- Department of Neurotoxicology, Mossakowski Medical Research Institute-Polish Academy of Sciences, Warsaw, Poland
Acute liver failure (ALF) impairs ammonia clearance from blood, which gives rise to acute hyperammonemia and increased ammonia accumulation in the brain. Since in brain glutamine synthesis is the only route of ammonia detoxification, hyperammonemia is as a rule associated with increased brain glutamine content (glutaminosis) which correlates with and contributes along with ammonia itself to hyperammonemic brain edema-associated with ALF. This review focuses on the effects of hyperammonemia on the two glutamine carriers located in the astrocytic membrane: Slc38a3 (SN1, SNAT3) and Slc7a6 (y + LAT2). We emphasize the contribution of the dysfunction of either of the two carriers to glutaminosis- related aspects of brain edema: retention of osmotically obligated water (Slc38a3) and induction of oxidative/nitrosative stress (Slc7a6). The changes in glutamine transport link glutaminosis- evoked mitochondrial dysfunction to oxidative-nitrosative stress as formulated in the “Trojan Horse” hypothesis.
Metabolic Characteristics of Glutamine in Mammalian Tissues: A Brief Account
The presence and roles of glutamine common to all mammalian tissues have been dealt with in many excellent reviews (Häussinger, 1990; Labow and Souba, 2000; Tapiero et al., 2002; Oehler and Roth, 2003; Bak et al., 2006; Hakvoort et al., 2017), and therefore are mentioned here only briefly. Glutamine is the most abundant free amino acid and accounts for ∼50% of the total free amino acids in the human body (Spodenkiewicz et al., 2016). In peripheral tissues, glutamine is most abundantly represented in muscles (more than 40% of the free amino acid pool) and blood plasma (more than 20%, concentrations ranging from 0.4 to 0.7 mM) (Bergström et al., 1974; Kuhn et al., 1999). Glutamine participates in a variety of metabolic pathways, thereby controlling an abundance of biological processes. Ubiquitously, it serves as a precursor of purines and pyrimidines, is involved in the synthesis of amino sugars, regulation of pH, glutathione homeostasis, energy production, etc. (Cory and Cory, 2006; Albrecht et al., 2010). Systemically, enhanced glutamine synthesis exerts beneficial effects on the immune system (Castell et al., 2004) or gut barrier (Krishna Rao, 2012; Wang et al., 2015). Glutamine also stimulates branched-chain amino acids catabolism in skeletal muscle (Mann et al., 2021).
Glutamine Functioning in the Brain Depends on Its Mobility
In the mammalian brain, glutamine is a conditionally essential amino acid, synthesized from glutamate and ammonia, in a reaction mediated by glutamine synthetase (GS), an enzyme overwhelmingly (Martinez-Hernandez et al., 1977), albeit not exclusively (Amaral et al., 2013), located in astrocytes. Astrocytic glutamine is a precursor of the releasable pool of the excitatory neurotransmitter amino acid glutamate and the inhibitory amino acid, γ-aminobutyric acid (GABA), a sequence of reactions characterized by rapid cellular turnover rates (Bak et al., 2006; Rose et al., 2013). Glutamine released by astrocytes is taken up by neurons. In the mitochondria of neurons, glutamine is transformed by phosphate-activated glutaminase (PAG) into glutamate, released as a neurotransmitter into the synaptic space, from where it is taken up by astrocytes, and conjugates with ammonia to form glutamine, coined the glutamine/glutamate cycle (GGC) (see reviews by Yudkoff et al., 2000; Rothman et al., 2003; Bak et al., 2006; Albrecht et al., 2010). Of note, a significant portion glutamate is not converted to glutamine, but is taken up by astrocytes undergoes oxidation subsequent to its conversion to TCA cycle intermediates (McKenna et al., 2016). A portion of glutamine not utilized in GGC leaves the brain via the blood-brain barrier (Hawkins and Viña, 2016; Zaragozá, 2020) or blood-CSF barrier (Dolgodilina et al., 2020), by a mechanism involving exchange with large neutral amino acids leucine or tryptophan (Mans et al., 1982; del Pino et al., 1995).
Transport of glutamine within GGC involves transporter members belonging to A and N transport systems that differ in their properties (Bröer, 2007; Leke and Schousboe, 2016). Of note, the issue of the specific, GGC-related roles of glutamine will not be dealt with here, as the effects of hyperammonemia-related excess of glutamine on amino acidergic neurotransmission have so far escaped conclusive elaboration. Here we focus on the implications of the involvement of glutamine in brain ammonia turnover. Because in the brain the urea cycle is incomplete, glutamine synthesis remains the only efficient route of ammonia neutralization and, along with glutamine efflux from the brain to blood, constitutes the critical mechanism for excreting excess ammonia (Cooper and Jeitner, 2016). Since an overwhelming proportion (up to 80%) of astrocytic glutamate is converted to glutamine, glutamate uptake is a critical factor in regulation of glutamine efflux (McKenna et al., 1996, 2016). In line with the above, under controlled ammonia load, glutamine transporters localized on the astrocytic side suffice with facilitated diffusion controlled by the intracellular concentration of glutamine. However, in the setting of ALF, or of acute hyperammonemia evoked by other causes, increased glutamine accumulation resulting from ammonia overload per se induces astrocytic swelling, which leads to the often-mortal cytotoxic brain edema. This issue will form a specific theme of the review.
Both ammonia detoxification and the GGC put high demands on the availability of glutamine at its destination loci and rapid escape from its locus of synthesis (Daikhin and Yudkoff, 2000; Schousboe et al., 2014). As discussed in further sections of this review, the escape routes are critical for preventing excessive, edema-inducing accumulation in astrocytes under conditions of ammonia overload. Intercellular mobility of glutamine is facilitated, by its extraordinary abundance in the extracellular space. While brain tissue glutamine concentrations are more or less equal to other amino acids, its concentrations in the extracellular fluid or the cerebrospinal fluid (∼0.5–1 mM) exceeds by one order of magnitude other amino acids in these compartments (Perry et al., 1975; Jacobson et al., 1985; Xu et al., 1998). Most importantly, glutamine mobility is secured by active, highly controlled transport between different cell types of the CNS, at the brain-blood and blood-CSF barrier, executed by glutamine transporters.
Cell Membrane Glutamine Transporting Systems
Glutamine membrane transporters belong to different protein families that differ in properties, and most of them also accept other neutral or cationic amino acids (Albrecht and Zielińska, 2019). Glutamine transporters share an affinity toward glutamine but show differences in transport modes concerning Na+ or H+ coupling. The cotransporters efficiently accumulate glutamine while antiporters determine the balance between glutamine and other amino acids. The most acknowledged glutamine transporters belong to the Slc1, 6, 7, and 38 families (Pochini et al., 2014). The pleiotropic role of glutamine may be a reason why glutamine transporters, belonging to several protein families, are both redundant and ubiquitous. In general, none of the proteins is unequivocally specific (Pochini et al., 2014). Glutamine transporter classification originally was based on functional properties, such as substrate specificity, ion and pH dependence, kinetics, and regulatory properties, and led to clustering the transporters in systems. In the mammalian brain, glutamine transport systems are classified into two distinct groups termed sodium-dependent (systems A, ASC, and N) and sodium-independent (system L and y + L). System A and system L, the first defined, indicate carriers’ “alanine-preference” and “leucine-preference,” respectively (Kanai and Endou, 2001; Solbu, 2010). The systems include one or even more transporters, still not identified as specific proteins, due to the difficulties in discriminating a single function in entire cells. Neither system A nor system N-mediated transport requires counter-transport of other amino acids and determines the actual net amino acid flux (Meier, 2002). Table 1 presents astrocyte-localized glutamine transporting moieties with their transport features and, so far identified, functional importance.
Glutamine Cell Membrane Transporters in Focus of This Review
We specifically focus in this review on the ammonia-induced changes in the operation of astrocytic glutamine transporters that, with the evidence presented, have a contributing impact on the development of brain edema in the setting of ALF. Among the glutamine transporting moieties present on the membranes of astrocytes (Table 1), two, according to current data (Table 2), have attracted attention in this context. One of these, Slc38a3, is responsible for effective egress of newly synthesized glutamine from astrocytes and, this is well suited to prevent its excessive intra-astrocytic accumulation. The other one, Slc7A6, promotes glutamine exchange for essential cationic amino acids including, arginine (Bröer et al., 2000).
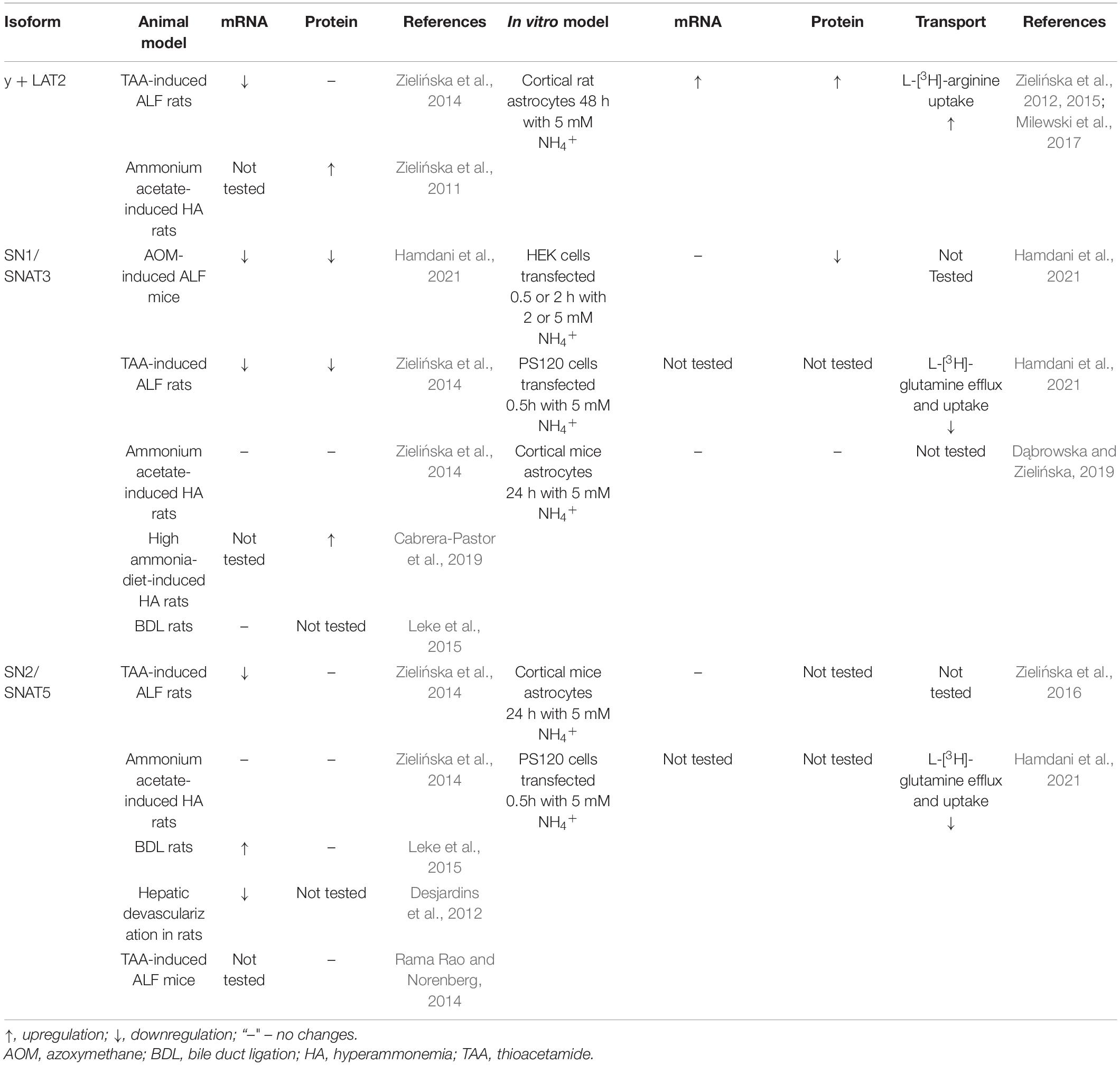
Table 2. ALF or ammonia in vitro- induced alterations in astrocyte-localized glutamine transporters.
Glutamine Transport in Astrocytic Mitochondria
Intracellular transport from cytoplasm to mitochondria is one other factor contributing to intracellular (especially intra-astrocytic) glutamine homeostasis. It was demonstrated before that glutamine enters through the mitochondrial outer membrane (Zalman et al., 1980) and passes through the inner mitochondrial membrane using the mitochondrial glutamine carrier (Matés et al., 2009). The transport has been characterized in peripheral mammalian tissues e.g., kidneys (Indiveri et al., 1998), liver (Kovacević et al., 1970; Kovacević and Bajin, 1982; Haussinger et al., 1985), and tumor cells (Molina et al., 1995). Recently, the SLC1A5 carrier variant transporting glutamine has been characterized in mitochondria of cancer cells (Yoo et al., 2020). Although its in-depth characterization in brain mitochondria is pending, there is firm evidence of a saturable, carrier-mediated transport of glutamine in both synaptic and non-synaptic, astrocytic mitochondria (Minn, 1982; Steib et al., 1986; Roberg, 1995; Dolińska et al., 1996). However, the molecular identity of mitochondrial glutamine transporter in control brain tissue has not yet been definitively revealed. Most interestingly glutamine uptake to mitochondrial fractions enriched in large, astrocytic mitochondria is strongly inhibited by histidine (Albrecht et al., 2000; Ziemińska et al., 2000a), and is coupled to its intra-mitochondrial degradation to glutamate and ammonia by PAG (Albrecht et al., 2000; Ziemińska et al., 2004).
