- 1Department of Anatomy and Medical Imaging, Faculty of Medical and Health Sciences, Centre for Brain Research, University of Auckland, Auckland, New Zealand
- 2Pharmacology and Therapeutics, Galway Neuroscience Centre, School of Medicine, Ollscoil na Gaillimhe – University of Galway, Galway, Ireland
Glutamate is the main excitatory neurotransmitter in the human central nervous system, responsible for a wide variety of normal physiological processes. Glutamatergic metabolism and its sequestration are tightly regulated in the normal human brain, and it has been demonstrated that dysregulation of the glutamatergic system can have wide-ranging effects both in acute brain injury and neurodegenerative diseases. The excitatory amino acid transporter 2 (EAAT2) is the dominant glutamatergic transporter in the human brain, responsible for efficient removal of glutamate from the synaptic cleft for recycling within glial cells. As such, it has a key role in maintaining excitatory-inhibitory homeostasis. Animal studies have demonstrated dysregulation or alterations of EAAT2 expression can have implications in neurodegenerative disorders. Despite extensive research into glutamatergic alterations in AD mouse models, there is a lack of studies examining the expression of EAAT2 within the AD human brain. In this systematic review, 29 articles were identified that either analyzed EAAT2 expression in the AD human brain or used a human-derived cell culture. Studies were inconclusive as to whether EAAT2 was upregulated or downregulated in AD. However, changes in localization and correlation between EAAT2 expression and symptomatology was noted. These findings implicate EAAT2 alterations as a key process in AD progression and highlight the need for further research into the characterization of EAAT2 processes in normal physiology and disease in human tissue and to identify compounds that can act as EAAT2 neuromodulators.
Introduction
Alzheimer's disease (AD) is a progressive neurodegenerative condition that is the most common form of dementia, causing impairments in memory and cognitive function (Revi, 2020). Due to an aging population worldwide, the incidence of AD is predicted to increase rapidly in the coming years (Brookmeyer et al., 2007). As such, there is now an increasing need to develop a disease-modifying treatment or preventative approach for AD due to the social and economic burden associated with rising incidence. Many promising scientific interventions based on animal models have failed to translate into effective therapies in humans, raising doubts about the current leading theories behind AD's etiology (Tolar et al., 2019). Analyzing postmortem human brain tissue to determine cellular alterations to disease-relevant proteins is, therefore, a very valuable method to identify potential human-specific therapeutic targets for AD.
The main pathological hallmarks of AD are the aggregation of extracellular amyloid-beta (Aβ) plaques and intracellular tangles of the microtubule-associated protein tau (Bloom, 2014). These pathologies severely affect the hippocampal regions and the entorhinal cortex, causing neuronal cell loss and memory impairment (Braak and Braak, 1991). Neuronal death eventually spreads throughout the temporal cortex and to other brain regions in late disease (Braak and Braak, 1991). Other notable pathological features of AD include vascular deficits, mitochondrial damage, inflammation, and damage to neurotransmitter systems, which are contributing factors to extensive neuronal death (Šerý et al., 2013; Wang and Reddy, 2017; Hampel et al., 2018).
Glutamate is the primary excitatory neurotransmitter of the central nervous system (CNS), involved in a variety of vital functions, including neuronal communication and regulation of neuronal activity (Danbolt, 2001). Glutamate concentrations in the extracellular space are usually tightly regulated, and dysregulation can lead to overexcitation of postsynaptic neurons, potentially causing glutamate excitotoxicity. A growing body of evidence suggests glutamate dysfunction as a core component in the pathogenesis of neurodegenerative diseases, including AD (Hynd et al., 2004). Glutamate reuptake from the extracellular space is regulated by a class of transporters known as the excitatory amino acid transporters (EAATs).
There are five isoforms of the EAATs, with their expression being cell type and brain region specific (Malik and Willnow, 2019). The isoforms EAAT1 and EAAT2 are primarily expressed on astrocytes, with high expression in the cerebellum and throughout the entire brain, respectively (Kim et al., 2011; Pajarillo et al., 2019). EAAT3 is expressed throughout the brain, while EAAT4 expression is predominantly found in the cerebellum (Malik and Willnow, 2019). EAAT5 expression is mainly restricted to photoreceptors and bipolar cells in the retina (Arriza et al., 1997). EAAT2, encoded by the SLC1A2 gene and also referred to by its rodent nomenclature, glutamate transporter 1 (GLT-1), is responsible for 90% of glutamate uptake in the mature brain (Kim et al., 2011; Pajarillo et al., 2019). Its expression is hence critical for controlling extracellular glutamate concentrations.
Several past animal studies have suggested that EAAT2 alteration occurs in AD. EAAT2 translocation has also been demonstrated in AD models and may play a role in EAAT2 dysfunction. EAAT2 haploinsufficiency has been shown to hasten cognitive decline in an AD mouse model, indicating that EAAT2 expression may play a critical factor in dictating AD progression (Mookherjee et al., 2011). Cross-linking of EAAT2 both in vitro and ex vivo in mouse hippocampal brain was found to alter astrocytic currents and neuronal EPSCs, although transporter function was preserved (Murphy-Royal et al., 2015). These findings support the notion that astrocytes are actively involved in shaping excitatory neurotransmission and highlight the importance of normal surface diffusion of glutamate transporters at the synapse in maintaining homeostasis and cellular signaling processes. In mouse hippocampal slices, Aβ1−42 induces rapid EAAT2 mislocalization and internalization in astrocytes, which leads to reduced cell surface expression and a marked reduction in glutamate reuptake from the extracellular space (Scimemi et al., 2013). Despite significant evidence pointing to glutamatergic dysfunction in AD, the majority of current literature has used mouse or other animal tissue studies to assess EAAT2 alterations and test therapeutic strategies for AD. However, only a limited amount of literature has used human tissue or cells to understand what may be happening to EAAT2 in AD.
Overall, this review aims to discuss the current evidence of EAAT2 expression and functional alteration in AD, a critical knowledge gap given that AD is ultimately a human disease. In addition, whether the existing literature warrants further research to investigate this glutamate transporter as a therapeutic target for the treatment or intervention of AD will be discussed.
Methodology
We performed a comprehensive literature search of PubMed, Web of Science and Scopus databases from 1991 through to 2022. Specific search terms used were Title, Abstract, Keywords/MeSH terms (“Excitatory Amino Acid Transporter 2” [Mesh] OR “SLC1A2 protein, human” [Supplementary Concept]) OR “Glt-1” OR “Glutamate transporter 1” OR “solute carrier family 1 member 2”) AND Title, Abstract, Keywords/MeSH terms: (Alzheimer Disease). Five hundred and six relevant articles were identified and collated in EndNote X8 (Clarivate Analytics, Philadelphia, PA, USA). Duplicates were removed using the Rayyan systematic review software (Rayyan QCRI, RRID:SCR_017584), and then sorted for inclusion or exclusion. Each abstract was screened independently by the first two authors to identify the articles that met our inclusion criteria of human-based studies with an EAAT2 focus. All authors discussed any conflicts to determine whether to include or exclude articles from this review. The inclusion criteria for this review were any EAAT2 study relevant to AD, including human-derived cultured cells, even those where EAAT2 may not have necessarily been the main focus of the study. Hence, animal-based studies or studies with no relevance to EAAT2 or AD were excluded. Twenty-nine articles were selected based on this criterion, and all authors agreed on this selection. The article selection process is shown in Figure 1, and the included studies are shown in Table 1 (postmortem studies) and Table 2 (cell cultures and other studies).
