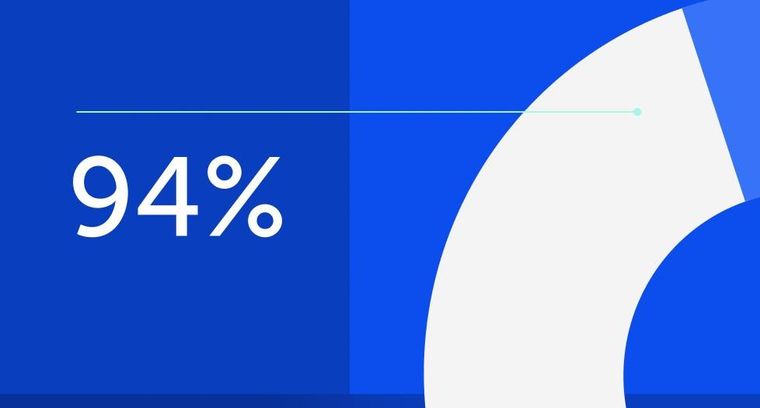
94% of researchers rate our articles as excellent or good
Learn more about the work of our research integrity team to safeguard the quality of each article we publish.
Find out more
PERSPECTIVE article
Front. Neurosci., 31 March 2022
Sec. Neurogenesis
Volume 16 - 2022 | https://doi.org/10.3389/fnins.2022.878875
This article is part of the Research TopicInsights in Neurogenesis: 2021View all 10 articles
The majority of adult neural stem cells (aNSCs) are in a distinct metabolic state of reversible cell cycle exit also known as quiescence. The rate of aNSC activation determines the number of new neurons generated and directly influences the long-term maintenance of neurogenesis. Despite its relevance, it is still unclear how aNSC quiescence is regulated. Many factors contribute to this, like aNSC heterogeneity, the lack of reliable quiescence markers, the complexity of the neurogenic niches or the intricacy of the transcriptional and post-transcriptional mechanisms involved. In this perspective article I discuss possible solutions to these problems. But, first and foremost, I believe we require a model that goes beyond a simple transition toward activation. Instead, we must acknowledge the full complexity of aNSC states, which include not only activation but also differentiation and survival as behavioural outcomes. I propose a model where aNSCs dynamically transition through a cloud of highly interlinked cellular states driven by intrinsic and extrinsic cues. I also show how a new perspective enables us to integrate current results into a coherent framework leading to the formulation of new testable hypothesis. This model, like all others, is still far from perfect and will be reshaped by future findings. I believe that having a more complete view of aNSC transitions and embracing their complexity will bring us closer to understand how aNSC activity and neurogenesis are controlled throughout life.
Neurogenesis is preserved in specific regions or neurogenic niches in the adult brains of most mammals (Ming and Song, 2011; Bond et al., 2015). The subependymal zone (SEZ) of the lateral ventricles and the subgranular zone (SGZ) of the dentate gyrus (DG) in the hippocampal formation are the two main neurogenic niches in the mouse brain (Gonçalves et al., 2016; Obernier and Alvarez-Buylla, 2019). Adult neural stem cells (aNSCs) in both niches are largely quiescent (Urbán et al., 2019). Quiescence is not only the reversible exit of stem cells from the cell cycle, but also involves significant metabolic changes and the maintenance of low transcription and translation levels (Urbán and Cheung, 2021). It is hypothesised that the quiescent state protects stem cells from DNA and protein damage (Cheung and Rando, 2013). Adult NSCs have a poor ability to self-renew, and consequently, their numbers (and thus the amount of newly generated neurons) fall significantly with age in an activation-dependent way (Encinas et al., 2011; Kalamakis et al., 2019). Quiescence prevents them from being depleted, ensuring a steady supply of new cells. Therefore, the rate of aNSC activation determines the number of new neurons generated and directly influences the long-term maintenance of neurogenesis. Adult-born neurons play important functions in olfaction, memory and mood regulation, with loss of neurogenesis associated with the loss of cognitive and affective functions (Bowers and Jessberger, 2016). Despite our best efforts, we still lack a clear picture of how the transitions of aNSCs from quiescent to active states are controlled, due to several reasons (Figure 1):
Figure 1. Summary of the current roadblocks in our understanding of adult neural stem cell activation. IPC, Intermediate progenitor cell.
- We still ignore how signal integration works in aNSCs. A variety of different signals have been involved in balancing quiescence and activation in these cells. For instance, NOTCH and BMP are pro-quiescence signals while WNT and SHH are considered pro-activation ones (Ahn and Joyner, 2005; Lie et al., 2005; Imayoshi et al., 2010; Mira et al., 2010). Metabolic cues and neurotransmitters such as GABA are also heavily involved (Alfonso et al., 2012; Berg et al., 2013; Knobloch and Jessberger, 2017; Paul et al., 2017; Catavero et al., 2018; Adusumilli et al., 2020). But in reality, cells rarely respond to a single signal, instead receiving a complex mix of signalling and metabolic cues simultaneously. The mechanisms aNSCs employ to integrate these signals and how signal dynamics influence quiescence are still poorly explored.
