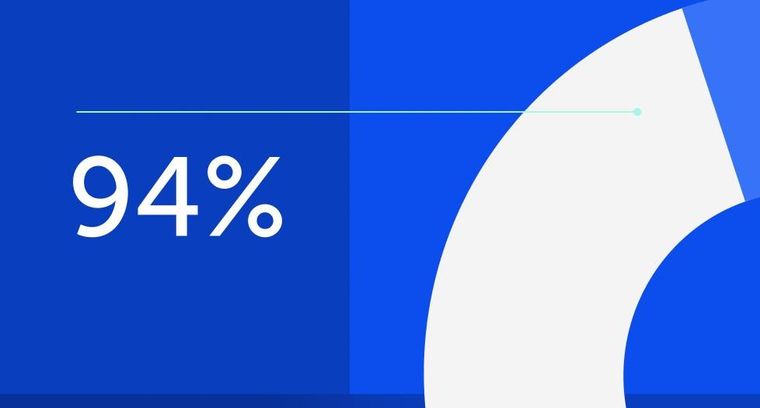
94% of researchers rate our articles as excellent or good
Learn more about the work of our research integrity team to safeguard the quality of each article we publish.
Find out more
REVIEW article
Front. Neurosci., 16 June 2022
Sec. Autonomic Neuroscience
Volume 16 - 2022 | https://doi.org/10.3389/fnins.2022.897303
This article is part of the Research TopicThe Heart-Brain Connection in Emotions, Cognition, and DementiaView all 11 articles
The vagus nerve is the longest nerve in the human body, providing afferent information about visceral sensation, integrity and somatic sensations to the CNS via brainstem nuclei to subcortical and cortical structures. Its efferent arm influences GI motility and secretion, cardiac ionotropy, chonotropy and heart rate variability, blood pressure responses, bronchoconstriction and modulates gag and cough responses via palatine and pharyngeal innervation. Vagus nerve stimulation has been utilized as a successful treatment for intractable epilepsy and treatment-resistant depression, and new non-invasive transcutaneous (t-VNS) devices offer equivalent therapeutic potential as invasive devices without the surgical risks. t-VNS offers exciting potential as a therapeutic intervention in cognitive decline and aging populations, classically affected by reduced cerebral perfusion by modulating both limbic and frontal cortical structures, regulating cerebral perfusion and improving parasympathetic modulation of the cardiovascular system. In this narrative review we summarize the research to date investigating the cognitive effects of VNS therapy, and its effects on neurocardiovascular stability.
Vagus nerve stimulation (VNS) as a neurostimulation technique and has received renewed attention in recent years. Traditionally invasive VNS (iVNS) devices were sutured under the skin of the chest with a lateral left neck dissection undertaken to expose the left cervical vagus nerve and wrap a stimulating electrode around it. Each iVNS device is costly and up to 30% of patients have side effects post implantation (Morris and Mueller, 1999). Since the development in the early 2000s of peripheral stimulating devices that harness the vagus nerve’s innervation of the skin of the external ear demonstrating efficacy in treating epilepsy, depression and headaches, interest in wider therapeutic potentials of this treatment have grown (Yap et al., 2020).
Declining cognition associated with aging is a burgeoning global health crisis, with at least 152.8 million persons projected to have dementia worldwide by the year 2050 (Nichols et al., 2022). There are few effective treatments for cognitive decline and dementia, with no current cure (Cummings et al., 2021) and although the first disease modifying anti-amyloid agent has been licensed by the FDA (Steinbrook, 2021), more therapies are urgently needed to help alleviate the personal, societal and economic cost of increasing dementia diagnoses (Xu et al., 2017). Impaired cognition is associated with impaired autonomic function, specifically impaired parasympathetic measures of heart rate variability (HRV) (Forte et al., 2019; Cheng et al., 2022; Liu et al., 2022) likely reflective of the complex interplay between cognition and cardiac modulation, via the central autonomic network.
Studies of patients with intractable epilepsy and treatment -resistant depression treated with iVNS devices showed signals indicating increased alertness and potentially cognitive improvements (Ghacibeh et al., 2006a; McGlone et al., 2008; Schevernels et al., 2016; Sun et al., 2017; van Bochove et al., 2018) and a small pilot study investigated iVNS devices in patients with Alzheimer’s Disease with overall positive results (Sjögren et al., 2002; Merrill et al., 2006). Recent meta-analysis of t-VNS in young healthy adults has found an overall moderate effect especially for improved cognitive performance especially executive function (Ridgewell et al., 2021). However the neuroanatomical substrates of persons with treatment-resistant depression or epilepsy are likely both widely variable, and grossly different to both a young cognitively healthy adult and a person with mild cognitive impairment (MCI) or dementia and dedicated larger studies are required to investigate if t-VNS has therapeutic potential in this population.
The purpose of this narrative review will be to outline the research to date investigating both cognitive outcomes of VNS in healthy and clinical populations, and the effect VNS has on HRV as a measure of autonomic tone. The mechanisms of action of VNS including neurotransmitter release, local increased cerebral blood flow and modulation of peripheral hemodynamics are discussed and future research recommendations outlined.
The longest nerve in the body, the vagus nerve derives its name from the Latin for ‘straying’ or ‘wandering.’ Aptly named, the nerve has an extensive course, traveling from the medulla to the gut. The vagus nerve’s function is to transmit information to and from the central nervous system (CNS) regarding control of the gastrointestinal, cardiovascular, and respiratory systems. It is comprised of approximately 80% afferent and 20% efferent fibers (Foley and Dubois, 1937; Agostini et al., 1957) including A, B and C fibers classified by conduction velocity (Erlanger and Gasser, 1937). Vagus neurons may involve visceral (cardiac, bronchopulmonary, gastrointestinal) or somatic (soft tissues, muscles of palate, pharynx) modulation. Afferent fibers are further sub classified as general visceral afferent, general somatic afferent, or special visceral afferent. Two efferent fiber types are recognized, namely special visceral efferent and general visceral efferent (see Table 1). Fibers connect centrally to four vagal nuclei; the nucleus of the solitary tract (NTS) and spinal trigeminal nucleus which contain vagal afferent fibers and the nucleus ambiguous and dorsal motor nucleus of the vagus (DMN) from where vagal efferent fibers leave (Rutecki, 1990; Berthoud and Neuhuber, 2000).
Afferent vagus fibers enter the medulla at the level of the olive, and terminate primarily in the NTS (Beckstead and Norgren, 1979; Kalia and Sullivan, 1982). Each vagus nerve (VN) synapses bilaterally in the NTS; so vagal afferent information is processed bilaterally in the CNS (Henry, 2002). Second order afferent fibers from the NTS project most densely to the parabrachial nucleus of the pons (PBN) with the NTS also projecting to noradrenergic (locus coeruleus) and serotonergic (raphe nuclei) neuromodulatory systems (Rutecki, 1990; Saper, 2000). From here vagal information is relayed to a number of mostly subcortical structures, including the hypothalamus, the central nucleus of the amygdala, the bed nucleus of the stria terminalis, and the intralaminar thalamic nucleus. Vagal afferent information is also sent to the anterior insular cortex which communicates with more rostral regions of the cortex (orbital and ventrolateral prefrontal cortex) and also indirectly with the medial prefrontal cortex (Öngür and Price, 2000; Saleem et al., 2008).
These central structures are part of the central autonomic network (CAN) which is thought to be the origin of autonomic, behavioral, cognitive, and endocrine responses, capable of modulating the functioning of the autonomic nervous system (ANS) via descending pathways projecting onto sympathetic pre-ganglionic neurons in the spinal cord and onto the DMN at the origin of vagal efferents (Benarroch, 1993). The central connections of the DMN are considerable, with afferent projections arising from sites including the NST, magnocellular paraventricular nuclei and several medullary nuclei (Roges et al., 1980; Hansen, 2019). Whilst a minority of efferent fibers connect centrally, most DMN fibers project to GI organs via parasympathetic ganglia located close to or in the walls of viscera. Further efferent fibers originate from the nucleus ambiguous (NA), a motor nucleus located in the reticular formation of the medulla which gives rise to preganglionic neurons innervating the heart and lungs (Llewellyn-Smith and Verberne, 2011) which exert a cardio-inhibitory effect mediated via the sinoatrial and atrioventricular ganglia (Massari et al., 1995; Gatti et al., 1996). The right vagus nerve mostly innervates the sinoatrial node (involved in the pacemaker function of the heart) whereas the left vagus is mostly thought to innervate the atrioventricular node (regulating the force of contraction of the cardiac myocytes with less influence over heart rate) however comprehensive human studies confirming this precise delineation are needed (Coote, 2013). The dorsal branchiomotor division of the NA is the site of origin of efferent fibers innervating striated muscle of the palate, pharynx, larynx and upper esophagus.
See Figure 1 for a schematic representation of the VN fibers and central projections.
Figure 1. Schematic representation of afferent and efferent fibers of the vagus nerve and central projections.
Vagus nerve stimulation was initially proposed as a therapeutic intervention in 1871 (Neftel, 1871) and a device was designed to stimulate bilateral vagus nerves in the late 19th century (Lanska, 2002). Preclinical studies in the 1930–1950s demonstrated via EEG signaling that VNS had cortical stimulating activity (Bailey and Bremer, 1938; Zanchetti et al., 1952), and could terminate canine seizures (Zabara, 1985, 1992).
Invasive VNS (iVNS) received United States regulatory approval for the adjunctive treatment of refractory seizures in 1997 and for use in treatment resistant depression in 2005 (O’Reardon et al., 2006). However, given the invasive nature of iVNS (requiring general anesthesia, thoracic implantation of a battery generator, and neck dissection to attach stimulating electrodes to the left cervical vagus nerve), the concept of non-invasive VNS was proposed in 2000 whereby, drawing on evidence from studies of auricular acupuncture, it was postulated that transcutaneous vagal stimulation could represent a valuable tool in epilepsy treatment (Ventureyra, 2000). Non-invasive VNS involves using stimulating electrodes on the skin to excite afferent vagal fibers and can be performed via the ear (transcutaneous auricular VNS: t-VNS) or the neck (transcutaneous cervical VNS: tcVNS). For the purposes of this narrative review non-invasive VNS will refer to auricular t-VNS.
The technique of t-VNS exploits the peripheral anatomy of the vagus nerve, activating vagal afferent projections through stimulation of the auricular branch of vagus nerve (ABVN) at the ear (Peuker and Filler, 2002; Mercante et al., 2018) see Figure 2 for a schematic representation of the anatomy of the ABVN and central structures it modulates. Anti-seizure efficacy equivalent to iVNS was demonstrated in preclinical studies before the feasibility and therapeutic significance of this technique in humans were demonstrated (Stefan et al., 2012) and evidence from multiple functional brain imaging studies confirms significant activation of central vagal projections via this non-invasive method (Kraus et al., 2013; Frangos et al., 2015; Yakunina et al., 2017; Badran et al., 2018a).
Figure 2. Schematic diagram of innervation of ABVN and central projections, adapted with permission from Kaniusas et al. (2019).
Transcutaneous auricular vagus nerve stimulation waveforms can be delivered at a variety of different parameter settings which vary frequency (Hz), amplitude (mA), pulse width (μs-msec) and duration of stimulation. It is currently being investigated as a therapeutic intervention for a variety of medical disorders including epilepsy, migraine and cluster headaches, tinnitus, atrial fibrillation, Parkinson’s disease, schizophrenia, impaired glucose tolerance, obesity, and pain (Goadsby et al., 2014; Huang et al., 2014; Laqua et al., 2014; Hasan et al., 2015; Hyvarinen et al., 2015; Nesbitt et al., 2015; Stavrakis et al., 2015; Cakmak et al., 2017; Obst et al., 2020). There is particular interest in the evolving literature reporting the use of t-VNS in cognitive disorders (Broncel et al., 2020; Lam et al., 2021). Potential mechanisms of action include modulation of HRV, impacts on cerebral perfusion, and noradrenergic neuromodulation. The complementary role of vagus nerve stimulation in modulating neuro-cardiovascular and cognitive performance is explored in detail below.
See Figure 2 for a schematic diagram of the area of innervation of the ABVN and its central projections.
Brain imaging during t-VNS demonstrates strong activation of vagal projections to subcortical nuclei and frontal brain regions, i.e., superior frontal gyrus and medial frontal gyrus during stimulation (Kraus et al., 2013) (See below in “Mechanisms of Action” for further detailed discussion regarding the neuroanatomical structures modulated during VNS). Cognitive effects of both iVNS and t-VNS in both clinical populations and healthy volunteers will be examined under the following themes: Cognitive control, i.e., the non-automatic regulation of behavior to achieve a goal (Gonthier, 2014) a primarily executive function that involves suppression of goal-irrelevant stimuli via response and attention-inhibition (Tiego et al., 2018) and it primarily involves the lateral prefrontal cortex (Dixon, 2015); Language, both assessing categorical fluency a semantic memory language task involving the temporal lobe, and word recognition and retrieval which mostly involves episodic working memory, involving prefrontal cortex and medial temporal structures (Squire and Zola, 1998; Camina and Güell, 2017); Associative memory, a subcategory of declarative episodic memory and involves the ability to link disparate novel stimuli (Naveh-Benjamin, 2000); Emotion recognition as a subtype of cognition involves areas of the brain involved in perceiving social information including the medial prefrontal cortex and the orbitofrontal cortex (Bachmann et al., 2018) and regions implicated in emotional processing, including the cortical orbitofrontal cortex and the anterior cingulate cortex but also subcortical structures including the amygdala, hypothalamus, basal ganglia and the periaqueductal gray matter (van den Stock et al., 2011).
