- 1Sorbonne Université, INSERM, CNRS, Institut de la Vision, Paris, France
- 2Lab for Retinal Cell Biology, Department of Ophthalmology, University Hospital Zurich, University of Zurich, Zurich, Switzerland
- 3Neuroscience Center Zurich (ZNZ), University of Zurich, Zurich, Switzerland
- 4Faculty of Medicine and Health, Children’s Medical Research Institute, University of Sydney, Westmead, NSW, Australia
- 5Zurich Center for Integrative Human Physiology (ZIHP), University of Zurich, Zurich, Switzerland
The quest for neuroprotective factors that can prevent or slow down the progression of retinal degeneration is still ongoing. Acute hypoxic stress has been shown to provide transient protection against subsequent damage in the retina. Stanniocalcins – STC1 and STC2 – are secreted glycoproteins that are hypoxia-regulated and were shown to be cytoprotective in various in vitro studies. Hence, we investigated the expression of stanniocalcins in the normal, degenerating and hypoxic retina. We show that the expression of Stc1 and Stc2 in the retina was detectable as early as postnatal day 10 and persisted during aging. Retinal expression of Stc2, but not Stc1, was induced in mice in an in vivo model of acute hypoxia and a genetic model of chronic hypoxia. Furthermore, we show that HIF1, not HIF2, is responsible for regulating Stc2 in cells with the molecular response to hypoxia activated due to the absence of von Hippel Lindau protein. Surprisingly, Stc2 was not normally expressed in photoreceptors but in the inner retina, as shown by laser capture microdissection and immunofluorescence data. The expression of both Stc1 and Stc2 remained unchanged in the degenerative retina with an almost complete loss of photoreceptors, confirming their expression in the inner retina. However, the absence of either Stc1 or Stc2 had no effect on retinal architecture, as was evident from retinal morphology of the respective knockout mice. Taken together our data provides evidence for the differential regulation of STC1 and STC2 in the retina and the prospect of investigating STC2 as a retinal neuroprotective factor.
Introduction
Retinal degenerative diseases not only reduce the quality of life of the patients but are also a huge economic burden. Strategies that focus on supplying neuroprotective factors as treatment for these diseases are promising. Hence, there is an immense interest in identification and characterization of such neuroprotective factors that can promote survival of retinal neurons and slow down or prevent the progression of disease (Pardue and Allen, 2018). Subjecting the retinal tissue to acute stress such as hypoxic stress can result in the secretion of such neuroprotective factors (Thiersch et al., 2008).
The corpuscle of Stannius (named after Hermann Friedrich Stannius who discovered it in 1839 in bony fish) secrets a glycoprotein hormone named stanniocalcin (STC) (reviewed in Chang et al., 2003). The human STC1 gene was first identified in a screen for genes involved in the control of proliferation and almost 60% of the amino acids it encodes are identical to fish STC (Chang et al., 1995; Olsen et al., 1996). STC2 was identified later (Chang and Reddel, 1998; DiMattia et al., 1998; Ishibashi et al., 1998) and shares only 30% nucleotide identity with STC1 suggesting a common ancestor (Wagner and DiMattia, 2006). Both STC1 and STC2 have signal peptides of about 24 amino acids suggesting that they are secreted proteins (Moore et al., 1999).
Both stanniocalcins have been implicated in cancer formation (Yeung et al., 2012; Joshi, 2020). The tumor microenvironment is often hypoxic (Vaupel et al., 1989), and several studies have shown Stc1 and Stc2 to be regulated by hypoxia. Increased expression of Stc1 was reported in mice that were preconditioned by hypoxia resulting in ischemic tolerance in the heart (Westberg et al., 2007a) and brain (Westberg et al., 2007b). Additionally, hypoxic exposure of various cancer cell lines resulted in the upregulation of STC1 expression (Yeung et al., 2005). Similarly, STC2 was induced by hypoxia in proximal tubular epithelial cells (Leonard et al., 2003). Analysis of promoters of both STC1 and STC2 revealed putative hypoxia responsive elements (HRE) and in vitro studies using mutated versions of these sites suggested that both STC1 and STC2 are HIF1 (hypoxia inducible factor 1) target genes, although the association for STC1 was not consistent (Law et al., 2008, 2010; Law and Wong, 2010b).
A few studies have investigated the molecular mechanisms and subcellular functions of the stanniocalcins, with several focusing on their role in calcium homeostasis (Zeiger et al., 2011; López et al., 2018). Other studies reported the identification of STC1 binding sites on the mitochondrial membrane (McCudden et al., 2002) or an uncoupling effect of STC1 on oxidative phosphorylation, suggesting a role in intracellular metabolism (Ellard et al., 2007), despite the presence of a signal peptide for secretion. Using fibroblasts from Stc2 knockout mice, Zeiger et al. (2011) showed that STC2 is a negative regulator of store-operated calcium entry and several cell culture studies have reported cytoprotective effects of STC2 on mesenchymal cells (Kim et al., 2015), hepatocytes (Joshi et al., 2015), and neuronal cells (Zhang et al., 2000; Ito et al., 2004). Studies in mice have shown in vivo protective effects of STC2 on pancreas (Fazio et al., 2011), liver (Zhao J. et al., 2018), and adipose tissue (Sarapio et al., 2019).
Not much is known about the function of stanniocalcins in the retina. However, STC1 increased cell viability of the retinal cell line RGC-5 (Kim et al., 2013) and rescued retinal degeneration in two rat models after intravitreal protein injection or AAV-mediated gene delivery (Roddy et al., 2012, 2017). STC1 was protective in a model of retinopathy of prematurity (Dalvin et al., 2020), and was implicated in the development of choroidal neovascularization (Zhao M. et al., 2018), glaucoma pathogenesis (Lu et al., 2019), and the protection of ganglion cells (Kim et al., 2013). No studies report on the role of STC2 in the retina so far. As the retina is a tissue with a high oxygen demand, it is very sensitive to variations in oxygen levels and hypoxia has been implicated in degenerative diseases of the retina (Arjamaa and Nikinmaa, 2006; Grimm and Willmann, 2012; Lange and Bainbridge, 2012). Here we report for the first time on differences between the hypoxic regulation of Stc1 and Stc2 in the mouse retina.
Materials and Methods
Animals and Hypoxic Exposure
Animals were treated in accordance with the regulations of the veterinary authority of Zurich and the statement of the Association for Research in Vision and Ophthalmology (ARVO) for the use of animals in vision research. All mice were kept at the Laboratory Animal Services Center (LASC) of the University of Zurich in a light-dark (12 h:12 h) cycle with food and water ad libitum. Light was maintained at 60 lux at cage level during the light period. Mice were euthanized by CO2 inhalation followed by cervical dislocation. C57BL/6 mice were purchased from Charles River (Chatillon-sur-Chalaronne, France), 129S6 mice from Taconic (Ejby, Denmark), rd10 mice (C57BL/6 background) from Jackson Laboratories (Bar Harbor, ME, United States) and Balb/c mice were offspring from an in-house breeding. The master regulator of the hypoxic response is the hypoxia-inducible factor 1 (HIF1) belonging to a family of proteins that also includes HIF2 and HIF3 (Semenza, 2012). HIF1 is a heterodimer composed of a nuclear-localized beta subunit and an oxygen labile alpha subunit (Wang et al., 1995) that is constitutively produced but undergoes immediate degradation in normoxia via an oxygen-dependent mechanism (Maxwell et al., 1999). Since degradation requires VHL (von Hippel Lindau protein), the absence of functional VHL leads to a stabilization of HIFA subunits (Jaakkola et al., 2001), the activation of HIF transcription factors and induction of the hypoxic response even in normoxic cells (Krieg et al., 2000; Lange et al., 2011). The mice lacking the VHL in the rod photoreceptors serve as a model for chronic hypoxia-like response in the retina. Opsin-cre;Vhlflox/flox (rodΔVhl), opsin-cre;Vhlflox/flox;Hif1aflox/flox (rodΔVhl;Hif1a), opsin-cre;Vhlflox/flox;Hif2aflox/flox (rodΔVhl;Hif2a) and opsin-cre;Vhlflox/flox;Hif1flox/flox;Hif2aflox/flox (rodΔVhl;Hif1a;Hif2a) mice were generated and genotyped as described (Lange et al., 2011; Barben et al., 2018). Mice with floxed genes but without Opsin-cre served as controls. The STC1-/- and STC2-/- mice were generated as described earlier (Chang et al., 2005, 2008). 129S6 and Balb/C wild type mice were exposed to hypoxia in a hypoxia chamber by reducing O2 levels by 2% every 10 min until a level of 7% O2 was reached. Hypoxia was maintained for 6 h before mice were either euthanized (immediate time-point) or reoxygenated in normal room air (up to 16 h) before euthanasia and tissue collection (Grimm et al., 2002). Since the genetic background of the mouse strain does not influence their response to hypoxia, different strains of WT mice were used for hypoxia experiments as per availability at various time and age-points. Balb/c mice were used for hypoxia and reoxygenation experiment. C57BL/6 mice at 7 weeks and 7 months of age were used for testing gene expression post-hypoxia at different ages. Gene expression in rd10 mice was compared to C57BL/6 WT animals and the strain used for laser capture microdissection experiment was 129S6.