- Transcriptional profiling is not an accurate readout of the position of NSCs along the quiescence to activation transition. Recent data has pointed out that unlike other cell types, the correlation between mRNA and protein content in quiescent NSCs is very poor (Rossi et al., 2021). In addition, several pieces of evidence point to post-transcriptional and post-translational mechanisms being the main drivers of the transition of adult NSCs between quiescence and activation. A good example is the transcription factor ASCL1, which is essential for aNSCs to exit quiescence (Andersen et al., 2014). ASCL1 is controlled by many niche signals at different levels, from transcription to protein stability, and is therefore a perfect candidate (although likely not the only one) to integrate stimuli to make cellular fate choices. Remarkably, while ASCL1 protein is detected only in active aNSCs, Ascl1 mRNA levels are similar in quiescent and active DG aNSCs (Blomfield et al., 2019).
- Quiescence-specific markers do not exist for aNSCs. Despite many genes being enriched in quiescent NSCs compared with active NSCs, none of them has been proven to be a reliable quiescence-specific marker. The main reason for this is the huge overlap in expression profile between astrocytes and aNSCs (which are correctly called radial glia-like cells) (Urbán et al., 2019). This problem clearly demonstrates how little we know about what defines quiescence, aside from the absence of cell cycle markers.
- Adult neural stem cells are heterogeneous. SEZ NSCs are spatially heterogeneous, as they retain the regional identity that was instructed to them during development (Merkle et al., 2014; Fiorelli et al., 2015; Mizrak et al., 2019). Spatial heterogeneity has so far not been described for the DG but there, aNSCs labelled with different lineage tracing strategies show distinct activation and differentiation potential (Bonaguidi et al., 2011; Bottes et al., 2021). Not only that, but they behave differently depending on their previous activation history, with those that recently activated (resting) being much more likely to proliferate again than the ones which have remained quiescent for a long time (dormant) (Urbán et al., 2016). However, direct proof of the existence of independent lineages of DG aNSCs is still missing, as their behavioural heterogeneity has so far not been confirmed by single cell RNA sequencing (scRNAseq) data. In addition, aNSCs are heterogeneous in their response to signalling cues. In active NSCs, WNT stimulation promotes exit from the cell cycle and neuronal differentiation but the very same signal triggers proliferation in quiescent aNSCs (Austin et al., 2021). The nature and consequences of aNSC heterogeneity remain unexplored, but heterogeneity has been proposed to avoid the exhaustion of the stem cell pool upon receiving a pro-activation signal (Kalamakis et al., 2019; Martín-Suárez et al., 2019; Harris et al., 2020).
- Likely underpinning all the above, we are still lacking a true profile of NSC quiescence in vivo. The maintenance of quiescence depends on intrinsic as well as niche signals. The mere fact of dissociating the tissue for subsequent sorting and sequencing is enough to induce the activation of satellite cells, the resident quiescent cells of the muscle (Machado et al., 2017; van Velthoven et al., 2017). Adult NSCs are also highly dependent on niche signals and cell-cell contacts for maintaining quiescence and thus likely to suffer from similar dissociation-dependent artefacts. This could explain the high percentage of activated and primed populations of NSCs identified using RNA sequencing in comparison to data obtained through immunohistochemistry.
The points above show that improved methods are needed to reliably detect the steps followed by adult NSCs as they exit quiescence and the mechanisms that drive their switch to activation.
Even though mRNA content might not be the biggest determinant of aNSC fate, heterogeneity is noticeable in published scRNAseq data in both SEZ and DG NSCs (Llorens-Bobadilla et al., 2015; Shin et al., 2015; Artegiani et al., 2017; Dulken et al., 2017; Basak et al., 2018; Hochgerner et al., 2018). But in order to capture the true signature of quiescent NSCs we must make sure to avoid dissociation artefacts. One way to do so would be to perform spatial transcriptomics on brain samples. However, even with the latest advances, the sequencing depth and cellular resolution of this technique is not yet enough for assessing differences between aNSCs. Alternatively, we could adapt to the brain niches the method that was used to solve the very same problem in satellite stem cells. By mildly perfusing the mice prior to tissue dissection we should be able to lock NSCs states while preserving RNA of enough quality for subsequent sequencing (Yue and Cheung, 2020).
But since changes in signalling and protein stability are crucial for aNSC decisions, we cannot rely solely on transcriptional data. One way to complement transcriptional approaches is by multiplexed immunohistochemistry, with which it will be possible to identify stem cells along with signalling and cell fate readouts (Cole et al., 2022). One immediate caveat of this technique is that it relies on the quality of the available antibodies, which is often sub-optimal for phospho-proteins and other signalling-related post-transcriptional modifications (PTMs). It is therefore necessary to generate reporter lines to closely monitor signalling pathway activation along aNSC transitions.
