- 1Laboratorio de Neurofarmacología, Facultad de Ciencias Químicas, Benemérita Universidad Autónoma de Puebla, Puebla, Mexico
- 2Facultad de Ciencias Biológicas, Benemérita Universidad Autónoma de Puebla, Puebla, Mexico
- 3Laboratorio de Biología Molecular y Virología, Centro de Investigación Biomédica de Oriente, Instituto Mexicano del Seguro Social, Atlixco, Mexico
- 4Centro de Detección Biomolecular, Benemérita Universidad Autónoma de Puebla, Puebla, Mexico
- 5Department of Pharmacology, Howard University College of Medicine, Washington, DC, United States
Evidence suggests that SARS-CoV-2 entry into the central nervous system can result in neurological and/or neurodegenerative diseases. In this review, routes of SARS-Cov-2 entry into the brain via neuroinvasive pathways such as transcribrial, ocular surface or hematogenous system are discussed. It is argued that SARS-Cov-2-induced cytokine storm, neuroinflammation and oxidative stress increase the risk of developing neurodegenerative diseases such as Alzheimer’s disease and Parkinson’s disease. Further studies on the effects of SARS-CoV-2 and its variants on protein aggregation, glia or microglia activation, and blood-brain barrier are warranted.
Introduction
On December 12, 2019, in Wuhan City, Hubei Province, China, the first cases of an unexplained pneumonia failing to respond to the standard treatment regimen led to an exhaustive search for a new virus. The clinical symptoms of the condition were fever, dry cough, sore throat, pneumonia, severe dyspnea, and myalgia (Huang C. et al., 2020; Zhu N. et al., 2020). Subsequently, on February 11, 2020, the new coronavirus was identified and was termed severe acute respiratory syndrome coronavirus 2 (SARS-CoV-2). Coincidentally, a day earlier, the first draft genome of the virus was made publicly available. This enabled research groups to develop different molecular diagnostics such as RT-PCR and immunological assays. Later, CRISPR-based assays, nucleic acid microarray assays, and next generation sequencing were added (Habibzadeh et al., 2021).
The World Health Organization (WHO) is responsible for declaring a pandemic. WHO monitors disease activity on a global scale through a network of centers located in countries worldwide and has a pandemic preparedness plan that consists of six phases of pandemic alert. Phase 1 represents the lowest level of alert and usually indicates that a newly emerged or previously existing virus is circulating among animals, with low risk of transmission to humans. Phase 6, the pandemic phase, is declared when an outbreak is characterized by globally widespread and sustained disease transmission among humans (Rogers, 2022). Since by the end of February 2020, COVID-19 had already registered 83,652 cases globally (Wassie et al., 2020; Carvalho et al., 2021). On March 11, 2020, WHO declared COVID-19 a pandemic.
Patients who have concomitant comorbidities and patients admitted to the intensive care unit are significantly more likely to develop complications from COVID-19 (Sanyaolu et al., 2020). Elderly and seriously ill patients with a clinical history of cardiovascular, liver, and/or kidney disease carry the highest risk of mortality. Obesity is also a risk factor for all ages (Huang Y. et al., 2020), and age, sex, ethnicity, socioeconomic group, and geographical location may also influence the outcome (Harwood et al., 2022).
The SARS-CoV-2 belongs to the Coronaviridae family of the genus betacoronavirus (βCoV) and was identified as the etiological agent of COVID-19. SARS-CoV-2, like other known coronaviruses, is an enveloped virus with single-stranded positive sense RNA and a genome approximately 29.9 kb in size (Masters, 2006; Rastogi et al., 2020). Genetically, SARS-CoV-2 and severe acute respiratory syndrome coronavirus (SARS-CoV) both have characteristically high homologous sequence, unlike the Middle East Syndrome (MERS)-CoV virus (Yu et al., 2020). The SARS-CoV-2 envelope is associated with four structural proteins: membrane protein (M); spike protein (S); envelope protein (E); and nucleocapsid protein (N) (Lu R. et al., 2020; Figure 1). Details on the structure and molecular biology of the SARS-CoV-2 virus have been recently reviewed by several groups (Arya et al., 2021; Hu et al., 2021; Peng et al., 2021; Rahimi et al., 2021).
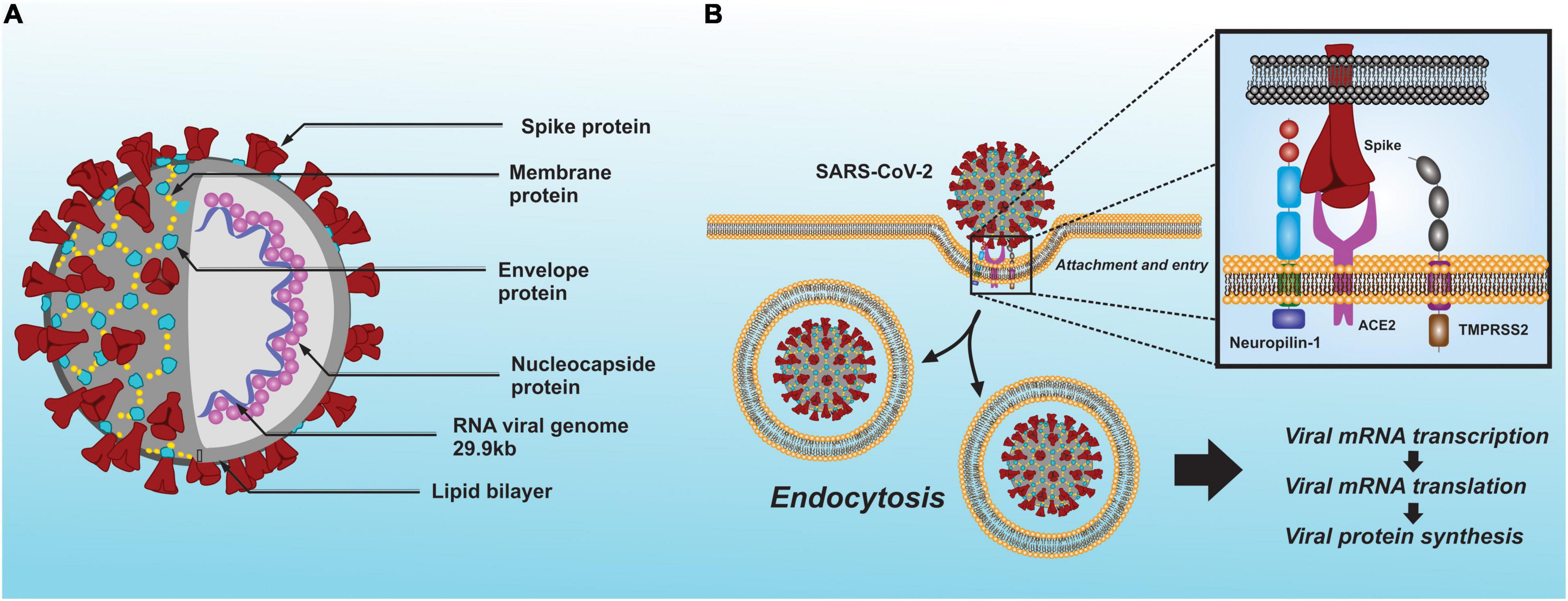
Figure 1. Schematic model of the SARS-CoV-2 whit its structural proteins. (A) The virus envelope is associated with four structural proteins: membrane protein (M); spike protein (S); envelope protein (E); and nucleocapsid protein (N). Moreover, it has a single-stranded positive sense RNA and a genome approximately 29.9 kb in size. (B) To enter a host cell, the SARS-CoV-2 spike protein interacts with the ACE2 and neuropilin-1, while TMPRSS2 to activate its membrane-fusion capacity and subsequent endocytosis.
Analysis of mutations in the coding and non-coding regions, genetic diversity, and pathogenicity of SARS-CoV-2 has also been carried out and based on the results it was suggested that a minimal variation in the genome sequence of SARS-CoV-2 may be responsible for a drastic change in the structures of target proteins, making available drugs ineffective (Naqvi et al., 2020). Clinical data show that the main structures of the body that are affected by COVID-19 are the respiratory and cardiovascular systems (Kapoor, 2020; Lazzeri et al., 2020; Yi et al., 2021). However, SARS-CoV-2 is able to infect other systems, such as the digestive, urogenital and nervous systems (Zhang Y. et al., 2020; Spudich and Nath, 2022). In this review, we discuss the recent reports on the infectivity of SARS-CoV-2 in the central nervous system (CNS) and the probable impact of COVID-19 on neurodegenerative diseases.
Neurological Consequences of COVID-19
There are reports of COVID-19 exerting neurological effects, the most common being hypogeusia (diminished sense of taste) and anosmia (loss of sense of smell) (Gilani et al., 2020; Spudich and Nath, 2022), and cerebrovascular damage (Beyrouti et al., 2020; Meegada et al., 2020; Gilpin et al., 2021). However, encephalopathies (Helms et al., 2020), demyelination (Domingues et al., 2020; Zanin et al., 2020), edema and symptom presentations similar to multiple sclerosis and Guillain-Barré have also been observed with COVID-19 (Toscano et al., 2020; Spudich and Nath, 2022). Other neurological/neuropsychiatric symptoms such as alterations in consciousness and hallucinations in COVID-19 have been attributed to SARS-CoV-2’s effect on the frontal lobe cortex, an area intimately involved in perception (Ellul et al., 2020; Ferrando et al., 2020; Paniz-Mondolfi et al., 2020).
Because COVID-19 is a relatively recent disease, its full pathogenic mechanisms and possible sequelae in the nervous system remain unclear. Fortunately, novel molecular biology techniques, such as RT-PCR, RT-qPCR, CRISPR-based assays, and nucleic acid microarray assays have made it possible to elucidate some general aspects of the disease (Habibzadeh et al., 2021) and relate them to other public health emergencies caused by other coronaviruses, such as SARS in 2002–2003 and MERS in 2012 (Zhu Z. et al., 2020). Researchers have been able to ascertain complications associated with pre-existing conditions and the key role played by the immune system in resolution or further complication of the disease (de Wit et al., 2016; Abdelrahman et al., 2020; Ansariniya et al., 2021). In addition, SARS-CoV-2-induced acute and long-term neurological effects are a subject of intense investigation and a main focus of this article.
Neuroinvasive Mechanisms of Severe Acute Respiratory Syndrome Coronavirus 2
Human coronaviruses not only cause common colds but can also infect neural cells as evidenced by neurotropism and neuroinvasion (Arbour et al., 2000). Studies carried out on brain samples taken from patients with SARS disease detected the presence of the SARS-CoV virus in nervous tissue (Ding et al., 2004; Xu et al., 2005). Moreover, SARS-CoV-2 has been detected in the brain and cerebrospinal fluid of COVID-19 patients using RT-qPCR and immunohistochemistry techniques (Domingues et al., 2020; Huang Y. H. et al., 2020; Moriguchi et al., 2020; Liu J. M. et al., 2021; Serrano et al., 2021; Song et al., 2021). Although the exact mechanism of neurological complications in COVID-19 patients is unknown, it has been shown that infection with SARS-CoV-2 damages the choroid plexus epithelium, leading to leakage across the blood brain barrier (Pellegrini et al., 2020). Nonetheless, potential mechanism (s) of SARS-CoV-2 entry into the CNS are a subject of intense relevance and interest (Hu et al., 2020).
It is known that both SARS-CoV and SARS-CoV-2 occupy the primary receptor angiotensin-converting enzyme 2 (ACE2) (Li et al., 2003; Lu R. et al., 2020) and can form a complex with other cofactors such as transmembrane serine protease 2 (TMPRSS2) (Hoffmann et al., 2020) and neuropilin-1 (Cantuti-Castelvetri et al., 2020; Daly et al., 2020). This interaction between SARS-CoV-2 and ACE2 is essential for the complex to be internalized into the cells (Figure 1). TMPRSS2 is vital for SARS-CoV-2 infection, although it has a low expression in the brain. However, SARS-CoV-2 can also infect cells via neuropilin-1 and furin protease which have a higher and broader expression in the brain compared to TMPRSS2 or ACE2 (Davies et al., 2020). Moreover, SARS-CoV-2 is likely to infect glutamatergic neurons due to higher expression of ACE2, neuropilin-1 and furin protease than GABAergic neurons (Dobrindt et al., 2021). Thus, other proteins could be involved in SARS-CoV-2 entry into the brain. Indeed, a recent study suggests that SARS-CoV-2 may interact with metabotropic glutamate receptor 2 (mGluR2), which may play a role in internalization and perhaps in SARS-CoV-2 neurotropism (Wang J. et al., 2021).
ACE2 is highly expressed in adipose tissue and organs such as the kidney, small intestine, heart, and testicles, and to a lesser extent in the lung, liver, colon, spleen, muscle, blood, and brain (Li et al., 2020). Moreover, a low but constant expression of ACE2 has been revealed via the use of transcriptomic techniques on various brain structures, such as the brainstem, cortex, striatum, hypothalamus, choroid plexuses, and the paraventricular nuclei of the hypothalamus (Xia and Lazartigues, 2008; Chen R. et al., 2020). Given the evidence for the distribution of ACE2 in the brain, it can be inferred that multiple regions may be affected during SARS-CoV-2 infection. Furthermore, SARS-CoV-2 has a higher affinity for ACE2 than SARS-CoV and therefore, could have a major detrimental effect on the brain (Natoli et al., 2020).
The clinical severity of COVID-19 has been correlated with the frequency of neurological complications, while meningitis and encephalitis have been associated with paranasal sinusitis and could, in severe cases of SARS-CoV-2 infection, be an indicator of an aggravated viral infection due to an obstruction in the paranasal lymphatic vessels (Bridwell et al., 2020; Moriguchi et al., 2020). On the other hand, the glymphatic system, a glia-dependent elimination pathway for soluble wastes and metabolic products in the brain, is believed to play an important role in paranasal sinusitis. Serving as the brain’s “front end,” the glymphatic system is interconnected with the lymphatic network of the dura, cranial nerves, and veins of the skull (Benveniste et al., 2019). This interconnection could be used by SARS-CoV-2 to gain access to the brain in order to be internalized by the neurons. A compromised blood-brain barrier (BBB) and the perforation of the ethmoid bone are other suggested routes via which the virus enters the brain (Zubair et al., 2020; Pacheco-Herrero et al., 2021). Overall, three main routes for viral entry to the CNS have been proposed: (1) the transcribrial neuroinvasive route; (2) the neuroinvasive route via the ocular surface; and (3) the hematogenous neuroinvasive route (Figure 2). These proposed routes are discussed in the following sections.