Glutaminosis: A Consistent Neuropathological Component Of Hyperammonemic Encephalopathies
Hyperammonemia defines metabolic disorders characterized by elevated levels of ammonia in the blood. Hyperammonemia occurs in a course of many and often causally unrelated diseases, such as chronic and acute encephalopathy, Reye’s syndrome, congenital deficits in urea cycle enzymes (UCDs), uremic encephalopathy, diabetic encephalopathy, or hypoglycemic encephalopathy.
Typically and most frequently, hyperammonemia is a secondary complication of primary liver disease, a leading factor in hepatic encephalopathy (HE). HE is a multi-symptomatic neurological syndrome related to acute (drug-induced) or chronic (cirrhosis associated) liver failure, where blood-derived ammonia (Ong et al., 2003; Jayakumar and Norenberg, 2018), and blood-derived and endogenous inflammatory intermediates, are the key pathogens (Rodrigo et al., 2010; Coltart et al., 2013; Balzano et al., 2020).
By definition, in hyperammonemic encephalopathies circulating ammonia concentration is significantly increased, reaching high micromolar or even millimolar concentrations in UCDs (Michalak et al., 1996) and ALF patients (Clemmesen et al., 1999). However, biochemical analysis of these patients’ brain tissue, neuroimaging (Kreis et al., 1990; Binesh et al., 2005) and brain microdialysis (Bjerring et al., 2008) showed significantly increased cerebral glutamine concentrations, consistent with the conversion of ammonia into glutamine. While, this did not per se prove the pathogenic role of glutamine, more recent studies do. In patients with acute-upon-chronic liver failure, a condition where stable liver cirrhosis undergoes instant exacerbation, the severity of clinical symptoms correlated well with brain glutamine as quantitated by NMR (Laubenberger et al., 1997). In line with the above, in patients with hepatic coma awaiting liver transplantation, the brain’s extracellular level of glutamine measured by microdialysis correlated as faithfully as arterial blood ammonia with intracranial pressure (Tofteng et al., 2006). Of note, increased glutamine content in the extracellular space content is thought to faithfully reflect the excess of glutamine that leaves astrocytes (Bosman et al., 1992; Rao et al., 1995; Hilgier et al., 1999). Notably, the high activity of GS and efficient incorporation of ammonia into glutamine result in a large ammonia concentration gradient between the brain and blood, which, together with the pH difference between the brain (∼7.1) and blood (∼7.4), promotes the penetration of ammonia into the brain (Ott and Larsen, 2004).
Brain Edema: The Ultimate Phase of Acute Hyperammonemia
The most life-threatening complication associated with acute HE is cerebral edema, in extreme cases resulting in intracranial hypertension severe enough to cause a patient’s death by brain herniation (reviewed in Blei, 2007; Bosoi and Rose, 2013; Scott, 2013). It has been documented that in ALF patients, intracranial pressure positively correlates with arterial ammonia levels (Bernal et al., 2007). The cerebral edema development in ALF progresses quickly and remains the leading cause of high patient mortality (∼50–80% of deaths). To this date, no specific treatment of acute HE-associated brain edema and coma other than whole-body cooling is available (Vaquero and Blei, 2004).
Histopathologic evaluation of ALF patient’s brain tissue revealed characteristic features of brain edema encompassing alterations in the morphology of astrocyte and endothelial cells (Martinez, 1968; Norenberg, 1977; Kato et al., 1992). Increased number of vacuoles and thickened basement membrane were visible in the endothelial cells whereas, swollen astrocytes with swollen mitochondria in the cytoplasm, and enlarged basal membrane, were observed in regard to the astrocyte cell population (Martinez, 1968; Norenberg, 1977; Kato et al., 1992). Of note in this context, characteristic pathomorphologic features of astrocytes, with swollen nuclei, glycogen deposition, and margination of chromatin, known as Alzheimer type 2 astrocytosis, are observed irrespective of the duration of hyperammonemia and its origin (inherited vs. acquired) (Norenberg, 1987). Moreover, imaging studies based on T2-weighted MRI scans in patients with ALF displayed decreased value of an apparent diffusion coefficient, indicating an increase in intracellular water in the brain and the cytotoxic nature of brain edema in ALF (Chavarria et al., 2010). Morphological (Traber et al., 1987, 1989; Kato et al., 1989; Chadipiralla et al., 2012) and MRI-derived evidence obtained in animal models of ALF (Obara-Michlewska et al., 2018; Hamdani et al., 2021) corroborated with observations on patients, further supporting the cytotoxic mechanism of ALF-induced brain edema.
Acute Hyperammonemic Brain Edema Is Primarily Cytotoxic in Nature
By analogy to other edema-associated brain pathologies, the relative roles of cytotoxic and vasogenic factors in the development of brain edema associated with acute HE has long remained a matter of debate (Blei, 2007). In agreement with the long-held view that HE is primarily an “astrogliopathy” (Norenberg, 1987; Albrecht et al., 2010), current evidence supports its primarily cytotoxic nature, primarily reflecting swelling of astrocytes by mechanisms related to intracellular metabolic and ion imbalance and the ensuing intracellular accumulation of water (Haussinger and Schliess, 2008; Bémeur et al., 2016). The current view of edema pathophysiology, is that ammonia induces astrocytic swelling by a complex interplay of (i) impairment of in- and out-transport of different osmolytes leading to intracellular osmotic imbalance, (ii) mitochondrial dysfunction related to excessive accumulation of ammonia-derived glutamine and successive intra-mitochondrial release of toxic levels of ammonia, (iii) oxidative/nitrosative stress. Evidence presented below strongly suggests that glutamine is involved in a vicious cycle of (i), (ii), and (iii).
Glutaminosis: A Causative Factor In Cytotoxic Brain Edema And Its Mechanistic Basis
As mentioned in the section: “Glutaminosis: a consistent neuropathological component of hyperammonemic encephalopathies,” clinical studies revealed a good correlation between brain glutamine content and the severity of edema-related manifestations of ALF. While correlation strongly suggested a mechanistic link, it could not be interpreted as causal nexus. A proof of concept came from experimental ALF or acute-upon-chronic liver failure models, inhibition of GS activity by a GS inhibitor, methionine sulfoximine, alleviated astrocytic swelling, brain edema, and a set of other pathophysiological symptoms of acute HE (Takahashi et al., 1991; Willard-Mack et al., 1996; Master et al., 1999; Tanigami et al., 2005). Contrary to efficient ammonia-lowering treatments, which are effective in reducing brain edema in animal models of ALF, a decrease in high cerebral glutamine levels were not observed (Zwingmann et al., 2004; Ytrebø et al., 2009).
Slc38A3: The Transporter Involved in Glutamine Trapping Within Astrocytes
Studies in vitro on primary astrocyte cultures indicated that the contribution of system N in glutamine transport varies from 10 to 50% of the total glutamine transport capacity (Nagaraja and Brookes, 1996; Deitmer et al., 2003; Heckel et al., 2003; Dolińska et al., 2004; Sidoryk-Węgrzynowicz et al., 2009). System N silencing in neonatal astrocytes inhibited glutamine efflux out of the cells and documented its prevailing functioning in the direction of glutamine release (Zielińska et al., 2016). Glutamine efflux is preferably mediated by the Slc38a3 system N glutamine transporter, much more so than by one other system N transporter Slc38a2, as the latter shows relatively high affinity for serine, alanine, and glycine (Nakanishi et al., 2001a,b; Hamdani et al., 2012). Immunohistochemical data revealed localization of the system N transporters Slc38a3 (Boulland et al., 2002) and Slc38a5 (Cubelos et al., 2005) on astrocytic processes located adjacent to glutamatergic and GABAergic synapses, confirming the presence of the protein in native tissue. More recently, Slc38a5 was shown to be located intracellularly (Hamdani et al., 2012), and functional expression of Slcs8a3 was demonstrated in the astrocytic plasma membrane in situ (Todd et al., 2017). Hence, over thirty years after its initial discovery, it has become clear that Slc38a3 plays a critical role in regulating the astrocytic leg of GGC.
Evidence implicates Slc38a3 transporter to act as a hub for glutamine transport, recruiting a range of accessory complexes that enhance and regulate its function in response to different protein (Boulland et al., 2002; Rubio-Aliaga and Wagner, 2016). Interestingly enough, the physical coupling of Slc1a3-Slc38a3 proteins demonstrated in cultured Bergmann glia (Martínez-Lozada et al., 2013) and kinetics data of glutamine fluxes driven mainly by the increased astrocytic [Na+] (Todd et al., 2017) support Slc38a3-mediated glutamine transport in glutamate recycling. Since Slc38a3 preferentially controls the efflux of newly synthesized glutamine from astrocytes (Chaudhry et al., 1999), it prevents excessive accumulation in there and, subsequently, retention of osmotically obligated water. Accordingly, its decreased activity in ALF leads to enhanced intracellular glutamine trapping, in this way contributing to cytotoxic brain edema (Hamdani et al., 2021).
Kanamori and Ross (2005) were the first to hint, albeit indirectly, at the possibility that system N-mediated glutamine transport may be controlled by hyperammonemia. In their study, continuous infusion of [15N]ammonium acetate resulted increased glutamine accumulation in brain microdialysate. Since extracellular glutamine sufficiently saturated system A-mediated glutamine transport, the authors interpreted their finding as reflecting inhibition of glutamine efflux from astrocytes by reversal of system N transport. Our recent studies extended this original finding and directly implicated the involvement Slc38a3 in the sequence of events leading to astrocytic swelling and brain edema. Decreased Slc38a3 expression was recorded in the cerebral cortex of rats with thioacetamide-induced ALF, but not in those with ammonium-acetate-induced simple hyperammonemia (Zielińska et al., 2014). Most interestingly, thioacetamide-induced ALF, but not hyperammonemia was earlier found associated with increased cerebro-cortical volume (Hilgier et al., 1996). In azoxymethan-induced mice model of ALF, decreased expression of Slc38a3 in the brain coincided with (i) excessive brain glutamine (ii) increased astrocytic cell volume and (iii) decreased apparent diffusion coefficient marking cytotoxic brain edema (Hamdani et al., 2021). As a proof of concept, local Slc38a3 silencing using vivo morpholino technique resulted in astrocytic swelling and increased volume of the brain region subjected to silencing (Hamdani et al., 2021).
The mechanism underlying reduction of Slc38a3 expression in the ammonia- (or otherwise glutamine)- overexposed brain may be subject to regulation by a wide spectrum of transcriptional, translational, or epigenetic factor which remain to be analyzed in detail. Ammonia treatment in vitro strongly inhibited the release of newly loaded radiolabeled glutamine from cultured astrocytes (Dąbrowska et al., 2018) and the inhibition involved changes in protein kinase C signaling (Dąbrowska et al., 2018), Sp1-Nrf2 transcription factor complex formation (Dąbrowska and Zielińska, 2019), and activation of Nrf2 transcription factor per se (Dąbrowska et al., 2021). Of note in this context, the study by Lister et al. (2018) identified Nrf2 transcription factor control in the regulation of Slc38a3mRNA expression (Lister et al., 2018). In addition, in vitro experiments on Slc38a3 transfected oocytes revealed that depression Slc38a3-mediated glutamine transport engages direct interaction of ammonium ions with the Slc38a3 protein moiety (Hamdani et al., 2021). Clearly, the mechanisms underlying the responses of Slc38a3 to ammonia at the transcriptional/translational/posttranslational levels deserve further investigation.
Slc38a3 carrier is believed to bridge inactivation of glutamate in astrocytes to neurotransmitter glutamate synthesis in the nerve endings (Todd et al., 2017; Verkhratsky et al., 2021). Therefore, its excessive involvement in glutamine trapping may contribute to the imbalance between excitatory and inhibitory neurotransmission as well. However, proper glutamatergic transmission seems to be less dependent and may occur after inhibition or reduction of GS (Liang et al., 2006; Kam and Nicoll, 2007) or after local knock out-induced reduction of astrocytic Slc38a3 transporter, mediating glutamine efflux (Popek et al., 2020). It remains to be seen whether structural and electrophysiological impairment of glutamatergic synapse noted in ALF mice is in anyway related to Slc38a3 depletion in the same model (Popek et al., 2018).
