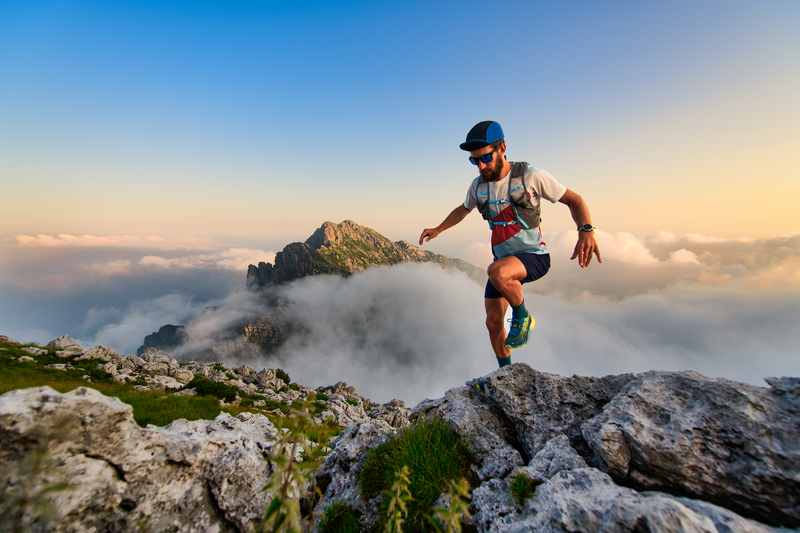
95% of researchers rate our articles as excellent or good
Learn more about the work of our research integrity team to safeguard the quality of each article we publish.
Find out more
REVIEW article
Front. Neurosci. , 22 July 2022
Sec. Neural Technology
Volume 16 - 2022 | https://doi.org/10.3389/fnins.2022.964060
This article is part of the Research Topic Ultrasound Neuromodulation: Mechanisms and Applications View all 8 articles
Ischemic stroke is a serious medical condition that is caused by cerebral vascular occlusion and leads to neurological dysfunction. After stroke, patients suffer from long-term sensory, motor and cognitive impairment. Non-invasive neuromodulation technology has been widely studied in the field of stroke rehabilitation. Transcranial ultrasound stimulation (TUS), as a safe and non-invasive technique with deep penetration ability and a tiny focus, is an emerging technology. It can produce mechanical and thermal effects by delivering sound waves to brain tissue that can induce the production of neurotrophic factors (NFs) in the brain, and reduce cell apoptosis and the inflammatory response. TUS, which involves application of an acoustic wave, can also dissolve blood clots and be used to deliver therapeutic drugs to the ischemic region. TUS has great potential in the treatment of ischemic stroke. Future advancements in imaging and parameter optimization will improve the safety and efficacy of this technology in the treatment of ischemic stroke.
Stroke is one of the major causes of chronic disability worldwide (Zhu et al., 2022). Stroke can be classified as ischemic or hemorrhagic stroke, with ischemic stroke accounting for approximately 75% of all stroke cases (Donkor, 2018). This cerebrovascular condition can cause a considerable number of functional limitations and can lead to death in severe cases (Sousa et al., 2009). In the clinic, stroke treatment often involves thrombolysis and surgical recanalization (Paul and Candelario-Jalil, 2021). Thrombolytic therapy includes drug thrombolysis and interventional thrombectomy. At present, intravenous thrombolysis has certain limitations. For example, the classic treatment, i.e., intravenous injection of tissue plasminogen activator (tPA), has a short treatment window, and the risk of bleeding complications is high. tPA is also not suitable for patients with comorbidities, such as bleeding, hypertension and those who are on anticoagulation therapy. The clinical application of interventional is limited by technical challenges, equipment requirements and high cost (Powers et al., 2019). Therefore, research on safe and effective new treatment approaches for promoting nerve recovery after stroke is of great significance.
Non-invasive neuromodulation techniques, including transcranial magnetic stimulation (TMS), transcranial direct current stimulation (tDCS) and transcranial alternating current stimulation (tACS), have been applied in clinical settings to promote neural plasticity and improve function in stroke patients (Cantone et al., 2021). TMS and tDCS have different effects on neurons (Woods et al., 2016). TMS modulates neuronal excitability by generating a magnetic field that induces neuronal depolarization (Antczak et al., 2021), and it can also cause vascular cognitive impairment, which may lead to dementia (Cantone et al., 2020; Di Lazzaro et al., 2021). tDCS and tACS can modulate cortical excitability by hyperpolarization or depolarization neuronal resting membrane potentials (Pol et al., 2021). However, Airan reported that the absorption and scattering of magnetic and electrical signals within the brain limits the spatial resolution and penetration depth of these techniques, which further hinders their application for the treatment of stroke (Airan, 2017). Studies have found that transcranial focused ultrasound stimulation (TFUS), a non-invasive, high resolution and safe technology, can modulate neural activity and exert neuroprotective effects (Li et al., 2016; Baek et al., 2018). Ultrasound stimulation has been used to treat cancer, neurodegenerative diseases, diabetes and thrombosis (Miller and O’Callaghan, 2017; Tharkar et al., 2019; Zhang et al., 2019; Ma et al., 2021). Low-intensity focused ultrasound stimulation (LIFUS) has been shown to be efficacious in the treatment of a variety of neurological and psychiatric disorders (Li et al., 2017; Wang et al., 2022b) such as Parkinson’s disease (PD), Alzheimer’s disease (AD) epilepsy and stroke, and animal experiments have shown that LIFUS does not cause any tissue damage when used to treat stroke, proving that transcranial ultrasound stimulation (TUS) in the treatment of ischemic stroke has good research prospects and has the potential to become a non-invasive treatment method (Min et al., 2011; Liu et al., 2019; Shin et al., 2019; Ma et al., 2021).
This narrative review provides an overview of the current literature on the application of TUS in animal models and human subjects, discusses the potential effects of TUS in the treatment of ischemic stroke and the various treatment methods, and provide further theoretical and technical support for the clinical application of TUS.
The applications of focused ultrasound have been increasing, and diagnostic ultrasound has been established as a critical clinical imaging modality (Trockel et al., 1984; Fu et al., 2015; Nainwal, 2017; Darrow, 2019; di Biase et al., 2021; Liu et al., 2021). TFUS is a non-invasive technology that can be used to monitor cerebral circulation (Thomassen et al., 2021). Spatially limited energy can be delivered to brain tissue at a wide range of intensities, allowing high temporal resolution and spatial visualization of the intracranial and extracranial arteries (Sharma et al., 2011). In addition, TFUS can be used to enhance or inhibit nervous system activity by adjusting the frequency (Kubanek, 2018b), intensity and stimulation time (Kubanek, 2018b; Tyler et al., 2018).
