- 1Department of Rehabilitation Medicine, The First Affiliated Hospital of Nanjing Medical University, Nanjing, China
- 2Department of Otolaryngology, Jiangsu Province Hospital of Chinese Medicine, Affiliated Hospital of Nanjing University of Chinese Medicine, Nanjing, China
- 3Spine Disease Institute, Longhua Hospital, Shanghai University of Traditional Chinese Medicine, Shanghai, China
- 4Key Laboratory of Theory and Therapy of Muscles and Bones, Ministry of Education, Shanghai University of Traditional Chinese Medicine, Shanghai, China
Spinal cord injury (SCI) is a devastating condition with few treatment options. Metformin, a classical antidiabetic and antioxidant, has extended its application to experimental SCI treatment. Here, we performed a systematic review to evaluate the neurobiological roles of metformin for treating SCI in rats, and to assess the potential for clinical translation. PubMed, Embase, China National Knowledge Infrastructure, WanFang data, SinoMed, and Vip Journal Integration Platform databases were searched from their inception dates to October 2021. Two reviewers independently selected controlled studies evaluating the neurobiological roles of metformin in rats following SCI, extracted data, and assessed the quality of methodology and evidence. Pairwise meta-analyses, subgroup analyses and network analysis were performed to assess the roles of metformin in neurological function and tissue damage in SCI rats. Twelve articles were included in this systematic review. Most of them were of moderate-to-high methodological quality, while the quality of evidence from those studies was not high. Generally, Basso, Beattie, and Bresnahan scores were increased in rats treated with metformin compared with controls, and the weighted mean differences (WMDs) between metformin and control groups exhibited a gradual upward trend from the 3rd (nine studies, n = 164, WMD = 0.42, 95% CI = −0.01 to 0.85, P = 0.06) to the 28th day after treatment (nine studies, n = 136, WMD = 3.48, 95% CI = 2.04 to 4.92, P < 0.00001). Metformin intervention was associated with improved inclined plane scores, tissue preservation ratio and number of anterior horn motor neurons. Subgroup analyses indicated an association between neuroprotection and metformin dose. Network meta-analysis showed that 50 mg/kg metformin exhibited greater protection than 10 and 100 mg/kg metformin. The action mechanisms behind metformin were associated with activating adenosine monophosphate-activated protein kinase signaling, regulating mitochondrial function and relieving endoplasmic reticulum stress. Collectively, this review indicates that metformin has a protective effect on SCI with satisfactory safety and we demonstrate a rational mechanism of action; therefore, metformin is a promising candidate for future clinical trials. However, given the limitations of animal experimental methodological and evidence quality, the findings of this pre-clinical review should be interpreted with caution.
Introduction
Spinal cord injury (SCI) is a catastrophic event associated with high morbidity and mortality (Lucchesi et al., 2019). Most of the SCIs arise from physical trauma, with an incidence of 210 cases and a prevalence of 5,410 cases per million in the United States (Feigin et al., 2021). Worldwide, there are over 700,000 new cases of traumatic SCI annually and ~27.04 million people are affected by SCI, which equates to 9.5 million years of life lived with disability (Kumar et al., 2018; Gbd, 2019; Lucchesi et al., 2019). However, effective therapeutic strategies for this condition remain limited.
The pathological progression of SCI is multifactorial. Contusion or transection is frequently the initial insult, which leads to structural disruption and breakdown of tissue homeostasis promptly (Anjum et al., 2020; Lin et al., 2022). Subsequently, the acute phase of secondary damage, involving oxidative stress, membrane and ionic dysregulation, neurotransmitter toxicity, and vascular dysfunction, glial cell activation, and neuronal death, is triggered (Tran et al., 2018; Anjum et al., 2020; Xu et al., 2020). Despite the wide range of mechanisms involved in these pathological processes, oxidative stress, and adenosine monophosphate-activated protein kinase (AMPK) are believed to be critical for progression of SCI (Visavadiya et al., 2016; Wang et al., 2018). For instance, increased generation of reactive oxygen species (ROS) following central nervous system (CNS) injury can activate mitogen-activated protein kinase (MAPK) or the nod-like receptor family, pyrin domain-containing three (NLRP3) inflammasome to induce an neuroinflammation response, and may also lead to neuronal damage via a wide range of mechanisms (Sarkar et al., 2017; Khatri et al., 2018). Then, activation of AMPK signaling can downregulate the formation of ROS, thereby exerting anti-inflammatory and neuroprotective effects on CNS injury (Jiang et al., 2020; Hu et al., 2021).
Metformin is a classical antidiabetic drug that can activate AMPK signaling and reduce ROS production (Apostolova et al., 2020). With a satisfactory safety profile, metformin has been approved by the Food and Drug Administration as a conventional treatment for type 2 diabetes. Recently, metformin has shown promise in the treatment of obesity, cancer, stroke and cardiovascular disease (Luo et al., 2019; Ma et al., 2020). Given the important role of AMPK signaling and excessive ROS production in SCI, metformin is trying to find its new application in flied of SCI (Wang et al., 2020; Zhou et al., 2020a). Multiple pre-clinical studies have reported favorable neuroprotection by metformin in the treatment of SCI (Wang et al., 2020; Zhang et al., 2020). While, Lin et al. (2015) demonstrated a negative result of metformin for SCI. The issues regarding the candidate administration does, time window of intervention and administration times of metformin for SCI remain poorly understood. Additionally, the systematic knowledges of the safety and pharmacological mechanism of metformin in treating SCI are still limited.
Comprehensively understanding of efficacy, administration details, safety, and pharmacological mechanism is critical for evaluating clinical translation. Chen et al. (2021) conducted a systematic review including seven studies to indicate the effects of metformin on locomotor function recovery in rats after SCI, hinting an application prospective of metformin in SCI. Here, we performed an updated and combined review for analyzing all of those parameters. Compared with Chen et al. (2021), we identified 12 references with inconsistent result tendencies to conducted the systematic review. For the parameter of efficacy, we primarily focused on the dynamic changes of locomotor function after metformin intervention, and paid attention to the effects of metformin on the tissue damage in SCI. In regard to administration details, series of variables concerning metformin dose, intervention timing and administration times participate in subgroup analyses. And a network meta-analysis was carried out for predicting the candidate administration does. Furthermore, we combined the advantages of a traditional review for the knowledges about the safety, pharmacokinetics in the CNS, and mechanism in treating SCI of metformin. Under the comprehensive analysis, this review was aimed to adequately evaluate the neurobiological roles of metformin for treating SCI in rats and describe the potential for future clinical trials and applications.
Materials and methods
Literature search
PubMed, Embase, China National Knowledge Infrastructure, WanFang data, Vip Journal Integration Platform, and SinoMed databases were searched from their inception dates to October 2021. According to the guide of search strategy (Leenaars et al., 2012), “spinal cord injuries,” “spinal cord injury,” “spinal cord diseases,” “spinal cord compression,” “spinal cord trauma,” “metformin,” “biguanides,” “guanidines,” “rats,” and “murinae” were used as the key search terms. Reference lists of selected articles were also searched to find additional studies. Appendix 1 contains the search strategy in PubMed database.
Selection of studies
According to the eligibility criteria, two reviewers independently selected the articles by screening the abstracts and full texts. Disagreements were resolved by consensus following discussion with a third reviewer.
Eligibility criteria
Types of study
Controlled studies evaluating the neurobiological roles of metformin in rats following SCI were searched. All clinical case reports or in vitro studies were excluded. No language, publication date, or publication status were restricted (Yao et al., 2015).
Types of participants
Laboratory rats of any age, sex, or strain that were subjected to SCI induced by compression or contusion were included. Studies with local or global ischemia, traumatic root avulsion, chronic SCI or genetically modified models were excluded (Oliveri et al., 2014; Sng et al., 2022). Additionally, laceration/transection types of SCI were also excluded because these models do not represent the typical crush injury mechanism in humans (Dietz and Curt, 2006; Oliveri et al., 2014).
Types of intervention
Any type of metformin intervention compared with a placebo control was included. Placebo controls included saline, vehicle, dimethyl sulfoxide, or no treatment (Tian et al., 2020). Multiple treatment combinations (e.g., metformin plus methylprednisolone) were excluded.
Types of outcome measure
The 21-point Basso, Beattie, and Bresnahan (BBB) locomotor rating scale is a widely used ordinal scale involving assessments of hindlimb joints, paw placement during stepping, weight support, and forelimb-hindlimb coordination of animals (Basso et al., 1995). Briefly, examiners subjectively registered the limb movements and walking characteristics in an open-field arena, then assigned a BBB scale score ranging from 0 (complete paralysis) to 21 (normal locomotion) for indicating the basic locomotion of an animal (Zhou et al., 2020b). Only data at the same time points were used in the analyses of BBB scores.
The inclined plane test describes the maximal angle at which rats can maintain themselves on an inclined plane measured in 0–90° (Rivlin and Tator, 1978), and is used to evaluate limb muscle strength. Animals were placed transversely on the rubber inclined plane and the highest angle the rat could maintain for 5 s was recorded (Chen et al., 2018).
In histopathological analysis, tissue preservation area and the number of motor neurons in anterior horn area of the spinal cord are widely applied indicators for injury severity and the prospect of locomotor recovery after SCI (Li et al., 2017; Wang et al., 2020). To reduce the risk of data heterogeneity, only data obtained by the same detection method was used in the analyses.
Data extraction
Data including the name of the first author, publication year, animal strain and sex, animal age and weight, number of animals, SCI model, spinal level of trauma, metformin administration, and measured outcomes were extracted from included studies. Moreover, to investigate the potential therapeutic mechanism of metformin for SCI, the proposed mechanisms and changes of related molecules were also extracted from those studies. In studies with multiple intervention arms, only data from the metformin and negative control groups were used in our analysis. If data were not numerically described in the text, we estimated the values from graphs using GetData Graph Digitizer 2.26 (http://getdata-graph-digitizer.com; Yao et al., 2015). If data were missing, authors were contacted.
Risk of bias assessment
The SYstematic Review Centre for Laboratory animal Experimentation risk of bias (SYRCLE's RoB) tool, a specially designed system for animal studies, was employed to assess the methodological quality of the studies (Hooijmans et al., 2014). This tool encompasses 10 items for evaluating selection bias, performance bias, detection bias, attrition bias, reporting bias, and other biases. The items were scored with “yes,” “no,” or “unclear,” indicating a low risk, a high risk or insufficient details to assess the risk of bias, respectively. Two investigators independently assessed the methodological quality according to this tool.