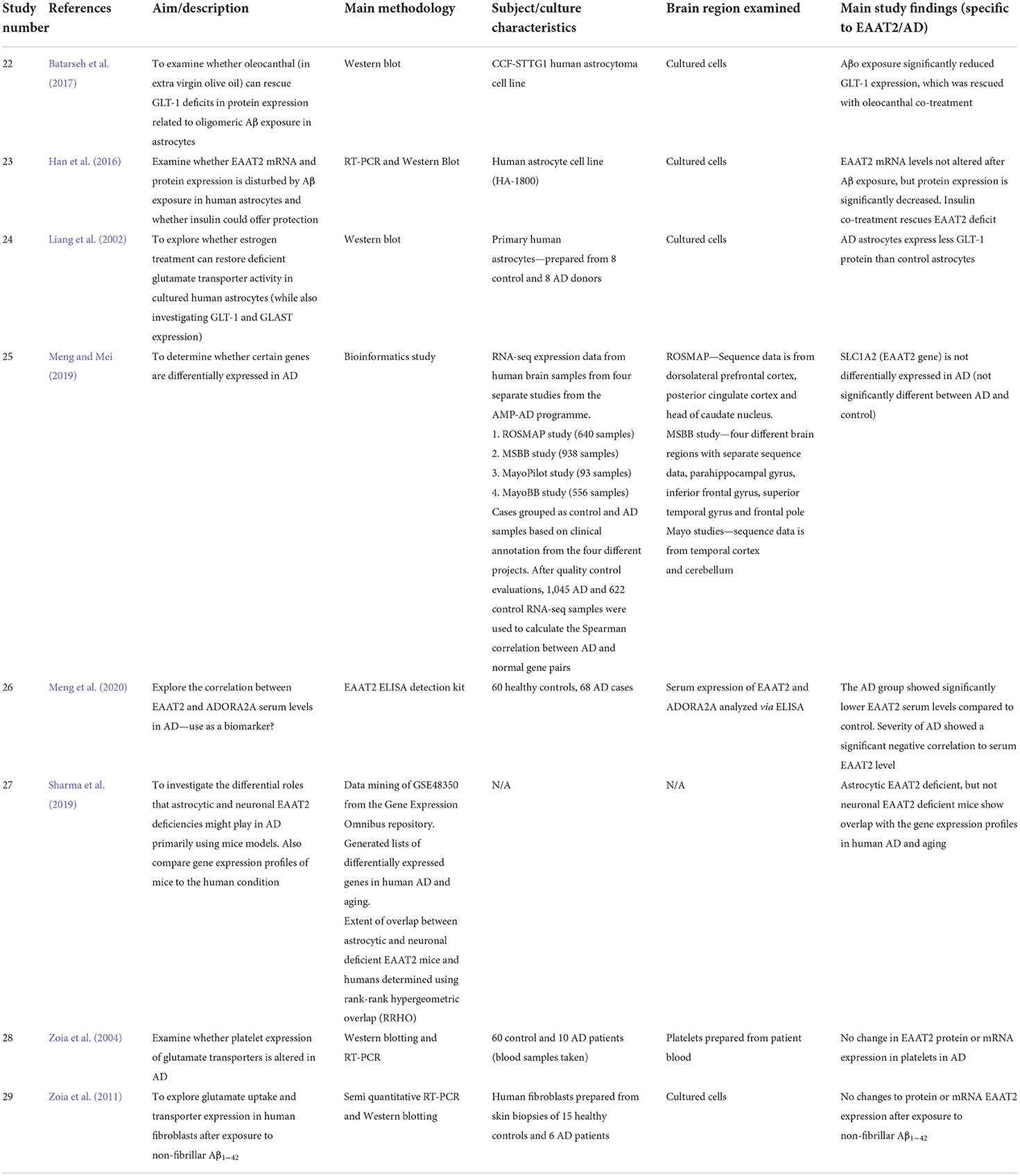
Table 2. Summary of cell culture, gene expression and other human EAAT2 studies included in this systematic review.
Summary of findings
Only a small group of studies in current literature have investigated the expression of EAAT2 in human postmortem tissue, and this is reflected by the relatively small number of papers that met the inclusion criteria for this review. These articles have yielded contradictory findings regarding EAAT2 expression in AD, even for some studies investigating the same brain regions. In addition, a handful of studies have utilized human-derived cultured cells to investigate EAAT2 dysfunction in AD. Overall, despite a small sample size, it would appear these results broadly support findings from animal work and suggest a role for Aβ induced dysfunction of EAAT2 in AD.
Postmortem human tissue
Temporal cortex and hippocampus
Some research has concluded EAAT2 protein expression is reduced in AD postmortem hippocampal (Abdul et al., 2009) and temporal gyrus tissue (Hoshi et al., 2018). However, a recently published study by our laboratory group revealed no quantitative EAAT2 alterations in the hippocampus, subiculum, entorhinal cortex, or superior temporal gyrus (Yeung et al., 2021). Despite this, changes to the expression pattern of EAAT2 were evident, with higher immunoreactivity noticeable in the neuropil (Yeung et al., 2021). The notion that EAAT2 expression level may not be altered in AD is further supported by earlier evidence from (Beckstrøm et al. 1999), who found varying EAAT2 levels in the inferior temporal gyrus for both control and AD cases, but no clear association with AD. However, Jacob et al. (2007) and Scott et al. (2011) provided evidence for a decrease in EAAT2 mRNA expression in the AD temporal cortex, complicating current literature. Further results include those from Simpson et al. (2010), who reported variability in EAAT2 staining patterns between AD cases and a significant inverse relationship between GFAP and EAAT2 expression. Rothstein et al. (1992) reported no change to high-affinity glutamate transport. In addition, Woltjer et al. (2010) found an increase in detergent-insoluble EAAT2 in both the AD hippocampus and frontal cortex.
Interestingly, one study reported that cognitively intact patients with AD-related pathology in the entorhinal cortex showed greater GLT-1 immunoreactivity and greater mRNA expression than those with symptoms of dementia (Kobayashi et al., 2018). This is a fascinating result as no other study in postmortem tissue has revealed that different levels of EAAT2 expression might be correlated to clinical symptoms in the presence of AD pathology.
Frontal cortex
Similar to the temporal cortex, there is conflicting information in the frontal cortex regarding potential changes to the expression of EAAT2 in AD. Several studies have suggested no changes to EAAT2 expression in AD and dementia (Kirvell et al., 2010; Garcia-Esparcia et al., 2018; Poirel et al., 2018). Others have reported a decreased mRNA (Jacob et al., 2007; Tian et al., 2010; Scott et al., 2011) and protein (Li et al., 1997) EAAT2 expression. Tian et al. (2010) also found evidence for a decreased association of EAAT2 with lipid rafts in AD cases, potentially indicating a reduction in membrane expression.
Other anatomical areas
Compared to the temporal and frontal cortices, the expression of EAAT2 in other brain areas has not been as extensively studied. Jacob et al. (2007) found no evidence of EAAT2 alteration in AD postmortem cerebellar tissue. In the parietal cortex, there is evidence that a lipid peroxidation product 4-hydroxy-2-nonenal (HNE) is co-expressed with EAAT2, potentially indicating oxidative damage and loss of transporter function (Lauderback et al., 2001). However, the significance of this finding and whether it is true for other brain regions needs further investigation.