Glutamine: An Osmotic Stress- Inducing Factor
Cerebral glutamine concentration above 2 mM saturates active glutamine transport out of the brain (O’Kane and Hawkins, 2003) and, thereby, favors its accumulation. Accumulation of synthesized in glial cells glutamine, might cause the osmotic pressure increase and generate glutamine concentration gradient leading to the development of intracranial pressure (ICP) (Master et al., 1999; Tofteng et al., 2002, 2006). In the brain, the difference between 1 mmol/L in blood and 4 mmol/L would cause a pressure gradient of 35 mmHg that is well above the critical level to cause brain edema. Thus, simultaneously triggered brain compensatory mechanisms are critical and encompass a removal from the brain other osmotically active compounds, e.g., myo-inositol, choline, or taurine, which at least partially reduce brain osmotic effects (Sterns and Silver, 2006; Heins and Zwingmann, 2010; Jiménez et al., 2017). Importantly, biochemical changes of different osmotically active substances in the brain are frequently observed even before the onset of clinical symptoms (Larsen, 2002). Studies performed by proton magnetic resonance spectroscopy (1H MRS) in symptomatic patients with severe ornithine transcarbamylase deficiency showed that a significantly elevated level of glutamine was accompanied by a simultaneous decrease in myo-inositol and choline, which is considered compensatory in nature (Gropman et al., 2009; Sen et al., 2021). However, at a very high concentration of ammonia, osmoregulation may be insufficient to compensate for the rapid cerebral glutamine increase. The above is supported by the results delivered from animal models: as outlined in the previous section, brain edema caused by acute ammonia poisoning was prevented by the inhibition of glutamine synthesis. However, arguments may be raised against the contribution of excess glutamine to increased intracellular osmotic pressure. Mild hypothermia prevented brain edema development although, glutamine brain content remained relatively stable under these conditions (Vaquero and Butterworth, 2007; Stravitz and Larsen, 2009). Moreover, in the healthy brain glutamine accounts for less than 2% of the total pool of organic osmolytes and electrolytes (Pasantes-Morales et al., 2002). Therefore, the ∼3–4-fold increase of brain glutamine observed in ALF patients (Bjerring et al., 2010), and experimental animals (Takahashi et al., 1991; Master et al., 1999), is unlikely to be enough to significantly account for brain edema, even if not compensated by the efflux of other osmolytes. Of note, water flow through the cell membrane is at least in part regulated by the activity of aquaporin water channels which additionally may modify the cell’s osmotic status (Papadopoulos and Verkman, 2007; Thrane et al., 2011). All in all, although perhaps significant locally, on a global scale, the osmotic effect of excess glutamine alone is unlikely to be the predominating edema-eliciting factor.
Glutamine: Inducer of Mitochondrial Dysfunction and Swelling in the “Trojan Horse” Hypothesis
According to the “Trojan Horse” hypothesis (Albrecht and Norenberg, 2006), glutamine accumulating in excess in the cytoplasm enters mitochondria and opens the megachannel in the inner mitochondrial membrane, mitochondrial permeability transition pore (mPTP), which is sensitive to cyclosporine A (Ziemińska et al., 2000b). Available evidence suggests that glutamine enters mitochondria by active transport rather than by diffusion. It was shown that glutamine entry to astrocytic mitochondria is stimulated by pathopysiologically relevant concentrations of glutamine (Dolińska et al., 1996). Histidine, the strongest inhibitor of mitochondrial glutamine among all the amino acids (see section “Glutamine Transport in Astrocytic Mitochondria”), attenuated opening of mPTP and mitochondrial swelling in isolated astrocytic mitochondria (Ziemińska et al., 2000a), in cultured astrocytes (Pichili et al., 2007) and in the brain of ALF-affected rats (Rama Rao et al., 2010).
In mammalian cells, mPTP elicits a rapid increase in the permeability of e.g., protons, ions, and other molecules of MW < 1,500 Da through the inner mitochondrial membrane. The mega-channels opening leads to a collapse of the mitochondrial inner membrane potential, created by protons through the mitochondrial electron transport chains (Guo et al., 2018; Fernandez-Vizarra and Zeviani, 2021). This causes osmotic swelling of the mitochondrial matrix and movement of metabolites through the inner membrane that leads to defective oxidative phosphorylation decrease of ATP synthesis (Vercellino and Sazanov, 2022). Consequently, the mitochondrial membrane potential loss leads to reactive oxygen species production that further stimulates the mPTP process.
In astrocytes, mitochondrial dysfunction elicited by glutamine leads to the formation of reactive oxygen species and astrocytic cell swelling (Norenberg et al., 2004; Albrecht and Norenberg, 2006; Rama Rao and Norenberg, 2012). Upon entry to mitochondria, glutamine is metabolized by the mitochondrial enzyme PAG to ammonia and glutamate and, ammonia is the trigger of subsequent deleterious events. This sequence of events has been documented in cultured astrocytes, where the glutamine-induced intra-astrocytic accumulation of reactive oxygen species (Jayakumar et al., 2004) and astrocytic swelling (Jayakumar et al., 2006) were attenuated, by the PAG synthetic inhibitor, DON (Crosby, 2015).
Evidence that served to formulate the “Trojan Horse” hypothesis has been considered somewhat ambiguous. The criticism raised was that the hypothesis was overwhelmingly based on results of ex vivo experiments (isolated mitochondria), or in vitro (cultured astrocytes treated with 5 mM ammonia for 24 h) (see the above paras). As a step forward, in one in vivo the mPTP induction was detected in brain homogenates of rats with thioacetamide-induced ALF, and reduced after the administration of glutamine transport competitor, histidine. Histidine also attenuated oxidative stress, and brain edema (Rama Rao et al., 2010). Toward the same end, intracellular lactate accumulation in the brain and elevated ICP occurred notwithstanding unchanged mitochondrial function in hyperammonemia brain tissue (Witt et al., 2017). In support of the “Trojan Horse” glutamine mode of action, elevated concentrations of ATP degradation products hypoxanthine and inosine, markers of mitochondrial dysfunction, in brain dialysates from ALF patients, have been found to correlate with extracellular glutamine (Bjerring et al., 2010). Less directly supportive of, but consistent with the hypothesis, reduced metabolic rates of oxygen were very previously noted in the brain of rats with thioacetamide-induced ALF (Pluta and Albrecht, 1986), and more recently in the brain of patients with overt hepatic encephalopathy (Iversen et al., 2009).
One other criticism of the Trojan Horse hypothesis has been raised based on the purported absence of PAG in native astrocytes and its exclusive location in neurons (Laake et al., 1999). In line with the discussed view, ameliorating effects of astrocyte swelling in vitro, induced by the PAG inhibitor, were interpreted as solely reflecting phenotypic alteration of astrocytes upon culturing. However, the skepticism was balanced by solid evidence that both PAG isoforms abound in the rat and human brain (Cardona et al., 2015; Milewski et al., 2019).
Slc7A6: Contributor to Ammonia-Induced Oxidative/Nitrosative Stress in Astrocytic Swelling
Compelling evidence documents that ammonia generates free radicals (Murthy et al., 2001; Görg et al., 2008) and ammonia-induced astrocytic swelling that per se appears to be a trigger of oxidative/nitrosative stress and subsequent protein tyrosine nitration, is a critical event en route to astrocytic and neuronal dysfunction (Schliess et al., 2002; Kruczek et al., 2011). Induction of peroxynitrite activates volume-regulated anion channels in astrocytes and induces astrocytic swelling which was coupled to enhanced release of the glutamate surrogate [3H]D-aspartate (Haskew et al., 2002). Free radicals formation was observed in a rat model of hyperammonemia (Kosenko et al., 1997, 1998), and was associated with decreased activity of antioxidant enzymes (glutathione peroxidase, superoxide dismutase and catalase) (Kosenko et al., 1997). Ammonia-induced cerebrocortical tissue swelling was ameliorated by the NMDA/nitric oxide (NO) pathway inhibitors, indicating involvement of oxidative-nitrosative stress (Zielińska et al., 2003).
The system y + L member, Slc7a6 (4F2hc/y + LAT2) carrier is a heterodimer, composed of a glycosylated heavy chain (SLC3a2/4F2hc/CD98 and a catalytic light chain subunit (SLC7) (Torrents et al., 1998). The heavy chain is necessary for trafficking of the light chain to the plasma membrane, whereas the light chain determines the transport characteristics of the respective heterodimer. Slc7a6 has a wide tissue distribution including brain, heart, kidney, and promotes glutamine exchange for essential cationic amino acids including arginine (Pfeiffer et al., 1998; Torrents et al., 1998; Bröer et al., 2000).
The role of Slc7a6-mediated glutamine-arginine exchange in the modulation of NO synthesis under hyperammonemic conditions in vivo has been demonstrated in this laboratory (Hilgier et al., 2008, 2009). Specifically, in ammonia perfused rat brain, pharmacological inhibition of extracellular glutamine degradation or glutamine transport to neurons reduced NO synthesis and its accumulation in the extracellular space (Hilgier et al., 2009). Attenuation of NO synthesis was reproduced by intracerebral administration of glutamine, or two other y + LAT2 substrates, leucine and cyclo-leucine (Hilgier et al., 2009), while inhibition of glutamine synthesis reversed this attenuating effect (Hilgier et al., 2009). Collectively the results underscored the contribution of y + LAT2 transporter to glutamine/arginine imbalance associated with hyperammonemia. The link of y + LAT2 activity to ammonia-induced oxidative-nitrosative stress came to light in studies on cultured astrocytes and animal models (Zielińska et al., 2011, 2012, 2014). Those studies documented reduced arginine delivery due to enhanced y + LAT2-mediated exchange of extracellular glutamine for intracellular arginine, contributing to decreased NO and cGMP formation in the rat brain by hyperammonemia.
More recent studies revealed that induction of iNOS in astrocytes and stimulation of NO synthesis by ammonia is coupled to increased arginine uptake, a process promoted by a selective increase of the expression and activity of y + LAT2, whereas the unidirectional basic amino acid carrier y + was not affected by ammonia (Zielińska et al., 2012, 2015). In cultured astrocytes, ammonia-evoked oxidative-nitrosative stress also involves induction of iNOS (Schliess et al., 2002), mediated by activation of the nuclear factor κB (Sinke et al., 2008). Of note, cytotoxic brain edema in ALF is also related to the central or peripheral inflammation (Wilkinson et al., 1974; Wyke et al., 1982; Odeh et al., 2004; Shawcross et al., 2007; Butterworth, 2011; Scott, 2013; Jayakumar et al., 2015), whose deleterious effects are mediated by oxidative-nitrosative stress. However, specific contribution of glutamine transport to this phenomenon has to our knowledge not been investigated as yet.
Concluding Remarks
In conclusion, data presented in this review support the view that altered functioning of glutamine transporting moieties residing in astrocytes, contribute in a vicious circle mode, to the glutamine-driven aspect of astrocytic swelling and cytotoxic brain edema associated with ALF (Figure 1). Decreased Slc38a3 activity reduces efflux of newly synthesized glutamine, augmenting its intra-astrocytic residence. The resulting glutamine surplus aggravates glutamine-induced osmotic stress in the cytoplasm, at the same time rendering more glutamine available for (i) its Slc7a6-mediated exchange for extracellular arginine, which increases intra-astrocytic accumulation of reactive nitrogen species (ii) glutamine transport to astrocytic mitochondria, which leads to mitochondrial dysfunction (the “Trojan Horse” mode of action). The effects of (i) and (ii) converge at the level of oxidative/nitrosative stress, a critical driver of astrocytic swelling. Clearly, evidence for the above reasoning, especially regarding glutamine transport to mitochondria needs to be fine-tuned to achieve full symmetry between the documentation in vivo and in vitro.