Assessment of quality of evidence
The assessment of quality of evidence from included studies was based on the previously reported strategy (Charalambous et al., 2014, 2016). This strategy classifies the experimental laboratory animal studies (ELAS) into three groups. The first group includes blinded randomized ELAS (bRELAS) and non-blinded RELAS (nbRELAS). Non-randomized RELAS (nRELAS) and uncontrolled ELAS (UELAS) were defined as the second group. Case series and reports belong to the third group. The studies in the first group were considered to provide higher quality evidence, followed by the studies in the second and third group. Additionally, a three-part system of evidence quality assessment was used to indicate the strengths and weaknesses of each study within each group, including study group sizes, subject enrolment quality and overall risk of bias based on SYRCLE's RoB tool. For example, bRELAS with large group sizes, clear inclusion criteria, thorough diagnostic investigations and low overall risk of bias were considered to provide the highest available quality of evidence (Charalambous et al., 2016).
Statistical analyses
Pairwise meta-analyses and subgroup analyses were produced with Review Manager version 5.3 (provided by the Cochrane Collaboration). For all outcome measures, differences were considered statistically significant at P < 0.05 (two-tailed). Pooled data for each outcome were reported as weighted mean differences (WMDs) with 95% confidence intervals (CIs). Heterogeneity between studies was evaluated by χ2 and Cochrane's I2 (Higgins and Green, 2011). The random effect model was applied because it provides the expected average of all samples of individual true effect sizes, rather than a single common true effect size from dispersed studies caused by sampling errors. Line graphs were constructed by GraphPad Prism 8.0.2 software (GraphPad Software, San Diego, California USA) to highlight the dynamic WMDs of BBB scores and dynamic BBB score improvements in both groups (Sng et al., 2022).
To explore the appropriate dose of metformin, a network meta-analysis was preformed according to the Bayesian method using Stata 12.0 (StataCorp LP, College Station, Texas, USA) to simultaneously compare direct and indirect treatment regimens. Probability of being the best intervention and surface under cumulative ranking (SUCRA) are usually applied to determine the rank order of interventions (Mbuagbaw et al., 2017; Owen et al., 2020). Stata reports both the probabilities and SUCRA based on the reported computational formula (Chiocchia et al., 2020). For every treatment dose, we calculated the probabilities of its efficacy, and then ranked the treatments according to SUCRA.
Results
Study selection
Our systematic search identified 1,562 potentially relevant references. Following the removal of duplications and title/abstract screening, 14 papers were selected for full-text screening. Two publications (Zhang, 2017; Zhao, 2021) were excluded at the full-text stage because the data in these articles were reported in Zhang (2017) and Zhao (2021), respectively. Twelve articles were, therefore, included in this systematic review and meta-analysis (Figure 1; Lin et al., 2015; Wang et al., 2016, 2018, 2020; Zhang et al., 2017a,b, 2020; Afshari et al., 2018; Guo et al., 2018, 2019; Wu et al., 2021; Zhao et al., 2021).
Characteristics of the included studies
Of the 12 publications from 2015 to 2021, 10 were presented in English, the other two in Chinese (Guo et al., 2019; Zhao et al., 2021). The sample size ranged from 18 to 114 animals. Seven publications demonstrated an SCI model induced by aneurysm clip compression, and the others used a weight-drop impactor method (Wang et al., 2016; Guo et al., 2018, 2019; Zhang et al., 2020; Zhao et al., 2021). All studies caused trauma at the T7–T11 level. Eight studies reported the source of metformin (Lin et al., 2015; Wang et al., 2016, 2018; Zhang et al., 2017a,b; Afshari et al., 2018; Guo et al., 2019; Wu et al., 2021). The placebo controls included saline and dimethyl sulfoxide diluted with saline. Metformin dose ranged from 10 to 320 mg/kg, and all studies used intraperitoneal injection except Lin et al. (2015). Most studies injected metformin immediately post injury, while five studies treated SCI from the 1st day or 1st week after trauma (Lin et al., 2015; Wang et al., 2016; Guo et al., 2018; Zhang et al., 2020; Zhao et al., 2021). Ten studies applied repeated administration of metformin, once daily for 3–28 days after SCI, whereas a single dose of metformin was employed in the remaining studies (Table 1; Afshari et al., 2018; Wang et al., 2018).
Risk of bias within studies
The mean number of reported items in SYRCLE's RoB tools was 4.5. Six studies (50%) adequately described 5–6 items in the standard checklist list (Lin et al., 2015; Zhang et al., 2017a,b, 2020; Afshari et al., 2018; Wu et al., 2021). The items of “baseline characteristics,” “selective outcome reporting,” and “outcome assessor blinding” were well-evaluated items, with an appropriate description in 100, 100, and 91.7% of included studies, respectively. In contrast, the items of “sequence generation,” “allocation concealment,” “random housing,” “investigator blinding,” and “random outcome assessment” were poorly delineated in included studies, since none or few of them reported those details. Incomplete outcome data was adequately addressed in four studies (Lin et al., 2015; Zhang et al., 2017a,b; Wu et al., 2021). In additional, we did not identify any other sources of bias such as pooling of drugs, dropouts, unit of analysis error, design-specific bias, and bias due to inappropriate influence of funder (Table 2).
Quality of evidence within studies
Overall, the majority of the studies included in this review did not offer high quality of evidence (Table 3). Half of included studies were classified into bRELAS or nbRELAS (Wang et al., 2016; Guo et al., 2018, 2019; Zhang et al., 2020; Wu et al., 2021; Zhao et al., 2021), the remaining belonged to the nRELAS. In addition, five study included a small study population size (Zhang et al., 2017a,b; Guo et al., 2018; Wang et al., 2018; Zhao et al., 2021). Only three studies adequately addressed details of the subject enrolment quality (Zhang et al., 2020; Wu et al., 2021; Zhao et al., 2021). A potential risk of bias was widely presented in methodological quality of included studies. Thus, the results based on those studies should be interpreted with caution.
Overall analysis of the effects of metformin
BBB scores was employed in all included studies to evaluate locomotor recovery in rats. Nine studies collected dynamic BBB score information from 1 to 28 days after SCI (Lin et al., 2015; Wang et al., 2016, 2018; Zhang et al., 2017a, 2020; Afshari et al., 2018; Guo et al., 2018; Wu et al., 2021; Zhao et al., 2021). All studies except one (Lin et al., 2015) reported improved BBB scores in metformin-treated rats compared with placebo intervention. The meta-analyses and dynamic BBB scores in both groups also revealed that BBB scores were increased in metformin-treated animals compared with controls over the 7th (12 studies, n = 198, WMD = 1.41, 95% CI = 1.01–1.80, P < 0.00001) to 28th day after injury (nine studies, n = 136, WMD = 3.48, 95% CI = 2.04–4.92, P < 0.00001), and the WMDs between the two groups showed a gradual upward trend with time (Figures 2A,C,D and Table 4). Furthermore, meta-analyses regarding inclined plane scores confirmed the positive recovery effects of metformin for rats subjected to SCI (Figure 2B and Table 4).
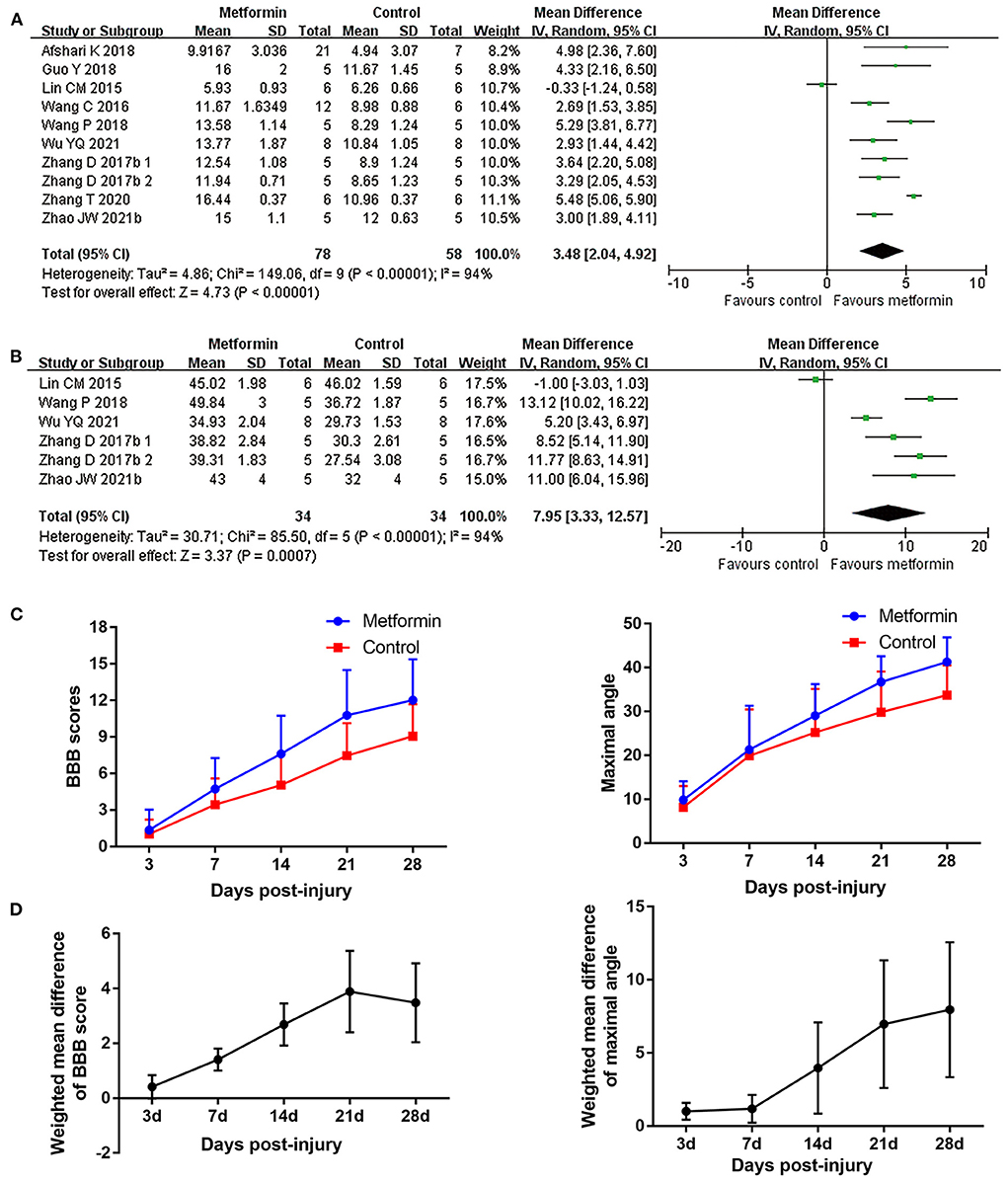
Figure 2. Overall analyses of the effects of metformin on dynamic changes of neurological function. (A,B) BBB scale, inclined plane test meta-analysis at 28th day after SCI. (C) The BBB scores and inclined plane scores in each group over time. (D) The WMDs of BBB score between metformin and control groups from 3rd to 28th day after SCI.
