysiology of ischemic stroke. Front. Cell Neurosci. 9:108. doi: 10.3389/fncel.2015.00108
Virani, S. S., Alonso, A., Aparicio, H. J., Benjamin, E. J., Bittencourt, M. S., Callaway, C. W., et al. (2021). Heart disease and stroke statistics-2021 update: A report from the american heart association. Circulation 143, e254–e743. doi: 10.1161/CIR.0000000000000950
Volny, O., Cimflova, P., and Mikulik, R. (2016). Ipsilateral sinus hypoplasia and poor leptomeningeal collaterals as midline shift predictors. J. Stroke Cerebrovasc. Dis. 25, 1792–1796. doi: 10.1016/j.jstrokecerebrovasdis.2016.04.004
Vorasayan, P., Bevers, M. B., Beslow, L. A., Sze, G., Molyneaux, B. J., Hinson, H. E., et al. (2019). Intravenous glibenclamide reduces lesional water uptake in large hemispheric infarction. Stroke 50, 3021–3027. doi: 10.1161/STROKEAHA.119.026036
Walberer, M., Blaes, F., Stolz, E., Muller, C., Schoenburg, M., Tschernatsch, M., et al. (2007). Midline-shift corresponds to the amount of brain edema early after hemispheric stroke–an MRI study in rats. J. Neurosurg. Anesthesiol. 19, 105–110. doi: 10.1097/ANA.0b013e31802c7e33
Wang, G., Yuan, Y., Zhang, J., Gao, L., Tan, X., Yang, G., et al. (2014). Roles of aquaporins and matrix metalloproteinases in mouse brain edema formation induced by subacute exposure to 1,2-dichloroethane. Neurotoxicol. Teratol. 44, 105–112. doi: 10.1016/j.ntt.2014.06.005
Wang, J., Ma, C., Zhu, J., Rao, G., and Li, H. (2020a). Effect of 3-aminobenzamide on the ultrastructure of astrocytes and microvessels after focal cerebral ischemia in rats. Dose Response 18:1559325819901242. doi: 10.1177/1559325819901242
Wang, L., Deng, L., Yuan, R., Liu, J., Li, Y., and Liu, M. (2020b). Association of matrix metalloproteinase 9 and cellular fibronectin and outcome in acute ischemic stroke: A systematic review and meta-analysis. Front. Neurol. 11:523506. doi: 10.3389/fneur.2020.523506
Wang, Q., Deng, Y., Huang, L., Zeng, W., Chen, S., Lv, B., et al. (2019). Hypertonic saline downregulates endothelial cell-derived VEGF expression and reduces blood-brain barrier permeability induced by cerebral ischaemia via the VEGFR2/eNOS pathway. Int. J. Mol. Med. 44, 1078–1090. doi: 10.3892/ijmm.2019.4262
Wang, X., Chang, Y., He, Y., Lyu, C., Li, H., Zhu, J., et al. (2020c). Glimepiride and glibenclamide have comparable efficacy in treating acute ischemic stroke in mice. Neuropharmacology 162:107845. doi: 10.1016/j.neuropharm.2019.107845
Wang, Y., Hong, F., and Yang, S. (2022). Roles of nitric oxide in brain ischemia and reperfusion. Int. J. Mol. Sci. 23, 4243. doi: 10.3390/ijms23084243
Warach, S., Mosley, M., Sorensen, A. G., and Koroshetz, W. (1996). Time course of diffusion imaging abnormalities in human stroke. Stroke 27, 1254–1256.