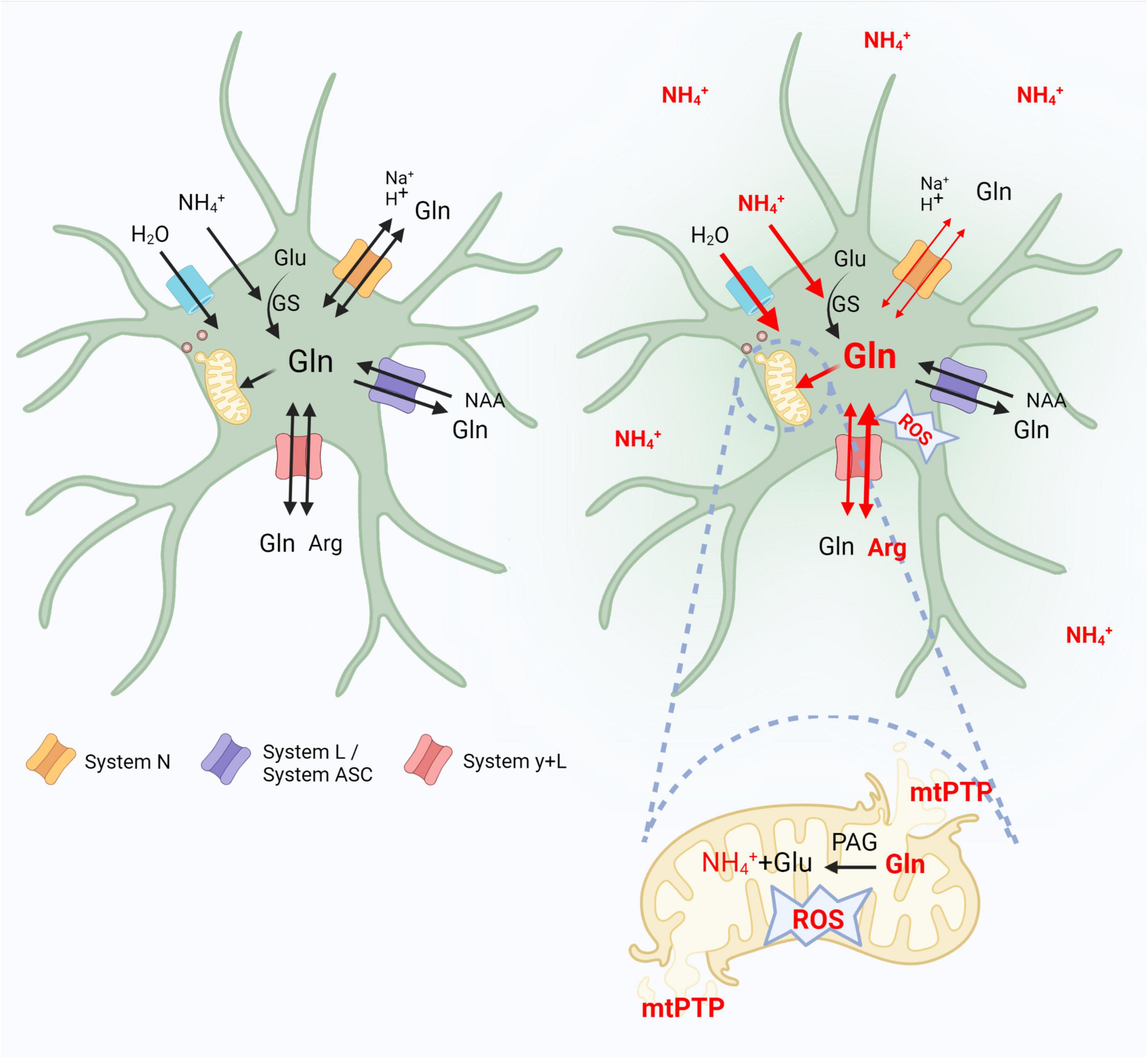
Figure 1. Contribution of astrocytic glutamine transporters belonging to systems N, L/ASC and y + L to the glutaminosis-driven aspect of brain edema in acute hyperammonemia. Left panel indicates control conditions. In the right, where the effects of hyperammonemia are outlined, thin or thick red lines indicate decreased or increased activity of a given event, respectively. Glu, glutamate; Gln, glutamine; Arg, arginine; GS, glutamine synthetase; NAA, neutral amino acids; ROS, reactive oxygen species; mtPTP, mitochondria permeability transition pore. Created with BioRender.com.
In astrocytes, hyperammonemia (ammonium ions, NH4+) alters the functioning of glutamine transporters that contribute in a vicious circle mode to the glutamine-driven aspect of astrocytic swelling and cytotoxic brain edema. Decreased system N (Slc38a3) activity reduces efflux of synthesized by glutamine synthetase (GS) glutamine, augmenting its intra-astrocytic residence. The resulting glutamine surplus aggravates glutamine-induced osmotic stress in the cytoplasm, rendering more glutamine available for: (i) its system y + L (Slc7a6)-mediated exchange for extracellular arginine (Arg), which increases the intra-astrocytic accumulation of reactive oxygen-nitrogen species (ROS), (ii) glutamine enter to astrocytic mitochondria, where, hydrolyzed to glutamate and NH4+ by PAG leads to open mitochondrial permeability transition pore (mPTP), and mitochondrial dysfunction (the “Trojan Horse” mode of action).
Author Contributions
MZ, JA, and MP wrote the manuscript. MZ and MP designed the figure, tables, and contributed to the revision of the manuscript. All authors contributed to the article and approved the submitted version.
Funding
This work was supported by funds from the National Centre for Research (NCBiR) Polish-Norwegian Research Program (Grant no. Pol-Nor/196190/23/2013) and the National Science Centre of the Republic of Poland (NCN), Grant no. 2015/19/B/NZ4/01902.
Conflict of Interest
The authors declare that the research was conducted in the absence of any commercial or financial relationships that could be construed as a potential conflict of interest.
Publisher’s Note
All claims expressed in this article are solely those of the authors and do not necessarily represent those of their affiliated organizations, or those of the publisher, the editors and the reviewers. Any product that may be evaluated in this article, or claim that may be made by its manufacturer, is not guaranteed or endorsed by the publisher.
References
Albrecht, J., and Norenberg, M. D. (2006). Glutamine: a Trojan horse in ammonia neurotoxicity. Hepatology 44, 788–794. doi: 10.1002/hep.21357
Albrecht, J., and Zielińska, M. (2019). Exchange-mode glutamine transport across CNS cell membranes. Neuropharmacology 161:107560. doi: 10.1016/j.neuropharm.2019.03.003
Albrecht, J., Dolińska, M., Hilgier, W., Lipkowski, A. W., and Nowacki, J. (2000). Modulation of glutamine uptake and phosphate-activated glutaminase activity in rat brain mitochondria by amino acids and their synthetic analogues. Neurochem. Int. 36, 341–347. doi: 10.1016/S0197-0186(99)00142-4
Albrecht, J., Sidoryk-Węgrzynowicz, M., Zielińska, M., and Aschner, M. (2010). Roles of glutamine in neurotransmission. Neuron Glia Biol. 6, 263–276. doi: 10.1017/S1740925X11000093
Amaral, A. I., Meisingset, T. W., Kotter, M. R., and Sonnewald, U. (2013). Metabolic aspects of neuron-oligodendrocyte-astrocyte interactions. Front. Endocrinol. 4:54. doi: 10.3389/fendo.2013.00054
Bak, L. K., Schousboe, A., and Waagepetersen, H. S. (2006). The glutamate/GABA-glutamine cycle: aspects of transport, neurotransmitter homeostasis and ammonia transfer. J. Neurochem. 98, 641–653. doi: 10.1111/j.1471-4159.2006.03913.x
Balzano, T., Dadsetan, S., Forteza, J., Cabrera-Pastor, A., Taoro-Gonzalez, L., Malaguarnera, M., et al. (2020). Chronic hyperammonemia induces peripheral inflammation that leads to cognitive impairment in rats: reversed by anti-TNF-α treatment. J. Hepatol. 73, 582–592. doi: 10.1016/j.jhep.2019.01.008
Barollo, S., Bertazza, L., Watutantrige-Fernando, S., Censi, S., Cavedon, E., Galuppini, F., et al. (2016). Overexpression of L-Type Amino acid transporter 1 (LAT1) and 2 (LAT2): novel markers of neuroendocrine tumors. PLoS One 11:e0156044. doi: 10.1371/journal.pone.0156044
Bémeur, C., Cudalbu, C., Dam, G., Thrane, A. S., Cooper, A. J. L., and Rose, C. F. (2016). Brain edema: a valid endpoint for measuring hepatic encephalopathy? Metab. Brain Dis. 31, 1249–1258. doi: 10.1007/s11011-016-9843-9
Bergström, J., Fürst, P., Norée, L. O., and Vinnars, E. (1974). Intracellular free amino acid concentration in human muscle tissue. J. Appl. Physiol. 36, 693–697. doi: 10.1152/jappl.1974.36.6.693
Bernal, W., Hall, C., Karvellas, C. J., Auzinger, G., Sizer, E., and Wendon, J. (2007). Arterial ammonia and clinical risk factors for encephalopathy and intracranial hypertension in acute liver failure. Hepatology 46, 1844–1852. doi: 10.1002/hep.21838
Bhutia, Y. D., Babu, E., Ramachandran, S., and Ganapathy, V. (2015). Amino acid transporters in cancer and their relevance to “glutamine addiction”: novel targets for the design of a new class of anticancer drugs. Cancer Res. 75, 1782–1788. doi: 10.1158/0008-5472.CAN-14-3745
Binesh, N., Huda, A., Bugbee, M., Gupta, R., Rasgon, N., Kumar, A., et al. (2005). Adding another spectral dimension to1H magnetic resonance spectroscopy of hepatic encephalopathy. J. Magn. Reson. Imaging 21, 398–405. doi: 10.1002/jmri.20291
Bjerring, P. N., Hauerberg, J., Frederiksen, H.-J., Jorgensen, L., Hansen, B. A., Tofteng, F., et al. (2008). Cerebral glutamine concentration and lactate–pyruvate ratio in patients with acute liver failure. Neurocrit. Care 9, 3–7. doi: 10.1007/s12028-008-9060-4
Bjerring, P. N., Hauerberg, J., Jørgensen, L., Frederiksen, H.-J., Tofteng, F., Hansen, B. A., et al. (2010). Brain hypoxanthine concentration correlates to lactate/pyruvate ratio but not intracranial pressure in patients with acute liver failure. J. Hepatol. 53, 1054–1058. doi: 10.1016/j.jhep.2010.05.032
Blei, A. T. (2007). Brain edema in acute liver failure: can it be prevented? Can it be treated? J. Hepatol. 46, 564–569. doi: 10.1016/j.jhep.2007.01.011
Bosman, D. K., Deutz, N. E. P., Maas, M. A. W., Eijk, H. M. H., Smit, J. J. H., Haan, J. G., et al. (1992). Amino acid release from cerebral cortex in experimental acute liver failure. studied by in vivo cerebral cortex microdialysis. J. Neurochem. 59, 591–599. doi: 10.1111/j.1471-4159.1992.tb09410.x
Bosoi, C. R., and Rose, C. F. (2013). Brain edema in acute liver failure and chronic liver disease: similarities and differences. Neurochem. Int. 62, 446–457. doi: 10.1016/j.neuint.2013.01.015
Boulland, J.-L., Osen, K. K., Levy, L. M., Danbolt, N. C., Edwards, R. H., Storm-Mathisen, J., et al. (2002). Cell-specific expression of the glutamine transporter SN1 suggests differences in dependence on the glutamine cycle: glutamine transporter SN1 and transmitter recycling. Eur. J. Neurosci. 15, 1615–1631. doi: 10.1046/j.1460-9568.2002.01995.x
Braun, D., Kinne, A., Bräuer, A. U., Sapin, R., Klein, M. O., Köhrle, J., et al. (2011). Developmental and cell type-specific expression of thyroid hormone transporters in the mouse brain and in primary brain cells. Glia 59, 463–471. doi: 10.1002/glia.21116
Bröer, A., Brookes, N., Ganapathy, V., Dimmer, K. S., Wagner, C. A., Lang, F., et al. (1999). The astroglial ASCT2 amino acid transporter as a mediator of glutamine efflux. J. Neurochem. 73, 2184–2194.
Bröer, A., Rahimi, F., and Bröer, S. (2016). Deletion of amino acid transporter ASCT2 (SLC1A5) reveals an essential role for transporters SNAT1 (SLC38A1) and SNAT2 (SLC38A2) to sustain glutaminolysis in cancer cells. J. Biol. Chem. 291, 13194–13205. doi: 10.1074/jbc.M115.700534
Bröer, A., Wagner, C. A., Lang, F., and Bröer, S. (2000). The heterodimeric amino acid transporter 4F2hc/y+LAT2 mediates arginine efflux in exchange with glutamine. Biochem. J. 349(Pt 3), 787–795. doi: 10.1042/bj3490787
Bröer, S. (2007). “SLC38 family of transporters for neutral amino acids,”,” in Handbook of Neurochemistry and Molecular Neurobiology, eds A. Lajtha and M. E. A. Reith (Boston, MA: Springer US), 327–338. doi: 10.1007/978-0-387-30380-2_16
Butterworth, R. F. (2011). Hepatic encephalopathy: a central neuroinflammatory disorder? Hepatology 53, 1372–1376. doi: 10.1002/hep.24228
Cabrera-Pastor, A., Arenas, Y. M., Taoro-Gonzalez, L., Montoliu, C., and Felipo, V. (2019). Chronic hyperammonemia alters extracellular glutamate, glutamine and GABA and membrane expression of their transporters in rat cerebellum. Modulation by extracellular cGMP. Neuropharmacology 161:107496. doi: 10.1016/j.neuropharm.2019.01.011
Cardona, C., Sánchez-Mejías, E., Dávila, J. C., Martín-Rufián, M., Campos-Sandoval, J. A., Vitorica, J., et al. (2015). Expression of Gls and Gls2 glutaminase isoforms in astrocytes: functional glutaminase expression in astroglia. Glia 63, 365–382. doi: 10.1002/glia.22758
Castell, L., Vance, C., Abbott, R., Marquez, J., and Eggleton, P. (2004). Granule localization of glutaminase in human neutrophils and the consequence of glutamine utilization for neutrophil activity. J. Biol. Chem. 279, 13305–13310. doi: 10.1074/jbc.M309520200
Chadipiralla, K., Reddanna, P., Chinta, R. M., and Reddy, P. V. B. (2012). Thioacetamide-Induced fulminant hepatic failure induces cerebral mitochondrial dysfunction by altering the electron transport chain complexes. Neurochem. Res. 37, 59–68. doi: 10.1007/s11064-011-0583-2
Chaudhry, F. A. (2001). Coupled and uncoupled proton movement by amino acid transport system N. EMBO J. 20, 7041–7051. doi: 10.1093/emboj/20.24.7041
Chaudhry, F. A., Reimer, R. J., Krizaj, D., Barber, D., Storm-Mathisen, J., Copenhagen, D. R., et al. (1999). Molecular analysis of system N suggests novel physiological roles in nitrogen metabolism and synaptic transmission. Cell 99, 769–780. doi: 10.1016/s0092-8674(00)81674-8
Chavarria, L., Oria, M., Romero-Gimenez, J., Alonso, J., Lope-Piedrafita, S., and Cordoba, J. (2010). Diffusion tensor imaging supports the cytotoxic origin of brain edema in a rat model of acute liver failure. Gastroenterology 138, 1566–1573. doi: 10.1053/j.gastro.2009.10.003
Clemmesen, J. O., Larsen, F. S., Kondrup, J., Hansen, B. A., and Ott, P. (1999). Cerebral herniation in patients with acute E liver failure is correlated with arterial ammonia concentration. Hepatology 29, 648–653. doi: 10.1002/hep.510290309
Coltart, I., Tranah, T. H., and Shawcross, D. L. (2013). Inflammation and hepatic encephalopathy. Arch. Biochem. Biophys. 536, 189–196. doi: 10.1016/j.abb.2013.03.016
Cooper, A., and Jeitner, T. (2016). Central role of glutamate metabolism in the maintenance of nitrogen homeostasis in normal and hyperammonemic brain. Biomolecules 6:16. doi: 10.3390/biom6020016
Coothankandaswamy, V., Cao, S., Xu, Y., Prasad, P. D., Singh, P. K., Reynolds, C. P., et al. (2016). Amino acid transporter SLC6A14 is a novel and effective drug target for pancreatic cancer: SLC6A14 is a drug target for pancreatic cancer. Br. J. Pharmacol. 173, 3292–3306. doi: 10.1111/bph.13616
Cory, J. G., and Cory, A. H. (2006). Critical roles of glutamine as nitrogen donors in purine and pyrimidine nucleotide synthesis: asparaginase treatment in childhood acute lymphoblastic leukemia. Vivo 20, 587–589.