Alternatively-spliced variants and neuronal expression of EAAT2 in Alzheimer's disease
Several studies have researched whether alternatively spliced variants of the EAAT2 protein have altered expression and subsequently play a role in the pathophysiology of AD. Scott et al. (2011) found that both the exon seven and exon nine skipping variants have an increased mRNA copy number with increasing pathological severity in AD cases. Neuronal expression of the exon nine skipping EAAT2 variant has been reported in AD postmortem tissue via immunohistochemistry (Pow and Cook, 2009). However, Flowers et al. (2001) found no change in the expression of intron seven retaining and exon nine skipping transcripts in AD, although this was a small sample of AD cases. Thai (2002) also provided evidence for EAAT2 accumulation in neurons in AD but not in control postmortem brains, but it is uncertain if this is the full length or a splice variant of EAAT2.
Human cell culture studies
Several studies utilizing human-derived cultured cells have also been used to investigate potential alterations to EAAT2 expression in AD. Batarseh et al. (2017) found reduced GLT-1 protein expression in a human astrocyte cell line after Aβ exposure. Another study confirmed this result but revealed that while EAAT2 protein levels are decreased after Aβ exposure, there was no change to EAAT2 mRNA transcript levels (Han et al., 2016). This discrepancy between mRNA and protein EAAT2 levels has also been documented in AD postmortem tissue (Li et al., 1997; Kobayashi et al., 2018). Thus, it is postulated that modifications to EAAT2 protein expression in AD result from posttranslational disturbances.
Zoia et al. (2011) found no change to both EAAT2 mRNA and protein in fibroblasts derived from AD patients after Aβ exposure, but this is perhaps unrelated to what is happening to astrocytic expression of EAAT2 in AD. Liang et al. (2002) also found a decrease in GLT-1 protein expression in astrocytes cultured from AD patients. Therefore, these studies suggest that exposure to Aβ may induce a loss of the EAAT2 protein in astrocytes, while mRNA levels are not affected. However, the functional consequences for glutamate uptake are still unknown and the exact reason behind the mRNA-protein discrepancy needs more clarification.
Other relevant studies
One study found that serum levels of EAAT2 were lower in AD patients than in control, and this decrease was inversely correlated to the clinical symptoms of AD (Meng et al., 2020). This finding indicates that ELISA analysis of EAAT2 levels in patient serum may be a potential diagnostic tool for AD, although more work is needed to establish whether this change is specific to AD alone.
Only one paper from the reviewed studies used a bioinformatic approach to investigate EAAT2 gene expression in AD. Meng and Mei (2019) found that by analyzing RNA-seq datasets from four different projects, SLC1A2 was not a differentially expressed gene in AD. Multiple brain regions were represented in the mRNAseq data including the dorsolateral prefrontal cortex, parahippocampal gyrus, inferior frontal gyrus, temporal cortex, and cerebellum (see Table 2, study 25 for full details).
Discussion
There are several possible explanations for the differing results regarding the expression of both EAAT2 mRNA and protein in AD postmortem tissue from the studies included in this review. These results could simply indicate that there is high patient variability of EAAT2 mRNA or protein expression in AD. Additionally, low sample sizes often seen in human studies, mainly due to tissue availability, can produce potentially variable and confounding results. For example, some studies included in this review only used three AD and three control cases (Pow and Cook, 2009). This sample size does not give confidence that these results are replicable across the population. Other factors to consider when looking at the results of postmortem studies are the heterogeneity of AD, stage of disease, age and sex differences, postmortem delay, and medication effects. At this stage, it is unclear how these factors may influence the EAAT2 mRNA and protein expression as well as preservation in the postmortem human brain, an important fact to consider when interpreting variable results between studies. A final point is that different EAAT2/GLT-1 antibodies between studies, likely targeting different regions of the protein, may also be playing a role in conflicting results.
EAAT2 protein expression
Based on early previous evidence from radiolabeling, an ~30% reduction in [3H] aspartate binding was revealed in the postmortem AD brain, signaling a significant dysfunction in glutamate uptake (Masliah et al., 1996). This is likely to play a role in AD progression, with implications such as glutamate excitotoxicity, a well-documented concept in the literature (Conway, 2020; Manisha et al., 2020). However, how this might relate to EAAT2 expression in the human AD brain is still unclear. Animal studies have suggested that Aβ may be responsible for decreases in EAAT2 expression (Takahashi et al., 2015; Huang et al., 2018). In addition, amyloid-beta administration has been shown to result in the mislocalization of EAAT2 (Manisha et al., 2020). However, it is uncertain if this is the reason behind EAAT2 alterations (both expressional and qualitative) previously documented in AD postmortem tissue (Li et al., 1997; Abdul et al., 2009; Hoshi et al., 2018; Yeung et al., 2021). After Aβ treatment, HNE and GLT-1 conjugation and impairments in glutamate uptake were reported in rat cortical synaptosomes (Keller et al., 1997). This result potentially indicates that the same changes seen with HNE and GLT-1 co-precipitation in the human brain by Lauderback et al. (2001) are related to Aβ exposure. More work is needed to establish the exact cellular mechanisms behind Aβ induced dysfunction of EAAT2. Interestingly, certain studies within the same brain region have shown contradictory results regarding EAAT2 protein expression (Abdul et al., 2009; Yeung et al., 2021). A possible explanation could be the methodology used. Abdul et al. (2009) reported a significant decrease in EAAT2 protein expression in the AD hippocampus via analysis of the membrane fraction in a Western blot. This result possibly indicates that EAAT2 expression on astrocytic membranes is reduced in AD, which seems to be in agreement with Tian et al.'s (2010) finding of a significantly decreased association between lipid rafts and EAAT2 in AD. However, our previous study reported no significant quantitative differences in EAAT2 staining in hippocampal regions. Despite this, we did note higher EAAT2 staining in the neuropil that appeared to show less co-localization with GFAP stained astrocytic main branches, also perhaps indicative of EAAT2 astrocytic loss (Yeung et al., 2021). Interestingly, this EAAT2 expression pattern in AD cases (higher staining in the neuropil) appears similar to immunohistochemical images from the hippocampus published by Li et al. (1997). However, no quantitative data was provided in this study for hippocampal areas, raising validity concerns about this comparison. Further analysis is needed to determine if this expression pattern seen in the AD hippocampus represents a loss of EAAT2 on astrocytic membranes or whether this is simply EAAT2 staining on fine astrocytic processes that were not stained with GFAP. Animal work also supports that the underlying pathophysiology is related more to a displacement of transporters than reduced transporter expression, as total EAAT2 expression from the same whole-cell protein lysates was not changed by Aβ1−42 in mouse hippocampal slices (Scimemi et al., 2013). More research is needed to validate this theory of EAAT2 astrocytic displacement, investigating if EAAT2 cell surface trafficking pathways are altered in AD, as this can have functional implications on glutamate uptake and thus play a key role in neurodegeneration.