Transcranial focused ultrasound stimulation waves are transmitted to human tissue in a continuous or pulsed form through an ultrasonic probe (Zhang et al., 2022). They can modulate neural excitability (Kamimura et al., 2020) by producing mechanical effects (Blackmore et al., 2019) through alterations in ion channels (Yoo et al., 2011), membrane capacitance (Plaksin et al., 2014), the generation of sonopores (Tata and Dunn, 1992) and interfacial elastic wave coupling, and by producing thermal effects through the rise in temperature caused by sound waves (Cesare et al., 1999; Kamimura et al., 2020), thus, ultrasonic stimulation is a potential non-invasive treatment for neurological diseases. The therapeutic effect of TUS is mainly influenced by the carrier frequency, peak intensity, duration, pulse repetition frequency and duty cycle (Kubanek, 2018a). Ultrasound stimulation at different parameters induces different therapeutic effects. TFUS can utilize ultrasound phased array technology to electronically drive an ultrasound transducer array to direct focused ultrasound beams to different neural targets, enabling large-scale ultrasound neuromodulation within a given tissue volume (Monteith et al., 2013; Ilham et al., 2021), it can also transmit acoustic energy to a target area in the brain through single-element transducers, acting on a focal point (Park et al., 2022). LIFU (frequency:<1 MHz; intensity: 0.5-100 W/cm2) (Tyler et al., 2018) stimulates nerve tissue mainly through the pressure generated by ultrasonic radiation, improving the blood supply around the brain lesion by means of neural regulation without causing tissue damage (Bystritsky et al., 2011; Fomenko et al., 2018; Wang et al., 2022a). Therefore, LIFU is a good option for non-invasive treatment of ischemic stroke.
It is well known that some important neural factors, such as vascular endothelial growth factor (VEGF) and brain-derived neurotrophic factor (BDNF), have a protective effect against ischemic brain injury and are potential therapeutic targets. Angiogenesis is closely related to neurogenesis in the adult mammalian brain (Palmer et al., 2000). VEGF can regulate axonal growth, neuronal survival, and neovascularization (Greenberg and Jin, 2005). BDNF is a neurotrophin that is widely expressed in the central nervous system and plays a key role in memory, the differentiation and survival of neurons and synaptic plasticity (Bartkowska et al., 2007; Balkaya and Cho, 2019). Low-intensity pulsed ultrasound stimulation (LIPUS) has been proven to have a neuroprotective effect on brain injury (Bretsztajn and Gedroyc, 2018). Short-term application of transcranial pulse ultrasound stimulation can increase in the density of BDNF-positive lacrimal spots in the hippocampus, suggesting that ultrasound can stimulate hippocampal neuronal activity and promote endogenous brain plasticity (Tufail et al., 2010). LIPUS can also increase the expression of BDNF and VEGF in astrocytes and inhibit cell apoptosis (Yang et al., 2015; Su et al., 2017). LIPUS was applied to the brains of mice before middle cerebral artery occlusion (MCAO)-induced cerebral ischemic injury and was found to significantly reduce neuron apoptosis of neurons in brain tissue, prevent a decline in cell viability, ameliorate neuronal injury, and alleviate ischemic stroke (Chen et al., 2018). Application of LIPUS for 9 consecutive days before secondary MCAO was shown to reduce mortality, attenuate pathological changes, and significantly reduce neuronal apoptosis in mice with recurrent stroke (Wu et al., 2019). Postischemia angiogenesis regulates axon growth and neurogenesis, including the proliferation, migration, maturation of nerve stem/progenitor which contribute to functional recovery (Taguchi et al., 2015). Ultrasound has the ability to vascularize endothelial cells (ECs) and promote angiogenesis (Imashiro et al., 2021). Application of LIPUS for 20 min a day for 4 weeks was shown to upregulate VEGF expression and enhance endothelial nitric oxide synthase (eNOS) activity in vitro (Hanawa et al., 2014). LIPUS was a found to significantly increase the number of peripheral CD31-positive blood vessels and ischemic striatum doublecortin (DCX)-positive neurons, and the gene expression levels of eNOS, VEGF, and fibroblast growth factor. Activated ECs secrete VEGF to increase neurogenesis. LIPUS is likely to promote the proliferation and migration of neural stem cells by establishing suitable blood vessels as scaffolds, and promote the repair of brain injury after stroke by inducing expression of a series of neurotrophic factors (NFs) (Ichijo et al., 2021).
Transcranial ultrasound stimulation can increase the expression of BDNF and VEGF, which play a protective role during brain injury rehabilitation, in the brain. Thus, TUS may be applied a novel approach for clinical stroke treatment (Figure 1).
Oxygen and energy consumption after ischemic stroke trigger a cascade of damage, including an inflammatory response that leads to severe brain damage. After ischemic stroke, microglia are activated and produce both harmful and neuroprotective mediators. The balance between these types of mediators determines the outcome of neuronal damage (Zhao et al., 2017). Any disruption or loss of cerebral homeostasis with real or potential effects on the central nervous system causes rapid and intense changes in microglial shape, gene expression, and function. These changes are known as microglial activation (Kettenmann et al., 2011). When brain injury occurs, microglia become activated and are polarized toward the M1 or M2 phenotype. Inflammatory M1 microglia secrete inflammatory factors, which may exacerbate autologous brain injury in early ischemic stroke, and M2 microglia secrete the anti-inflammatory cytokine IL-10, which in turn can induce the polarization of microglia toward the M2 phenotype. IL-10 is an important immunomodulator in the central nervous system and a key factor in poststroke recovery. It can not only suppress the inflammatory response but also alter neurogenesis and promote synaptic remodeling (Wang et al., 2021). LIPUS can significantly reduce the levels of TNF-α, IL-1β, and IL-6 in microglia, and inhibit the expression of proinflammatory mediators in microglia and microglial apoptosis to control the inflammatory response (Chang et al., 2020). In a previous study, TUS was applied to stimulate the ischemic hemisphere in mice 1 week after cerebral ischemia. Microglia in the ischemic brain area were polarized toward the M2 phenotype, the expression levels of IL-10R and IL-10 in the brain were increased, the cerebral infarct volume was significantly reduced, and neurological severity scores and behavioral scores were improved (Wang et al., 2021). Ultrasound can induce the polarization of microglia toward the M2 phenotype through the IL-4 signaling pathway, protect against brain injury in mice and promote functional recovery by alleviating nerve function defect, inhibiting nerve cell apoptosis and reducing the destruction of the blood-brain barrier (BBB). It can also promote tissue reconstruction and nerve regeneration. Engineered platelet-fused microglia can achieve functional neuronal regeneration after ischemic stroke (Li et al., 2021). After stroke, the number of neutrophils increases rapidly, which leads to the disruption of the BBB, cerebral edema, and brain damage. In response to the mutual interaction between neutrophils and the cerebral endothelium, a large amount of neutrophil-derived reactive oxygen species, proteases and cytokines are released in the brain (Jickling et al., 2015). Reactive microglia surrounding the infarct can engulf neutrophils, preventing neutrophils from accumulating in this area (Otxoa-de-Amezaga et al., 2019). Applying low-intensity pulsed transcranial ultrasound stimulation (PTUS) with a frequency of 0.5 MHz to the ischemic cortex after distal occlusion of the middle cerebral artery can reduce the number of neutrophils in the damaged area and the inflammatory response in the brain (Guo et al., 2015).
TUS and LIPUS can modulate neural activity in different brain regions by affecting the polarization of microglia in the brain. TUS and LIPUS can induce the polarization of microglia toward the protective phenotype and reduce the detrimental effect of neutrophil injury; thus, they are effective in reducing inflammation after stroke.