Crosby, H. (2015). Evaluating the toxicity of the analgesic glutaminase inhibitor 6-Diazo-5-Oxo-L-norleucine in vitro and on rat dermal skin fibroblasts. MOJT 1:5. doi: 10.15406/mojt.2015.01.00005
Cubelos, B., González-González, I. M., Giménez, C., and Zafra, F. (2005). Amino acid transporter SNAT5 localizes to glial cells in the rat brain. Glia 49, 230–244. doi: 10.1002/glia.20106
Czeredys, M., Mysiorek, C., Kulikova, N., Samluk, L., Berezowski, V., Cecchelli, R., et al. (2008). A polarized localization of amino acid/carnitine transporter B0,+ (ATB0,+) in the blood–brain barrier. Biochem. Biophys. Res. Commun. 376, 267–270. doi: 10.1016/j.bbrc.2008.08.122
Dąbrowska, K., Albrecht, J., and Zielińska, M. (2018). Protein kinase C-mediated impairment of glutamine outward transport and SN1 transporter distribution by ammonia in mouse cortical astrocytes. Neurochem. Int. 118, 225–232. doi: 10.1016/j.neuint.2018.07.001
Dąbrowska, K., and Zielińska, M. (2019). Silencing of transcription factor Sp1 promotes SN1 transporter regulation by ammonia in mouse cortical astrocytes. IJMS 20:234. doi: 10.3390/ijms20020234
Dąbrowska, K., Skowrońska, K., Popek, M., Albrecht, J., and Zielińska, M. (2021). The role of Nrf2 transcription factor and Sp1-Nrf2 protein complex in glutamine transporter sn1 regulation in mouse cortical astrocytes exposed to ammonia. IJMS 22:11233. doi: 10.3390/ijms222011233
Daikhin, Y., and Yudkoff, M. (2000). Compartmentation of brain glutamate metabolism in neurons and glia. J. Nutr. 130, 1026S–1031S. doi: 10.1093/jn/130.4.1026S
Deitmer, J. W., Bröer, A., and Bröer, S. (2003). Glutamine efflux from astrocytes is mediated by multiple pathways. J. Neurochem. 87, 127–135. doi: 10.1046/j.1471-4159.2003.01981.x
del Pino, M. M. S., Peterson, D. R., and Hawkins, R. A. (1995). Neutral amino acid transport characterization of isolated luminal and abluminal membranes of the blood-brain barrier. J. Biol. Chem. 270, 14913–14918. doi: 10.1074/jbc.270.25.14913
Desjardins, P., Du, T., Jiang, W., Peng, L., and Butterworth, R. F. (2012). Pathogenesis of hepatic encephalopathy and brain edema in acute liver failure: role of glutamine redefined. Neurochem. Int. 60, 690–696. doi: 10.1016/j.neuint.2012.02.001
Dolgodilina, E., Camargo, S. M., Roth, E., Herzog, B., Nunes, V., Palacín, M., et al. (2020). Choroid plexus LAT2 and SNAT3 as partners in CSF amino acid homeostasis maintenance. Fluids Barriers CNS 17:17. doi: 10.1186/s12987-020-0178-x
Dolińska, M., Hilgier, W., and Albrecht, J. (1996). Ammonia stimulates glutamine uptake to the cerebral non-synaptic mitochondria of the rat. Neurosci. Lett. 213, 45–48. doi: 10.1016/0304-3940(96)12827-5
Dolińska, M., Zabłocka, B., Sonnewald, U., and Albrecht, J. (2004). Glutamine uptake and expression of mRNA’s of glutamine transporting proteins in mouse cerebellar and cerebral cortical astrocytes and neurons. Neurochem. Int. 44, 75–81. doi: 10.1016/s0197-0186(03)00123-2
Feng, M., Xiong, G., Cao, Z., Yang, G., Zheng, S., Qiu, J., et al. (2018). LAT2 regulates glutamine-dependent mTOR activation to promote glycolysis and chemoresistance in pancreatic cancer. J. Exp. Clin. Cancer Res. 37:274. doi: 10.1186/s13046-018-0947-4
Fernandez-Vizarra, E., and Zeviani, M. (2021). Mitochondrial disorders of the OXPHOS system. FEBS Lett. 595, 1062–1106. doi: 10.1002/1873-3468.13995
Fotiadis, D., Kanai, Y., and Palacín, M. (2013). The SLC3 and SLC7 families of amino acid transporters. Mol. Aspects Med. 34, 139–158. doi: 10.1016/j.mam.2012.10.007
Gliddon, C. M., Shao, Z., LeMaistre, J. L., and Anderson, C. M. (2009). Cellular distribution of the neutral amino acid transporter subtype ASCT2 in mouse brain. J. Neurochem. 108, 372–383. doi: 10.1111/j.1471-4159.2008.05767.x
Görg, B., Qvartskhava, N., Keitel, V., Bidmon, H. J., Selbach, O., Schliess, F., et al. (2008). Ammonia induces RNA oxidation in cultured astrocytes and brain in vivo. Hepatology 48, 567–579. doi: 10.1002/hep.22345
Gropman, A. L., Sailasuta, N., Harris, K. C., Abulseoud, O., and Ross, B. D. (2009). Ornithine transcarbamylase deficiency with persistent abnormality in cerebral glutamate metabolism in adults. Radiology 252, 833–841. doi: 10.1148/radiol.2523081878
Guo, R., Gu, J., Zong, S., Wu, M., and Yang, M. (2018). Structure and mechanism of mitochondrial electron transport chain. Biomed. J. 41, 9–20. doi: 10.1016/j.bj.2017.12.001
Hakvoort, T. B. M., He, Y., Kulik, W., Vermeulen, J. L. M., Duijst, S., Ruijter, J. M., et al. (2017). Pivotal role of glutamine synthetase in ammonia detoxification. Hepatology 65, 281–293. doi: 10.1002/hep.28852
Hamdani, E. H., Gudbrandsen, M., Bjørkmo, M., and Chaudhry, F. A. (2012). The system N transporter SN2 doubles as a transmitter precursor furnisher and a potential regulator of NMDA receptors. Glia 60, 1671–1683. doi: 10.1002/glia.22386
Hamdani, E. H., Popek, M., Frontczak-Baniewicz, M., Utheim, T. P., Albrecht, J., Zielińska, M., et al. (2021). Perturbation of astroglial Slc38 glutamine transporters by NH4 + contributes to neurophysiologic manifestations in acute liver failure. FASEB J. 35:e21588. doi: 10.1096/fj.202001712RR
Haskew, R. E., Mongin, A. A., and Kimelberg, H. K. (2002). Peroxynitrite enhances astrocytic volume-sensitive excitatory amino acid release via a src tyrosine kinase-dependent mechanism: peroxynitrite enhances EAA release. J. Neurochem. 82, 903–912. doi: 10.1046/j.1471-4159.2002.01037.x
Häussinger, D. (1990). Liver glutamine metabolism. J. Parenter. Enteral Nutr. 14, 56S–62S. doi: 10.1177/014860719001400405
Haussinger, D., and Schliess, F. (2008). Pathogenetic mechanisms of hepatic encephalopathy. Gut 57, 1156–1165. doi: 10.1136/gut.2007.122176
Haussinger, D., Soboll, S., Meijer, A. J., Gerok, W., Tager, J. M., and Sies, H. (1985). Role of plasma membrane transport in hepatic glutamine metabolism. Eur. J. Biochem. 152, 597–603. doi: 10.1111/j.1432-1033.1985.tb09237.x
Hawkins, R., and Viña, J. (2016). How glutamate is managed by the blood–brain barrier. Biology 5:37. doi: 10.3390/biology5040037
Heckel, T., Bröer, A., Wiesinger, H., Lang, F., and Bröer, S. (2003). Asymmetry of glutamine transporters in cultured neural cells. Neurochem. Int. 43, 289–298. doi: 10.1016/s0197-0186(03)00014-7
Heins, J., and Zwingmann, C. (2010). Organic osmolytes in hyponatremia and ammonia toxicity. Metab. Brain Dis. 25, 81–89. doi: 10.1007/s11011-010-9170-5
Hilgier, W., Fręśko, I., Klemenska, E., Beręsewicz, A., Oja, S. S., Saransaari, P., et al. (2009). Glutamine inhibits ammonia-induced accumulation of cGMP in rat striatum limiting arginine supply for NO synthesis. Neurobiol. Dis. 35, 75–81. doi: 10.1016/j.nbd.2009.04.004
Hilgier, W., Olson, J. E., and Albrecht, J. (1996). Relation of taurine transport and brain edema in rats with simple hyperammonemia or liver failure. J. Neurosci. Res. 45, 69–74. doi: 10.1002/(SICI)1097-4547(19960701)45:1<69::AID-JNR6<3.0.CO;2-F
Hilgier, W., Wegrzynowicz, M., Maczewski, M., Beresewicz, A., Oja, S. S., Saransaari, P., et al. (2008). Effect of glutamine synthesis inhibition with methionine sulfoximine on the nitric oxide-cyclic GMP pathway in the rat striatum treated acutely with ammonia: a microdialysis study. Neurochem. Res. 33, 267–272. doi: 10.1007/s11064-007-9455-1
Hilgier, W., Zielińska, M., Borkowska, H. D., Gadamski, R., Walski, M., Oja, S. S., et al. (1999). Changes in the extracellular profiles of neuroactive amino acids in the rat striatum at the asymptomatic stage of hepatic failure. J. Neurosci. Res. 56, 76–84. doi: 10.1002/(SICI)1097-4547(19990401)56:1<76::AID-JNR10<3.0.CO;2-Y
Huttunen, J., Peltokangas, S., Gynther, M., Natunen, T., Hiltunen, M., Auriola, S., et al. (2019). l-Type amino acid transporter 1 (LAT1/Lat1)-utilizing prodrugs can improve the delivery of drugs into neurons, astrocytes and microglia. Sci. Rep. 9:12860. doi: 10.1038/s41598-019-49009-z
Indiveri, C., Abruzzo, G., Stipani, I., and Palmieri, F. (1998). Identification and purification of the reconstitutively active glutamine carrier from rat kidney mitochondria. Biochem. J. 333, 285–290. doi: 10.1042/bj3330285
Iversen, P., Sørensen, M., Bak, L. K., Waagepetersen, H. S., Vafaee, M. S., Borghammer, P., et al. (2009). Low cerebral oxygen consumption and blood flow in patients with cirrhosis and an acute episode of hepatic encephalopathy. Gastroenterology 136, 863–871. doi: 10.1053/j.gastro.2008.10.057
Jacobson, I., Sandberg, M., and Hamberger, A. (1985). Mass transfer in brain dialysis devices—a new method for the estimation of extracellular amino acids concentration. J. Neurosci. Methods 15, 263–268. doi: 10.1016/0165-0270(85)90107-4
Jayakumar, A. R., and Norenberg, M. D. (2018). Hyperammonemia in hepatic encephalopathy. J. Clin. Exp. Hepatol. 8, 272–280. doi: 10.1016/j.jceh.2018.06.007
Jayakumar, A. R., Rama Rao, K. V., and Norenberg, M. D. (2015). Neuroinflammation in hepatic encephalopathy: mechanistic aspects. J. Clin. Exp. Hepatol. 5, S21–S28. doi: 10.1016/j.jceh.2014.07.006
Jayakumar, A. R., Rama Rao, K. V., Schousboe, A., and Norenberg, M. D. (2004). Glutamine-induced free radical production in cultured astrocytes. Glia 46, 296–301. doi: 10.1002/glia.20003
Jayakumar, A. R., Rao, K. V. R., Murthy, C. R. K., and Norenberg, M. D. (2006). Glutamine in the mechanism of ammonia-induced astrocyte swelling. Neurochem. Int. 48, 623–628. doi: 10.1016/j.neuint.2005.11.017
Jiménez, J. V., Carrillo-Pérez, D. L., Rosado-Canto, R., García-Juárez, I., Torre, A., Kershenobich, D., et al. (2017). Electrolyte and acid–base disturbances in end-stage liver disease: a physiopathological approach. Dig. Dis. Sci. 62, 1855–1871. doi: 10.1007/s10620-017-4597-8
Kageyama, T., Nakamura, M., Matsuo, A., Yamasaki, Y., Takakura, Y., Hashida, M., et al. (2000). The 4F2hc/LAT1 complex transports l-DOPA across the blood–brain barrier. Brain Res. 879, 115–121. doi: 10.1016/S0006-8993(00)02758-X
Kam, K., and Nicoll, R. (2007). Excitatory synaptic transmission persists independently of the glutamate-glutamine cycle. J. Neurosci. 27, 9192–9200. doi: 10.1523/JNEUROSCI.1198-07.2007
Kanai, Y., and Endou, H. (2001). Heterodimeric amino acid transporters: molecular biology and pathological and pharmacological relevance. CDM 2, 339–354. doi: 10.2174/1389200013338324
