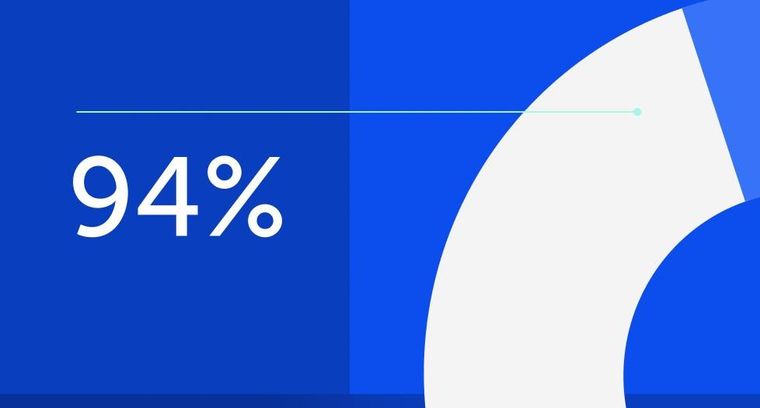
94% of researchers rate our articles as excellent or good
Learn more about the work of our research integrity team to safeguard the quality of each article we publish.
Find out more
REVIEW article
Front. Neurosci., 09 November 2022
Sec. Gut-Brain Axis
Volume 16 - 2022 | https://doi.org/10.3389/fnins.2022.981772
This article is part of the Research TopicSystems Biology in Brain-Gut Axis ResearchView all 20 articles
Spinal cord injury (SCI) is a central nervous system (CNS) disease that can cause sensory and motor impairment below the level of injury. Currently, the treatment scheme for SCI mainly focuses on secondary injury and complications. Recent studies have shown that SCI leads to an imbalance of intestinal microbiota and the imbalance is also associated with complications after SCI, possibly through the microbial-brain-gut axis. Melatonin is secreted in many parts of the body including pineal gland and gut, effectively protecting the spinal cord from secondary damage. The secretion of melatonin is affected by circadian rhythms, known as the dark light cycle, and SCI would also cause dysregulation of melatonin secretion. In addition, melatonin is closely related to the intestinal microbiota, which protects the barrier function of the gut through its antioxidant and anti-inflammatory effects, and increases the abundance of intestinal microbiota by influencing the metabolism of the intestinal microbiota. Furthermore, the intestinal microbiota can influence melatonin formation by regulating tryptophan and serotonin metabolism. This paper summarizes and reviews the knowledge on the relationship among intestinal microbiota, melatonin, and SCI in recent years, to provide new theories and ideas for clinical research related to SCI treatment.
SCI is a highly disabling disease caused by various events, including traffic accidents, fall injuries, sports injuries, and tumors triggering spinal cord compression, traction, and contusion (Zhang et al., 2017). Besides the primary injury, the subsequent secondary injury caused by inflammation, edema, free radical peroxidation, local circulatory disorder, abnormal energy metabolism, electrolyte disorder, programmed apoptosis, nitric oxide and endogenous opioid peptides, and accumulation of excitatory neurotransmitters further exacerbate the damage to the spinal cord tissue (Anjum et al., 2020). Furthermore, multiple complications after SCI including respiratory and urinary tract infections, pressure ulcer, deep vein thrombosis and pulmonary embolism, neurogenic bladder, gastrointestinal dysfunction, sexual dysfunction and so on, have become significant causes of death and low quality of life in the recovery period (Rogers and Todd, 2016). The prognosis of SCI is usually related to the degree of primary injury and the treatment of segments, injury time, secondary injury and complications. Surgery to relieve compression after injury (within 24–36 h) is considered an effective and critically effective treatment to improve the prognosis of SCI (Badhiwala et al., 2021). In addition, hyperbaric oxygen, pulse electrical stimulation, mild hypothermia therapy, acupuncture, laser puncture, rehabilitation training, and other therapies could be used to assist in the recovery of function (Huang et al., 2020). Pharmacological treatment, neurotrophic factor therapy, cell transplantation therapy, gene therapy, and intervention in signaling pathways are thought to be potentially efficient in improving the prognosis of SCI, but more clinical trials are still needed to prove their effectiveness (Badhiwala et al., 2021). SCI has different pathological changes in various periods. Acute (= 2–48 h), and sub-acute (=14 days) after SCI, pathological changes such as vascular injury, ionic imbalance, free radical production, lipid peroxidation, accumulation of excitatory neurotransmitters, edema, necrosis and apoptosis occur at this stage (Faden et al., 1989; Oyinbo, 2011; Borgens and Liu-Snyder, 2012; Anjum et al., 2020). In the chronic SCI stage (> 1 month post-injury), characterized by chronic inflammation, axonal loss and maturation of glial scarring (Tran et al., 2018; Quadri et al., 2020). In addition, there are differences between acute and chronic inflammation. Following the primary injury, microglia at the injury site release proinflammatory and chemotactic factors, as well as recruit central granulocytes to mediate acute inflammation. At this time, M1 and M2 macrophages coexist (Zhou et al., 2014; Gensel and Zhang, 2015; Hellenbrand et al., 2021). In the chronic stage, M1 macrophages exist for a long time and mediate chronic inflammation with mature lymphocytes (Bastien and Lacroix, 2014; Schwab et al., 2014). Melatonin (N-acetyl-5-methoxy tryptamine) is an indole hormone secreted by the pineal gland. The pineal gland takes tryptophan in the blood as raw material and synthesizes 5-methoxy-n-acetamide through various enzymatic reactions (Vasey et al., 2021; Figure 1). Its secretion is regulated by circadian rhythm, inhibited during the day and active at night. In addition to the pineal gland, melatonin can also come from the intestines, skin, retina, bone marrow, platelets and other structures. However, it acts primarily locally and rarely throughout the whole body via blood circulation (Huether, 1993; Bubenik, 2002; Ren et al., 2017).
Figure 1. Melatonin synthesis: Pineal cells absorb tryptophan (Trp) and produce melatonin through intermediates such as 5-hydroxytryptophan (5-HTP), serotonin and N-acetyl-5-hydroxytryptamine (NAS). Substances such as indole and short chain fatty acids produced by gut microbiota also promote the synthesis of gut-derived melatonin. Light signal regulation of melatonin: Light signal received by the optic apparatus transmits to the supraoptic nucleus (SCN) of the hypothalamus, then it continues to transmit to the sympathetic cervical ganglion (SCG) through the brainstem and spinal cord, and finally arrived at the pineal gland to regulate the secretion of melatonin. Spinal cord direct damage, sleep disorders, microbiota imbalance and inflammatory will inhibit the synthesis and secretion of melatonin after SCI. IDO, Indoleamine 2, 3-dioxygenase; TDO, Tryptophan dioxygenase. Created with BioRender.com.
In terms of dosage, there is little difference between the physiological and pharmacological effects of melatonin, physiological dose provides plasma melatonin levels of the same order of magnitude as a nocturnal peak (less or around 100 pg/ml or 400 pM). Oral administration of 0.3 mg can reach the endogenous level at night (Dubocovich et al., 2010; Claustrat and Leston, 2015). In addition, continuous desensitization of receptors caused by excessive use of melatonin may affect sleep and circadian rhythm (Gerdin et al., 2004; Wurtman, 2006).
Melatonin which the CNS synthesizes has good blood-brain barrier permeability. It determines that melatonin can ensure sufficient concentration when interacting with other CNS parts through blood circulation. Melatonin is known to have a variety of biological functions, including circadian rhythm regulation, anti-inflammatory, free radical scavenging, edema reduction, suppression of the hypothalamic-pituitary endocrine axis, suppression of cancer, stimulation of mitochondrial biogenesis, immunomodulation, blood pressure regulation and the epigenetic regulation, and has therapeutic effects on a variety of secondary injuries after SCI (Giannoulia-Karantana et al., 2006; de Faria Poloni et al., 2011; Mazzoccoli et al., 2011; Xu L.X. et al., 2017; Chang et al., 2018; Onaolapo et al., 2020; Cho et al., 2021; Vasey et al., 2021). Melatonin can also regulate the intestinal biological clock and the types of intestinal microbiota, improve the metabolic disorder caused by intestinal microbiota imbalance after SCI, and indirectly play a role in the recovery of function and the treatment of complications (Paulose et al., 2016). The route of administration and detection method are simple and convenient, which are conducive to investigation and evaluation. The exogenous melatonin can be administered orally or intravenously and its concentration can be assessed by blood, saliva and so on. It is considered a more convenient and accessible approach to provide greater comfort to patients and improve their quality of life (Dermanowski et al., 2022).
The intestinal microbiota can act on the CNS through the microbial gut-brain axis. The normal microbial ecology of the intestinal tract helps the human body generate a range of vitamins, engage in carbohydrate, protein, and lipid metabolism, promote trace element absorption, proper intestinal peristalsis, feces excretion, and prevent the growth of dangerous bacteria (Blaut, 2013). The brain-gut axis seems to provide new discoveries in some CNS diseases. The brain can transmit information from top to bottom via the autonomic nervous system, the gut nervous system, the hypothalamus pituitary adrenal axis (HPA), and the meningeal lymphatic vessels (Yuan et al., 2021). many neurological diseases, such as depression, multiple sclerosis (MS), Alzheimer’s disease (AD), Parkinson’s disease, and others, can cause changes in the structure of the intestinal microbiota (Joscelyn and Kasper, 2014; Williams and Murray, 2015; Sun et al., 2018; Anderson et al., 2019). In addition, the intestine can also transmit signals from bottom to top through the vagus and immune pathways (Yuan et al., 2021). Patients with Parkinson’s disease have higher levels of lipopolysaccharide (LPS) and lower levels of short-chain fatty acid (SCFA) in the intestine, leading to chronic inflammation in the CNS and promoting disease progression (Keshavarzian et al., 2015; Perez-Pardo et al., 2019). Increasing the percentage of intestinal regulatory T (Treg) cells but decreasing interleukin-17-gamma-delta T cells can alleviate nerve defects and anti-inflammation after stroke (Lee et al., 2020). Intestinal microbiota promotes the occurrence of the intracranial aneurysm by regulating plasma taurine and fatty acid levels (Li et al., 2020). It also improves neuronal degeneration, brain edema and blood-brain-barrier damage by increasing the level of glucagon-like peptide 1 (Li et al., 2018), and improves spatial learning through SCFA after traumatic brain injury (Opeyemi et al., 2021). In addition, the secretion of intestinal bacterial amyloid protein and lipopolysaccharide (LPS) promotes neuroinflammation and causes neuronal death in AD (Pistollato et al., 2016; Kesika et al., 2021). Some studies suggest that regulating stress state and HPA through fecal bacteria transplantation may be significant in treating depression (Liang et al., 2018).