Transcranial focused ultrasound stimulation can improve or restore blood flow in blood vessels blocked by a clot (Birnbaum et al., 1998). However, an important factor to be considered in ultrasound thrombolysis is the size and concentration of the clot fragments, as they may block the micro vessels in the distal vascular bed. It is generally believed that the efficacy of ultrasound thrombolysis is based on its cavitation effect, which facilitates the dissolution of thrombi (Table 1). Under ultrasonic irradiation, tiny bubbles in the blood vibrate and grow under the action of the ultrasonic field and continuously gather the energy of the sound field; when the energy reaches a certain threshold, the cavitated bubbles collapse, promoting the dissolution of the thrombus (Kubanek, 2018a). However, TFUS is often used as an auxiliary thrombolytic method to enhance the efficacy of intravenous thrombolytic drugs (Wright et al., 2012). It has been reported that ultrasound at a frequency of 490 kHz and an intensity of 0.13 W/cm2 can promote tPA thrombolytic effect and improve blood perfusion (Ishibashi et al., 2002). Ultrasound stimulation at a frequency of 490kHz and an intensity of 0.8 W/cm2 can enhance the thrombolytic effect of tPA, significantly reducing the cerebral infarct volume and improving neurological function without causing hemorrhagic complications (Saguchi et al., 2008). A clinical study also confirmed that continuous TUS can enhance tPA-induced arterial recanalization (Alexandrov et al., 2004). In addition to thrombolytic drugs, microbubble-assisted thrombolysis can greatly enhance the effect of thrombolytic therapy, and there is no difference in effectiveness between microbubble-assisted thrombolysis and thrombolytic therapy involving different microbubbles (Schleicher et al., 2016). Ultrasound combined with microbubbles can effectively remove thrombi when combined with a very low dose of tPA or in the absence of tPA, and significantly reduce the infarct volume without causing obvious side effects (Brown et al., 2011; Culp et al., 2011). High mechanical index (MI) PTUS combined with microbubbles can dissolve thrombi and improve ipsilateral and contralateral cerebral blood flow after acute cerebral embolism (Gao et al., 2014). A combination of microbubbles with transcranial TUS at a frequency of 1 MHz and intensity of 2.0 W/cm2 can rapidly resolve acute intracranial thrombotic occlusions (Culp et al., 2004). Thrombolytic efficacy can be further enhanced through the use of targeted microbubbles (TMBs). This may be because adhesion between microbubbles and the thrombus is enhanced and the concentration of bubbles around the thrombosis increased, leading to promotion of the cavitation effect and thus enhancement of the thrombolytic effect. The combination of thrombolytic therapy and recombinant tissue plasminogen activator (r-tPA) is safer than the application of r-tPA alone and decreases the risk of intracranial hemorrhage (Ren et al., 2012). Since the combination of ultrasound and microbubbles has an enhanced thrombolytic effect and does not increase the incidence of cerebral hemorrhage, r-tPA can be administered during thrombolytic therapy (Lu et al., 2016). It has also been reported that the addition of microbubbles enhances the effects of transcranial ultrasound-assisted urokinase thrombolysis, significantly reducing the infarct size without increasing the risk of cerebral hemorrhage (Liu et al., 2012).
Table 1. Summary of transcranial focused ultrasound in neuromodulation and reduction of inflammatory responses.
The cavitation effect is the main mechanism underlying the effect of ultrasonic thrombolysis. The effectiveness and safety of ultrasonic thrombolysis have also been confirmed by a large number of experimental studies. However, to date, there is no consensus on the optimal parameters of ultrasonic thrombolysis, and further exploration is needed.
Ultrasound combined with microbubble-mediated drug delivery is a non-invasive, targeted drug delivery approach that can be guided by imaging technology through the interaction of microbubbles undergoing acoustic cavitation and cells. Delivery of therapeutic substances to the target tissue or organ through an ultrasonic microbubble contrast agent mainly relies on ultrasonic TMB destruction technology. The therapeutic agent is released at a specific point under the action of ultrasonic irradiation for diagnosis or treatment. Oscillation and implosion of the microbubbles result in an increase in temperature (Wood and Sehgal, 2015), shock waves, the shear stress, mechanical stress, and the generation of free radicals (Barnett et al., 1994; Rosenthal et al., 2004; Ashokkumar, 2011; Sanwal et al., 2021). These phenomena are the main mechanisms of ultrasound-enhanced targeted drug delivery (Shin Low et al., 2021). The BBB is a key factor affecting the delivery of drugs to the central nervous system. Ultrasound can induce opening of the BBB and promote drug delivery. Ohta et al. (2020) investigated the effect of size on the ability of nanoparticles to be delivered to the brain via FUS-induced BBB opening, using gold nanoparticles (AuNPs) with diameters of 3, 15, and 120 nm. They found that medium-sized (15 nm) AuNPs showed the highest delivery efficiency (0.22% ID). The steady and inertial cavitation doses were quantified by labeling lipid microvesicles with the fluorophore 5-dodecylamino fluorescein and applying FUS to the rat head to aid the transport of lipid microvesicles across the BBB; in this way, the cavitation dose threshold was determined for the first time (Sierra et al., 2017). FUS-mediated delivery of NFs, including BDNF (Baseri et al., 2012), neurturin and GDNF (Wang et al., 2012), has been shown to result in high enough levels of these factors to induce neuroprotection and survival. In the treatment of AD, focused ultrasound combined with microbubbles formed by embedding quercetin-modified sulfur nanoparticles (Qc@SNPs) can induce opening of the BBB and allow the delivery of Qc@SNPs. This can effectively protect nerve cells by reducing neuronal apoptosis, the inflammatory response, calcium homeostasis imbalance and oxidative stress (Liu et al., 2020). FUS combined with MBs can be used to deliver erythropoietin (EPO) to the injured area, increases the vascular permeability, promotes the recovery of neurons in the ischemic area, reduces the infarct volume, and exerts a significant neuroprotective effect (Wu et al., 2014). Ultrasound-targeted microbubble destruction (UTMD) can facilitate the delivery of NF genes into brain to protect against the development of neurodegenerative diseases (Lin et al., 2020). UTMD can be used to deliver BDNF to the brain, to promote functional recovery and white matter repair without increasing BBB damage (Rodriguez-Frutos et al., 2016). In addition, FUS can facilitate the delivery of isopropanol in the brains of epileptic rats to exert therapeutic effects against epilepsy (Airan et al., 2017). Magnetic resonance imaging-guided FUS (MRgFUS), enhances endogenous antibody delivery in AD rats to rapidly reduce the number of amyloid beta (Aβ) plaques and increase endogenous immunoglobulin levels and microglial activation (Jordao et al., 2013). MRgFUS is also a safer method used to deliver drugs across the BBB in vivo (Nance et al., 2014). A clinical study demonstrated that MRgFUS can be used for non-invasive, spatially targeted delivery of the monoclonal antibody trastuzumab in the brain via the BBB in patients with Her2-positive brain metastases (Meng et al., 2021). When cerebral ischemia occurs, the BBB will be opened, and macromolecular drugs can enter the ischemic area. However, the time and the therapeutic effect are limited due to the inability to achieve a sufficiently high dose of the drug in the infarcted area (Menzies et al., 1993). FUS combined with microbubbles can open the BBB and improve the permeability of blood vessels, thereby prolongs the time of drug treatment and improves the treatment effect. This technology has good prospects in the treatment of ischemic stroke.