Interest in the potential role of VNS as a cognitive enhancer started following a preclinical rodent study of an inhibitory-avoidance task. Subjects received a single exposure to a foot shock followed immediately by VNS or sham. Those undergoing true VNS stimulation had longer step times demonstrating enhanced avoidance and this effect was modulated by the intensity of the stimulus, with 0.4 mA being an effective level of stimulation and 0.2 and 0.8 mA having no significant effect (Clark et al., 1995). Subsequent in-human trials tested word recognition in patients with intractable epilepsy who had iVNS devices implanted 2–24 weeks prior to testing. The stimulation parameters were 30Hz, 0.5 mA at 0.5 ms pulse width compared to an amplitude of 0.75–1 mA, and improved word recognition was only found in the group stimulated at the lower amplitude (Clark et al., 1999). These results paved the way for further investigation in this area as detailed below.
Inhibitory control is commonly measured using performance on tasks such as the Stroop, Eriksen Flanker (Flanker), and Simon tasks, i.e., forced-choice reaction time tasks that require participants to selectively attend and respond to target stimuli whilst ignoring goal-irrelevant distracting stimuli (Kornblum et al., 1990; MacLeod, 1991; Eriksen, 1995).
Enhanced response times, as reflected by participants’ ability to stop a process and change to another response simultaneously and sequentially, and increased post error slowing were demonstrated during t-VNS (Sellaro et al., 2015; Steenbergen et al., 2015). Post error slowing refers to appropriate slowing after negative feedback or unforeseen errors and is linked to the activity of the locus coeruleus–norepinephrine (LC–NE) system and therefore postulated to be enhanced by VNS. As with the above trials, there were fewer false alarms during a more challenging paradigm with t-VNS when working memory processes were simultaneously engaged (Beste et al., 2016) and improved response selection and control performance was demonstrated with t-VNS in a serial reaction time test in young volunteers (Jongkees et al., 2018). In a sequence learning paradigm, the presentation of so-called reversal trials is associated with longer response latencies as compared to non-reversal trials, a result attributable to the ‘inhibition of return’ type phenomenon. Inhibition of return refers to an inhibitory after-effect of attention whereby, following exogenous orientation of attention to a stimulus, processing of stimuli at this location is first facilitated and then inhibited (Wang et al., 2018). Jongkees et al. (2018) demonstrated that active t-VNS, as compared to sham stimulation in the context of a serial reaction time test, reduced reaction time for reversal trials, eliminating the inhibition of return like effect described above.
In a similar experimental set up, increased attention, globally enhanced accuracy and reduced performance costs were demonstrated in a Stop-Change paradigm with t-VNS (Keute et al., 2020).
Results in this area have not been uniformly positive. In a testing paradigm in healthy volunteers using higher than average amplitude settings (see Table 2) there were no improvements in a Stroop test, Modified Flanker test or a number/letter working memory task with t-VNS. Improved accuracy in a dimensional change card sorting task was however noted (Borges et al., 2020). Similar previous studies failed to show improved behavioral performance with t-VNS (Fischer et al., 2018; Ventura-Bort et al., 2018) however non-performance parameters, namely a frontal EEG signal (P3 amplitude) thought to change with response inhibition and higher salivary amylase levels, were noted in the intervention group (Ventura-Bort et al., 2018). Further studies investigating EEG amplitudes affected by t-VNS and cognitive control paradigms included one involving an acoustic rather than visual oddball paradigm. In this context, t-VNS augmented the P3 amplitude, and with random noise stimulation with t-VNS reaction times were reduced (Rufener et al., 2018). There are myriad potential reasons for replication challenges in this newly expanding area of research and may include stimulation parameter differences including lack of pre-testing active stimulation.
The most recent studies in this area have involved a spatial stimulation and response inhibition multitask, with notable improved results in accuracy with 25 min pre-assessment t-VNS stimulation (Sun et al., 2021) and improved objective attention, arousal and multitasking ability in sleep deprived military personnel (McIntire et al., 2021).
Fluency scores in healthy volunteers during a convergent and divergent thinking task were significantly higher during active t-VNS at the left conchae, and categorical flexibility (i.e., participants’ ability to think of more and varied categories of nouns) was also significantly improved (Colzato et al., 2018). However, an experimental design investigating the difference in effect of t-VNS on word recognition memory in young compared to older volunteers (average age 22.2 and 55.1) whereby t-VNS was delivered for 30 s during the consolidation phase of a word recognition memory task showed no improvement in accuracy scores for immediate recall or delayed recognition in both age groups (Mertens et al., 2020). Possible reasons for this may be that 30 s of t-VNS may be insufficient for a non-invasive device to effectively stimulate the vagal afferent pathway, that longer and more repetitive stimulation of the vagus nerve might be required to effectively modulate hippocampal processes via synaptic plasticity. A recent investigation of word retention, stimulating the left tragus with t-VNS at again similar parameters but wider amplitude found improved accuracy in word retention but only in items that rhymed, i.e., were phonologically similar (Kaan et al., 2021).
Transcutaneous auricular vagus nerve stimulation has been tested in a group of healthy older adults to determine the technique’s impact on performance in a face-name association task (Jacobs et al., 2015). VNS was employed in the encoding and consolidation phases of the task with active and sham stimulation compared in a randomized crossover design. Active t-VNS was demonstrated to increase the number of ‘hits’ on the memory task. Stimulation parameters employed differed somewhat from those seen in the broader literature concerning the impact of VNS on cognitive function. A stimulation intensity of 5.0 mA, a pulse width of 0.2 ms, and a frequency of 8Hz were utilized, citing previous functional and electrophysiological studies (Kraus et al., 2007; Polak et al., 2009). A stimulation lead in time of 17 min was also utilized, which has been theorized to be beneficial for targeted neuronal plasticity (Hays et al., 2013).
This ability to recognize different emotions in others was investigated and found to be enhanced by t-VNS at the left outer auditory canal in young healthy adults but only for objectively easy, not challenging, items via the Reading the Mind in the Eyes test (Colzato et al., 2017). Subsequent investigations of fear conditioning and extinction in young volunteers, after previous positive studies, found that t-VNS at the left cymba conchae did not infer any difference in physiological or declarative indices of fear or improve fear extinction (Burger et al., 2019). Further studies are needed in this area to elucidate if t-VNS has a specific beneficial effect, given its ability to modulate both cortical and subcortical structures.
See Table 2 for parameters settings and outcomes in trials of VNS in healthy volunteers.
In this section we highlight the studies to date investigating the cognitive effects of VNS on clinical populations, mostly with treatment-resistant depression or epilepsy. Many studies investigating the role of VNS in clinical populations has involved invasive VNS (iVNS). A further potential confounder is the impact some of these underlying pathologies have on cognition, the altered medial temporal anatomy especially in cases of epilepsy and the medications used to manage these conditions can also have deleterious effects on cognition.
Vagus nerve stimulation has been shown experimentally to have mixed results when examining the subdomain of decision making, specifically on the Iowa Gambling Task (IGT). In one paradigm eleven patients with refractory epilepsy and iVNS devices completed a gambling task involving control and experimental trials with active VNS synchronized to stimulate in the latter. Whilst improved performance was demonstrated in the earlier part of the task, this trend was reversed later in the experimental trial with active stimulation trending toward being detrimental to performance (Martin et al., 2004). Technical failure and a cumulative stimulation-dose effect were amongst the potential explanations proposed by the authors to explain this phenomenon. Decision-making may depend on intact working memory (Bechara and Martin, 2004) and several studies have demonstrated working memory involvement in the IGT (Bagneux et al., 2013) which may have affected results in this study.
Working memory refers to a cognitive process that provides temporary storage and manipulation of the information necessary for complex cognitive tasks (Baddeley, 2010). Literature concerning the impact of acutely administered VNS on working memory is promising but limited to a small number of studies. In one experimental paradigm, twenty participants with poorly controlled epilepsy were required to perform a computer-based Executive-Reaction Time (Executive RT) Test, wherein ability to memorize and store the orientation of a triangle and indicate its position in response to a go signal were assessed whilst VNS was delivered in a cyclic fashion. Active iVNS stimulation was associated with fewer errors in the subtask relying on working memory (Sun et al., 2017).
The effect of active iVNS on response inhibition was also assessed by employing a classic stop-signal task in participants with refractory epilepsy (Schevernels et al., 2016). Quicker response inhibition has been demonstrated during active stimulation in patients who had previously shown a larger therapeutic effect of VNS. The beneficial effects of VNS on cognitive control may be maximally demonstrated in so-called ‘VNS responders’ (for the primary clinical indication) as demonstrated by patients with iVNS devices who undertook the Eriksen Flanker task during both VNS ‘on’ and ‘off’ stimulation. Only those deemed VNS responders (i.e., those whose seizure frequency had decreased by >50% post-device implantation) had demonstrable improved reaction times and reduced distractor interference during active stimulation (van Bochove et al., 2018). There is a subcategory of patients with refractory epilepsy who do not respond to iVNS therapy, i.e., do not have seizure reduction of 50%, and deemed “non-responders.” It is notable that a current output of 2.28 mA was utilized in the VNS “responder” group and it’s possible that, in keeping with previous studies examining optimal amplitude for stimulation, that the higher amplitudes employed exceeded that at which cognitive control is optimized for the iVNS “non-responders.” Further research is needed in this area in particular regarding stimulation parameters and iVNS responders.
In the first study of its kind, building on previous preclinical research, the impact of iVNS on word retrieval memory was assessed via an experimental protocol whereby participants with iVNS devices inserted for epilepsy control, were required to read a series of paragraphs, and subsequently identify words that were highlighted in the text. The study population comprised two groups of patients who were administered active (0.5–1.5 mA) or sham VNS, delivered 2-min after learning in the memory consolidation phase. An inverted U-shaped relationship was demonstrated regarding stimulus intensity and modulation of cognitive performance, with memory enhancing effects demonstrated only at moderate intensities, namely 0.5 mA (Clark et al., 1999). These results were in part corroborated by a subsequent study which employed higher stimulation intensities (>1.0 mA) and failed to demonstrate enhancement of verbal recognition memory, in fact demonstrating a reversible deterioration in figural memory (Helmstaedter et al., 2001). However, study design may have impacted cognitive outcomes here as delivery of stimulation was not restricted to the consolidation period. The propensity for iVNS to positively impact word retrieval memory in a population of patients being treated with iVNS for intractable epilepsy was highlighted again in 2006 whereby the impact of iVNS on performance in the Hopkins Verbal Learning Test was assessed, demonstrating a significant improvement in word retention when active (amplitude 0.5 mA) as opposed to sham stimulation was applied during memory consolidation (Ghacibeh et al., 2006b).
The effect of t-VNS on participants’ ability to recognize facial emotions in three experimental paradigms (graded presentation, static images and in a go-no-go task) was assessed in a group of adolescents diagnosed with major depressive disorder (MDD). In non-depressed controls t-VNS delivered at 1Hz, 0.5 mA 30 s block with 15 min lead in time, demonstrated enhanced recognition of emotions but notably led to a significant decrease in the ability of those with MDD to recognize sad emotions (Koenig et al., 2021).
See Table 3 for parameters settings and outcomes in trials of VNS in clinical populations and please see below “VNS, cognition and HRV” for a discussion of VNS in Alzheimer’s disease.
There are many potential mechanisms through which VNS may exert its cognitive enhancing effects, including direct neurotransmitter release, increased cerebral perfusion to discreet neuroanatomical structures, reduced neuro-inflammation and via modulation of peripheral hemodynamics. For the purposes of this narrative review, we will analyze the link between cerebral blood flow, cerebral autoregulation and cardiac modulation. Beyond the scope of this review is how t-VNS may therapeutically affect the inflammatory cascade via activating the cholinergic anti-inflammatory pathway and the beneficial effects this may have in aging populations.
The main neurotransmitters centrally released via the afferent projections of the vagus nerve are thought to be GABA and Norepinephrine (NE). For a comprehensive review of the preclinical and clinical studies detailing the evidence supporting the modulation of these neurotransmitters during iVNS and t-VNS see (Colzato and Beste, 2020).
As the primary inhibitory neurotransmitter in the brain, higher levels of GABA decrease cortical excitability, and is the accepted proposed method for VNS’ anti-seizure efficacy. It has been suggested that increased cortical inhibition due to high GABA levels can sharpen task-relevant representations in the cortex and inhibit competing responses, thereby facilitating response selection and inhibition processes (Munakata et al., 2011; de la Vega et al., 2014).
Norepinephrine is a crucial neurotransmitter modulating arousal and attention, and is primarily released via the locus coeruleus (LC). There are two distinct modes of LC firing that are associated with equally distinct modes of attentional strategy. Connections with the orbitofrontal cortex and anterior cingulate cortex are thought to drive the LC-NE system into one of these two stable states of activity, a high tonic (sustained) mode or a phasic (bursting) mode accompanied by moderate tonic activity (Aston-Jones and Cohen, 2005). This switching of attentional state via tonic LC activity is thought to result in a flexible attentional system that allows cycling between behaviors to find and meet task demands in one’s environment, i.e., the adaptive gain theory (Aston-Jones and Cohen, 2005).