SCI is usually accompanied by the destruction of intestinal ecology through mechanisms associated with disruption of signals from higher control center, autonomic nerve dysfunction, intestinal barrier permeability change, mesenteric lymph node immune regulation, and long-term use of antibiotics (Cervi et al., 2014; Kigerl et al., 2016; Tate et al., 2016; Jing et al., 2019). Studies have shown that intestinal microbiota modulates the functional recovery after SCI through SCFA and the immune system (Jing et al., 2021b; Rodenhouse et al., 2022). Alternatively, there is evidence that fecal flora transplantation (FMT) may be effective in improving complications such as gastrointestinal dysfunction, bloating and constipation after SCI (Jing et al., 2021a). Furthermore, intestinal microbiota could directly or indirectly participate in the synthesis of melatonin. Melatonin is derived from serotonin (5-hydroxytryptamine, 5-HT). Intestinal bacteria may directly produce SCFAs, which stimulate the production of 5-HT. Then, the 5-HT is successively converted into N-acetyl-5-hydroxytryptamine (NAS) and melatonin by arylalkylamine N-acetyltransferase (AANAT, EC: 2.3.1.87) and acetylserotonin O-methyltransferase (ASMT, EC: 2.1.1.4) (Benabou et al., 2017; Figure 1).
As a result, a bidirectional link is observed, in which changes in intestinal microbiota influence the regulation of the melatonergic system, and treatment of melatonin cause changes in microbiota, with both factors contributing to the pathobiology and treatment of SCI. This review aims to describe the potential links among gut microbiota, melatonin, and SCI and to explore the synergistic effects of melatonin and the regulation of gut microbiota in improving the prognosis of SCI.
Melatonin regulate the intestinal microbiota, which improve the reduction of intestinal operational taxonomic unit (OTU), increase ACE index and Shannon index, decrease Simpson index, increase the diversity and abundance of intestinal microbiota, increase the abundance of firmicum and decrease the abundance of Bacteroides (Ren et al., 2018; Gao T. et al., 2020; Kim et al., 2020). Melatonin also increases Akkermansia flora’s abundance (Hagi and Belzer, 2021). In the SCI mouse model, melatonin reduce the abundance of Clostridium and increase the abundance of Lactobacillus (Jing et al., 2019). Also, melatonin could increase the abundance of Myxobacteria and Streptococcus mucophaphagus (Park et al., 2020), protect intestinal barrier and reduce intestinal inflammation. In addition, melatonin enhances the abundance of bacteria and decrease the ratio of firmicum to Bacteroides in a high-fat diet-induced obesity mouse model (Xu P. et al., 2017; Yin et al., 2018). In the ochratoxina induced liver injury model, melatonin increase the abundance of Bacteroides, verrucous microorganisms and actinomycetes, while decrease the abundance of firmicum and Lactobacillus (Zhang H. et al., 2021; Zhao et al., 2021). In terms of microbial metabolism, melatonin significantly reduces the relative abundance of the microbiome in the cysteine and methionine metabolism and peptidoglycan biosynthesis pathway in the restraint stress mouse model, while increasing the relative abundance of the microbiome in the tryptophan metabolism, aminobenzoic acid degradation, renin angiotensin system, γ-aminobutyric acid ergic synapses and type II diabetes pathway (Lin et al., 2021).
There are three interconnected barriers in the intestine, a biological barrier made up of the intestinal microbiota; an intestinal immune barrier made up of the intestinal lymphatic system; and a physical barrier comprising the voluntary movement of the intestine and the mucosal epithelial system (Gao et al., 2019). The fluorescein isothiocyanate labeled dextran experiment proved that the intestinal permeability in stress state would increase (Jing et al., 2019). Under stress, the number of intestinal goblet cells and intestinal gland proliferating cell nuclear antigen positive cells decreased, which affect mucus secreted and intestinal cells proliferated, and then the repair effect of intestine barrier was damaged. Meanwhile, the activation of nuclear factor-kappaB (NF-κB) pathway induced autophagy and eventually damages the intestinal mechanical barrier (Gao et al., 2019).
The expression of tight junction-related proteins claudin-1, occludin and zonula occludens-1 (ZO-1) decreased in stress models such as SCI and sleep deprivation, and melatonin is effective in improving the reduction of occludin and ZO-1 (Gao et al., 2019; Jing et al., 2019). It suggested that melatonin may strengthen the tight connection between cells, regulate intestinal permeability, repair and maintain the stability of intestinal mechanical barrier, which effectively prevent toxins and other bacterial metabolites in the intestine from entering the blood and cause cascading chain inflammatory reaction. A similar effect was also observed in the oxazolone induced colitis model (Zhao et al., 2021), indicating that melatonin’s preservation of the intestinal barrier was not confined to stress damage and that its barrier protective role had a broader application. In addition, melatonin accelerates the emptying effect of the gastrointestinal tract in mice after SCI (Jing et al., 2019), demonstrating that melatonin enhances gastrointestinal function, promotes peristalsis and strengthens the mechanical barrier.
In prolonged paradoxical sleep deprivation mouse model, with the activation of the HPA, a large amount of cortisol were released into the systemic circulation, which would mediate the disturbance of intestinal microbiota (Galvão Mde et al., 2009), stimulate a cascade of transcription factors and associated signaling pathways. Cortisol activated the protein expression of the signal transducer and activator of transcription 3 (STAT-3)/activator protein 1 (AP-1)/NF-κB pathway, such as phosphorylated-signal transducer and activator of transcription-3 (p-STAT3), AP-1, p-P65 and phosphorylated-inhibitor of nuclear factor-kappaB (p-IκB), while melatonin decreased the expression of the above proteins, suggesting that melatonin suppressed inflammation and autophagy by inhibiting NF-κB pathway (Gao et al., 2021). Research has shown that the stress induces changes in intestinal permeability leading to the secretion of LPS by the intestinal microbiota which in turn activates the TLR4 inflammatory signaling pathway to release of several proinflammatory cytokine such as interleukin-1β (IL-1β), IL-6 and tumor necrosis factor-α (TNF-α) (Ghareghani et al., 2018). In a mouse model of colitis, melatonin inhibited the increase of IL-1β, IL-17 and TNF-α, whereas in TLR4 knockout mice melatonin has been found to fail to inhibit effectively the increased levels of IL-1β, IL-17, and TNF-α (Kim et al., 2020), suggesting that melatonin may suppress the LPS/TLR4 signaling pathway by inhibiting expression of pro-inflammation cytokines. In addition, the expression of anti-inflammatory factors (IL-5, IL-10, IL-22) was regulated by administration of melatonin on in a stress model (Gao et al., 2019, Gao K. et al., 2020; Lin et al., 2021), and further inhibit intestinal inflammation. It was demonstrated that in a mouse model of SCI, melatonin displayed anti-inflammatory effects by reducing the upregulation of IL-17, interferon-gamma (IFN- γ) and monocyte chemoattractant protein-1 (MCP1) (Jing et al., 2019). In the oxazolone induced colitis model, melatonin could inhibit the production of IL-5, IL-13 and their mRNA in type 2 innate lymphocytes (ILC2s) and affect the type 2 immune response (Zhao et al., 2021). Ghareghani et al. (2018) and Xiong et al. (2022) demonstrated that melatonin modulates intestinal immune function through the expression of nod-like receptor-related genes and TLRs. By differential expression gene (DEG) and gene ontology (GO) analysis, Tlr1, Tlr2, Tlr7, Cd14, Naip, Cxcl8 and Nlrp1, Nlrp3 gene expression levels were found to be significantly increased in ASMT (a key enzyme in the acetyl serine synthesis melatonin pathway) overexpressing transgenic sheep (Li et al., 2021). It is suggested that melanin plays an important role by regulating anti-inflammatory related factors and signaling pathways. In general, melatonin can regulate the immune response by regulating intestinal TLRs and nod like receptors, and affect the type 2 immune response by inhibiting ILC2s. It also inhibits the STAT-3/AP-1/NF-κB pathway, down-regulating the release of inflammatory factors and up-regulating anti-inflammatory factors, suppressing the intestinal inflammatory response and setting the stage for ecological recovery of the microbiota.
Melatonin is abundant in the gastrointestinal tract, with approximately 10–100 times more melatonin in the gastrointestinal tissues than in the blood and 400 times more in the gut than in the pineal gland (Huether, 1993; Bubenik, 2002). Intestinal microbiota can affect melatonin secretion through a variety of mechanisms. High dietary fiber diet promoted the increase of intestinal short chain fatty acid concentration and 5-HT concentration in serum and intestinal mucosa, and promoted the up-regulation of tryptophan hydroxylase (TPH1) mRNA level (Zhuo et al., 2021). Besides, intestinal microbial metabolites, short chain fatty acids such as propionic acid and butyric acid, and tryptophan metabolite indoles can promote the production of 5-HT, which in turn further affects melatonin secretion via AANAT and ASMT (Gao et al., 2018; Figure 1).
Intestinal microbial sulfatase can promote melatonin secretion (Ervin et al., 2020). The experiments in zebrafish have proved that probiotics and dark conditions can increase the mRNA expression of melatonin receptor genes, mainly melatonin-1 related receptor genes (Mtnr1ba and Mtnr1bb) (Lutfi et al., 2021). The disturbance of intestinal microbiota affect the absorption of vitamins, which cause deficiencies in enzyme cofactors. In addition, the disruption of intestinal microbiota induces intestinal inflammatory reaction. Inflammatory and deficiencies of enzymatic cofactors would trigger the kynurenine metabolic pathway, promoting the synthesis of tryptophan into kynurenine and inhibiting the synthesis of tryptophan into 5-hydroxytryptamine and melatonin (Rudzki et al., 2021).
The imbalance of intestinal microbiota has been proved to be related to various CNS diseases. Firmicutes and Bacteroides are two phylum of intestinal microbiota, both of them contain probiotics and opportunistic pathogens, which have dual effects on human body (Wexler and Goodman, 2017; Heintz-Buschart and Wilmes, 2018). Intestinal microbiota can produce short-chain fatty acids by metabolizing dietary fiber (Markowiak-Kopeć and liżewska, 2020). Previous studies found that obese people have higher level of Firmicutes and lower level of Bacteroides (Ley et al., 2005, 2006). In addition, another study found that obese people have higher levels of SCFA (Schwiertz et al., 2010), proving that the level of SCFA is directly proportional to Firmicutes.