Wei, X., Zhang, B., Cheng, L., Chi, M., Deng, L., Pan, H., et al. (2015). Hydrogen sulfide induces neuroprotection against experimental stroke in rats by down-regulation of AQP4 via activating PKC. Brain Res. 1622, 292–299. doi: 10.1016/j.brainres.2015.07.001
Winkler, L., Blasig, R., Breitkreuz-Korff, O., Berndt, P., Dithmer, S., Helms, H. C., et al. (2021). Tight junctions in the blood-brain barrier promote edema formation and infarct size in stroke - Ambivalent effects of sealing proteins. J. Cereb. Blood Flow Metab. 41, 132–145. doi: 10.1177/0271678X20904687
Woo, S. K., Kwon, M. S., Geng, Z., Chen, Z., Ivanov, A., Bhatta, S., et al. (2012). Sequential activation of hypoxia-inducible factor 1 and specificity protein 1 is required for hypoxia-induced transcriptional stimulation of Abcc8. J. Cereb. Blood Flow Metab. 32, 525–536. doi: 10.1038/jcbfm.2011.159
Woo, S. K., Kwon, M. S., Ivanov, A., Gerzanich, V., and Simard, J. M. (2013). The sulfonylurea receptor 1 (Sur1)-transient receptor potential melastatin 4 (Trpm4) channel. J. Biol. Chem. 288, 3655–3667. doi: 10.1074/jbc.M112.428219
Wu, W., Zhong, W., Lang, B., Hu, Z., He, J., and Tang, X. (2018). Thrombopoietin could protect cerebral tissue against ischemia-reperfusion injury by suppressing NF-kappaB and MMP-9 expression in rats. Int. J. Med. Sci. 15, 1341–1348. doi: 10.7150/ijms.27543
Xing, L., Wang, B., Li, J., Guo, X., Lu, X., Chen, X., et al. (2022). A Fluorogenic ONOO(-)-Triggered carbon monoxide donor for mitigating brain ischemic damage. J. Am. Chem. Soc. 144, 2114–2119. doi: 10.1021/jacs.2c00094
Xiong, M., Feng, Y., Huang, S., Lv, S., Deng, Y., Li, M., et al. (2022). Teriparatide induces angiogenesis in ischemic cerebral infarction zones of rats through AC/PKA signaling and reduces ischemia-reperfusion injury. Biomed. Pharmacother. 148:112728. doi: 10.1016/j.biopha.2022.112728
Yang, B., Li, B., Xu, C., Hu, S., Dai, M., Xia, J., et al. (2019). Comparison of electrical impedance tomography and intracranial pressure during dehydration treatment of cerebral edema. Neuroimage Clin. 23:101909. doi: 10.1016/j.nicl.2019.101909
Yang, J., Yang, B., Xiu, B., Qi, J., and Liu, H. (2018). Effect of combination therapy with neuroprotective and vasoprotective agents on cerebral ischemia. Can. J. Neurol. Sci. 45, 325–331. doi: 10.1017/cjn.2018.8
Yang, Y., and Rosenberg, G. A. (2011). Blood-brain barrier breakdown in acute and chronic cerebrovascular disease. Stroke 42, 3323–3328. doi: 10.1161/STROKEAHA.110.608257
Yao, Y., Zhang, Y., Liao, X., Yang, R., Lei, Y., and Luo, J. (2020). Potential therapies for cerebral edema after ischemic stroke: A mini review. Front. Aging Neurosci. 12:618819. doi: 10.3389/fnagi.2020.618819
Yu, L., Fan, S. J., Liu, L., Xiao, M., Lin, X. J., Liu, Y., et al. (2015). Effect of ischemic postconditioning on cerebral edema and the AQP4 expression following hypoxic-eschemic brain damage in neonatal rats. World J. Pediatr. 11, 165–170. doi: 10.1007/s12519-014-0519-4
Yu, W., Rives, J., Welch, B., White, J., Stehel, E., and Samson, D. (2009). Hypoplasia or occlusion of the ipsilateral cranial venous drainage is associated with early fatal edema of middle cerebral artery infarction. Stroke 40, 3736–3739. doi: 10.1161/STROKEAHA.109.563080
Yu, X., and Li, X. (2020). microRNA-1906 protects cerebral ischemic injury through activating Janus kinase 2/signal transducer and activator of transcription 3 pathway in rats. Neuroreport 31, 871–878. doi: 10.1097/WNR.0000000000001456