Interestingly, and similar to the effects noted with iVNS stimulation levels and responses by Clark et al. (1995), moderate levels of NE augment prefrontal cortex function, whereas high and low concentrations of NE impair function, i.e., NE exhibits an inverted-U relationship between LC-NE activity and optimal performance on attention tasks (Berridge and Waterhouse, 2003). However, in general as NE levels rise executive function improves, likely via enhanced activation of the prefrontal cortex and frontoparietal control network (Xing et al., 2016; Unsworth and Robison, 2017). Inhibitory control for action cancelation is specifically enhanced with noradrenergic modulation, likely via this prefrontal cortical network (Chambers et al., 2009; Duann et al., 2009).
Older adults with more dense LC innervation (i.e., higher neuromelanin MRI contrast) had overall better performance on a reversal memory tasks (Hämmerer et al., 2018) and had improved cognitive reserve (Clewett et al., 2016). Similarly in a post-mortem study of patients with Alzheimer’s disease, lower LC cell integrity and greater cortical tangle density was associated with greater tau burden beyond the medial temporal lobes and worsening memory decline, identifying LC integrity as a promising indicator of initial AD-related processes (Jacobs et al., 2021).
Studies have also demonstrated a decline in GABA concentration in frontal and parietal regions in aging populations, areas crucial for cognitive control (Gao et al., 2013; Porges et al., 2017). NE and GABA may in fact work synergistically to facilitate executive functioning; GABA by encouraging response inhibition of task irrelevant stimuli and NE via the LC-NE system increasing frontal NE release and thus executive functioning (Ridgewell et al., 2021).
Cerebral autoregulation is the phenomenon by which the brain receives the same cerebral blood flow (CBF) despite variations in perfusion pressure. The aim of autoregulation is to protect the brain against hypoxia and edema as a result of decreased or critically high arterial blood pressures respectively. Multiple factors physiologically modify autoregulation including blood CO2 levels, hypoxia etc. While still controversial, the ANS may play a prominent role in cerebral autoregulation in response to such stimuli, inducing vasodilation or constriction, and parasympathetic and sympathetic nerves are anatomically located in the same perineural sheath innervating cerebral arteries (Tamayo and Siepmann, 2021). The means by which VNS exerts its cognitive enhancing effect is probably multimodal, however modulating CBF is likely a crucial factor.
Multiple modalities have been utilized to assess for CBF changes due to vagus nerve stimulation, including position emission tomography (PET), functional magnetic resonance imaging (fMRI) and single photon emission computed tomography (SPECT) studies and trials of patients with iVNS treatment for epilepsy and depression have demonstrated a variety of CBF modulatory effects at specific cortical and subcortical areas. Increased CBF at the orbitofrontal cortex (Henry et al., 1998; Bohning et al., 2001; Lomarev et al., 2002; Mu et al., 2004; Vonck et al., 2008), temporal lobe (Ko et al., 1996; Lomarev et al., 2002; Liu et al., 2003; Vonck et al., 2008; Conway et al., 2012), insular cortex (Liu and Hu, 1988; Henry et al., 1998, 2004), bilateral frontal lobes (Sucholeiki et al., 2002), left dorsolateral prefrontal cortex (Kosel et al., 2011) and subcortical structures including thalamus, hypothalamus, basal ganglia and other nuclei (Narayanan et al., 2002; Sucholeiki et al., 2002; Conway et al., 2012) has been observed. For a comprehensive review see Chae et al. (2003).
Notably analysis undertaken during acute iVNS has noted bilateral decreased hippocampal CBF (Henry et al., 1998; Mu et al., 2004; Vonck et al., 2008). This has been replicated in t-VNS functional imaging studies which have confirmed stimulation and increased CBF at vagally innervated brain regions during auricular t-VNS and notably decreased perfusion at hippocampal regions (Kraus et al., 2007, 2013; Frangos and Komisaruk, 2017). T-VNS has also demonstrated efficacy in increasing arousal in comatose patients who respond to auditory signaling and again the brain regions noted on fMRI to be activated were similar to previous iVNS studies, including left superior temporal gyrus, left prefrontal cortex, left insular cortex, left middle frontal gyrus among other cortical and subcortical structures (Yu et al., 2021).
It is worth considering that intermittently stimulating neurons at different frequencies produces drastically different changes in neuronal behavior with low frequency stimulation inducing long term depression (LTD) and less connectivity while intermittent high frequency stimulation produces long term potentiation (LTP) and increased signaling (Lomarev et al., 2002; Kealy and Commins, 2010). Therefore acute VNS stimulates brain regions mostly involved in alertness and frontal processing, whereas chronic stimulation may improve LTP in classic memory-associated regions, including the hippocampus. Evidence for this can be seen in preclinical studies (Zuo et al., 2007) but also significant increases in hippocampal gray matter volume over time has been observed in patients with iVNS devices inserted for treatment-resistant depression (Perini et al., 2017). More recently, Near Infrared Spectroscopy (NIRS) has been utilized to monitor cerebral blood flow and increased frontal perfusion in patients with epilepsy was noted during iVNS when paired with a cognitive task (Kunii et al., 2021).
Both dementia and even its prodromal stage, MCI, are characterized by a reduction in cerebral blood flow (Mazza et al., 2011; Sierra-Marcos, 2017). A meta-analysis of twenty-six studies investigating CBF in MCI found overall reduced tissue oxygenation, CBF and velocity in MCI compared to healthy controls (Beishon et al., 2017) and studies are underway investigating the CBF changes that may occur with cognitive stimulation in MCI and dementia (Beishon et al., 2019). Similar findings have been noted in patients with Alzheimer’s disease, with reduced CBF in many cortical regions including temporal (Sandson et al., 1996; Alsop et al., 2000; Asllani et al., 2008; Yoshiura et al., 2009; Ding et al., 2014) parietal (Alsop et al., 2000; Johnson et al., 2005) and other regions including precuneus, frontal and posterior cingulate cortex (Alsop et al., 2008; Yoshiura et al., 2009).
As well as modulating central neurotransmitter release and cerebral blood flow, VNS has been shown to have positive peripheral modulatory effects in pathological states characterized by impaired autonomic regulation including postural orthostatic tachycardia syndrome (POTS) (Petelin Gadze et al., 2018) specifically patients with POTS and impaired vagal cardiac control, as defined by reduced HRV (Jacob et al., 2019). T-VNS has also shown benefits in modulating blood pressure in induced orthostatic hypotension (Tobaldini et al., 2019). These studies suggest VNS may have a role in positively manipulating the peripheral baroreceptor-reflex and thus cerebral autoregulation, and potentially may improve cortical perfusion via this route, however further dedicated studies are required to precisely delineate this relationship.
Heart rate variability analysis can be performed via a variety of approaches and is based on the extrapolation of time intervals between each R wave peak (Shaffer and Ginsberg, 2017), discounting any ectopic beats or arrhythmias, e.g., atrial fibrillation. The most commonly applied methods to determine HRV are time-domain analysis and frequency/spectral analysis. Indices deriving from the time domain analysis quantify the amount of variance in the selected inter-beat interval employing statistical measures, such as the standard deviation of the normal beat intervals (SDNN) and the root mean square of successive differences between normal beats (RMSSD) (Shaffer et al., 2014). The spectral analysis of HRV identifies oscillatory rhythms that occur in specific frequency ranges. Three main components of the spectrums can be identified as: the very low frequency band (VLF), below 0.04 Hz, likely influenced by thermoregulatory mechanisms and circadian rhythms; the low-frequency band (LF) between 0.04 and 0.15 Hz in humans, a marker influenced by baroreflex (Furlan et al., 2019) sympathetic and parasympathetic modulation; the high-frequency band (HF) in the range from 0.15 to 0.4 Hz, a marker of vagal modulation that is influenced by respiratory activity (Montano et al., 2009; Shaffer et al., 2014). One of the limitations of HRV analysis is high within and between individual variability, which may be reduced by longer measurement intervals, i.e., 24 h but which is resultantly harder to process. For a comprehensive review on the various indices please see Merrick et al. (2017).
The ANS influences cardiac beat-to-beat interval length in response to several factors. The sympathetic and parasympathetic systems are the principal rapidly reacting systems that control heart rate. The two systems have different latency periods with sympathetic effects on heart rate slower than parasympathetic (Warner and Cox, 1962; Pickering and Davies, 1973; Koizumi et al., 1983) i.e., the parasympathetic system has the ability to alter heart rate within 1–2 beats, while sympathetic effects take up to 10 s to take effect.
Low HRV has been associated with poorer prognosis in cardiovascular diseases, cancer, Metabolic Syndrome and Alzheimer’s disease and it has been postulated that related pathophysiological mechanisms often contribute to their occurrence and progression, namely inflammatory responses, sympathetic overactivity, and oxidative stress (Entschladen et al., 2004; Thayer and Lane, 2007; de Couck et al., 2012). Lower vagal nerve activity has been found to be significantly correlated with oxidative stress (Tsutsumi et al., 2008), with inflammatory markers in healthy individuals as well as in those with cardiovascular diseases (Haensel et al., 2008) and anxiety disorders have also been characterized by low HRV (Chalmers et al., 2014). Experimental studies have long demonstrated the success of behavioral (Stein and Kleiger, 2003) and pharmacological (Sandrone et al., 1994) interventions in manipulating HRV. Increases in HRV seen with physical fitness training are associated with improvements in executive function (Hansen et al., 2004). The links between executive function and cardiac autonomic regulation were further highlighted by a recent study examining the impact of cognitive and motor training on HRV indices. Physical training alone failed to impact HRV in older adults whereas dual cognitive and motor training significantly improved global and parasympathetic autonomic nervous system activity (Eggenberger et al., 2020). These studies point toward a duality; the vagal communications between heart and mind can be bidirectionally manipulated to improve both parasympathetic control of HRV and, synergistically, executive cognitive function.
Preclinical research has noted that VNS, particularly to the right vagus nerve, increases vagally mediated (vm-) HRV measures (Huang et al., 2010; Sun et al., 2013). In a canine study, VNS treatment enhanced HRV at 4 and 8 weeks and reduced heart failure development (Zhang et al., 2009) and a Japanese study in rabbits founds that intermittent VNS, but not constant VNS, increased the HF (vagal) component of HRV (Iwao et al., 2000). Discrepancies in this preclinical work may be due to different species, devices and parameters but indicate that manipulating the vagus nerve electrically can have positive impacts on cardiac function and HRV.
Transcutaneous auricular vagus nerve stimulation devices and their stimulation effect on HRV have been examined in several experimental paradigms involving multiple auricular positions, left vs. right ear stimulation, and different stimulation settings. There is a trend toward positive findings, i.e., improved HRV indices, with t-VNS in healthy volunteer populations when the right auricular branch of the vagus is stimulated (de Couck et al., 2017; Machetanz et al., 2021). It is notable that greater responses to t-VNS (i.e., improved vagally medicated HRV signals) have been demonstrated in those with higher sympathetic balance at baseline in both younger and older volunteers, both acutely and with 2 weeks t-VNS at home for 15 min daily (Clancy et al., 2014; Bretherton et al., 2019). An experimental design comparing left and right t-VNS at multiple stimulation targets found that SDNN and RMSSD both were most significantly improved when the right cymba conchae and fossa triangularis were stimulated (Machetanz et al., 2021).
When specific parameters of stimulation at the left tragus were sequentially analyzed, the settings that had the most significant impact on heart rate analysis in young volunteers were 500 μs at 10 Hz (Badran et al., 2018b). Studies investigating the effect of t-VNS and 70-degree tilt table testing on HRV at the left tragus found that the RSA measure of HRV (HF domain) was also significantly increased during an orthostatic maneuver (Lamb et al., 2017) and similarly stimulation at the left cymba conchae during 75-degree tilt found that responsivity, i.e., degree of change of heart rate and systolic blood pressure during t-VNS were significantly higher during orthostasis compared to control (Tobaldini et al., 2019).
Research in this area has not been consistent. Some initial findings indicated improved HRV measures with t-VNS to the left cymba conchae but ultimately no difference compared to sham and at multiple intensities (Borges et al., 2019). In an experimental crossover design employing a variety of amplitudes at the right cymba, there was no positive signal in affecting HRV measures (Gauthey et al., 2020) and similarly t-VNS to the right tragus during rest and autonomic nervous system testing, with appreciably different stimulation parameters to what was previously cited in the literature, also did not have any effect on HRV (Sinkovec et al., 2021). Inconsistent results are likely due to the use of different anatomical sites and stimulation parameters being utilized, some with “lead in” times and some without, and reporting on this area has been of variable quality, and recent international consensus has called for standardized reporting of this research (Farmer et al., 2021).
Initial studies in clinical populations involved patients with iVNS devices inserted for control of refractory epilepsy. The earliest study demonstrated a reduction in LF:HF ratio and significantly higher HF power was noted in the higher stimulation group than lower stimulation (see Table 4; Kamath et al., 1992). These results were not however replicated in further studies of similar populations with comparable stimulation settings at timeframes ranging from minutes to 1 year of stimulation (Handforth et al., 1998; Setty et al., 1998; Galli et al., 2003; Ronkainen et al., 2006; Barone et al., 2007). A small study analyzing HRV in patients with iVNS devices implanted for management of treatment-resistant depression noted an increase in the RMSSD (increased vagal predominance) during stimulation compared to baseline and healthy controls (Sperling et al., 2010). It is notable that iVNS devices are for the most part inserted to activate the vagus via its left cervical branch, thereby appropriately reducing adverse cardiac effects but also not demonstrably influencing HRV measures in these populations.
Please see Table 4 for further analysis of the specific neurocardiovascular assessments, specific t-VNS parameters and outcomes measures in discreet populations in this area.