Four studies applied hematoxylin-eosin staining to describe the preservation area in the form residual tissue/cross-sectional area ratio (Wang et al., 2016, 2020; Zhang et al., 2017a, 2020). Meta-analyses revealed metformin treatment resulted in a higher percentage of preserved tissue compared with controls (four studies, n = 54, WMD = 13.29, 95% CI = 2.66–23.93, P = 0.01, Figure 3 and Table 4). Additionally, another study with lesion area data in longitudinal sections demonstrated suppression of lesion area in the injury site after metformin treatment (Wu et al., 2021).
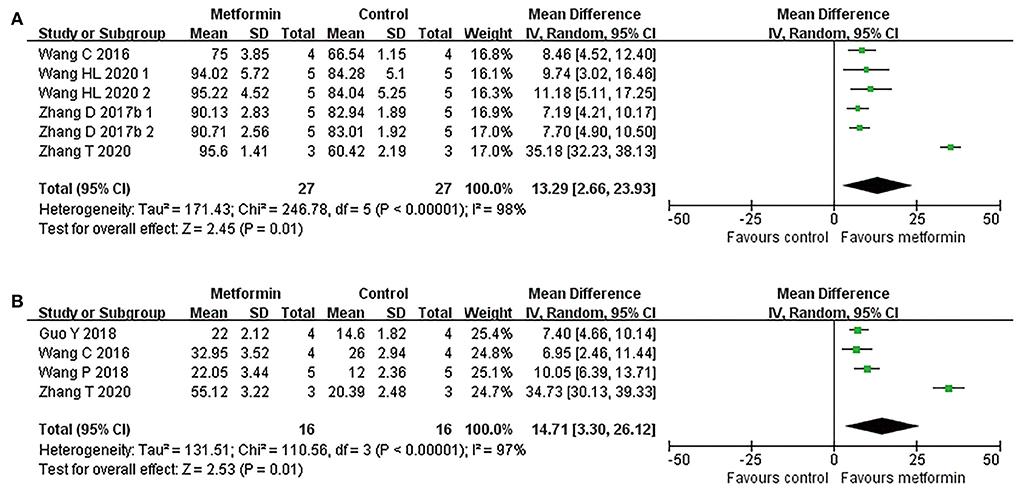
Figure 3. Overall analyses of the effects of metformin on tissue damage in lesion area. (A,B) Tissue preservation area ratio, number of survival motor neuron meta-analysis in lesion area and the effect sizes line chart.
By counting the Nissl positive cells in anterior horn area of the spinal cord, six publications reported the number of motor neurons in this area (Wang et al., 2016, 2018; Zhang et al., 2017a, 2020; Guo et al., 2018; Wu et al., 2021). Of the five studies reporting the mean number of anterior horn motor neuron in a single cross section, one presented these data in the epicenter and in both side-regions of the injury separately (Zhang et al., 2017a), while the remaining studies calculated this mean count in the global lesion site (Wang et al., 2016, 2018; Guo et al., 2018; Zhang et al., 2020). Consistently, data presented in different injury segments or meta-analyses of the four studies both suggested an increased number of survived motor neurons in metformin-treated rats compared with controls (four studies, n = 32, WMD = 14.71, 95% CI =3.30–26.12, P = 0.01, Figure 3 and Table 4).
Subgroup analyses of the effects of metformin
Subgroup analyses indicated that there was no statistical difference in BBB scores between rats based on gender, compression, or contusion injury model, timing of metformin administration (immediately or from the 1st day following injury), or number of injections Figures 4, 5, Supplementary Figure 1, and Table 5). Dose was argued to critically impact metformin efficacy (Riddle, 2000; Araújo et al., 2017). Interestingly, analyses with respect to metformin doses indicated an association of locomotor recovery with administration dose (Figure 5 and Table 5). Recent studies suggest that locomotor function, systemic symptoms, and pathology may differ depending on the level of SCI and rat strain used (Wilcox et al., 2017; Hong et al., 2018). However, analysis of distinct strains and injury levels was not preformed because these factors were consistent in our included studies.
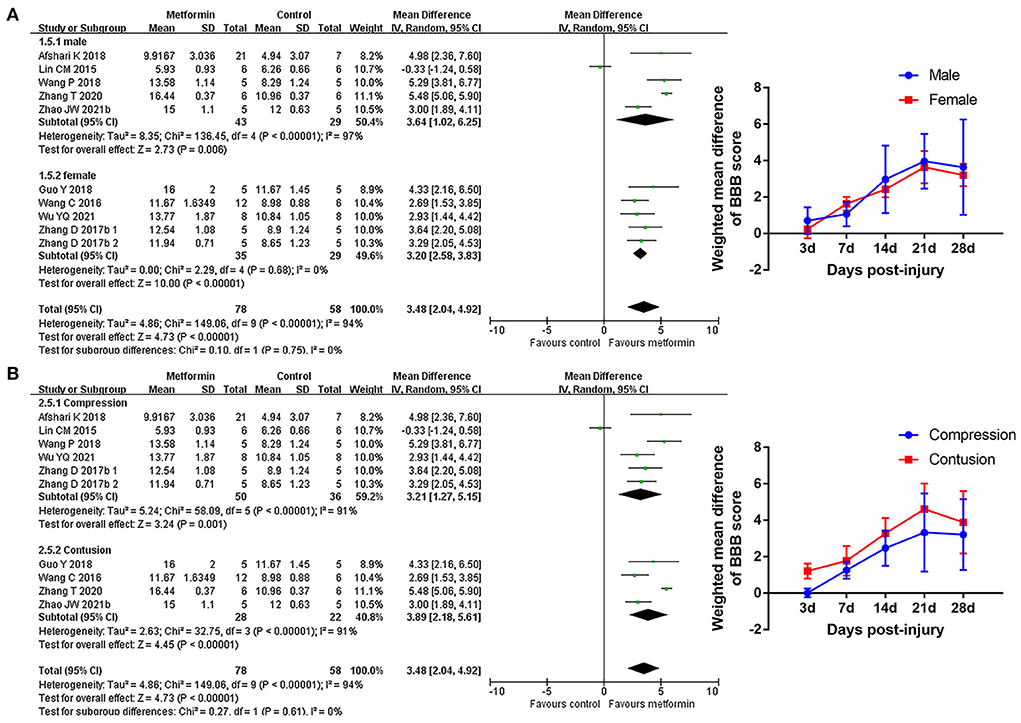
Figure 4. BBB scale subgroup analysis concerning rat gender and injury model type. (A,B) Subgroup analysis concerning rat gender and injury model type at 28th day after SCI and the WMDs of BBB score over time in different subgroups.
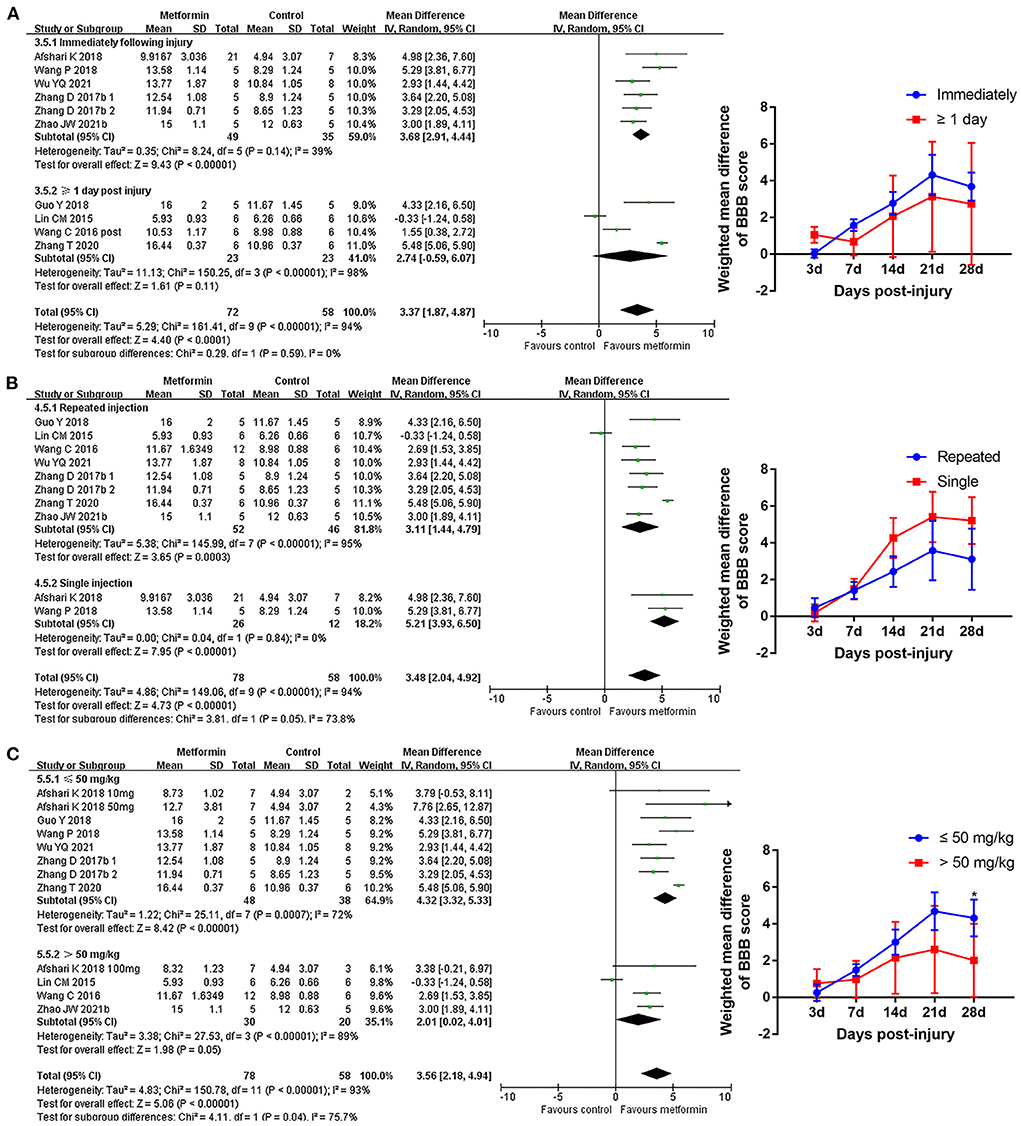
Figure 5. BBB scale subgroup analysis concerning administration details. (A–C) Subgroup analysis concerning administration timing, injection numbers and administration dose at 28th day after SCI and the WMDs of BBB score over time in different subgroups. *p < 0.05.