Studies have confirmed that intestinal microbiota is disordered after SCI, which is manifested by the decrease of the abundance of Firmicutes, with or without the increase of bacteroides, resulting in the change of the ratio of Firmicutes to Bacteroides, this change in microbiota structure affects the production of short-chain fatty acids (Gungor et al., 2016; Zhang et al., 2018; Jing et al., 2019; Bazzocchi et al., 2021; Rodenhouse et al., 2022), this appears to be associated with severe inflammation and complex infection after SCI. There are significant differences in intestinal microbiota composition in patients after SCI in the acute and chronic stages. There is greater abundance of genus Sutterella in the acute stage, it may be related to more severe inflammation (Santoru et al., 2017). In the chronic stage, it had lower abundances of the Burkholderiaceae family. But with the increase of exercise, the abundance of Burkholderiaceae increases. It suggests that long-term inactivity after SCI may lead to this difference (Li et al., 2022). The changes in intestinal microbiota after SCI are related to the degree of injury. In American Spinal Injury Association Impairment Scale (AIS) A or B patients, the abundance of lactobacillus is higher, and in AIS C or D patients, Bacteroides, Faecalibacterium, Lachnospiraceae are more abundant (Bazzocchi et al., 2021). A study of patients with complete spinal cord injury (CTSCI) and incomplete spinal cord injury (ITSCI) has found that the abundance of Synergistetes is significantly different between CTSCI and ITSCI. Coriobacteriae, Eubacterium, and Cloacibacillus is more abundant in CTSCI, Lactobacillaceae, Lachnospiraceae, Eubacteria, Clostridium, and Sutterella is more abundant in ITSCI (Yu et al., 2021). Different levels of SCI will also lead to the different intestinal microbiota. Zhang et al. (2018) found that the abundance of Bacteroides is higher in quadriplegia patient, while Acidaminococcaceae, Blautia, Porphyromonadaceae, and Lachnoclostridium is higher in paraplegia patient. Another study pointed out that the Firmicutes in T10 is low, while actinomycetes in T4 and T10 are increased (Du et al., 2021).
The regulation of intestinal function is actually co-regulated by sympathetic, parasympathetic and enteric nervous system (ENS) (Furness et al., 2014). After SCI, the injured ascending and descending neurons cannot dominate the lower neurons below the injured segment, nor can they transmit the information of lower neurons to the brain. The interruption of the control of the advanced center leads to the loss of gastrointestinal and bladder sympathetic control, smooth muscle dysfunction, impaired intestinal movement, fecal retention or incontinence (Holmes et al., 2020), and these variables are maintained throughout the acute to chronic phase of SCI. It has been studied to exclude the influence of sympathetic and parasympathetic on intestinal tract by injuring the high level thoracic spinal cord, in the mice with T8 SCI, it found that the number of nitroergic nerve cells decreased, and the number of acetylcholine decreased although the proportion of acetylcholinergic nerve did not change significantly (Lefèvre et al., 2020). Another study found that nitroergic and cholinergic neurons decreased in the mice with T3 SCI (White et al., 2020). These results suggest that the ENS is damaged after SCI, which lead to the destruction of colonic content secretion, propulsion and local reflex regulation related to colonic segmentation (Furness et al., 2014).
Gut-associated lymphoid tissue (GALT) is innervated by the sympathetic nerve of the spinal cord. The loss of control of the sympathetic nerve destroys the immune homeostasis of GALT after SCI (Straub et al., 2006), further affecting the regulation of the intestinal immune system on the intestinal microbiota. Kigerl et al. (2016) found that the expression of TNF, IL-10, IL-1β, and transforming growth factor-β (TGF-β) is increased in mesenteric lymph nodes on the third day after SCI, and Li et al. (2022) found that different flora changes in the feces of patients in the acute stage of SCI compared with those in the chronic stage. In 8-week SCI animals, it has been found that IL-1β is significantly associated with 23 OTUs, and 12 OTUs were found to be significantly correlated to IL-12. Although macrophage inflammatory protein 2 is especially related to the difference in microbial diversity, no significant OTU is detected (O’Connor et al., 2018). It indicates that the occurrence of acute and chronic inflammation after SCI mediates the destruction of intestinal ecology.
Intestinal epithelial barrier dysfunction has proved after SCI (Liu et al., 2020, 2021). The change leads to more opportunities for bacteria and their metabolites to enter the circulation, cause enterogenous infection, and further lead to the disorder of flora. Studies have shown that SCI affects the intestinal epithelial barrier and induces intestinal disturbances, leading to delayed motor recovery in mice (Kigerl et al., 2016).
Numerous studies have reported that many antibiotics exert anti-inflammatory and neuroprotective effects in different central and peripheral nervous system disorders, including SCI. But long-term use of antibiotics may further aggravate the disturbance of intestinal microbiota after SCI, inducing the increase of firmicum, Proteus and Actinobacillus the decrease of Bacteroides, and affecting the functional recovery after SCI (Jing et al., 2019; Bazzocchi et al., 2021; Rodenhouse et al., 2022). However, as an antibiotic, dimethylaminocycline has largely ignored its negative effects on intestinal microbiota and systemic immune response after SCI, which may be related to its direct anti-inflammatory, anti-oxidation, anti-anxiety, and neuroprotective properties (Schmidt et al., 2021).
Microglia can rapidly transform into activated macrophages and produce toxic molecules that mediate lipid peroxidation, eventually leading to subsequent tissue damage and axonal contraction after SCI (Busch et al., 2009; Kigerl et al., 2009). Moreover, glial cells increase pain hypersensitivity by releasing various signal molecules, such as proinflammatory cytokines (DeLeo and Yezierski, 2001; Watkins et al., 2001; Hanisch, 2002). Short-chain fatty acids have strong anti-inflammatory effects on macrophages (Park et al., 2005; Chen et al., 2007) and inhibit CNS inflammation through the brain-gut axis. Patients with SCI have significantly lower levels of butyric acid producing bacteria, and the decreased butyric acid levels lead to reduce inhibition of microglia, resulting in microglia-mediated neurotoxicity, which may also be responsible for the persistent triggering of neuropathic pain. Low butyric acid levels may affect long-term functional recovery after SCI (Gungor et al., 2016). Another study demonstrated that probiotic-transplanted mice could reduce inflammatory response by increasing the production of SCFAs, which modulate gut immunological and barrier functions by inhibiting NF-κB signaling (Jing et al., 2021b; Figure 2). At the early stage of SCI, the level of Firmicutes decreases and Bacteroides increases, which affect the production of SCFA (Jing et al., 2021a). However, most studies of FMT for SCI have chosen in the chronic phase of SCI. Therefore, the alteration of intestinal flora after the intervention of intestinal flora in the acute phase of SCI need to be studied in depth.
Figure 2. Intestinal microbiota protects neuron and improves complications after SCI. Microbiota secretes SCFA to regulate maturation and activation of microglia, thereby suppressing their neurotoxicity. Created with BioRender.com.
It is suggested that probiotic transplantation to reconstitute the intestinal microbiota is beneficial in promoting functional recovery after SCI. In mice involved in the transplantation of probiotics to reconstitute the gut microbiota after SCI, the action evoked potential (MEP) amplitude was significantly higher in the probiotic-transplanted mice than in the SCI group. In addition, the number of NeuN + cells, NF-200 -positive cells, and the expression of synapsin increased in probiotic-transplanted mice, which suggested that probiotic transplantation may promote the survival of neurons and axonal regeneration after SCI (Jing et al., 2021a; Figure 2). On the other hand, transplantation of probiotics before SCI could reduce the loss of function after injury, and can effectively improve the flora structure disorder caused by antibiotics, which is considered to be positive significance for the recovery of neural function after SCI (Rodenhouse et al., 2022).
There are many related chronic complications after SCI, such as intestinal dysfunction, bladder dysfunction and sexual dysfunction (Figure 3), severely impair the quality of life. Studies have shown that compared with other complications, patients with SCI want to solve the problem of intestinal dysfunction (Glickman and Kamm, 1996; Lynch et al., 2001; Anderson, 2004; Zhang et al., 2018). Neurogenic motor gut dysfunction usually occurs after SCI, and it can be further classified into upper motor meta gut syndrome (UMN) and lower motor gut syndrome (LMN). In the mouse model, the mice that recovered the structure of intestinal microbiota after fecal microbiota transplantation showed faster gastrointestinal transit through barium gavage followed by X-ray imaging (Jing et al., 2021b).
Figure 3. Smooth muscle dysfunction leads to constipation and fecal incontinence after SCI. Melatonin can enhance intestinal motility to promote emptying. It can also improve colonizing dominant bacteria abundance to reduce the probability of urinary infection. Melatonin inhibits the activation of M1 macrophage after SCI, the release of a variety of inflammatory factors are suppressed, and at the same time regulates apoptosis-related proteins to inhibit the apoptosis of nerve cells. In addition, melatonin regulates channel-related proteins to maintain the stability of blood-spinal cord barrier and reduce edema. Created with BioRender.com.
After SCI, neurogenic bladder would also occur, resulting in urinary retention and incontinence, which further cause urinary system infection. It has been reported that the annual urinary tract infection rates of upper motor neuron intestinal syndrome group and lower motor neuron intestinal syndrome group were 3.0 ± 1.8 and 2.6 ± 1.8, respectively (Gungor et al., 2016). It has been proved that the transplantation of intestinal microbiota could improve refractory urinary tract infection (Tariq et al., 2017; Biehl et al., 2018; Magruder et al., 2019, 2020), which may be related to the change of dominant bacteria inhibiting the growth and colonization of pathogenic bacteria, but it still needs more in-depth research and prospective studies to explore the molecular mechanisms and confirm. C. J. Worby summarized that the influence of intestinal microbiota of urinary tract infection may come from three aspects. Firstly, the causative organisms of urinary tract infections come from the gut microbiota. Furthermore, a specific gut microbiota provides a suitable living environment for urinary tract infections. Eventually disturbances in the gut microbiota modulate the immune system (Worby et al., 2022). Therefore, improving the gut microbiota positively affects urinary tract infections.