Heart rate variability can be conceptualized as a biomarker of parasympathetic modulation, and it is associated with a network of brain regions involved in autonomic nervous system regulation, known as the central autonomic network (Benarroch, 1993; Thayer et al., 2009). This network, which comprises prefrontal cortical (anterior cingulate, insula, orbitofrontal, and ventromedial cortices), limbic (central nucleus of the amygdala, hypothalamus), and brainstem regions, areas of the brain intimately involved in emotional regulation and executive functioning, leading to the proposal that vagally mediated HRV may index these aspects of prefrontal cortical function (Thayer and Lane, 2007; Thayer et al., 2009). Higher HRV has been linked to better cognitive function in healthy adults including healthy older individuals (Frewen et al., 2013; Grässler et al., 2020) and a meta-analysis found a positive overall correlation (r = 0.09) between vagally mediated HRV indices and emotional regulation processes (including executive functioning, emotion regulation, and effortful or self-control) in mostly healthy participants across a number of age groups (Holzman and Bridgett, 2017).
Autonomic system dysfunction is common in patients with MCI, with studies suggesting MCI participants are 5.6 times more likely than controls to have autonomic dysfunction, specifically on assessment of HRV and cardiac reflexes (Collins et al., 2012). A meta-analysis of MCI with dementia also found autonomic dysfunction, as defined by reduced HRV, was significantly associated with cognitive impairment (da Silva et al., 2017). Reduced HRV is associated with worse performance on tests of global cognitive function, more than cardiovascular risk factors (Zeki Al Hazzouri et al., 2014).
Recent meta-analyses of HRV in patients with neurodegenerative conditions including MCI, Alzheimer’s disease, Lewy Body dementia (DLB), vascular dementia, Parkinson’s disease and multiple sclerosis found a significant, moderate effect (r = 0.25) indicating that higher HRV was related to better cognitive and behavioral scores, which was not influenced by mean age or cognitive status (Liu et al., 2022). These results were mirrored in a similar recent meta-analysis of patients with dementia compared to healthy controls, which found significantly lower resting HRV for parasympathetic function and total variability in those with dementia. On subgroup analysis then most striking differences, i.e., worse HRV analysis was found in those with MCI or DLB (Cheng et al., 2022).
Heart rate variability and CBF are linked via vagal afferents, and a meta-analysis revealed that HRV was significantly associated with regional cerebral blood flow in the ventromedial prefrontal cortex (including anterior cingulate regions) and the amygdala (Thayer et al., 2012). In both younger and older adults scanned while at rest, higher HRV is associated with higher medial prefrontal cortex and amygdala functional connectivity (Sakaki et al., 2016). The Neurovisceral Integration Model holds that HRV, executive cognitive function, and prefrontal neural function are integrally associated (Thayer et al., 2009).
In an interesting Swedish clinical trial in 2002, iVNS devices were implanted in a small group of patients with likely Alzheimer’s Dementia (AD) as defined by the criteria of the National Institute of Neurological and Communicative Disorders and Stroke and the Alzheimer’s Disease and Related Disorders Association (NINCDS-ADRDA), with a view to assessing its impact on cognition via memory test scores. In the primary trial, 10 patients with average Mini Mental State Exam (MMSE) scores of 21 (range 16–24) had iVNS devices implanted and the median change in MMSE and Alzheimer’s Disease Assessment Scale-cognitive subscale (ADAS-cog) scores among a battery of tests was assessed at 3 and 6 months, with improvements in both assessments noted in the majority (6 out of 10) of cases (Sjögren et al., 2002). The follow up trial by the same research group involved 17 patients with likely AD, who had iVNS devices implanted and had outcomes measured and available at 1 year post implantation. At 1 year, 7 of 17 (41%) had improvement or no decline from baseline in ADAS-cog scores and 12 of 17 (70%) had improvement or no decline in MMSE scores. There was no change in noted in other outcomes including depressive symptoms (Merrill et al., 2006). There are a small number of trials registered investigating the therapeutic potential of t-VNS in older populations, both healthy and with cognitive impairment (for a recent review see (Vargas-Caballero et al., 2022)) however there are no known published studies to date investigating t-VNS in populations with dementia or MCI, and the associated effect on HRV.
There is mounting evidence of the potential benefits of VNS in myriad disease states, with notable promise in the area of cognition. VNS shows promise as a neuromodulatory technique in cognitive decline and this may be via its ability to regulate both cardiac autonomic function and increase cerebral perfusion. Dementia is a multifactorial process and together with reduced cerebral perfusion is associated with neuroinflammation and altered synaptic plasticity, both of which may also be favorably modulated by VNS. It has been noted that perfusion to cortical and subcortical areas increases with VNS, specifically to areas that modulate executive function and attention, i.e., insular, orbitofrontal and prefrontal cortex. These areas are hypothesized by the neurovisceral integration model to be crucial areas in modulating the ANS (Thayer et al., 2009). Given that the LC-NE system is intimately involved in the therapeutic effects of VNS, and likely improves cognition via norepinephrine release and improved executive performance, it is notable that the earliest stages of pathological tau accumulation in Alzheimer’s disease are seen in the LC. Whether this small midbrain nucleus will prove to be pivotal in our understanding of how to modulate the vagus nerve and harness its benefits cognitively remains to be elucidated. VNS can now be delivered safely and non-invasively via t-VNS devices with equivalent neuromodulatory effects on brain imaging as invasive devices, which broadens its therapeutic applicability considerably, especially to an older population with cognitive complaints for whom device implantation may not be feasible. Globally, the need for effective therapies to both treat the cause and symptoms of cognitive decline are needed urgently as rates of dementia increase due to population expansion. Dedicated studies into the potential therapeutic effects of t-VNS in early cognitive decline and dementia are needed. Research to date has been limited by myriad issues, including studies on cognition in clinical populations with altered neuroanatomy, lack of standardization in device usage, parameter settings, frequency of use, duration of stimulation. Minimum reporting standards have recently been published to help ameliorate some of these issues. Further rigorous studies of the therapeutic benefit of VNS are required, especially in populations with autonomic instability and cognitive decline.
HD did most of the research, writing, and editing of the article. Significant contributions were made by each author, specifically TD with manuscript reading, editing, and direction, SC with direction RE psychological assessments and plasticity, CF with neurocardiovascular assessments, ANS testing. PM and SK assisted significantly with overall editorial support and guidance. All authors contributed to the article and approved the submitted version.
This study is part of a research study generously funded by the Meath Foundation, Tallaght University Hospital.
The authors declare that the research was conducted in the absence of any commercial or financial relationships that could be construed as a potential conflict of interest.
All claims expressed in this article are solely those of the authors and do not necessarily represent those of their affiliated organizations, or those of the publisher, the editors and the reviewers. Any product that may be evaluated in this article, or claim that may be made by its manufacturer, is not guaranteed or endorsed by the publisher.
Agostini, E., Chinnock, J., de Daly, M., and Murray, J. (1957). Functional and histological studies of the vagus nerve and its branches to the heart, lungs and abdominal viscera in the cat. J. Physiol. 135, 182–205. doi: 10.1113/JPHYSIOL.1957.SP005703
Alsop, D., Detre, J., and Grossman, M. (2000). Assessment of cerebral blood flow in Alzheimer’s disease by spin-labeled magnetic resonance imaging. Ann. Neurol. 47, 93–100. doi: 10.1002/1531-8249(200001)47:1<93::AID-ANA15<3.0.CO;2-8
Alsop, D. C., Casement, M., de Bazelaire, C., Fong, T., and Press, D. Z. (2008). Hippocampal hyperperfusion in Alzheimer’s disease. NeuroImage 42, 1267–1274. doi: 10.1016/J.NEUROIMAGE.2008.06.006
Antonino, D., Teixeira, A. L., Maia-Lopes, P. M., Souza, M. C., Sabino-Carvalho, J. L., Murray, A. R., et al. (2017). Non-invasive vagus nerve stimulation acutely improves spontaneous cardiac baroreflex sensitivity in healthy young men: a randomized placebo-controlled trial. Brain Stimul. 10, 875–881. doi: 10.1016/j.brs.2017.05.006
Asllani, I., Habeck, C., Scarmeas, N., Borogovac, A., Brown, T. R., and Stern, Y. (2008). Multivariate and univariate analysis of continuous arterial spin labeling perfusion MRI in Alzheimer’s disease. J. Cereb. Blood Flow Metab. Off. J. Int. Soc. Cereb. Blood Flow Metab. 28, 725–736. doi: 10.1038/SJ.JCBFM.9600570
Aston-Jones, G., and Cohen, J. D. (2005). An integrative theory of locus coeruleus-norepinephrine function: adaptive gain and optimal performance. Annu. Rev. Neurosci. 28, 403–450. doi: 10.1146/ANNUREV.NEURO.28.061604.135709
Bachmann, J., Munzert, J., and Krüger, B. (2018). Neural underpinnings of the perception of emotional states derived from biological human motion: a review of neuroimaging research. Front. Psychol. 9:1763. doi: 10.3389/FPSYG.2018.01763
Badran, B. W., Dowdle, L. T., Mithoefer, O. J., LaBate, N. T., Coatsworth, J., Brown, J. C., et al. (2018a). Neurophysiologic effects of transcutaneous auricular vagus nerve stimulation (taVNS) via electrical stimulation of the tragus: a concurrent taVNS/fMRI study and review. Brain Stimul. 11, 492–500. doi: 10.1016/J.BRS.2017.12.009
Badran, B. W., Mithoefer, O. J., Summer, C. E., LaBate, N. T., Glusman, C. E., Badran, A. W., et al. (2018b). Short trains of transcutaneous auricular vagus nerve stimulation (taVNS) have parameter-specific effects on heart rate. Brain Stimul. Basic Transl. Clin. Res. Neuromodul. 11, 699–708. doi: 10.1016/J.BRS.2018.04.004
Bagneux, V., Thomassin, N., Gonthier, C., and Roulin, J. L. (2013). Working memory in the processing of the iowa gambling task: an individual differences approach. PLoS One 8:e81498. doi: 10.1371/JOURNAL.PONE.0081498
Bailey, P., and Bremer, F. (1938). A sensory cortical representation of the vagus nerve: with a note on the effects of low blood pressure on the cortical electrogram. J. Neurophysiol. 1, 405–412. doi: 10.1152/JN.1938.1.5.405
Barone, L., Colicchio, G., Policicchio, D., di Clemente, F., di Monaco, A., Meglio, M., et al. (2007). Effect of vagal nerve stimulation on systemic inflammation and cardiac autonomic function in patients with refractory epilepsy. Neuroimmunomodulation 14, 331–336. doi: 10.1159/000127360
Bechara, A., and Martin, E. M. (2004). Impaired decision making related to working memory deficits in individuals with substance addictions. Neuropsychology 18, 152–162. doi: 10.1037/0894-4105.18.1.152
Beckstead, R. M., and Norgren, R. (1979). An autoradiographic examination of the central distribution of the trigeminal, facial, glossopharyngeal, and vagal nerves in the monkey. J. Comparat. Neurol. 184, 455–472. doi: 10.1002/CNE.901840303
Beishon, L., Evley, R., Panerai, R. B., Subramaniam, H., Mukaetova-Ladinska, E., Robinson, T., et al. (2019). Effects of brain training on brain blood flow (The Cognition and Flow Study-CogFlowS): protocol for a feasibility randomised controlled trial of cognitive training in dementia. BMJ Open 9:27817. doi: 10.1136/bmjopen-2018-027817
Beishon, L., Haunton, V. J., Panerai, R. B., and Robinson, T. G. (2017). Cerebral hemodynamics in mild cognitive impairment: a systematic review. J. Alzheimer’s Dis. 59, 369–385. doi: 10.3233/JAD-170181
Benarroch, E. E. (1993). The central autonomic network: functional organization, dysfunction, and perspective. Mayo Clin. Proc. 68, 988–1001. doi: 10.1016/S0025-6196(12)62272-1
Berridge, C. W., and Waterhouse, B. D. (2003). The locus coeruleus–noradrenergic system: modulation of behavioral state and state-dependent cognitive processes. Brain Res. Rev. 42, 33–84. doi: 10.1016/S0165-0173(03)00143-7
Berthoud, H. R., and Neuhuber, W. L. (2000). Functional and chemical anatomy of the afferent vagal system. Auton. Neurosci. 85, 1–17. doi: 10.1016/S1566-0702(00)00215-0
Beste, C., Steenbergen, L., Sellaro, R., Grigoriadou, S., Zhang, R., Chmielewski, W., et al. (2016). Effects of concomitant stimulation of the GABAergic and norepinephrine system on inhibitory control – a study using transcutaneous vagus nerve stimulation. Brain Stimul. 9, 811–818. doi: 10.1016/J.BRS.2016.07.004
Bohning, D. E., Lomarev, M. P., Denslow, S., Nahas, Z., Shastri, A., and George, M. S. (2001). Feasibility of vagus nerve stimulation-synchronized blood oxygenation level-dependent functional MRI. Invest. Radiol. 36, 470–479. doi: 10.1097/00004424-200108000-00006
Borges, U., Knops, L., Laborde, S., Klatt, S., and Raab, M. (2020). Transcutaneous vagus nerve stimulation may enhance only specific aspects of the core executive functions. A randomized crossover trial. Front. Neurosci. 14:523. doi: 10.3389/FNINS.2020.00523
Borges, U., Laborde, S., and Raab, M. (2019). Influence of transcutaneous vagus nerve stimulation on cardiac vagal activity: not different from sham stimulation and no effect of stimulation intensity. PLoS One 14:e0223848. doi: 10.1371/JOURNAL.PONE.0223848
Bretherton, B., Atkinson, L., Murray, A., Clancy, J., Deuchars, S., and Deuchars, J. (2019). Effects of transcutaneous vagus nerve stimulation in individuals aged 55 years or above: potential benefits of daily stimulation. Aging 11, 4836–4857. doi: 10.18632/aging.102074
Broncel, A., Bocian, R., Kłos-Wojtczak, P., Kulbat-Warycha, K., and Konopacki, J. (2020). Vagal nerve stimulation as a promising tool in the improvement of cognitive disorders. Brain Res. Bull. 155, 37–47. doi: 10.1016/j.brainresbull.2019.11.011
Burger, A. M., Van Diest, I., Van der Does, W., Korbee, J. N., Waziri, N., Brosschot, J. F., et al. (2019). The effect of transcutaneous vagus nerve stimulation on fear generalization and subsequent fear extinction. Neurobiol. Learn. Mem. 161, 192–201. doi: 10.1016/J.NLM.2019.04.006
Cakmak, Y. O., Apaydin, H., Kiziltan, G., Gündüz, A., Ozsoy, B., Olcer, S., et al. (2017). Rapid alleviation of parkinson’s disease symptoms via electrostimulation of intrinsic auricular muscle zones. Front. Hum. Neurosci. 11:338. doi: 10.3389/FNHUM.2017.00338
Camina, E., and Güell, F. (2017). The neuroanatomical, neurophysiological and psychological basis of memory: current models and their origins. Front. Pharmacol. 8:438. doi: 10.3389/FPHAR.2017.00438
Chae, J. H., Nahas, Z., Lomarev, M., Denslow, S., Lorberbaum, J. P., Bohning, D. E., et al. (2003). A review of functional neuroimaging studies of vagus nerve stimulation (VNS). J. Psychiatric Res. 37, 443–455. doi: 10.1016/S0022-3956(03)00074-8
Chalmers, J. A., Quintana, D. S., Abbott, M. J. A., and Kemp, A. H. (2014). Anxiety disorders are associated with reduced heart rate variability: a meta-analysis. Front. Psychiatry 5:80. doi: 10.3389/FPSYT.2014.00080
Chambers, C. D., Garavan, H., and Bellgrove, M. A. (2009). Insights into the neural basis of response inhibition from cognitive and clinical neuroscience. Neurosci. Biobehav. Rev. 33, 631–646. doi: 10.1016/J.NEUBIOREV.2008.08.016
Cheng, Y. C., Huang, Y. C., and Huang, W. L. (2022). Heart rate variability in patients with dementia or neurocognitive disorders: a systematic review and meta-analysis. Aust. N. Z. J. Psychiatry 56, 16–27. doi: 10.1177/0004867420976853
Clancy, J. A., Mary, D. A., Witte, K. K., Greenwood, J. P., Deuchars, S. A., and Deuchars, J. (2014). Non-invasive Vagus nerve stimulation in healthy humans reduces sympathetic nerve activity. Brain Stimul. 7, 871–877.