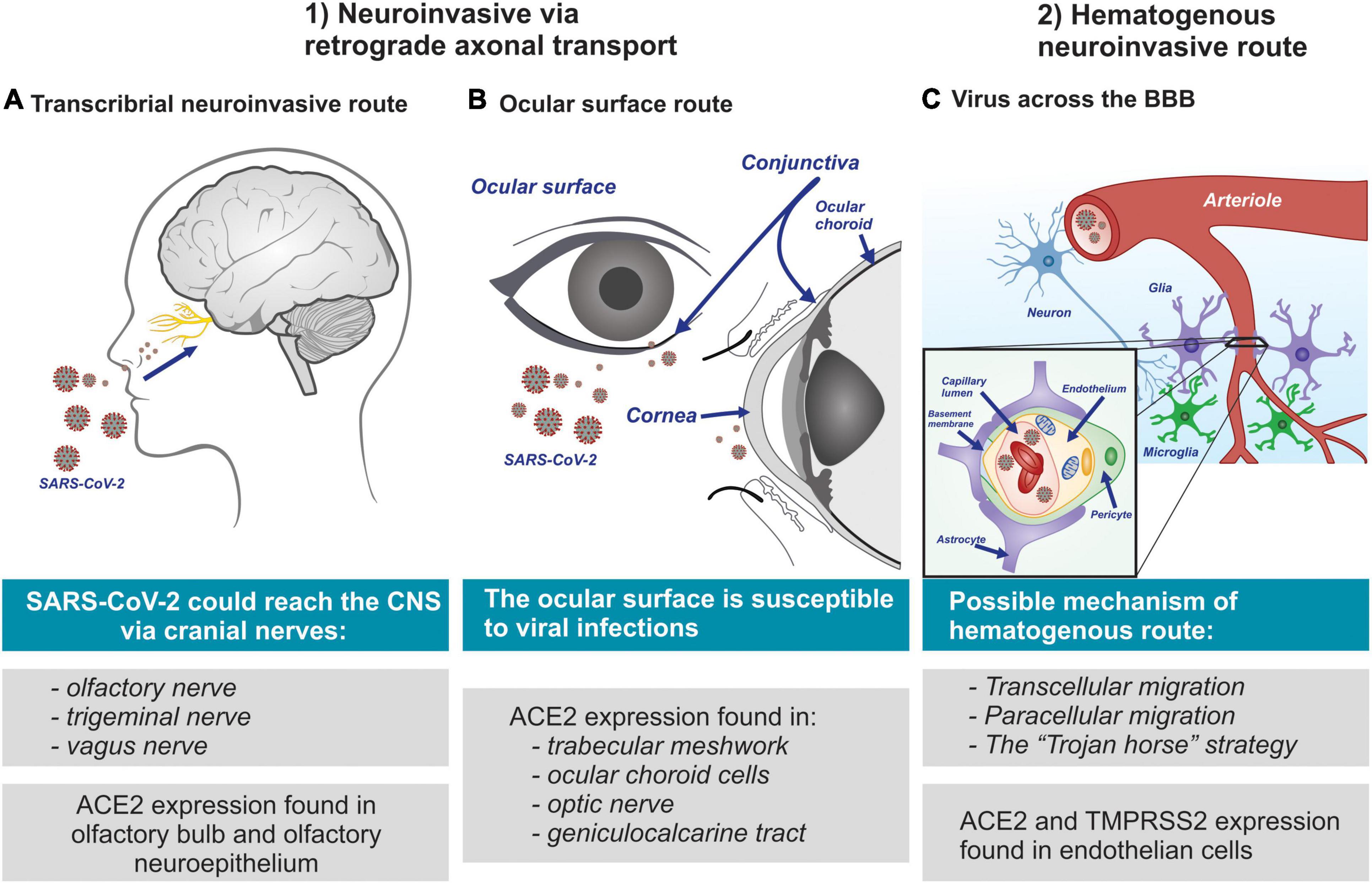
Figure 2. Mechanisms suggested through which SARS-CoV-2 invades the nervous system. (A) Transcribrial neuroinvasive route; SARS-CoV-2 may invade the brain through the olfactory nerve, and via cranial nerves. (B) Route ocular surface; multiple cell types of the visual system are suggested provide as potential entry points for SARS-CoV-2 invasion to the brain. (C) Hematogenous neuroinvasive route; SARS-CoV-2 possibly infects vascular endothelial cells via the ACE2 and TMPRSS2 receptors. Viral particles can reach the brain through the BBB by infecting and replicating inside brain microvascular endothelial cells. Moreover, SARS-CoV-2 infection can cause excessive peripheral immune responses to result in BBB dysfunction via cytokine storm.
Evidence of Neuroinvasive Routes via Retrograde Axonal Transport
It has been proposed that one of the main neuroinvasive pathways into the CNS by SARS-CoV-2, as well as other viruses is via tropism, where a virus can infect a distinct group of cells by binding to the virus receptors on the surface of the host cell (Bauer et al., 2022a). In fact, chronic viral infections can be a risk factor for neurodegenerative diseases. In this regard, potential involvement of Japanese encephalitic virus, the influenza virus, herpes simplex virus type-1 (HSV-1) and the human immunodeficiency virus (HIV) in etiology of Parkinson’s disease and for Alzheimer’s disease have been suggested (De Chiara et al., 2012; Limphaibool et al., 2019; Sait et al., 2021). It is worth noting that HSV-1 infections are common, and that the virus can persist in a latent form within the neurons of its human host. Therefore, viruses may cause damage in vulnerable brain areas leading to neurodegenerative disease (Lotz et al., 2021; Sait et al., 2021).
Transcribrial Neuroinvasive Route of Severe Acute Respiratory Syndrome Coronavirus 2
It is suggested that SARS-CoV-2 reaches the olfactory bulb via infection of the peripheral nerve terminals of the olfactory sensory neurons (Mori, 2015; Desforges et al., 2019; Paniz-Mondolfi et al., 2020; Kumar et al., 2021). This proposed transcribrial route is supported by reports of SARS-CoV-2 patients presenting symptoms of anosmia and dysgeusia (Klopfenstein et al., 2020; Levinson et al., 2020; Mutiawati et al., 2021). It is noteworthy that ACE2 protein is highly expressed in the olfactory bulb (Brann et al., 2020) and the olfactory neuroepithelium (Sungnak et al., 2020; Ziegler et al., 2020). Thus, it is postulated that SARS-CoV-2 forms complexes with TMPRSS2 and neuropilin-1 via interaction with olfactory neuroepithelial ACE2, allowing it to enter into the brain retrogradely via the cranial nerves (Reza-Zaldívar et al., 2020; Messlinger et al., 2021). Indeed, there are reports that when some viruses infect the nociceptive neurons of the nasal cavity, they are able to reach the CNS through the trigeminal nerve (Deatly et al., 1990; Lochhead and Thorne, 2012) and other sensory terminals of the vagus nerve (Driessen et al., 2016). Hence, SARS-CoV-2 could follow the cranial nerves (Bulfamante et al., 2021; Messlinger et al., 2021) from their origin in the nasal cavity to the olfactory nerve, and then to the olfactory bulb, and finally arriving at the brain stem (Bougakov et al., 2021; Figure 2A). This pathway is also followed by the OC43 coronavirus strain (Dubé et al., 2018).
Neuroinvasive Route of Severe Acute Respiratory Syndrome Coronavirus 2 via the Ocular Surface
Recent studies conducted on both humans (Zhou et al., 2020; Collin et al., 2021) and mice (Ma et al., 2020) have demonstrated the expression of ACE2 and TMPRSS2 receptors in ocular surface cells, a region comprising the epithelial cells of the cornea and conjunctiva. Furthermore, a remarkable level of ubiquity of ACE2 receptor expression was recently found in the trabecular meshwork and ocular choroid cells of the outer eye, the optic nerve, and optic radiation or geniculocalcarine tract to the occipital cortex, which suggests that multiple cell types of the visual system provide potential entry points for SARS-CoV-2 invasion (Hill et al., 2021). However, as ACE2 expression has been observed more in the conjunctiva than in the cornea, SARS-CoV-2 has greater neuroinvasive potential via the conjunctiva (Leonardi et al., 2020; Ma et al., 2020). For the ocular surface to be considered a SARS-CoV-2 infection route, TMPRSS2 must be co-expressed along with ACE2, as TMPRSS2 acts as a cofactor in internalization of the complex (de Freitas Santoro et al., 2021). It should be noted that TMPRSS2 expression has been observed in both the cornea and the conjunctiva (Leonardi et al., 2020; Collin et al., 2021). However, the likelihood of the ocular surface being an infection gateway is low despite the potential of SARS-CoV-2 causing conjunctivitis and other ocular discomfort (Chen X. et al., 2021). However, the actual conjunctival transmission of SARS-CoV-2 is yet to be confirmed (de Freitas Santoro et al., 2021).
The ocular surface is susceptible to viral infections by means of aerosols or direct contact with fomites resulting from exposure to external contaminants and is vulnerable to a higher level of exposure than the oral cavity or the nostrils (Coroneo, 2021; Figure 2B). In addition, clinical cases where conjunctivitis was the initial symptomatic manifestation in COVID-19 positive patients have been reported and confirmed via both nasopharyngeal swab samples and PCR test which detected the presence of SARS-CoV-2 RNA in tears (Zhang X. et al., 2020; Hassan et al., 2021). Nonetheless, confirmation of the conjunctival transmission of SARS-CoV-2 into the CNS requires further investigation.
Evidence of a Hematogenous Neuroinvasive Route of Entry for Severe Acute Respiratory Syndrome Coronavirus 2 to the Brain
The BBB tightly regulates the movement of molecules, ions, and cells between blood and the CNS and prevents the neurotoxic components of plasma, blood cells, and pathogens from entering the brain (Montagne et al., 2017). This regulatory characteristic is attributed to the arteries, arterioles, and capillaries that supply blood to the brain and that act in response to neuronal stimuli that trigger an increased rate of neurovascular coupling, a mechanism generated by the cerebral blood flow and the supply of oxygen. The neurovascular unit is made up of the following structural components: vascular cells (endothelium and wall cells, pericytes, and smooth muscle cells); glia (astrocytes and microglia); and neurons (Kisler et al., 2017; Sweeney et al., 2019; Sanchez-Cano et al., 2021). The regulation conducted by the BBB provides strict control over the cellular permeability of neuronal tissues, which is essential for proper neuronal function and which, furthermore, requires precise ionic concentrations in the surrounding environment (Daneman, 2012; Daneman and Prat, 2015). Therefore, the loss of homeostatic regulation and deterioration in the restrictive capacity of the BBB play important roles in the progression of neurological conditions such as brain trauma, and infectious and neurodegenerative diseases (Sanchez-Cano et al., 2021).
It is of particular interest to note that the ACE2 and TMPRSS2 receptors are expressed in the endothelial cells of the BBB (Chen R. et al., 2020; Qiao et al., 2020; Torices et al., 2021). Due to these findings and the interaction of the virus with the protein complex discussed earlier in this paper, it has been suggested that SARS-CoV-2 could reach the brain via systemic circulation by crossing the BBB and damage the choroid plexus (Baig, 2020; Pellegrini et al., 2020). The actual hematogenous mechanism by which SARS-CoV-2 gains entry to brain is not known. However, several mechanisms have been suggested (Achar and Ghosh, 2020; Pellegrini et al., 2020). These include: (a) transcellular migration, where the virus invades the host’s endothelial cells and is able to cross the BBB; (b) paracellular migration, where the virus invades the choroid plexus of the fenestrated endothelial cells and gets into the brain; and (c) the “Trojan horse” strategy, where the virus is internalized by phagocytic immune cells such as neutrophils and macrophages, and is subsequently replicated in the brain (Dahm et al., 2016; Figure 2C). Moreover, is likely that SARS-CoV-2 invades the brain by damaging the integral architecture of the BBB (Varghese et al., 2020). Thus, SARS-CoV-2 can get access into the brain by one or a combination of the above mechanisms.
Evidence of Neuroinvasive Mechanisms of Severe Acute Respiratory Syndrome Coronavirus 2 in Animal Models
Both the symptoms presented by infected patients and the findings of clinical pathology provide evidence of possible infection of the CNS by SARS-CoV-2. However, to further explore the viral pathogenesis in the host and characterize the mechanisms of viral access and dissemination in the CNS, a translational neuroscience approach (Johansen et al., 2020; Sanclemente-Alaman et al., 2020), similar to that employed in the early research conducted on SARS and MERS is necessary (Callaway, 2020; Natoli et al., 2020).
Because SARS-CoV-2 has a higher affinity for the human ACE2 receptor (hACE2) than animal ACE2, few studies have been carried out in animal models to determine the neuroinvasive pathways of the virus (Wan et al., 2020). In addition, hACE2 is structurally different from that in animal species. Hence, a significantly lower level of tropism is noted in animal vs. human tissue, particularly in relation to CNS (Natoli et al., 2020). Indeed, Brann et al. (2020) demonstrated that the olfactory sensory neurons of the whole olfactory mucosa of mice, unlike olfactory epithelial support cells, stem cells, and the cells of the nasal respiratory epithelium, do not express ACE2 and TMPRSS2 genes. Thus, it is argued that based off of animal studies, anosmia or other forms of olfactory dysfunction may not support olfactory bulb as an entry route for SARS-CoVs into the CNS (Brann et al., 2020; Natoli et al., 2020).
To overcome the discrepancy between animal and human studies, several animal models with closer resemblance to that of humans have been suggested. One suggestion is to develop humanized mouse models that express the hACE2 receptor (Sun et al., 2020), as the murine is the most widely used animal model for this purpose (Muñoz-Fontela et al., 2020). Another suggestion is to modify the SARS-CoV-2 spike to effectively bind with murine-ACE2 (Dinnon et al., 2020), however, this strategy is risky, since modifying the viruses can create a natural reservoir for a virus that might be completely different from the wild-type version. A third suggestion is to induce mice to be susceptible to SARS-CoV-2 infection by sensitizing the respiratory tract to the virus. The latter may be achieved via transduction with adenovirus or associated viruses that express hACE2 (Ad5-hACE2 or AAV-hACE2, respectively) (Israelow et al., 2020; Rathnasinghe et al., 2020). In all these suggestions, however, as mentioned earlier, it must be borne in mind that the co-expression of the ACE2 and TMPRSS2 receptors is necessary for the virus to be internalized.
It was recently demonstrated that neuroinvasion by SARS-CoV-2 could be achieved in an animal model where hACE2 was overexpressed by means of an adeno-associated virus infection (Song et al., 2021). Moreover, the neuronal infection could be prevented by blocking ACE2 with neutralizing antibodies or administering cerebrospinal fluid obtained from a COVID-19 patient, where presumably antibodies were present (Song et al., 2021). A recent study reported differences in the neuroinvasiveness and neurovirulence among the most relevant SARS-CoV-2 variants, D614G, Delta (B.1.617.2), and Omicron (B.1.1.529) 5 days post inoculation in a hamster model. The results showed that D614G variant had a high neuroinvasion via the olfactory nerve compared to the Delta and Omicron variants (Bauer et al., 2022b). While the results obtained provide evidence for the neuroinvasive capacity of SARS-CoV-2 in an animal model, the sequence of infection in different CNS cell types has not yet been determined. Therefore, more studies on detailed mechanism (s) of SARS-CoV-2 infection of the CNS are needed.