Morphology and Light Microscopy
Eyes were enucleated and fixed in 2.5% glutaraldehyde in 0.1 M cacodylate buffer (pH 7.3) at 4°C overnight. After fixation, cornea and lens were removed, and the eyecup was separated into a superior and an inferior half by cutting through the optic nerve head. The trimmed tissue was washed in cacodylate buffer, contrasted with osmium tetroxide (1%) for 1 h at room temperature, dehydrated by incubations in increasing ethanol concentrations, and embedded in Epon 812. Semi-thin sections (0.5 μm) were prepared and counterstained with toluidine blue. An Axioplan digitalized microscope (Zeiss Meditec, Jena, Germany) was used to examine the slides. Shown are representative images of N = 3 independent animals per strain.
RNA Isolation, cDNA Synthesis, and Semiquantitative Real-Time PCR
Retinas were isolated through a corneal incision and immediately snap frozen in liquid nitrogen. Total RNA was isolated using the RNeasy isolation kit (catalog number: 74104, Qiagen, Hilden, Germany) or the High Pure RNA isolation kit (catalog number: 11828665001, Roche Diagonistics, Basel, Switzerland). Residual genomic DNA was removed by a DNase I incubation step. Total RNA (650–1000 ng) was reverse transcribed using oligo(dT) and M-MLV reverse transcriptase (catalog number: M1701, Promega, Madison, WI, United States). 10 ng of cDNA was used for gene expression analysis by semiquantitative real-time PCR using a LightCycler 480 instrument (Roche Diagnostics), the LightCycler 480 SYBR Green I Master mix (catalog number: 04887352001, Roche Diagonistics), and specific primer pairs (Table 1). The primer pairs were designed to span large intronic sequences or to cover exon-intron boundaries. Gene expression was normalized to ß-actin (Actb), and relative quantification was calculated using the comparative threshold method (ΔΔCT).
Laser Capture Microdissection
Eyes from 8 weeks old wild type (129S6) mice were enucleated, immediately embedded in tissue freezing medium (Leica Microsystems Nussloch GmbH, Nussloch, Germany), and frozen in a 2-methylbutane bath cooled in liquid nitrogen. 20 μm thick retinal sections were collected on Arcturus PEN Membrane Glass Slides (Applied Biosystems, Foster City, CA, United States). The slides were fixed for 5 min in acetone, followed by 5 min of air drying and two dehydration steps (30 s with 100% ethanol and 5 min with xylol). Retinal layers were isolated using an Arcturus XT Laser Capture Microdissection system (Bucher Biotec AG, Basel, Switzerland) and Arcturus CapSure Macro LCM Caps (Applied Biosystems). Extraction of RNA was done using the Arcturus PicoPure RNA Isolation Kit (Applied Biosystems) according to the manufacturer’s instructions, including a DNase treatment to remove residual genomic DNA. cDNA was synthesized using random hexamer primers (High-Capacity cDNA Reverse Transcription Kit; Applied Biosystems), and gene expression analyzed by semi-quantitative real-time PCR as described in above.
Immunofluorescence on Retinal Cryosections and Flatmounts
To prepare cryosections, eyes were enucleated and fixed in 4% paraformaldehyde (PFA) overnight. The cornea and lens were removed and the eyecups immersed in 30% sucrose in Phosphate-buffered saline (PBS). Eyecups were embedded in tissue cryoprotective medium (Leica Microsystems Nussloch GmbH, Nussloch, Germany) and frozen in a 2-methylbutane bath cooled with liquid nitrogen. 12 μm thick sections were cut through the optic nerve head with a cryostat (Leica), air-dried, and stored at –80°C until further use. To prepare flatmounts, eyes were enucleated and incubated for 3–5 min in 2% paraformaldehyde prepared in phosphate-buffered saline (PBS). After having removed cornea and lens, the eyeball was cut in a cloverleaf shape by making four incisions. The retina was dissected from the sclera and placed in PBS in a 48-well plate. Dissected retinas were post-fixed at room temperature for 1 h with 4% paraformaldehyde in PBS and washed with PBS. Retinal sections or flatmounts were incubated with blocking solution (3% goat serum/0.3% Triton-X in PBS) for 1 h at room temperature. Primary antibodies were applied and left on sections at 4°C overnight (Table 2). Specificity of the anti-STC2 antibody was tested on Western blots with the antibody detecting a protein of the size of STC2 (34 kDa) but not of STC1 (28 kDa) (not shown). Slides were washed 3 times with PBS followed by incubation with Cy2 or Cy3-conjugated secondary antibodies (Jackson Immunoresearch, Soham, United Kingdom) in blocking solution (dilution = 1:500) for 1 h at room temperature. After 3 PBS washes the sections were stained with DAPI to visualize nuclei and slides mounted with the anti-fade medium Mowiol. Immunofluorescently labeled proteins were imaged using an Axioplan fluorescence microscope (Carl Zeiss AG). Shown are representative images of N = 3 independent animals per strain.
Statistical Analysis
Statistical analysis was performed using Prism software (GraphPad, San Diego, CA, United States). All data are presented as mean ± standard deviation (SD). The number of samples (N) and the statistical test used for individual experiments are given in the Figure captions. Student’s t-tests were used to measure the statistical differences of means and differences between two groups. One-way or two-way ANOVA with respective post hoc tests (see Figure captions) were used to compare several groups. P-value ≤ 0.05 was considered significant.
Results
Expression of Stc1 and Stc2 in the Mouse Retina
To investigate Stc1 and Stc2 in the retina, we compared their expression levels in the retina to those in other tissues. When normalized to Actb, Stc1 was expressed more strongly in the retina than in any other tissue investigated. Stc2 was also highly expressed in the retina but did not reach expression levels detected in heart and kidney (Figure 1A).
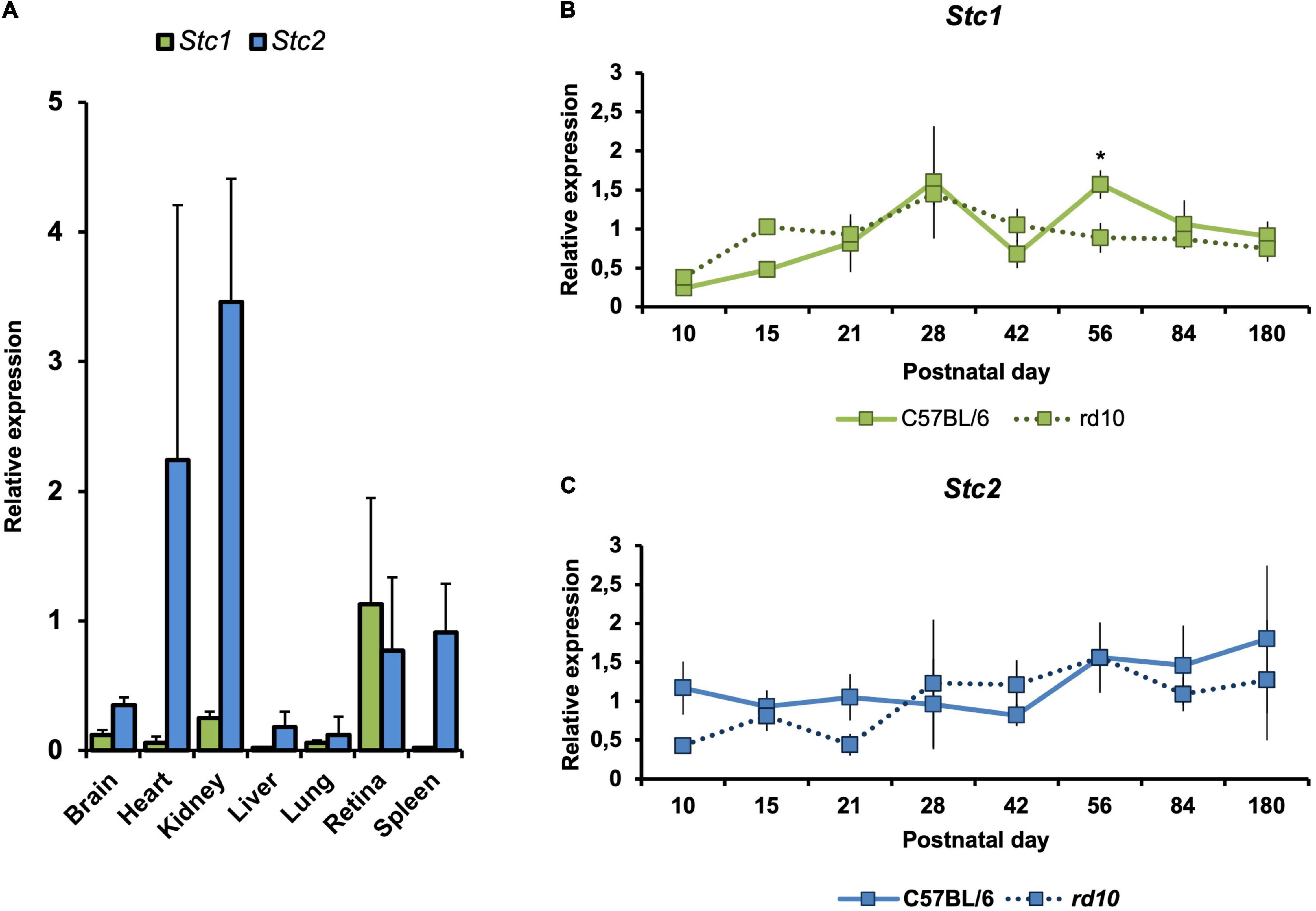
Figure 1. Gene expression of Stc1 and Stc2 in the retina during postnatal development, aging and degeneration. (A) Relative expression levels of Stc1 (green bars) and Stc2 (blue bars) in different tissues isolated from 11 weeks old wild type (C57BL/6) mice. Relative expression levels of Stc1 (B) and Stc2 (C) in the retina of wild type (C57BL/6) mice (solid line) and rd10 mice (dotted line) from PND10 to PND180. Expression was determined by semi-quantitative real-time PCR and normalized to Actb levels. Shown are mean values ± SD of N = 3. *P < 0.05. Two-way ANOVA with Sidak’s multiple comparison test.