Network analysis of the effects of metformin dose
To comprehensively compare the effects of metformin at different doses, BBB scores from day 14 to 28 after SCI were employed in our network analysis. A forest plot using data from the 28th day revealed that 50 mg/kg metformin exhibited a greater effect compared with 10, 100, and 200 mg/kg metformin (Figure 6), and seemed to be the best treatment regimen on the basis of the SUCRA value (Figures 7A,B). The superior treatment effect and SUCRA value for 50 mg/kg metformin was confirmed on the 14th and 21st days after SCI (Figures 7C,D, Supplementary Figure 2).
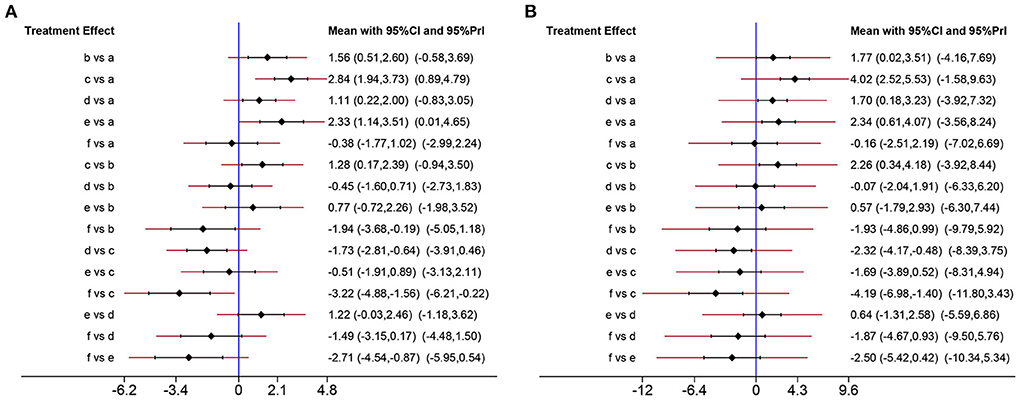
Figure 6. Network analysis of the effects of metformin at different doses. (A) Forest plot of effect size in different metformin doses according to data on 28th day. (B) Forest plot of effect size in different metformin doses according to data on 21th day. a, Control; b, 10 mg/kg metformin; c, 50 mg/kg metformin; d, 100 mg/kg metformin; e, 200 mg/kg metformin; f, 320 mg/kg metformin.
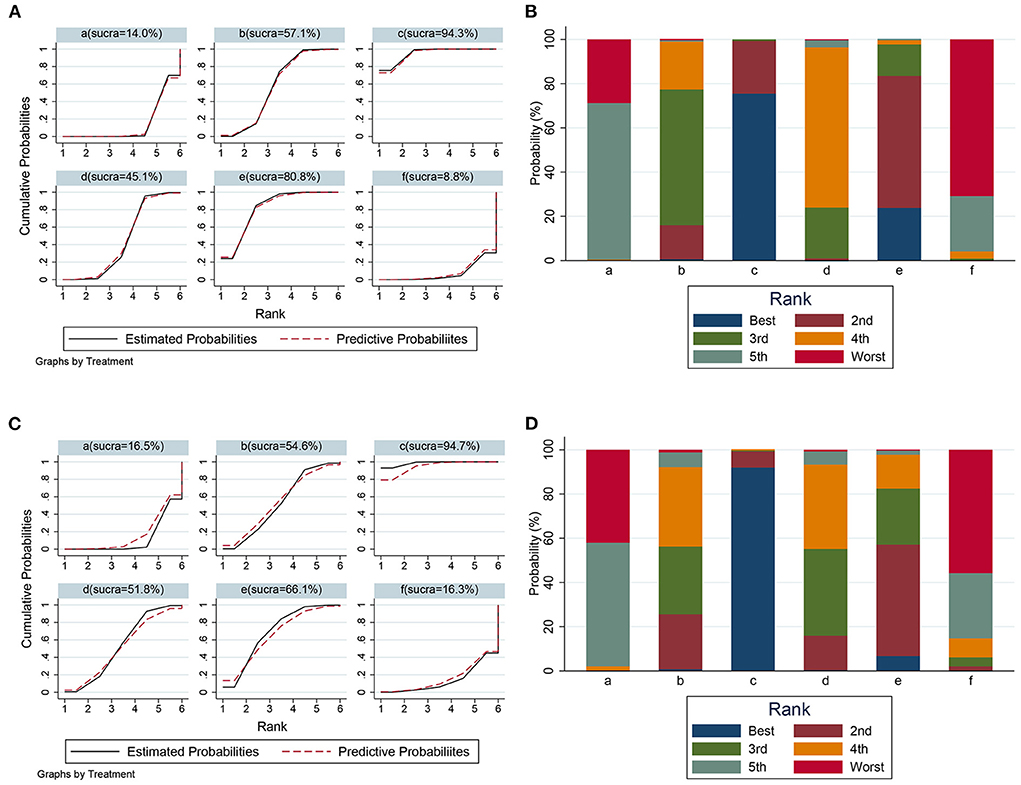
Figure 7. The SUCRA value and probabilities of each treatment doses. (A) SUCRA value ranking of different administration doses according to data on 28th day. (B) Histogram of ranking probability of each treatment dose according to data on 28th day. (C) SUCRA value ranking of different administration doses according to data on 21th day. (D) Histogram of ranking probability of each treatment dose according to data on 21th day. a, control; b, 10 mg/kg metformin; c, 50 mg/kg metformin; d, 100 mg/kg metformin, e, 200 mg/kg metformin; f, 320 mg/kg metformin.
Sensitivity analysis
We performed sensitivity analyses by excluding either non-randomized studies, small-sample-sized studies, or all single studies. Through heterogeneity among studies were partly reduced by excluding specific studies, a moderate or high heterogeneity remained. Moreover, the improvement of BBB scores with metformin was largely maintained (Supplementary Table 1), indicating a robust result concerning BBB scores.
Proposed therapeutic mechanisms
The proposed mechanisms behind metformin in included studies was presented in Table 6. Generally, activating adenosine monophosphate-activated protein kinase signaling, regulating mitochondrial function and relieving endoplasmic reticulum stress were showed to be highly related to the action mechanisms underlying the neuroprotective effects of metformin for experimental SCI.
Discussion
Treatments for SCI have been a research focus for a long time; however, this has produced few effective therapies. Therefore, it is of great importance that clinical translation of novel treatments is achieved. For translation to the clinic, treatments must be efficacious, safe with minor adverse effects, and a clear molecular mechanism need to be determined (Park et al., 2017). Metformin is a promising candidate for SCI treatment; therefore, we conducted a systematic review of all available studies to evaluate its potential for clinical translation.
Summary of evidence
This review identified 12 eligible studies that compared metformin with placebo controls in SCI models. Pairwise meta-analyses indicated increased BBB scores in the metformin group, and this effect tended to increase over time. There were no differences in locomotor recovery with respect to gender, compression or contusion injury, administration timing of metformin or number of injections; however, there were differences related to the administration dose. Network meta-analysis confirmed the candidate dose with the best neuroprotective effects.
Meta-analysis also revealed that the WMDs of the maximal angle maintained on the inclined plane showed a gradually increasing trend (from 1.00° on the 3rd day to 7.95° on the 28th day) between metformin and control groups. Compared with controls, metformin-treated animals exhibited a higher percentage of preserved tissue at injury sites. Additionally, an increased number of motor neurons in anterior horn area of the spinal cord was observed in metformin-treated rats vs. controls.
The proposed mechanisms in included studies implied that metformin could modulate the AMPK and mTORC1 signaling, ameliorate endoplasmic reticulum stress and regulate the oxidative stress, thereby exerting a satisfactory neuroprotective role in SCI rat.
The quality of included studies was the basis for the meta-analysis. Although most of included studies had moderate-to-high methodological quality, the quality of evidence from those studies was not high. The above results need to be interpreted with caution given those limitations.
Statistical heterogeneity
Substantial between-study heterogeneity on treatment effect were observed in our analyses, which may become the barrier to conduct the meaningful meta-analysis and conclude the correct decision. There are several variables concerning used animal, injury type, injury degree, administration details, random performance, and result tendency. In addition, though only data at the same time points were used in the analyses for BBB scores, the understanding of assessor for this indicator varies from each other. All of those factors may contribute to the heterogeneity among studies. Here, subgroup analyses and sensitive analyses targeted potential factors were employed for answering the heterogeneous reasons. In our study, subgroup analyses concerning variables rat gender, administration dose or number of injections all reduced the result heterogeneity to a certain degree. The Cochrane's I2 in subgroup of single injection ranged from 0 to 23%, and subgroup analyses regarding different metformin doses regulated the heterogeneities in both subgroups. Lin et al. (2015) showed a negative effect of metformin for SCI, which may be related to the application of metformin at a high dose. Our sensitivity analyses from day 7 to 28 after SCI stably suggested a minimum heterogeneity after excluding Lin et al. (2015) compared with excluding other studies. Importantly, SYRCLE's RoB tools revealed a potential bias in methodological quality, especially in the design of random and allocation concealment. Those bias may contribute to a heterogeneous classification between the studies and lead to the treatment effect heterogeneity. In summary, multiple variables among included studies may be associated with the presented heterogeneity; different administration details, distinct results, and varied methodological quality of studies appear to be the important factors increasing the heterogeneity in our meta-analyses. The subgroup analysis under subgroups to control multiple variables is the feasible measure for reducing heterogeneity and explore the heterogeneity sources, while this type of analysis is difficult to be conducted in our study due to the insufficiency in included studies.
Metformin dose
The efficacy of metformin decreases at both very low and high doses (Riddle, 2000). One included study reported that injured rats administrated with 320 mg/kg metformin did not show improvement in motor function or neuropathic pain (Lin et al., 2015). Afshari et al. (2018) indicated that metformin at 50 mg/kg had the highest effect on promoting locomotor recovery and alleviating SCI complications compared with 10 and 100 mg/kg doses. Another study showed higher efficacy in attenuating the inflammatory response with a metformin dose of 50 mg/kg vs. high doses of 100 and 200 mg/kg (Araújo et al., 2017). Consistently, our subgroup analyses demonstrated a superior locomotor recovery in administration of metformin at ≤ 50 mg/kg. Further network meta-analysis suggested that 50 mg/kg metformin may provide better neuroprotection than other doses of metformin. Equivalent dose calculation indicates that 50 mg/kg metformin in rodents is below the therapeutic dose used for diabetes treatment in humans (Sanchez-Rangel and Inzucchi, 2017), indicating that dose titration of metformin needs to be considered for human SCI trials.