SCI in different spinal cord segments lead to significantly disparate degrees of melatonin secretion. Studies have shown that the level of cortisol in thoracic SCI was higher, and the level of melatonin in cervical SCI was lower (Thøfner Hultén et al., 2018). It was found that melatonin levels were significantly lower in patients with completed cervical SCI than in the thoracolumbar SCI group and the normal group. Furthermore, melatonin levels appear to differ between patients with complete and incomplete SCI, but in vivo and in vitro experimental verification is lacking, and this hypothesis requires further experimental validation (Whelan et al., 2020). It has been previously confirmed that the secretion rhythm of melatonin is related to the suprachiasmatic nucleus (SCN) of the hypothalamus (Cardinali and Pévet, 1998). The nerve from SCN to the pineal gland passes through the upper part of the cervical spinal cord and connects with the preganglionic cells of the sympathetic cervical ganglion (SCG) (Møller and Baeres, 2002), which indicates that melatonin secretion is related to cervical spinal cord segments (Figure 1).
In addition, the change of melatonin secretion level may be related to sleep disorders after SCI (Hultén et al., 2020). An investigation on sleep disorders in patients with cervical myelopathy reported that spinal cord compression and area reduction were independent risk factors for sleep disorders. Therefore, patients with compression SCI caused by spinal canal stenosis are prone to sleep disorders, and subsequently affect melatonin secretion (Kim et al., 2021).
Melatonin has been proved by many studies to improve motor function recovery after SCI (Shen et al., 2017; Jing et al., 2019; Li et al., 2019; Wang K. et al., 2019; Xu et al., 2019; Gao K. et al., 2020; Majidpoor et al., 2020; Yang et al., 2020; Bi et al., 2021a), which is closely related to the anti-inflammatory, anti-apoptotic, tissue edema reducing, blood spinal cord barrier (BSCB) protection and nerve remodeling functions of melatonin (Figure 3). It was demonstrated that melatonin treatment significantly reduced the relative abundance of Clostridium perfringens, markedly increased the relative abundance of Lactobacillus, and dramatically improved function after SCI (Jing et al., 2019). In addition, melatonin can be used in combination with various treatment methods to treat SCI. For instance, polylactic acid glycolic acid copolymer sustained-release microspheres with melatonin and laponite hydrogel for mixed injection to treat SCI (Zhang M. et al., 2021). The use of melatonin in extracellular vesicles of stem cells reduces the levels of N6 methyladenosine modification (m6A) and methyltransferase 3 (METTL3) in SCI, and enhances the stability of USP29mRNA in MSCs, which also promote stem cell proliferation, differentiation, neurosphere formation and secretion of neurotrophic factors (Li et al., 2017; Zheng et al., 2017). In general, melatonin plays the role of anti-inflammatory, anti-apoptotic, tissue edema reducing, blood polar core barrier protection and nerve remodeling functions in acute and sub-acute stages.
Melatonin was effective in ameliorating neuronal apoptosis and consequently protecting neurons after SCI (Jing et al., 2017; Shen et al., 2017; Zheng et al., 2017; Li et al., 2019; Xu et al., 2019; Gao K. et al., 2020; Bi et al., 2021a). It has been found that melatonin treatment significantly increased the expression of apoptosis-related proteins, including phosphatidylinositol 3 kinase (PI3K) p85 and PI3K downstream proteins (phosphoinositide-dependent kinase-1, protein kinase B and NF-κB p65). The up-regulation of PI3K downstream protein was inhibited after using melatonin inhibitor luzindole, which indicates that melatonin inhibits apoptosis after SCI by activating PI3K pathway (Bi et al., 2021a,b). In addition, some studies found that melatonin down regulated the expression of Caspase3 and caspase9 after SCI, and the expression levels of autophagy marker protein (beclin-1), LC-3b and SITR1/AMPK signal pathway related proteins (SIRT1 and p-AMPK) increased significantly. The above effects disappeared after using SIRT1 inhibitor EX527, which indicates that melatonin can regulate autophagy and apoptosis through SIRT1/AMPK (Gao K. et al., 2020). Li et al. (2019) also demonstrated that melatonin enhances the autophagy of spinal cord neurons through PI3K/AKT/mTOR signaling pathway. Furthermore, melatonin significantly increased p-LRP6, β-catenin and LEF-1, the level of pro-apoptotic protein Bax was down-regulated, and the anti-apoptotic protein Bcl-2 was up-regulated, indicating that melatonin via Wnt/β- Catenin signaling pathway to regulate autophagy and apoptosis (Shen et al., 2017; Figure 3).
Melatonin treatment can significantly reverse the expression of inflammatory related factors after SCI, such as IL-17 and IFN-γ, MCP-1 (Jing et al., 2019), NOD-like receptor protein 3 (NLRP3), IL-1 β, and caspase-1 (Xu et al., 2019; Yang et al., 2020). Some studies have pointed out that melatonin can inhibit inflammation after SCI via TLR4/NF-κB and NOX2/TXNIP signaling pathways (Paterniti et al., 2017; Majidpoor et al., 2020). Majidpoor et al. (2021) found that melatonin decreased the activities of NLRP3, apoptosis associated spotted protein and caspase-1, down regulated the level of IL-1 β and IL-18. The sensitivity of different segmental injuries to melatonin treatment is different. Among them, T6 is the most sensitive to melatonin treatment, except for T6, melatonin treatment does not affect the level of NLRP3 after SCI. IL-18 does not change only after melatonin treatment for T1 segmental injury (Majidpoor et al., 2021), which is undoubtedly interesting, but the reasons for this deserve further study.
However, few studies are concluding that melatonin has different sensitivity in different segments, which still needs to be further verified by other experiments. Zhang et al. (2019) found that melatonin treatment after SCI reduced M1 microglia markers (CD16, iNOS, TNF-a), but increased the levels of the M2 microglia markers (Arg1, CD206, TGF-β), which proves that melatonin promotes the differentiation of macrophages toward M2 type after SCI. In addition, melatonin inhibits the aggregation of microglia and thus the activation and proliferation of microglia and astrocytes (Paterniti et al., 2017; Yang et al., 2020; Figure 3).
Studies have shown a reduction in the endothelial marker protein CD31 after SCI, suggesting damage to the blood-spinal cord barrier, and administration of melatonin increased the proportion of CD31-labeled vessels, suggesting that melatonin is effective in reducing the permeability of the blood-spinal cord barrier after SCI (Jing et al., 2017). Wang K. et al. (2019) proved that melatonin enhance the level of angiopoietin 1 (Ang1), reduce the expression of vascular endothelial growth factor (VEGF), adhesion factor intercellular adhesion molecule 1 and BSCB related factor matrix metallopeptidase 2 through insulin-like growth factor binding protein-3 (IGFBP-3), indicating that melatonin can maintain the stability of BSCB through interaction with IGFBP3 (Figure 3). Besides this, studies in animals have shown that melatonin administration reduces tissue edema after SCI. Xu et al. (2019) demonstrated that the water content of spinal cord tissue decreased significantly after melatonin administration and that melatonin downregulated the expression of the aquaporin aquaporin-4 (Li et al., 2014; Liu et al., 2015).
Melatonin treatment after SCI significantly increased the mRNA and protein expression levels of growth associated protein-43 (GAP-43), synapsin1, Neurofilament 200 (NF200) and Postsynaptic density protein 95 (PSD-95) (Bi et al., 2021a), which play an important role in the process of neuronal remodeling. Moreover, brain-derived neurotrophic factor (BDNF) is the neuronal plasticity promoter, which affects neuronal remodeling through synapsin1 and GAP-43 protein. After SCI, the expression of BDNF, synapsin1 and GAP-43 in the spinal cord decreases, while melatonin can partially reverse the decrease of them, suggesting that melatonin has a protective effect on neural plasticity after SCI (Jing et al., 2017).
The complications after SCI greatly impact quality of life and life expectancy, especially constipation and fecal incontinence caused by gastrointestinal and bladder dysfunction (Glickman and Kamm, 1996; Lynch et al., 2001; Anderson, 2004; Zhang et al., 2018). The intestinal microbiota is closely related to gastrointestinal dysfunction. After SCI, the loss of advanced central control of the sympathetic nerve, the destruction of intestinal immune homeostasis, the impairment of intestinal barrier function, inflammation and the use of antibiotics will lead to the imbalance of intestinal microbiota and further cause gastrointestinal dysfunction. Regulating the structural disorder of intestinal microbiota can effectively inhibit the secondary injury after SCI, improve function, treat gastrointestinal dysfunction, and have a positive effect on urinary tract infection, which is undoubtedly very important for treating complications and the improvement of patients’ quality of life. Melatonin not only effectively blocks the secondary injury process after SCI, but also promotes the recovery of intestinal microbiota structure. It plays a synergistic role in intestinal microbiota to improve the prognosis of SCI.
Melatonin plays an important role in the treatment of acute and subacute SCI, including anti-apoptosis, anti-inflammation, anti-free radical damage, alleviating edema, protecting BSCB, promoting neurons, reducing the accumulation of astrocytes, and further affecting disease’s development in the chronic phase, such as regulating chronic inflammation and reducing scar formation. Intestinal microbiota can promote neuronal survival and axonal regeneration, secrete SCFA to regulate inflammation and immune function, and has positive significance for the treatment of complications after SCI.
There is no direct research proving that melatonin and intestinal microbiota have synergistic effects on the treatment of SCI. However, some studies have proved the synergy between the two from the side. Intestinal microbiota down regulates NF-κB pathway by short-chain fatty acids in SCI. Melatonin is also proved that down-regulate the signal expression of the same pathway. On the other hand, melatonin can modulate the intestinal barrier and motor function to regulate the structure of the intestinal flora. In addition, some studies have confirmed that the positive effect of melatonin on SCI is reduced after the use of antibiotics. It also indirectly shows that the two have a synergistic effect on the recovery of SCI. Therefore, melatonin and gut microbiota may act synergistically in the treatment of SCI and further studies will be needed to confirm their role and underlying mechanisms.
The disorder of intestinal microbiota after SCI occurs in the acute stage. However, in the current study, FMT mostly occurs in the chronic stage after SCI. Therefore, whether early use of FMT and melatonin to regulate the structure of intestinal microbiota and produce significant therapeutic effects on SCI is worthy of further study.
In addition, Majidpoor et al. found that different sensitivity of melatonin treatment in different injured segments (Majidpoor et al., 2021). Few study has reached similar conclusions, so the underlying mechanism deserves further study.
The intestinal tract contains a variety of flora and abundant lymphoid tissue, which has a strong immunomodulatory function. Gut-derived Treg cells have been confirmed to feature in the prognosis of stroke, and studies have confirmed that programmed death protein 1 (PD-1) and CC-chemokine ligand 28 (CCL28) regulate Treg cells (Wang P. et al., 2019; He et al., 2021). In addition, melatonin also has a regulatory effect on Treg cells (Álvarez-Sánchez et al., 2015; Glebezdina et al., 2019). Therefore, the combined effect of melatonin and gut microbiota whether further affect the prognosis of SCI through Treg cells is worthy of in-depth study.