Neuropathological Features of Severe Acute Respiratory Syndrome Coronavirus 2
SARS-CoV-2 has been reported to manifest neurological symptoms that range from mild to fatal, while it can also occur asymptomatically in patients. Clinical studies conducted on patients hospitalized with COVID-19 report a level of neurological manifestation ranging from 15.2% (Flores-Silva et al., 2021), or 36.4% (Mao et al., 2020) to 54.7% (Romero-Sánchez et al., 2020), and up to 88% (García-Moncó et al., 2021). It should be noted that the frequency of neurological alterations observed in patients with COVID-19 depends on whether they have been evaluated by a neurologist and/or inclusion of patients with a history of neurological complication.
The most common early neurological manifestations in patients with COVID-19 are headache, dizziness, nausea, vomiting, myalgia, and neuralgia (Guan et al., 2020; Mao et al., 2020; Wang et al., 2020; Song et al., 2021). Anosmia and dysgeusia develop in the early stages of infection and are more frequent in less severe cases (Mao et al., 2020; Flores-Silva et al., 2021; García-Moncó et al., 2021). Late-infection neurological manifestations include acute cerebrovascular disease, meningoencephalitis, impaired consciousness, and skeletal muscle injury (Al Saiegh et al., 2020; Guan et al., 2020; Guidon and Amato, 2020; Parikh et al., 2020). Less-frequently reported symptoms include dysautonomia, seizures, movement disorders, Guillain Barré syndrome, Miller Fisher syndrome, and optic neuritis (Gutiérrez-Ortiz et al., 2020; Hwang et al., 2020; Manji et al., 2020). In addition, the University College London Queen Square Institute of Neurology has reported five categories of clinical presentations at a neurological level: (1) encephalopathy with delirium/psychosis and no magnetic resonance imaging or cerebrospinal fluid abnormalities; (2) inflammatory CNS syndromes including encephalitis and acute disseminated encephalomyelitis; (3) ischemic strokes; (4) peripheral neurological disorders including Guillain–Barré syndrome and brachial plexopathy; and (5) miscellaneous central nervous disorders (Paterson et al., 2020; Gasmi et al., 2021). Thus, neurological manifestations are variable and not uncommon in COVID-19 patients. Moreover, both morphological and biochemical pathological changes may be manifested as detailed below.
Morphological Changes
Research on the clinical and imaging aspects of COVID-19 infection as well as molecular biology studies conducted on both in vitro and in vivo models have provided valuable information in understanding the etiological mechanisms of SARS-CoV-2. However, despite the large amount of information available on the disease, there is little work conducted to characterize its pathological manifestations in the tissues of different systems of the body (Al Nemer, 2020; Skok et al., 2021; Table 1). Studies carried out on the anatomical brain pathology during autopsy reveal morphological alterations in the frontal and occipital lobes, olfactory bulb, cingulate gyrus, corpus callosum, hippocampus, basal ganglia, thalamus, cerebellum, midbrain, middle pons, medulla, brainstem, and the lateral ventricles (Barone et al., 2021; Caramaschi et al., 2021; Generoso et al., 2021). The most common gross findings are edema (Reichard et al., 2020), hemorrhagic lesions (Paniz-Mondolfi et al., 2020; Reichard et al., 2020), hydrocephalus (Lacy et al., 2020), atrophy and low brain mass (Lax et al., 2020; Wichmann et al., 2020), olfactory bulb asymmetry (Coolen et al., 2020), and infarcts (Solomon et al., 2020). SARS-CoV-2 has also been found to cause lesions and alterations in neuronal structures, while neuronal infection can cause encephalitis and the generation of lethal microthrombi (Bradley et al., 2020; von Weyhern et al., 2020). In addition, severe COVID-19 infection accompanied by multisystem inflammatory syndrome may cause fibrotic lesions and generate cerebral thrombosis (Turski et al., 2020).
Various studies have been carried out to ascertain the structural modifications in the brains of COVID-19 patients. Magnetic resonance techniques have shown a bilateral obliteration of the olfactory cleft in 50% of SARS-CoV-2-positive patients as well as a sudden loss of smell and subtle olfactory bulb asymmetry in 25% of the sampled patients (Niesen et al., 2021). Another study demonstrated microstructural changes using diffusion tensor imaging (DTI) and 3D high-resolution T1WI sequences in COVID-19 patients, where greater volume of bilateral gray matter (reported as gray matter volume) was observed in the hippocampus, olfactory cortices, insula, left rolandic operculum, left Heschl gyrus, and right cingulate gyrus (Lu Y. et al., 2020). These findings demonstrate possible alterations in the structural and functional integrity of brain microstructures in susceptible patients, and also suggest potential long-term consequences of SARS-CoV-2 infection, which may lead to or accelerate a variety of neurodegenerative diseases (ElBini Dhouib, 2021), discussed further below.
Molecular and Biochemical Changes
Given that most cases of SARS-CoV-2 infection present mild or moderate symptoms and that a group with severe infections develops multiple systemic dysfunctions as a consequence of imbalances in the immune and the oxidation-reduction systems, it is not surprising that inflammatory states and oxidative stress are commonly indicated in pathogenesis of COVID-19 (Mrityunjaya et al., 2020; Forcados et al., 2021). In addition, hyperactivation of the immune system leading to an exaggerated release of pro-inflammatory cytokines referred to as “cytokine storm” is not only associated with severe complications, but also poorer outcome (Chen G. et al., 2020; Noroozi et al., 2020; Tan et al., 2021; Yang et al., 2021).
Both the innate and adaptive immune systems have been widely described as working together, with the innate response representing the host’s first line of defense and the adaptive response becoming prominent several days after infection, when T and B cells have undergone clonal expansion (Strbo et al., 2014). Furthermore, the components of the innate system contribute to the activation of antigen-specific cells, which amplify their responses in order to achieve complete control over the pathogen by recruiting innate effector mechanisms. Therefore, the innate and adaptive responses are fundamentally different, although the synergy between them is essential for an effective immune response (Chaplin, 2010). Patients with severe SARS-CoV-2 infection manifest an increased innate immune response and a suppressed adaptive immunity, which is why a delayed elimination of the virus from the organism is observed (Pan Y. et al., 2021). This scenario aggravates the immune status of the patient as it increases the levels of various inflammatory factors and increases both the number and activation of immune cells at the site of the inflammation, a process from which cytokine storm originates (Catanzaro et al., 2020; Chen R. et al., 2021).
Neurons infected by SARS-CoV-2 release inflammatory mediators that are capable of activating adjacent cells such as glia, microglia, mast cells and endothelial cells, conditions which constitute the beginning of neuroinflammation (Almutairi et al., 2021; Frank et al., 2022). Elevated immunological mediators during SARS-CoV-2 infection include: tumor necrosis factor α (TNF-α); interferon gamma (INFγ); a series of chemokines such as CCL-2, CCL-5, and CXCL-10; a series of interleukins such as IL-1β, IL-6, IL-8, IL-12, IL-17, IL-18, and IL-33; and granulocyte macrophage colony stimulating factor (GM-CSF) (Kempuraj et al., 2020; Mehta and Fajgenbaum, 2021; Tripathy et al., 2021). SARS-CoV-2 infection induces the down-regulation of ACE2, disrupting the physiological balance between ACE/ACE2 and angiotensin II (Ang-II)/angiotensin and leading to severe multiple organ injury (Mehrabadi et al., 2021). In fact, it has been suggested that ACE2 downregulation may contribute to the pathogenesis of lung injury in COVID-19 (Ni et al., 2020). Angiotensin II stimulates gene expression of multiple inflammatory cytokines such as TNF-α and IL-6. TNF-α, in particular, induces macrophage differentiation of a pro-inflammatory phenotype, which exerts an antimicrobial effect. However, such differentiated macrophages are also responsible for recruiting more cell types via cytokine secretion, thus exacerbating the inflammatory response. Similarity, IL-6, essential for T cell differentiation, when elevated, signals a poorer SARS-CoV-2 prognosis (Banu et al., 2020; Patra et al., 2020; Ben Moftah and Eswayah, 2022).
Additionally, the pro-inflammatory factors discussed above are able to cross the BBB, increase vascular permeability, and trigger further release of pro-inflammatory cytokines from the microglia (da Fonseca et al., 2014; Zhang et al., 2021). This cascade results in increased apoptotic activity, increased levels of reactive oxygen species (ROS), mitochondrial dysfunction and eventual neurodegeneration (Chaudhry et al., 2020; Chiricosta et al., 2021; Kumar et al., 2021). Moreover, the high concentration of pro-inflammatory cytokines can lead to the activation of the coagulation cascade, suppression of anticoagulant factors, and hence increase the chance of thrombosis (Al Saiegh et al., 2020; Levi et al., 2020). An opposite scenario may also manifest itself where an increase in fibrinolytic activity leading to an increase in the level of fibrin degradation and hemorrhagic conditions including aneurysms may be observed in certain patients infected with SARS-CoV-2 (Al Saiegh et al., 2020).
Severe Acute Respiratory Syndrome Coronavirus 2 and Neurodegenerative Disease
The link between systemic and central inflammation, as well as between neurological and neuropsychiatric diseases, is well known (Hurley and Tizabi, 2013; Schwartz and Deczkowska, 2016; Skaper et al., 2018). In nervous tissue, the increased levels of inflammatory mediators and glial cell activity caused by SARS-CoV-2 infection may pose an increased risk of neurodegenerative disease such as Alzheimer’s disease (AD), Parkinson’s disease (PD) as well as multiple sclerosis (MS), stroke and neurological trauma (Mohammadi et al., 2020; Li et al., 2021; Tekiela and Majersik, 2021). SARS-CoV-2 infection in people with senility is likely to increase the neuropathological intensity and contribute to the worsening of motor and cognitive deficits (Wang Y. et al., 2021; Yu et al., 2021). Indeed, one of the main risk factors for both COVID-19 and neurodegenerative disease is age (Ferini-Strambi and Salsone, 2021; Figure 3). Older people comprise the section of the population most prone to developing neurodegenerative diseases (Hou et al., 2019) and present with more severe clinical reaction to COVID-19 (Lebrasseur et al., 2021). In addition, lifestyle and preexisting conditions such as trauma, infection, metabolic disease, and stress can accelerate the onset and progression of neurodegenerative diseases (Graham and Sharp, 2019; Madore et al., 2020; Lotz et al., 2021).
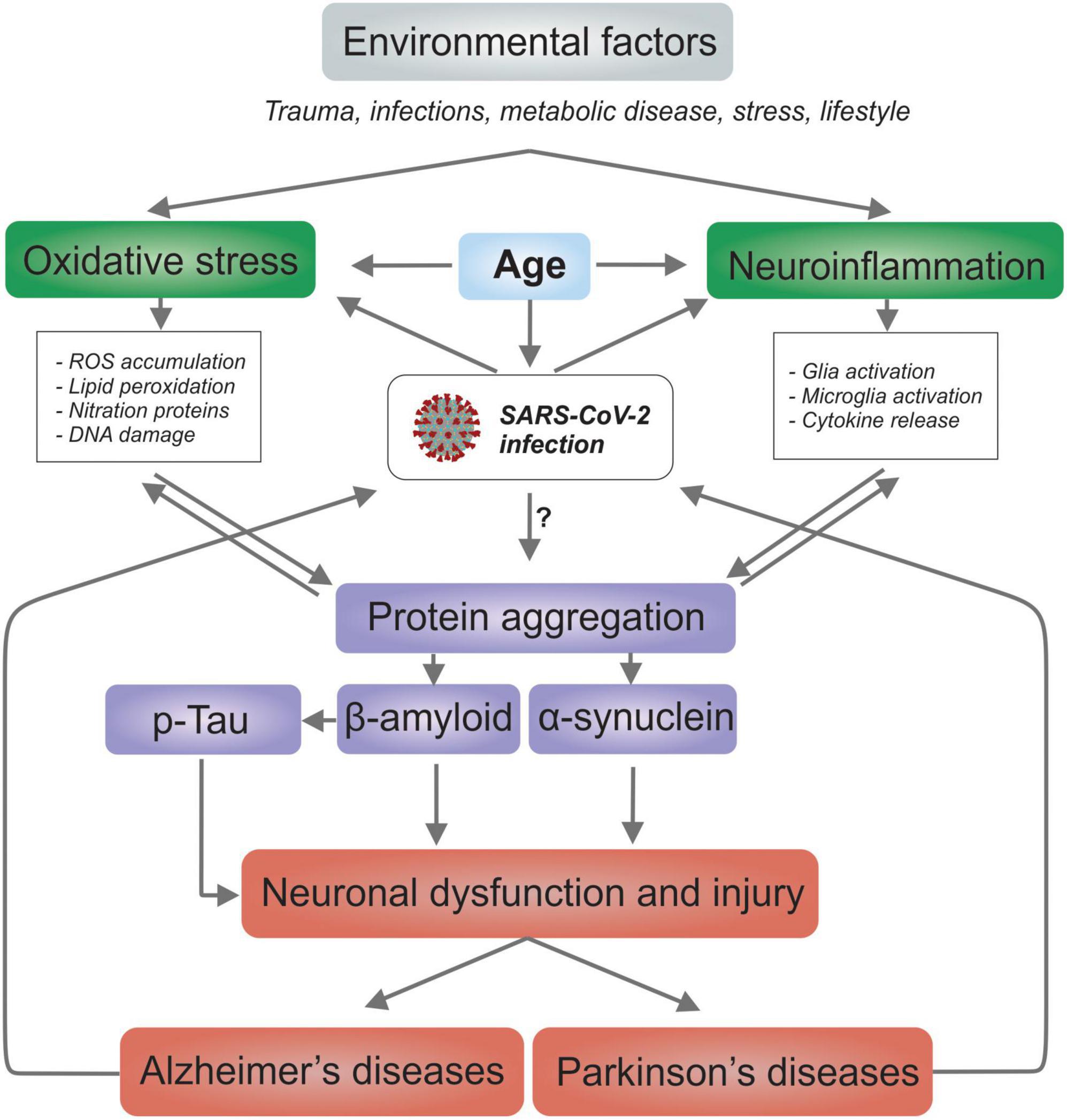
Figure 3. SARS-CoV-2 infection and possible links for both Alzheimer’s and Parkinson’s disease. The common risk factors between COVID-19 and neurodegenerative diseases is the age; the oxidative stress; and, neuroinflammation. It’s possible that COVID-19 patients are more susceptible to developing neurodegenerative diseases and that patients with neurodegenerative diseases are more susceptible to contracting COVID-19.
It is important to note that protein aggregation in the brain is considered as one of the main reasons behind neurodegeneration. Protein aggregation has been observed for tau protein and Aβ peptide in AD; and α-synuclein for PD. The aggregation process spreads from one cell to another, and the aggregates and deposits formed impair brain function. It is unclear whether viral infections directly or indirectly cause neurodegeneration (Liu S. et al., 2021). However, it has been suggested that viruses can initiate pathological protein aggregation via a direct mechanism in Aβ peptide aggregation in vitro and in animal models infected with HSV-1 and respiratory syncytial virus (Ezzat et al., 2019). A recent study has shown direct interactions between the N-protein of SARS-CoV-2 and α-synuclein as the molecular basis for the observed correlation between SARS-CoV-2 infections and parkinsonism (Semerdzhiev et al., 2022). Given that cell-surface glycans function as initial, usually low-affinity attachment factors, these receptors play key roles in SARS-CoV-2 infection (Koehler et al., 2020; Prasad et al., 2021). Moreover, the ability of S1 protein of SARS-CoV-2 to interact with heparin receptor can lead to many misfolded brain proteins including amyloid complex and ultimately lead to neurodegeneration (Tavassoly et al., 2020; Idrees and Kumar, 2021).