We also analyzed the expression of Stc1 and Stc2 in the retina during postnatal development and aging from postnatal day 10 (PND10) to PND180. Both genes were expressed as early as PND10 in the retinas of wild type mice. While expression of Stc2 was quite steady during the time frame analyzed, expression of Stc1 slightly increased during postnatal development and aging (Figures 1B,C – solid lines; period between PND10 and PND28). To investigate whether expression of Stc1 and Stc2 is affected by retinal degeneration, we analyzed their mRNA levels in rd10 mice that are characterized by a progressive loss of rod photoreceptors starting around PND15 and a loss of almost all photoreceptors at 6 months of age (Samardzija et al., 2012). With the exception of PND56 for Stc1, expression of Stc1 and Stc2 in rd10 and wild type retinas was comparable at all time points tested (Figures 1B,C – dotted lines), suggesting that these genes may not have a direct or important role in the degenerating retina. Since almost all photoreceptors are lost in rd10 mice at the late timepoints, the data also suggest that Stc1 and Stc2 are not expressed in photoreceptors, or are expressed at very low levels.
In the Retina Stc2 but Not Stc1 Is Responsive to Hypoxia
The retina is a metabolically active tissue with a high oxygen consumption rate (Yu and Cringle, 2001) and therefore is very sensitive to hypoxia (Caprara and Grimm, 2012). Since previous studies have shown STC1 and STC2 to be responsive to hypoxia (see section “Introduction”), we examined Stc1 and Stc2 expression in the retina of mice that have been exposed for 6 h to 7% O2. Surprisingly, expression of Stc1 remained unaltered in the hypoxic retina. In contrast, expression of Stc2 was upregulated ninefold immediately after hypoxia and was still fourfold elevated at 2 h of reoxygenation before decreasing to basal levels after 4 h of reoxygenation (Figure 2A). To test if the hypoxic response was age-related, 7 weeks old and 7 months old mice were exposed to hypoxia. Again, Stc1 did not respond to the treatment whereas Stc2 showed significant upregulation in young and a trend toward upregulation in old mice suggesting that the hypoxic induction did not depend on the age of the mice. The known hypoxia-regulated genes Adm (adrenomedullin) (Zhao et al., 1996) and Epo (erythropoetin) (Grimm et al., 2002) were used as controls and showed an upregulation post-hypoxia as expected (Figure 2B).
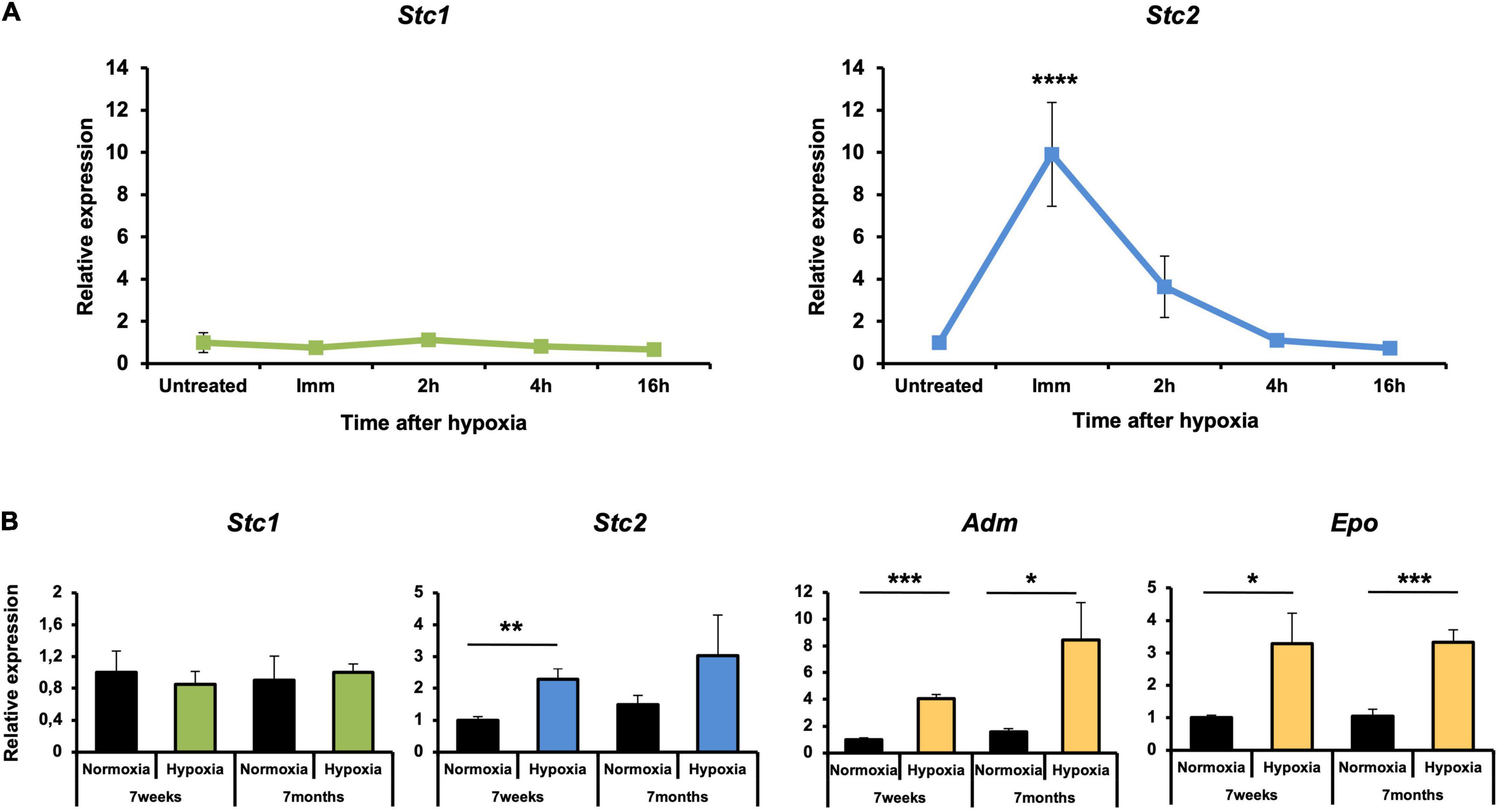
Figure 2. Upregulation of Stc2 gene expression in response to hypoxia. (A) Relative expressions of Stc1 (left panel) and Stc2 (right panel) in retinas of 6 weeks old wild type (Balb/c) mice exposed to hypoxia (6 h, 7% O2). Tissue was collected immediately after hypoxia (Imm) or after 2, 4, and 16 h of reoxygenation following hypoxia. (B) Relative expression of Stc1, Stc2, Adm, and Epo in retinas of wild-type (129S6) mice exposed to normoxia (black bars) or hypoxia (tissue collected immediately after 6 h at 7% O2) (colored bars) at 7 weeks or 7 months of age. Expression levels were determined by semi-quantitative real-time PCR, normalized to Actb and shown relative to the levels in untreated controls (set to 1) (A) or to normoxic controls at 7 weeks of age (set to 1) (B). Shown are mean values ± SD of N = 3. Significance was tested using one-way ANOVA with Dunnett’s multiple comparison test (A) and by Student’s t-test (B) (*P < 0.05, **P < 0.01, ***P < 0.001, P < 0.0001****).