Yu, X., Ji, C., and Shao, A. (2020). Neurovascular unit dysfunction and neurodegenerative disorders. Front. Neurosci. 14:334. doi: 10.3389/fnins.2020.00334
Yu, X., Yuan, L., Jackson, A., Sun, J., Huang, P., Xu, X., et al. (2016). Prominence of medullary veins on susceptibility-weighted images provides prognostic information in patients with subacute stroke. AJNR Am. J. Neuroradiol. 37, 423–429. doi: 10.3174/ajnr.A4541
Zeuthen, T., Meinild, A. K., Klaerke, D. A., Loo, D. D., Wright, E. M., Belhage, B., et al. (1997). Water transport by the Na+/glucose cotransporter under isotonic conditions. Biol. Cell 89, 307–312. doi: 10.1016/s0248-4900(97)83383-7
Zhang, C., Jiang, M., Wang, W. Q., Zhao, S. J., Yin, Y. X., Mi, Q. J., et al. (2020a). Selective mGluR1 negative allosteric modulator reduces blood-brain barrier permeability and cerebral edema after experimental subarachnoid hemorrhage. Transl. Stroke Res. 11, 799–811. doi: 10.1007/s12975-019-00758-z
Zhang, J., Bhuiyan, M. I. H., Zhang, T., Karimy, J. K., Wu, Z., Fiesler, V. M., et al. (2020b). Modulation of brain cation-Cl(-) cotransport via the SPAK kinase inhibitor ZT-1a. Nat Commun 11:78. doi: 10.1038/s41467-019-13851-6
Zhang, S., Lin, L., Zhang, R., Wang, M., Yu, Y., Shi, Z., et al. (2020c). Absent contrast filling of ipsilateral superficial middle cerebral vein predicts midline shift in acute middle cerebral artery occlusion. Front. Neurol. 11:570844. doi: 10.3389/fneur.2020.570844
Zhang, W., Neal, J., Lin, L., Dai, F., Hersey, D. P., McDonagh, D. L., et al. (2019). Mannitol in critical care and surgery over 50+ years: A systematic review of randomized controlled trials and complications with meta-analysis. J. Neurosurg. Anesthesiol. 31, 273–284. doi: 10.1097/ANA.0000000000000520
Zhang, W., Zhu, L., An, C., Wang, R., Yang, L., Yu, W., et al. (2020d). The blood brain barrier in cerebral ischemic injury–Disruption and repair. Brain Hemorrhages 1, 34–53. doi: 10.1016/j.hest.2019.12.004
Zhang, Z. G., Zhang, L., Jiang, Q., Zhang, R., Davies, K., Powers, C., et al. (2000). VEGF enhances angiogenesis and promotes blood-brain barrier leakage in the ischemic brain. J. Clin. Invest. 106, 829–838. doi: 10.1172/JCI9369
Zhou, Y. F., Li, Y. N., Jin, H. J., Wu, J. H., He, Q. W., Wang, X. X., et al. (2018). Sema4D/PlexinB1 inhibition ameliorates blood-brain barrier damage and improves outcome after stroke in rats. FASEB J. 32, 2181–2196. doi: 10.1096/fj.201700786RR
Keywords: blood-brain barrier, cerebrovascular disease, cerebral edema, ischemic stroke, pathophysiology
Citation: Gu Y, Zhou C, Piao Z, Yuan H, Jiang H, Wei H, Zhou Y, Nan G and Ji X (2022) Cerebral edema after ischemic stroke: Pathophysiology and underlying mechanisms. Front. Neurosci. 16:988283. doi: 10.3389/fnins.2022.988283
Received: 07 July 2022; Accepted: 01 August 2022;
Published: 18 August 2022.
Edited by:
Ji He, Peking University Third Hospital, ChinaReviewed by:
Felix Ng, University of Melbourne, AustraliaAnuska V. Andjelkovic, University of Michigan, United States
Copyright © 2022 Gu, Zhou, Piao, Yuan, Jiang, Wei, Zhou, Nan and Ji. This is an open-access article distributed under the terms of the Creative Commons Attribution License (CC BY). The use, distribution or reproduction in other forums is permitted, provided the original author(s) and the copyright owner(s) are credited and that the original publication in this journal is cited, in accordance with accepted academic practice. No use, distribution or reproduction is permitted which does not comply with these terms.
*Correspondence: Guangxian Nan, bmFuZ3hAamx1LmVkdS5jbg==; Xunming Ji, aml4bUBjY211LmVkdS5jbg==