The decreased efficacy of metformin at high doses on SCI may be related to its role on metabolism. Metformin does not significantly impact the plasma glucose levels in healthy participants (Sambol et al., 1996). However, a rise in postprandial plasma glucose or insulin resistance were observed in SCI or brain injury, 100 mg/kg metformin obviously lowered the plasma glucose to a physiological level in rats subjected to those diseases (Elder et al., 2004; Zhang et al., 2022). The transport of glucose from the periphery to lesion site is reported to be diminished post-SCI (Jaiswal et al., 2022), thus administration of metformin at dose of ≥100 mg/kg may further deteriorate the glucose uptake impairment in vulnerable lesion site. Given the critical role of glucose metabolism for CNS developing and homeostasis (Veys et al., 2020), this negative impact of high doses of metformin seems be detrimental for recovery of SCI. Secondly, in the internal environment of CNS, metformin was suggested to accelerate consumption of extracellular glucose (Westhaus et al., 2017; Blumrich and Dringen, 2019). While, due to its inhibition role on mitochondrial respiration chain, metformin at a high dose (comparable to 140 mg/kg in rats) strongly elevated glycolysis (Li et al., 2019). Consequently, the ATP synthesis pathway is disrupted and lactate largely accumulates in cells (Blumrich and Dringen, 2019; Li et al., 2019), which is detrimental for neuroregeneration and may cause the further damage of neurol cells in spinal cord (Ohnishi et al., 2021). Collectively, metformin at a high dose may notably impact the glucose uptake of CNS, dimmish the ATP synthesis in CNS and lead to the lactate accumulation in neurol cells, thereby neutralizing its neuroprotective effects. However, the specific dose at which metformin obviously abolishes its neuroprotection in SCI remain obscure and may warrant the further investigation.
Timing of metformin administration
The progress of pathology following SCI has a distinct timeframe (Tran et al., 2018). Most included studies performed the design of administrating metformin immediately or from the 1st day following SCI. Our subgroup analyses indicated the neurological improvement did not differ between administrating metformin immediately and from the 1st day following SCI. However, due to those reported administration timing all located in the acute phase of SCI, this result does not mean delayed administration shows no impact the efficacy of metformin. Early treatment of secondary damage is critical for neurological recovery in SCI participants (Badhiwala et al., 2019). Consistently, in a study in which metformin was administrated 1 week after SCI, no significant difference in locomotor function was observed between metformin and placebo treatment (Lin et al., 2015). Meanwhile, the action of metformin against SCI was shown to partly depend on its modulation of mTORC1 activation, oxidative stress and the inflammatory response, which is usually triggered a few hours after trauma to the spinal cord (Anjum et al., 2020; Wang et al., 2020). Therefore, early metformin administration needs to be considered although clinical interventions applied within a few hours after SCI are not practical.
Pharmacokinetics of metformin
Accumulation in the CNS by crossing the blood–brain barrier is powerful evidence for a drug's potential effect on a CNS disease. Pharmacokinetic detection suggested oral administration of metformin can rapidly lead to its accumulation in brain tissues (Hong et al., 2019), and rats under inflammatory conditions exhibited brain region-specific differences in metformin distribution compared with normal rats (łabuzek et al., 2010). Meanwhile, łabuzek et al. (2010) reported a higher metformin concentration in cerebrospinal fluid compared with that in plasma 6 h after administration. The pharmacokinetic parameters of metformin have also been established in healthy, young and old individuals, pregnant women, and in patients with diabetes and brain injury (Graham et al., 2011; Jang et al., 2016). Generally, metformin pharmacokinetics were closely related to the conditions of subjects (Graham et al., 2011). A decrease in maximum concentration and elimination rate constant were shown in traumatic brain injury patients vs. healthy subjects, while the apparent distribution volume and peak time displayed an opposite trend, indicating delayed metformin absorption in traumatic brain injury patients (Taheri et al., 2019). Despite an absence of metformin pharmacokinetic information in SCI, the above findings indicate that monitoring metformin plasma levels may need to be considered in human trials.
Safety
Metformin is approved by the Food and Drug Administration for type II diabetes mellitus typically, and is exploring new use in the field of obesity, cancer and aging (Ayoub et al., 2020; Samaras et al., 2020; Masarwa et al., 2021). Side effects of metformin are primarily mild-to-moderate digestive disturbance, and rarely hypoglycemia, anemia or lactic acidosis (Mccreight et al., 2016). Although high doses of metformin present some toxic or adverse effects, there is a wide range between the median effective dose and the median lethal dose. For instance, in animal studies, metformin at doses of 10–200 mg/kg exert neuroprotective effects (Afshari et al., 2018; Zhao et al., 2021), even at a dose of 500 mg/kg, which mimics accumulation, the mortality rate in animals with sepsis is not aggravated (Gras et al., 2006). In human trials focused on type II diabetes mellitus, benefits were observed with as little as 500 mg/day, while only mild or moderate adverse effects were reported with the maximum recommended dose of 2,500 mg/day (Garber et al., 1997). Clearance of metformin is mainly implemented in the kidney (Lalau et al., 2018). Despite general avoidance of metformin for stage 4–5 chronic kidney disease, these patients show a relatively low occurrence of lactic acidosis or hypoglycemia, even under repeated administration of a high dose (2,000 mg/day; Tanner et al., 2019). Importantly, no events of hypoglycemia or lactic acidosis occurred in severe traumatic brain injury patients treated with 2,000 mg/day metformin (Taheri et al., 2019). However, the metformin safety specially targeted SCI patients remains to be confirmed due to the lack of related human clinical trials.
Potential therapeutic mechanisms
According to the proposed mechanisms in included studies and related evidences, we speculated the potential mechanisms of metformin, which are described as follows.
Activation of AMPK
AMPK is a well-known cellular energy sensor. Progression of SCI is accompanied by disruption of energy metabolism, excessive oxidative stress and a reactive increase of AMPK (Hu et al., 2021). Further activation of AMPK can effectively alleviate the inflammatory response and neuronal apoptosis in SCI by regulating oxidative stress, autophagy or the family of caspase proteins (Jiang et al., 2020; Zhou et al., 2020a). Metformin has been generally argued to be an activator of AMPK signaling (Apostolova et al., 2020). Interestingly, AMPK is notably activated by metformin treatment in animals with SCI, which subsequently enhances the autophagy and alleviates neuronal damage (Zhang et al., 2017a; Wang et al., 2018; Wu et al., 2021). Thus, based on the critical role of AMPK in CNS diseases, activation of AMPK may be an important mechanism of metformin for ameliorating neuronal damage and neurological function deficits.
Inhibition of mTOR complex 1
mTOR complex 1 (mTORC1) is a direct target of AMPK, and plays an important role in anabolic and catabolic processes. Insult to the spinal cord triggers mTORC1 activation in the lesion area (Kjell et al., 2014). The activation of mTORC1 in SCI and other CNS diseases contributes to microglia activation, astrogliosis and neuronal death (Nikolaeva et al., 2016; Lin et al., 2020). Suppression of mTORC1 signaling is a major pathway affected by metformin in the treatment of diabetes, cancer and cardiovascular disease (Efentakis et al., 2019). Importantly, the activity of mTOR and p70S6 kinase, which is a subunit or substrate of mTORC1, is downregulated in a rat model of SCI after metformin intervention (Wang et al., 2016; Zhang et al., 2017a; Guo et al., 2018). Taken together, these findings indicate that the action of metformin against SCI may partly depend on its negative effect on mTORC1.
Regulation of mitochondrial function
Mitochondrial disorder can lead to aberrant electron flow and a consequent increase in ROS production (Scialò et al., 2020). The generated ROS further activate the inflammasome and apoptosis signaling to induce neuroinflammation or neural damage (Wu et al., 2020). Numerous studies have suggested a specific inhibitory action of metformin on mitochondrial complex I (Apostolova et al., 2020). Metformin significantly reduces mitochondrial ROS formation by selective inhibition of the reverse electron flow through its mild inhibitory action on complex I (Batandier et al., 2006). Interestingly, improved mitochondrial function and reduced oxidative stress was exhibited in SCI rats after treatment by metformin (Wang et al., 2020), indicating involvement of a mitochondrial mechanism in metformin neuroprotection after SCI.
Alleviating endoplasmic reticulum stress
Finally, metformin can ameliorate endoplasmic reticulum stress and the unfolded protein response in SCI through modulation of CCAAT/enhancer-binding protein homologous protein or X-box binding protein 1 signaling (Guo et al., 2019). Suppression of the pathways involved in endoplasmic reticulum stress was suggested to promote neuronal survival in CNS diseases (Zhong et al., 2020; Chuan et al., 2021). Therefore, we speculate that alleviating endoplasmic reticulum stress represents another potential mechanism by which metformin protects the neurons in lesion area.
In addition to the mechanisms mentioned, metformin also acts on TET2- FoxO3a axis, Wnt/β-catenin signaling and neuroinflammation responses (Afshari et al., 2018; Zhang et al., 2020; Zhao et al., 2021). The therapeutic mechanisms by which metformin affects SCI appear to be multiple (Figure 8) and further research will clarify its actions.
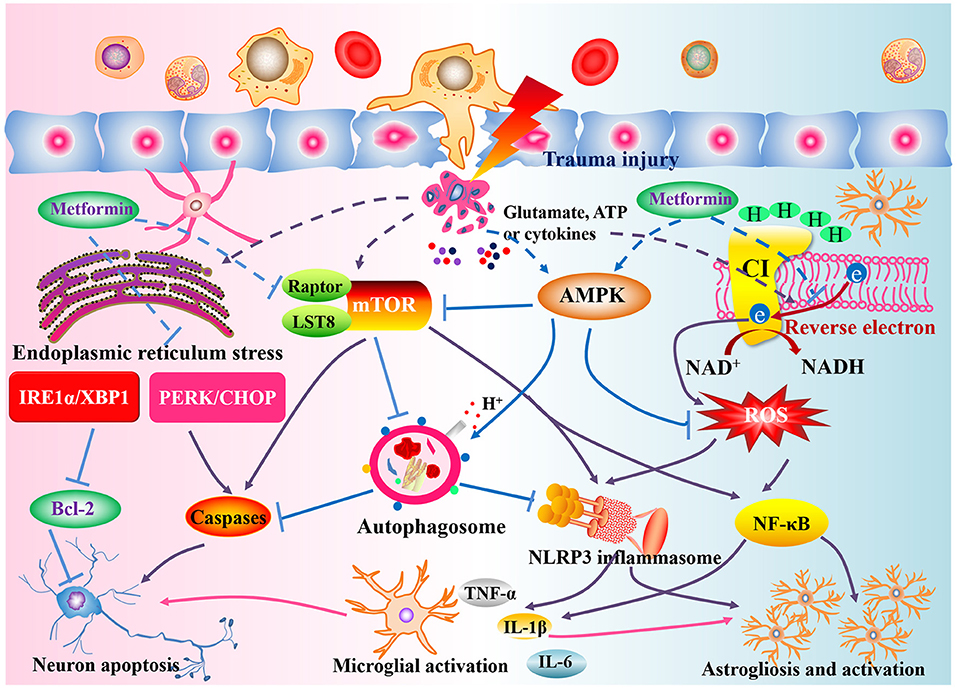
Figure 8. Potential action mechanisms of metformin against SCI. Following the primary insult, structural damage and a loss of homeostasis trigger the disturbance of energy metabolism, oxidative stress, mitochondrial disorder, endoplasmic reticulum stress, and increased mTORC1 expression. Metformin, the activator of AMPK and well-antioxidant, can modulate the AMPK and reverse electron flow in mitochondria to suppress the ROS generation and activation of NLRP3 inflammasome or NF-κB signaling, ameliorate endoplasmic reticulum stress to regulate the Bcl-2 and caspase-3, attenuate mTORC1 activation to induce the autophagosome formation, thereby exerting a satisfactory neuroprotective role in SCI rat.