Basic and clinical studies have demonstrated the efficacy of TFUS. It has been found to enhance drug delivery and neuroprotection. However, due to differences between animals and humans, making the effectiveness of this technology needs to be further verified. Moreover, given the diversity of stroke types, TUS may not necessarily have a beneficial effect in every patient.
The application of TFUS is limited by acoustics-related factors. Because the skull has a high attenuation coefficient, it can absorb and reflect a large amount of ultrasonic energy (Quadri et al., 2018). The absorption of ultrasonic energy can interfere with the transmission of ultrasound, preventing it from acting effectively on the target area and reducing the effectiveness of treatment. In addition, the TFUS focus is relatively small and fixed; however, it has already been demonstrated that through phased array technology, TFUS can be used to treat multiple areas in the brain (Khanna et al., 2017).
Ultrasonic energy is a double-edged sword. Application of ultrasound at an inappropriate power and at the wrong time can cause damage to brain tissue. An almost infinite range of parameters and brain regions need to be studied. Future studies are needed to determine the optimal pulse parameters and dose-response effect on region-specific neural activity (Fomenko et al., 2018). In addition, the electrophysiological and functional responses of cortical and subcortical encephalic regions to ultrasound have yet to be explored.
TUS has the potential to become a clinical treatment for stroke. It can promote functional recovery from nerve recovery promotion, inflammatory response reduction, and will not cause serious tissue damage. It can also improve the curative efficacy by thrombolysis and drugs delivery. Because of the advantages of safety, non-invasiveness, deep penetration and tiny focus, it is gradually used in clinic. However, the clinical evidence regarding the efficacy of TUS is still very limited. In addition, findings from animal studies, such as those related to effective drugs and treatment parameters, may not be applicable to human beings. Especially in terms of thrombolysis, the currently approved thrombolytic drug is tPA, and the improper use of parameters will increase the risk of injury. More experiments are needed to provide strong theoretical support for the clinical application of ultrasound stimulation.
LL and LY: conceptualization. LL, LY, and HH: methodology. JG and LY: writing – original draft preparation. LY, JG and WL: writing – review and editing. All authors contributed to the article and approved the submitted version.
This work was supported by the National Natural Science Foundation of China (Nos. 32071316 and 32211530049), the Fundamental Research Funds for the Central Universities (Grant Nos. G2021KY05101, G2021KY05105, G2021KY05107, and G2022WD01006), the Key Research and Development Project of Shaanxi Province (2022SF-117), the Natural Science Foundation of Shaanxi province (2022-JM482), and the Education and Teaching Reform Funds for the Central Universities (No. 22GZ230101).
The authors declare that the research was conducted in the absence of any commercial or financial relationships that could be construed as a potential conflict of interest.
All claims expressed in this article are solely those of the authors and do not necessarily represent those of their affiliated organizations, or those of the publisher, the editors and the reviewers. Any product that may be evaluated in this article, or claim that may be made by its manufacturer, is not guaranteed or endorsed by the publisher.
TUS, transcranial ultrasound stimulation; TMS, transcranial magnetic stimulation; tDCS, transcranial direct current stimulation; tACS, transcranial alternating current stimulation; tPA, tissue plasminogen activator; TFUS, transcranial focused ultrasound; PD, Parkinson’s disease; AD, Alzheimer’s disease; LIFU, low intensity focused ultrasound; LIPUS, low intensity pulsed ultrasound; BDNF, brain derived neurotrophic factor; VEGF, vascular endothelial growth factor; MCAO, middle cerebral artery occlusion; BBB, blood-brain barrier; PTUS, pulsed transcranial ultrasound stimulation.
Airan, R. D., Meyer, R. A., Ellens, N. P. K., Rhodes, K. R., Farahani, K., Pomper, M. G., et al. (2017). Noninvasive Targeted Transcranial Neuromodulation via Focused Ultrasound Gated Drug Release from Nanoemulsions. Nano Lett. 17, 652–659. doi: 10.1021/acs.nanolett.6b03517
Alexandrov, A. V., Molina, C. A., Grotta, J. C., Garami, Z., Ford, S. R., Alvarez-Sabin, J., et al. (2004). Ultrasound-enhanced systemic thrombolysis for acute ischemic stroke. N. Engl. J. Med. 351, 2170–2178. doi: 10.1056/NEJMoa041175
Antczak, J., Rusin, G., and Slowik, A. (2021). Transcranial Magnetic Stimulation as a Diagnostic and Therapeutic Tool in Various Types of Dementia. J. Clin. Med. 10:2875. doi: 10.3390/jcm10132875
Ashokkumar, M. (2011). The characterization of acoustic cavitation bubbles - An overview. Ultrason. Sonochem. 18, 864–872.
Baek, H., Pahk, K. J., Kim, M. J., Youn, I., and Kim, H. (2018). Modulation of Cerebellar Cortical Plasticity Using Low-Intensity Focused Ultrasound for Poststroke Sensorimotor Function Recovery. Neurorehabil Neural Repair 32, 777–787. doi: 10.1177/1545968318790022
Balkaya, M., and Cho, S. (2019). Genetics of stroke recovery: BDNF val66met polymorphism in stroke recovery and its interaction with aging. Neurobiol. Dis. 126, 36–46. doi: 10.1016/j.nbd.2018.08.009
Barnett, S. B., ter Haar, G. R., Ziskin, M. C., Nyborg, W. L., Maeda, K., and Bang, J. (1994). Current status of research on biophysical effects of ultrasound. Ultrasound Med. Biol. 20, 205–218. doi: 10.1016/0301-5629(94)90060-4
Bartkowska, K., Paquin, A., Gauthier, A. S., Kaplan, D. R., and Miller, F. D. (2007). Trk signaling regulates neural precursor cell proliferation and differentiation during cortical development. Development 134, 4369–4380. doi: 10.1242/dev.008227
Baseri, B., Choi, J. J., Deffieux, T., Samiotaki, G., Tung, Y. S., Olumolade, O., et al. (2012). Activation of signaling pathways following localized delivery of systemically administered neurotrophic factors across the blood-brain barrier using focused ultrasound and microbubbles. Phys. Med. Biol. 57, N65–N81. doi: 10.1088/0031-9155/57/7/N65
Birnbaum, Y., Luo, H., Nagai, T., Fishbein, M. C., Peterson, T. M., Li, S. P., et al. (1998). Noninvasive in vivo clot dissolution without a thrombolytic drug - Recanalization of thrombosed iliofemoral arteries by transcutaneous ultrasound combined with intravenous infusion of microbubbles. Circulation 97, 130–134. doi: 10.1161/01.cir.97.2.130
Blackmore, J., Shrivastava, S., Sallet, J., Butler, C. R., and Cleveland, R. O. (2019). Ultrasound Neuromodulation: A Review of Results, Mechanisms and Safety. Ultrasound Med. Biol. 45, 1509–1536.