Hypoxic Upregulation of Stc2 Requires HIF1
To analyze the molecular requirements for hypoxic Stc2 induction in the retina, we took advantage of several mouse lines lacking either Vhl (rodΔVhl), Vhl and Hif1a (rodΔVhl;Hif1a), Vhl and Hif2a (rodΔVhl;Hif2a) or Vhl, Hif1a and Hif2a (rodΔVhl;Hif1a;Hif2a) in rod photoreceptors (Lange et al., 2011; Barben et al., 2018). The absence of VHL activated HIF transcription factors and thus resulted in a chronic hypoxia-like response in rods. This enabled us to investigate the potential involvement of HIF1 and HIF2 transcription factors in the activation of Stc2 in photoreceptors with an activated hypoxic response. Although our data (Figures 1B,C) suggested only a very weak or even absent (Figure 4, see below) expression of Stc1 and Stc2 in normal photoreceptors, activation of HIF transcription factors by means of Vhl deletion in rods resulted in increased retinal Stc2 expression (Figure 3, rodΔVhl). Stc1, however, was not induced in the absence of Vhl, resembling the results from the acute hypoxia experiment (Figure 2). Stc2 expression was abolished in retinas of mice with the additional deletion of Hif1a (rodΔVhl;Hif1a) or of both Hif1a and Hif2a (rodΔ Vhl;Hif1a;Hif2a) whereas the retinas of mice lacking only Hif2a in addition to Vhl (rodΔ Vhl;Hif2a) had Stc2 levels comparable to those in rodΔ Vlhl mice (Figure 3). These data indicate that HIF1, not HIF2, is responsible for regulating Stc2 in retina during hypoxic events.
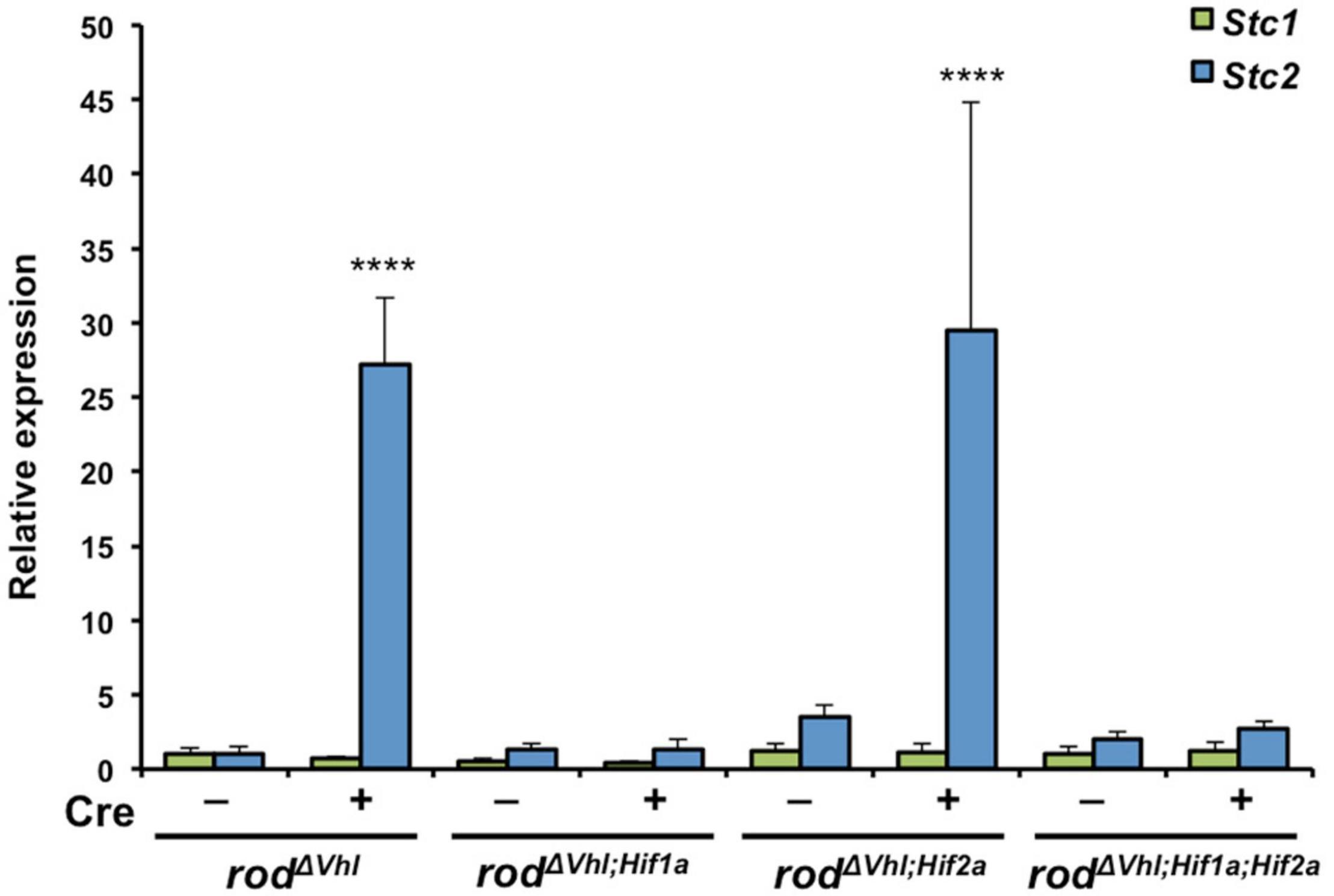
Figure 3. Upregulation of Stc2 upon stabilization of HIF1A in rod photoreceptors. Relative expression of Stc1 (green bars) and Stc2 (blue bars) in rodΔVhl, rodΔVhl;Hif1a, rodΔVhl;Hif2a, and rodΔVhl;Hif1a;Hif2a mice and their control lines that carried the respective floxed genes without Opsin-cre (–). Expression was determined by semi-quantitative real-time PCR and normalized to Actb levels. Shown are mean values ± SD of N = 3. ****P < 0.0001. Two-way ANOVA with Sidak’s multiple comparison test.