EAAT2 mRNA expression
Both cell culture models and human postmortem studies have reported a loss of EAAT2 protein expression in AD, despite no disturbances to EAAT2 mRNA expression (Li et al., 1997; Han et al., 2016). It suggests that any modification to EAAT2 protein expression by Aβ may be posttranslational, but how this may occur is uncertain. However, two studies included in this review reported decreased EAAT2 mRNA in AD tissue (Jacob et al., 2007; Scott et al., 2011), highlighting the contradictory nature of these results, and the inability to rely on the current literature available to accurately determine the processes impacting on EAAT2 mRNA and protein expression in AD. It is also possible that both protein and mRNA expression are differentially affected by AD in distinct brain regions. However, the theory that EAAT2 mRNA expression may be preserved in AD, is supported by findings that SLC1A2 gene expression is not altered in AD (Meng and Mei, 2019). Although the RNAseq data used was from multiple brain regions, it cannot be ruled out that there might be subtle anatomical and layer-specific variations to SLC1A2 expression in response to AD. Further work is needed to confirm EAAT2 mRNA and protein expression in AD, given the contradictory results reported in the above discussed studies.
EAAT2 solubility and aggregation
A decrease in EAAT2 astrocytic protein expression was noted in an AD model by Scimemi et al. (2013), who found Aβ1−42 can cause rapid mislocalization of EAAT2 cell surface expression on astrocytes. Additionally, Woltjer et al. (2010) revealed that detergent-insoluble EAAT2 in the hippocampus and frontal cortex of AD patients was higher than control cases, indicating possible aggregation of EAAT2 in AD. Further analysis, though, revealed no evidence of an association between EAAT2 and amyloid plaques (Woltjer et al., 2010). Perhaps EAAT2 expression is lost from astrocytic membranes in AD and forms insoluble structures, independent of Aβ plaques which could explain these results. The increased EAAT2 in the neuropil seen in our earlier study (Yeung et al., 2021) could also be indicative of aggregation. Sasaki et al. (2009) noted that high molecular weight EAAT2 was detected in the detergent-insoluble fraction of their analyses, again potentially suggesting aggregation of EAAT2 in AD. However, it is uncertain if this is insoluble EAAT2 alone or its co-association with tau or amyloid aggregates. This study did indicate EAAT2 co-precipitates with p-tau (Sasaki et al., 2009), so EAAT2 and tau co-aggregates may occur in AD. Additionally, Hoshi et al. (2018) reported plaque-like staining of EAAT2 in AD, which was co-expressed with aquaporin-4. These results seem to suggest EAAT2 protein expression may be lost from astrocytic membranes in AD and form aggregates. However, it is not clear where EAAT2 expression might be translocated to, or what consequences this may have for progression of disease or neurodegeneration.
EAAT2 as a neuroprotective factor
There is evidence that the preservation of EAAT2 expression on astrocytes may be neuroprotective in the presence of AD pathology (Kobayashi et al., 2018). Lower immunoreactivity of GLT-1 was seen in entorhinal layers I/II and III–VI of cases with dementia (AD-D) compared to control, but those with AD pathology but no dementia (AD-N) showed no change to GLT-1 positive area over control (Kobayashi et al., 2018). This result implies that the maintenance of EAAT2 expression in the presence of AD pathology is neuroprotective and may even be a key factor in determining AD symptomatology, more so than other established pathological AD changes. This study is the only postmortem human evidence supporting the maintenance of or increased EAAT2 expression as a therapeutic target in AD. A study investigating potential differences in serum expression of EAAT2 between control and AD patients found significantly lower expression of EAAT2 in their AD group (Meng et al., 2020). Interestingly, when AD subjects were grouped into “mild,” “moderate,” and “severe” based on the severity of the disease, severe subjects had significantly lower serum expression of EAAT2, and a significant negative correlation between severity and EAAT2 serum expression was noted (Meng et al., 2020). Although it cannot be certain that serum levels of EAAT2 reflect the expression of the protein in the brain, these results broadly seem to support the Kobayashi study. Numerous research from animal studies further supports that higher EAAT2 transporter activity in the presence of AD pathology may be neuroprotective and these results are discussed in the subsequent therapeutics chapter.
EAAT2 splice variants
Alternatively-spliced variants of the EAAT2 protein (intron seven retaining and exon nine skipping) have been documented in both control and AD postmortem tissue, although there was no alteration to their mRNA expression noted in neurological disease (Flowers et al., 2001). However, conflicting evidence suggests an increased mRNA expression of exon 7 and 9 skipping splice variants in AD cases, which was found to correlate with increased pathological severity (Scott et al., 2011). Gebhardt et al. (2010) found evidence for the formation of heteromeric wild-type EAAT2 and variant protein complexes in HEK293 cells. In addition, it was found that cells co-transfected with wild-type and variant EAAT2 showed a reduction in glutamate-dependent activity measured by EC50 and Hill coefficients (Gebhardt et al., 2010). It remains to be determined if this reduction is relevant in the human brain. Due to the small number of studies in current literature, more investigation is needed into the splice variants of EAAT2 and their relationship to AD.
Neuronal expression of EAAT2
Neuronal expression of EAAT2 is still a highly debated area of research. Historically, EAAT2 expression and thus glutamate uptake was thought to be localized exclusively to astrocytes (Milton et al., 1997; Rimmele and Rosenberg, 2016). More recent work though has indicated a small amount of EAAT2 immunoreactivity is likely to be localized to neurons (Roberts et al., 2014). In one immunohistochemistry study, in both the white and gray matter, ~80% of identifiable immunoreactivity for EAAT2 was deemed attributable to astrocytic processes. The remaining immunoreactivity was seen in axon terminals, dendrites or was unidentifiable (Melone et al., 2011). However, the exact differences in physiological function between neuronal and astrocytic EAAT2 are still not well-understood, although EAAT2 expressed on astrocytic processes perform the vast majority of glutamate reuptake and is also responsible for protection against glutamate excitotoxicity (Petr et al., 2015; Danbolt et al., 2016). The study from Sharma et al. (2019) found that an astrocytic deficiency of EAAT2 in mice, rather than neuronal, is more correlated with gene expression profiles seen in aging and AD, indicating that it is likely astrocytic EAAT2 that is affected in AD. Without further study though, it cannot be conclusively ruled out that changes to neuronal EAAT2 may have a role to play in AD.
It has been suggested that neuronal accumulation of EAAT2 may be relevant to neuron degeneration in AD (Pow and Cook, 2009). Some studies have found EAAT2 immunoreactive neurons throughout many brain regions in AD (Thai, 2002). Others have suggested that splice variants of EAAT2 may become translated in stressed neurons and have provided evidence for their accumulation in AD (Pow and Cook, 2009). However, it is inconclusive whether these results reflect true neuronal staining, as neither of these studies included a neuronal marker. Therefore, these immunoreactive “neurons” described in AD tissue may be astrocytic labeling wrapping around neurons or staining of other neuronal-like structures. Future research needs to establish whether EAAT2 accumulation in neurons is relevant in AD and whether this could contribute to AD pathophysiology.