Kanamori, K., and Ross, B. D. (2005). Suppression of glial glutamine release to the extracellular fluid studied in vivo by NMR and microdialysis in hyperammonemic rat brain. J. Neurochem. 94, 74–85. doi: 10.1111/j.1471-4159.2005.03170.x
Kato, M., Hughes, R. D., Keays, R. T., and Williams, R. (1992). Electron microscopic study of brain capillaries in cerebral edema from fulminant hepatic failure. Hepatology 15, 1060–1066. doi: 10.1002/hep.1840150615
Kato, M., Sugihara, J., Nakamura, T., and Muto, Y. (1989). Electron microscopic study of the blood-brain barrier in rats with brain edema and encephalopathy due to acute hepatic failure. Gastroenterol. Jpn. 24, 135–142. doi: 10.1007/BF02774187
Kosenko, E., Felipo, V., Montoliu, C., Grisolía, S., and Kaminsky, Y. (1997). Effects of acute hyperammonemia in vivo on oxidative metabolism in nonsynaptic rat brain mitochondria. Metab. Brain Dis. 12, 69–82. doi: 10.1007/BF02676355
Kosenko, E., Kaminsky, Y., Lopata, O., Muravyov, N., Kaminsky, A., Hermenegildo, C., et al. (1998). Nitroarginine, an inhibitor of nitric oxide synthase, prevents changes in superoxide radical and antioxidant enzymes induced by ammonia intoxication. Metab. Brain Dis. 13, 29–41. doi: 10.1023/a:1020626928259
Kovacević, Z., and Bajin, K. (1982). Kinetics of glutamine efflux from liver mitochondria loaded with the 14C-labeled substrate. Biochim. Biophys. Acta 687, 291–295. doi: 10.1016/0005-2736(82)90557-0
Kovacević, Z., McGivan, J. D., and Chappell, J. B. (1970). Conditions for activity of glutaminase in kidney mitochondria. Biochem. J. 118, 265–274. doi: 10.1042/bj1180265
Kreis, R., Farrow, N., and Ross BrianD. (1990). Diagnosis of hepatic encephalopathy by proton magnetic resonance spectroscopy. Lancet 336, 635–636. doi: 10.1016/0140-6736(90)93439-V
Krishna Rao, R. (2012). Role of glutamine in protection of intestinal epithelial tight junctions. JEBP 5, 47–54. doi: 10.2174/1875044301205010047
Kruczek, C., Görg, B., Keitel, V., Bidmon, H. J., Schliess, F., and Häussinger, D. (2011). Ammonia increases nitric oxide, free Zn(2+), and metallothionein mRNA expression in cultured rat astrocytes. Biol. Chem. 392, 1155–1165. doi: 10.1515/BC.2011.199
Kuhn, K. S., Schuhmann, K., Stehle, P., Darmaun, D., and Fürst, P. (1999). Determination of glutamine in muscle protein facilitates accurate assessment of proteolysis and de novo synthesis–derived endogenous glutamine production. Am. J. Clin. Nutr. 70, 484–489. doi: 10.1093/ajcn/70.4.484
Laake, J. H., Takumi, Y., Eidet, J., Torgner, I. A., Roberg, B., Kvamme, E., et al. (1999). Postembedding immunogold labelling reveals subcellular localization and pathway-specific enrichment of phosphate activated glutaminase in rat cerebellum. Neuroscience 88, 1137–1151. doi: 10.1016/S0306-4522(98)00298-X
Labow, B. I., and Souba, W. W. (2000). Glutamine. World J. Surg. 24, 1503–1513. doi: 10.1007/s002680010269
Larsen, F. S. (2002). Cerebral blood flow in hyperammonemia: heterogeneity and starling forces in capillaries. Metab. Brain Dis. 17, 229–235. doi: 10.1023/a:1021941414605
Laubenberger, J., Haussinger, D., Bayer, S., Gufler, H., Hennig, J., and Langer, M. (1997). Proton magnetic resonance spectroscopy of the brain in symptomatic and asymptomatic patients with liver cirrhosis. Gastroenterology 112, 1610–1616. doi: 10.1016/S0016-5085(97)70043-X
Leke, R., and Schousboe, A. (2016). The glutamine transporters and their role in the glutamate/GABA-glutamine cycle. Adv. Neurobiol. 13, 223–257. doi: 10.1007/978-3-319-45096-4_8
Leke, R., Escobar, T. D. C., Rama Rao, K. V., Silveira, T. R., Norenberg, M. D., and Schousboe, A. (2015). Expression of glutamine transporter isoforms in cerebral cortex of rats with chronic hepatic encephalopathy. Neurochem. Int. 88, 32–37. doi: 10.1016/j.neuint.2015.03.005
Liang, S.-L., Carlson, G. C., and Coulter, D. A. (2006). Dynamic regulation of synaptic GABA release by the glutamate-glutamine cycle in hippocampal area CA1. J. Neurosci. 26, 8537–8548. doi: 10.1523/JNEUROSCI.0329-06.2006
Lister, A., Bourgeois, S., Imenez Silva, P. H., Rubio-Aliaga, I., Marbet, P., Walsh, J., et al. (2018). NRF2 regulates the glutamine transporter Slc38a3 (SNAT3) in kidney in response to metabolic acidosis. Sci. Rep. 8:5629. doi: 10.1038/s41598-018-24000-2
Liu, R., Hong, R., Wang, Y., Gong, Y., Yeerken, D., Yang, D., et al. (2020). Defect of SLC38A3 promotes epithelial mesenchymal transition and predicts poor prognosis in esophageal squamous cell carcinoma. Chin. J. Cancer Res. 32, 547–563. doi: 10.21147/j.issn.1000-9604.2020.05.01
Lyck, R., Ruderisch, N., Moll, A. G., Steiner, O., Cohen, C. D., Engelhardt, B., et al. (2009). Culture-induced changes in blood—brain barrier transcriptome: implications for amino-acid transporters in vivo. J. Cereb. Blood Flow Metab. 29, 1491–1502. doi: 10.1038/jcbfm.2009.72
Mann, G., Mora, S., Madu, G., and Adegoke, O. A. J. (2021). Branched-chain amino acids: catabolism in skeletal muscle and implications for muscle and whole-body metabolism. Front. Physiol. 12:702826. doi: 10.3389/fphys.2021.702826
Mans, A. M., Biebuyck, J. F., Shelly, K., and Hawkins, R. A. (1982). Regional blood-brain barrier permeability to amino acids after portacaval anastomosis. J. Neurochem. 38, 705–717. doi: 10.1111/j.1471-4159.1982.tb08689.x
Martinez, A. (1968). Electron microscopy in human hepatic encephalopathy. Acta Neuropathol. 11, 82–86. doi: 10.1007/BF00692797
Martinez-Hernandez, A., Bell, K. P., and Norenberg, M. D. (1977). Glutamine synthetase: glial localization in brain. Science 195, 1356–1358. doi: 10.1126/science.14400
Martínez-Lozada, Z., Guillem, A. M., Flores-Méndez, M., Hernández-Kelly, L. C., Vela, C., Meza, E., et al. (2013). GLAST/EAAT1-induced glutamine release via SNAT3 in Bergmann glial cells: evidence of a functional and physical coupling. J. Neurochem. 125, 545–554. doi: 10.1111/jnc.12211
Master, S., Gottstein, J., and Blei, A. T. (1999). Cerebral blood flow and the development of ammonia-induced brain edema in rats after portacaval anastomosis. Hepatology 30, 876–880. doi: 10.1002/hep.510300428
Matés, J. M., Segura, J. A., Campos-Sandoval, J. A., Lobo, C., Alonso, L., Alonso, F. J., et al. (2009). Glutamine homeostasis and mitochondrial dynamics. Int. J. Biochem. Cell Biol. 41, 2051–2061. doi: 10.1016/j.biocel.2009.03.003
McKenna, M. C., Sonnewald, U., Huang, X., Stevenson, J., and Zielke, H. R. (1996). Exogenous glutamate concentration regulates the metabolic fate of glutamate in astrocytes. J. Neurochem. 66, 386–393. doi: 10.1046/j.1471-4159.1996.66010386.x
McKenna, M. C., Stridh, M. H., McNair, L. F., Sonnewald, U., Waagepetersen, H. S., and Schousboe, A. (2016). Glutamate oxidation in astrocytes: roles of glutamate dehydrogenase and aminotransferases: role of GDH in glutamate oxidation in astrocytes. J. Neurosci. Res. 94, 1561–1571. doi: 10.1002/jnr.23908
Meier, C. (2002). Activation of system L heterodimeric amino acid exchangers by intracellular substrates. EMBO J. 21, 580–589. doi: 10.1093/emboj/21.4.580
Michalak, A., Rose, C., Butterworth, J., and Butterworth, R. F. (1996). Neuroactive amino acids and glutamate (n.d.) receptors in frontal cortex of rats with experimental acute liver failure. Hepatology 24, 908–913. doi: 10.1002/hep.510240425
Michalec, K., Mysiorek, C., Kuntz, M., Bérézowski, V., Szczepankiewicz, A. A., Wilczyński, G. M., et al. (2014). Protein kinase C restricts transport of carnitine by amino acid transporter ATB0,+ apically localized in the blood–brain barrier. Arch. Biochem. Biophys. 554, 28–35. doi: 10.1016/j.abb.2014.05.006
Milewski, K., Bogacińska-Karaś, M., Fręśko, I., Hilgier, W., Jaźwiec, R., Albrecht, J., et al. (2017). Ammonia reduces intracellular asymmetric dimethylarginine in cultured astrocytes stimulating its y+LAT2 carrier-mediated loss. IJMS 18:2308. doi: 10.3390/ijms18112308
Milewski, K., Bogacińska-Karaś, M., Hilgier, W., Albrecht, J., and Zielińska, M. (2019). TNFα increases STAT3-mediated expression of glutaminase isoform KGA in cultured rat astrocytes. Cytokine 123:54774. doi: 10.1016/j.cyto.2019.154774
Minn, A. (1982). Glutamine uptake by isolated rat brain mitochondria. Neuroscience 7, 2859–2865. doi: 10.1016/0306-4522(82)90108-7
Molina, M., Segura, J. A., Aledo, J. C., Medina, M. A., Núnez de Castro, I., and Márquez, J. (1995). Glutamine transport by vesicles isolated from tumour-cell mitochondrial inner membrane. Biochem. J. 308, 629–633. doi: 10.1042/bj3080629
Murthy, C. R., Rama Rao, K. V., Bai, G., and Norenberg, M. D. (2001). Ammonia-induced production of free radicals in primary cultures of rat astrocytes. J. Neurosci. Res. 66, 282–288. doi: 10.1002/jnr.1222
Nagaraja, T. N., and Brookes, N. (1996). Glutamine transport in mouse cerebral astrocytes. J. Neurochem. 66, 1665–1674. doi: 10.1046/j.1471-4159.1996.66041665.x
Nakanishi, T., Kekuda, R., Fei, Y. J., Hatanaka, T., Sugawara, M., Martindale, R. G., et al. (2001a). Cloning and functional characterization of a new subtype of the amino acid transport system N. Am. J. Physiol. Cell Physiol. 281, C1757–C1768. doi: 10.1152/ajpcell.2001.281.6.C1757
Nakanishi, T., Sugawara, M., Huang, W., Martindale, R. G., Leibach, F. H., Ganapathy, M. E., et al. (2001b). Structure, function, and tissue expression pattern of human SN2, a subtype of the amino acid transport system N. Biochem. Biophys. Res. Commun. 281, 1343–1348. doi: 10.1006/bbrc.2001.4504
Nałęcz, K. A. (2020). Amino acid transporter SLC6A14 (ATB0,+) – a target in combined anti-cancer therapy. Front. Cell Dev. Biol. 8:594464. doi: 10.3389/fcell.2020.594464
Nicklin, P., Bergman, P., Zhang, B., Triantafellow, E., Wang, H., Nyfeler, B., et al. (2009). Bidirectional transport of amino acids regulates mTOR and autophagy. Cell 136, 521–534. doi: 10.1016/j.cell.2008.11.044
Nissen-Meyer, L. S. H., and Chaudhry, F. A. (2013). Protein kinase c phosphorylates the system n glutamine transporter SN1 (Slc38a3) and regulates its membrane trafficking and degradation. Front. Endocrinol. 4:138. doi: 10.3389/fendo.2013.00138
Norenberg, M. D. (1977). A light and electron microscopic study of experimental portal-systemic (ammonia) encephalopathy. Progression and reversal of the disorder. Lab. Invest. 36, 618–627.