This review discusses the relationship among melatonin, intestinal microbiota and SCI, and summarizes the progress of melatonin and intestinal microbiota in treating SCI. By elucidating the link among melatonin, the brain-gut axis and SCI, new insights and ideas for the treatment of SCI are provided, furthering the development of SCI treatment.
YZ, YY, CB, and WD conceived the perspective of the work. YZ, RL, and SG drafted the manuscript. HL and CL designed the figures. All authors revised and approved the final version of the manuscript.
This work was supported by the National Natural Science Foundation of China (31871031 and 32170968 to WD), the Fund of Key Laboratory of Medical Electrophysiology in 2021 (KeyME-2021-01 to YY), China Postdoctoral Science Foundation (2021M692700 to YY), and Sichuan Science and Technology Program (22ZDYF3809 to YY and 22ZDYF3783 to WD).
The authors declare that the research was conducted in the absence of any commercial or financial relationships that could be construed as a potential conflict of interest.
All claims expressed in this article are solely those of the authors and do not necessarily represent those of their affiliated organizations, or those of the publisher, the editors and the reviewers. Any product that may be evaluated in this article, or claim that may be made by its manufacturer, is not guaranteed or endorsed by the publisher.
Álvarez-Sánchez, N., Cruz-Chamorro, I., López-González, A., Utrilla, J. C., Fernández-Santos, J. M., Martínez-López, A., et al. (2015). ‘Melatonin controls experimental autoimmune encephalomyelitis by altering the t effector/regulatory balance’. Brain Behav. Immun. 50, 101–114. doi: 10.1016/j.bbi.2015.06.021
Anderson, G., Rodriguez, M., and Reiter, R. J. (2019). ‘Multiple sclerosis: Melatonin, orexin, and ceramide interact with platelet activation coagulation factors and gut-microbiome-derived butyrate in the circadian dysregulation of mitochondria in glia and immune cells’. Int. J. Mol. Sci. 20:5500. doi: 10.3390/ijms20215500
Anderson, K. D. (2004). ‘Targeting recovery: Priorities of the spinal cord-injured population’. J. Neurotrauma 21, 1371–1383.
Anjum, A., Yazid, M. D., Fauzi Daud, M., Idris, J., Ng, A. M. H., Selvi Naicker, A., et al. (2020). Spinal cord injury: Pathophysiology, multimolecular interactions, and underlying recovery mechanisms’. Int. J. Mol. Sci. 21:7533. doi: 10.3390/ijms21207533
Badhiwala, J. H., Wilson, J. R., Witiw, C. D., Harrop, J. S., Vaccaro, A. R., Aarabi, B., et al. (2021). ‘The influence of timing of surgical decompression for acute spinal cord injury: A pooled analysis of individual patient data’. Lancet Neurol. 20, 117–126.
Bastien, D., and Lacroix, S. (2014). ‘Cytokine pathways regulating glial and leukocyte function after spinal cord and peripheral nerve injury’. Exp. Neurol. 258, 62–77. doi: 10.1016/j.expneurol.2014.04.006
Bazzocchi, G., Turroni, S., Bulzamini, M. C., D’Amico, F., Bava, A., Castiglioni, M., et al. (2021). ‘Changes in gut microbiota in the acute phase after spinal cord injury correlate with severity of the lesion’. Sci. Rep. 11:12743. doi: 10.1038/s41598-021-92027-z
Benabou, M., Rolland, T., Leblond, C. S., Millot, G. A., Huguet, G., Delorme, R., et al. (2017). ‘Heritability of the melatonin synthesis variability in autism spectrum disorders’. Sci. Rep. 7:17746. doi: 10.1038/s41598-017-18016-3
Bi, J., Shen, J., Chen, C., Li, Z., Tan, H., Sun, P., et al. (2021a). ‘Role of melatonin in the dynamics of acute spinal cord injury in rats’. J. Cell. Mol. Med. 25, 2909–2917. doi: 10.1111/jcmm.16325
Bi, J., Sun, P., Feng, E., Shen, J., Chen, C., Tan, H., et al. (2021b). ‘Melatonin synergizes with methylprednisolone to ameliorate acute spinal cord injury’. Front. Pharmacol. 12:723913. doi: 10.3389/fphar.2021.723913
Biehl, L. M., Cruz Aguilar, R., Farowski, F., Hahn, W., Nowag, A., and Wisplinghoff, H. (2018). Fecal microbiota transplantation in a kidney transplant recipient with recurrent urinary tract infection. Infection 46, 871–874. doi: 10.1007/s15010-018-1190-9
Blaut, M. (2013). ‘Ecology and physiology of the intestinal tract’. Curr. Top. Microbiol. Immunol. 358, 247–272.
Borgens, R. B., and Liu-Snyder, P. (2012). ‘Understanding secondary injury’. Q. Rev. Biol. 87, 89–127.
Bubenik, G. A. (2002). ‘Gastrointestinal melatonin: Localization, function, and clinical relevance’. Dig. Dis. Sci. 47, 2336–2348.
Busch, S. A., Horn, K. P., Silver, D. J., and Silver, J. (2009). ‘Overcoming macrophage-mediated axonal dieback following CNS injury’. J. Neurosci. 29, 9967–9976. doi: 10.1523/JNEUROSCI.1151-09.2009
Cardinali, D. P., and Pévet, P. (1998). ‘Basic aspects of melatonin action’. Sleep Med. Rev. 2, 175–190.
Cervi, A. L., Lukewich, M. K., and Lomax, A. E. (2014). ‘Neural regulation of gastrointestinal inflammation: Role of the sympathetic nervous system’. Auton. Neurosci. 182, 83–88.
Chang, C. C., Huang, T. Y., Chen, H. Y., Huang, T. C., Lin, L. C., Chang, Y. J., et al. (2018). ‘Protective effect of melatonin against oxidative stress-induced apoptosis and enhanced autophagy in human retinal pigment epithelium cells’. Oxid. Med. Cell. Longev. 2018:9015765. doi: 10.1155/2018/9015765
Chen, P. S., Wang, C. C., Bortner, C. D., Peng, G. S., Wu, X., Pang, H., et al. (2007). ‘Valproic acid and other histone deacetylase inhibitors induce microglial apoptosis and attenuate lipopolysaccharide-induced dopaminergic neurotoxicity’. Neuroscience 149, 203–212. doi: 10.1016/j.neuroscience.2007.06.053
Cho, J. H., Bhutani, S., Kim, C. H., and Irwin, M. R. (2021). ‘Anti-inflammatory effects of melatonin: A systematic review and meta-analysis of clinical trials’. Brain Behav. Immun. 93, 245–253.
Claustrat, B., and Leston, J. (2015). ‘Melatonin: Physiological effects in humans’. Neurochirurgie 61, 77–84.
de Faria Poloni, J., Feltes, B. C., and Bonatto, D. (2011). Melatonin as a central molecule connecting neural development and calcium signaling. Funct. Integr. Genom. 11, 383–388. doi: 10.1007/s10142-011-0221-8
DeLeo, J. A., and Yezierski, R. P. (2001). ‘The role of neuroinflammation and neuroimmune activation in persistent pain’. Pain 90, 1–6.
Dermanowski, M. M., Hejduk, A., Kuczyñska, J., Wichniak, A., Urbañska, A., and Mierzejewski, P. (2022). ‘Assessment of dim light melatonin onset based on plasma and saliva samples’. Chronobiol. Int. 39, 626–635.
Du, J., Zayed, A. A., Kigerl, K. A., Zane, K., Sullivan, M. B., and Popovich, P. G. (2021). ‘Spinal cord injury changes the structure and functional potential of gut bacterial and viral communities’. mSystems 6:e01356–20. doi: 10.1128/mSystems.01356-20
Dubocovich, M. L., Delagrange, P., Krause, D. N., Sugden, D., Cardinali, D. P., and Olcese, J. (2010). ‘International union of basic and clinical pharmacology. LXXV. nomenclature, classification, and pharmacology of G protein-coupled melatonin receptors’. Pharmacol. Rev. 62, 343–380. doi: 10.1124/pr.110.002832
Ervin, S. M., Simpson, J. B., Gibbs, M. E., Creekmore, B. C., Lim, L., Walton, W. G., et al. (2020). ‘Structural insights into endobiotic reactivation by human gut microbiome-encoded sulfatases’. Biochemistry 59, 3939–3950. doi: 10.1021/acs.biochem.0c00711
Faden, A. I., Demediuk, P., Panter, S. S., and Vink, R. (1989). ‘The role of excitatory amino acids and NMDA receptors in traumatic brain injury’. Science 244, 798–800.
Furness, J. B., Callaghan, B. P., Rivera, L. R., and Cho, H. J. (2014). ‘The enteric nervous system and gastrointestinal innervation: Integrated local and central control’. Adv. Exp. Med. Biol. 817, 39–71.
Galvão Mde, O., Sinigaglia-Coimbra, R., Kawakami, S. E., Tufik, S., and Suchecki, D. (2009). Paradoxical sleep deprivation activates hypothalamic nuclei that regulate food intake and stress response. Psychoneuroendocrinology 34, 1176–1183. doi: 10.1016/j.psyneuen.2009.03.003
Gao, J., Xu, K., Liu, H., Liu, G., Bai, M., Peng, C., et al. (2018). ‘Impact of the gut microbiota on intestinal immunity mediated by tryptophan metabolism’. Front. Cell. Infect. Microbiol. 8:13. doi: 10.3389/fcimb.2018.00013
Gao, K., Niu, J., and Dang, X. (2020). ‘Neuroprotection of melatonin on spinal cord injury by activating autophagy and inhibiting apoptosis via SIRT1/AMPK signaling pathway’. Biotechnol. Lett. 42, 2059–2069.