Ultimately, we will need proof that given signalling or metabolic cues are responsible for the transition of aNSCs through different states. For this, we must be able to manipulate those cues on aNSCs and evaluate their functional outcome. The scarcity of aNSCs, the lack of reliable unique markers for them and the complexity of their surrounding niches make such an approach extremely challenging in vivo. This problem can be overcome by using a simplified, controlled in vitro system. Adult NSCs can be isolated from both the SEZ and DG and remain in a proliferative, undifferentiated state for many passages in culture. Upon addition of BMP4, known to maintain quiescence in vivo, the cells enter a reversible quiescence-like state. The system, first described by Mira et al. (2010) and further developed by many other groups, recapitulates important aspects of quiescence, including withdrawal from the cell cycle, upregulation of astroglial markers and profound metabolic changes (Mira et al., 2010; Martynoga et al., 2013; Knobloch et al., 2017; Leeman et al., 2018). The simplicity of the system allows to directly measure the effects of signalling and metabolic cues on aNSC behaviour.
Last but not least, in order to integrate recent discoveries on the tremendous complexity of quiescence regulation in adult NSCs, we need a new framework that goes beyond our existing concept of a linear shift from quiescence to activation.
Single cell RNA sequencing changed our view from discrete active or quiescent populations of aNSCs to a continuous transition of states (Urbán et al., 2019). Pseudo-time analysis then helped streamline the data and focus the attention on those genes expressed highest or lowest in quiescence and which are down or upregulated, respectively, during activation (Shin et al., 2015; Dulken et al., 2017). Different degrees of quiescence have been identified, depending on how close the transcriptional profile is to the deepest or shallowest end of the quiescence-to-activation spectrum (deep, dormant, primed, active, etc.). While this has been extremely helpful, it has also oversimplified our view of quiescence.
To fully understand the quiescence to activation transitions of aNSCs (and in fact any cellular transition), we must embrace their complexity in full. Therefore, instead of the classical view of a linear transition from quiescence to activation, I propose a model where adult NSCs exist in a vast matrix of cellular states (Figure 2). These states are not restricted to a switch between activation and quiescence, but also involve other stem cell characteristics. In this complete view, NSCs transit through a wide spectrum of states which differ in their differentiation, proliferation, metabolic, fitness and survival capabilities. In the case of adult NSCs, the drains (i.e., the ways in which NSCs stop being stem cells) are four: neurogenesis, gliogenesis, senescence and apoptosis. For NSCs in the DG, neurogenesis is the one with the most weight, as gliogenesis (mostly astrogliogenesis) happens rarely (Figure 2A). For the SEZ, both gliogenesis (preferentially oligodendrogenesis) and neurogenesis constitute important drains (Figure 2B). In both niches, apoptosis of NSCs is relatively low and senescence gains importance as ageing progresses.
Figure 2. A complete view of aNSC quiescence. (A) Representation of the states matrix for the DG showing the four main outlets, neurogenesis, astrogenesis, senescence, and apoptosis. Their relative weight depends on the age and condition (e.g., stress levels, disease, etc.) of the animal. (B) Representation of the states matrix for the SEZ, in which oligodendrogenesis is also an important outlet. S, senescence; A, astrogenesis; O, oligodendrogenesis; N, neurogenesis. (C) cell can reversibly transition through the matrix space and several paths are possible for the same outcome (in this case, neurogenesis). (D) One of our hypotheses is that systemic stimuli, such as diet of exercise, will shift the cells in the matrix to a slightly different state. This could then affect how they respond to additional stimuli. (E) We also notice that proliferation is inversely correlated with stemness, which suggests that quiescence and stemness could be functionally linked. aNSCs lose self-renewing potential as they continue proliferating. S.R.: symmetric self-renewal, A.R.: asymmetric self-renewal, S.D.: symmetric differentiation.
At one specific time each NSC cell occupies a defined position in the states matrix. But if we were able to follow that same NSC over time we would be able to observe it travelling (or reversibly transitioning) through different positions (Figure 2C). NSCs in opposite sides of the matrix can present very different transcriptional profiles, with cells leaning toward activation expressing cell cycle genes and those close to the astrogliogenic output expressing astrocyte-enriched genes. ScRNAseq data could therefore be used as a starting point to roughly position cells in the matrix and identify the factors and cues driving NSC behaviour. Provided sequencing data is of enough quality and depth, we could even gain insight into the intrinsic and niche-related differences between SEZ and DG and identify age-related changes in NSC states. This has already been possible with current sequencing pipelines and should be very much improved when paired to techniques aimed at conserving the quiescence signature in vivo (Dulken et al., 2017; Kalamakis et al., 2019; Mizrak et al., 2019; Borrett et al., 2020, 2022). The use of tools such RNAvelocity (La Manno et al., 2018) and CellRank (Lange et al., 2022) on scRNAseq data will allow us to measure the direction and speed of cells travelling through the matrix and help us identify regions where one direction is favoured (where the probability of going back to the previous state is much lower than that of continuing in the same direction).