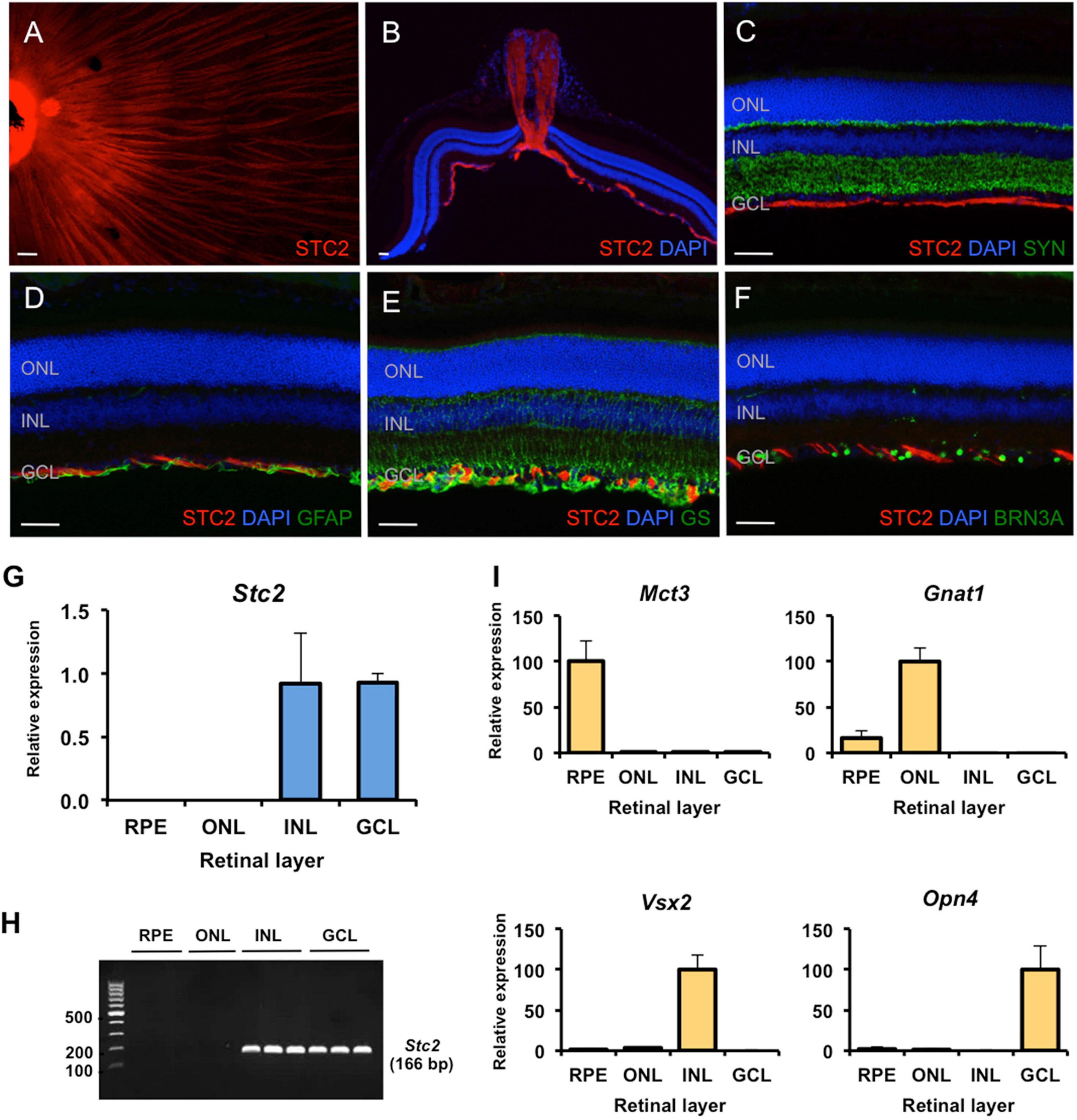
Figure 4. Site-specific detection of STC2 protein and mRNA expression in retinal tissue. (A) Immunostaining for STC2 (red) in a retinal flatmount of a 7 weeks old wild type (129S6) mouse. (B) Immunostaining for STC2 in a retinal section of an 11 weeks old wild type (129S6) mouse. Co-immunostainings for STC2 (red) and SYN (C, green), GFAP (D, green), GS (E, green), and BRN3A (F, green). Nuclei are stained with DAPI (blue). Scale bar: 50 μM. (G) Relative expression of Stc2 mRNA in the RPE (retinal pigment epithelium), ONL (outer nuclear layer), INL (inner nuclear layer), and GCL (ganglion cell layer) that were isolated by laser capture microdissection. (H) The amplified PCR products from experiments shown in panel (G) were run on an agarose gel for visualization. N = 3. (I) Relative expression of layer-specific control genes: Mct3 for RPE, Gnat1 for ONL, Vsx2 for INL, and Opn4 for GCL. Expression was determined by semi-quantitative real-time PCR and normalized to Actb. Shown are mean values ± SD of N = 3.
Stc2 Is Expressed in the Inner Retina
Immunostaining on retinal flatmounts and sections of wild type mice revealed that the STC2 protein localized to axonal neurofilaments in the ganglion cell layer (GCL, Figures 4A,B). Co-stainings for markers of various retinal cell types, including SYP (synaptophysin, Figure 4C) for synapses in the plexiform layers, GFAP (glial fibrillary acidic protein, Figure 4D) for activated Müller cells and astrocytes, GS (glutamine synthetase, Figure 4E) for Müller cells and BRN3A (brain-specific homeobox protein 3A, Figure 4F) for retinal ganglion cells, suggested that STC2 localized to the neurofilament layer and did not co-localize with any of the above markers (Figures 4C–F).
Since expression of Stc2 was not reduced in degenerated rd10 retinas lacking all photoreceptors, Stc2 may not normally be expressed in rods (see above). To elucidate the retinal layers expressing Stc2 in more detail, we separated the retinal pigment epithelium (RPE), outer nuclear layer (ONL), inner nuclear layer (INL), and the GCL by laser capture microdissection and analyzed Stc2 expression by real-time PCR. Results showed that Stc2 was strongly and exclusively expressed in the INL and GCL, but not in the ONL or RPE of the normal retina (Figures 4G–I). Layer specific markers for the RPE (Mct3, monocarboxylate transporter 3), ONL (Gnat1, guanine nucleotide-binding protein G subunit alpha-1, for rods), INL (Vsx2, visual system homeobox 2, for bipolar cells), and GCL (Opn4, melanopsin, for ganglion cells) verified the separation of the individual retinal layers (Figure 4I).
Both Stc1–/– and Stc2–/– Mice Have a Normal Retinal Morphology
Two Stc1 knockout mouse lines display a dwarf phenotype (Filvaroff et al., 2002; Varghese et al., 2002) while a third line was reported without a significant phenotype (Chang et al., 2005). Transgenic mice overexpressing human STC2 are about 45% smaller than their littermates (Gagliardi et al., 2005) while Stc2–/– mice are 10–15% larger and grow faster than wild type mice (Chang et al., 2008). Although not completely congruent, published data thus indicate a potential involvement of stanniocalcins in development (Filvaroff et al., 2002; Varghese et al., 2002). To test a potential influence of stanniocalcins on retinal development and/or maintenance we investigated the retinal morphology in eyes of 9 months old Stc1–/– and Stc2–/– mice. Surprisingly, morphology and retinal architecture of both knockout mice were indistinguishable from wild type mice (Figures 5A–C). This was further supported by the similar retinal thickness of wild type and Stc2–/– mice (Figure 5D). Immunolabeling of STC2 in wild type mice was detected in the NFL and was absent in the Stc2–/– mice as expected, but interestingly, was also not detectable in Stc1–/– mice (Figures 5E–G). Immunolabeling for BRN3A, SYN and GFAP in Stc2–/– mice showed normal expression patterns (Figures 5H–J).
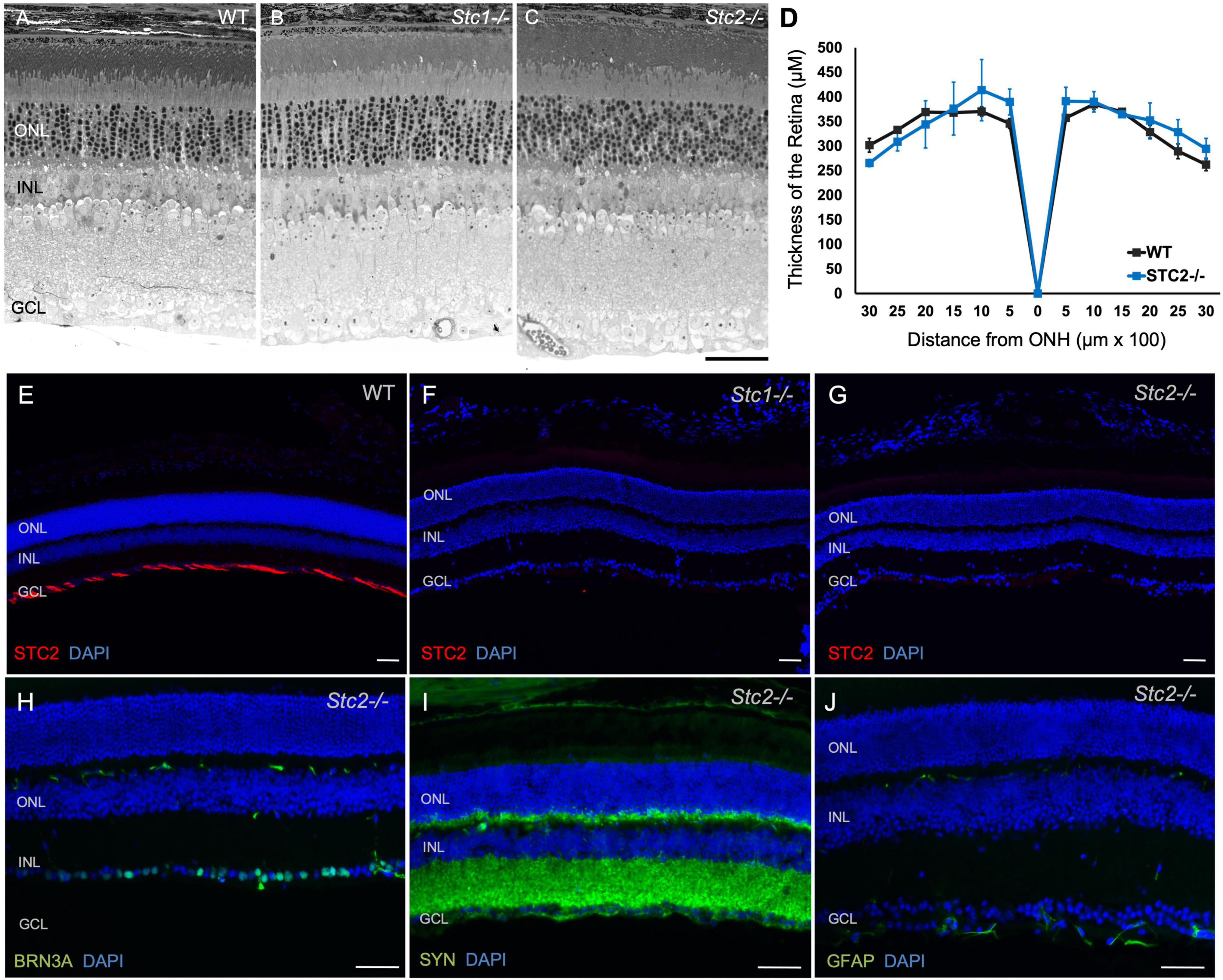
Figure 5. Retinal morphology in the absence of STC1 and STC2. Retinal morphology of wild type (WT, C57BL/6) (A), Stc1–/– (B) and Stc2–/–, (C) mice. (D) Spidergram of the retinal thickness of WT (C57BL/6, black line) and Stc2–/– (blue line) mice; N = 3, ONH: Optic Nerve Head Immunostainings for STC2 (red) in retinal sections of wild type (C57BL/6) (E), Stc1–/– (F), and Stc2–/– (G) mice. Immunostainings for BRN3A (H), SYN (I), and GFAP (J) in retinal sections of Stc2–/– mice. DAPI (blue) was used as nuclear stain. Images were acquired at 200 μm from the optic nerve head. Mice were 6 months (wild type) or 9 months (Stc1–/– and Stc2–/–) of age. Shown are representative images of N = 3 retinas per genotype. Scale bar: 50 μm.