Bretsztajn, L., and Gedroyc, W. (2018). Brain-focussed ultrasound: what’s the “FUS” all about? A review of current and emerging neurological applications. Br. J. Radiol. 91:20170481. doi: 10.1259/bjr.20170481
Brown, A. T., Flores, R., Hamilton, E., Roberson, P. K., Borrelli, M. J., and Culp, W. C. (2011). Microbubbles improve sonothrombolysis in vitro and decrease hemorrhage in vivo in a rabbit stroke model. Invest. Radiol. 46, 202–207. doi: 10.1097/RLI.0b013e318200757a
Bystritsky, A., Korb, A. S., Douglas, P. K., Cohen, M. S., Melega, W. P., Mulgaonkar, A. P., et al. (2011). A review of low-intensity focused ultrasound pulsation. Brain Stimul. 4, 125–136. doi: 10.1016/j.brs.2011.03.007
Cantone, M., Lanza, G., Fisicaro, F., Pennisi, M., Bella, R., Di Lazzaro, V., et al. (2020). Evaluation and Treatment of Vascular Cognitive Impairment by Transcranial Magnetic Stimulation. Neural. Plast. 2020:8820881. doi: 10.1155/2020/8820881
Cantone, M., Lanza, G., Ranieri, F., Opie, G. M., and Terranova, C. (2021). Editorial: non-invasive Brain Stimulation in the Study and Modulation of Metaplasticity in Neurological Disorders. Front. Neurol. 12:721906. doi: 10.3389/fneur.2021.721906
Cesare, P., Moriondo, A., Vellani, V., and McNaughton, P. A. (1999). Ion channels gated by heat. Proc. Natl. Acad. Sci. U. S. A. 96, 7658–7663.
Chang, J. W., Wu, M. T., Song, W. S., and Yang, F. Y. (2020). Ultrasound Stimulation Suppresses LPS-Induced Proinflammatory Responses by Regulating NF-kappaB and CREB Activation in Microglial Cells. Cereb. Cortex 30, 4597–4606. doi: 10.1093/cercor/bhaa062
Chen, C. M., Wu, C. T., Yang, T. H., Liu, S. H., and Yang, F. Y. (2018). Preventive Effect of Low Intensity Pulsed Ultrasound against Experimental Cerebral Ischemia/Reperfusion Injury via Apoptosis Reduction and Brain-derived Neurotrophic Factor Induction. Sci. Rep. 8:5568. doi: 10.1038/s41598-018-23929-8
Culp, W. C., Flores, R., Brown, A. T., Lowery, J. D., Roberson, P. K., Hennings, L. J., et al. (2011). Successful microbubble sonothrombolysis without tissue-type plasminogen activator in a rabbit model of acute ischemic stroke. Stroke 42, 2280–2285. doi: 10.1161/STROKEAHA.110.607150
Culp, W. C., Porter, T. R., Lowery, J., Xie, F., Roberson, P. K., and Marky, L. (2004). Intracranial clot lysis with intravenous microbubbles and transcranial ultrasound in swine. Stroke 35, 2407–2411. doi: 10.1161/01.STR.0000140890.86779.79
Darrow, D. P. (2019). Focused Ultrasound for Neuromodulation. Neurotherapeutics 16, 88–99. doi: 10.1007/s13311-018-00691-3
di Biase, L., Falato, E., Caminiti, M. L., Pecoraro, P. M., Narducci, F., and Lazzaro, V. Di (2021). Focused Ultrasound (FUS) for Chronic Pain Management: Approved and Potential Applications. Neurol. Res. Int. 2021:8438498. doi: 10.1155/2021/8438498
Di Lazzaro, V., Bella, R., Benussi, A., Bologna, M., Borroni, B., Capone, F., et al. (2021). Diagnostic contribution and therapeutic perspectives of transcranial magnetic stimulation in dementia. Clin. Neurophysiol. 132, 2568–2607. doi: 10.1016/j.clinph.2021.05.035
Donkor, E. S. (2018). Stroke in the 21(st) Century: A Snapshot of the Burden, Epidemiology, and Quality of Life. Stroke Res. Treat. 2018:3238165. doi: 10.1155/2018/3238165
Fomenko, A., Neudorfer, C., Dallapiazza, R. F., Kalia, S. K., and Lozano, A. M. (2018). Low-intensity ultrasound neuromodulation: an overview of mechanisms and emerging human applications. Brain Stimul. 11, 1209–1217. doi: 10.1016/j.brs.2018.08.013
Fu, W. X., Wang, Q., Zhang, Y. S., Li, Y., Xu, T., He, S., et al. (2015). Application of ultrasound technology in the diagnosis and treatment of digestive tract diseases. Eur. Rev. Med. Pharmacol. Sci. 19, 602–606.
Gao, S., Zhang, Y., Wu, J., Shi, W. T., Lof, J., Vignon, F., et al. (2014). Improvements in cerebral blood flow and recanalization rates with transcranial diagnostic ultrasound and intravenous microbubbles after acute cerebral emboli. Invest. Radiol. 49, 593–600. doi: 10.1097/RLI.0000000000000059
Greenberg, D. A., and Jin, K. (2005). From angiogenesis to neuropathology. Nature 438, 954–959. doi: 10.1038/nature04481
Guo, T., Li, H., Lv, Y., Lu, H., Niu, J., Sun, J., et al. (2015). Pulsed Transcranial Ultrasound Stimulation Immediately After The Ischemic Brain Injury is Neuroprotective. IEEE Trans. Biomed. Eng. 62, 2352–2357. doi: 10.1109/TBME.2015.2427339
Hanawa, K., Ito, K., Aizawa, K., Shindo, T., Nishimiya, K., Hasebe, Y., et al. (2014). Low-intensity pulsed ultrasound induces angiogenesis and ameliorates left ventricular dysfunction in a porcine model of chronic myocardial ischemia. PLoS One 9:e104863. doi: 10.1371/journal.pone.0104863
Ichijo, S., Shindo, T., Eguchi, K., Monma, Y., Nakata, T., Morisue, Y., et al. (2021). Low-intensity pulsed ultrasound therapy promotes recovery from stroke by enhancing angio-neurogenesis in mice in vivo. Sci. Rep. 11:4958. doi: 10.1038/s41598-021-84473-6
Ilham, S. J., Kashani, Z., and Kiani, M. (2021). Design and Optimization of Ultrasound Phased Arrays for Large-Scale Ultrasound Neuromodulation. IEEE Trans. Biomed. Circ. Syst. 15, 1454–1466. doi: 10.1109/TBCAS.2021.3133133
Imashiro, C., Azuma, T., Itai, S., Kuribara, T., Totani, K., Onoe, H., et al. (2021). Travelling ultrasound promotes vasculogenesis of three-dimensional-monocultured human umbilical vein endothelial cells. Biotechnol. Bioeng. 118, 3760–3769. doi: 10.1002/bit.27852
Ishibashi, T., Akiyama, M., Onoue, H., Abe, T., and Furuhata, H. (2002). Can transcranial ultrasonication increase recanalization flow with tissue plasminogen activator? Stroke 33, 1399–1404. doi: 10.1161/01.str.0000013789.15436.42
Jickling, G. C., Liu, D., Ander, B. P., Stamova, B., Zhan, X., and Sharp, F. R. (2015). Targeting neutrophils in ischemic stroke: translational insights from experimental studies. J. Cereb. Blood Flow Metab. 35, 888–901. doi: 10.1038/jcbfm.2015.45
Jordao, J. F., Thevenot, E., Markham-Coultes, K., Scarcelli, T., Weng, Y. Q., Xhima, K., et al. (2013). Amyloid-beta plaque reduction, endogenous antibody delivery and glial activation by brain-targeted, transcranial focused ultrasound. Exp. Neurol. 248, 16–29. doi: 10.1016/j.expneurol.2013.05.008
Kamimura, H. A. S., Conti, A., Toschi, N., and Konofagou, E. E. (2020). Ultrasound Neuromodulation: Mechanisms and the Potential of Multimodal Stimulation for Neuronal Function Assessment. Front. Phys. 8:150. doi: 10.3389/fphy.2020.00150