However at shorter distances, which are those important for the actual behavioural decisions of aNSCs, transcriptional differences can be small, noisy and, I would argue, rather meaningless. The main drivers of the movement of adult NSCs through different states are signalling cues and the activity of intrinsic factors. To fully understand how NSCs navigate through these spaces, the focus of future research should lay on signal integration, protein stability and PTMs. One way to integrate signalling activity with aNSC behavioural output would be to focus on known direct readouts of signalling pathways (through direct detection or the use of reporters) and correlate them with stemness, proliferation, differentiation, cell death and senescence markers. This, while extremely challenging in vivo, would be fully possible to achieve thanks to the in vitro system. In vitro aNSC states exist in a fully defined and stable environment and are thus unlikely to be common (or even exist) in vivo. Direct manipulation of signalling and metabolism in aNSC in vitro will also allow evaluating the effects of single and combined cues on the transition of aNSCs between states. In addition, the in vitro system will enable us to determine the degree of intrinsic heterogeneity of adult NSCs in different conditions. Intrinsic heterogeneity can be driven, for instance, by oscillations in the levels of key transcription factors [ASCL1 oscillates in embryonic NSCs (Imayoshi et al., 2013)] and the rhythmicity of signalling cascades (like NOTCH or WNT). Cyclic expression of fate determinants belonging to gene regulatory networks has been suggested to underlie the multipotency of neural crest and neural stem cells (Imayoshi and Kageyama, 2014; Kelsh et al., 2021).
The new framework immediately prompts us to think of the heterogeneity of stem cells in a different way and allows the integration of clonal and label-retention data without the need of invoking the existence of distinct stem cell types. The clonal approaches typically used for the study of adult neurogenesis label those cells with the highest activity of a particular promoter at the time of tamoxifen administration. They will therefore label the positions in the matrix where that promoter is most active. But labelled cells might not constitute a separate lineage, as they do not necessarily need to be restricted to one single area of the matrix and could partially overlap with the high activity of other promoters also used for clonal analysis. For instance, high activity of the Gli1 promoter could happen anywhere where SHH signalling is high and is likely to overlap, at least partially, with high expression of unrelated genes such as Nestin or Ascl1. It is important to note that promoter activity and gene function do not always come hand in hand. Even less in adult NSCs, where mRNA and protein are poorly correlated. Nevertheless, the observed behavioural biases could still be linked to the function of the gene used for labelling the cells. For instance, high Ascl1 mRNA expression, although does not always lead to activation due to post-transcriptional regulation, could increase the probability of NSCs to activate. On the other hand, it is easy to imagine that resting NSCs, which recently proliferated, are likely to remain in a pro-activation zone after division. This alone will make them more likely to become activated again than dormant NSCs, which did not activate during the labelling period. Finally, cells which are very distant in the matrix might have very distinct signal transduction capabilities, both due to differential expression of signalling pathway components and different metabolic characteristics. This readily explains how NSCs respond differently to the same stimuli depending on their state (e.g., response to WNT in active vs. quiescent cells).
The model makes it clear that the likeliness of a NSC becoming activated depends on two things: their current state (the closer to the activation zone, the most likely it is that they will become activated), and their signalling environment (how likely it is that they will receive the appropriate activation signals). But what I personally find most exciting about this framework is that it allows us to generate hypothesis and design specific experiments to test them. Two particularly tantalising ones are:
One still unanswered question in the field is how interventions that affect neurogenesis impact the maintenance of aNSCs over long periods of time. Stimuli such as exercise, dietary changes or an enriched environment affect adult neurogenesis (Trejo et al., 2001; Overall et al., 2016; Kempermann, 2019; Dias et al., 2021). However, virtually nothing is known about their long-term influence on NSC quiescence. In the light of the new model, I propose that these stimuli shift aNSCs to a different state, changing the whole range of aNSC states (Figure 2D). This resembles how ageing pushes aNSCs toward senescence, injury toward activation or epilepsy toward astrogenesis. Indeed, the global changes in aNSC behaviour over time suggest that ageing heavily influences the positioning of aNSC in the matrix (Kalamakis et al., 2019; Martín-Suárez et al., 2019; Harris et al., 2020). For more subtle stimuli, the shift might not result in an obvious, immediate phenotype. But it will affect the response of NSCs to additional signals and systemic cues, including ageing. Evaluating potential effects on quiescence is of particular importance for interventions suggested to counteract ageing, as we risk generating a brief burst in neurogenesis at the cost of exhausting aNSCs at an even faster pace.
Several pieces of independent evidence suggest that quiescence does not serve the sole purpose of pausing the cell cycle. Stem cell-related markers (e.g., Sox9, Hopx) are highest in the most quiescent cells and repeated activation of DG NSCs in vivo is associated with the loss of their self-renewing ability (Llorens-Bobadilla et al., 2015; Shin et al., 2015; Pilz et al., 2018; Bottes et al., 2021). This suggests that quiescence is important for the self-renewal capacity of adult NSCs (Figure 2E). Such a connection is evident from our model, which also points that in order to measure it, we need to go beyond merely assessing proliferation levels and jointly analyse fitness and self-renewal capacity of adult NSCs as they transition from quiescent to active states. Determining if and how quiescence and self-renewal are linked will help devise better strategies to avoid NSC exhaustion while still generating enough neurons to maintain functionality throughout life.