Gao, T., Wang, Z., Cao, J., Dong, Y., and Chen, Y. (2020). ‘Melatonin attenuates microbiota dysbiosis of jejunum in short-term sleep deprived mice’. J. Microbiol. 58, 588–597. doi: 10.1007/s12275-020-0094-4
Gao, T., Wang, Z., Cao, J., Dong, Y., and Chen, Y. (2021). Melatonin ameliorates corticosterone-mediated oxidative stress-induced colitis in sleep-deprived mice involving gut microbiota. Oxid. Med. Cell. Longev. 2021:9981480. doi: 10.1155/2021/9981480
Gao, T., Wang, Z., Dong, Y., Cao, J., Lin, R., Wang, X., et al. (2019). ‘Role of melatonin in sleep deprivation-induced intestinal barrier dysfunction in mice’. J. Pineal Res. 67:e12574. doi: 10.1111/jpi.12574
Gensel, J. C., and Zhang, B. (2015). ‘Macrophage activation and its role in repair and pathology after spinal cord injury’. Brain Res. 1619, 1–11.
Gerdin, M. J., Masana, M. I., and Dubocovich, M. L. (2004). ‘Melatonin-mediated regulation of human MT(1) melatonin receptors expressed in mammalian cells’. Biochem. Pharmacol. 67, 2023–2030.
Ghareghani, M., Reiter, R. J., Zibara, K., and Farhadi, N. (2018). ‘Latitude, vitamin d, melatonin, and gut microbiota act in concert to initiate multiple sclerosis: A new mechanistic pathway’. Front. Immunol. 9:2484. doi: 10.3389/fimmu.2018.02484
Giannoulia-Karantana, A., Vlachou, A., Polychronopoulou, S., Papassotiriou, I., and Chrousos, G. P. (2006). ‘Melatonin and immunomodulation: Connections and potential clinical applications’. Neuroimmunomodulation 13, 133–144. doi: 10.1159/000097258
Glebezdina, N. S., Olina, A. A. I., Nekrasova, V., and Kuklina, E. M. (2019). ‘Molecular mechanisms of control of differentiation of regulatory T-lymphocytes by exogenous melatonin’. Dokl. Biochem. Biophys. 484, 13–16. doi: 10.1134/S1607672919010058
Glickman, S., and Kamm, M. A. (1996). ‘Bowel dysfunction in spinal-cord-injury patients’. Lancet 347, 1651–1653.
Gungor, B., Adiguzel, E., Gursel, I., Yilmaz, B., and Gursel, M. (2016). ‘Intestinal microbiota in patients with spinal cord injury’. PLoS One 11:e0145878. doi: 10.1371/journal.pone.0145878
Hagi, T., and Belzer, C. (2021). ‘The interaction of akkermansia muciniphila with host-derived substances, bacteria and diets’. Appl. Microbiol. Biotechnol. 105, 4833–4841. doi: 10.1007/s00253-021-11362-3
He, X., Lin, S., Yang, L., Tan, P., Ma, P., Qiu, P., et al. (2021). ‘Programmed death protein 1 is essential for maintaining the anti-inflammatory function of infiltrating regulatory T cells in a murine spinal cord injury model’. J. Neuroimmunol. 354:577546. doi: 10.1016/j.jneuroim.2021.577546
Heintz-Buschart, A., and Wilmes, P. (2018). ‘Human gut microbiome: Function matters’. Trends Microbiol. 26, 563–574.
Hellenbrand, D. J., Quinn, C. M., Piper, Z. J., Morehouse, C. N., Fixel, J. A., and Hanna, A. S. (2021). ‘Inflammation after spinal cord injury: A review of the critical timeline of signaling cues and cellular infiltration’. J. Neuroinflamm. 18:284. doi: 10.1186/s12974-021-02337-2
Holmes, G. M., Hubscher, C. H., Krassioukov, A., Jakeman, L. B., and Kleitman, N. (2020). ‘Recommendations for evaluation of bladder and bowel function in pre-clinical spinal cord injury research’. J. Spinal Cord Med. 43, 165–176. doi: 10.1080/10790268.2019.1661697
Huang, H., Young, W., Skaper, S., Chen, L., Moviglia, G., Saberi, H., et al. (2020). ‘Clinical neurorestorative therapeutic guidelines for spinal cord injury (IANR/CANR version 2019)’. J. Orthop. Translat. 20, 14–24. doi: 10.1016/j.jot.2019.10.006
Huether, G. (1993). ‘The contribution of extrapineal sites of melatonin synthesis to circulating melatonin levels in higher vertebrates’. Experientia 49, 665–670. doi: 10.1007/BF01923948
Hultén, V. D. T., Biering-Sørensen, F., Jørgensen, N. R., and Jennum, P. J. (2020). ‘A review of sleep research in patients with spinal cord injury’. J. Spinal Cord Med. 43, 775–796.
Jing, Y., Yu, Y., Bai, F., Wang, L., Yang, D., Zhang, C., et al. (2021b). ‘Effect of fecal microbiota transplantation on neurological restoration in a spinal cord injury mouse model: Involvement of brain-gut axis’. Microbiome 9:59. doi: 10.1186/s40168-021-01007-y
Jing, Y., Bai, F., and Yu, Y. (2021a). ‘Spinal cord injury and gut microbiota: A review’. Life Sci. 266:118865.
Jing, Y., Bai, F., Chen, H., and Dong, H. (2017). ‘Melatonin prevents blood vessel loss and neurological impairment induced by spinal cord injury in rats’. J. Spinal Cord Med. 40, 222–229. doi: 10.1080/10790268.2016.1227912
Jing, Y., Yang, D., Bai, F., Zhang, C., Qin, C., Li, D., et al. (2019). ‘Melatonin treatment alleviates spinal cord injury-induced gut dysbiosis in mice’. J. Neurotrauma 36, 2646–2664. doi: 10.1089/neu.2018.6012
Joscelyn, J., and Kasper, L. H. (2014). ‘Digesting the emerging role for the gut microbiome in central nervous system demyelination’. Mult. Scler. 20, 1553–1559.
Keshavarzian, A., Green, S. J., Engen, P. A., Voigt, R. M., Naqib, A., Forsyth, C. B., et al. (2015). ‘Colonic bacterial composition in Parkinson’s disease’. Mov. Disord. 30, 1351–1360.
Kesika, P., Suganthy, N., Sivamaruthi, B. S., and Chaiyasut, C. (2021). ‘Role of gut-brain axis, gut microbial composition, and probiotic intervention in Alzheimer’s disease’. Life Sci. 264:118627.
Kigerl, K. A., Gensel, J. C., Ankeny, D. P., Alexander, J. K., Donnelly, D. J., and Popovich, P. G. (2009). ‘Identification of two distinct macrophage subsets with divergent effects causing either neurotoxicity or regeneration in the injured mouse spinal cord’. J. Neurosci. 29, 13435–13444.
Kigerl, K. A., Hall, J. C., Wang, L., Mo, X., Yu, Z., and Popovich, P. G. (2016). ‘Gut dysbiosis impairs recovery after spinal cord injury’. J. Exp. Med. 213, 2603–2620.
Kim, J., Oh, J. K., Kim, S. W., Yee, J. S., and Kim, T. H. (2021). ‘Risk factors for sleep disturbance in patients with cervical myelopathy and its clinical significance: A cross-sectional study’. Spine J. 21, 96–104. doi: 10.1016/j.spinee.2020.09.002
Kim, S. W., Kim, S., Son, M., Cheon, J. H., and Park, Y. S. (2020). ‘Melatonin controls microbiota in colitis by goblet cell differentiation and antimicrobial peptide production through Toll-like receptor 4 signalling’. Sci. Rep. 10:2232. doi: 10.1038/s41598-020-59314-7
Lee, J., d’Aigle, J., Atadja, L., Quaicoe, V., Honarpisheh, P., Ganesh, B. P., et al. (2020). ‘Gut microbiota-derived short-chain fatty acids promote poststroke recovery in aged mice’. Circ. Res. 127, 453–465. doi: 10.1161/CIRCRESAHA.119.316448
Lefèvre, C., Bessard, A., Aubert, P., Joussain, C., Giuliano, F., Behr-Roussel, D., et al. (2020). ‘Enteric nervous system remodeling in a rat model of spinal cord injury: A pilot study’. Neurotrauma Rep. 1, 125–136. doi: 10.1089/neur.2020.0041
Ley, R. E., Bäckhed, F., Turnbaugh, P., Lozupone, C. A., Knight, R. D., and Gordon, J. I. (2005). ‘Obesity alters gut microbial ecology’. Proc. Natl. Acad. Sci. U.S.A. 102, 11070–11075.
Ley, R. E., Turnbaugh, P. J., Klein, S., and Gordon, J. I. (2006). ‘Microbial ecology: Human gut microbes associated with obesity’. Nature 444, 1022–1023.
Li, C., Chen, X., Qiao, S., Liu, X., Liu, C., Zhu, D., et al. (2014). Melatonin lowers edema after spinal cord injury. Neural Regen. Res. 9, 2205–2210. doi: 10.4103/1673-5374.147954
Li, G., Lv, D., Yao, Y., Wu, H., Wang, J., Deng, S., et al. (2021). ‘Overexpression of ASMT likely enhances the resistance of transgenic sheep to brucellosis by influencing immune-related signaling pathways and gut microbiota’. FASEB J. 35:e21783. doi: 10.1096/fj.202100651R
Li, H., Sun, J., Du, J., Wang, F., Fang, R., Yu, C., et al. (2018). ‘Clostridium butyricum exerts a neuroprotective effect in a mouse model of traumatic brain injury via the gut-brain axis’. Neurogastroenterol. Motil. 30:e13260. doi: 10.1111/nmo.13260
Li, H., Xu, H., Li, Y., Jiang, Y., Hu, Y., Liu, T., et al. (2020). ‘Alterations of gut microbiota contribute to the progression of unruptured intracranial aneurysms’. Nat. Commun. 11:3218. doi: 10.1038/s41467-020-16990-3
Li, J., Van Der Pol, W., Eraslan, M., McLain, A., Cetin, H., Cetin, B., et al. (2022). ‘Comparison of the gut microbiome composition among individuals with acute or long-standing spinal cord injury vs. able-bodied controls’. J. Spinal Cord Med. 45, 91–99.
Li, Y., Guo, Y., Fan, Y., Tian, H., Li, K., and Mei, X. (2019). ‘Melatonin enhances autophagy and reduces apoptosis to promote locomotor recovery in spinal cord injury via the PI3K/AKT/mTOR signaling pathway’. Neurochem. Res. 44, 2007–2019. doi: 10.1007/s11064-019-02838-w
Li, Z., Li, X., Chan, M. T. V., Wu, W. K. K., Tan, D., and Shen, J. (2017). ‘Melatonin antagonizes interleukin-18-mediated inhibition on neural stem cell proliferation and differentiation’. J. Cell. Mol. Med. 21, 2163–2171. doi: 10.1111/jcmm.13140
Liang, S., Wu, X., Hu, X., Wang, T., and Jin, F. (2018). ‘Recognizing depression from the microbiota-gut-brain axis’. Int. J. Mol. Sci. 19:1592.