Strengths and limitations
Chen et al. have performed the first meta-analysis to evaluate the efficacy of metformin on rats with SCI, revealing a potential therapeutic method for this disease. While, understanding of efficacy, dose and timing of administration, safety, and pharmacological mechanism is critical for effective clinical translation. Here, we employed a combination of updated systematic and traditional review to comprehensively analyze all of these parameters. The findings indicate that metformin warrants further investigation with respect to SCI intervention. Greater knowledge of metformin will facilitate research into SCI pathology and promote investigation of new drugs to achieve clinical translation.
It is important to note that there are several limitations of our study. Firstly, substantial heterogeneity in treatment effects between the studies was presented in our review. The heterogeneity in our study seems stubborn and complicated, which may be a barrier to meaningful analysis. While, the robust results in the sensitivity analyses, dynamic changes of locomotor function and nearly consistent result tendency among studies enhance the strength of evidence regarding metformin efficacy. Secondly, most of our findings were based on the analysis of BBB scores. This scale is a well-documented tool, while the accuracy of this outcome largely depends on assessments of locomotion that are made subjectively. The possibility of subjective bias in included studies may cause misinterpretation of metformin effects. Thirdly, although we applied an extensive search strategy, the risk of missing potentially relevant articles remains because we did not have access to all possible databases and only searched for studies in English or Chinese. Additionally, subgroup analysis concerning injury level or rat strains was not performed because of the inconsistency of these factors in our included studies.
Conclusion
In summary, our present review shows that metformin can promote motor recovery and attenuate tissue damage in SCI. Metformin shows satisfactory safety in animal studies of SCI and human trials, with a potential mechanism of action. Hence, we suggest that metformin is suitable for further confirmation in clinical trials, which may result in a new clinical therapy for SCI. Nonetheless, to move toward the clinical trials, extensive pre-clinical studies must be performed to understand the mechanism of metformin. Besides, in light of the limitations of methodological and evidence quality within included studies, the findings of this pre-clinical review should be interpreted with caution.
Data availability statement
The original contributions presented in the study are included in the article/Supplementary material, further inquiries can be directed to the corresponding author/s.
Author contributions
Review concept and design and manuscript revision: MY and XL. Electronic and manual literature searches: L-yZ, X-qC, M-xP, and LF. Study selection and data extraction, quality control, and external adviser: L-yZ, X-qC, B-bY, JL, X-jC, and MY. Review analysis: L-yZ, X-qC, and XL. Initial draft writing: L-yZ, X-qC, and B-bY. All authors contributed to the article and approved the submitted version.
Funding
This study was supported by the National Natural Science Foundation of China (81804152, 82072546, 82074454, and 82174409).
Acknowledgments
We thank Jeremy Allen, Ph.D., from Liwen Bianji (Edanz) (www.liwenbianji.cn), for editing a draft of this manuscript.
Conflict of interest
The authors declare that the research was conducted in the absence of any commercial or financial relationships that could be construed as a potential conflict of interest.
Publisher's note
All claims expressed in this article are solely those of the authors and do not necessarily represent those of their affiliated organizations, or those of the publisher, the editors and the reviewers. Any product that may be evaluated in this article, or claim that may be made by its manufacturer, is not guaranteed or endorsed by the publisher.
Supplementary material
The Supplementary Material for this article can be found online at: https://www.frontiersin.org/articles/10.3389/fnins.2022.946879/full#supplementary-material
References
Afshari, K., Dehdashtian, A., Haddadi, N. S., Haj-Mirzaian, A., Iranmehr, A., Ebrahimi, M. A., et al. (2018). Anti-inflammatory effects of Metformin improve the neuropathic pain and locomotor activity in spinal cord injured rats: introduction of an alternative therapy. Spinal Cord 56, 1032–1041. doi: 10.1038/s41393-018-0168-x
Anjum, A., Yazid, M. D. I., Fauzi Daud, M., Idris, J., Ng, A. M. H., Selvi Naicker, A., et al. (2020). Spinal cord injury: pathophysiology, multimolecular interactions, and underlying recovery mechanisms. Int. J. Mol. Sci. 21:7533. doi: 10.3390/ijms21207533
Apostolova, N., Iannantuoni, F., Gruevska, A., Muntane, J., Rocha, M., and Victor, V. M. (2020). Mechanisms of action of metformin in type 2 diabetes: effects on mitochondria and leukocyte-endothelium interactions. Redox Biol. 34:101517. doi: 10.1016/j.redox.2020.101517
Araújo, A. A. D., Pereira, A. D. S. B., Medeiros, C. A. C. X., Brito, G. A. D. C., Leitão, R. F. D. C., Araújo, L. D. S., et al. (2017). Effects of metformin on inflammation, oxidative stress, and bone loss in a rat model of periodontitis. PLoS ONE 12:e183506. doi: 10.1371/journal.pone.0183506
Ayoub, R., Ruddy, R. M., Cox, E., Oyefiade, A., Derkach, D., Laughlin, S., et al. (2020). Assessment of cognitive and neural recovery in survivors of pediatric brain tumors in a pilot clinical trial using metformin. Nat. Med. 26, 1285–1294. doi: 10.1038/s41591-020-0985-2
Badhiwala, J. H., Ahuja, C. S., and Fehlings, M. G. (2019). Time is spine: a review of translational advances in spinal cord injury. J. Neurosurg. 30, 1–18. doi: 10.3171/2018.9.SPINE18682
Basso, D. M., Beattie, M. S., and Bresnahan, J. C. (1995). A sensitive and reliable locomotor rating scale for open field testing in rats. J. Neurotrauma 12, 1–21. doi: 10.1089/neu.1995.12.1
Batandier, C., Guigas, B., Detaille, D., El-Mir, M., Fontaine, E., Rigoulet, M., et al. (2006). The ROS production induced by a reverse-electron flux at respiratory-chain complex 1 is hampered by metformin. J. Bioenerg. Biomembr. 38, 33–42. doi: 10.1007/s10863-006-9003-8
Blumrich, E., and Dringen, R. (2019). Metformin accelerates glycolytic lactate production in cultured primary cerebellar granule neurons. Neurochem. Res. 44, 188–199. doi: 10.1007/s11064-017-2346-1
Charalambous, M., Brodbelt, D., and Volk, H. A. (2014). Treatment in canine epilepsy-a systematic review. BMC Vet Res. 10:257. doi: 10.1186/s12917-014-0257-9
Charalambous, M., Shivapour, S. K., Brodbelt, D. C., and Volk, H. A. (2016). Antiepileptic drugs' tolerability and safety-a systematic review and meta-analysis of adverse effects in dogs. BMC Vet. Res. 12:79. doi: 10.1186/s12917-016-0703-y
Chen, Q., Xie, D., Yao, Q., and Yang, L. (2021). Effect of metformin on locomotor function recovery in rat spinal cord injury model: a meta-analysis. Oxid. Med. Cell. Longev. 2021:8302831. doi: 10.1155/2021/8302831
Chen, S., Ye, J., Chen, X., Shi, J., Wu, W., Lin, W., et al. (2018). Valproic acid attenuates traumatic spinal cord injury-induced inflammation via STAT1 and NF-κB pathway dependent of HDAC3. J. Neuroinflamm. 15:150. doi: 10.1186/s12974-018-1193-6
Chiocchia, V., Nikolakopoulou, A., Papakonstantinou, T., Egger, M., and Salanti, G. (2020). Agreement between ranking metrics in network meta-analysis: an empirical study. BMJ Open 10:e37744. doi: 10.1136/bmjopen-2020-037744
Chuan, L., Huang, X., Fan, C., Wen, S., Yang, X., Wang, J., et al. (2021). Metformin ameliorates brain damage caused by cardiopulmonary resuscitation via targeting endoplasmic reticulum stress-related proteins GRP78 and XBP1. Eur. J. Pharmacol. 891:173716. doi: 10.1016/j.ejphar.2020.173716
Dietz, V., and Curt, A. (2006). Neurological aspects of spinal-cord repair: promises and challenges. Lancet Neurol. 5, 688–694. doi: 10.1016/S1474-4422(06)70522-1
Efentakis, P., Kremastiotis, G., Varela, A., Nikolaou, P., Papanagnou, E., Davos, C. H., et al. (2019). Molecular mechanisms of carfilzomib-induced cardiotoxicity in mice and the emerging cardioprotective role of metformin. Blood 133, 710–723. doi: 10.1182/blood-2018-06-858415
Elder, C. P., Apple, D. F., Bickel, C. S., Meyer, R. A., and Dudley, G. A. (2004). Intramuscular fat and glucose tolerance after spinal cord injury–a cross-sectional study. Spinal Cord 42, 711–716. doi: 10.1038/sj.sc.3101652
Feigin, V. L., Vos, T., Alahdab, F., Amit, A. M. L., Bärnighausen, T. W., Beghi, E., et al. (2021). Burden of neurological disorders across the US from 1990-2017. JAMA Neurol. 78:165. doi: 10.1001/jamaneurol.2020.4152
Garber, A. J., Duncan, T. G., Goodman, A. M., Mills, D. J., and Rohlf, J. L. (1997). Efficacy of metformin in type II diabetes: results of a double-blind, placebo-controlled, dose-response trial. Am. J. Med. 103, 491–497. doi: 10.1016/S0002-9343(97)00254-4
Gbd, N. C.. (2019). Global, regional, and national burden of neurological disorders, 1990-2016: a systematic analysis for the Global Burden of Disease Study 2016. Lancet Neurol. 18, 459–480. doi: 10.1016/S1474-4422(18)30499-X
Graham, G. G., Punt, J., Arora, M., Day, R. O., Doogue, M. P., Duong, J. K., et al. (2011). Clinical pharmacokinetics of metformin. Clin. Pharmacokinet. 50, 81–98. doi: 10.2165/11534750-000000000-00000
Gras, V., Bouffandeau, B., Montravers, P. H., and Lalau, J. D. (2006). Effect of metformin on survival rate in experimental sepsis. Diabetes Metab. 32, 147–150. doi: 10.1016/S1262-3636(07)70261-6
Guo, W. D., Li, G., and Fan, Z. K. (2019). Effects of metformin on endoplasmic reticulum stress and apoptosis after spinal cord injury in rats. Chinese J. Anat. 42, 161–166. doi: 10.3969/j.issn.1001-1633.2019.02.012
Guo, Y., Wang, F., Li, H., Liang, H., Li, Y., Gao, Z., et al. (2018). Metformin protects against spinal cord injury by regulating autophagy via the mTOR signaling pathway. Neurochem. Res. 43, 1111–1117. doi: 10.1007/s11064-018-2525-8
Higgins, J., and Green, S. (2011). Cochrane Handbook for Systematic Reviews of Interventions Version 5.1.0. The Cochrane Collaboration. Available online at: www.handbook.cochrane.org.