The way we think about a subject often affects the questions we ask and due to the oversimplification associated with models, significant features could be overlooked. I believe it is time to incorporate the intricacy of stem cell behaviour into our working models, even if we still do not have enough data to fully support them. In fact, this, as any model is only meant to serve as a starting point, since it will continuously evolve through the addition of new data. Experiments, on the other hand, are independent of the model and will remain significant regardless of it. Although my focus is on aNSCs, the proposed framework will be useful for other adult and embryonic stem cell transitions, reprogramming strategies and cancer research. One interesting avenue would be to further explore the continuum of states that exist between glia and neural stem cells. Glial cells respond to injury and have the potential to generate new neurons in the adult brain. By investigating the aNSC to glia transition as well as the reactivation path of glial cells, we could identify important roadblocks for glia-to-neuron transitions (Gascón et al., 2017). Other systems, particularly simpler models, could teach us a lot about the basic principles governing cell fate transitions. Similarly, looking at other cells in the niche will provide a more complete picture of aNSC regulation and help us understand the significance of cell-cell interactions. Through the combination of new approaches and this framework we may soon be able to understand the rules of the game and make sense of the apparent stochasticity of aNSC activation.
The original contributions presented in the study are included in the article/supplementary material, further inquiries can be directed to the corresponding author.
NU conceived and wrote the manuscript.
NU was supported by IMBA, from the Austrian Academy of Sciences (ÖAW). Work in her lab was further supported with funding from the Austrian Science Fund (FWF, programs SFB-78, SFB-79, docfunds SCORPION and docfunds SMICH).
The author declares that the research was conducted in the absence of any commercial or financial relationships that could be construed as a potential conflict of interest.
The reviewer JE-P declared a past co-authorship with the author NU to the handling editor.
All claims expressed in this article are solely those of the authors and do not necessarily represent those of their affiliated organizations, or those of the publisher, the editors and the reviewers. Any product that may be evaluated in this article, or claim that may be made by its manufacturer, is not guaranteed or endorsed by the publisher.
I would like to thank our graphics department, and in particular Johannes Tkadletz, for help with the figures and all Urbán lab members for lively discussions and helpful comments.
Adusumilli, V. S., Walker, T. L., Overall, R. W., Klatt, G. M., Zeidan, S. A., Zocher, S., et al. (2020). ROS Dynamics Delineate Functional States of Hippocampal Neural Stem Cells and Link to Their Activity-Dependent Exit from Quiescence. Cell Stem Cell 28, 300–314.e6. doi: 10.1016/j.stem.2020.10.019
Ahn, S., and Joyner, A. L. (2005). In vivo analysis of quiescent adult neural stem cells responding to Sonic hedgehog. Nature 437, 894–897. doi: 10.1038/nature03994
Alfonso, J., Le Magueresse, C., Zuccotti, A., Khodosevich, K., and Monyer, H. (2012). Diazepam binding inhibitor promotes progenitor proliferation in the postnatal SVZ by reducing GABA signaling. Cell Stem Cell 10, 76–87. doi: 10.1016/j.stem.2011.11.011
Andersen, J., Urbán, N., Achimastou, A., Ito, A., Simic, M., Ullom, K., et al. (2014). A transcriptional mechanism integrating inputs from extracellular signals to activate hippocampal stem cells. Neuron 83, 1085–1097. doi: 10.1016/j.neuron.2014.08.004
Artegiani, B., Lyubimova, A., Muraro, M., van Es, J. H., van Oudenaarden, A., and Clevers, H. (2017). A Single-Cell RNA Sequencing Study Reveals Cellular and Molecular Dynamics of the Hippocampal Neurogenic Niche. Cell Rep. 21, 3271–3284. doi: 10.1016/j.celrep.2017.11.050
Austin, S. H. L., Gabarró-Solanas, R., Rigo, P., Paun, O., Harris, L., Guillemot, F., et al. (2021). Wnt/β-catenin signalling is dispensable for adult neural stem cell homeostasis and activation. Development 148:dev199629. doi: 10.1242/dev.199629