Norenberg, M. D. (1987). The role of astrocytes in hepatic encephalopathy. Neurochem. Pathol. 6, 13–33. doi: 10.1007/BF02833599
Norenberg, M. D., Rama Rao, K. V., and Jayakumar, A. R. (2004). Ammonia neurotoxicity and the mitochondrial permeability transition. J. Bioenerg. Biomembr. 36, 303–307. doi: 10.1023/B:JOBB.0000041758.20071.19
O’Brien, K. B., Miller, R. F., and Bowser, M. T. (2005). D-Serine uptake by isolated retinas is consistent with ASCT-mediated transport. Neurosci. Lett. 385, 58–63. doi: 10.1016/j.neulet.2005.05.009
O’Kane, R. L., and Hawkins, R. A. (2003). Na + –dependent transport of large neutral amino acids occurs at the abluminal membrane of the blood-brain barrier. Am. J. Physiol. Endocrinol. Metab. 285, E1167–E1173. doi: 10.1152/ajpendo.00193.2003
Obara-Michlewska, M., Ding, F., Popek, M., Verkhratsky, A., Nedergaard, M., Zielinska, M., et al. (2018). Interstitial ion homeostasis and acid-base balance are maintained in oedematous brain of mice with acute toxic liver failure. Neurochem. Int. 118, 286–291. doi: 10.1016/j.neuint.2018.05.007
Odeh, M., Sabo, E., Srugo, I., and Oliven, A. (2004). Serum levels of tumor necrosis factor-alpha correlate with severity of hepatic encephalopathy due to chronic liver failure. Liver Int. 24, 110–116. doi: 10.1111/j.1478-3231.2004.0894.x
Oehler, R., and Roth, E. (2003). Regulative capacity of glutamine. Curr. Opin. Clin. Nutr. Metab. Care 6, 277–282. doi: 10.1097/01.mco.0000068962.34812.ac
Ong, J. P., Aggarwal, A., Krieger, D., Easley, K. A., Karafa, M. T., Van Lente, F., et al. (2003). Correlation between ammonia levels and the severity of hepatic encephalopathy. Am. J. Med. 114, 188–193. doi: 10.1016/S0002-9343(02)01477-8
Ott, P., and Larsen, F. S. (2004). Blood–brain barrier permeability to ammonia in liver failure: a critical reappraisal. Neurochem. Int. 44, 185–198. doi: 10.1016/S0197-0186(03)00153-0
Papadopoulos, M. C., and Verkman, A. S. (2007). Aquaporin-4 and brain edema. Pediatr. Nephrol. 22, 778–784. doi: 10.1007/s00467-006-0411-0
Pasantes-Morales, H., Franco, R., Ordaz, B., and Ochoa, L. D. (2002). Mechanisms counteracting swelling in brain cells during hyponatremia. Arch. Med. Res. 33, 237–244. doi: 10.1016/S0188-4409(02)00353-3
Perry, T. L., Hansen, S., and Kennedy, J. (1975). CSF amino acids and plasma-CSF amino acid ratios in adults. J. Neurochem. 24, 587–589. doi: 10.1111/j.1471-4159.1975.tb07680.x
Pfeiffer, R., Spindler, B., Loffing, J., Skelly, P. J., Shoemaker, C. B., and Verrey, F. (1998). Functional heterodimeric amino acid transporters lacking cysteine residues involved in disulfide bond. FEBS Lett. 439, 157–162. doi: 10.1016/S0014-5793(98)01359-3
Pichili, V. B. R., Rao, K. V. R., Jayakumar, A. R., and Norenberg, M. D. (2007). Inhibition of glutamine transport into mitochondria protects astrocytes from ammonia toxicity. Glia 55, 801–809. doi: 10.1002/glia.20499
Pluta, R., and Albrecht, J. (1986). Changes in arterial and cerebral venous blood gases, cerebral blood flow and cerebral oxygen consumption at different stages of thioacetamide-induced hepatogenic encephalopathy in rat. Resuscitation 14, 135–139. doi: 10.1016/0300-9572(86)90117-6
Pochini, L., Scalise, M., Galluccio, M., and Indiveri, C. (2014). Membrane transporters for the special amino acid glutamine: structure/function relationships and relevance to human health. Front. Chem. 2:61. doi: 10.3389/fchem.2014.00061
Popek, M., Bobula, B., Sowa, J., Hess, G., Frontczak-Baniewicz, M., Albrecht, J., et al. (2020). Physiology and morphological correlates of excitatory transmission are preserved in glutamine transporter SN1-depleted mouse frontal cortex. Neuroscience 446, 124–136. doi: 10.1016/j.neuroscience.2020.08.019
Popek, M., Bobula, B., Sowa, J., Hess, G., Polowy, R., Filipkowski, R. K., et al. (2018). Cortical synaptic transmission and plasticity in acute liver failure are decreased by presynaptic events. Mol. Neurobiol. 55, 1244–1258. doi: 10.1007/s12035-016-0367-4
Rama Rao, K. V., and Norenberg, M. D. (2012). Brain energy metabolism and mitochondrial dysfunction in acute and chronic hepatic encephalopathy. Neurochem. Int. 60, 697–706. doi: 10.1016/j.neuint.2011.09.007
Rama Rao, K. V., and Norenberg, M. D. (2014). Glutamine in the pathogenesis of hepatic encephalopathy: the trojan horse hypothesis revisited. Neurochem. Res. 39, 593–598. doi: 10.1007/s11064-012-0955-2
Rama Rao, K. V., Reddy, P. V. B., Tong, X., and Norenberg, M. D. (2010). Brain edema in acute liver failure. Am. J. Pathol. 176, 1400–1408. doi: 10.2353/ajpath.2010.090756
Rao, V. L., Audet, R. M., and Butterworth, R. F. (1995). Selective alterations of extracellular brain amino acids in relation to function in experimental portal-systemic encephalopathy: results of an in vivo microdialysis study. J. Neurochem. 65, 1221–1228. doi: 10.1046/j.1471-4159.1995.65031221.x
Roberg, B. (1995). The orientation of phosphate activated glutaminase in the inner mitochondrial membrane of synaptic and non-synaptic rat brain mitochondria. Neurochem. Int. 27, 367–376. doi: 10.1016/0197-0186(95)00018-4
Rodrigo, R., Cauli, O., Gomez-Pinedo, U., Agusti, A., Hernandez-Rabaza, V., Garcia-Verdugo, J., et al. (2010). Hyperammonemia induces neuroinflammation that contributes to cognitive impairment in rats with hepatic encephalopathy. Gastroenterology 139, 675–684. doi: 10.1053/j.gastro.2010.03.040
Rose, C. F., Verkhratsky, A., and Parpura, V. (2013). Astrocyte glutamine synthetase: pivotal in health and disease. Biochem. Soc. Trans. 41, 1518–1524. doi: 10.1042/BST20130237
Rothman, D. L., Behar, K. L., Hyder, F., and Shulman, R. G. (2003). In vivo NMR studies of the glutamate neurotransmitter flux and neuroenergetics: implications for brain function. Annu. Rev. Physiol. 65, 401–427. doi: 10.1146/annurev.physiol.65.092101.142131
Rubio-Aliaga, I., and Wagner, C. A. (2016). Regulation and function of the SLC38A3/SNAT3 glutamine transporter. Channels 10, 440–452. doi: 10.1080/19336950.2016.1207024
Samluk, L., Czeredys, M., and Nałęcz, K. A. (2010). Regulation of amino acid/carnitine transporter B0,+ (ATB0,+) in astrocytes by protein kinase C: independent effects on raft and non-raft transporter subpopulations: regulation of ATB0,+ by protein kinase C. J. Neurochem. 115, 1386–1397. doi: 10.1111/j.1471-4159.2010.07040.x
Scalise, M., Galluccio, M., Console, L., Pochini, L., and Indiveri, C. (2018a). The human SLC7A5 (LAT1): the intriguing histidine/large neutral amino acid transporter and its relevance to human health. Front. Chem. 6:243. doi: 10.3389/fchem.2018.00243
Scalise, M., Pochini, L., Console, L., Losso, M. A., and Indiveri, C. (2018b). The human SLC1A5 (ASCT2) amino acid transporter: from function to structure and role in cell biology. Front. Cell Dev. Biol. 6:96. doi: 10.3389/fcell.2018.00096
Schliess, F., Görg, B., Fischer, R., Desjardins, P., Bidmon, H. J., Herrmann, A., et al. (2002). Ammonia induces MK-801-sensitive nitration and phosphorylation of protein tyrosine residues in rat astrocytes. FASEB J. 16, 739–741. doi: 10.1096/fj.01-0862fje
Schousboe, A., Scafidi, S., Bak, L. K., Waagepetersen, H. S., and McKenna, M. C. (2014). “Glutamate metabolism in the brain focusing on astrocytes,” in Glutamate and ATP at the Interface of Metabolism and Signaling in the Brain Advances in Neurobiology, eds V. Parpura, A. Schousboe, and A. Verkhratsky (Cham: Springer International Publishing), 13–30. doi: 10.1007/978-3-319-08894-5_2
Scott, T. R. (2013). Pathophysiology of cerebral oedema in acute liver failure. WJG 19:9240. doi: 10.3748/wjg.v19.i48.9240
Sen, K., Castillo Pinto, C., and Gropman, A. L. (2021). Expanding role of proton magnetic resonance spectroscopy: timely diagnosis and treatment initiation in partial ornithine transcarbamylase deficiency. J. Pediatr. Genet. 10, 77–80. doi: 10.1055/s-0040-1709670
Shawcross, D. L., Wright, G., Olde Damink, S. W. M., and Jalan, R. (2007). Role of ammonia and inflammation in minimal hepatic encephalopathy. Metab. Brain Dis. 22, 125–138. doi: 10.1007/s11011-006-9042-1
Sidoryk-Węgrzynowicz, M., Lee, E., Albrecht, J., and Aschner, M. (2009). Manganese disrupts astrocyte glutamine transporter expression and function. J. Neurochem. 110, 822–830. doi: 10.1111/j.1471-4159.2009.06172.x
Sinke, A. P., Jayakumar, A. R., Panickar, K. S., Moriyama, M., Reddy, P. V. B., and Norenberg, M. D. (2008). NFB in the mechanism of ammonia-induced astrocyte swelling in culture. J. Neurochem. 106, 2302–2311. doi: 10.1111/j.1471-4159.2008.05549.x
Sivaprakasam, S., Sikder, M. O. F., Ramalingam, L., Kaur, G., Dufour, J. M., Moustaid-Moussa, N., et al. (2021). SLC6A14 deficiency is linked to obesity, fatty liver, and metabolic syndrome but only under conditions of a high-fat diet. Biochim. Biophys. Acta 1867:166087. doi: 10.1016/j.bbadis.2021.166087
Sloan, J. L., and Mager, S. (1999). Cloning and functional expression of a human Na+and Cl–dependent neutral and cationic amino acid transporter B0+. J. Biol. Chem. 274, 23740–23745. doi: 10.1074/jbc.274.34.23740
Solbu, T. T. (2010). SAT1, a glutamine transporter, is preferentially expressed in GABAergic neurons. Front. Neuroanat 4:1. doi: 10.3389/neuro.05.001.2010
Spodenkiewicz, M., Diez-Fernandez, C., Rüfenacht, V., Gemperle-Britschgi, C., and Häberle, J. (2016). Minireview on glutamine synthetase deficiency, an ultra-rare inborn error of amino acid biosynthesis. Biology 5:40. doi: 10.3390/biology5040040
Steib, A., Rendon, A., Mark, J., and Borg, J. (1986). Preferential glutamine uptake in rat brain synaptic mitochondria. FEBS Lett. 207, 63–68. doi: 10.1016/0014-5793(86)80013-8
Sterns, R. H., and Silver, S. M. (2006). Brain volume regulation in response to hypo-osmolality and its correction. Am. J. Med. 119, S12–S16. doi: 10.1016/j.amjmed.2006.05.003
Stravitz, R. T., and Larsen, F. S. (2009). Therapeutic hypothermia for acute liver failure. Crit. Care Med. 37, S258–S264. doi: 10.1097/CCM.0b013e3181aa5fb8
Takahashi, H., Koehler, R. C., Brusilow, S. W., and Traystman, R. J. (1991). Inhibition of brain glutamine accumulation prevents cerebral edema in hyperammonemic rats. Am. J. Physiol. 261, H825–H829. doi: 10.1152/ajpheart.1991.261.3.H825