Kettenmann, H., Hanisch, U. K., Noda, M., and Verkhratsky, A. (2011). Physiology of microglia. Physiol. Rev. 91, 461–553. doi: 10.1152/physrev.00011.2010
Khanna, N., Gandhi, D., Steven, A., Frenkel, V., and Melhem, E. R. (2017). Intracranial Applications of MR Imaging-Guided Focused Ultrasound. AJNR Am. J. Neuroradiol. 38, 426–431. doi: 10.3174/ajnr.A4902
Kubanek, J. (2018a). Neuromodulation with transcranial focused ultrasound. Neurosurg. Focus 44:E14. doi: 10.3171/2017.11.FOCUS17621
Li, G. F., Zhao, H. X., Zhou, H., Yan, F., Wang, J. Y., Xu, C. X., et al. (2016). Improved Anatomical Specificity of Non-invasive Neuro-stimulation by High Frequency (5 MHz) Ultrasound. Sci. Rep. 6:24738. doi: 10.1038/srep24738
Li, H. D., Sun, J. F., Zhang, D. Q., Omire-Mayor, D., Lewin, P. A., and Tong, S. B. (2017). Low-intensity (400 mW/cm(2), 500 kHz) pulsed transcranial ultrasound preconditioning may mitigate focal cerebral ischemia in rats. Brain Stimul. 10, 695–702. doi: 10.1016/j.brs.2017.02.008
Li, Y., Teng, X., Yang, C., Wang, Y., Wang, L., Dai, Y., et al. (2021). Ultrasound Controlled Anti-Inflammatory Polarization of Platelet Decorated Microglia for Targeted Ischemic Stroke Therapy. Angew Chem. Int. Ed. Engl. 60, 5083–5090. doi: 10.1002/anie.202010391
Lin, C. Y., Lin, Y. C., Huang, C. Y., Wu, S. R., Chen, C. M., and Liu, H. L. (2020). Ultrasound-responsive neurotrophic factor-loaded microbubble- liposome complex: preclinical investigation for Parkinson’s disease treatment. J. Control. Release 321, 519–528. doi: 10.1016/j.jconrel.2020.02.044
Liu, L., Du, J., Zheng, T., Hu, S., Dong, Y., Du, D., et al. (2019). Protective effect of low-intensity transcranial ultrasound stimulation after differing delay following an acute ischemic stroke. Brain Res. Bull. 146, 22–27. doi: 10.1016/j.brainresbull.2018.12.004
Liu, W. S., Huang, Z. Z., Wang, X. W., and Zhou, J. (2012). Effects of microbubbles on transcranial Doppler ultrasound-assisted intracranial urokinase thrombolysis. Thromb Res. 130, 547–551. doi: 10.1016/j.thromres.2012.06.020
Liu, X., Naomi, S. S. M., Sharon, W. L., and Russell, E. J. (2021). The Applications of Focused Ultrasound (FUS) in Alzheimer’s Disease Treatment: A Systematic Review on Both Animal and Human Studies. Aging Dis. 12, 1977–2002. doi: 10.14336/AD.2021.0510
Liu, Y. A., Gong, Y. C., Xie, W. J., Huang, A. L., Yuan, X. Y., Zhou, H., et al. (2020). Microbubbles in combination with focused ultrasound for the delivery of quercetin-modified sulfur nanoparticles through the blood brain barrier into the brain parenchyma and relief of endoplasmic reticulum stress to treat Alzheimer’s disease. Nanoscale 12, 6498–6511. doi: 10.1039/c9nr09713a
Lu, Y., Wang, J., Huang, R., Chen, G., Zhong, L., Shen, S., et al. (2016). Microbubble-Mediated Sonothrombolysis Improves Outcome After Thrombotic Microembolism-Induced Acute Ischemic Stroke. Stroke 47, 1344–1353. doi: 10.1161/STROKEAHA.115.012056
Ma, H., Jiang, Z., Xu, J., Liu, J., and Guo, Z. N. (2021). Targeted nano-delivery strategies for facilitating thrombolysis treatment in ischemic stroke. Drug Deliv. 28, 357–371. doi: 10.1080/10717544.2021.1879315
Meng, Y., Reilly, R. M., Pezo, R. C., Trudeau, M., Sahgal, A., Singnurkar, A., et al. (2021). MR-guided focused ultrasound enhances delivery of trastuzumab to Her2-positive brain metastases. Sci. Transl. Med. 13:eabj4011. doi: 10.1126/scitranslmed.abj4011
Menzies, S. A., Betz, A. L., and Hoff, J. T. (1993). Contributions of Ions and Albumin To the Formation and Resolution of Ischemic Brain Edema. J. Neurosurg. 78, 257–266. doi: 10.3171/jns.1993.78.2.0257
Miller, D. B., and O’Callaghan, J. P. (2017). New horizons for focused ultrasound (FUS) - therapeutic applications in neurodegenerative diseases. Metabolism 69S, S3–S7. doi: 10.1016/j.metabol.2017.01.012
Min, B. K., Bystritsky, A., Jung, K. I., Fischer, K., Zhang, Y., Maeng, L. S., et al. (2011). Focused ultrasound-mediated suppression of chemically-induced acute epileptic EEG activity. BMC Neurosci. 12:23. doi: 10.1186/1471-2202-12-23
Monteith, S., Sheehan, J., Medel, R., Wintermark, M., Eames, M., Snell, J., et al. (2013). Potential intracranial applications of magnetic resonance-guided focused ultrasound surgery. J. Neurosurg. 118, 215–221. doi: 10.3171/2012.10.JNS12449
Nainwal, N. (2017). Recent Advances in Transcranial Focused Ultrasound (FUS) Triggered Brain Delivery. Curr. Drug Targets 18, 1225–1232. doi: 10.2174/1389450117666161222160025
Nance, E., Timbie, K., Miller, G. W., Song, J., Louttit, C., Klibanov, A. L., et al. (2014). Non-invasive delivery of stealth, brain-penetrating nanoparticles across the blood - brain barrier using MRI-guided focused ultrasound. J. Control. Release 189, 123–132. doi: 10.1016/j.jconrel.2014.06.031
Ohta, S., Kikuchi, E., Ishijima, A., Azuma, T., Sakuma, I., and Ito, T. (2020). Investigating the optimum size of nanoparticles for their delivery into the brain assisted by focused ultrasound-induced blood-brain barrier opening. Sci. Rep. 10:18220. doi: 10.1038/s41598-020-75253-9
Otxoa-de-Amezaga, A., Miro-Mur, F., Pedragosa, J., Gallizioli, M., Justicia, C., Gaja-Capdevila, N., et al. (2019). Microglial cell loss after ischemic stroke favors brain neutrophil accumulation. Acta Neuropathol. 137, 321–341. doi: 10.1007/s00401-018-1954-4
Palmer, T. D., Willhoite, A. R., and Gage, F. H. (2000). Vascular niche for adult hippocampal neurogenesis. J. Comp. Neurol. 425, 479–494. doi: 10.1002/1096-9861(20001002)425:4<479::aid-cne2<3.0.co;2-3
Park, T. Y., Kim, H. J., Park, S. H., Chang, W. S., Kim, H., and Yoon, K. (2022). Differential evolution method to find optimal location of a single-element transducer for transcranial focused ultrasound therapy. Comp. Methods Prog. Biomed. 219:106777. doi: 10.1016/j.cmpb.2022.106777
Paul, S., and Candelario-Jalil, E. (2021). Emerging neuroprotective strategies for the treatment of ischemic stroke: an overview of clinical and preclinical studies. Exp. Neurol. 335:113518. doi: 10.1016/j.expneurol.2020.113518
Plaksin, M., Shoham, S., and Kimmel, E. (2014). Intramembrane cavitation as a predictive bio-piezoelectric mechanism for ultrasonic brain stimulation. J. Mol. Neurosci. 53, S103–S103.