Alzheimer’s Disease
One of the main causes of disability among older people around the world is dementia, with AD as the most common form (Alzheimer’s-Association, 2020). The mechanisms involved in the pathogenesis of AD are complex and not fully understood. However, the most accepted hypothesis involves molecular changes such as extracellular deposition of the β-amyloid protein and intracellular phosphorylation of the tau protein, causing the formation of amyloid plaques and neurofibrillary tangles, respectively (Liu et al., 2019). In addition, intense neuroinflammation, oxidative stress, mitochondrial dysfunction, and protein misfolding have also been implicated (Gandhi et al., 2019; Perez Ortiz and Swerdlow, 2019; Leng and Edison, 2021; Figure 3). Patients with AD are more susceptible to contracting COVID-19 (Finelli, 2021; Pan A. P. et al., 2021; Wang Q. et al., 2021), and COVID-19 patients are more susceptible to developing AD (Brown et al., 2020; Chiricosta et al., 2021; Villa et al., 2022). This might not be surprising due to the presence of common risk factors such as age, cardiovascular disease, metabolic, and psychological disorders between the two diseases (Brown et al., 2020; Finelli, 2021). Generally, inflammation increases with age, wherein higher levels of pro-inflammatory cytokines have been quantified in older people (Niraula et al., 2017; Rea et al., 2018). Furthermore, given that infection with a wide variety of pathogens is suspected to be a risk factor for the onset of AD (Seaks and Wilcock, 2020; Vigasova et al., 2021), an increased risk of developing AD and cognitive impairment in susceptible populations after SARS-CoV-2 infection would also be expected. Moreover, these patients often face social stigma and mental stress, which can further aggravate neuroinflammatory processes and result in psychiatric disorders (Justice, 2018; Milligan Armstrong et al., 2021). The presence of pathogens and other factors such as age, alcohol and tobacco consumption, cerebral hypoxia, metabolic diseases, pollution, sedentary lifestyle, or sleep disorders may cause BBB malfunction (Noe et al., 2020; Hussain et al., 2021), and hence lead to the infiltration of neurotoxic proteins such as the β-amyloid peptide (Wang D. et al., 2021). SARS-CoV-2 infection accompanied by a local immune response incorporating astrocytes and microglia could generate a state of neuroinflammation in susceptible patients that can manifest for a long term (Kumar et al., 2021; Villa et al., 2022). Such a scenario would be expected to exacerbate the current pathology in AD patients.
Genetically, apolipoprotein E ε4 allele (APOE4) has been determined the strongest risk factor for AD (Serrano-Pozo et al., 2021). Furthermore, APOE4 has been associate with increased susceptibility to SARS-CoV-2 infection and COVID-19 (Kurki et al., 2021). Cerebral microvasculature complications may be the basis of neurological issues in hospitalized COVID-19 patients (Miners et al., 2020; Lee et al., 2021). Interestingly APOE4 is also involved in BBB dysfunction and cerebrovascular diseases (Montagne et al., 2021). However, further research is needed to determine the exact role of APOE4 in COVID-19-induced AD.
Parkinson’s Disease
Similar to AD, PD is related to COVID-19, sharing risk factors such as advanced age, cardiovascular and metabolic diseases (Park et al., 2020; Fearon and Fasano, 2021; Sharifi et al., 2021). Moreover, patients with PD may be immunosuppressed, which makes them more susceptible to infections of any type including SARS-CoV-2 (Tansey and Romero-Ramos, 2019; Prasad et al., 2020).
Histologically, PD is characterized by the loss of dopaminergic neurons in the substantia nigra pars compacta (SNpc) and cytoplasmic inclusions, mainly composed of α-synuclein aggregates called Lewy bodies (Henderson et al., 2019; Figure 3). Although the precise etiology of PD is not well known, some hypotheses for its pathogenesis and development point to oxidative stress, neuroinflammation, mitochondrial dysfunction, synaptic pathogenesis, and also as a result of infection (Hurley and Tizabi, 2013; Meng et al., 2019). As patients in an advanced stage of PD have difficulties in chewing and swallowing, they commonly experience salivary accumulation and aspiration (Kwon and Lee, 2019). In addition, the stiffness of the chest wall common in PD inhibits the cough reflex, forming a favorable environment for SARS-CoV-2 infection. In the most severe cases, SARS-CoV-2 infection progresses to pneumonia, which is one of the leading causes of death in PD patients (Bhidayasiri et al., 2020). It is already well known that various viral infections can accentuate the pathological sequelae of PD (Jang et al., 2009).
Recently, a study demonstrated the ability of the H1N1 influenza virus to block protein degradation pathways and promoting the formation of α-synuclein aggregates in dopaminergic neurons in vitro (Marreiros et al., 2020), and a more recent study provided similar results in an in vivo model (Bantle et al., 2021). Furthermore, increased amounts of phosphorylated α-synuclein, activation of microglia and astrocytes, and selective loss of dopaminergic neurons in the SNpc with behavioral and motor consequences have been observed as secondary consequences of infection with the western equine encephalitis virus (WEEV) (Bantle et al., 2019). Thus, it is not unreasonable to expect similar consequences with SARS-CoV-2 infection (Chaná-Cuevas et al., 2020). Moreover, COVID-19 pandemic causing at least partial confinement and social distancing may not only limit the mobility but may also aggravate depression, mental stress, and loneliness in PD patients. It is noteworthy that there exists a substantial co-morbid condition of PD and depression (Tizabi et al., 2019, 2021).
Immediate Prospects
In this article, we have reviewed the possible routes by means of which SARS-Cov-2 may enter the CNS, including transcribrial or the ocular surface, and the hematogenous neuroinvasive pathways. The increased occurrence of neuroinflammation, oxidative stress, and cytokine storm caused by the virus also increases the level of cellular damage in the CNS, thus increasing the risk of developing neurodegenerative diseases such as AD and PD. Further studies on effects of SARS-CoV-2 and its variants on protein aggregation, glia or microglia activation, BBB damage, oxidative stress, and neuroinflammation is warranted.
Author Contributions
JS, FP, AP-M, and IL designed the sections and contents of the review article. IL and FP oversaw the organization to distribute the writing tasks among the authors and participated in article writing. FP made all the figures. JS, FP, AP-M, GS-L, LC, YT, and IL performed literature search and participated in the article writing. All authors critically reviewed and approved the final version of the article.
Funding
This study was supported by grants from the BUAP Vicerrectoría de Investigación y Estudios de Posgrado (Vice-rectory for Research and Postgraduate Study) 2021–2022, awarded to IL, while the support provided via CONACYT-Mexico grant (732793) was awarded to FP.
Conflict of Interest
The authors declare that the research was conducted in the absence of any commercial or financial relationships that could be construed as a potential conflict of interest.
Publisher’s Note
All claims expressed in this article are solely those of the authors and do not necessarily represent those of their affiliated organizations, or those of the publisher, the editors and the reviewers. Any product that may be evaluated in this article, or claim that may be made by its manufacturer, is not guaranteed or endorsed by the publisher.
References
Abdelrahman, Z., Li, M., and Wang, X. (2020). Comparative review of SARS-CoV-2. SARS-CoV, MERS-CoV, and influenza a respiratory viruses. Front. Immunol. 11:552909. doi: 10.3389/fimmu.2020.552909
Achar, A., and Ghosh, C. (2020). COVID-19-associated neurological disorders: the potential route of CNS invasion and blood-brain relevance. Cells 9:2360. doi: 10.3390/cells9112360
Al Nemer, A. (2020). Histopathologic and autopsy findings in patients diagnosed with coronavirus disease 2019 (COVID-19): what we know so far based on correlation with clinical, morphologic and pathobiological aspects. Adv. Anat. Pathol. 27, 363–370. doi: 10.1097/PAP.0000000000000276
Al Saiegh, F., Ghosh, R., Leibold, A., Avery, M. B., Schmidt, R. F., Theofanis, T., et al. (2020). Status of SARS-CoV-2 in cerebrospinal fluid of patients with COVID-19 and stroke. J. Neurol. Neurosurg. Psychiatry 91, 846–848. doi: 10.1136/jnnp-2020-323522
Almutairi, M. M., Sivandzade, F., Albekairi, T. H., Alqahtani, F., and Cucullo, L. (2021). Neuroinflammation and its impact on the pathogenesis of COVID-19. Front. Med. 8:745789. doi: 10.3389/fmed.2021.745789
Alzheimer’s-Association (2020). 2020 Alzheimer’s disease facts and figures. Alzheimers Dement 16, 391–460. doi: 10.1002/alz.12068
Ansariniya, H., Seifati, S. M., Zaker, E., and Zare, F. (2021). Comparison of immune response between SARS, MERS, and COVID-19 infection, perspective on vaccine design and development. Biomed. Res. Int. 2021:8870425. doi: 10.1155/2021/8870425
Arbour, N., Day, R., Newcombe, J., and Talbot, P. J. (2000). Neuroinvasion by human respiratory coronaviruses. J. Virol. 74, 8913–8921. doi: 10.1128/jvi.74.19.8913-8921.2000
Arya, R., Kumari, S., Pandey, B., Mistry, H., Bihani, S. C., Das, A., et al. (2021). Structural insights into SARS-CoV-2 proteins. J. Mol. Biol. 433:166725. doi: 10.1016/j.jmb.2020.11.024
Baig, A. M. (2020). Neurological manifestations in COVID-19 caused by SARS-CoV-2. CNS Neurosci. Ther. 26, 499–501. doi: 10.1111/cns.13372
Bantle, C. M., French, C. T., Cummings, J. E., Sadasivan, S., Tran, K., Slayden, R. A., et al. (2021). Manganese exposure in juvenile C57BL/6 mice increases glial inflammatory responses in the substantia nigra following infection with H1N1 influenza virus. PLoS One 16:e0245171. doi: 10.1371/journal.pone.0245171
Bantle, C. M., Phillips, A. T., Smeyne, R. J., Rocha, S. M., Olson, K. E., and Tjalkens, R. B. (2019). Infection with mosquito-borne alphavirus induces selective loss of dopaminergic neurons, neuroinflammation and widespread protein aggregation. NPJ Parkinsons Dis. 5:20. doi: 10.1038/s41531-019-0090-8
Banu, N., Panikar, S. S., Leal, L. R., and Leal, A. R. (2020). Protective role of ACE2 and its downregulation in SARS-CoV-2 infection leading to macrophage activation syndrome: therapeutic implications. Life Sci. 256:117905. doi: 10.1016/j.lfs.2020.117905
Barone, R., Marino Gammazza, A., Paladino, L., Pitruzzella, A., Spinoso, G., Salerno, M., et al. (2021). Morphological alterations and stress protein variations in lung biopsies obtained from autopsies of COVID-19 subjects. Cells 10:3136. doi: 10.3390/cells10113136
Bauer, L., Laksono, B. M., de Vrij, F. M. S., Kushner, S. A., Harschnitz, O., and van Riel, D. (2022a). The neuroinvasiveness, neurotropism, and neurovirulence of SARS-CoV-2. Trends Neurosci. 45:6. doi: 10.1016/j.tins.2022.02.006
Bauer, L., Rissmann, M., Benavides, F. F. W., Lonneke, L., Leijten, L., Begeman, L., et al. (2022b). Differences in neuroinflammation in the olfactory bulb between D614G, Delta and Omicron BA.1 SARS-CoV-2 variants in the hamster model. biorxiv [Preprint]. doi: 10.1101/2022.03.24.485596
Ben Moftah, M., and Eswayah, A. (2022). Intricate relationship between SARS-CoV-2-induced shedding and cytokine storm generation: a signaling inflammatory pathway augmenting COVID-19. Health Sci. Rev. 2:100011. doi: 10.1016/j.hsr.2021.100011
Benveniste, H., Liu, X., Koundal, S., Sanggaard, S., Lee, H., and Wardlaw, J. (2019). The glymphatic system and waste clearance with brain aging: a review. Gerontology 65, 106–119. doi: 10.1159/000490349
Beyrouti, R., Adams, M. E., Benjamin, L., Cohen, H., Farmer, S. F., Goh, Y. Y., et al. (2020). Characteristics of ischaemic stroke associated with COVID-19. J. Neurol. Neurosurg. Psychiatry 91, 889–891. doi: 10.1136/jnnp-2020-323586
Bhidayasiri, R., Virameteekul, S., Kim, J. M., Pal, P. K., and Chung, S. J. (2020). COVID-19: an early review of its global impact and considerations for parkinson’s disease patient care. J. Mov. Disord. 13, 105–114. doi: 10.14802/jmd.20042
Bougakov, D., Podell, K., and Goldberg, E. (2021). Multiple neuroinvasive pathways in COVID-19. Mol. Neurobiol. 58, 564–575. doi: 10.1007/s12035-020-02152-5
Bradley, B. T., Maioli, H., Johnston, R., Chaudhry, I., Fink, S. L., Xu, H., et al. (2020). Histopathology and ultrastructural findings of fatal COVID-19 infections in Washington State: a case series. Lancet 396, 320–332. doi: 10.1016/S0140-6736(20)31305-2
Brann, D. H., Tsukahara, T., Weinreb, C., Lipovsek, M., Van den Berge, K., Gong, B., et al. (2020). Non-neuronal expression of SARS-CoV-2 entry genes in the olfactory system suggests mechanisms underlying COVID-19-associated anosmia. Sci. Adv. 6:eabc5801. doi: 10.1126/sciadv.abc5801
Bridwell, R., Long, B., and Gottlieb, M. (2020). Neurologic complications of COVID-19. Am. J. Emerg. Med. 38, e1549e3–e1549e7. doi: 10.1016/j.ajem.2020.05.024
Brown, E. E., Kumar, S., Rajji, T. K., Pollock, B. G., and Mulsant, B. H. (2020). Anticipating and mitigating the impact of the COVID-19 pandemic on alzheimer’s disease and related dementias. Am. J. Geriatr. Psychiatry 28, 712–721. doi: 10.1016/j.jagp.2020.04.010
Bulfamante, G., Bocci, T., Falleni, M., Campiglio, L., Coppola, S., Tosi, D., et al. (2021). Brainstem neuropathology in two cases of COVID-19: SARS-CoV-2 trafficking between brain and lung. J. Neurol. 268, 4486–4491. doi: 10.1007/s00415-021-10604-8