Clark, K. B., Krahl, S. E., Smith, D. C., and Jensen, R. A. (1995). Post-training unilateral vagal stimulation enhances retention performance in the rat. Neurobiol. Learn. Mem. 63, 213–216. doi: 10.1006/NLME.1995.1024
Clark, K. B., Naritoku, D. K., Smith, D. C., Browning, R. A., and Jensen, R. A. (1999). Enhanced recognition memory following vagus nerve stimulation in human subjects. Nat. Neurosci. 2, 94–98. doi: 10.1038/4600
Clewett, D. V., Lee, T. H., Greening, S., Ponzio, A., Margalit, E., and Mather, M. (2016). Neuromelanin marks the spot: identifying a locus coeruleus biomarker of cognitive reserve in healthy aging. Neurobiol. Aging 37:117. doi: 10.1016/J.NEUROBIOLAGING.2015.09.019
Collins, O., Dillon, S., Finucane, C., Lawlor, B., and Kenny, R. A. (2012). Parasympathetic autonomic dysfunction is common in mild cognitive impairment. Neurobiol. Aging 33, 2324–2333. doi: 10.1016/J.NEUROBIOLAGING.2011.11.017
Colzato, L., and Beste, C. (2020). A literature review on the neurophysiological underpinnings and cognitive effects of transcutaneous vagus nerve stimulation: challenges and future directions. J. Neurophysiol. 123, 1739–1755. doi: 10.1152/jn.00057.2020.-Brain
Colzato, L. S., Ritter, S. M., and Steenbergen, L. (2018). Transcutaneous vagus nerve stimulation (tVNS) enhances divergent thinking. Neuropsychologia 111, 72–76. doi: 10.1016/J.NEUROPSYCHOLOGIA.2018.01.003
Colzato, L. S., Sellaro, R., and Beste, C. (2017). Darwin revisited: the vagus nerve is a causal element in controlling recognition of other’s emotions. Cortex 92, 95–102. doi: 10.1016/J.CORTEX.2017.03.017
Conway, C. R., Sheline, Y. I., Chibnall, J. T., Bucholz, R. D., Price, J. L., Gangwani, S., et al. (2012). Brain blood-flow change with acute vagus nerve stimulation in treatment-refractory major depressive disorder. Brain Stimul. 5, 163–171. doi: 10.1016/J.BRS.2011.03.001
Coote, J. H. (2013). Myths and realities of the cardiac vagus. J. Physiol. 591, 4073–4085. doi: 10.1113/JPHYSIOL.2013.257758
Cummings, J., Lee, G., Zhong, K., Fonseca, J., and Taghva, K. (2021). Alzheimer’s disease drug development pipeline: 2021. Alzheimer’s Dementia Transl. Res. Clin. Intervent. 7:e12179. doi: 10.1002/TRC2.12179
da Silva, V. P., Ramalho Oliveira, B. R., Tavares Mello, R. G., Moraes, H., Deslandes, A. C., and Laks, J. (2017). Heart rate variability indexes in dementia: a systematic review with a quantitative analysis. Curr. Alzheimer Res. 15, 80–88. doi: 10.2174/1567205014666170531082352
de Couck, M., Cserjesi, R., Caers, R., Zijlstra, W. P., Widjaja, D., Wolf, N., et al. (2017). Effects of short and prolonged transcutaneous vagus nerve stimulation on heart rate variability in healthy subjects. Autonomic Neurosci. Basic Clin. 203, 88–96. doi: 10.1016/j.autneu.2016.11.003
de Couck, M., Mravec, B., and Gidron, Y. (2012). You may need the vagus nerve to understand pathophysiology and to treat diseases. Clin. Sci. 122, 323–328. doi: 10.1042/CS20110299
de la Vega, A., Brown, M. S., Snyder, H. R., Singel, D., Munakata, Y., and Banich, M. T. (2014). Individual differences in the balance of GABA to glutamate in pFC predict the ability to select among competing options. J. Cogn. Neurosci. 26, 2490–2502. doi: 10.1162/JOCN_A_00655
Ding, B., Ling, H. W., Zhang, Y., Huang, J., Zhang, H., Wang, T., et al. (2014). Pattern of cerebral hyperperfusion in Alzheimer’s disease and amnestic mild cognitive impairment using voxel-based analysis of 3D arterial spin-labeling imaging: initial experience. Clin. Intervent. Aging 9, 493–500. doi: 10.2147/CIA.S58879
Dixon, M. L. (2015). Cognitive control, emotional value, and the lateral prefrontal cortex. Front. Psychol. 6:758. doi: 10.3389/FPSYG.2015.00758
Dodrill, C. B., and Morris, G. L. (2001). Effects of vagal nerve stimulation on cognition and quality of life in epilepsy. Epilepsy Behav. 2, 46–53. doi: 10.1006/EBEH.2000.0148
Duann, J. R., Ide, J. S., Luo, X., and Li, C. S. R. (2009). Functional connectivity delineates distinct roles of the inferior frontal cortex and presupplementary motor area in stop signal inhibition. J. Neurosci. 29, 10171–10179. doi: 10.1523/JNEUROSCI.1300-09.2009
Eggenberger, P., Annaheim, S., Kündig, K. A., Rossi, R. M., Münzer, T., and de Bruin, E. D. (2020). Heart rate variability mainly relates to cognitive executive functions and improves through exergame training in older adults: a secondary analysis of a 6-month randomized controlled trial. Front. Aging Neurosci. 12:197. doi: 10.3389/FNAGI.2020.00197
Entschladen, F., Drell, T. L., Lang, K., Joseph, J., and Zaenker, K. S. (2004). Tumour-cell migration, invasion, and metastasis: navigation by neurotransmitters. Lancet Oncol. 5, 254–258. doi: 10.1016/S1470-2045(04)01431-7
Eriksen, C. W. (1995). The flankers task and response competition: a useful tool for investigating a variety of cognitive problems. Visual Cogn. 2, 101–118. doi: 10.1080/13506289508401726
Erlanger, J., and Gasser, H. (1937). Electrical signs of nervous activity. Am. J. Psychiatry 93, 1472–a–1473. doi: 10.1176/AJP.93.6.1472-A
Farmer, A. D., Strzelczyk, A., Finisguerra, A., Gourine, A. V., Gharabaghi, A., Hasan, A., et al. (2021). International consensus based review and recommendations for minimum reporting standards in research on transcutaneous vagus nerve stimulation (Version 2020). Front. Hum. Neurosci. 14:568051. doi: 10.3389/FNHUM.2020.568051
Fischer, R., Ventura-Bort, C., Hamm, A., and Weymar, M. (2018). Transcutaneous vagus nerve stimulation (tVNS) enhances conflict-triggered adjustment of cognitive control. Cogn. Affect. Behav. Neurosci. 18, 680–693. doi: 10.3758/s13415-018-0596-2
Foley, J., and Dubois, F. S. (1937). Quantitative studies of the vagus nerve in the cat. I. The ratio of sensory to motor fibres. J. Comp. Neurol. 67, 49–64.
Forte, G., Favieri, F., and Casagrande, M. (2019). Heart rate variability and cognitive function: a systematic review. Front. Neurosci. 13:710. doi: 10.3389/FNINS.2019.00710
Frangos, E., Ellrich, J., and Komisaruk, B. R. (2015). Non-invasive access to the vagus nerve central projections via electrical stimulation of the external ear: fMRI evidence in humans. Brain Stimul. 8, 624–636. doi: 10.1016/J.BRS.2014.11.018
Frangos, E., and Komisaruk, B. R. (2017). Access to vagal projections via cutaneous electrical stimulation of the neck: fMRI evidence in healthy humans. Brain Stimul. 10, 19–27. doi: 10.1016/J.BRS.2016.10.008
Frewen, J., Finucane, C., Savva, G. M., Boyle, G., Coen, R. F., and Kenny, R. A. (2013). Cognitive function is associated with impaired heart rate variability in ageing adults: the Irish longitudinal study on ageing wave one results. Clin. Autonomic Res. 23, 313–323. doi: 10.1007/S10286-013-0214-X
Furlan, R., Heusser, K., Minonzio, M., Shiffer, D., Cairo, B., Tank, J., et al. (2019). Cardiac and vascular sympathetic baroreflex control during orthostatic pre-syncope. J. Clin. Med. 8:1434. doi: 10.3390/JCM8091434
Galli, R., Limbruno, U., Pizzanelli, C., Giorgi, F. S., Lutzemberger, L., Strata, G., et al. (2003). Analysis of RR variability in drug-resistant epilepsy patients chronically treated with vagus nerve stimulation. Autonomic Neurosci. Basic Clin. 107, 52–59. doi: 10.1016/S1566-0702(03)00081-X
Gao, F., Edden, R. A. E., Li, M., Puts, N. A. J., Wang, G., Liu, C., et al. (2013). Edited magnetic resonance spectroscopy detects an age-related decline in brain GABA levels. Neuroimage 78, 75–82. doi: 10.1016/j.neuroimage.2013.04.012
Gatti, P. J., Johnson, T. A., and Massari, V. J. (1996). Can neurons in the nucleus ambiguus selectively regulate cardiac rate and atrio-ventricular conduction? J. Autonomic Nerv. Syst. 57, 123–127. doi: 10.1016/0165-1838(95)00104-2
Gauthey, A., Morra, S., van de Borne, P., Deriaz, D., Maes, N., and le Polain de Waroux, J. B. (2020). Sympathetic effect of auricular transcutaneous vagus nerve stimulation on healthy subjects: a crossover controlled clinical trial comparing vagally mediated and active control stimulation using microneurography. Front. Physiol. 11:599896. doi: 10.3389/FPHYS.2020.599896
Ghacibeh, G. A., Shenker, J. I., Shenal, B., Uthman, B. M., and Heilman, K. M. (2006a). Effect of vagus nerve stimulation on creativity and cognitive flexibility. Epilepsy Behav. 8, 720–725. doi: 10.1016/j.yebeh.2006.03.008
Ghacibeh, G. A., Shenker, J. I., Shenal, B., Uthman, B. M., and Heilman, K. M. (2006b). The influence of vagus nerve stimulation on memory. Cogn. Behav. Neurol. Off. J. Soc. Behav. Cogn. Neurol. 19, 119–122. doi: 10.1097/01.WNN.0000213908.34278.7D
Giraudier, M., Ventura-Bort, C., and Weymar, M. (2020). Transcutaneous Vagus Nerve Stimulation (tVNS) improves high-confidence recognition memory but not emotional word processing. Front. Psychol. 11:1276. doi: 10.3389/FPSYG.2020.01276
Goadsby, P. J., Grosberg, B. M., Mauskop, A., Cady, R., and Simmons, K. A. (2014). Effect of noninvasive vagus nerve stimulation on acute migraine: an open-label pilot study. Cephalalgia 34, 986–993. doi: 10.1177/0333102414524494
Gonthier, C. (2014). Cognitive Control in Working Memory: an Individual Differences Approach Based on the Dual Mechanisms of Control Framework. Saint-Martin-d’Hères: University of Grenoble.