Lin, R., Wang, Z., Cao, J., Gao, T., Dong, Y., and Chen, Y. (2021). ‘Role of melatonin in murine “restraint stress”-induced dysfunction of colonic microbiota’. J. Microbiol. 59, 500–512. doi: 10.1007/s12275-021-0305-7
Liu, X., Liang, F., Song, W., Diao, X., Zhu, W., and Yang, J. (2021). ‘Effect of Nrf2 signaling pathway on the improvement of intestinal epithelial barrier dysfunction by hyperbaric oxygen treatment after spinal cord injury’. Cell Stress Chaperones 26, 433–441. doi: 10.1007/s12192-020-01190-1
Liu, X., Liang, F., Zhang, J., Li, Z., Yang, J., and Kang, N. (2020). ‘Hyperbaric oxygen treatment improves intestinal barrier function after spinal cord injury in rats’. Front. Neurol. 11:563281. doi: 10.3389/fneur.2020.563281
Liu, X., Wang, Y., Yang, J., Liu, Y., Zhou, D., Hou, M., et al. (2015). Anti-edema effect of melatonin on spinal cord injury in rats. Biomed. Pap. Med. Fac. Univ. Palacky Olomouc Czech. Repub. 159, 220–226. doi: 10.5507/bp.2015.012
Lutfi, E., Basili, D., Falcinelli, S., Morillas, L., Carnevali, O., Capilla, E., et al. (2021). ‘The probiotic lactobacillus rhamnosus mimics the dark-driven regulation of appetite markers and melatonin receptors’ expression in zebrafish (Danio rerio) larvae: Understanding the role of the gut microbiome’. Comp. Biochem. Physiol. B Biochem. Mol. Biol. 256:110634. doi: 10.1016/j.cbpb.2021.110634
Lynch, A. C., Antony, A., Dobbs, B. R., and Frizelle, F. A. (2001). ‘Bowel dysfunction following spinal cord injury’. Spinal Cord 39, 193–203.
Magruder, M., Edusei, E., Zhang, L., Albakry, S., Satlin, M. J., Westblade, L. F., et al. (2020). ‘Gut commensal microbiota and decreased risk for Enterobacteriaceae bacteriuria and urinary tract infection’. Gut Microbes 12:1805281. doi: 10.1080/19490976.2020.1805281
Magruder, M., Sholi, A. N., Gong, C., Zhang, L., Edusei, E., Huang, J., et al. (2019). ‘Gut uropathogen abundance is a risk factor for development of bacteriuria and urinary tract infection’. Nat. Commun. 10:5521. doi: 10.1038/s41467-019-13467-w
Majidpoor, J., Khezri, Z., Rostamzadeh, P., Mortezaee, K., Rezaie, M. J., Fathi, F., et al. (2020). ‘The expressions of NLRP1, NLRP3, and AIM2 inflammasome complexes in the contusive spinal cord injury rat model and their responses to hormonal therapy’. Cell Tissue Res. 381, 397–410. doi: 10.1007/s00441-020-03250-5
Majidpoor, J., Mortezaee, K., Khezri, Z., Fathi, F., Zali, A., Derakhshan, H. B., et al. (2021). ‘The effect of the “segment” of spinal cord injury on the activity of the nucleotide-binding domain-like receptor protein 3 inflammasome and response to hormonal therapy’. Cell Biochem. Funct. 39, 267–276. doi: 10.1002/cbf.3574
Markowiak-Kopeć, P., and liżewska, K. (2020). ‘The effect of probiotics on the production of short-chain fatty acids by human intestinal microbiome’. Nutrients 12:1107.
Mazzoccoli, G., Carughi, S., Sperandeo, M., Pazienza, V., Giuliani, F., and Tarquini, R. (2011). ‘Neuro-endocrine correlations of hypothalamic-pituitary-thyroid axis in healthy humans’. J. Biol. Regul. Homeost. Agents 25, 249–257.
Møller, M., and Baeres, F. M. (2002). ‘The anatomy and innervation of the mammalian pineal gland’. Cell Tissue Res. 309, 139–150.
O’Connor, G., Jeffrey, E., Madorma, D., Marcillo, A., Abreu, M. T., Deo, S. K., et al. (2018). ‘Investigation of microbiota alterations and intestinal inflammation post-spinal cord injury in rat model’. J. Neurotrauma 35, 2159–2166. doi: 10.1089/neu.2017.5349
Onaolapo, O. J., Onaolapo, A. Y., Olowe, O. A., Udoh, M. O., Udoh, D. O., and Nathaniel, T. I. (2020). ‘Melatonin and melatonergic influence on neuronal transcription factors: Implications for the development of novel therapies for neurodegenerative disorders’. Curr. Neuropharmacol. 18, 563–577. doi: 10.2174/1570159X18666191230114339
Opeyemi, O. M., Rogers, M. B., Firek, B. A., Janesko-Feldman, K., Vagni, V., Mullett, S. J., et al. (2021). ‘Sustained dysbiosis and decreased fecal short-chain fatty acids after traumatic brain injury and impact on neurologic outcome’. J. Neurotrauma 38, 2610–2621. doi: 10.1089/neu.2020.7506
Oyinbo, C. A. (2011). ‘Secondary injury mechanisms in traumatic spinal cord injury: A nugget of this multiply cascade’. Acta Neurobiol. Exp. 71, 281–299.
Park, J. S., Woo, M. S., Kim, S. Y., Kim, W. K., and Kim, H. S. (2005). ‘Repression of interferon-gamma-induced inducible nitric oxide synthase (iNOS) gene expression in microglia by sodium butyrate is mediated through specific inhibition of ERK signaling pathways’. J. Neuroimmunol. 168, 56–64. doi: 10.1016/j.jneuroim.2005.07.003
Park, Y. S., Kim, S. H., Park, J. W., Kho, Y., Seok, P. R., Shin, J. H., et al. (2020). ‘Melatonin in the colon modulates intestinal microbiota in response to stress and sleep deprivation’. Intest. Res. 18, 325–336. doi: 10.5217/ir.2019.00093
Paterniti, I., Campolo, M., Cordaro, M., Impellizzeri, D., Siracusa, R., Crupi, R., et al. (2017). ‘PPAR-α modulates the anti-inflammatory effect of melatonin in the secondary events of spinal cord injury’. Mol. Neurobiol. 54, 5973–5987. doi: 10.1007/s12035-016-0131-9
Paulose, J. K., Wright, J. M., Patel, A. G., and Cassone, V. M. (2016). ‘Human gut bacteria are sensitive to melatonin and express endogenous circadian rhythmicity’. PLoS One 11:e0146643. doi: 10.1371/journal.pone.0146643
Perez-Pardo, P., Dodiya, H. B., Engen, P. A., Forsyth, C. B., Huschens, A. M., Shaikh, M., et al. (2019). ‘Role of TLR4 in the gut-brain axis in Parkinson’s disease: A translational study from men to mice’. Gut 68, 829–843. doi: 10.1136/gutjnl-2018-316844
Pistollato, F., Sumalla Cano, S., Elio, I., Masias Vergara, M., Giampieri, F., and Battino, M. (2016). ‘Role of gut microbiota and nutrients in amyloid formation and pathogenesis of Alzheimer disease’. Nutr. Rev. 74, 624–634.
Quadri, S. A., Farooqui, M., Ikram, A., Zafar, A., Khan, M. A., Suriya, S. S., et al. (2020). ‘Recent update on basic mechanisms of spinal cord injury’. Neurosurg. Rev. 43, 425–441.
Ren, W., Liu, G., Chen, S., Yin, J., Wang, J., Tan, B., et al. (2017). ‘Melatonin signaling in T cells: Functions and applications’. J. Pineal Res. 62:12394. doi: 10.1111/jpi.12394
Ren, W., Wang, P., Yan, J., Liu, G., Zeng, B., Hussain, T., et al. (2018). ‘Melatonin alleviates weanling stress in mice: Involvement of intestinal microbiota’. J. Pineal Res. 64:12448. doi: 10.1111/jpi.12448
Rodenhouse, A., Talukder, M. A. H., Lee, J. I., Govindappa, P. K., O’Brien, M., Manto, K. M., et al. (2022). ‘Altered gut microbiota composition with antibiotic treatment impairs functional recovery after traumatic peripheral nerve crush injury in mice: Effects of probiotics with butyrate producing bacteria’. BMC Res. Notes 15:80. doi: 10.1186/s13104-022-05967-8
Rogers, W. K., and Todd, M. (2016). ‘Acute spinal cord injury’. Best Pract. Res. Clin. Anaesthesiol. 30, 27–39.
Rudzki, L., Stone, T. W., Maes, M., Misiak, B., Samochowiec, J., and Szulc, A. (2021). ‘Gut microbiota-derived vitamins - underrated powers of a multipotent ally in psychiatric health and disease’. Prog. Neuropsychopharmacol. Biol. Psychiatry 107:110240. doi: 10.1016/j.pnpbp.2020.110240
Santoru, M. L., Piras, C., Murgia, A., Palmas, V., Camboni, T., and Liggi, S. (2017). ‘Cross sectional evaluation of the gut-microbiome metabolome axis in an Italian cohort of IBD patients’. Sci. Rep. 7:9523.
Schmidt, E. K. A., Raposo, P. J. F., Torres-Espin, A., Fenrich, K. K., and Fouad, K. (2021). ‘Beyond the lesion site: Minocycline augments inflammation and anxiety-like behavior following SCI in rats through action on the gut microbiota’. J. Neuroinflamm. 18:144. doi: 10.1186/s12974-021-02123-0
Schwab, J. M., Zhang, Y., Kopp, M. A., Brommer, B., and Popovich, P. G. (2014). ‘The paradox of chronic neuroinflammation, systemic immune suppression, autoimmunity after traumatic chronic spinal cord injury’. Exp. Neurol. 258, 121–129. doi: 10.1016/j.expneurol.2014.04.023
Schwiertz, A., Taras, D., Schäfer, K., Beijer, S., Bos, N. A., Donus, C., et al. (2010). ‘Microbiota and SCFA in lean and overweight healthy subjects’. Obesity 18, 190–195.
Shen, Z., Zhou, Z., Gao, S., Guo, Y., Gao, K., Wang, H., et al. (2017). ‘Melatonin inhibits neural cell apoptosis and promotes locomotor recovery via activation of the Wnt/β-catenin signaling pathway after spinal cord injury’. Neurochem. Res. 42, 2336–2343.