Callaway, E. (2020). Labs rush to study coronavirus in transgenic animals - some are in short supply. Nature 579:183. doi: 10.1038/d41586-020-00698-x
Cantuti-Castelvetri, L., Ojha, R., Pedro, L. D., Djannatian, M., Franz, J., Kuivanen, S., et al. (2020). Neuropilin-1 facilitates SARS-CoV-2 cell entry and infectivity. Science 370, 856–860. doi: 10.1126/science.abd2985
Caramaschi, S., Kapp, M. E., Miller, S. E., Eisenberg, R., Johnson, J., Epperly, G., et al. (2021). Histopathological findings and clinicopathologic correlation in COVID-19: a systematic review. Mod. Pathol. 34, 1614–1633. doi: 10.1038/s41379-021-00814-w
Carvalho, T., Krammer, F., and Iwasaki, A. (2021). The first 12 months of COVID-19: a timeline of immunological insights. Nat. Rev. Immunol. 21, 245–256. doi: 10.1038/s41577-021-00522-1
Catanzaro, M., Fagiani, F., Racchi, M., Corsini, E., Govoni, S., and Lanni, C. (2020). Immune response in COVID-19: addressing a pharmacological challenge by targeting pathways triggered by SARS-CoV-2. Signal Transduct. Target. Ther. 5:84. doi: 10.1038/s41392-020-0191-1
Chaná-Cuevas, P., Salles-Gándara, P., Rojas-Fernandez, A., Salinas-Rebolledo, C., and Milán-Solé, A. (2020). The potential role of SARS-COV-2 in the pathogenesis of parkinson’s disease. Front. Neurol. 11:1044. doi: 10.3389/fneur.2020.01044
Chaplin, D. D. (2010). Overview of the immune response. J. Allergy Clin. Immunol. 125(2 Suppl. 2) S3–S23. doi: 10.1016/j.jaci.2009.12.980
Chaudhry, Z. L., Klenja, D., Janjua, N., Cami-Kobeci, G., and Ahmed, B. Y. (2020). COVID-19 and parkinson’s disease: shared inflammatory pathways under oxidative stress. Brain Sci. 10:807. doi: 10.3390/brainsci10110807
Chen, G., Wu, D., Guo, W., Cao, Y., Huang, D., Wang, H., et al. (2020). Clinical and immunological features of severe and moderate coronavirus disease 2019. J. Clin. Invest. 130, 2620–2629. doi: 10.1172/JCI137244
Chen, R., Lan, Z., Ye, J., Pang, L., Liu, Y., Wu, W., et al. (2021). Cytokine storm: the primary determinant for the pathophysiological evolution of COVID-19 deterioration. Front. Immunol. 12:589095. doi: 10.3389/fimmu.2021.589095
Chen, R., Wang, K., Yu, J., Howard, D., French, L., Chen, Z., et al. (2020). The spatial and cell-type distribution of SARS-CoV-2 receptor ACE2 in the human and mouse brains. Front. Neurol. 11:573095. doi: 10.3389/fneur.2020.573095
Chen, X., Yu, H., Mei, T., Chen, B., Chen, L., Li, S., et al. (2021). SARS-CoV-2 on the ocular surface: is it truly a novel transmission route? Br. J. Ophthalmol. 105, 1190–1195. doi: 10.1136/bjophthalmol-2020-316263
Chiricosta, L., Gugliandolo, A., and Mazzon, E. (2021). SARS-CoV-2 exacerbates beta-amyloid neurotoxicity, inflammation and oxidative stress in alzheimer’s disease patients. Int. J. Mol. Sci. 22:13603. doi: 10.3390/ijms222413603
Collin, J., Queen, R., Zerti, D., Dorgau, B., Georgiou, M., Djidrovski, I., et al. (2021). Co-expression of SARS-CoV-2 entry genes in the superficial adult human conjunctival, limbal and corneal epithelium suggests an additional route of entry via the ocular surface. Ocul. Surf. 19, 190–200. doi: 10.1016/j.jtos.2020.05.013
Coolen, T., Lolli, V., Sadeghi, N., Rovai, A., Trotta, N., Taccone, F. S., et al. (2020). Early postmortem brain MRI findings in COVID-19 non-survivors. Neurology 95, e2016–e2027. doi: 10.1212/WNL.0000000000010116
Coroneo, M. T. (2021). The eye as the discrete but defensible portal of coronavirus infection. Ocul. Surf. 19, 176–182. doi: 10.1016/j.jtos.2020.05.011
da Fonseca, A. C., Matias, D., Garcia, C., Amaral, R., Geraldo, L. H., Freitas, C., et al. (2014). The impact of microglial activation on blood-brain barrier in brain diseases. Front. Cell Neurosci. 8:362. doi: 10.3389/fncel.2014.00362
Dahm, T., Rudolph, H., Schwerk, C., Schroten, H., and Tenenbaum, T. (2016). Neuroinvasion and inflammation in viral central nervous system infections. Mediators Inflamm. 2016:8562805. doi: 10.1155/2016/8562805
Daly, J. L., Simonetti, B., Klein, K., Chen, K. E., Williamson, M. K., Antón-Plágaro, C., et al. (2020). Neuropilin-1 is a host factor for SARS-CoV-2 infection. Science 370, 861–865. doi: 10.1126/science.abd3072
Daneman, R. (2012). The blood-brain barrier in health and disease. Ann. Neurol. 72, 648–672. doi: 10.1002/ana.23648
Daneman, R., and Prat, A. (2015). The blood-brain barrier. Cold Spring Harb. Perspect. Biol. 7:a020412. doi: 10.1101/cshperspect.a020412
Davies, J., Randeva, H. S., Chatha, K., Hall, M., Spandidos, D. A., Karteris, E., et al. (2020). Neuropilin−1 as a new potential SARS−CoV−2 infection mediator implicated in the neurologic features and central nervous system involvement of COVID−19. Mol. Med. Rep. 22, 4221–4226. doi: 10.3892/mmr.2020.11510
De Chiara, G., Marcocci, M. E., Sgarbanti, R., Civitelli, L., Ripoli, C., Piacentini, R., et al. (2012). Infectious agents and neurodegeneration. Mol. Neurobiol. 46, 614–638. doi: 10.1007/s12035-012-8320-7
de Freitas Santoro, D., de Sousa, L. B., Câmara, N. O. S., de Freitas, D., and de Oliveira, L. A. (2021). SARS-COV-2 and ocular surface: from physiology to pathology, a route to understand transmission and disease. Front. Physiol. 12:612319. doi: 10.3389/fphys.2021.612319
de Wit, E., van Doremalen, N., Falzarano, D., and Munster, V. J. (2016). SARS and MERS: recent insights into emerging coronaviruses. Nat. Rev. Microbiol. 14, 523–534. doi: 10.1038/nrmicro.2016.81
Deatly, A. M., Haase, A. T., Fewster, P. H., Lewis, E., and Ball, M. J. (1990). Human herpes virus infections and Alzheimer’s disease. Neuropathol. Appl. Neurobiol. 16, 213–223. doi: 10.1111/j.1365-2990.1990.tb01158.x
Desforges, M., Le Coupanec, A., Dubeau, P., Bourgouin, A., Lajoie, L., Dubé, M., et al. (2019). Human coronaviruses and other respiratory viruses: underestimated opportunistic pathogens of the central nervous system? Viruses 12:14. doi: 10.3390/v12010014
Ding, Y., He, L., Zhang, Q., Huang, Z., Che, X., Hou, J., et al. (2004). Organ distribution of severe acute respiratory syndrome (SARS) associated coronavirus (SARS-CoV) in SARS patients: implications for pathogenesis and virus transmission pathways. J. Pathol. 203, 622–630. doi: 10.1002/path.1560
Dinnon, K. H., Leist, S. R., Schäfer, A., Edwards, C. E., Martinez, D. R., Montgomery, S. A., et al. (2020). A mouse-adapted model of SARS-CoV-2 to test COVID-19 countermeasures. Nature 586, 560–566. doi: 10.1038/s41586-020-2708-8
Dobrindt, K., Hoagland, D. A., Seah, C., Kassim, B., O’Shea, C. P., Murphy, A., et al. (2021). Common genetic variation in humans impacts in vitro susceptibility to SARS-CoV-2 infection. Stem Cell Rep. 16, 505–518. doi: 10.1016/j.stemcr.2021.02.010
Domingues, R. B., Mendes-Correa, M. C., de Moura Leite, F. B. V., Sabino, E. C., Salarini, D. Z., Claro, I., et al. (2020). First case of SARS-COV-2 sequencing in cerebrospinal fluid of a patient with suspected demyelinating disease. J. Neurol. 267, 3154–3156. doi: 10.1007/s00415-020-09996-w
Driessen, A. K., Farrell, M. J., Mazzone, S. B., and McGovern, A. E. (2016). Multiple neural circuits mediating airway sensations: recent advances in the neurobiology of the urge-to-cough. Respir. Physiol. Neurobiol. 226, 115–120. doi: 10.1016/j.resp.2015.09.017
Dubé, M., Le Coupanec, A., Wong, A. H. M., Rini, J. M., Desforges, M., and Talbot, P. J. (2018). Axonal transport enables neuron-to-neuron propagation of human coronavirus OC43. J. Virol. 92, e00404–e00418. doi: 10.1128/JVI.00404-18
ElBini Dhouib, I. (2021). Does coronaviruses induce neurodegenerative diseases? A systematic review on the neurotropism and neuroinvasion of SARS-CoV-2. Drug Discov. Ther. 14, 262–272. doi: 10.5582/ddt.2020.03106
Ellul, M. A., Benjamin, L., Singh, B., Lant, S., Michael, B. D., Easton, A., et al. (2020). Neurological associations of COVID-19. Lancet Neurol. 19, 767–783. doi: 10.1016/S1474-4422(20)30221-0
Ezzat, K., Pernemalm, M., Pålsson, S., Roberts, T. C., Järver, P., Dondalska, A., et al. (2019). The viral protein corona directs viral pathogenesis and amyloid aggregation. Nat. Commun. 10:2331. doi: 10.1038/s41467-019-10192-2
Fearon, C., and Fasano, A. (2021). Parkinson’s Disease and the COVID-19 Pandemic. J. Parkinsons Dis. 11, 431–444. doi: 10.3233/JPD-202320
Ferini-Strambi, L., and Salsone, M. (2021). COVID-19 and neurological disorders: are neurodegenerative or neuroimmunological diseases more vulnerable? J. Neurol. 268, 409–419. doi: 10.1007/s00415-020-10070-8
Ferrando, S. J., Klepacz, L., Lynch, S., Tavakkoli, M., Dornbush, R., Baharani, R., et al. (2020). COVID-19 psychosis: a potential new neuropsychiatric condition triggered by novel coronavirus infection and the inflammatory response? Psychosomatics 61, 551–555. doi: 10.1016/j.psym.2020.05.012
Finelli, C. (2021). Metabolic syndrome, alzheimer’s disease, and Covid 19: a possible correlation. Curr. Alzheimer Res. 18, 915–924. doi: 10.2174/1567205018666211209095652
Flores-Silva, F. D., García-Grimshaw, M., Valdés-Ferrer, S. I., Vigueras-Hernández, A. P., Domínguez-Moreno, R., Tristán-Samaniego, D. P., et al. (2021). Neurologic manifestations in hospitalized patients with COVID-19 in Mexico City. PLoS One 16:e0247433. doi: 10.1371/journal.pone.0247433
Forcados, G. E., Muhammad, A., Oladipo, O. O., Makama, S., and Meseko, C. A. (2021). Metabolic implications of oxidative stress and inflammatory process in SARS-CoV-2 pathogenesis: therapeutic potential of natural antioxidants. Front. Cell Infect. Microbiol. 11:654813. doi: 10.3389/fcimb.2021.654813
Frank, M. G., Nguyen, K. H., Ball, J. B., Hopkins, S., Kelley, T., Baratta, M. V., et al. (2022). SARS-CoV-2 spike S1 subunit induces neuroinflammatory, microglial and behavioral sickness responses: evidence of PAMP-like properties. Brain Behav. Immun. 100, 267–277. doi: 10.1016/j.bbi.2021.12.007
Gandhi, J., Antonelli, A. C., Afridi, A., Vatsia, S., Joshi, G., Romanov, V., et al. (2019). Protein misfolding and aggregation in neurodegenerative diseases: a review of pathogeneses, novel detection strategies, and potential therapeutics. Rev. Neurosci. 30, 339–358. doi: 10.1515/revneuro-2016-0035
García-Moncó, J. C., Cabrera Muras, A., Erburu Iriarte, M., Rodrigo Armenteros, P., Collía Fernández, A., Arranz-Martínez, J., et al. (2021). Neurologic manifestations in a prospective unselected series of hospitalized patients with COVID-19. Neurol. Clin. Pract. 11, e64–e72. doi: 10.1212/CPJ.0000000000000913
Gasmi, A., Tippairote, T., Mujawdiya, P. K., Gasmi Benahmed, A., Menzel, A., Dadar, M., et al. (2021). Neurological involvements of SARS-CoV2 infection. Mol. Neurobiol. 58, 944–949. doi: 10.1007/s12035-020-02070-6
Generoso, J. S., Barichello de Quevedo, J. L., Cattani, M., Lodetti, B. F., Sousa, L., Collodel, A., et al. (2021). Neurobiology of COVID-19: how can the virus affect the brain? Braz. J. Psychiatry 43, 650–664. doi: 10.1590/1516-4446-2020-1488
Gilani, S., Roditi, R., and Naraghi, M. (2020). COVID-19 and anosmia in Tehran, Iran. Med. Hypotheses 141:109757. doi: 10.1016/j.mehy.2020.109757
Gilpin, S., Byers, M., Byrd, A., Cull, J., Peterson, D., Thomas, B., et al. (2021). Rhabdomyolysis as the initial presentation of SARS-CoV-2 in an adolescent. Pediatrics 147:e2020019273. doi: 10.1542/peds.2020-019273
Graham, N. S., and Sharp, D. J. (2019). Understanding neurodegeneration after traumatic brain injury: from mechanisms to clinical trials in dementia. J. Neurol. Neurosurg. Psychiatry 90, 1221–1233. doi: 10.1136/jnnp-2017-317557
Guan, W. J., Ni, Z. Y., Hu, Y., Liang, W. H., Ou, C. Q., He, J. X., et al. (2020). Clinical characteristics of coronavirus disease 2019 in China. N. Engl. J. Med. 382, 1708–1720. doi: 10.1056/NEJMoa2002032
Guidon, A. C., and Amato, A. A. (2020). COVID-19 and neuromuscular disorders. Neurology 94, 959–969. doi: 10.1212/WNL.0000000000009566
Gutiérrez-Ortiz, C., Méndez-Guerrero, A., Rodrigo-Rey, S., San Pedro-Murillo, E., Bermejo-Guerrero, L., Gordo-Mañas, R., et al. (2020). Miller Fisher syndrome and polyneuritis cranialis in COVID-19. Neurology 95, e601–e605. doi: 10.1212/WNL.0000000000009619
Habibzadeh, P., Mofatteh, M., Silawi, M., Ghavami, S., and Faghihi, M. A. (2021). Molecular diagnostic assays for COVID-19: an overview. Crit. Rev. Clin. Lab. Sci. 58, 385–398. doi: 10.1080/10408363.2021.1884640
Harwood, R., Yan, H., Talawila Da Camara, N., Smith, C., Ward, J., Tudur-Smith, C., et al. (2022). Which children and young people are at higher risk of severe disease and death after hospitalisation with SARS-CoV-2 infection in children and young people: a systematic review and individual patient meta-analysis. EClinical Med. 44:101287. doi: 10.1016/j.eclinm.2022.101287
Hassan, M., Mustafa, F., Syed, F., Mustafa, A., Mushtaq, H. F., Khan, N. U., et al. (2021). Ocular surface: a route for SARS CoV-2 transmission- a case report. Brain Hemorrhages 2, 139–140. doi: 10.1016/j.hest.2021.09.003
Helms, J., Kremer, S., Merdji, H., Clere-Jehl, R., Schenck, M., Kummerlen, C., et al. (2020). Neurologic features in severe SARS-CoV-2 infection. N. Engl. J. Med. 382, 2268–2270. doi: 10.1056/NEJMc2008597
Henderson, M. X., Trojanowski, J. Q., and Lee, V. M. (2019). α-Synuclein pathology in Parkinson’s disease and related α-synucleinopathies. Neurosci. Lett. 709:134316. doi: 10.1016/j.neulet.2019.134316
Hill, J. M., Clement, C., Arceneaux, L., and Lukiw, W. J. (2021). Angiotensin converting enzyme 2 (ACE2) expression in the aged brain and visual system. J. Aging Sci. 9(Suppl.):7.