EAAT2 transporter activity in Alzheimer's disease
There are several previous studies in the literature that have investigated the functionality of the EAAT2 transporter in postmortem brains by assessing glutamate uptake. Some of these studies have shown a reduction in glutamate uptake in postmortem AD tissue (Hardy et al., 1987; Procter et al., 1988), while others have found no change (Beckstrøm et al., 1999). An article from Petr et al. (2015), suggests that these discrepancies may be due to the methodology used. From using both astrocytic and neuronal specific knockouts of GLT-1 in mice, they noted that interestingly, neuronal but not astrocytic GLT-1/EAAT2 made a significant contribution to glutamate uptake measured in synaptosomes (Petr et al., 2015). This is paradoxical, as neuronal or axon terminal EAAT2 only accounts for ~20% of its total labeling (Melone et al., 2011), so the astrocytic contribution to uptake would be expected to be much greater. The authors postulate that tissue homogenization may differentially affect astrocytic and neuronal EAAT2 function, with the former being suggested to be localized to leaky compartments and thus is unable to play a role in glutamate uptake. An alternative explanation is that the astrocytic EAAT2 (or a large proportion) may not be functional, perhaps due to environmental context (Petr et al., 2015). Reconstituting synaptosomes in liposomes removed this bias in their study, a step which they suggest may have caused discrepancies in earlier studies, as without reconstitution, astrocytic EAAT2 activity may be completely removed in a fashion unrelated to AD (i.e., unable to contribute to glutamate uptake or not functional; Petr et al., 2015). It is also entirely plausible that the differing results reported in literature may just reflect low sample sizes and variability associated with human tissue use. Moreover, the Petr et al. (2015) study is ultimately an animal study, so we cannot be sure this effect also occurs in human tissue. Nevertheless, these findings highlight the need to carefully consider methodology when comparing results between studies measuring glutamate uptake in AD, as well as the complicated nature of assessing glutamate uptake from both astrocytes and neurons in postmortem tissue.
Evidence for EAAT2 modulation as a valid therapeutic target for the treatment of AD
Modulation of EAATs may have positive therapeutic effects, with their ability to alter glutamate levels providing a logical link in managing glutamate excitotoxicity in the AD brain. Upregulation of EAAT2 has been shown to reduce excitotoxic damage seen in a variety of acute and chronic neurological diseases, and over-expression of EAAT3 appears to protect against toxicity by decreasing the levels of extracellular glutamate (Lewerenz et al., 2006; Sheldon and Robinson, 2007). Furthermore, beneficial effects of memantine, an NMDAR antagonist approved by the FDA for the symptomatic management of AD, indicate that a reduction in circulating glutamate may provide another avenue toward reducing overexcitation of glutamatergic neurons (Plosker and Lyseng-Williamson, 2005).
There has been a focus on identifying and testing novel compounds with EAAT2-enhancing properties to identify their effect on AD models. Synthetic compound LDN/OSU-0212320 improved cognitive functions in the APPSw,Ind mouse model by increasing EAAT2 expression through translational activation (Takahashi et al., 2015). Other methods of EAAT2 modulation have also been explored. A recent medical hypothesis published by Manisha et al. (2020) described increasing EAAT2 activity by promoting glutamate ligand binding through allosteric modulator GT949. Enhancement of glutamate ligand binding is effective in promoting EAAT2 activity independent of substrate pharmacokinetics (Kortagere et al., 2018). Improving EAAT2 activity through increased allosteric binding might be an effective mechanism through which AD-associated EAAT2 dysfunction can be ameliorated.
The antibiotic ceftriaxone is a known activator of EAAT2 transcription, and several studies have investigated whether treatment with this compound may be beneficial in animal models of AD (Zumkehr et al., 2015; Fan et al., 2018; Hamidi et al., 2019). These results suggest that the upregulation of EAAT2 has beneficial effects on cognition and neuronal activity in animal models of AD and is a promising therapeutic target. Human research shows that increased EAAT2 expression is associated with cognitively intact subjects with AD pathology (Kobayashi et al., 2018), potentially validating enhancement of EAAT2 activity as a good therapeutic approach for AD. However, as an antibiotic compound, there are likely to be numerous off-target effects and problems with delivering this drug across the blood-brain barrier. Human research from Lee et al. (2008) has indicated that the NF-kB pathway is responsible for the ceftriaxone dependent upregulation of EAAT2. Perhaps this information could be used to design or search for treatment options that can act similarly to ceftriaxone to induce EAAT2 upregulation. However, more clarification and research is needed before upregulating EAAT2 can be considered a valid strategy to treat the human condition.
Additional novel and well-established compounds have also been examined for their potential affinity and synergistic properties in modulating the EAAT2 transporter. For example, the vitamin E derivative trolox is effective in ameliorating mislocalization of astrocytic EAAT2 in mice exposed to Aβ (Scimemi et al., 2013). Medications used for non-neurodegenerative conditions, such as minocycline, dexamethasone, and histamine, have been shown to have neuroprotective effects through the upregulation of EAAT2 (Fontana, 2015). Examination of current therapeutics used for other neurodegenerative disorders might also be useful as potential drugs for AD. Riluzole, currently approved for the management of amyotrophic lateral sclerosis, has been shown to promote activation of EAAT2 translation and reducing excitotoxic cellular injury in cultured neurons (Kong et al., 2014). Overall, EAAT2 modulation does appear to have neuroprotective effects in AD models, although further studies will be required to explore the applicability of these compounds and their suitability as disease-modifying therapies for AD. A short summary of EAAT2 modulation strategies in the current literature are outlined in Table 3.
Future implications and conclusion
Overall, in this review, we highlight the contradictory nature of existing literature and indicate a potential for maintained EAAT2 astrocytic expression as a potential therapeutic option in AD. First and foremost, though, more research is needed to truly establish what happens to EAAT2 expression on astrocytic membranes in AD, how this may be related to Aβ and tau pathologies, and what effect this may have on excitotoxic glutamate disturbances. In addition, the potential contribution of these changes to AD's clinical symptoms and progression has to be explored. EAAT2 modulation may still be a valid therapeutic strategy for AD treatment or prevention, but these unknowns need answering before compounds aiming to restore EAAT2 function or expression are trialed extensively in clinics to treat AD.
Data availability statement
The original contributions presented in the study are included in the article/Supplementary material, further inquiries can be directed to the corresponding author/s.
Author contributions
OW, JY, and AK: conceptualization, methodology, and writing—original draft preparation. OW, JY, AK, and RF: writing—review and editing. AK and RF: supervision and funding acquisition. AK: project administration. All authors have read and agreed to the published version of the manuscript.
Funding
This work was supported by Alzheimer's New Zealand Charitable Trust (AK; 370836), Alzheimer's New Zealand (AK; 3718869), Freemasons New Zealand (AK; 3719321), Aotearoa Foundation, Centre for Brain Research and University of Auckland (AK; 3705579), and Health Research Council of New Zealand (RLF; 3627373).
Conflict of interest
The authors declare that the research was conducted in the absence of any commercial or financial relationships that could be construed as a potential conflict of interest.
Publisher's note
All claims expressed in this article are solely those of the authors and do not necessarily represent those of their affiliated organizations, or those of the publisher, the editors and the reviewers. Any product that may be evaluated in this article, or claim that may be made by its manufacturer, is not guaranteed or endorsed by the publisher.