Discussion
Neuroprotection slows the progression of retinal degeneration and extends the therapeutic window for additional treatments. Hence there is an active pursuit for factors that can protect retinal cells. Protective factors are often involved in pro-survival signaling pathways that are activated when the tissue experiences stress. Such a stress can be caused by reduced oxygen availability leading to local hypoxia that has been implicated in retinal degeneration and disease (Lange and Bainbridge, 2012). Since several studies have implicated both secreted glycoproteins STC1 and STC2 in the response to hypoxia (Chang et al., 2003; Joshi, 2020), we investigated expression and hypoxic regulation of these two genes in the retina. Surprisingly, and in contrast to other tissues (Yeung et al., 2005; Westberg et al., 2007a,b), only Stc2 and not Stc1 was responsive to hypoxia in the retina (Figure 2). Two consensus HIF1 binding sites were recognized and characterized in the promoter region of the Stc2 gene in previous in vitro studies (Law and Wong, 2010a). In line with this finding, we showed that upregulation of Stc2 expression in rodΔ Vhl mice depended on the transcription factor HIF1A (Figure 3). A search for hypoxia-response elements in the promoter of Stc1 did not yield conclusive results but suggested that Stc1 was inhibited by factor inhibiting HIF (FIH) (Law et al., 2010). However, unless Stc1 and Stc2 are expressed in separate cell types, it seems unlikely that FIH accounts for the lack of Stc1 induction in hypoxia in the retina as its action would affect HIF1 activity in general and thus expression of Stc2 as well.
Our results showed that Stc1 and Stc2 were highly expressed in the retina of wild type mice and their expression was largely unaffected by the loss of photoreceptors during retinal degeneration (Figure 1). This suggests that the two stanniocalcin genes are mainly expressed in cells of the inner retina of wild type mice, a conclusion supported by our laser capture microdissection data (Figure 4). The localization of STC2 protein on neurofilaments further strengthens this conclusion. In the rodΔ Vhl mice that serve as a model for an active hypoxic response, Stc2 gene is also expressed in photoreceptors where HIF1 is stabilized (Figure 3). However, the protein is still localized in the neurofilaments, and to some extent in Muller cells. STCs are secreted glycoproteins and we hypothesize that they may be produced by cells of the INL and GCL in the normal retina, or in response to hypoxia in other cell types, and secreted and transported to the NFL where they are required. The exact mechanism of this secretion, transport and their function in the NFL is not clear and merits further investigation.
The function of stanniocalcins in the retina is unknown but the surprising lack of STC2 protein in Stc1–/– mice hints at a potential cross-talk in the regulation of the two stanniocalcins (Figure 5). Following this intriguing result, it would have been interesting to know if STC1 is missing from Stc2–/– mice as well. However, our primary goal was to analyze hypoxia-regulated genes in the retina, and since the expression level of Stc1 was low and not regulated by hypoxia we did not further test STC1 protein levels. Nevertheless, stanniocalcins may not have essential functions for retinal development and maintenance as the retinal morphologies of Stc1–/– and Stc2–/– mice appeared normal (Figure 5) despite reported effects of the knockouts on general animal size (Filvaroff et al., 2002; Varghese et al., 2002; Chang et al., 2005, 2008). It is possible that lack of STC1 and/or STC2 does not affect the retina under normal physiological conditions, but that the two stanniocalcins are needed under stress conditions. Hence, it would be interesting to investigate how Stc1–/– and Stc2–/– mice respond when subjected to hypoxic stress or to photoreceptor loss in models of retinal degeneration.
Several publications report protective activities of STC1 in the retina including a study concluding that intravitreal injections of STC1 rescued degeneration in S334ter-3 and RCS rats, which are models for fast and slow, respectively, photoreceptor degeneration (Roddy et al., 2012). It would be of interest to investigate whether maintaining high retinal levels of STC1 prevents or slows the progression of degeneration in the rd10 mouse, as it was reported for rats (Roddy et al., 2012). Since Stc2 showed a strongly elevated expression during hypoxic stress, it may be equally important to test whether high levels of STC2 would be neuroprotective in the retina, similar to STC1 in other tissues. Such data may make stanniocalcins attractive targets for the development of potential therapeutic strategies.
Taken together our study investigates for the first time the expression of stanniocalcins in the normal, degenerating and hypoxic retina. The differential regulation of Stc1 and Stc2 under hypoxic conditions indicates the possibility of distinct and non-redundant functions for the two proteins in the retina. We show HIF1A-mediated regulation of Stc2 in photoreceptors lacking Vhl, which merits further study of the potential of STC2 as a retinal neuroprotective candidate.
Data Availability Statement
The original contributions presented in this study are included in the article/supplementary material, further inquiries can be directed to the corresponding author.
Ethics Statement
The animal study was reviewed and approved by the Veterinary authority of Zurich, Switzerland.
Author Contributions
CG and DA: conceptualization and analysis. DA, MS, and JK: experiments. AC and RR: STC KO mice related experiments. DA: writing – original draft. DA, MS, RR, and CG: writing – revision and editing. CG: project administration and funding acquisition. All authors contributed to the article and approved the submitted version.
Funding
This work was supported by the Swiss National Science Foundation (31003A_133043 to CG).
Conflict of Interest
The authors declare that the research was conducted in the absence of any commercial or financial relationships that could be construed as a potential conflict of interest.
Publisher’s Note
All claims expressed in this article are solely those of the authors and do not necessarily represent those of their affiliated organizations, or those of the publisher, the editors and the reviewers. Any product that may be evaluated in this article, or claim that may be made by its manufacturer, is not guaranteed or endorsed by the publisher.
Acknowledgments
We thank Cornelia Imsand, Andrea Gubler, and Christel Beck for their skillful assistance in experimental procedures.