Hoffmann, M., Kleine-Weber, H., Schroeder, S., Krüger, N., Herrler, T., Erichsen, S., et al. (2020). SARS-CoV-2 cell entry depends on ACE2 and TMPRSS2 and is blocked by a clinically proven protease inhibitor. Cell 181, 271.e–280.e. doi: 10.1016/j.cell.2020.02.052
Hou, Y., Dan, X., Babbar, M., Wei, Y., Hasselbalch, S. G., Croteau, D. L., et al. (2019). Ageing as a risk factor for neurodegenerative disease. Nat. Rev. Neurol. 15, 565–581. doi: 10.1038/s41582-019-0244-7
Hu, B., Guo, H., Zhou, P., and Shi, Z. L. (2021). Characteristics of SARS-CoV-2 and COVID-19. Nat. Rev. Microbiol. 19, 141–154. doi: 10.1038/s41579-020-00459-7
Hu, J., Jolkkonen, J., and Zhao, C. (2020). Neurotropism of SARS-CoV-2 and its neuropathological alterations: similarities with other coronaviruses. Neurosci. Biobehav. Rev. 119, 184–193. doi: 10.1016/j.neubiorev.2020.10.012
Huang, C., Wang, Y., Li, X., Ren, L., Zhao, J., Hu, Y., et al. (2020). Clinical features of patients infected with 2019 novel coronavirus in Wuhan, China. Lancet 395, 497–506. doi: 10.1016/S0140-6736(20)30183-5
Huang, Y. H., Jiang, D., and Huang, J. T. (2020). SARS-CoV-2 detected in cerebrospinal fluid by pcr in a case of COVID-19 encephalitis. Brain Behav. Immun. 87:149. doi: 10.1016/j.bbi.2020.05.012
Huang, Y., Lu, Y., Huang, Y. M., Wang, M., Ling, W., Sui, Y., et al. (2020). Obesity in patients with COVID-19: a systematic review and meta-analysis. Metabolism 113:154378. doi: 10.1016/j.metabol.2020.154378
Hurley, L. L., and Tizabi, Y. (2013). Neuroinflammation, neurodegeneration, and depression. Neurotox Res. 23, 131–144. doi: 10.1007/s12640-012-9348-1
Hussain, B., Fang, C., and Chang, J. (2021). Blood-brain barrier breakdown: an emerging biomarker of cognitive impairment in normal aging and dementia. Front. Neurosci. 15:688090. doi: 10.3389/fnins.2021.688090
Hwang, S. T., Ballout, A. A., Mirza, U., Sonti, A. N., Husain, A., Kirsch, C., et al. (2020). Acute seizures occurring in association with SARS-CoV-2. Front. Neurol. 11:576329. doi: 10.3389/fneur.2020.576329
Idrees, D., and Kumar, V. (2021). SARS-CoV-2 spike protein interactions with amyloidogenic proteins: potential clues to neurodegeneration. Biochem. Biophys. Res. Commun. 554, 94–98. doi: 10.1016/j.bbrc.2021.03.100
Israelow, B., Song, E., Mao, T., Lu, P., Meir, A., Liu, F., et al. (2020). Mouse model of SARS-CoV-2 reveals inflammatory role of type I interferon signaling. J. Exp. Med. 217:e20201241. doi: 10.1084/jem.20201241
Jang, H., Boltz, D. A., Webster, R. G., and Smeyne, R. J. (2009). Viral parkinsonism. Biochim. Biophys. Acta 1792, 714–721. doi: 10.1016/j.bbadis.2008.08.001
Johansen, M. D., Irving, A., Montagutelli, X., Tate, M. D., Rudloff, I., Nold, M. F., et al. (2020). Animal and translational models of SARS-CoV-2 infection and COVID-19. Mucosal Immunol. 13, 877–891. doi: 10.1038/s41385-020-00340-z
Justice, N. J. (2018). The relationship between stress and Alzheimer’s disease. Neurobiol. Stress 8, 127–133. doi: 10.1016/j.ynstr.2018.04.002
Kapoor, M. C. (2020). Respiratory and cardiovascular effects of COVID-19 infection and their management. J. Anaesthesiol. Clin. Pharmacol. 36(Suppl. 1) S21–S28. doi: 10.4103/joacp.JOACP_242_20
Kempuraj, D., Selvakumar, G. P., Ahmed, M. E., Raikwar, S. P., Thangavel, R., Khan, A., et al. (2020). COVID-19, mast cells, cytokine storm, psychological stress, and neuroinflammation. Neuroscientist 26, 402–414. doi: 10.1177/1073858420941476
Kisler, K., Nelson, A. R., Rege, S. V., Ramanathan, A., Wang, Y., Ahuja, A., et al. (2017). Pericyte degeneration leads to neurovascular uncoupling and limits oxygen supply to brain. Nat. Neurosci. 20, 406–416. doi: 10.1038/nn.4489
Klopfenstein, T., Kadiane-Oussou, N. J., Toko, L., Royer, P. Y., Lepiller, Q., Gendrin, V., et al. (2020). Features of anosmia in COVID-19. Med. Mal. Infect. 50, 436–439. doi: 10.1016/j.medmal.2020.04.006
Koehler, M., Delguste, M., Sieben, C., Gillet, L., and Alsteens, D. (2020). Initial step of virus entry: virion binding to cell-surface glycans. Ann. Rev. Virol. 7, 143–165. doi: 10.1146/annurev-virology-122019-070025
Kumar, D., Jahan, S., Khan, A., Siddiqui, A. J., Redhu, N. S., Khan, J., et al. (2021). Neurological manifestation of SARS-CoV-2 induced inflammation and possible therapeutic strategies against COVID-19. Mol. Neurobiol. 58, 3417–3434. doi: 10.1007/s12035-021-02318-9
Kurki, S. N., Kantonen, J., Kaivola, K., Hokkanen, L., Mäyränpää, M. I., Puttonen, H., et al. (2021). APOE ε4 associates with increased risk of severe COVID-19, cerebral microhaemorrhages and post-COVID mental fatigue: a finnish biobank, autopsy and clinical study. Acta Neuropathol. Commun. 9:199. doi: 10.1186/s40478-021-01302-7
Kwon, M., and Lee, J. H. (2019). Oro-pharyngeal dysphagia in parkinson’s disease and related movement disorders. J. Mov. Disord. 12, 152–160. doi: 10.14802/jmd.19048
Lacy, J. M., Brooks, E. G., Akers, J., Armstrong, D., Decker, L., Gonzalez, A., et al. (2020). COVID-19: postmortem diagnostic and biosafety considerations. Am. J. For. Med. Pathol. 41, 143–151. doi: 10.1097/PAF.0000000000000567
Lax, S. F., Skok, K., Zechner, P., Kessler, H. H., Kaufmann, N., Koelblinger, C., et al. (2020). Pulmonary arterial thrombosis in COVID-19 with fatal outcome : results from a prospective. single-center, clinicopathologic case series. Ann. Intern. Med. 173, 350–361. doi: 10.7326/M20-2566
Lazzeri, C., Bonizzoli, M., Batacchi, S., Cianchi, G., Franci, A., Fulceri, G. E., et al. (2020). Cardiac involvment in COVID-19-related acute respiratory distress syndrome. Am. J. Cardiol. 132, 147–149. doi: 10.1016/j.amjcard.2020.07.010
Lebrasseur, A., Fortin-Bédard, N., Lettre, J., Raymond, E., Bussières, E. L., Lapierre, N., et al. (2021). Impact of the COVID-19 pandemic on older adults: rapid review. JMIR Aging 4:e26474. doi: 10.2196/26474
Lee, M. H., Perl, D. P., Nair, G., Li, W., Maric, D., Murray, H., et al. (2021). Microvascular injury in the brains of patients with Covid-19. N. Engl. J. Med. 384, 481–483. doi: 10.1056/NEJMc2033369
Leng, F., and Edison, P. (2021). Neuroinflammation and microglial activation in Alzheimer disease: where do we go from here? Nat. Rev. Neurol. 17, 157–172. doi: 10.1038/s41582-020-00435-y
Leonardi, A., Rosani, U., and Brun, P. (2020). Ocular surface expression of SARS-CoV-2 receptors. Ocul. Immunol. Inflamm. 28, 735–738. doi: 10.1080/09273948.2020.1772314
Levi, M., Thachil, J., Iba, T., and Levy, J. H. (2020). Coagulation abnormalities and thrombosis in patients with COVID-19. Lancet Haematol. 7, e438–e440. doi: 10.1016/S2352-3026(20)30145-9
Levinson, R., Elbaz, M., Ben-Ami, R., Shasha, D., Levinson, T., Choshen, G., et al. (2020). Time course of anosmia and dysgeusia in patients with mild SARS-CoV-2 infection. Infect. Dis. 52, 600–602. doi: 10.1080/23744235.2020.1772992
Li, L., Acioglu, C., Heary, R. F., and Elkabes, S. (2021). Role of astroglial toll-like receptors (TLRs) in central nervous system infections, injury and neurodegenerative diseases. Brain Behav. Immun. 91, 740–755. doi: 10.1016/j.bbi.2020.10.007
Li, M. Y., Li, L., Zhang, Y., and Wang, X. S. (2020). Expression of the SARS-CoV-2 cell receptor gene ACE2 in a wide variety of human tissues. Infect. Dis. Poverty 9:45. doi: 10.1186/s40249-020-00662-x
Li, W., Moore, M. J., Vasilieva, N., Sui, J., Wong, S. K., Berne, M. A., et al. (2003). Angiotensin-converting enzyme 2 is a functional receptor for the SARS coronavirus. Nature 426, 450–454. doi: 10.1038/nature02145
Limphaibool, N., Iwanowski, P., Holstad, M. J. V., Kobylarek, D., and Kozubski, W. (2019). Infectious etiologies of parkinsonism: pathomechanisms and clinical implications. Front. Neurol. 10:652. doi: 10.3389/fneur.2019.00652
Liu, J. M., Tan, B. H., Wu, S., Gui, Y., Suo, J. L., and Li, Y. C. (2021). Evidence of central nervous system infection and neuroinvasive routes, as well as neurological involvement, in the lethality of SARS-CoV-2 infection. J. Med. Virol. 93, 1304–1313. doi: 10.1002/jmv.26570
Liu, P. P., Xie, Y., Meng, X. Y., and Kang, J. S. (2019). History and progress of hypotheses and clinical trials for Alzheimer’s disease. Signal Transduct. Target. Ther. 4:29. doi: 10.1038/s41392-019-0063-8
Liu, S., Hossinger, A., Heumüller, S. E., Hornberger, A., Buravlova, O., Konstantoulea, K., et al. (2021). Highly efficient intercellular spreading of protein misfolding mediated by viral ligand-receptor interactions. Nat. Commun. 12:5739. doi: 10.1038/s41467-021-25855-2
Lochhead, J. J., and Thorne, R. G. (2012). Intranasal delivery of biologics to the central nervous system. Adv. Drug. Deliv. Rev. 64, 614–628. doi: 10.1016/j.addr.2011.11.002
Lotz, S. K., Blackhurst, B. M., Reagin, K. L., and Funk, K. E. (2021). Microbial infections are a risk factor for neurodegenerative diseases. Front. Cell Neurosci. 15:691136. doi: 10.3389/fncel.2021.691136
Lu, R., Zhao, X., Li, J., Niu, P., Yang, B., Wu, H., et al. (2020). Genomic characterisation and epidemiology of 2019 novel coronavirus: implications for virus origins and receptor binding. Lancet 395, 565–574. doi: 10.1016/S0140-6736(20)30251-8
Lu, Y., Li, X., Geng, D., Mei, N., Wu, P. Y., Huang, C. C., et al. (2020). Cerebral micro-structural changes in COVID-19 patients - an MRI-based 3-month follow-up study. EClinicalMedicine 25:100484. doi: 10.1016/j.eclinm.2020.100484
Ma, D., Chen, C. B., Jhanji, V., Xu, C., Yuan, X. L., Liang, J. J., et al. (2020). Expression of SARS-CoV-2 receptor ACE2 and TMPRSS2 in human primary conjunctival and pterygium cell lines and in mouse cornea. Eye 34, 1212–1219. doi: 10.1038/s41433-020-0939-4
Madore, C., Yin, Z., Leibowitz, J., and Butovsky, O. (2020). Microglia, lifestyle stress, and neurodegeneration. Immunity 52, 222–240. doi: 10.1016/j.immuni.2019.12.003
Manji, H. K., George, U., Mkopi, N. P., and Manji, K. P. (2020). Guillain-Barré syndrome associated with COVID-19 infection. Pan. Afr. Med. J. 35(Suppl. 2):118. doi: 10.11604/pamj.supp.2020.35.2.25003
Mao, L., Jin, H., Wang, M., Hu, Y., Chen, S., He, Q., et al. (2020). Neurologic manifestations of hospitalized patients with coronavirus disease 2019 in Wuhan, China. JAMA Neurol. 77, 683–690. doi: 10.1001/jamaneurol.2020.1127
Marreiros, R., Müller-Schiffmann, A., Trossbach, S. V., Prikulis, I., Hänsch, S., Weidtkamp-Peters, S., et al. (2020). Disruption of cellular proteostasis by H1N1 influenza A virus causes α-synuclein aggregation. Proc. Natl. Acad. Sci. U.S.A. 117, 6741–6751. doi: 10.1073/pnas.1906466117