Pol, F., Salehinejad, M. A., Baharlouei, H., and Nitsche, M. A. (2021). The effects of transcranial direct current stimulation on gait in patients with Parkinson’s disease: a systematic review. Trans. Neurodegen. 10:22.
Powers, W. J., Rabinstein, A. A., Ackerson, T., Adeoye, O. M., Bambakidis, N. C., Becker, K., et al. (2019). Guidelines for the Early Management of Patients With Acute Ischemic Stroke: 2019 Update to the 2018 Guidelines for the Early Management of Acute Ischemic Stroke: A Guideline for Healthcare Professionals From the American Heart Association/American Stroke Association. Stroke 50, e344–e418. doi: 10.1161/STR.0000000000000211
Quadri, S. A., Waqas, M., Khan, I., Khan, M. A., Suriya, S. S., Farooqui, M., et al. (2018). High-intensity focused ultrasound: past, present, and future in neurosurgery. Neurosurg. Focus 44:E16. doi: 10.3171/2017.11.FOCUS17610
Ren, S. T., Long, L. H., Wang, M., Li, Y. P., Qin, H., Zhang, H., et al. (2012). Thrombolytic effects of a combined therapy with targeted microbubbles and ultrasound in a 6 h cerebral thrombosis rabbit model. J. Thromb Thromb. 33, 74–81. doi: 10.1007/s11239-011-0644-z
Rodriguez-Frutos, B., Otero-Ortega, L., Ramos-Cejudo, J., Martinez-Sanchez, P., Barahona-Sanz, I., Navarro-Hernanz, T., et al. (2016). Enhanced brain-derived neurotrophic factor delivery by ultrasound and microbubbles promotes white matter repair after stroke. Biomaterials 100, 41–52. doi: 10.1016/j.biomaterials.2016.05.028
Rosenthal, I., Sostaric, J. Z., and Riesz, P. (2004). Sonodynamic therapy–a review of the synergistic effects of drugs and ultrasound. Ultrason. Sonochem. 11, 349–363. doi: 10.1016/j.ultsonch.2004.03.004
Saguchi, T., Onoue, H., Urashima, M., Ishibashi, T., Abe, T., and Furuhata, H. (2008). Effective and safe conditions of low-frequency transcranial ultrasonic thrombolysis for acute ischemic stroke: neurologic and histologic evaluation in a rat middle cerebral artery stroke model. Stroke 39, 1007–1011. doi: 10.1161/STROKEAHA.107.496117
Sanwal, R., Joshi, K., Ditmans, M., Tsai, S. S. H., and Lee, W. L. (2021). Ultrasound and Microbubbles for Targeted Drug Delivery to the Lung Endothelium in ARDS: Cellular Mechanisms and Therapeutic Opportunities. Biomedicines 9:803. doi: 10.3390/biomedicines9070803
Schleicher, N., Tomkins, A. J., Kampschulte, M., Hyvelin, J. M., Botteron, C., Juenemann, M., et al. (2016). Sonothrombolysis with BR38 Microbubbles Improves Microvascular Patency in a Rat Model of Stroke. PLoS One 11:e0152898. doi: 10.1371/journal.pone.0152898
Sharma, V. K., Paliwal, P. R., Teoh, H. L., Venketasubramanian, N., and Chan, B. P. (2011). Role of diagnostic ultrasound in patient selection for stroke intervention. Recent Pat. CNS Drug Discov. 6, 181–195. doi: 10.2174/157488911796957995
Shin Low, S., Nong, L. C., Yew, M., Siong Chai, W., Low, L. E., Manickam, S., et al. (2021). Recent ultrasound advancements for the manipulation of nanobiomaterials and nanoformulations for drug delivery. Ultrason. Sonochem. 80:105805. doi: 10.1016/j.ultsonch.2021.105805
Shin, J., Kong, C., Lee, J., Choi, B. Y., Sim, J., Koh, C. S., et al. (2019). Focused ultrasound-induced blood-brain barrier opening improves adult hippocampal neurogenesis and cognitive function in a cholinergic degeneration dementia rat model. Alzheimers Res. Ther. 11:110. doi: 10.1186/s13195-019-0569-x
Sierra, C., Acosta, C., Chen, C., Wu, S. Y., Karakatsani, M. E., Bernal, M., et al. (2017). Lipid microbubbles as a vehicle for targeted drug delivery using focused ultrasound-induced blood-brain barrier opening. J. Cereb. Blood Flow Metabol. 37, 1236–1250. doi: 10.1177/0271678X16652630
Sousa, R. M., Ferri, C. P., Acosta, D., Albanese, E., Guerra, M., Huang, Y., et al. (2009). Contribution of chronic diseases to disability in elderly people in countries with low and middle incomes: a 10/66 Dementia Research Group population-based survey. Lancet 374, 1821–1830. doi: 10.1016/S0140-6736(09)61829-8
Su, W. S., Wu, C. H., Chen, S. F., and Yang, F. Y. (2017). Transcranial ultrasound stimulation promotes brain-derived neurotrophic factor and reduces apoptosis in a mouse model of traumatic brain injury. Brain Stimul. 10, 1032–1041. doi: 10.1016/j.brs.2017.09.003
Taguchi, A., Sakai, C., Soma, T., Kasahara, Y., Stern, D. M., Kajimoto, K., et al. (2015). Intravenous Autologous Bone Marrow Mononuclear Cell Transplantation for Stroke: Phase1/2a Clinical Trial in a Homogeneous Group of Stroke Patients. Stem Cells Dev. 24, 2207–2218. doi: 10.1089/scd.2015.0160
Tata, D. B., and Dunn, F. (1992). Interaction of Ultrasound and Model Membrane Systems - Analyses and Predictions. J. Phys. Chem. 96, 3548–3555.