Basak, O., Krieger, T. G., Muraro, M. J., Wiebrands, K., Stange, D. E., Frias-Aldeguer, J., et al. (2018). Troy+ brain stem cells cycle through quiescence and regulate their number by sensing niche occupancy. Proc. Natl. Acad. Sci. U. S. A. 115, E610–E619. doi: 10.1073/pnas.1715911114
Berg, D. A., Belnoue, L., Song, H., and Simon, A. (2013). Neurotransmitter-mediated control of neurogenesis in the adult vertebrate brain. Development 140, 2548–2561. doi: 10.1242/dev.088005
Blomfield, I. M., Rocamonde, B., Masdeu, M. D. M., Mulugeta, E., Vaga, S., van den Berg, D. L., et al. (2019). Id4 promotes the elimination of the pro-activation factor Ascl1 to maintain quiescence of adult hippocampal stem cells. Elife 8:e48561. doi: 10.7554/eLife.48561
Bonaguidi, M. A., Wheeler, M. A., Shapiro, J. S., Stadel, R. P., Sun, G. J., Ming, G., et al. (2011). In vivo clonal analysis reveals self-renewing and multipotent adult neural stem cell characteristics. Cell 145, 1142–1155. doi: 10.1016/j.cell.2011.05.024
Bond, A. M., Ming, G.-L., and Song, H. (2015). Adult Mammalian Neural Stem Cells and Neurogenesis: five Decades Later. Cell Stem Cell 17, 385–395. doi: 10.1016/j.stem.2015.09.003
Borrett, M. J., Innes, B. T., Jeong, D., Tahmasian, N., Storer, M. A., Bader, G. D., et al. (2020). Single-Cell Profiling Shows Murine Forebrain Neural Stem Cells Reacquire a Developmental State when Activated for Adult Neurogenesis. Cell Rep. 32:108022. doi: 10.1016/j.celrep.2020.108022
Borrett, M. J., Innes, B. T., Tahmasian, N., Bader, G. D., Kaplan, D. R., and Miller, F. D. (2022). A Shared Transcriptional Identity for Forebrain and Dentate Gyrus Neural Stem Cells from Embryogenesis to Adulthood. Eneuro 9, ENEURO.0271–21.2021. doi: 10.1523/ENEURO.0271-21.2021
Bottes, S., Jaeger, B. N., Pilz, G.-A., Jörg, D. J., Cole, J. D., Kruse, M., et al. (2021). Long-term self-renewing stem cells in the adult mouse hippocampus identified by intravital imaging. Nat. Neurosci. 24, 225–233. doi: 10.1038/s41593-020-00759-4
Bowers, M., and Jessberger, S. (2016). Linking adult hippocampal neurogenesis with human physiology and disease. Dev. Dyn. 245, 702–709. doi: 10.1002/dvdy.24396
Catavero, C., Bao, H., and Song, J. (2018). Neural mechanisms underlying GABAergic regulation of adult hippocampal neurogenesis. Cell Tissue Res. 371, 33–46. doi: 10.1007/s00441-017-2668-y
Cheung, T. H., and Rando, T. A. (2013). Molecular regulation of stem cell quiescence. Nat. Rev. Mol. Cell. Biol. 14, 329–340. doi: 10.1038/nrm3591
Cole, J. D., Sarabia Del Castillo, J., Gut, G., Gonzalez-Bohorquez, D., Pelkmans, L., and Jessberger, S. (2022). Characterization of the neurogenic niche in the aging dentate gyrus using iterative immunofluorescence imaging. Elife 11:e68000. doi: 10.7554/eLife.68000
Dias, G. P., Murphy, T., Stangl, D., Ahmet, S., Morisse, B., Nix, A., et al. (2021). Intermittent fasting enhances long-term memory consolidation, adult hippocampal neurogenesis, and expression of longevity gene Klotho. Mol. Psychiatry 26, 6365–6379. doi: 10.1038/s41380-021-01102-4
Dulken, B. W., Leeman, D. S., Boutet, S. C., Hebestreit, K., and Brunet, A. (2017). Single-Cell Transcriptomic Analysis Defines Heterogeneity and Transcriptional Dynamics in the Adult Neural Stem Cell Lineage. Cell Rep. 18, 777–790. doi: 10.1016/j.celrep.2016.12.060
Encinas, J. M., Michurina, T. V., Peunova, N., Park, J.-H., Tordo, J., Peterson, D. A., et al. (2011). Division-Coupled Astrocytic Differentiation and Age-Related Depletion of Neural Stem Cells in the Adult Hippocampus. Cell Stem Cell 8, 566–579. doi: 10.1016/j.stem.2011.03.010
Fiorelli, R., Azim, K., Fischer, B., and Raineteau, O. (2015). Adding a spatial dimension to postnatal ventricular-subventricular zone neurogenesis. Development 142, 2109–2120. doi: 10.1242/dev.119966
Gascón, S., Masserdotti, G., Russo, G. L., and Götz, M. (2017). Direct Neuronal Reprogramming: achievements, Hurdles, and New Roads to Success. Cell Stem Cell 21, 18–34. doi: 10.1016/j.stem.2017.06.011
Gonçalves, J. T., Schafer, S. T., and Gage, F. H. (2016). Adult Neurogenesis in the Hippocampus: from Stem Cells to Behavior. Cell 167, 897–914. doi: 10.1016/j.cell.2016.10.021
Harris, L., Rigo, P., Stiehl, T., Gaber, Z., Austin, S. H. L., Masdeu, M., et al. (2020). Progressive changes in hippocampal stem cell properties ensure lifelong neurogenesis. Biorxiv doi: 10.1101/2020.03.12.987107