Tanigami, H., Rebel, A., Martin, L. J., Chen, T.-Y., Brusilow, S. W., Traystman, R. J., et al. (2005). Effect of glutamine synthetase inhibition on astrocyte swelling and altered astroglial protein expression during hyperammonemia in rats. Neuroscience 131, 437–449. doi: 10.1016/j.neuroscience.2004.10.045
Tapiero, H., Mathé, G., Couvreur, P., and Tew, K. D. (2002). II. Glutamine and glutamate. Biomed. Pharmacother. 56, 446–457. doi: 10.1016/S0753-3322(02)00285-8
Tǎrlungeanu, D. C., Deliu, E., Dotter, C. P., Kara, M., Janiesch, P. C., Scalise, M., et al. (2016). Impaired amino acid transport at the blood brain barrier is a cause of autism spectrum disorder. Cell 167, 1481.e–1494.e. doi: 10.1016/j.cell.2016.11.013
Thrane, A. S., Rappold, P. M., Fujita, T., Torres, A., Bekar, L. K., Takano, T., et al. (2011). Critical role of aquaporin-4 (AQP4) in astrocytic Ca2+ signaling events elicited by cerebral edema. Proc. Natl. Acad. Sci. U.S.A. 108, 846–851. doi: 10.1073/pnas.1015217108
Todd, A. C., Marx, M.-C., Hulme, S. R., Bröer, S., and Billups, B. (2017). SNAT3-mediated glutamine transport in perisynaptic astrocytes in situ is regulated by intracellular sodium. Glia 65, 900–916. doi: 10.1002/glia.23133
Tofteng, F., Hauerberg, J., Hansen, B. A., Pedersen, C. B., Jørgensen, L., and Larsen, F. S. (2006). Persistent arterial hyperammonemia increases the concentration of glutamine and alanine in the brain and correlates with intracranial pressure in patients with fulminant hepatic failure. J. Cereb. Blood Flow Metab. 26, 21–27. doi: 10.1038/sj.jcbfm.9600168
Tofteng, F., Jorgensen, L., Hansen, B. A., Ott, P., Kondrup, J., and Larsen, F. S. (2002). Cerebral microdialysis in patients with fulminant hepatic failure: cerebral microdialysis in patients with fulminant hepatic failure. Hepatology 36, 1333–1340. doi: 10.1002/hep.1840360607
Torrents, D., Estévez, R., Pineda, M., Fernández, E., Lloberas, J., Shi, Y. B., et al. (1998). Identification and characterization of a membrane protein (y+L amino acid transporter-1) that associates with 4F2hc to encode the amino acid transport activity y+L. A candidate gene for lysinuric protein intolerance. J. Biol. Chem. 273, 32437–32445. doi: 10.1074/jbc.273.49.32437
Traber, P. G., Canto, M. D., Ganger, D. R., and Blei, A. T. (1987). Electron microscopic evaluation of brain edema in rabbits with galactosamine-induced fulminant hepatic failure: ultrastructure and integrity of the blood-brain barrier. Hepatology 7, 1272–1277. doi: 10.1002/hep.1840070616
Traber, P., DalCanto, M., Ganger, D., and Blei, A. T. (1989). Effect of body temperature on brain edema and encephalopathy in the rat after hepatic devascularization. Gastroenterology 96, 885–891.
Vaquero, J., and Blei, A. T. (2004). Cooling the patient with acute liver failure. Gastroenterology 127, 1626–1629. doi: 10.1053/j.gastro.2004.09.058
Vaquero, J., and Butterworth, R. F. (2007). Mechanisms of brain edema in acute liver failure and impact of novel therapeutic interventions. Neurol. Res. 29, 683–690. doi: 10.1179/016164107X240099
Vercellino, I., and Sazanov, L. A. (2022). The assembly, regulation and function of the mitochondrial respiratory chain. Nat. Rev. Mol. Cell Biol. 23, 141–161. doi: 10.1038/s41580-021-00415-0
Verkhratsky, A., Parpura, V., Li, B., and Scuderi, C. (2021). “Astrocytes: the housekeepers and guardians of the CNS,” in Astrocytes in Psychiatric Disorders Advances in Neurobiology, eds B. Li, V. Parpura, A. Verkhratsky, and C. Scuderi (Cham: Springer International Publishing), 21–53. doi: 10.1007/978-3-030-77375-5_2
Wagner, C. A., Lang, F., and Bröer, S. (2001). Function and structure of heterodimeric amino acid transporters. Am. J. Physiol. 281, C1077–C1093. doi: 10.1152/ajpcell.2001.281.4.C1077
Wang, B., Wu, G., Zhou, Z., Dai, Z., Sun, Y., Ji, Y., et al. (2015). Glutamine and intestinal barrier function. Amino Acids 47, 2143–2154. doi: 10.1007/s00726-014-1773-4
Wang, Q., and Holst, J. (2015). L-type amino acid transport and cancer: targeting the mTORC1 pathway to inhibit neoplasia. Am. J. Cancer Res. 5, 1281–1294.
Wang, Y., Fu, L., Cui, M., Wang, Y., Xu, Y., Li, M., et al. (2017). Amino acid transporter SLC38A3 promotes metastasis of non-small cell lung cancer cells by activating PDK1. Cancer Lett. 393, 8–15. doi: 10.1016/j.canlet.2017.01.036
Wilkinson, S. P., Arroyo, V., Moodie, H., and Williams, R. (1974). Proceedings: endotoxaemia in fulminant hepatic failure. Clin. Sci. Mol. Med. 46, 30–31. doi: 10.1042/cs046030pb
Willard-Mack, C. L., Koehler, R. C., Hirata, T., Cork, L. C., Takahashi, H., Traystman, R. J., et al. (1996). Inhibition of glutamine synthetase reduces ammonia-induced astrocyte swelling in rat. Neuroscience 71, 589–599. doi: 10.1016/0306-4522(95)00462-9
Wise, D. R., DeBerardinis, R. J., Mancuso, A., Sayed, N., Zhang, X.-Y., Pfeiffer, H. K., et al. (2008). Myc regulates a transcriptional program that stimulates mitochondrial glutaminolysis and leads to glutamine addiction. Proc. Natl. Acad. Sci. U.S.A. 105, 18782–18787. doi: 10.1073/pnas.0810199105
Witt, A. M., Larsen, F. S., and Bjerring, P. N. (2017). Accumulation of lactate in the rat brain during hyperammonaemia is not associated with impaired mitochondrial respiratory capacity. Metab. Brain Dis. 32, 461–470. doi: 10.1007/s11011-016-9934-7
Wyke, R. J., Canalese, J. C., Gimson, A. E., and Williams, R. (1982). Bacteraemia in patients with fulminant hepatic failure. Liver 2, 45–52. doi: 10.1111/j.1600-0676.1982.tb00177.x
Xu, G.-Y., McAdoo, D. J., Hughes, M. G., Robak, G., and de Castro, R. (1998). Considerations in the determination by microdialysis of resting extracellular amino acid concentrations and release upon spinal cord injury. Neuroscience 86, 1011–1021. doi: 10.1016/S0306-4522(98)00063-3
Yoo, H. C., Park, S. J., Nam, M., Kang, J., Kim, K., Yeo, J. H., et al. (2020). A Variant of SLC1A5 is a mitochondrial glutamine transporter for metabolic reprogramming in cancer cells. Cell Metab. 31, 267.e–283.e. doi: 10.1016/j.cmet.2019.11.020
Ytrebø, L. M., Kristiansen, R. G., Maehre, H., Fuskevåg, O. M., Kalstad, T., Revhaug, A., et al. (2009). L-ornithine phenylacetate attenuates increased arterial and extracellular brain ammonia and prevents intracranial hypertension in pigs with acute liver failure. Hepatology 50, 165–174. doi: 10.1002/hep.22917
Yudkoff, M., Daikhin, Y., Nissim, I., and Nissim, I. (2000). Acidosis and astrocyte amino acid metabolism. Neurochem. Int. 36, 329–339. doi: 10.1016/S0197-0186(99)00141-2
Zalman, L. S., Nikaido, H., and Kagawa, Y. (1980). Mitochondrial outer membrane contains a protein producing nonspecific diffusion channels. J. Biol. Chem. 255, 1771–1774.
Zaragozá, R. (2020). Transport of amino acids across the blood-brain barrier. Front. Physiol. 11:973. doi: 10.3389/fphys.2020.00973
Zielińska, M., Dąbrowska, K., Hadera, M. G., Sonnewald, U., and Albrecht, J. (2016). System N transporters are critical for glutamine release and modulate metabolic fluxes of glucose and acetate in cultured cortical astrocytes: changes induced by ammonia. J. Neurochem. 136, 329–338. doi: 10.1111/jnc.13376
Zielińska, M., Law, R. O., and Albrecht, J. (2003). Excitotoxic mechanism of cell swelling in rat cerebral cortical slices treated acutely with ammonia. Neurochem. Int. 43, 299–303. doi: 10.1016/s0197-0186(03)00015-9
Zielińska, M., Milewski, K., Skowrońska, M., Gajos, A., Ziemińska, E., Beręsewicz, A., et al. (2015). Induction of inducible nitric oxide synthase expression in ammonia-exposed cultured astrocytes is coupled to increased arginine transport by upregulated y(+)LAT2 transporter. J. Neurochem. 135, 1272–1281. doi: 10.1111/jnc.13387
Zielińska, M., Popek, M., and Albrecht, J. (2014). Roles of changes in active glutamine transport in brain edema development during hepatic encephalopathy: an emerging concept. Neurochem. Res. 39, 599–604. doi: 10.1007/s11064-013-1141-x
Zielińska, M., Ruszkiewicz, J., Hilgier, W., Fręśko, I., and Albrecht, J. (2011). Hyperammonemia increases the expression and activity of the glutamine/arginine transporter y+LAT2 in rat cerebral cortex: implications for the nitric oxide/cGMP pathway. Neurochem. Int. 58, 190–195. doi: 10.1016/j.neuint.2010.11.015
Zielińska, M., Skowrońska, M., Fręśko, I., and Albrecht, J. (2012). Upregulation of the heteromeric y+LAT2 transporter contributes to ammonia-induced increase of arginine uptake in rat cerebral cortical astrocytes. Neurochem. Int. 61, 531–535. doi: 10.1016/j.neuint.2012.02.021
Ziemińska, E., Dolińska, M., Łazarewicz, J. W., and Albrecht, J. (2000a). Induction of permeability transition and swelling of rat brain mitochondria by glutamine. Neurotoxicology 21, 295–300.
Ziemińska, E., Matyja, E., Nałecz, M., Salińska, E., Ziembowicz, A., and Łazarewicz, J. W. (2000b). In vivo brain microdialysis as a tool in studies of neuroprotective effects of cyclosporin A in acute excitotoxicity. Acta Pol. Pharm. 57(Suppl.), 129–133.
Ziemińska, E., Hilgier, W., Waagepetersen, H. S., Hertz, L., Sonnewald, U., Schousboe, A., et al. (2004). Analysis of glutamine accumulation in rat brain mitochondria in the presence of a glutamine uptake inhibitor. histidine, reveals glutamine pools with a distinct access to deamidation. Neurochem. Res. 29, 2121–2123. doi: 10.1007/s11064-004-6885-x
Keywords: glutamine, hyperammonemia, astrocytes, edema, Slc38a3, Slc7a6
Citation: Zielińska M, Albrecht J and Popek M (2022) Dysregulation of Astrocytic Glutamine Transport in Acute Hyperammonemic Brain Edema. Front. Neurosci. 16:874750. doi: 10.3389/fnins.2022.874750
Received: 14 February 2022; Accepted: 17 May 2022;
Published: 06 June 2022.
Edited by:
Lori A. Knackstedt, University of Florida, United StatesReviewed by:
Larissa Daniele Bobermin, University of Rio Grande do Sul, BrazilGustavo C. Ferreira, Federal University of Rio de Janeiro, Brazil
Copyright © 2022 Zielińska, Albrecht and Popek. This is an open-access article distributed under the terms of the Creative Commons Attribution License (CC BY). The use, distribution or reproduction in other forums is permitted, provided the original author(s) and the copyright owner(s) are credited and that the original publication in this journal is cited, in accordance with accepted academic practice. No use, distribution or reproduction is permitted which does not comply with these terms.
*Correspondence: Magdalena Zielińska, bXppZWxpbnNrYUBpbWRpay5wYW4ucGw=