Masters, P. S. (2006). The molecular biology of coronaviruses. Adv. Virus Res. 66, 193–292. doi: 10.1016/S0065-3527(06)66005-3
Meegada, S., Muppidi, V., Wilkinson, D. C., Siddamreddy, S., and Katta, S. K. (2020). Coronavirus disease 2019-induced rhabdomyolysis. Cureus 12:e10123. doi: 10.7759/cureus.10123
Mehrabadi, M. E., Hemmati, R., Tashakor, A., Homaei, A., Yousefzadeh, M., Hemati, K., et al. (2021). Induced dysregulation of ACE2 by SARS-CoV-2 plays a key role in COVID-19 severity. Biomed. Pharmacother. 137:111363. doi: 10.1016/j.biopha.2021.111363
Mehta, P., and Fajgenbaum, D. C. (2021). Is severe COVID-19 a cytokine storm syndrome: a hyperinflammatory debate. Curr. Opin. Rheumatol. 33, 419–430. doi: 10.1097/BOR.0000000000000822
Meng, L., Shen, L., and Ji, H. F. (2019). Impact of infection on risk of Parkinson’s disease: a quantitative assessment of case-control and cohort studies. J. Neurovirol. 25, 221–228. doi: 10.1007/s13365-018-0707-4
Messlinger, K., Neuhuber, W., and May, A. (2021). Activation of the trigeminal system as a likely target of SARS-CoV-2 may contribute to anosmia in COVID-19. Cephalalgia 42, 176–180. doi: 10.1177/03331024211036665
Milligan Armstrong, A., Porter, T., Quek, H., White, A., Haynes, J., Jackaman, C., et al. (2021). Chronic stress and Alzheimer’s disease: the?interplay between the hypothalamic-pituitary-adrenal axis, genetics and microglia. Biol. Rev. Camb. Philos. Soc. 96, 2209–2228. doi: 10.1111/brv.12750
Miners, S., Kehoe, P. G., and Love, S. (2020). Cognitive impact of COVID-19: looking beyond the short term. Alzheimers Res. Ther. 12:170. doi: 10.1186/s13195-020-00744-w
Mohammadi, S., Moosaie, F., and Aarabi, M. H. (2020). Understanding the immunologic characteristics of neurologic manifestations of SARS-CoV-2 and potential immunological mechanisms. Mol. Neurobiol. 57, 5263–5275. doi: 10.1007/s12035-020-02094-y
Montagne, A., Nikolakopoulou, A. M., Huuskonen, M. T., Sagare, A. P., Lawson, E. J., Lazic, D., et al. (2021). Accelerates advanced-stage vascular and neurodegenerative disorder in old Alzheimer’s mice via cyclophilin A independently of amyloid-β. Nat. Aging 1, 506–520. doi: 10.1038/s43587-021-00073-z
Montagne, A., Zhao, Z., and Zlokovic, B. V. (2017). Alzheimer’s disease: a matter of blood-brain barrier dysfunction? J. Exp. Med. 214, 3151–3169. doi: 10.1084/jem.20171406
Mori, I. (2015). Transolfactory neuroinvasion by viruses threatens the human brain. Acta Virol. 59, 338–349. doi: 10.4149/av_2015_04_338
Moriguchi, T., Harii, N., Goto, J., Harada, D., Sugawara, H., Takamino, J., et al. (2020). A first case of meningitis/encephalitis associated with SARS-Coronavirus-2. Int. J. Infect. Dis. 94, 55–58. doi: 10.1016/j.ijid.2020.03.062
Mrityunjaya, M., Pavithra, V., Neelam, R., Janhavi, P., Halami, P. M., and Ravindra, P. V. (2020). Immune-boosting, antioxidant and anti-inflammatory food supplements targeting pathogenesis of COVID-19. Front. Immunol. 11:570122. doi: 10.3389/fimmu.2020.570122
Muñoz-Fontela, C., Dowling, W. E., Funnell, S. G. P., Gsell, P. S., Riveros-Balta, A. X., Albrecht, R. A., et al. (2020). Animal models for COVID-19. Nature 586, 509–515. doi: 10.1038/s41586-020-2787-6
Mutiawati, E., Fahriani, M., Mamada, S. S., Fajar, J. K., Frediansyah, A., Maliga, H. A., et al. (2021). Anosmia and dysgeusia in SARS-CoV-2 infection: incidence and effects on COVID-19 severity and mortality, and the possible pathobiology mechanisms - a systematic review and meta-analysis. F1000Res 10:40. doi: 10.12688/f1000research.28393.1
Naqvi, A. A. T., Fatima, K., Mohammad, T., Fatima, U., Singh, I. K., Singh, A., et al. (2020). Insights into SARS-CoV-2 genome, structure, evolution, pathogenesis and therapies: structural genomics approach. Biochim. Biophys. Acta Mol. Basis Dis. 1866:165878. doi: 10.1016/j.bbadis.2020.165878
Natoli, S., Oliveira, V., Calabresi, P., Maia, L. F., and Pisani, A. (2020). Does SARS-Cov-2 invade the brain? Translational lessons from animal models. Eur. J. Neurol. 27, 1764–1773. doi: 10.1111/ene.14277
Ni, W., Yang, X., Yang, D., Bao, J., Li, R., Xiao, Y., et al. (2020). Role of angiotensin-converting enzyme 2 (ACE2) in COVID-19. Crit. Care 24:422. doi: 10.1186/s13054-020-03120-0
Niesen, M., Trotta, N., Noel, A., Coolen, T., Fayad, G., Leurkin-Sterk, G., et al. (2021). Structural and metabolic brain abnormalities in COVID-19 patients with sudden loss of smell. Eur. J. Nucl. Med. Mol. Imaging 48, 1890–1901. doi: 10.1007/s00259-020-05154-6
Niraula, A., Sheridan, J. F., and Godbout, J. P. (2017). Microglia priming with aging and stress. Neuropsychopharmacology 42, 318–333. doi: 10.1038/npp.2016.185
Noe, C. R., Noe-Letschnig, M., Handschuh, P., Noe, C. A., and Lanzenberger, R. (2020). Dysfunction of the blood-brain barrier-a key step in neurodegeneration and dementia. Front. Aging Neurosci. 12:185. doi: 10.3389/fnagi.2020.00185
Noroozi, R., Branicki, W., Pyrc, K., Łabaj, P. P., Pospiech, E., Taheri, M., et al. (2020). Altered cytokine levels and immune responses in patients with SARS-CoV-2 infection and related conditions. Cytokine 133:155143. doi: 10.1016/j.cyto.2020.155143
Pacheco-Herrero, M., Soto-Rojas, L. O., Harrington, C. R., Flores-Martinez, Y. M., Villegas-Rojas, M. M., León-Aguilar, A. M., et al. (2021). Elucidating the neuropathologic mechanisms of SARS-CoV-2 infection. Front. Neurol. 12:660087. doi: 10.3389/fneur.2021.660087
Pan, A. P., Meeks, J., Potter, T., Masdeu, J. C., Seshadri, S., Smith, M. L., et al. (2021). SARS-CoV-2 susceptibility and COVID-19 mortality among older adults with cognitive impairment: cross-sectional analysis from hospital records in a diverse us metropolitan area. Front. Neurol. 12:692662. doi: 10.3389/fneur.2021.692662
Pan, Y., Jiang, X., Yang, L., Chen, L., Zeng, X., Liu, G., et al. (2021). SARS-CoV-2-specific immune response in COVID-19 convalescent individuals. Signal Transduct. Target. Ther. 6:256. doi: 10.1038/s41392-021-00686-1
Paniz-Mondolfi, A., Bryce, C., Grimes, Z., Gordon, R. E., Reidy, J., Lednicky, J., et al. (2020). Central nervous system involvement by severe acute respiratory syndrome coronavirus-2 (SARS-CoV-2). J. Med. Virol. 92, 699–702. doi: 10.1002/jmv.25915
Parikh, N. S., Merkler, A. E., and Iadecola, C. (2020). Inflammation, autoimmunity, infection, and stroke: epidemiology and lessons from therapeutic intervention. Stroke 51, 711–718. doi: 10.1161/STROKEAHA.119.024157
Park, J. H., Kim, D. H., Park, Y. G., Kwon, D. Y., Choi, M., Jung, J. H., et al. (2020). Association of parkinson disease with risk of cardiovascular disease and all-cause mortality: a nationwide. Population-Based Cohort Study. Circulation 141, 1205–1207. doi: 10.1161/CIRCULATIONAHA.119.044948
Paterson, R. W., Brown, R. L., Benjamin, L., Nortley, R., Wiethoff, S., Bharucha, T., et al. (2020). The emerging spectrum of COVID-19 neurology: clinical, radiological and laboratory findings. Brain 143, 3104–3120. doi: 10.1093/brain/awaa240
Patra, T., Meyer, K., Geerling, L., Isbell, T. S., Hoft, D. F., Brien, J., et al. (2020). SARS-CoV-2 spike protein promotes IL-6 trans-signaling by activation of angiotensin II receptor signaling in epithelial cells. PLoS Pathog. 16:e1009128. doi: 10.1371/journal.ppat.1009128
Pellegrini, L., Albecka, A., Mallery, D. L., Kellner, M. J., Paul, D., Carter, A. P., et al. (2020). SARS-CoV-2 infects the brain choroid plexus and disrupts the blood-CSF barrier in human brain organoids. Cell Stem Cell 27, 951.e–961.e. doi: 10.1016/j.stem.2020.10.001
Peng, R., Wu, L. A., Wang, Q., Qi, J., and Gao, G. F. (2021). Cell entry by SARS-CoV-2. Trends Biochem. Sci. 46, 848–860. doi: 10.1016/j.tibs.2021.06.001
Perez Ortiz, J. M., and Swerdlow, R. H. (2019). Mitochondrial dysfunction in Alzheimer’s disease: role in pathogenesis and novel therapeutic opportunities. Br. J. Pharmacol. 176, 3489–3507. doi: 10.1111/bph.14585
Prasad, K., AlOmar, S. Y., Alqahtani, S. A. M., Malik, M. Z., and Kumar, V. (2021). Brain disease network analysis to elucidate the neurological manifestations of COVID-19. Mol. Neurobiol. 58, 1875–1893. doi: 10.1007/s12035-020-02266-w
Prasad, S., Holla, V. V., Neeraja, K., Surisetti, B. K., Kamble, N., Yadav, R., et al. (2020). Parkinson’s disease and COVID-19: perceptions and implications in patients and caregivers. Mov. Disord. 35, 912–914. doi: 10.1002/mds.28088
Qiao, J., Li, W., Bao, J., Peng, Q., Wen, D., Wang, J., et al. (2020). The expression of SARS-CoV-2 receptor ACE2 and CD147, and protease TMPRSS2 in human and mouse brain cells and mouse brain tissues. Biochem. Biophys. Res. Commun. 533, 867–871. doi: 10.1016/j.bbrc.2020.09.042
Rahimi, A., Mirzazadeh, A., and Tavakolpour, S. (2021). Genetics and genomics of SARS-CoV-2: a review of the literature with the special focus on genetic diversity and SARS-CoV-2 genome detection. Genomics 113(1 Pt 2), 1221–1232. doi: 10.1016/j.ygeno.2020.09.059
Rastogi, M., Pandey, N., Shukla, A., and Singh, S. K. (2020). SARS coronavirus 2: from genome to infectome. Respir. Res. 21:318. doi: 10.1186/s12931-020-01581-z
Rathnasinghe, R., Strohmeier, S., Amanat, F., Gillespie, V. L., Krammer, F., García-Sastre, A., et al. (2020). Comparison of transgenic and adenovirus hACE2 mouse models for SARS-CoV-2 infection. Emerg. Microbes Infect. 9, 2433–2445. doi: 10.1080/22221751.2020.1838955
Rea, I. M., Gibson, D. S., McGilligan, V., McNerlan, S. E., Alexander, H. D., and Ross, O. A. (2018). Age and age-related diseases: role of inflammation triggers and cytokines. Front. Immunol. 9:586. doi: 10.3389/fimmu.2018.00586
Reichard, R. R., Kashani, K. B., Boire, N. A., Constantopoulos, E., Guo, Y., and Lucchinetti, C. F. (2020). Neuropathology of COVID-19: a spectrum of vascular and acute disseminated encephalomyelitis (ADEM)-like pathology. Acta Neuropathol. 140, 1–6. doi: 10.1007/s00401-020-02166-2
Reza-Zaldívar, E. E., Hernández-Sapiéns, M. A., Minjarez, B., Gómez-Pinedo, U., Márquez-Aguirre, A. L., Mateos-Díaz, J. C., et al. (2020). Infection mechanism of SARS-COV-2 and its implication on the nervous system. Front. Immunol. 11:621735. doi: 10.3389/fimmu.2020.621735
Rogers, K. (2022). Who Can Declare a Pandemic and What Criteria Are Required for an Outbreak to Be Called a Pandemic?. Available online at: https://www.britannica.com/story/who-can-declare-a-pandemic-and-what-criteria-are-required-for-an-outbreak-to-be-called-a-pandemic. (accessed April 7, 2022).