Hochgerner, H., Zeisel, A., Lönnerberg, P., and Linnarsson, S. (2018). Conserved properties of dentate gyrus neurogenesis across postnatal development revealed by single-cell RNA sequencing. Nat. Neurosci. 21, 290–299. doi: 10.1038/s41593-017-0056-2
Imayoshi, I., Isomura, A., Harima, Y., Kawaguchi, K., Kori, H., Miyachi, H., et al. (2013). Oscillatory control of factors determining multipotency and fate in mouse neural progenitors. Science 342, 1203–1208. doi: 10.1126/science.1242366
Imayoshi, I., and Kageyama, R. (2014). Oscillatory control of bHLH factors in neural progenitors. Trends Neurosci. 37, 531–538. doi: 10.1016/j.tins.2014.07.006
Imayoshi, I., Sakamoto, M., Yamaguchi, M., Mori, K., and Kageyama, R. (2010). Essential roles of Notch signaling in maintenance of neural stem cells in developing and adult brains. J. Neurosci. 30, 3489–3498. doi: 10.1523/JNEUROSCI.4987-09.2010
Kalamakis, G., Brüne, D., Ravichandran, S., Bolz, J., Fan, W., Ziebell, F., et al. (2019). Quiescence Modulates Stem Cell Maintenance and Regenerative Capacity in the Aging Brain. Cell 176, 1407–1419.e14. doi: 10.1016/j.cell.2019.01.040
Kelsh, R. N., Camargo Sosa, K., Farjami, S., Makeev, V., Dawes, J. H. P., and Rocco, A. (2021). Cyclical fate restriction: a new view of neural crest cell fate specification. Development 148:dev176057. doi: 10.1242/dev.176057
Kempermann, G. (2019). Environmental enrichment, new neurons and the neurobiology of individuality. Nat. Rev. Neurosci. 20, 235–245. doi: 10.1038/s41583-019-0120-x
Knobloch, M., and Jessberger, S. (2017). Metabolism and neurogenesis. Curr. Opin. Neurobiol. 42, 45–52. doi: 10.1016/j.conb.2016.11.006
Knobloch, M., Pilz, G.-A., Ghesquière, B., Kovacs, W. J., Wegleiter, T., Moore, D. L., et al. (2017). A Fatty Acid Oxidation-Dependent Metabolic Shift Regulates Adult Neural Stem Cell Activity. Cell Rep. 20, 2144–2155. doi: 10.1016/j.celrep.2017.08.029
La Manno, G., Soldatov, R., Zeisel, A., Braun, E., Hochgerner, H., Petukhov, V., et al. (2018). RNA velocity of single cells. Nature 560, 494–498. doi: 10.1038/s41586-018-0414-6
Lange, M., Bergen, V., Klein, M., Setty, M., Reuter, B., Bakhti, M., et al. (2022). CellRank for directed single-cell fate mapping. Nat. Methods 19, 159–170. doi: 10.1038/s41592-021-01346-6
Leeman, D. S., Hebestreit, K., Ruetz, T., Webb, A. E., McKay, A., Pollina, E. A., et al. (2018). Lysosome activation clears aggregates and enhances quiescent neural stem cell activation during aging. Science 359, 1277–1283. doi: 10.1126/science.aag3048
Lie, D.-C., Colamarino, S. A., Song, H.-J., Désiré, L., Mira, H., Consiglio, A., et al. (2005). Wnt signalling regulates adult hippocampal neurogenesis. Nature 437, 1370–1375. doi: 10.1038/nature04108
Llorens-Bobadilla, E., Zhao, S., Baser, A., Saiz-Castro, G., Zwadlo, K., and Martin-Villalba, A. (2015). Single-Cell Transcriptomics Reveals a Population of Dormant Neural Stem Cells that Become Activated upon Brain Injury. Cell Stem Cell 17, 329–340. doi: 10.1016/j.stem.2015.07.002
Machado, L., Esteves de Lima, J., Fabre, O., Proux, C., Legendre, R., Szegedi, A., et al. (2017). In Situ Fixation Redefines Quiescence and Early Activation of Skeletal Muscle Stem Cells. Cell Rep. 21, 1982–1993. doi: 10.1016/j.celrep.2017.10.080
Martín-Suárez, S., Valero, J., Muro-García, T., and Encinas, J. M. (2019). Phenotypical and functional heterogeneity of neural stem cells in the aged hippocampus. Aging Cell 18:e12958. doi: 10.1111/acel.12958
Martynoga, B., Mateo, J. L., Zhou, B., Andersen, J., Achimastou, A., Urbán, N., et al. (2013). Epigenomic enhancer annotation reveals a key role for NFIX in neural stem cell quiescence. Genes Dev. 27, 1769–1786. doi: 10.1101/gad.216804.113
Merkle, F. T., Fuentealba, L. C., Sanders, T. A., Magno, L., Kessaris, N., and Alvarez-Buylla, A. (2014). Adult neural stem cells in distinct microdomains generate previously unknown interneuron types. Nat. Neurosci. 17, 207–214. doi: 10.1038/nn.3610
Ming, G., and Song, H. (2011). Adult Neurogenesis in the Mammalian Brain: significant Answers and Significant Questions. Neuron 70, 687–702. doi: 10.1016/j.neuron.2011.05.001
Mira, H., Andreu, Z., Suh, H., Lie, D. C., Jessberger, S., Consiglio, A., et al. (2010). Signaling through BMPR-IA Regulates Quiescence and Long-Term Activity of Neural Stem Cells in the Adult Hippocampus. Cell Stem Cell 7, 78–89. doi: 10.1016/j.stem.2010.04.016