References
Abdul, H. M., Sama, M. A., Furman, J. L., Mathis, D. M., Beckett, T. L., Weidner, A. M., et al. (2009). Cognitive decline in Alzheimer's disease is associated with selective changes in calcineurin/NFAT signaling. J. Neurosci. 29, 12957–12969. doi: 10.1523/JNEUROSCI.1064-09.2009
Arriza, J. L., Eliasof, S., Kavanaugh, M. P., and Amara, S. G. (1997). Excitatory amino acid transporter 5, a retinal glutamate transporter coupled to a chloride conductance. Proc. Natl. Acad. Sci. U.S.A. 94, 4155–4160. doi: 10.1073/pnas.94.8.4155
Batarseh, Y. S., Mohamed, L. A., Al Rihani, S. B., Mousa, Y. M., Siddique, A. B., El Sayed, K. A., et al. (2017). Oleocanthal ameliorates amyloid-β oligomers' toxicity on astrocytes and neuronal cells: in vitro studies. Neuroscience 352, 204–215. doi: 10.1016/j.neuroscience.2017.03.059
Beckstrøm, H., Julsrud, L., Haugeto, O., Dewar, D., Graham, D. I., Lehre, K. P., et al. (1999). Interindividual differences in the levels of the glutamate transporters GLAST and GLT, but no clear correlation with Alzheimer's disease. J. Neurosci. Res. 55, 218–229. doi: 10.1002/(SICI)1097-4547(19990115)55:2<218::AID-JNR9>3.0.CO
Bloom, G. S. (2014). Amyloid-β and tau: the trigger and bullet in Alzheimer disease pathogenesis. JAMA Neurol. 71, 505–508. doi: 10.1001/jamaneurol.2013.5847
Braak, H., and Braak, E. (1991). Neuropathological stageing of Alzheimer-related changes. Acta Neuropathol. 82, 239–259. doi: 10.1007/BF00308809
Brookmeyer, R., Johnson, E., Ziegler-Graham, K., and Arrighi, H. M. (2007). Forecasting the global burden of Alzheimer's disease. Alzheimers Dement. 3, 186–191. doi: 10.1016/j.jalz.2007.04.381
Conway, M. E. (2020). Alzheimer's disease: targeting the glutamatergic system. Biogerontology 21, 257–274. doi: 10.1007/s10522-020-09860-4
Danbolt, N. C. (2001). Glutamate uptake. Prog. Neurobiol. 65, 1–105. doi: 10.1016/S0301-0082(00)00067-8
Danbolt, N. C., Furness, D. N., and Zhou, Y. (2016). Neuronal vs. glial glutamate uptake: Resolving the conundrum. Neurochem. Int. 98, 29–45. doi: 10.1016/j.neuint.2016.05.009
Fan, S., Xian, X., Li, L., Yao, X., Hu, Y., Zhang, M., et al. (2018). Ceftriaxone improves cognitive function and upregulates GLT-1-related glutamate-glutamine cycle in APP/PS1 mice. J. Alzheimers. Dis. 66, 1731–1743. doi: 10.3233/JAD-180708
Flowers, J. M., Powell, J. F., Leigh, P. N., Andersen, P., and Shaw, C. E. (2001). Intron 7 retention and exon 9 skipping EAAT2 mRNA variants are not associated with amyotrophic lateral sclerosis. Ann. Neurol. 49, 643–649. doi: 10.1002/ana.1029
Fontana, A. C. (2015). Current approaches to enhance glutamate transporter function and expression. J. Neurochem. 134, 982–1007. doi: 10.1111/jnc.13200
Garcia-Esparcia, P., Diaz-Lucena, D., Ainciburu, M., Torrejón-Escribano, B., Carmona, M., Llorens, F., et al. (2018). Glutamate transporter GLT1 expression in Alzheimer disease and dementia with lewy bodies. Front. Aging Neurosci. 10, 122. doi: 10.3389/fnagi.2018.00122
Gebhardt, F. M., Mitrovic, A. D., Gilbert, D. F., Vandenberg, R. J., Lynch, J. W., and Dodd, P. R. (2010). Exon-skipping splice variants of excitatory amino acid transporter-2 (EAAT2) form heteromeric complexes with full-length EAAT2. J. Biol. Chem. 285, 31313–31324. doi: 10.1074/jbc.M110.153494
Hamidi, N., Nozad, A., Sheikhkanloui Milan, H., Salari, A. A., and Amani, M. (2019). Effect of ceftriaxone on paired-pulse response and long-term potentiation of hippocampal dentate gyrus neurons in rats with Alzheimer-like disease. Life Sci. 238, 116969. doi: 10.1016/j.lfs.2019.116969
Hampel, H., Mesulam, M. M., Cuello, A. C., Farlow, M. R., Giacobini, E., Grossberg, G. T., et al. (2018). The cholinergic system in the pathophysiology and treatment of Alzheimer's disease. Brain 141, 1917–1933. doi: 10.1093/brain/awy132
Han, X., Yang, L., Du, H., Sun, Q., Wang, X., Cong, L., et al. (2016). Insulin attenuates beta-amyloid-associated Insulin/AKT/EAAT signaling perturbations in human astrocytes. Cell. Mol. Neurobiol. 36, 851–864. doi: 10.1007/s10571-015-0268-5
Hardy, J., Cowburn, R., Barton, A., Reynolds, G., Lofdahl, E., O'carroll, A.-M., et al. (1987). Region-specific loss of glutamate innervation in Alzheimer's disease. Neurosci. Lett. 73, 77–80. doi: 10.1016/0304-3940(87)90034-6
Hoshi, A., Tsunoda, A., Yamamoto, T., Tada, M., Kakita, A., and Ugawa, Y. (2018). Altered expression of glutamate transporter-1 and water channel protein aquaporin-4 in human temporal cortex with Alzheimer's disease. Neuropathol. Appl. Neurobiol. 44, 628–638. doi: 10.1111/nan.12475
Huang, S., Tong, H., Lei, M., Zhou, M., Guo, W., Li, G., et al. (2018). Astrocytic glutamatergic transporters are involved in Aβ-induced synaptic dysfunction. Brain Res. 1678, 129–137. doi: 10.1016/j.brainres.2017.10.011
Hynd, M. R., Scott, H. L., and Dodd, P. R. (2004). Glutamate-mediated excitotoxicity and neurodegeneration in Alzheimer's disease. Neurochem. Int. 45, 583–595. doi: 10.1016/j.neuint.2004.03.007
Jacob, C. P., Koutsilieri, E., Bartl, J., Neuen-Jacob, E., Arzberger, T., Zander, N., et al. (2007). Alterations in expression of glutamatergic transporters and receptors in sporadic Alzheimer's disease. J. Alzheimers Dis. 11, 97–116. doi: 10.3233/JAD-2007-11113
Keller, J. N., Mark, R. J., Bruce, A. J., Blanc, E., Rothstein, J. D., Uchida, K., et al. (1997). 4-Hydroxynonenal, an aldehydic product of membrane lipid peroxidation, impairs glutamate transport and mitochondrial function in synaptosomes. Neuroscience 80, 685–696. doi: 10.1016/S0306-4522(97)00065-1
Kim, K., Lee, S. G., Kegelman, T. P., Su, Z. Z., Das, S. K., Dash, R., et al. (2011). Role of excitatory amino acid transporter-2 (EAAT2) and glutamate in neurodegeneration: opportunities for developing novel therapeutics. J. Cell. Physiol. 226, 2484–2493. doi: 10.1002/jcp.22609
Kirvell, S. L., Elliott, M. S., Kalaria, R. N., Hortobágyi, T., Ballard, C. G., and Francis, P. T. (2010). Vesicular glutamate transporter and cognition in stroke: a case-control autopsy study. Neurology 75, 1803–1809. doi: 10.1212/WNL.0b013e3181fd6328