Straub, R. H., Wiest, R., Strauch, U. G., Härle, P., and Schölmerich, J. (2006). ‘The role of the sympathetic nervous system in intestinal inflammation’. Gut 55, 1640–1649.
Sun, M. F., Zhu, Y. L., Zhou, Z. L., Jia, X. B., Xu, Y. D., Yang, Q., et al. (2018). ‘Neuroprotective effects of fecal microbiota transplantation on MPTP-induced Parkinson’s disease mice: Gut microbiota, glial reaction and TLR4/TNF-α signaling pathway’. Brain Behav. Immun. 70, 48–60. doi: 10.1016/j.bbi.2018.02.005
Tariq, R., Pardi, D. S., Tosh, P. K., Walker, R. C., Razonable, R. R., and Khanna, S. (2017). ‘Fecal microbiota transplantation for recurrent clostridium difficile infection reduces recurrent urinary tract infection frequency’. Clin. Infect. Dis. 65, 1745–1747. doi: 10.1093/cid/cix618
Tate, D. G., Forchheimer, M., Rodriguez, G., Chiodo, A., Cameron, A. P., Meade, M., et al. (2016). ‘Risk factors associated with neurogenic bowel complications and dysfunction in spinal cord injury’. Arch. Phys. Med. Rehabil. 97, 1679–1686.
Thøfner Hultén, V. D., Biering-Sørensen, F., Jørgensen, N. R., and Jennum, P. J. (2018). ‘Melatonin and cortisol in individuals with spinal cord injury’. Sleep Med. 51, 92–98.
Tran, A. P., Warren, P. M., and Silver, J. (2018). ‘The biology of regeneration failure and success after spinal cord injury’. Physiol. Rev. 98, 881–917.
Vasey, C., McBride, J., and Penta, K. (2021). ‘Circadian rhythm dysregulation and restoration: The role of melatonin’. Nutrients 13:3480. doi: 10.3390/nu13103480
Wang, K., Li, M., Jin, L., Deng, C., Chen, Z., Chen, H., et al. (2019). ‘Retracted article: Melatonin protects spinal cord injury by up-regulating IGFBP3 through the improvement of microcirculation in a rat model’. RSC Adv. 9, 32072–32080. doi: 10.1039/c9ra04591k
Wang, P., Qi, X., Xu, G., Liu, J., Guo, J., Li, X., et al. (2019). ‘CCL28 promotes locomotor recovery after spinal cord injury via recruiting regulatory T cells’. Aging 11, 7402–7415.
Watkins, L. R., Milligan, E. D., and Maier, S. F. (2001). ‘Spinal cord glia: New players in pain’. Pain 93, 201–205.
Wexler, A. G., and Goodman, A. L. (2017). ‘An insider’s perspective: Bacteroides as a window into the microbiome’. Nat. Microbiol. 2:17026.
Whelan, A., Halpine, M., Christie, S. D., and McVeigh, S. A. (2020). ‘Systematic review of melatonin levels in individuals with complete cervical spinal cord injury’. J. Spinal Cord Med. 43, 565–578. doi: 10.1080/10790268.2018.1505312
White, A. R., Werner, C. M., and Holmes, G. M. (2020). ‘Diminished enteric neuromuscular transmission in the distal colon following experimental spinal cord injury’. Exp. Neurol. 331:113377. doi: 10.1016/j.expneurol.2020.113377
Williams, R., and Murray, A. (2015). ‘Prevalence of depression after spinal cord injury: A meta-analysis’. Arch. Phys. Med. Rehabil. 96, 133–140.
Worby, C. J., Olson, B. S., Dodson, K. W., Earl, A. M., and Hultgren, S. J. (2022). Establishing the role of the gut microbiota in susceptibility to recurrent urinary tract infections. J. Clin. Invest. 132:158497. doi: 10.1172/jci158497
Wurtman, R. (2006). ‘Ramelteon: A novel treatment for the treatment of insomnia’. Expert Rev. Neurother. 6, 957–964.
Xiong, X., Bao, Z., Mi, Y., Wang, X., and Zhu, J. (2022). ‘Melatonin alleviates neonatal necrotizing enterocolitis by repressing the activation of the NLRP3 inflammasome’. Gastroenterol. Res. Pract. 2022:6920577. doi: 10.1155/2022/6920577
Xu, G., Shi, D., Zhi, Z., Ao, R., and Yu, B. (2019). ‘Melatonin ameliorates spinal cord injury by suppressing the activation of inflammasomes in rats’. J. Cell. Biochem. 120, 5183–5192.
Xu, L. X., Lv, Y., Li, Y. H., Ding, X., Wang, Y., Han, X., et al. (2017). ‘Melatonin alleviates brain and peripheral tissue edema in a neonatal rat model of hypoxic-ischemic brain damage: The involvement of edema related proteins’. BMC Pediatr. 17:90. doi: 10.1186/s12887-017-0824-x
Xu, P., Wang, J., Hong, F., Wang, S., Jin, X., Xue, T., et al. (2017). ‘Melatonin prevents obesity through modulation of gut microbiota in mice’. J. Pineal Res. 62:12399. doi: 10.1111/jpi.12399
Yang, Z., Bao, Y., Chen, W., and He, Y. (2020). ‘Melatonin exerts neuroprotective effects by attenuating astro- and microgliosis and suppressing inflammatory response following spinal cord injury’. Neuropeptides 79:102002. doi: 10.1016/j.npep.2019.102002
Yin, J., Li, Y., Han, H., Chen, S., Gao, J., Liu, G., et al. (2018). ‘Melatonin reprogramming of gut microbiota improves lipid dysmetabolism in high-fat diet-fed mice’. J. Pineal Res. 65:e12524. doi: 10.1111/jpi.12524
Yu, B., Qiu, H., Cheng, S., Ye, F., Li, J., Chen, S., et al. (2021). ‘Profile of gut microbiota in patients with traumatic thoracic spinal cord injury and its clinical implications: A case-control study in a rehabilitation setting’. Bioengineered 12, 4489–4499. doi: 10.1080/21655979.2021.1955543
Yuan, B., Lu, X. J., and Wu, Q. (2021). ‘Gut microbiota and acute central nervous system injury: A new target for therapeutic intervention’. Front. Immunol. 12:800796. doi: 10.3389/fimmu.2021.800796
Zhang, C., Zhang, W., Zhang, J., Jing, Y., Yang, M., Du, L., et al. (2018). ‘Gut microbiota dysbiosis in male patients with chronic traumatic complete spinal cord injury’. J. Transl. Med. 16:353. doi: 10.1186/s12967-018-1735-9
Zhang, H., Yan, A., Liu, X., Ma, Y., Zhao, F., Wang, M., et al. (2021). ‘Melatonin ameliorates ochratoxin A induced liver inflammation, oxidative stress and mitophagy in mice involving in intestinal microbiota and restoring the intestinal barrier function’. J. Hazard. Mater. 407:124489. doi: 10.1016/j.jhazmat.2020.124489
Zhang, J., Li, S., and Wu, Y. (2017). ‘Recovery of spinal cord injury following electroacupuncture in rats through enhancement of Wnt/β-catenin signaling’. Mol. Med. Rep. 16, 2185–2190. doi: 10.3892/mmr.2017.6801
Zhang, M., Bai, Y., Xu, C., Lin, J., Jin, J., Xu, A., et al. (2021). ‘Novel optimized drug delivery systems for enhancing spinal cord injury repair in rats’. Drug Deliv. 28, 2548–2561. doi: 10.1080/10717544.2021.2009937
Zhang, Y., Liu, Z., Zhang, W., Wu, Q., Zhang, Y., Liu, Y., et al. (2019). ‘Melatonin improves functional recovery in female rats after acute spinal cord injury by modulating polarization of spinal microglial/macrophages’. J. Neurosci. Res. 97, 733–743. doi: 10.1002/jnr.24409
Zhao, Z. X., Yuan, X., Cui, Y. Y., Liu, J., Shen, J., Jin, B. Y., et al. (2021). ‘Melatonin mitigates oxazolone-induced colitis in microbiota-dependent manner’. Front. Immunol. 12:783806. doi: 10.3389/fimmu.2021.783806
Zheng, B., Hao, D., Guo, H., and He, B. (2017). ‘Melatonin alleviates acute spinal cord injury in rats through promoting on progenitor cells proliferation’. Saudi Pharm. J. 25, 570–574.
Zhou, X., He, X., and Ren, Y. (2014). ‘Function of microglia and macrophages in secondary damage after spinal cord injury’. Neural Regen. Res. 9, 1787–1795.
Keywords: spinal cord injury, melatonin, intestinal microbiota, secondary injury, complications, treatment spinal cord injury, treatment
Citation: Zhang Y, Lang R, Guo S, Luo X, Li H, Liu C, Dong W, Bao C and Yu Y (2022) Intestinal microbiota and melatonin in the treatment of secondary injury and complications after spinal cord injury. Front. Neurosci. 16:981772. doi: 10.3389/fnins.2022.981772
Received: 29 June 2022; Accepted: 24 October 2022;
Published: 09 November 2022.
Edited by:
Xiaoming Jin, Indiana University–Purdue University Indianapolis, United StatesReviewed by:
Yue-Li Sun, Shanghai University of Traditional Chinese Medicine, ChinaCopyright © 2022 Zhang, Lang, Guo, Luo, Li, Liu, Dong, Bao and Yu. This is an open-access article distributed under the terms of the Creative Commons Attribution License (CC BY). The use, distribution or reproduction in other forums is permitted, provided the original author(s) and the copyright owner(s) are credited and that the original publication in this journal is cited, in accordance with accepted academic practice. No use, distribution or reproduction is permitted which does not comply with these terms.
*Correspondence: Wei Dong, ZG9uZ3dlaUBzd211LmVkdS5jbg==; Changshun Bao, YmNzNzU2QDEyNi5jb20=; Yang Yu, eXV5YW5nODBAc3dtdS5lZHUuY24=
†These authors have contributed equally to this work and share first authorship
Disclaimer: All claims expressed in this article are solely those of the authors and do not necessarily represent those of their affiliated organizations, or those of the publisher, the editors and the reviewers. Any product that may be evaluated in this article or claim that may be made by its manufacturer is not guaranteed or endorsed by the publisher.
Research integrity at Frontiers
Learn more about the work of our research integrity team to safeguard the quality of each article we publish.