Mizrak, D., Levitin, H. M., Delgado, A. C., Crotet, V., Yuan, J., Chaker, Z., et al. (2019). Single-Cell Analysis of Regional Differences in Adult V-SVZ Neural Stem Cell Lineages. Cell Rep. 26, 394–406.e5. doi: 10.1016/j.celrep.2018.12.044
Obernier, K., and Alvarez-Buylla, A. (2019). Neural stem cells: origin, heterogeneity and regulation in the adult mammalian brain. Development 146:dev156059. doi: 10.1242/dev.156059
Overall, R. W., Walker, T. L., Fischer, T. J., Brandt, M. D., and Kempermann, G. (2016). Different Mechanisms Must Be Considered to Explain the Increase in Hippocampal Neural Precursor Cell Proliferation by Physical Activity. Front. Neurosci. 10:362. doi: 10.3389/fnins.2016.00362
Paul, A., Chaker, Z., and Doetsch, F. (2017). Hypothalamic regulation of regionally distinct adult neural stem cells and neurogenesis. Science 356, 1383–1386. doi: 10.1126/science.aal3839
Pilz, G.-A., Bottes, S., Betizeau, M., Jörg, D. J., Carta, S., Simons, B. D., et al. (2018). Live imaging of neurogenesis in the adult mouse hippocampus. Science 359, 658–662. doi: 10.1126/science.aao5056
Rossi, A., Coum, A., Madelenat, M., Harris, L., Miedzik, A., Strohbuecker, S., et al. (2021). Neural stem cells alter nucleocytoplasmic partitioning and accumulate nuclear polyadenylated transcripts during quiescence. Biorxiv doi: 10.1101/2021.01.06.425462
Shin, J., Berg, D. A., Zhu, Y., Shin, J. Y., Song, J., Bonaguidi, M. A., et al. (2015). Single-Cell RNA-Seq with Waterfall Reveals Molecular Cascades underlying Adult Neurogenesis. Cell Stem Cell 17, 360–372. doi: 10.1016/j.stem.2015.07.013
Trejo, J. L., Carro, E., and Torres-Alemán, I. (2001). Circulating Insulin-Like Growth Factor I Mediates Exercise-Induced Increases in the Number of New Neurons in the Adult Hippocampus. J. Neurosci. 21, 1628–1634. doi: 10.1523/JNEUROSCI.21-05-01628.2001
Urbán, N., Blomfield, I. M., and Guillemot, F. (2019). Quiescence of Adult Mammalian Neural Stem Cells: a Highly Regulated Rest. Neuron 104, 834–848. doi: 10.1016/j.neuron.2019.09.026
Urbán, N., and Cheung, T. H. (2021). Stem cell quiescence: the challenging path to activation. Development 148, dev165084. doi: 10.1242/dev.165084
Urbán, N., van den Berg, D. L. C., Forget, A., Andersen, J., Demmers, J. A. A., Hunt, C., et al. (2016). Return to quiescence of mouse neural stem cells by degradation of a proactivation protein. Science 353, 292–295. doi: 10.1126/science.aaf4802
van Velthoven, C. T. J., de Morree, A., Egner, I. M., Brett, J. O., and Rando, T. A. (2017). Transcriptional Profiling of Quiescent Muscle Stem Cells In Vivo. Cell Rep. 21, 1994–2004. doi: 10.1016/j.celrep.2017.10.037
Keywords: adult neurogenesis, adult stem cells, adult neural stem cells, activation, working model, NSC transitions, dentate gyrus, subependymal zone
Citation: Urbán N (2022) Could a Different View of Quiescence Help Us Understand How Neurogenesis Is Regulated? Front. Neurosci. 16:878875. doi: 10.3389/fnins.2022.878875
Received: 18 February 2022; Accepted: 09 March 2022;
Published: 31 March 2022.
Edited by:
Luca Bonfanti, University of Turin, ItalyReviewed by:
Juan Manuel Encinas-Pérez, Achucarro Basque Center for Neuroscience, SpainCopyright © 2022 Urbán. This is an open-access article distributed under the terms of the Creative Commons Attribution License (CC BY). The use, distribution or reproduction in other forums is permitted, provided the original author(s) and the copyright owner(s) are credited and that the original publication in this journal is cited, in accordance with accepted academic practice. No use, distribution or reproduction is permitted which does not comply with these terms.
*Correspondence: Noelia Urbán, bm9lbGlhLnVyYmFuQGltYmEub2Vhdy5hYy5hdA==
Disclaimer: All claims expressed in this article are solely those of the authors and do not necessarily represent those of their affiliated organizations, or those of the publisher, the editors and the reviewers. Any product that may be evaluated in this article or claim that may be made by its manufacturer is not guaranteed or endorsed by the publisher.
Research integrity at Frontiers
Learn more about the work of our research integrity team to safeguard the quality of each article we publish.