Kobayashi, E., Nakano, M., Kubota, K., Himuro, N., Mizoguchi, S., Chikenji, T., et al. (2018). Activated forms of astrocytes with higher GLT-1 expression are associated with cognitive normal subjects with Alzheimer pathology in human brain. Sci. Rep. 8, 1712. doi: 10.1038/s41598-018-19442-7
Kong, Q., Chang, L. C., Takahashi, K., Liu, Q., Schulte, D. A., Lai, L., et al. (2014). Small-molecule activator of glutamate transporter EAAT2 translation provides neuroprotection. J. Clin. Invest. 124, 1255–1267. doi: 10.1172/JCI66163
Kortagere, S., Mortensen, O. V., Xia, J., Lester, W., Fang, Y., Srikanth, Y., et al. (2018). Identification of novel allosteric modulators of glutamate transporter EAAT2. ACS Chem. Neurosci. 9, 522–534. doi: 10.1021/acschemneuro.7b00308
Lauderback, C. M., Hackett, J. M., Huang, F. F., Keller, J. N., Szweda, L. I., Markesbery, W. R., et al. (2001). The glial glutamate transporter, GLT-1, is oxidatively modified by 4-hydroxy-2-nonenal in the Alzheimer's disease brain: the role of Abeta1-42. J. Neurochem. 78, 413–416. doi: 10.1046/j.1471-4159.2001.00451.x
Lee, S. G., Su, Z. Z., Emdad, L., Gupta, P., Sarkar, D., Borjabad, A., et al. (2008). Mechanism of ceftriaxone induction of excitatory amino acid transporter-2 expression and glutamate uptake in primary human astrocytes. J. Biol. Chem. 283, 13116–13123. doi: 10.1074/jbc.M707697200
Lewerenz, J., Klein, M., and Methner, A. (2006). Cooperative action of glutamate transporters and cystine/glutamate antiporter system Xc- protects from oxidative glutamate toxicity. J. Neurochem. 98, 916–925. doi: 10.1111/j.1471-4159.2006.03921.x
Li, S., Mallory, M., Alford, M., Tanaka, S., and Masliah, E. (1997). Glutamate transporter alterations in Alzheimer disease are possibly associated with abnormal APP expression. J. Neuropathol. Exp. Neurol. 56, 901–911. doi: 10.1097/00005072-199708000-00008
Liang, Z., Valla, J., Sefidvash-Hockley, S., Rogers, J., and Li, R. (2002). Effects of estrogen treatment on glutamate uptake in cultured human astrocytes derived from cortex of Alzheimer's disease patients. J. Neurochem. 80, 807–814. doi: 10.1046/j.0022-3042.2002.00779.x
Malik, A. R., and Willnow, T. E. (2019). Excitatory amino acid transporters in physiology and disorders of the central nervous system. Int. J. Mol. Sci. 20, 5671. doi: 10.3390/ijms20225671
Manisha, C., Selvaraj, A., Jubie, S., Moola Joghee Nanjan, C., Moola Joghee, N., Clement, J. P., et al. (2020). Positive allosteric activation of glial EAAT-2 transporter protein: a novel strategy for Alzheimer's disease. Med Hypothes. 142, 109794. doi: 10.1016/j.mehy.2020.109794
Masliah, E., Hansen, L., Alford, M., Deteresa, R., and Mallory, M. (1996). Deficient glutamate tranport is associated with neurodegeneration in Alzheimer's disease. Ann. Neurol. 40, 759–766. doi: 10.1002/ana.410400512
Melone, M., Bellesi, M., Ducati, A., Iacoangeli, M., and Conti, F. (2011). Cellular and synaptic localization of EAAT2a in human cerebral cortex. Front. Neuroanat. 4, 151. doi: 10.3389/fnana.2010.00151
Meng, G., and Mei, H. (2019). Transcriptional dysregulation study reveals a core network involving the progression of Alzheimer's disease. Front. Aging Neurosci. 11, 101. doi: 10.3389/fnagi.2019.00101
Meng, S. X., Wang, B., and Li, W. T. (2020). Serum expression of EAAT2 and ADORA2A in patients with different degrees of Alzheimer's disease. Eur. Rev. Med. Pharmacol. Sci. 24, 11783–11792. doi: 10.26355/eurrev_202011_23833
Milton, I. D., Banner, S. J., Ince, P. G., Piggott, N. H., Fray, A. E., Thatcher, N., et al. (1997). Expression of the glial glutamate transporter EAAT2 in the human CNS: an immunohistochemical study. Mol. Brain Res. 52, 17–31. doi: 10.1016/S0169-328X(97)00233-7
Mookherjee, P., Green, P. S., Watson, G. S., Marques, M. A., Tanaka, K., Meeker, K. D., et al. (2011). GLT-1 loss accelerates cognitive deficit onset in an Alzheimer's disease animal model. J. Alzheimers. Dis. 26, 447–455. doi: 10.3233/JAD-2011-110503
Murphy-Royal, C., Dupuis, J. P., Varela, J. A., Panatier, A., Pinson, B., Baufreton, J., et al. (2015). Surface diffusion of astrocytic glutamate transporters shapes synaptic transmission. Nat. Neurosci. 18, 219–226. doi: 10.1038/nn.3901
Pajarillo, E., Rizor, A., Lee, J., Aschner, M., and Lee, E. (2019). The role of astrocytic glutamate transporters GLT-1 and GLAST in neurological disorders: potential targets for neurotherapeutics. Neuropharmacology 161, 107559. doi: 10.1016/j.neuropharm.2019.03.002
Petr, G. T., Sun, Y., Frederick, N. M., Zhou, Y., Dhamne, S. C., Hameed, M. Q., et al. (2015). Conditional deletion of the glutamate transporter GLT-1 reveals that astrocytic GLT-1 protects against fatal epilepsy while neuronal GLT-1 contributes significantly to glutamate uptake into synaptosomes. J. Neurosci. 35, 5187–5201. doi: 10.1523/JNEUROSCI.4255-14.2015
Plosker, G. L., and Lyseng-Williamson, K. A. (2005). Memantine: a pharmacoeconomic review of its use in moderate-to-severe Alzheimer's disease. Pharmacoeconomics 23, 193–206. doi: 10.2165/00019053-200523020-00010
Poirel, O., Mella, S., Videau, C., Ramet, L., Davoli, M. A., Herzog, E., et al. (2018). Moderate decline in select synaptic markers in the prefrontal cortex (BA9) of patients with Alzheimer's disease at various cognitive stages. Sci. Rep. 8, 938. doi: 10.1038/s41598-018-19154-y
Pow, D. V., and Cook, D. G. (2009). Neuronal expression of splice variants of “glial” glutamate transporters in brains afflicted by Alzheimer's disease: unmasking an intrinsic neuronal property. Neurochem. Res. 34, 1748–1757. doi: 10.1007/s11064-009-9957-0
Procter, A. W., Palmer, A. M., Francis, P. T., Lowe, S. L., Neary, D., Murphy, E., et al. (1988). Evidence of glutamatergic denervation and possible abnormal metabolism in Alzheimer's disease. J. Neurochem. 50, 790–802. doi: 10.1111/j.1471-4159.1988.tb02983.x
Revi, M. (2020). Alzheimer's disease therapeutic approaches. Adv. Exp. Med. Biol. 1195, 105–116. doi: 10.1007/978-3-030-32633-3_15
Rimmele, T. S., and Rosenberg, P. A. (2016). GLT-1: the elusive presynaptic glutamate transporter. Neurochem. Int. 98, 19–28. doi: 10.1016/j.neuint.2016.04.010