Grässler, B., Hökelmann, A., and Cabral, R. H. (2020). Resting heart rate variability as a possibly marker of cognitive decline. Kinesiology 52, 72–84. doi: 10.26582/k.52.1.9
Groves, D. A., Bowman, E. M., and Brown, V. J. (2005). Recordings from the rat locus coeruleus during acute vagal nerve stimulation in the anaesthetised rat. Neurosci. Lett. 379, 174–179. doi: 10.1016/j.neulet.2004.12.055
Haensel, A., Mills, P. J., Nelesen, R. A., Ziegler, M. G., and Dimsdale, J. E. (2008). The relationship between heart rate variability and inflammatory markers in cardiovascular diseases. Psychoneuroendocrinology 33, 1305–1312. doi: 10.1016/J.PSYNEUEN.2008.08.007
Hämmerer, D., Callaghan, M. F., Hopkins, A., Kosciessa, J., Betts, M., Cardenas-Blanco, A., et al. (2018). Locus coeruleus integrity in old age is selectively related to memories linked with salient negative events. Proc. Natl. Acad. Sci. U.S.A. 115, 2228–2233. doi: 10.1073/PNAS.1712268115
Handforth, A., DeGiorgio, C. M., Schachter, S. C., Uthman, B. M., Naritoku, D. K., Tecoma, E. S., et al. (1998). Vagus nerve stimulation therapy for partial-onset seizures: a randomized active-control trial. Neurology 51, 48–55. doi: 10.1212/WNL.51.1.48
Hansen, A. L., Johnsen, B. H., Sollers, J. J., Stenvik, K., and Thayer, J. F. (2004). Heart rate variability and its relation to prefrontal cognitive function: the effects of training and detraining. Eur. J. Appl. Physiol. 93, 263–272. doi: 10.1007/S00421-004-1208-0
Hansen, N. (2019). Memory reinforcement and attenuation by activating the human locus coeruleus via transcutaneous vagus nerve stimulation. Front. Neurosci. 13:955. doi: 10.3389/FNINS.2018.00955
Hasan, A., Wolff-Menzler, C., Pfeiffer, S., Falkai, P., Weidinger, E., Jobst, A., et al. (2015). Transcutaneous noninvasive vagus nerve stimulation (tVNS) in the treatment of schizophrenia: a bicentric randomized controlled pilot study. Eur. Arch. Psychiatry Clin. Neurosci. 265, 589–600. doi: 10.1007/S00406-015-0618-9
Hays, S. A., Rennaker, R. L., and Kilgard, M. P. (2013). Targeting plasticity with vagus nerve stimulation to treat neurological disease. Progr. Brain Res. 207, 275–299. doi: 10.1016/B978-0-444-63327-9.00010-2
Helmstaedter, C., Hoppe, C., and Elger, C. E. (2001). Memory alterations during acute high-intensity vagus nerve stimulation. Epilepsy Res. 47, 37–42. doi: 10.1016/S0920-1211(01)00291-1
Henry, T. R. (2002). Therapeutic mechanisms of vagus nerve stimulation. Neurology 59(Suppl. 4), S3–S14. doi: 10.1212/WNL.59.6_SUPPL_4.S3
Henry, T. R., Bakay, R. A. E., Pennell, P. B., Epstein, C. M., and Votaw, J. R. (2004). Brain blood-flow alterations induced by therapeutic vagus nerve stimulation in partial epilepsy: II. Prolonged effects at high and low levels of stimulation. Epilepsia 45, 1064–1070. doi: 10.1111/J.0013-9580.2004.03104.X
Henry, T. R., Bakay, R. A. E., Votaw, J. R., Pennell, P. B., Epstein, C. M., Faber, T. L., et al. (1998). Brain blood flow alterations induced by therapeutic vagus nerve stimulation in partial epilepsy: I. Acute effects at high and low levels of stimulation. Epilepsia 39, 983–990. doi: 10.1111/J.1528-1157.1998.TB01448.X
Holzman, J. B., and Bridgett, D. J. (2017). Heart rate variability indices as bio-markers of top-down self-regulatory mechanisms: a meta-analytic review. Neurosci. Biobehav. Rev. 74, 233–255. doi: 10.1016/J.NEUBIOREV.2016.12.032
Huang, F., Dong, J., Kong, J., Wang, H., Meng, H., Spaeth, R. B., et al. (2014). Effect of transcutaneous auricular vagus nerve stimulation on impaired glucose tolerance: a pilot randomized study. BMC Complement. Alternat. Med. 14:203. doi: 10.1186/1472-6882-14-203
Huang, J., Wang, Y., Jiang, D., Zhou, J., and Huang, X. (2010). The sympathetic-vagal balance against endotoxemia. J. Neural Transm. 117, 729–735. doi: 10.1007/S00702-010-0407-6
Hyvarinen, P., Yrttiaho, S., Lehtimaki, J., Ilmoniemi, R. J., Makitie, A., Ylikoski, J., et al. (2015). Transcutaneous vagus nerve stimulation modulates tinnitus-related beta-and gamma-band activity. Ear Hear. 36, e76–e85. doi: 10.1097/AUD.0000000000000123
Iwao, T., Yonemochi, H., Nakagawa, M., Takahashi, N., Saikawa, T., and Ito, M. (2000). Effect of constant and intermittent vagal stimulation on the heart rate and heart rate variability in rabbits. Jpn. J. Physiol. 50, 33–39. doi: 10.2170/JJPHYSIOL.50.33
Jacob, G., Diedrich, L., Sato, K., Brychta, R. J., Raj, S. R., Robertson, D., et al. (2019). Vagal and sympathetic function in neuropathic postural tachycardia syndrome. Hypertension 73, 1087–1096. doi: 10.1161/HYPERTENSIONAHA.118.11803/FORMAT/EPUB
Jacobs, H. I. L., Becker, J. A., Kwong, K., Engels-Domínguez, N., Prokopiou, P. C., Papp, K. V., et al. (2021). In vivo and neuropathology data support locus coeruleus integrity as indicator of Alzheimer’s disease pathology and cognitive decline. Sci. Transl. Med. 13:eabj2511. doi: 10.1126/scitranslmed.abj2511
Jacobs, H. I. L., Riphagen, J. M., Razat, C. M., Wiese, S., and Sack, A. T. (2015). Transcutaneous vagus nerve stimulation boosts associative memory in older individuals. Neurobiol. Aging 36, 1860–1867. doi: 10.1016/j.neurobiolaging.2015.02.023
Johnson, N. A., Jahng, G. H., Weiner, M. W., Miller, B. L., Chui, H. C., Jagust, W. J., et al. (2005). Pattern of cerebral hypoperfusion in Alzheimer disease and mild cognitive impairment measured with arterial spin-labeling MR imaging: initial experience. Radiology 234, 851–859. doi: 10.1148/RADIOL.2343040197
Jongkees, B. J., Immink, M. A., Finisguerra, A., and Colzato, L. S. (2018). Transcutaneous vagus nerve stimulation (tVNS) Enhances response selection during sequential action. Front. Psychol. 9:1159. doi: 10.3389/FPSYG.2018.01159
Kaan, E., De Aguiar, I., Clarke, C., Lamb, D. G., Williamson, J. B., and Porges, E. C. (2021). A transcutaneous vagus nerve stimulation study on verbal order memory. J. Neurolinguist. 59:100990. doi: 10.1016/j.jneuroling.2021.100990
Kalia, M., and Sullivan, J. M. (1982). Brainstem projections of sensory and motor components of the vagus nerve in the rat. J. Comparat. Neurol. 211, 248–264. doi: 10.1002/CNE.902110304
Kamath, M., Upton, A., Talalla, A., and Fallen, E. (1992). Neurocardiac responses to vagoafferent electrostimulation in humans. Pacing Clin. Electrophysiol. PACE 15(Pt 2), 1581–1587. doi: 10.1111/J.1540-8159.1992.TB02937.X
Kaniusas, E., Kampusch, S., Tittgemeyer, M., Panetsos, F., Gines, R. F., Papa, M., et al. (2019). Current directions in the auricular vagus nerve stimulation II - An engineering perspective. Front. Neurosci. 13:772. doi: 10.3389/FNINS.2019.00772
Kealy, J., and Commins, S. (2010). Frequency-dependent changes in synaptic plasticity and brain-derived neurotrophic factor (BDNF) expression in the CA1 to perirhinal cortex projection. Brain Res. 1326, 51–61. doi: 10.1016/j.brainres.2010.02.065
Keute, M., Barth, D., Liebrand, M., Heinze, H.-J., Kraemer, U., and Zaehle, T. (2020). Effects of Transcutaneous Vagus Nerve Stimulation (tVNS) on conflict-related behavioral performance and frontal midline theta activity. J. Cogn. Enhanc. 4, 121–130. doi: 10.1007/S41465-019-00152-5
Ko, D., Heck, C., Grafton, S., Apuzzo, M. L. J., Couldwell, W. T., Chen, T., et al. (1996). Vagus nerve stimulation activates central nervous system structures in epileptic patients during PET H215O blood flow imaging. Neurosurgery 39, 426–431. doi: 10.1097/00006123-199608000-00061
Koenig, J., Parzer, P., Haigis, N., Liebemann, J., Jung, T., Resch, F., et al. (2021). Effects of acute transcutaneous vagus nerve stimulation on emotion recognition in adolescent depression. Psychol. Med. 51, 511–520. doi: 10.1017/S0033291719003490
Koizumi, K., Terui, N., and Kollai, M. (1983). Neural control of the heart: significance of double innervation re-examined. J. Autonomic Nerv. Syst. 7, 279–294. doi: 10.1016/0165-1838(83)90081-4
Kornblum, S., Hasbroucq, T., and Osman, A. (1990). Dimensional overlap: cognitive basis for stimulus-response compatibility-a model and taxonomy. Psychol. Rev. 97, 253–270. doi: 10.1037/0033-295X.97.2.253
Kosel, M., Brockmann, H., Frick, C., Zobel, A., and Schlaepfer, T. E. (2011). Chronic vagus nerve stimulation for treatment-resistant depression increases regional cerebral blood flow in the dorsolateral prefrontal cortex. Psychiatry Res. Neuroimaging 191, 153–159. doi: 10.1016/J.PSCYCHRESNS.2010.11.004
Kraus, T., Hösl, K., Kiess, O., Schanze, A., Kornhuber, J., and Forster, C. (2007). BOLD fMRI deactivation of limbic and temporal brain structures and mood enhancing effect by transcutaneous vagus nerve stimulation. J. Neural Transm. 114, 1485–1493. doi: 10.1007/S00702-007-0755-Z
Kraus, T., Kiess, O., Hösl, K., Terekhin, P., Kornhuber, J., and Forster, C. (2013). CNS BOLD fMRI effects of sham-controlled transcutaneous electrical nerve stimulation in the left outer auditory canal - A pilot study. Brain Stimul. 6, 798–804. doi: 10.1016/j.brs.2013.01.011
Kunii, N., Koizumi, T., Kawai, K., Shimada, S., and Saito, N. (2021). Vagus nerve stimulation amplifies task-induced cerebral blood flow increase. Front. Hum. Neurosci. 15:726087. doi: 10.3389/FNHUM.2021.726087
Lam, J., Williams, M., Ashla, M., and Lee, D. J. (2021). Cognitive outcomes following vagus nerve stimulation, responsive neurostimulation and deep brain stimulation for epilepsy: a systematic review. Epilepsy Res. 172:106591. doi: 10.1016/J.EPLEPSYRES.2021.106591
Lamb, D. G., Porges, E. C., Lewis, G. F., and Williamson, J. B. (2017). Non-invasive vagal nerve stimulation effects on hyperarousal and autonomic state in patients with Posttraumatic Stress Disorder and history of mild traumatic brain injury: preliminary evidence. Front. Med. 4:124. doi: 10.3389/FMED.2017.00124
Lanska, D. J. (2002). J.L. Corning and vagal nerve stimulation for seizures in the 1880s. Neurology 58, 452–459. doi: 10.1212/WNL.58.3.452
Laqua, R., Leutzow, B., Wendt, M., and Usichenko, T. (2014). Transcutaneous vagal nerve stimulation may elicit anti- and pro-nociceptive effects under experimentally-induced pain - a crossover placebo-controlled investigation. Autonomic Neurosci. Basic Clin. 185, 120–122. doi: 10.1016/J.AUTNEU.2014.07.008
Liu, D., and Hu, Y. (1988). The central projections of the great auricular nerve primary afferent fibers - an HRP transganglionic tracing method. Brain Res. 445, 205–210. doi: 10.1016/0006-8993(88)91179-1
Liu, K. Y., Elliott, T., Knowles, M., and Howard, R. (2022). Heart rate variability in relation to cognition and behavior in neurodegenerative diseases: a systematic review and meta-analysis. Ageing Res. Rev. 73:101539. doi: 10.1016/J.ARR.2021.101539
Liu, W. C., Mosier, K., Kalnin, A. J., and Marks, D. (2003). BOLD fMRI activation induced by vagus nerve stimulation in seizure patients. J. Neurol. Neurosurg. Psychiatry 74, 811–813. doi: 10.1136/JNNP.74.6.811
Llewellyn-Smith, I. J., and Verberne, A. J. M. (2011). Central Regulation of Autonomic Functions. Oxford: Oxford University, 1–400.