Tharkar, P., Varanasi, P., Wong, W. S. F., Jin, C. T., and Chrzanowski, W. (2019). Nano-Enhanced Drug Delivery and Therapeutic Ultrasound for Cancer Treatment and Beyond. Front. Bioeng. Biotechnol. 7:324. doi: 10.3389/fbioe.2019.00324
Thomassen, L., Fromm, A., Aarli, S., and Logallo, N. (2021). Transcranial ultrasound monitoring in acute stroke. Tidsskr Nor. Laegeforen. 141. doi: 10.4045/tidsskr.21.0180 [Epub ahead of print].
Trockel, U., Hennerici, M., Aulich, A., and Sandmann, W. (1984). The Superiority of Combined Continuous Wave Doppler Examination over Periorbital Doppler for the Detection of Extracranial Carotid Disease. J. Neurol. Neurosurg. Psychiat. 47, 43–50. doi: 10.1136/jnnp.47.1.43
Tufail, Y., Matyushov, A., Baldwin, N., Tauchmann, M. L., Georges, J., Yoshihiro, A., et al. (2010). Transcranial pulsed ultrasound stimulates intact brain circuits. Neuron 66, 681–694. doi: 10.1016/j.neuron.2010.05.008
Tyler, W. J., Lani, S. W., and Hwang, G. M. (2018). Ultrasonic modulation of neural circuit activity. Curr. Opin. Neurobiol. 50, 222–231.
Wang, F. Q., Wang, Q., Wang, L., Ren, J., Song, X. Z., Tian, Y. T., et al. (2022b). Low-Intensity Focused Ultrasound Stimulation Ameliorates Working Memory Dysfunctions in Vascular Dementia Rats via Improving Neuronal Environment. Front. Aging Neurosci. 14:814560. doi: 10.3389/fnagi.2022.814560
Wang, F., Shi, Y., Lu, L., Liu, L., Cai, Y. L., Zheng, H. R., et al. (2012). Targeted Delivery of GDNF through the Blood-Brain Barrier by MRI-Guided Focused Ultrasound. PLoS One 7:e52925. doi: 10.1371/journal.pone.0052925
Wang, F., Wang, Q., Wang, L., Ren, J., Song, X., Tian, Y., et al. (2022a). Low-Intensity Focused Ultrasound Stimulation Ameliorates Working Memory Dysfunctions in Vascular Dementia Rats via Improving Neuronal Environment. Front. Aging Neurosci. 14:814560.
Wang, J., Li, G., Deng, L., Mamtilahun, M., Jiang, L., Qiu, W., et al. (2021). Transcranial Focused Ultrasound Stimulation Improves Neurorehabilitation after Middle Cerebral Artery Occlusion in Mice. Aging Dis. 12, 50–60. doi: 10.14336/AD.2020.0623
Wood, A. K., and Sehgal, C. M. (2015). A review of low-intensity ultrasound for cancer therapy. Ultrasound Med. Biol. 41, 905–928. doi: 10.1016/j.ultrasmedbio.2014.11.019
Woods, A. J., Antal, A., Bikson, M., Boggio, P. S., Brunoni, A. R., Celnik, P., et al. (2016). A technical guide to tDCS, and related non-invasive brain stimulation tools. Clin. Neurophys. 127, 1031–1048. doi: 10.1016/j.clinph.2015.11.012
Wright, C., Hynynen, K., and Goertz, D. (2012). In vitro and in vivo high-intensity focused ultrasound thrombolysis. Invest. Radiol. 47, 217–225. doi: 10.1097/RLI.0b013e31823cc75c
Wu, C. T., Yang, T. H., Chen, M. C., Chung, Y. P., Guan, S. S., Long, L. H., et al. (2019). Low Intensity Pulsed Ultrasound Prevents Recurrent Ischemic Stroke in a Cerebral Ischemia/Reperfusion Injury Mouse Model via Brain-derived Neurotrophic Factor Induction. Int. J. Mol. Sci. 20:5169. doi: 10.3390/ijms20205169
Wu, S. K., Yang, M. T., Kang, K. H., Liou, H. C., Lu, D. H., Fu, W. M., et al. (2014). Targeted delivery of erythropoietin by transcranial focused ultrasound for neuroprotection against ischemia/reperfusion-induced neuronal injury: a long-term and short-term study. PLoS One 9:e90107. doi: 10.1371/journal.pone.0090107
Yang, F. Y., Lu, W. W., Lin, W. T., Chang, C. W., and Huang, S. L. (2015). Enhancement of Neurotrophic Factors in Astrocyte for Neuroprotective Effects in Brain Disorders Using Low-intensity Pulsed Ultrasound Stimulation. Brain Stimul. 8, 465–473. doi: 10.1016/j.brs.2014.11.017
Yoo, S. S., Bystritsky, A., Lee, J. H., Zhang, Y., Fischer, K., Min, B. K., et al. (2011). Focused ultrasound modulates region-specific brain activity. Neuroimage 56, 1267–1275. doi: 10.1016/j.neuroimage.2011.02.058
Zhang, M., Rodrigues, A., Zhou, Q., and Li, G. (2022). Focused ultrasound: growth potential and future directions in neurosurgery. J. Neurooncol. 156, 23–32. doi: 10.1007/s11060-021-03820-9
Zhang, Y., Yu, J., Kahkoska, A. R., Wang, J., Buse, J. B., and Gu, Z. (2019). Advances in transdermal insulin delivery. Adv. Drug Deliv. Rev. 139, 51–70. doi: 10.1016/j.addr.2018.12.006
Zhao, S. C., Ma, L. S., Chu, Z. H., Xu, H., Wu, W. Q., and Liu, F. (2017). Regulation of microglial activation in stroke. Acta Pharmacol. Sin. 38, 445–458. doi: 10.1038/aps.2016.162
Keywords: transcranial ultrasound, stroke, thrombosis, intervention, drug delivery
Citation: Guo J, Lo WLA, Hu H, Yan L and Li L (2022) Transcranial ultrasound stimulation applied in ischemic stroke rehabilitation: A review. Front. Neurosci. 16:964060. doi: 10.3389/fnins.2022.964060
Received: 08 June 2022; Accepted: 04 July 2022;
Published: 22 July 2022.
Edited by:
Zhihai Qiu, Guangdong Institute of Intelligence Science and Technology, ChinaReviewed by:
Defei Liao, Sonix Inc., United StatesCopyright © 2022 Guo, Lo, Hu, Yan and Li. This is an open-access article distributed under the terms of the Creative Commons Attribution License (CC BY). The use, distribution or reproduction in other forums is permitted, provided the original author(s) and the copyright owner(s) are credited and that the original publication in this journal is cited, in accordance with accepted academic practice. No use, distribution or reproduction is permitted which does not comply with these terms.
*Correspondence: Li Yan, eWFubGkxMTMwQG53cHUuZWR1LmNu; Le Li, bGlsZTVAbndwdS5lZHUuY24=
Disclaimer: All claims expressed in this article are solely those of the authors and do not necessarily represent those of their affiliated organizations, or those of the publisher, the editors and the reviewers. Any product that may be evaluated in this article or claim that may be made by its manufacturer is not guaranteed or endorsed by the publisher.
Research integrity at Frontiers
Learn more about the work of our research integrity team to safeguard the quality of each article we publish.