References
Arjamaa, O., and Nikinmaa, M. (2006). Oxygen-dependent diseases in the retina: role of hypoxia-inducible factors. Exp. Eye Res. 83, 473–483. doi: 10.1016/j.exer.2006.01.016
Barben, M., Ail, D., Storti, F., Klee, K., Schori, C., Samardzija, M., et al. (2018). Hif1a inactivation rescues photoreceptor degeneration induced by a chronic hypoxia-like stress. Cell Death Differ. 25, 2071–2085. doi: 10.1038/s41418-018-0094-7
Caprara, C., and Grimm, C. (2012). From oxygen to erythropoietin: relevance of hypoxia for retinal development, health and disease. Prog. Retin. Eye Res. 31, 89–119. doi: 10.1016/j.preteyeres.2011.11.003
Chang, A. C. M., Cha, J., Koentgen, F., and Reddel, R. R. (2005). The murine stanniocalcin 1 gene is not essential for growth and development. Mol. Cell. Biol. 25, 10604–10610. doi: 10.1128/mcb.25.23.10604-10610.2005
Chang, A. C. M., Hook, J., Lemckert, F. A., McDonald, M. M., Nguyen, M. A. T., Hardeman, E. C., et al. (2008). The murine stanniocalcin 2 gene is a negative regulator of postnatal growth. Endocrinology 149, 2403–2410. doi: 10.1210/en.2007-1219
Chang, A. C. M., Janosi, J., Hulsbeek, M., de Jong, D., Jeffrey, K. J., Noble, J. R., et al. (1995). A novel human cDNA highly homologous to the fish hormone stanniocalcin. Mol. Cell. Endocrinol. 112, 241–247. doi: 10.1016/0303-7207(95)03601-3
Chang, A. C. M., Jellinek, D. A., and Reddel, R. R. (2003). Mammalian stanniocalcins and cancer. Endocr. Relat. Cancer 10, 359–373. doi: 10.1677/erc.0.0100359
Chang, A. C. M., and Reddel, R. R. (1998). Identification of a second stanniocalcin cDNA in mouse and human: stanniocalcin 2. Mol. Cell Endocrinol. 141, 95–99. doi: 10.1016/s0303-7207(98)00097-5
Dalvin, L. A., Hartnett, M. E., Bretz, C. A., Hann, C. R., Cui, R. Z., Marmorstein, A. D., et al. (2020). Stanniocalcin-1 is a modifier of oxygen induced retinopathy severity. Curr. Eye Res. 45, 46–51. doi: 10.1080/02713683.2019.1645184
DiMattia, G. E., Varghese, R., and Wagner, G. F. (1998). Molecular cloning and characterization of stanniocalcin-related protein. Mol. Cell. Endocrinol. 146, 137–140. doi: 10.1016/S0303-7207(98)00163-4
Ellard, J. P., McCudden, C. R., Tanega, C., James, K. A., Ratkovic, S., Staples, J. F., et al. (2007). The respiratory effects of stanniocalcin-1 (STC-1) on intact mitochondria and cells: STC-1 uncouples oxidative phosphorylation and its actions are modulated by nucleotide triphosphates. Mol. Cell. Endocrinol. 264, 90–101. doi: 10.1016/j.mce.2006.10.008
Fazio, E. N., DiMattia, G. E., Chadi, S. A., Kernohan, K. D., and Pin, C. L. (2011). Stanniocalcin 2 alters PERK signalling and reduces cellular injury during cerulein induced pancreatitis in mice. BMC Cell Biol. 12:17. doi: 10.1186/1471-2121-12-17
Filvaroff, E. H., Guillet, S., Zlot, C., Bao, M. I. N., Ingle, G., Steinmetz, H., et al. (2002). Stanniocalcin 1 alters muscle and bone structure and function in transgenic mice. Endocrinology 143, 3681–3690. doi: 10.1210/en.2001-211424
Gagliardi, A. D., Kuo, E. Y. W., Raulic, S., Wagner, G. F., and DiMattia, G. E. (2005). Human stanniocalcin-2 exhibits potent growth-suppressive properties in transgenic mice independently of growth hormone and IGFs. Am. J. Physiol. Endocrinol. Metab. 288, 92–105. doi: 10.1152/ajpendo.00268.2004
Grimm, C., Wenzel, A., Groszer, M., Mayser, H., Seeliger, M., Samardzija, M., et al. (2002). HIF-1-induced erythropoietin in the hypoxic retina protects against light-induced retinal degeneration. Nat. Med. 8, 718–724. doi: 10.1038/nm723
Grimm, C., and Willmann, G. (2012). Hypoxia in the eye: a two-sided coin. High Alt. Med. Biol. 13, 169–175. doi: 10.1089/ham.2012.1031
Ishibashi, K., Miyamoto, K., Taketani, Y., Morita, K., Takeda, E., Sasaki, S., et al. (1998). Molecular cloning of a second human stanniocalcin homologue (STC2). Biochem. Biophys. Res. Commun. 250, 252–258. doi: 10.1006/bbrc.1998.9300
Ito, D., Walker, J. R., Thompson, C. S., Moroz, I., Lin, W., Veselits, M. L., et al. (2004). Characterization of stanniocalcin 2, a novel target of the mammalian unfolded protein response with cytoprotective properties. Mol. Cell. Biol. 24, 9456–9469. doi: 10.1128/mcb.24.21.9456-9469.2004
Jaakkola, P., Mole, D. R., Tian, Y.-M., Wilson, M. I., Gielbert, J., Gaskell, S. J., et al. (2001). Targeting of HIF-α to the von Hippel-Lindau ubiquitylation complex by O2-regulated prolyl hydroxylation. Science 292, 468–472. doi: 10.1126/science.1059796
Joshi, A. D. (2020). New insights into physiological and pathophysiological functions of stanniocalcin 2. Front. Endocrinol. 11:172. doi: 10.3389/fendo.2020.00172
Joshi, A. D., Carter, D. E., Harper, T. A., and Elferink, C. J. (2015). Aryl hydrocarbon receptor-dependent stanniocalcin 2 induction by cinnabarinic acid provides cytoprotection against endoplasmic reticulum and oxidative stress. J. Pharmacol. Exp. Ther. 353, 201–212. doi: 10.1124/jpet.114.222265
Kim, P. H., Na, S. S., Lee, B., Kim, J. H., and Cho, J. Y. (2015). Stanniocalcin 2 enhances mesenchymal stem cell survival by suppressing oxidative stress. BMB Rep. 48, 702–707. doi: 10.5483/BMBRep.2015.48.12.158
Kim, S. J., Ko, J. H., Yun, J. H., Kim, J. A., Kim, T. E., Lee, H. J., et al. (2013). Stanniocalcin-1 protects retinal ganglion cells by inhibiting apoptosis and oxidative damage. PLoS One 8:e63749. doi: 10.1371/journal.pone.0063749
Krieg, M., Haas, R., Brauch, H., Acker, T., Flamme, I., and Plate, K. H. (2000). Up-regulation of hypoxia-inducible factors HIF-1α and HIF-2α under normoxic conditions in renal carcinoma cells by von Hippel-Lindau tumor suppressor gene loss of function. Oncogene 19, 5435–5443. doi: 10.1038/sj.onc.1203938
Lange, C., Caprara, C., Tanimoto, N., Beck, S., Huber, G., Samardzija, M., et al. (2011). Retina-specific activation of a sustained hypoxia-like response leads to severe retinal degeneration and loss of vision. Neurobiol. Dis. 41, 119–130. doi: 10.1016/j.nbd.2010.08.028
Lange, C. A. K., and Bainbridge, J. W. B. (2012). Oxygen sensing in retinal health and disease. Ophthalmologica 227, 115–131. doi: 10.1159/000331418
Law, A. Y. S., Ching, L. Y., Lai, K. P., and Wong, C. K. C. (2010). Identification and characterization of the hypoxia-responsive element in human stanniocalcin-1 gene. Mol. Cell. Endocrinol. 314, 118–127. doi: 10.1016/j.mce.2009.07.007
Law, A. Y. S., Lai, K. P., Ip, C. K. M., Wong, A. S. T., Wagner, G. F., and Wong, C. K. C. (2008). Epigenetic and HIF-1 regulation of stanniocalcin-2 expression in human cancer cells. Exp. Cell Res. 314, 1823–1830. doi: 10.1016/j.yexcr.2008.03.001
Law, A. Y. S., and Wong, C. K. C. (2010b). Stanniocalcin-2 promotes epithelial-mesenchymal transition and invasiveness in hypoxic human ovarian cancer cells. Exp. Cell Res. 316, 3425–3434. doi: 10.1016/j.yexcr.2010.06.026
Law, A. Y. S., and Wong, C. K. C. (2010a). Stanniocalcin-2 is a HIF-1 target gene that promotes cell proliferation in hypoxia. Exp. Cell Res. 316, 466–476. doi: 10.1016/j.yexcr.2009.09.018
Leonard, M. O., Cottell, D. C., Godson, C., Brady, H. R., and Taylor, C. T. (2003). The role of HIF-1α in transcriptional regulation of the proximal tubular epithelial cell response to hypoxia. J. Biol. Chem. 278, 40296–40304. doi: 10.1074/jbc.M302560200
López, E., Gómez-Gordo, L., Cantonero, C., Bermejo, N., Pérez-Gómez, J., Granados, M. P., et al. (2018). Stanniocalcin 2 regulates non-capacitative Ca2+ entry and aggregation in mouse platelets. Front. Physiol. 9:266. doi: 10.3389/fphys.2018.00266
Lu, Y., Zhou, D., Lu, H., Xu, F., Yue, J., Tong, J., et al. (2019). Investigating a downstream gene of Gpnmb using the systems genetics method. Mol. Vis. 25, 222–236.