Lomarev, M., Denslow, S., Nahas, Z., Chae, J. H., George, M. S., and Bohning, D. E. (2002). Vagus nerve stimulation (VNS) synchronized BOLD fMRI suggests that VNS in depressed adults has frequency/dose dependent effects. J. Psychiatric Res. 36, 219–227. doi: 10.1016/S0022-3956(02)00013-4
Machetanz, K., Berelidze, L., Guggenberger, R., and Gharabaghi, A. (2021). Transcutaneous auricular vagus nerve stimulation and heart rate variability: analysis of parameters and targets. Autonomic Neurosci. Basic Clin. 236:102894. doi: 10.1016/j.autneu.2021.102894
MacLeod, C. M. (1991). Half a century of reseach on the stroop effect: an integrative review. Psychol. Bull. 109, 163–203. doi: 10.1037/0033-2909.109.2.163
Maharjan, A., Wang, E., Peng, M., and Cakmak, Y. O. (2018). Improvement of olfactory function with high frequency non-invasive auricular electrostimulation in healthy humans. Front. Neurosci. 12:225. doi: 10.3389/FNINS.2018.00225
Martin, C. O., Denburg, N. L., Tranel, D., Granner, M. A., and Bechara, A. (2004). The effects of vagus nerve stimulation on decision-making. Cortex 40, 605–612. doi: 10.1016/S0010-9452(08)70156-4
Massari, V. J., Johnson, T. A., and Gatti, P. J. (1995). Cardiotopic organization of the nucleus ambiguus? An anatomical and physiological analysis of neurons regulating atrioventricular conduction. Brain Res. 679, 227–240. doi: 10.1016/0006-8993(95)00227-H
Mazza, M., Marano, G., Traversi, G., Bria, P., and Mazza, S. (2011). Primary cerebral blood flow deficiency and Alzheimer’s disease: shadows and lights. J. Alzheimer’s Dis. 23, 375–389. doi: 10.3233/JAD-2010-090700
McGlone, J., Valdivia, I., Penner, M., Williams, J., Sadler, R. M., and Clarke, D. B. (2008). Quality of life and memory after vagus nerve stimulator implantation for epilepsy. Can. J. Neurol. Sci. J. Can. Sci. Neurol. 35, 287–296. doi: 10.1017/S0317167100008854
McIntire, L. K., McKinley, R. A., Goodyear, C., McIntire, J. P., and Brown, R. D. (2021). Cervical transcutaneous vagal nerve stimulation (ctVNS) improves human cognitive performance under sleep deprivation stress. Commun. Biol. 4:634. doi: 10.1038/s42003-021-02145-7
Mercante, B., Deriu, F., and Rangon, C.-M. (2018). Auricular neuromodulation: the emerging concept beyond the stimulation of vagus and trigeminal nerves. Medicines 5:10. doi: 10.3390/medicines5010010
Merrick, J., Grippo, A. J., Bartlett, G., Shaffer, F., and Ginsberg, J. P. (2017). An overview of heart rate variability metrics and norms. Front. Public Health 5:258. doi: 10.3389/fpubh.2017.00258
Merrill, C. A., Jonsson, M. A. G., Minthon, L., Ejnell, H., Silander, H. C. S., Blennow, K., et al. (2006). Vagus nerve stimulation in patients with Alzheimer’s disease: additional follow-up results of a pilot study through 1 year. J. Clin. Psychiatry 67, 1171–1178. doi: 10.4088/JCP.V67N0801
Mertens, A., Naert, L., Miatton, M., Poppa, T., Carrette, E., Gadeyne, S., et al. (2020). Transcutaneous vagus nerve stimulation does not affect verbal memory performance in healthy volunteers. Front. Psychol. 11:551. doi: 10.3389/FPSYG.2020.00551
Montano, N., Porta, A., Cogliati, C., Costantino, G., Tobaldini, E., Casali, K. R., et al. (2009). Heart rate variability explored in the frequency domain: a tool to investigate the link between heart and behavior. Neurosci. Biobehav. Rev. 33, 71–80. doi: 10.1016/J.NEUBIOREV.2008.07.006
Morris, G. L., and Mueller, W. M. (1999). Long-term treatment with vagus nerve stimulation in patients with refractory epilepsy. The vagus nerve stimulation study group E01-E05. Neurology 53, 1731–1735. doi: 10.1212/WNL.53.8.1731
Mu, Q., Bohning, D. E., Nahas, Z., Walker, J., Anderson, B., Johnson, K. A., et al. (2004). Acute vagus nerve stimulation using different pulse widths produces varying brain effects. Biol. Psychiatry 55, 816–825. doi: 10.1016/J.BIOPSYCH.2003.12.004
Munakata, Y., Herd, S. A., Chatham, C. H., Depue, B. E., Banich, M. T., and O’Reilly, R. C. (2011). A unified framework for inhibitory control. Trends Cogn. Sci. 15:453. doi: 10.1016/J.TICS.2011.07.011
Narayanan, J. T., Watts, R., Haddad, N., Labar, D. R., Li, P. M., and Filippi, C. G. (2002). Cerebral activation during vagus nerve stimulation: a functional MR study. Epilepsia 43, 1509–1514. doi: 10.1046/J.1528-1157.2002.16102.X
Naveh-Benjamin, M. (2000). Adult age differences in memory performance: tests of an associative deficit hypothesis. J. Exp. Psychol. Learn. Mem. Cogn. 26, 1170–1187. doi: 10.1037/0278-7393.26.5.1170
Neftel, W. B. (1871). Galvano-Therapeutics. The Physiological and Therapeutical Action of the Galvanic Current Upon the Acoustic, Optic, Sympathetic, and Pneumogastric Nerves. New York, NY: D Appleton & Company.
Nesbitt, A. D., Marin, J. C. A., Tompkins, E., Ruttledge, M. H., and Goadsby, P. J. (2015). Initial use of a novel noninvasive vagus nerve stimulator for cluster headache treatment. Neurology 84, 1249–1253. doi: 10.1212/WNL.0000000000001394
Nichols, E., Steinmetz, J. D., Vollset, S. E., Fukutaki, K., Chalek, J., Abd-Allah, F., et al. (2022). Estimation of the global prevalence of dementia in 2019 and forecasted prevalence in 2050: an analysis for the Global Burden of Disease Study 2019. Lancet Public Health 7, e105–e125. doi: 10.1016/S2468-2667(21)00249-8/ATTACHMENT/60E03FD1-38B2-4B40-A91D-9AFDDA22B45E/MMC1.PDF
Obst, M. A., Heldmann, M., Alicart, H., Tittgemeyer, M., and Münte, T. F. (2020). Effect of Short-Term Transcutaneous Vagus Nerve Stimulation (tVNS) on brain processing of food cues: an electrophysiological study. Front. Hum. Neurosci. 14:206. doi: 10.3389/FNHUM.2020.00206
Öngür, D., and Price, J. L. (2000). The organization of networks within the orbital and medial prefrontal cortex of rats, monkeys and humans. Cereb. Cortex (New York, N.Y.: 1991) 10, 206–219. doi: 10.1093/CERCOR/10.3.206
O’Reardon, J. P., Cristancho, P., and Peshek, A. D. (2006). Vagus Nerve Stimulation (VNS) and treatment of depression: to the brainstem and beyond. Psychiatry (Edgmont) 3:54.
Perini, G. I., Toffanin, T., Pigato, G., Ferri, G., Follador, H., Zonta, F., et al. (2017). Hippocampal gray volumes increase in treatment-resistant depression responding to vagus nerve stimulation. J. ECT 33, 160–166. doi: 10.1097/YCT.0000000000000424
Petelin Gadze, Z., Bujan Kovac, A., Adamec, I., Milekic, N., and Sulentic, V. (2018). Vagal nerve stimulation is beneficial in postural orthostatic tachycardia syndrome and epilepsy. Seizure 57, 11–13. doi: 10.1016/J.SEIZURE.2018.03.001
Peuker, E. T., and Filler, T. J. (2002). The nerve supply of the human auricle. Clin. Anatomy 15, 35–37. doi: 10.1002/ca.1089
Pickering, T. G., and Davies, J. (1973). Estimation of the conduction time of the baroreceptor-cardiac reflex in man. Cardiovasc. Res. 7, 213–219. doi: 10.1093/CVR/7.2.213
Polak, T., Markulin, F., Ehlis, A. C., Langer, J. B. M., Ringel, T. M., and Fallgatter, A. J. (2009). Far field potentials from brain stem after transcutaneous Vagus nerve stimulation: optimization of stimulation and recording parameters. J. Neural Transm. 116, 1237–1242. doi: 10.1007/S00702-009-0282-1
Porges, E. C., Woods, A. J., Edden, R. A. E., Puts, N. A. J., Harris, A. D., Chen, H., et al. (2017). Frontal gamma-aminobutyric acid concentrations are associated with cognitive performance in older adults. Biol. Psychiatry Cogn. Neurosci. Neuroimaging 2:38. doi: 10.1016/J.BPSC.2016.06.004
Ridgewell, C., Heaton, K. J., Hildebrandt, A., Couse, J., Leeder, T., and Neumeier, W. H. (2021). The effects of transcutaneous auricular vagal nerve stimulation on cognition in healthy individuals: a meta-analysis. Neuropsychology 35, 352–365. doi: 10.1037/NEU0000735
Roges, R. C., Kita, H., Butcher, L. L., and Novin, D. (1980). Afferent projections to the dorsal motor nucleus of the vagus. Brain Res. Bull. 5, 365–373. doi: 10.1016/S0361-9230(80)80006-2
Ronkainen, E., Korpelainen, J. T., Heikkinen, E., Myllylä, V. V., Huikuri, H. V., and Isojärvi, J. I. T. (2006). Cardiac autonomic control in patients with refractory epilepsy before and during vagus nerve stimulation treatment: a one-year follow-up study. Epilepsia 47, 556–562. doi: 10.1111/J.1528-1167.2006.00467.X
Rufener, K. S., Geyer, U., Janitzky, K., Heinze, H. J., and Zaehle, T. (2018). Modulating auditory selective attention by non-invasive brain stimulation: differential effects of transcutaneous vagal nerve stimulation and transcranial random noise stimulation. Eur. J. Neurosci. 48, 2301–2309. doi: 10.1111/ejn.14128
Rutecki, P. (1990). Anatomical, physiological, and theoretical basis for the antiepileptic effect of vagus nerve stimulation. Epilepsia 31(Suppl. 2), S1–S6. doi: 10.1111/J.1528-1157.1990.TB05843.X
Sakaki, M., Yoo, H. J., Nga, L., Lee, T.-H., Thayer, J. F., and Mather, M. (2016). Heart rate variability is associated with amygdala functional connectivity with MPFC across younger and older adults HHS Public Access. Neuroimage 139, 44–52. doi: 10.1016/j.neuroimage.2016.05.076
Saleem, K. S., Kondo, H., and Price, J. L. (2008). Complementary circuits connecting the orbital and medial prefrontal networks with the temporal, insular, and opercular cortex in the macaque monkey. J. Comparat. Neurol. 506, 659–693. doi: 10.1002/CNE.21577
Sandrone, G., Mortara, A., Torzillo, D., la Rovere, M. T., Malliani, A., and Lombardi, F. (1994). Effects of beta blockers (atenolol or metoprolol) on heart rate variability after acute myocardial infarction. Am. J. Cardiol. 74, 340–345. doi: 10.1016/0002-9149(94)90400-6
Sandson, T. A., O’Connor, M., Sperling, R. A., Edelman, R. R., and Warach, S. (1996). Noninvasive perfusion MRI in Alzheimer’s disease: a preliminary report. Neurology 47, 1339–1342. doi: 10.1212/WNL.47.5.1339
Saper, C. B. (2000). Hypothalamic connections with the cerebral cortex. Progr. Brain Res. 126, 39–48. doi: 10.1016/S0079-6123(00)26005-6
Schevernels, H., van Bochove, M. E., de Taeye, L., Bombeke, K., Vonck, K., van Roost, D., et al. (2016). The effect of vagus nerve stimulation on response inhibition. Epilepsy Behav. 64, 171–179. doi: 10.1016/J.YEBEH.2016.09.014
Sclocco, R., Garcia, R. G., Kettner, N. W., Isenburg, K., Fisher, H. P., Hubbard, C. S., et al. (2019). The influence of respiration on brainstem and cardiovagal response to auricular vagus nerve stimulation: a multimodal ultrahigh-field (7T) fMRI study. Brain Stimul. 12, 911–921. doi: 10.1016/J.BRS.2019.02.003
Sellaro, R., van Leusden, J. W. R., Tona, K. D., Verkuil, B., Nieuwenhuis, S., and Colzato, L. S. (2015). Transcutaneous vagus nerve stimulation enhances post-error slowing. J. Cogn. Neurosci. 27, 2126–2132. doi: 10.1162/JOCN_A_00851
Setty, A. B., Vaughn, B. V., Quint, S. R., Robertson, K. R., and Messenheimer, J. A. (1998). Heart period variability during vagal nerve stimulation. Seizure 7, 213–217. doi: 10.1016/S1059-1311(98)80038-1
Shaffer, F., and Ginsberg, J. P. (2017). An overview of heart rate variability metrics and norms. Front. Public Health 5:258.