Roberts, R. C., Roche, J. K., and Mccullumsmith, R. E. (2014). Localization of excitatory amino acid transporters EAAT1 and EAAT2 in human postmortem cortex: a light and electron microscopic study. Neuroscience 277, 522–540. doi: 10.1016/j.neuroscience.2014.07.019
Rothstein, J. D., Martin, L. J., and Kuncl, R. W. (1992). Decreased glutamate transport by the brain and spinal cord in amyotrophic lateral sclerosis. N. Engl. J. Med. 326, 1464–1468. doi: 10.1056/NEJM199205283262204
Sasaki, K., Shimura, H., Itaya, M., Tanaka, R., Mori, H., Mizuno, Y., et al. (2009). Excitatory amino acid transporter 2 associates with phosphorylated tau and is localized in neurofibrillary tangles of tauopathic brains. FEBS Lett. 583, 2194–2200. doi: 10.1016/j.febslet.2009.06.015
Scimemi, A., Meabon, J. S., Woltjer, R. L., Sullivan, J. M., Diamond, J. S., and Cook, D. G. (2013). Amyloid-beta1-42 slows clearance of synaptically released glutamate by mislocalizing astrocytic GLT-1. J. Neurosci. 33, 5312–5318. doi: 10.1523/JNEUROSCI.5274-12.2013
Scott, H. A., Gebhardt, F. M., Mitrovic, A. D., Vandenberg, R. J., and Dodd, P. R. (2011). Glutamate transporter variants reduce glutamate uptake in Alzheimer's disease. Neurobiol. Aging 32, 553.e1–553.e11. doi: 10.1016/j.neurobiolaging.2010.03.008
Šerý, O., Povová, J., Míšek, I., Pešák, L., and Janout, V. (2013). Review paperMolecular mechanisms of neuropathological changes in Alzheimer's disease: a review. Folia Neuropathol. 51, 1–9. doi: 10.5114/fn.2013.34190
Sharma, A., Kazim, S. F., Larson, C. S., Ramakrishnan, A., Gray, J. D., McEwen, B. S., et al. (2019). Divergent roles of astrocytic versus neuronal EAAT2 deficiency on cognition and overlap with aging and Alzheimer's molecular signatures. Proc. Natl. Acad. Sci. U.S.A. 116, 21800–21811. doi: 10.1073/pnas.1903566116
Sheldon, A. L., and Robinson, M. B. (2007). The role of glutamate transporters in neurodegenerative diseases and potential opportunities for intervention. Neurochem. Int. 51, 333–355. doi: 10.1016/j.neuint.2007.03.012
Simpson, J. E., Ince, P. G., Lace, G., Forster, G., Shaw, P. J., Matthews, F., et al. (2010). Astrocyte phenotype in relation to Alzheimer-type pathology in the ageing brain. Neurobiol. Aging 31, 578–590. doi: 10.1016/j.neurobiolaging.2008.05.015
Takahashi, K., Kong, Q., Lin, Y., Stouffer, N., Schulte, D. A., Lai, L., et al. (2015). Restored glial glutamate transporter EAAT2 function as a potential therapeutic approach for Alzheimer's disease. J. Exp. Med. 212, 319–332. doi: 10.1084/jem.20140413
Thai, D. R. (2002). Excitatory amino acid transporter EAAT-2 in tangle-bearing neurons in Alzheimer's disease. Brain Pathol. 12, 405–411. doi: 10.1111/j.1750-3639.2002.tb00457.x
Thal, D. R., Papassotiropoulos, A., Saido, T. C., Griffin, W. S., Mrak, R. E., Kölsch, H., et al. (2010). Capillary cerebral amyloid angiopathy identifies a distinct APOE epsilon4-associated subtype of sporadic Alzheimer's disease. Acta Neuropathol. 120, 169–183. doi: 10.1007/s00401-010-0707-9
Tian, G., Kong, Q., Lai, L., Ray-Chaudhury, A., and Lin, C. L. (2010). Increased expression of cholesterol 24S-hydroxylase results in disruption of glial glutamate transporter EAAT2 association with lipid rafts: a potential role in Alzheimer's disease. J. Neurochem. 113, 978–989. doi: 10.1111/j.1471-4159.2010.06661.x
Tolar, M., Abushakra, S., and Sabbagh, M. (2019). The path forward in Alzheimer's disease therapeutics: reevaluating the amyloid cascade hypothesis. Alzheimer's Dement. 16, 1553–1560. doi: 10.1016/j.jalz.2019.09.075
Wang, R., and Reddy, P. H. (2017). Role of glutamate and NMDA receptors in Alzheimer's disease. J. Alzheimers. Dis. 57, 1041–1048. doi: 10.3233/JAD-160763
Woltjer, R. L., Duerson, K., Fullmer, J. M., Mookherjee, P., Ryan, A. M., Montine, T. J., et al. (2010). Aberrant detergent-insoluble excitatory amino acid transporter 2 accumulates in Alzheimer disease. J. Neuropathol. Exp. Neurol. 69, 667–676. doi: 10.1097/NEN.0b013e3181e24adb
Yeung, J. H. Y., Palpagama, T. H., Wood, O. W. G., Turner, C., Waldvogel, H. J., Faull, R. L. M., et al. (2021). EAAT2 expression in the hippocampus, subiculum, entorhinal cortex and superior temporal gyrus in Alzheimer's disease. Front. Cell. Neurosci. 15, 702824. doi: 10.3389/fncel.2021.702824
Zoia, C., Cogliati, T., Tagliabue, E., Cavaletti, G., Sala, G., Galimberti, G., et al. (2004). Glutamate transporters in platelets: EAAT1 decrease in aging and in Alzheimer's disease. Neurobiol. Aging 25, 149–157. doi: 10.1016/S0197-4580(03)00085-X
Zoia, C. P., Riva, C., Isella, V., Proserpio, P., Terruzzi, A., Arban, S., et al. (2011). Nonfibrillar Abeta 1–42 inhibits glutamate uptake and phosphorylates p38 in human fibroblasts. Alzheimer Dis. Assoc. Disord. 25, 1641172. doi: 10.1097/WAD.0b013e3181f9860f
Zumkehr, J., Rodriguez-Ortiz, C. J., Cheng, D., Kieu, Z., Wai, T., Hawkins, C., et al. (2015). Ceftriaxone ameliorates tau pathology and cognitive decline via restoration of glial glutamate transporter in a mouse model of Alzheimer's disease. Neurobiol. Aging 36, 2260–2271. doi: 10.1016/j.neurobiolaging.2015.04.005
Keywords: glutamate transporter, EAAT2, hippocampus, Alzheimer's disease, human brain
Citation: Wood OWG, Yeung JHY, Faull RLM and Kwakowsky A (2022) EAAT2 as a therapeutic research target in Alzheimer's disease: A systematic review. Front. Neurosci. 16:952096. doi: 10.3389/fnins.2022.952096
Received: 24 May 2022; Accepted: 14 July 2022;
Published: 10 August 2022.
Edited by:
Yang Zhang, Chongqing University, ChinaReviewed by:
Sinead M. O'Donovan, University of Toledo, United StatesKejing Lao, Xi'an Medical University, China
Copyright © 2022 Wood, Yeung, Faull and Kwakowsky. This is an open-access article distributed under the terms of the Creative Commons Attribution License (CC BY). The use, distribution or reproduction in other forums is permitted, provided the original author(s) and the copyright owner(s) are credited and that the original publication in this journal is cited, in accordance with accepted academic practice. No use, distribution or reproduction is permitted which does not comply with these terms.
*Correspondence: Andrea Kwakowsky, YW5kcmVhLmt3YWtvd3NreUBudWlnYWx3YXkuaWU=
†These authors have contributed equally to this work