Maxwell, P. H., Wiesener, M. S., Chang, G.-W., Clifford, S. C., Vaux, E. C., Cockman, M. E., et al. (1999). The tumour suppressorprotein VHL targetshypoxia-inducible factors foroxygen-dependent proteolysis. Nature 399, 271–275.
McCudden, C. R., James, K. A., Hasilo, C., and Wagner, G. F. (2002). Characterization of mammalian stanniocalcin receptors: mitochondrial targeting of ligand and receptor for regulation of cellular metabolism. J. Biol. Chem. 277, 45249–45258. doi: 10.1074/jbc.M205954200
Moore, E. E., Kuestner, R. E., Conklin, D. C., Whitmore, T. E., Downey, W., Buddle, M. M., et al. (1999). Stanniocalcin 2: characterization of the protein and its localization to human pancreatic alpha cells. Horm. Metab. Res. 31, 406–414. doi: 10.1055/s-2007-978764
Olsen, H. S., Cepeda, M. A., Zhang, Q. Q., Rosen, C. A., Vozzolo, B. L., and Wagner, G. F. (1996). Human stanniocalcin: a possible hormonal regulator of mineral metabolism. Proc. Natl. Acad. Sci. U. S. A. 93, 1792–1796. doi: 10.1073/pnas.93.5.1792
Pardue, M. T., and Allen, R. S. (2018). Neuroprotective strategies for retinal disease. Prog. Retin. Eye Res. 65, 50–76. doi: 10.1016/j.preteyeres.2018.02.002
Roddy, G. W., Rosa, R. H. Jr., Oh, J. Y., Ylostalo, J. H., Bartosh, T. J. Jr., Choi, H., et al. (2012). Stanniocalcin-1 rescued photoreceptor degeneration in two rat models of inherited retinal degeneration. Mol. Ther. 20, 788–797. doi: 10.1038/mt.2011.308
Roddy, G. W., Yasumura, D., Matthes, M. T., Alavi, M. V., Boye, S. L., Rosa, H. R., et al. (2017). Long-term photoreceptor rescue in two rodent models of retinitis pigmentosa by adeno-associated virus delivery of Stanniocalcin-1. Exp. Eye Res. 65, 175–181. doi: 10.1016/j.exer.2017.09.011
Samardzija, M., Wariwoda, H., Imsand, C., Huber, P., Heynen, S. R., Gubler, A., et al. (2012). Activation of survival pathways in the degenerating retina of rd10 mice. Exp. Eye Res. 99, 17–26. doi: 10.1016/j.exer.2012.04.004
Sarapio, E., Souza, S. K., Vogt, E. L., Rocha, D. S., Fabres, R. B., Trapp, M., et al. (2019). Effects of stanniocalcin hormones on rat brown adipose tissue metabolism under fed and fasted conditions. Mol. Cell. Endocrinol. 485, 81–87. doi: 10.1016/j.mce.2019.02.004
Semenza, G. L. (2012). Hypoxia-inducible factors in physiology and medicine gregg. Cell 148, 399–408.
Thiersch, M., Raffelsberger, W., Frigg, R., Samardzija, M., Wenzel, A., Poch, O., et al. (2008). Analysis of the retinal gene expression profile after hypoxic preconditioning identifies candidate genes for neuroprotection. BMC Genomics 9:73. doi: 10.1186/1471-2164-9-73
Varghese, R., Gagliardi, A. D., Bialek, P. E., Yee, S. P., Wagner, G. F., and Dimattia, G. E. (2002). Overexpression of human stanniocalcin affects growth and reproduction in transgenic mice. Endocrinology 143, 868–876. doi: 10.1210/endo.143.3.8671
Vaupel, P., Kallinowski, F., and Okunieff, P. (1989). Blood flow, oxygen and nutrient supply, and metabolic microenvironment of human tumors: a review. Cancer Res. 49, 6449–6465.
Wagner, G. F., and DiMattia, G. E. (2006). The stanniocalcin family of proteins. J. Exp. Zool. 305A, 883–889. doi: 10.1002/jez.a.313
Wang, G. L., Jiang, B. H., Rue, E. A., and Semenza, G. L. (1995). Hypoxia-inducible factor 1 is a basic-helix-loop-helix-PAS heterodimer regulated by cellular O2 tension. Proc. Natl. Acad. Sci. U. S. A. 92, 5510–5514. doi: 10.1073/pnas.92.12.5510
Westberg, J. A., Serlachius, M., Lankila, P., and Andersson, L. C. (2007a). Hypoxic preconditioning induces elevated expression of stanniocalcin-1 in the heart. Am. J. Physiol. Heart Circ. Physiol. 93, 1766–1771. doi: 10.1152/ajpheart.00017.2007
Westberg, J. A., Serlachius, M., Lankila, P., Penkowa, M., Hidalgo, J., and Andersson, L. C. (2007b). Hypoxic preconditioning induces neuroprotective stanniocalcin-1 in brain via IL-6 signaling. Stroke 38, 1025–1030. doi: 10.1161/01.STR.0000258113.67252.fa
Yeung, B. H. Y., Law, A. Y. S., and Wong, C. K. C. (2012). Evolution and roles of stanniocalcin. Mol. Cell. Endocrinol. 349, 272–280. doi: 10.1016/j.mce.2011.11.007
Yeung, H. Y., Lai, K. P., Chan, H. Y., Mak, N. K., Wagner, G. F., and Wong, C. K. C. (2005). Hypoxia-inducible factor-1-mediated activation of stanniocalcin-1 in human cancer cells. Endocrinology 146, 4951–4960. doi: 10.1210/en.2005-0365
Yu, D.-Y., and Cringle, S. J. (2001). Oxygen distribution and consumption within the retina in vascularised and avascular retinas and in animal models of retinal disease. Prog. Retin. Eye Res. 20, 175–208. doi: 10.1016/s1350-9462(00)00027-6
Zeiger, W., Ito, D., Swetlik, C., Oh-hora, M., Villereal, M. L., and Thinakaran, G. (2011). Stanniocalcin 2 is a negative modulator of store-operated calcium entry. Mol. Cell. Biol. 31, 3710–3722. doi: 10.1128/mcb.05140-11
Zhang, K., Lindsberg, P. J., Tatlisumak, T., Kaste, M., Olsen, H. S., and Andersson, L. C. (2000). Stanniocalcin: a molecular guard of neurons during cerebral ischemia. Proc. Natl. Acad. Sci. 97, 3637–3642. doi: 10.1073/pnas.070045897
Zhao, J., Jiao, Y., Song, Y., Liu, J., Li, X., Zhang, H., et al. (2018). Stanniocalcin 2 ameliorates hepatosteatosis through activation of STAT3 signaling. Front. Physiol. 9:873. doi: 10.3389/fphys.2018.00873
Zhao, L., Brown, L. A., Owji, A. A., Nunez, D. J., Smith, D. M., Ghatei, M. A., et al. (1996). Adrenomedullin activity in chronically hypoxic rat lungs. Am. J. Physiol. 271, 622–629. doi: 10.1152/ajpheart.1996.271.2.H622
Keywords: stanniocalcin1 (Stc1), stanniocalcin2 (Stc2), hypoxia, hypoxia-inducible factor 1a (HIF1a), retinal stress
Citation: Ail D, Samardzija M, Chang ACM, Keck J, Reddel RR and Grimm C (2022) Stanniocalcin2, but Not Stanniocalcin1, Responds to Hypoxia in a HIF1-Dependent Manner in the Retina. Front. Neurosci. 16:882559. doi: 10.3389/fnins.2022.882559
Received: 23 February 2022; Accepted: 02 June 2022;
Published: 23 June 2022.
Edited by:
Markus Tschopp, University of Bern, SwitzerlandReviewed by:
Tudor Constantin Badea, Transilvania University of Braşov, RomaniaYonju Ha, University of Texas Medical Branch at Galveston, United States
Ying Xu, Jinan University, China
Copyright © 2022 Ail, Samardzija, Chang, Keck, Reddel and Grimm. This is an open-access article distributed under the terms of the Creative Commons Attribution License (CC BY). The use, distribution or reproduction in other forums is permitted, provided the original author(s) and the copyright owner(s) are credited and that the original publication in this journal is cited, in accordance with accepted academic practice. No use, distribution or reproduction is permitted which does not comply with these terms.
*Correspondence: Christian Grimm, Y2dyaW1tQG9waHQudXpoLmNo