Shaffer, F., McCraty, R., and Zerr, C. L. (2014). A healthy heart is not a metronome: an integrative review of the heart’s anatomy and heart rate variability. Front. Psychol. 5:1040. doi: 10.3389/FPSYG.2014.01040
Sierra-Marcos, A. (2017). Regional cerebral blood flow in mild cognitive impairment and Alzheimer’s disease measured with arterial spin labeling magnetic resonance imaging. Int. J. Alzheimer’s Dis. 2017:5479597. doi: 10.1155/2017/5479597
Sinkovec, M., Trobec, R., and Meglic, B. (2021). Cardiovascular responses to low-level transcutaneous vagus nerve stimulation. Autonomic Neurosci. 236:102851. doi: 10.1016/J.AUTNEU.2021.102851
Sjögren, M. J. C., Hellström, P. T. O., Jonsson, M. A. G., Runnerstam, M., Silander, H. C. S., and Ben-Menachem, E. (2002). Cognition-enhancing effect of vagus nerve stimulation in patients with Alzheimer’s disease: a pilot study. J. Clin. Psychiatry 63, 972–980. doi: 10.4088/jcp.v63n1103
Sperling, W., Reulbach, U., Bleich, S., Padberg, F., Kornhuber, J., and Mueck-Weymann, M. (2010). Cardiac effects of vagus nerve stimulation in patients with major depression. Pharmacopsychiatry 43, 7–11. doi: 10.1055/S-0029-1237374
Squire, L., and Zola, S. (1998). Episodic memory, semantic memory, and amnesia. Hippocampus 8, 205–211.
Stavrakis, S., Humphrey, M. B., Scherlag, B. J., Hu, Y., Jackman, W. M., Nakagawa, H., et al. (2015). Low-level transcutaneous electrical vagus nerve stimulation suppresses atrial fibrillation. J. Am. College Cardiol. 65, 867–875. doi: 10.1016/j.jacc.2014.12.026
Steenbergen, L., Sellaro, R., Stock, A. K., Verkuil, B., Beste, C., and Colzato, L. S. (2015). Transcutaneous vagus nerve stimulation (tVNS) enhances response selection during action cascading processes. Eur. Neuropsychopharmacol. J. Eur. College Neuropsychopharmacol. 25, 773–778. doi: 10.1016/J.EURONEURO.2015.03.015
Stefan, H., Kreiselmeyer, G., Kerling, F., Kurzbuch, K., Rauch, C., Heers, M., et al. (2012). Transcutaneous vagus nerve stimulation (t-VNS) in pharmacoresistant epilepsies: a proof of concept trial. Epilepsia 53, e115–e118. doi: 10.1111/J.1528-1167.2012.03492.X
Stein, P. K., and Kleiger, R. E. (2003). Insights from the study of heart rate variability. Annu. Rev. Med. 50, 249–261. doi: 10.1146/ANNUREV.MED.50.1.249
Steinbrook, R. (2021). The accelerated approval of aducanumab for treatment of patients with Alzheimer disease. JAMA Internal Med. 181:1281. doi: 10.1001/JAMAINTERNMED.2021.4622
Sucholeiki, R., Alsaadi, T. M., Morris, G. L., Ulmer, J. L., Biswal, B., and Mueller, W. M. (2002). fMRI in patients implanted with a vagal nerve stimulator. Seizure 11, 157–162. doi: 10.1053/SEIZ.2001.0601
Sun, J.-B., Cheng, C., Tian, Q.-Q., Yuan, H., Yang, X.-J., Deng, H., et al. (2021). Transcutaneous auricular vagus nerve stimulation improves spatial working memory in healthy young adults. Front. Neurosci. 15:790793. doi: 10.3389/FNINS.2021.790793
Sun, L., Peräkylä, J., Holm, K., Haapasalo, J., Lehtimäki, K., Ogawa, K. H., et al. (2017). Vagus nerve stimulation improves working memory performance. J. Clin. Exp. Neuropsychol. 39, 954–964. doi: 10.1080/13803395.2017.1285869
Sun, P., Zhou, K., Wang, S., Li, P., Chen, S., Lin, G., et al. (2013). Involvement of MAPK/NF-κB signaling in the activation of the cholinergic anti-inflammatory pathway in experimental colitis by chronic vagus nerve stimulation. PLoS One 8:e69424. doi: 10.1371/JOURNAL.PONE.0069424
Tamayo, A., and Siepmann, T. (2021). Regulation of blood flow in the cerebral posterior circulation by parasympathetic nerve fibers: physiological background and possible clinical implications in patients with vertebrobasilar stroke. Front. Neurol. 12:660373. doi: 10.3389/FNEUR.2021.660373
Thayer, J. F., Åhs, F., Fredrikson, M., Sollers, J. J., and Wager, T. D. (2012). A meta-analysis of heart rate variability and neuroimaging studies: implications for heart rate variability as a marker of stress and health. Neurosci. Biobehav. Rev. 36, 747–756. doi: 10.1016/J.NEUBIOREV.2011.11.009
Thayer, J. F., Hansen, A. L., Saus-Rose, E., and Johnsen, B. H. (2009). Heart rate variability, prefrontal neural function, and cognitive performance: the neurovisceral integration perspective on self-regulation, adaptation, and health. Ann. Behav. Med. 37, 141–153. doi: 10.1007/S12160-009-9101-Z
Thayer, J. F., and Lane, R. D. (2007). The role of vagal function in the risk for cardiovascular disease and mortality. Biol. Psychol. 74, 224–242. doi: 10.1016/J.BIOPSYCHO.2005.11.013
Tiego, J., Testa, R., Bellgrove, M. A., Pantelis, C., and Whittle, S. (2018). A hierarchical model of inhibitory control. Front. Psychol. 9:1339. doi: 10.3389/FPSYG.2018.01339
Tobaldini, E., Toschi-Dias, E., Appratto de Souza, L., Rabello Casali, K., Vicenzi, M., Sandrone, G., et al. (2019). Cardiac and peripheral autonomic responses to orthostatic stress during transcutaneous vagus nerve stimulation in healthy subjects. J. Clin. Med. 8:496. doi: 10.3390/jcm8040496
Tsutsumi, T., Ide, T., Yamato, M., Kudou, W., Andou, M., Hirooka, Y., et al. (2008). Modulation of the myocardial redox state by vagal nerve stimulation after experimental myocardial infarction. Cardiovasc. Res. 77, 713–721. doi: 10.1093/CVR/CVM092
Unsworth, N., and Robison, M. K. (2017). A locus coeruleus-norepinephrine account of individual differences in working memory capacity and attention control. Psychonomic Bull. Rev. 24, 1282–1311. doi: 10.3758/S13423-016-1220-5
van Bochove, M. E., de Taeye, L., Raedt, R., Vonck, K., Meurs, A., Boon, P., et al. (2018). Reduced distractor interference during vagus nerve stimulation. Int. J. Psychophysiol. Off. J. Int. Organ. Psychophysiol. 128, 93–99. doi: 10.1016/J.IJPSYCHO.2018.03.015
van den Stock, J., Tamietto, M., Sorger, B., Pichon, S., Grézes, J., and de Gelder, B. (2011). Cortico-subcortical visual, somatosensory, and motor activations for perceiving dynamic whole-body emotional expressions with and without striate cortex (V1). Proc. Natl. Acad. Sci. U.S.A. 108, 16188–16193. doi: 10.1073/PNAS.1107214108/-/DCSUPPLEMENTAL
Vargas-Caballero, M., Warming, H., Walker, R., Holmes, C., Cruickshank, G., and Patel, B. (2022). Vagus nerve stimulation as a potential therapy in Early Alzheimer’s disease: a review. Front. Hum. Neurosci. 16:866434. doi: 10.3389/FNHUM.2022.866434
Ventura-Bort, C., Wirkner, J., Genheimer, H., Wendt, J., Hamm, A. O., and Weymar, M. (2018). Effects of Transcutaneous Vagus Nerve Stimulation (tVNS) on the P300 and alpha-amylase level: a pilot study. Front. Hum. Neurosci. 12:202. doi: 10.3389/fnhum.2018.00202
Ventureyra, E. C. G. (2000). Transcutaneous vagus nerve stimulation for partial onset seizure therapy. A new concept. Child’s Nerv. Syst. ChNS Off. J. Int. Soc. Pediatric Neurosurg. 16, 101–102. doi: 10.1007/S003810050021
Vonck, K., de Herdt, V., Bosman, T., Dedeurwaerdere, S., van Laere, K., and Boon, P. (2008). Thalamic and limbic involvement in the mechanism of action of vagus nerve stimulation, a SPECT study. Seizure 17, 699–706. doi: 10.1016/J.SEIZURE.2008.05.001
Wang, B., Yan, C., Klein, R. M., and Wang, Z. (2018). Inhibition of return revisited: localized inhibition on top of a pervasive bias. Psychonomic Bull. Rev. 25, 1861–1867. doi: 10.3758/S13423-017-1410-9/TABLES/2
Warner, H., and Cox, A. (1962). A mathematical model of heart rate control by sympathetic and vagus efferent information. J. Appl. Physiol. 17, 349–355. doi: 10.1152/JAPPL.1962.17.2.349
Xing, B., Li, Y. C., and Gao, W. J. (2016). Norepinephrine versus dopamine and their interaction in modulating synaptic function in the prefrontal cortex. Brain Res. 1641(Pt B), 217. doi: 10.1016/J.BRAINRES.2016.01.005
Xu, J., Zhang, Y., Qiu, C., and Cheng, F. (2017). Global and regional economic costs of dementia: a systematic review. Lancet 390:S47. doi: 10.1016/S0140-6736(17)33185-9
Yakunina, N., Kim, S. S., and Nam, E. C. (2017). Optimization of transcutaneous vagus nerve stimulation using functional MRI. Neuromodulation 20, 290–300. doi: 10.1111/ner.12541
Yap, J. Y. Y., Keatch, C., Lambert, E., Woods, W., Stoddart, P. R., and Kameneva, T. (2020). Critical review of transcutaneous vagus nerve stimulation: challenges for translation to clinical practice. Front. Neurosci. 14:284. doi: 10.3389/FNINS.2020.00284
Yoshiura, T., Hiwatashi, A., Yamashita, K., Ohyagi, Y., Monji, A., Takayama, Y., et al. (2009). Simultaneous measurement of arterial transit time, arterial blood volume, and cerebral blood flow using arterial spin-labeling in patients with Alzheimer disease. AJNR. Am. J. Neuroradiol. 30, 1388–1393. doi: 10.3174/AJNR.A1562
Yu, Y., Yang, Y., Gan, S., Guo, S., Fang, J., Wang, S., et al. (2021). Cerebral hemodynamic correlates of transcutaneous auricular vagal nerve stimulation in consciousness restoration: an open-label pilot study. Front. Neurol. 12:684791. doi: 10.3389/FNEUR.2021.684791
Zabara, J. (1992). Inhibition of experimental seizures in canines by repetitive vagal stimulation. Epilepsia 33, 1005–1012. doi: 10.1111/J.1528-1157.1992.TB01751.X
Zanchetti, A., Wang, S. C., and Moruzzi, G. (1952). The effect of vagal afferent stimulation on the EEG pattern of the cat. Electroencephalogr. Clin. Neurophysiol. 4, 357–361. doi: 10.1016/0013-4694(52)90064-3
Zeki Al Hazzouri, A., Haan, M. N., Deng, Y., Neuhaus, J., and Yaffe, K. (2014). Reduced heart rate variability is associated with worse cognitive performance in elderly mexican americans. Hypertension 63, 181–187. doi: 10.1161/HYPERTENSIONAHA.113.01888
Zhang, Y., Popović, Z. B., Bibevski, S., Fakhry, I., Sica, D. A., Van Wagoner, D. R., et al. (2009). Chronic vagus nerve stimulation improves autonomic control and attenuates systemic inflammation and heart failure progression in a canine high-rate pacing model. Circ. Heart Fail. 2, 692–699. doi: 10.1161/CIRCHEARTFAILURE.109.873968
Keywords: vagus nerve stimulation, cognition, neurocardiovascular control, cerebral blood flow, LC-NE system, inhibitory control, executive function
Citation: Dolphin H, Dukelow T, Finucane C, Commins S, McElwaine P and Kennelly SP (2022) “The Wandering Nerve Linking Heart and Mind” – The Complementary Role of Transcutaneous Vagus Nerve Stimulation in Modulating Neuro-Cardiovascular and Cognitive Performance. Front. Neurosci. 16:897303. doi: 10.3389/fnins.2022.897303
Received: 17 March 2022; Accepted: 30 May 2022;
Published: 16 June 2022.
Edited by:
Knut Asbjorn Hestad, Hedmark University of Applied Sciences, NorwayReviewed by:
Lin Sørensen, University of Bergen, NorwayCopyright © 2022 Dolphin, Dukelow, Finucane, Commins, McElwaine and Kennelly. This is an open-access article distributed under the terms of the Creative Commons Attribution License (CC BY). The use, distribution or reproduction in other forums is permitted, provided the original author(s) and the copyright owner(s) are credited and that the original publication in this journal is cited, in accordance with accepted academic practice. No use, distribution or reproduction is permitted which does not comply with these terms.
*Correspondence: Helena Dolphin, aGVsZW5hZG9scGhpbkBnbWFpbC5jb20=
Disclaimer: All claims expressed in this article are solely those of the authors and do not necessarily represent those of their affiliated organizations, or those of the publisher, the editors and the reviewers. Any product that may be evaluated in this article or claim that may be made by its manufacturer is not guaranteed or endorsed by the publisher.
Research integrity at Frontiers
Learn more about the work of our research integrity team to safeguard the quality of each article we publish.