Hong, J., Chang, A., Zavvarian, M. M., Wang, J., Liu, Y., and Fehlings, M. G. (2018). Level-Specific differences in systemic expression of pro- and Anti-Inflammatory cytokines and chemokines after spinal cord injury. Int. J. Mol. Sci. 19:2167. doi: 10.3390/ijms19082167
Hong, L., Li, X., Bao, Y., Duvall, C. L., Zhang, C., Chen, W., et al. (2019). Preparation, preliminary pharmacokinetic and brain targeting study of metformin encapsulated W/O/W composite submicron emulsions promoted by borneol. Eur. J. Pharm. Sci. 133, 160–166. doi: 10.1016/j.ejps.2019.03.019
Hooijmans, C. R., Rovers, M. M., de Vries, R. B., Leenaars, M., Ritskes-Hoitinga, M., and Langendam, M. W. (2014). SYRCLE's risk of bias tool for animal studies. BMC Med. Res. Methodol. 14:43. doi: 10.1186/1471-2288-14-43
Hu, H., Xia, N., Lin, J., Li, D., Zhang, C., Ge, M., et al. (2021). Zinc regulates glucose metabolism of the spinal cord and neurons and promotes functional recovery after spinal cord injury through the AMPK signaling pathway. Oxid. Med. Cell. Longev. 2021:4331625. doi: 10.1155/2021/4331625
Jaiswal, S., Brabazon, F., von Leden, R., Acs, D., Collier, S., Allison, N., et al. (2022). Spinal cord injury chronically depresses glucose uptake in the rodent model. Neurosci. Lett. 771:136416. doi: 10.1016/j.neulet.2021.136416
Jang, K., Chung, H., Yoon, J., Moon, S., Yoon, S. H., Yu, K., et al. (2016). Pharmacokinetics, safety, and tolerability of metformin in healthy elderly subjects. J. Clin. Pharmacol. 56, 1104–1110. doi: 10.1002/jcph.699
Jiang, X., Shen, Z., Chen, J., Wang, C., Gao, Z., Yu, S., et al. (2020). Irisin protects against motor dysfunction of rats with spinal cord injury via adenosine 5'-Monophosphate (AMP)-Activated protein Kinase-Nuclear factor Kappa-B pathway. Front. Pharmacol. 11:582484. doi: 10.3389/fphar.2020.582484
Khatri, N., Thakur, M., Pareek, V., Kumar, S., Sharma, S., and Datusalia, A. K. (2018). Oxidative stress: major threat in traumatic brain injury. CNS Neurol. Disord. Drug Targets 17, 689–695. doi: 10.2174/1871527317666180627120501
Kjell, J., Codeluppi, S., Josephson, A., and Abrams, M. B. (2014). Spatial and cellular characterization of mTORC1 activation after spinal cord injury reveals biphasic increase mainly attributed to microglia/macrophages. Brain Pathol. 24, 557–567. doi: 10.1111/bpa.12135
Kumar, R., Lim, J., Mekary, R. A., Rattani, A., Dewan, M. C., Sharif, S. Y., et al. (2018). Traumatic spinal injury: global epidemiology and worldwide volume. World Neurosurg. 113, e345–e363. doi: 10.1016/j.wneu.2018.02.033
łabuzek, K., Suchy, D., Gabryel, B., Bielecka, A., Liber, S., and Okopień, B. (2010). Quantification of metformin by the HPLC method in brain regions, cerebrospinal fluid and plasma of rats treated with lipopolysaccharide. Pharmacol. Rep. 62, 956–965. doi: 10.1016/S1734-1140(10)70357-1
Lalau, J. D., Kajbaf, F., Bennis, Y., Hurtel-Lemaire, A. S., Belpaire, F., and De Broe, M. E. (2018). Metformin treatment in patients with type 2 diabetes and chronic kidney disease stages 3A, 3B, or 4. Diabetes Care 41, 547–553. doi: 10.2337/dc17-2231
Leenaars, M., Hooijmans, C. R., van Veggel, N., ter Riet, G., Leeflang, M., Hooft, L., et al. (2012). A step-by-step guide to systematically identify all relevant animal studies. Lab. Anim. 46, 24–31. doi: 10.1258/la.2011.011087
Li, G., Shen, F., Fan, Z., Wang, Y., Kong, X., Yu, D., et al. (2017). Dynasore improves motor function recovery via inhibition of neuronal apoptosis and astrocytic proliferation after spinal cord injury in rats. Mol. Neurobiol. 54, 7471–7482. doi: 10.1007/s12035-016-0252-1
Li, W., Chaudhari, K., Shetty, R., Winters, A., Gao, X., Hu, Z., et al. (2019). Metformin alters locomotor and cognitive function and brain metabolism in normoglycemic mice. Aging Dis. 10:949. doi: 10.14336/AD.2019.0120
Lin, C. M., Tsai, J. T., Chang, C. K., Cheng, J. T., and Lin, J. W. (2015). Development of telmisartan in the therapy of spinal cord injury: pre-clinical study in rats. Drug Des. Devel. Ther. 9, 4709–4717. doi: 10.2147/DDDT.S86616
Lin, J., Pan, X., Huang, C., Gu, M., Chen, X., Zheng, X., et al. (2020). Dual regulation of microglia and neurons by Astragaloside IV-mediated mTORC1 suppression promotes functional recovery after acute spinal cord injury. J. Cell. Mol. Med. 24, 671–685. doi: 10.1111/jcmm.14776
Lin, M., Fang, S., Hsu, J. C., Huang, C., Lee, P., Huang, C., et al. (2022). Mitochondrial transplantation attenuates neural damage and improves locomotor function after traumatic spinal cord injury in rats. Front. Neurosci. 16:800883. doi: 10.3389/fnins.2022.800883
Lucchesi, L. R., Agrawal, S., Ahmadi, A., Aichour, A. N., Altirkawi, K., Ariani, F., et al. (2019). Global, regional, and national burden of traumatic brain injury and spinal cord injury, 1990-2016: a systematic analysis for the Global Burden of Disease Study 2016. Lancet Neurol. 18, 56–87. doi: 10.1016/S1474-4422(18)30415-0
Luo, F., Das, A., Chen, J., Wu, P., Li, X., and Fang, Z. (2019). Metformin in patients with and without diabetes: a paradigm shift in cardiovascular disease management. Cardiovasc. Diabetol. 18:54. doi: 10.1186/s12933-019-0860-y
Ma, R., Yi, B., Riker, A. I., and Xi, Y. (2020). Metformin and cancer immunity. Acta Pharmacol. Sin. 41, 1403–1409. doi: 10.1038/s41401-020-00508-0
Masarwa, R., Brunetti, V. C., Aloe, S., Henderson, M., Platt, R. W., and Filion, K. B. (2021). Efficacy and safety of metformin for obesity: a systematic review. Pediatrics 147:e20201610. doi: 10.1542/peds.2020-1610
Mbuagbaw, L., Rochwerg, B., Jaeschke, R., Heels-Andsell, D., Alhazzani, W., Thabane, L., et al. (2017). Approaches to interpreting and choosing the best treatments in network meta-analyses. Syst. Rev. 6:79. doi: 10.1186/s13643-017-0473-z
Mccreight, L. J., Bailey, C. J., and Pearson, E. R. (2016). Metformin and the gastrointestinal tract. Diabetologia 59, 426–435. doi: 10.1007/s00125-015-3844-9
Nikolaeva, I., Crowell, B., Valenziano, J., Meaney, D., and D'Arcangelo, G. (2016). Beneficial effects of early mTORC1 inhibition after traumatic brain injury. J. Neurotrauma 33, 183–193. doi: 10.1089/neu.2015.3899
Ohnishi, Y., Yamamoto, M., Sugiura, Y., Setoyama, D., and Kishima, H. (2021). Rostro-caudal different energy metabolism leading to differences in degeneration in spinal cord injury. Brain Commun. 3:fcab058. doi: 10.1093/braincomms/fcab058
Oliveri, R. S., Bello, S., and Biering-Sørensen, F. (2014). Mesenchymal stem cells improve locomotor recovery in traumatic spinal cord injury: systematic review with meta-analyses of rat models. Neurobiol. Dis. 62, 338–353. doi: 10.1016/j.nbd.2013.10.014
Owen, P. J., Miller, C. T., Mundell, N. L., Verswijveren, S. J. J. M., Tagliaferri, S. D., Brisby, H., et al. (2020). Which specific modes of exercise training are most effective for treating low back pain? Network meta-analysis. Brit. J. Sport. Med. 54, 1279–1287. doi: 10.1136/bjsports-2019-100886
Park, Y. B., Ha, C. W., Lee, C. H., Yoon, Y. C., and Park, Y. G. (2017). Cartilage regeneration in osteoarthritic patients by a composite of allogeneic umbilical cord Blood-Derived mesenchymal stem cells and hyaluronate hydrogel: results from a clinical trial for safety and Proof-of-Concept with 7 years of extended Follow-Up. Stem Cells Transl. Med. 6, 613–621. doi: 10.5966/sctm.2016-0157
Riddle, M.. (2000). Combining sulfonylureas and other oral agents. Am. J. Med. 108, 15–22. doi: 10.1016/S0002-9343(00)00338-7
Rivlin, A. S., and Tator, C. H. (1978). Effect of duration of acute spinal cord compression in a new acute cord injury model in the rat. Surg Neurol. 10, 38–43.