Romero-Sánchez, C. M., Díaz-Maroto, I., Fernández-Díaz, E., Sánchez-Larsen, Á, Layos-Romero, A., and García-García, J. (2020). Neurologic manifestations in hospitalized patients with COVID-19: the ALBACOVID registry. Neurology 95, e1060–e1070. doi: 10.1212/WNL.0000000000009937
Sait, A., Angeli, C., Doig, A. J., and Day, P. J. R. (2021). Viral involvement in alzheimer’s disease. ACS Chem. Neurosci. 12, 1049–1060. doi: 10.1021/acschemneuro.0c00719
Sanchez-Cano, F., Hernández-Kelly, L. C., and Ortega, A. (2021). The blood-brain barrier: much more than a selective access to the brain. Neurotox. Res. 39, 2154–2174. doi: 10.1007/s12640-021-00431-0
Sanclemente-Alaman, I., Moreno-Jiménez, L., Benito-Martín, M. S., Canales-Aguirre, A., Matías-Guiu, J. A., Matías-Guiu, J., et al. (2020). Experimental models for the study of central nervous system infection by SARS-CoV-2. Front. Immunol. 11:2163. doi: 10.3389/fimmu.2020.02163
Sanyaolu, A., Okorie, C., Marinkovic, A., Patidar, R., Younis, K., Desai, P., et al. (2020). Comorbidity and its impact on patients with COVID-19. SN Compr. Clin. Med. 2, 1069–1076. doi: 10.1007/s42399-020-00363-4
Schwartz, M., and Deczkowska, A. (2016). Neurological disease as a failure of brain-immune crosstalk: the multiple faces of neuroinflammation. Trends Immunol. 37, 668–679. doi: 10.1016/j.it.2016.08.001
Seaks, C. E., and Wilcock, D. M. (2020). Infectious hypothesis of Alzheimer disease. PLoS Pathog. 16:e1008596. doi: 10.1371/journal.ppat.1008596
Semerdzhiev, S. A., Fakhree, M. A. A., Segers-Nolten, I., Blum, C., and Claessens, M. M. A. E. (2022). Interactions between SARS-CoV-2 N-protein and α-synuclein accelerate amyloid formation. ACS Chem. Neurosci. 13, 143–150. doi: 10.1021/acschemneuro.1c00666
Serrano, G. E., Walker, J. E., Arce, R., Glass, M. J., Vargas, D., Sue, L. I., et al. (2021). Mapping of SARS-CoV-2 brain invasion and histopathology in COVID-19 disease. medRxiv [Preprint]. doi: 10.1101/2021.02.15.21251511
Serrano-Pozo, A., Das, S., and Hyman, B. T. (2021). APOE and Alzheimer’s disease: advances in genetics, pathophysiology, and therapeutic approaches. Lancet Neurol. 20, 68–80. doi: 10.1016/S1474-4422(20)30412-9
Sharifi, Y., Payab, M., Mohammadi-Vajari, E., Aghili, S. M. M., Sharifi, F., Mehrdad, N., et al. (2021). Association between cardiometabolic risk factors and COVID-19 susceptibility, severity and mortality: a review. J. Diabetes Metab. Disord. 20, 1743–1765. doi: 10.1007/s40200-021-00822-2
Skaper, S. D., Facci, L., Zusso, M., and Giusti, P. (2018). An inflammation-centric view of neurological disease: beyond the neuron. Front. Cell Neurosci. 12:72. doi: 10.3389/fncel.2018.00072
Skok, K., Vander, K., Setaffy, L., Kessler, H. H., Aberle, S., Bargfrieder, U., et al. (2021). COVID-19 autopsies: procedure, technical aspects and cause of fatal course. Experiences from a single-center. Pathol. Res. Pract. 217:153305. doi: 10.1016/j.prp.2020.153305
Solomon, I. H., Normandin, E., Bhattacharyya, S., Mukerji, S. S., Keller, K., Ali, A. S., et al. (2020). Neuropathological features of Covid-19. N. Engl. J. Med. 383, 989–992. doi: 10.1056/NEJMc2019373
Song, E., Zhang, C., Israelow, B., Lu-Culligan, A., Prado, A. V., Skriabine, S., et al. (2021). Neuroinvasion of SARS-CoV-2 in human and mouse brain. J. Exp. Med. 218:e20202135. doi: 10.1084/jem.20202135
Spudich, S., and Nath, A. (2022). Nervous system consequences of COVID-19. Science 375, 267–269. doi: 10.1126/science.abm2052
Strbo, N., Yin, N., and Stojadinovic, O. (2014). Innate and adaptive immune responses in wound epithelialization. Adv. Wound Care 3, 492–501. doi: 10.1089/wound.2012.0435
Sun, S. H., Chen, Q., Gu, H. J., Yang, G., Wang, Y. X., Huang, X. Y., et al. (2020). A mouse model of SARS-CoV-2 infection and pathogenesis. Cell Host Microbe 28, 124.e–133.e. doi: 10.1016/j.chom.2020.05.020
Sungnak, W., Huang, N., Bécavin, C., Berg, M., Queen, R., Litvinukova, M., et al. (2020). SARS-CoV-2 entry factors are highly expressed in nasal epithelial cells together with innate immune genes. Nat. Med. 26, 681–687. doi: 10.1038/s41591-020-0868-6
Sweeney, M. D., Zhao, Z., Montagne, A., Nelson, A. R., and Zlokovic, B. V. (2019). Blood-brain barrier: from physiology to disease and back. Physiol. Rev. 99, 21–78. doi: 10.1152/physrev.00050.2017
Tan, L. Y., Komarasamy, T. V., and Rmt Balasubramaniam, V. (2021). Hyperinflammatory immune response and COVID-19: a double edged sword. Front. Immunol. 12:742941. doi: 10.3389/fimmu.2021.742941
Tansey, M. G., and Romero-Ramos, M. (2019). Immune system responses in Parkinson’s disease: early and dynamic. Eur. J. Neurosci. 49, 364–383. doi: 10.1111/ejn.14290
Tavassoly, O., Safavi, F., and Tavassoly, I. (2020). Seeding brain protein aggregation by SARS-CoV-2 as a possible long-term complication of COVID-19 infection. ACS Chem. Neurosci. 11, 3704–3706. doi: 10.1021/acschemneuro.0c00676
Tekiela, P., and Majersik, J. J. (2021). The impact of COVID-19 on developing neurologic disorders. Neurology 96, e647–e649. doi: 10.1212/WNL.0000000000011348
Tizabi, Y., Getachew, B., and Aschner, M. (2021). Novel pharmacotherapies in parkinson’s disease. Neurotox. Res. 39, 1381–1390. doi: 10.1007/s12640-021-00375-5
Tizabi, Y., Getachew, B., Csoka, A. B., Manaye, K. F., and Copeland, R. L. (2019). Novel targets for parkinsonism-depression comorbidity. Prog. Mol. Biol. Transl. Sci. 167, 1–24. doi: 10.1016/bs.pmbts.2019.06.004
Torices, S., Cabrera, R., Stangis, M., Naranjo, O., Fattakhov, N., Teglas, T., et al. (2021). Expression of SARS-CoV-2-related receptors in cells of the neurovascular unit: implications for HIV-1 infection. J. Neuroinflammation 18:167. doi: 10.1186/s12974-021-02210-2
Toscano, G., Palmerini, F., Ravaglia, S., Ruiz, L., Invernizzi, P., Cuzzoni, M. G., et al. (2020). Guillain-barré syndrome associated with SARS-CoV-2. N. Engl. J. Med. 382, 2574–2576. doi: 10.1056/NEJMc2009191
Tripathy, A. S., Vishwakarma, S., Trimbake, D., Gurav, Y. K., Potdar, V. A., Mokashi, N. D., et al. (2021). Pro-inflammatory CXCL-10, TNF-α, IL-1β, and IL-6: biomarkers of SARS-CoV-2 infection. Arch. Virol. 166, 3301–3310. doi: 10.1007/s00705-021-05247-z
Turski, W. A., Wnorowski, A., Turski, G. N., Turski, C. A., and Turski, L. (2020). AhR and IDO1 in pathogenesis of Covid-19 and the “Systemic AhR Activation Syndrome:” a translational review and therapeutic perspectives. Restor. Neurol. Neurosci. 38, 343–354. doi: 10.3233/RNN-201042
Varghese, P. M., Tsolaki, A. G., Yasmin, H., Shastri, A., Ferluga, J., Vatish, M., et al. (2020). Host-pathogen interaction in COVID-19: pathogenesis, potential therapeutics and vaccination strategies. Immunobiology 225:152008. doi: 10.1016/j.imbio.2020.152008
Vigasova, D., Nemergut, M., Liskova, B., and Damborsky, J. (2021). Multi-pathogen infections and Alzheimer’s disease. Microb. Cell Fact. 20:25. doi: 10.1186/s12934-021-01520-7
Villa, C., Rivellini, E., Lavitrano, M., and Combi, R. (2022). Can SARS-CoV-2 infection exacerbate alzheimer’s disease? An overview of shared risk factors and pathogenetic mechanisms. J. Pers. Med. 12:29. doi: 10.3390/jpm12010029
von Weyhern, C. H., Kaufmann, I., Neff, F., and Kremer, M. (2020). Early evidence of pronounced brain involvement in fatal COVID-19 outcomes. Lancet 395:e109. doi: 10.1016/S0140-6736(20)31282-4
Wan, Y., Shang, J., Graham, R., Baric, R. S., and Li, F. (2020). Receptor recognition by the novel coronavirus from wuhan: an analysis based on decade-long structural studies of SARS coronavirus. J. Virol. 94, e00127–e00220. doi: 10.1128/JVI.00127-20
Wang, D., Chen, F., Han, Z., Yin, Z., Ge, X., and Lei, P. (2021). Relationship between amyloid-β deposition and blood-brain barrier dysfunction in alzheimer’s disease. Front. Cell Neurosci. 15:695479. doi: 10.3389/fncel.2021.695479
Wang, D., Hu, B., Hu, C., Zhu, F., Liu, X., Zhang, J., et al. (2020). Clinical characteristics of 138 hospitalized patients with 2019 novel coronavirus-infected pneumonia in Wuhan, China. JAMA 323, 1061–1069. doi: 10.1001/jama.2020.1585
Wang, J., Yang, G., Wang, X., Wen, Z., Shuai, L., Luo, J., et al. (2021). SARS-CoV-2 uses metabotropic glutamate receptor subtype 2 as an internalization factor to infect cells. Cell Discov. 7:119. doi: 10.1038/s41421-021-00357-z
Wang, Q., Davis, P. B., Gurney, M. E., and Xu, R. (2021). COVID-19 and dementia: analyses of risk, disparity, and outcomes from electronic health records in the US. Alzheimers Dement 17, 1297–1306. doi: 10.1002/alz.12296
Wang, Y., Yang, Y., Ren, L., Shao, Y., Tao, W., and Dai, X. J. (2021). Preexisting mental disorders increase the risk of COVID-19 infection and associated mortality. Front. Public Health 9:684112. doi: 10.3389/fpubh.2021.684112
Wassie, G. T., Azene, A. G., Bantie, G. M., Dessie, G., and Aragaw, A. M. (2020). Incubation period of severe acute respiratory syndrome novel coronavirus 2 that causes coronavirus disease 2019: a systematic review and meta-analysis. Curr. Ther. Res. Clin. Exp. 93:100607. doi: 10.1016/j.curtheres.2020.100607
Wichmann, D., Sperhake, J. P., Lütgehetmann, M., Steurer, S., Edler, C., Heinemann, A., et al. (2020). Autopsy findings and venous thromboembolism in patients with COVID-19: a prospective cohort study. Ann. Intern. Med. 173, 268–277. doi: 10.7326/M20-2003
Xia, H., and Lazartigues, E. (2008). Angiotensin-converting enzyme 2 in the brain: properties and future directions. J. Neurochem. 107, 1482–1494. doi: 10.1111/j.1471-4159.2008.05723.x
Xu, J., Zhong, S., Liu, J., Li, L., Li, Y., Wu, X., et al. (2005). Detection of severe acute respiratory syndrome coronavirus in the brain: potential role of the chemokine mig in pathogenesis. Clin. Infect. Dis. 41, 1089–1096. doi: 10.1086/444461
Yang, L., Xie, X., Tu, Z., Fu, J., Xu, D., and Zhou, Y. (2021). The signal pathways and treatment of cytokine storm in COVID-19. Signal Transduct. Target. Ther. 6:255. doi: 10.1038/s41392-021-00679-0
Yi, Y., Xu, Y., Jiang, H., and Wang, J. (2021). Cardiovascular disease and COVID-19: insight from cases with heart failure. Front. Cardiovasc. Med. 8:629958. doi: 10.3389/fcvm.2021.629958
Yu, F., Du, L., Ojcius, D. M., Pan, C., and Jiang, S. (2020). Measures for diagnosing and treating infections by a novel coronavirus responsible for a pneumonia outbreak originating in Wuhan, China. Microbes Infect. 22, 74–79. doi: 10.1016/j.micinf.2020.01.003
Yu, Y., Travaglio, M., Popovic, R., Leal, N. S., and Martins, L. M. (2021). Alzheimer’s and parkinson’s diseases predict different COVID-19 outcomes: a uk biobank study. Geriatrics 6:10. doi: 10.3390/geriatrics6010010
Zanin, L., Saraceno, G., Panciani, P. P., Renisi, G., Signorini, L., Migliorati, K., et al. (2020). SARS-CoV-2 can induce brain and spine demyelinating lesions. Acta Neurochir. 162, 1491–1494. doi: 10.1007/s00701-020-04374-x
Zhang, L., Zhou, L., Bao, L., Liu, J., Zhu, H., Lv, Q., et al. (2021). SARS-CoV-2 crosses the blood-brain barrier accompanied with basement membrane disruption without tight junctions alteration. Signal Transduct. Target Ther. 6:337. doi: 10.1038/s41392-021-00719-9
Zhang, X., Chen, X., Chen, L., Deng, C., Zou, X., Liu, W., et al. (2020). The evidence of SARS-CoV-2 infection on ocular surface. Ocul. Surf. 18, 360–362. doi: 10.1016/j.jtos.2020.03.010
Zhang, Y., Geng, X., Tan, Y., Li, Q., Xu, C., Xu, J., et al. (2020). New understanding of the damage of SARS-CoV-2 infection outside the respiratory system. Biomed. Pharmacother. 127:110195. doi: 10.1016/j.biopha.2020.110195
Zhou, L., Xu, Z., Castiglione, G. M., Soiberman, U. S., Eberhart, C. G., and Duh, E. J. (2020). ACE2 and TMPRSS2 are expressed on the human ocular surface, suggesting susceptibility to SARS-CoV-2 infection. Ocul. Surf. 18, 537–544. doi: 10.1016/j.jtos.2020.06.007
Zhu, N., Zhang, D., Wang, W., Li, X., Yang, B., Song, J., et al. (2020). A novel coronavirus from patients with pneumonia in china, 2019. N. Engl. J. Med. 382, 727–733. doi: 10.1056/NEJMoa2001017
Zhu, Z., Lian, X., Su, X., Wu, W., Marraro, G. A., and Zeng, Y. (2020). From SARS and MERS to COVID-19: a brief summary and comparison of severe acute respiratory infections caused by three highly pathogenic human coronaviruses. Respir. Res. 21:224. doi: 10.1186/s12931-020-01479-w
Ziegler, C. G. K., Allon, S. J., Nyquist, S. K., Mbano, I. M., Miao, V. N., Tzouanas, C. N., et al. (2020). SARS-CoV-2 receptor ACE2 is an interferon-stimulated gene in human airway epithelial cells and is detected in specific cell subsets across tissues. Cell 181, 1016.e–1035.e. doi: 10.1016/j.cell.2020.04.035
Keywords: SARS-CoV-2, Alzheimer’s disease, Parkinson’s disease, neuroinvasive pathways, blood-brain barrier
Citation: Silva J, Patricio F, Patricio-Martínez A, Santos-López G, Cedillo L, Tizabi Y and Limón ID (2022) Neuropathological Aspects of SARS-CoV-2 Infection: Significance for Both Alzheimer’s and Parkinson’s Disease. Front. Neurosci. 16:867825. doi: 10.3389/fnins.2022.867825
Received: 01 February 2022; Accepted: 14 April 2022;
Published: 03 May 2022.
Edited by:
Natalia P. Rocha, University of Texas Health Science Center at Houston, United StatesReviewed by:
Ulises Gomez-Pinedo, Health Research Institute of the Hospital Clínico San Carlos, SpainRavi Kant Narayan, Dr. B. C. Roy Multispeciality Medical Research Center (Under IIT Kharagpur), India
Nicolas Alejandro Bravo-Vasquez, University of Texas Health Science Center at Houston, United States
Copyright © 2022 Silva, Patricio, Patricio-Martínez, Santos-López, Cedillo, Tizabi and Limón. This is an open-access article distributed under the terms of the Creative Commons Attribution License (CC BY). The use, distribution or reproduction in other forums is permitted, provided the original author(s) and the copyright owner(s) are credited and that the original publication in this journal is cited, in accordance with accepted academic practice. No use, distribution or reproduction is permitted which does not comply with these terms.
*Correspondence: Ilhuicamina Daniel Limón, aWxobGltb25AeWFob28uY29tLm14, ZGFuaWVsLmxpbW9uQGNvcnJlby5idWFwLm14
†These authors have contributed equally to this work and share first authorship