Samaras, K., Makkar, S., Crawford, J. D., Kochan, N. A., Wen, W., Draper, B., et al. (2020). Metformin use is associated with slowed cognitive decline and reduced incident dementia in older adults with type 2 diabetes: the sydney memory and ageing study. Diabetes Care 43, 2691–2701. doi: 10.2337/dc20-0892
Sambol, N. C., Chiang, J., O'Conner, M., Liu, C. Y., Lin, E. T., Goodman, A. M., et al. (1996). Pharmacokinetics and pharmacodynamics of metformin in healthy subjects and patients with noninsulin-dependent diabetes mellitus. J. Clin. Pharmacol. 36, 1012–1021. doi: 10.1177/009127009603601105
Sanchez-Rangel, E., and Inzucchi, S. E. (2017). Metformin: clinical use in type 2 diabetes. Diabetologia 60, 1586–1593. doi: 10.1007/s00125-017-4336-x
Sarkar, S., Malovic, E., Harishchandra, D. S., Ghaisas, S., Panicker, N., Charli, A., et al. (2017). Mitochondrial impairment in microglia amplifies NLRP3 inflammasome proinflammatory signaling in cell culture and animal models of Parkinson's disease. NPJ Parkinson's Dis. 3, 15–30. doi: 10.1038/s41531-017-0032-2
Scialò, F., Sriram, A., Stefanatos, R., Spriggs, R. V., Loh, S. H. Y., Martins, L. M., et al. (2020). Mitochondrial complex I derived ROS regulate stress adaptation in Drosophila melanogaster. Redox Biol. 32:101450. doi: 10.1016/j.redox.2020.101450
Sng, K. S., Li, G., Zhou, L. Y., Song, Y. J., Chen, X. Q., Wang, Y. J., et al. (2022). Ginseng extract and ginsenosides improve neurological function and promote antioxidant effects in rats with spinal cord injury: a meta-analysis and systematic review. J. Ginseng Res. 46, 11–22. doi: 10.1016/j.jgr.2021.05.009
Taheri, A., Emami, M., Asadipour, E., Kasirzadeh, S., Rouini, M., Najafi, A., et al. (2019). A randomized controlled trial on the efficacy, safety, and pharmacokinetics of metformin in severe traumatic brain injury. J. Neurol. 266, 1988–1997. doi: 10.1007/s00415-019-09366-1
Tanner, C., Wang, G., Liu, N., Andrikopoulos, S., Zajac, J. D., and Ekinci, E. I. (2019). Metformin: time to review its role and safety in chronic kidney disease. Med. J. Austr. 211, 37–42. doi: 10.5694/mja2.50239
Tian, Z. R., Yao, M., Zhou, L. Y., Song, Y. J., Ye, J., Wang, Y. J., et al. (2020). Effect of docosahexaenoic acid on the recovery of motor function in rats with spinal cord injury: a meta-analysis. Neural Regen. Res. 15, 537–547. doi: 10.4103/1673-5374.266065
Tran, A. P., Warren, P. M., and Silver, J. (2018). The biology of regeneration failure and success after spinal cord injury. Physiol. Rev. 98, 881–917. doi: 10.1152/physrev.00017.2017
Veys, K., Fan, Z., Ghobrial, M., Bouché, A., García-Caballero, M., Vriens, K., et al. (2020). Role of the GLUT1 glucose transporter in postnatal CNS angiogenesis and Blood-Brain barrier integrity. Circ. Res. 127, 466–482. doi: 10.1161/CIRCRESAHA.119.316463
Visavadiya, N. P., Patel, S. P., Vanrooyen, J. L., Sullivan, P. G., and Rabchevsky, A. G. (2016). Cellular and subcellular oxidative stress parameters following severe spinal cord injury. Redox Biol. 8, 59–67. doi: 10.1016/j.redox.2015.12.011
Wang, C., Liu, C., Gao, K., Zhao, H., Zhou, Z., Shen, Z., et al. (2016). Metformin preconditioning provide neuroprotection through enhancement of autophagy and suppression of inflammation and apoptosis after spinal cord injury. Biochem. Biophys. Res. Commun. 477, 534–540. doi: 10.1016/j.bbrc.2016.05.148
Wang, H., Zheng, Z., Han, W., Yuan, Y., Li, Y., Zhou, K., et al. (2020). Metformin promotes axon regeneration after spinal cord injury through inhibiting oxidative stress and stabilizing microtubule. Oxid. Med. Cell. Longev. 2020:9741369. doi: 10.1155/2020/9741369
Wang, P., Xie, Z. D., Xie, C. N., Lin, C. W., Wang, J. L., Xuan, L. N., et al. (2018). AMP-activated protein kinase-dependent induction of autophagy by erythropoietin protects against spinal cord injury in rats. CNS Neurosci. Ther. 24, 1185–1195. doi: 10.1111/cns.12856
Westhaus, A., Blumrich, E. M., and Dringen, R. (2017). The antidiabetic drug metformin stimulates glycolytic lactate production in cultured primary rat astrocytes. Neurochem. Res. 42, 294–305. doi: 10.1007/s11064-015-1733-8
Wilcox, J. T., Satkunendrarajah, K., Nasirzadeh, Y., Laliberte, A. M., Lip, A., Cadotte, D. W., et al. (2017). Generating level-dependent models of cervical and thoracic spinal cord injury: exploring the interplay of neuroanatomy, physiology, and function. Neurobiol. Dis. 105, 194–212. doi: 10.1016/j.nbd.2017.05.009
Wu, X., Luo, J., Liu, H., Cui, W., Guo, K., Zhao, L., et al. (2020). Recombinant adiponectin peptide ameliorates brain injury following intracerebral hemorrhage by suppressing Astrocyte-Derived inflammation via the inhibition of Drp1-Mediated mitochondrial fission. Transl. Stroke Res. 11, 924–939. doi: 10.1007/s12975-019-00768-x
Wu, Y. Q., Xiong, J., He, Z. L., Yuan, Y., Wang, B. N., Xu, J. Y., et al. (2021). Metformin promotes microglial cells to facilitate myelin debris clearance and accelerate nerve repairment after spinal cord injury. Acta Pharmacol. Sin. 43, 1360–1371. doi: 10.1038/s41401-021-00759-5
Xu, B., Yao, M., Li, Z., Tian, Z., Ye, J., Wang, Y., et al. (2020). Neurological recovery and antioxidant effects of resveratrol in rats with spinal cord injury: a meta-analysis. Neural Regen. Res. 15, 482–490. doi: 10.4103/1673-5374.266064
Yao, M., Yang, L., Wang, J., Sun, Y., Dun, R., Wang, Y., et al. (2015). Neurological recovery and antioxidant effects of curcumin for spinal cord injury in the rat: a network Meta-Analysis and systematic review. J. Neurotraum. 32, 381–391. doi: 10.1089/neu.2014.3520
Zhang, D.. (2017). Metformin Improves Functional Recovery After Spinal Cord Injury via Autophagy Flux Stimulation. Wenzhou Medical University.
Zhang, D., Tang, Q., Zheng, G., Wang, C., Zhou, Y., Wu, Y., et al. (2017b). Metformin ameliorates BSCB disruption by inhibiting neutrophil infiltration and MMP-9 expression but not direct TJ proteins expression regulation. J. Cell. Mol. Med. 21, 3322–3336. doi: 10.1111/jcmm.13235
Zhang, D., Xuan, J., Zheng, B. B., Zhou, Y. L., Lin, Y., Wu, Y. S., et al. (2017a). Metformin improves functional recovery after spinal cord injury via autophagy flux stimulation. Mol. Neurobiol. 54, 3327–3341. doi: 10.1007/s12035-016-9895-1
Zhang, T., Wang, F., Li, K., Lv, C., Gao, K., and Lv, C. (2020). Therapeutic effect of metformin on inflammation and apoptosis after spinal cord injury in rats through the Wnt/β-catenin signaling pathway. Neurosci. Lett. 739:135440. doi: 10.1016/j.neulet.2020.135440
Zhang, Y., Zhang, T., Li, Y., Guo, Y., Liu, B., Tian, Y., et al. (2022). Metformin attenuates early brain injury after subarachnoid hemorrhage in rats via AMPK-dependent mitophagy. Exp. Neurol. 353:114055. doi: 10.1016/j.expneurol.2022.114055
Zhao, J. W.. (2021). Metformin Inhibits Apoptosis by Regulating Tet2-Foxo3a Pathway After Spinal Cord Injury. Dalian Medical University
Zhao, J. W., Miao, Z. G., Sun, H. H., Hu, L., Sun, H., Zhong, X. L., et al. (2021). Metformin inhibits apoptosis by regulating TET2-Foxo3a pathway after spinal cord injury. Chinese J. Orthopaed. 41, 584–594. doi: 10.3760/cma.j.cn121113-20210201-00098
Zhong, H., Yu, H., Chen, J., Sun, J., Guo, L., Huang, P., et al. (2020). Hydrogen sulfide and endoplasmic reticulum stress: a potential therapeutic target for central nervous system degeneration diseases. Front. Pharmacol. 11:702. doi: 10.3389/fphar.2020.00702
Zhou, K., Zheng, Z., Li, Y., Han, W., Zhang, J., Mao, Y., et al. (2020a). TFE3, a potential therapeutic target for Spinal Cord Injury via augmenting autophagy flux and alleviating ER stress. Theranostics 10, 9280–9302. doi: 10.7150/thno.46566
Keywords: metformin, spinal cord injury, systematic review, neurological function, safety, action mechanism, clinical translation
Citation: Zhou L-y, Chen X-q, Yu B-b, Pan M-x, Fang L, Li J, Cui X-j, Yao M and Lu X (2022) The effect of metformin on ameliorating neurological function deficits and tissue damage in rats following spinal cord injury: A systematic review and network meta-analysis. Front. Neurosci. 16:946879. doi: 10.3389/fnins.2022.946879
Received: 18 May 2022; Accepted: 19 July 2022;
Published: 11 August 2022.
Edited by:
Pradeep Kumar, University of the Witwatersrand, South AfricaReviewed by:
Yubao Lu, Third Affiliated Hospital of Sun Yat-sen University, ChinaXiaobing Jiang, Guangzhou University of Chinese Medicine, China
Copyright © 2022 Zhou, Chen, Yu, Pan, Fang, Li, Cui, Yao and Lu. This is an open-access article distributed under the terms of the Creative Commons Attribution License (CC BY). The use, distribution or reproduction in other forums is permitted, provided the original author(s) and the copyright owner(s) are credited and that the original publication in this journal is cited, in accordance with accepted academic practice. No use, distribution or reproduction is permitted which does not comply with these terms.
*Correspondence: Min Yao, eWFvbWluMTk4NzEyMjMmI3gwMDA0MDsxMjYuY29t; Xiao Lu, bHV4aWFvMTk3MiYjeDAwMDQwOzE2My5jb20=
†These authors have contributed equally to this work