- 1Department of Physical Medicine and Rehabilitation, Tohoku University Graduates School of Medicine, Sendai, Japan
- 2Graduate School of Biomedical Engineering, Tohoku University, Sendai, Japan
Understanding the operation of cortical circuits is an important and necessary task in both neuroscience and neurorehabilitation. The functioning of the neocortex results from integrative neuronal activity, which can be probed non-invasively by transcranial magnetic stimulation (TMS). Despite a clear indication of the direct involvement of cortical neurons in TMS, no explicit connection model has been made between the microscopic neuronal landscape and the macroscopic TMS outcome. Here we have performed an integrative review of multidisciplinary evidence regarding motor cortex neurocytology and TMS-related neurophysiology with the aim of elucidating the micro–macro connections underlying TMS. Neurocytological evidence from animal and human studies has been reviewed to describe the landscape of the cortical neurons covering the taxonomy, morphology, circuit wiring, and excitatory–inhibitory balance. Evidence from TMS studies in healthy humans is discussed, with emphasis on the TMS pulse and paradigm selectivity that reflect the underlying neural circuitry constitution. As a result, we propose a preliminary neuronal model of the human motor cortex and then link the TMS mechanisms with the neuronal model by stimulus intensity, direction of induced current, and paired-pulse timing. As TMS bears great developmental potential for both a probe and modulator of neural network activity and neurotransmission, the connection model will act as a foundation for future combined studies of neurocytology and neurophysiology, as well as the technical advances and application of TMS.
Introduction
Neurons in the developed neocortex are highly diversified in their morphology, distribution, connection, and physiological function in the central nervous system. Approximately 70–85% of the neocortical neurons are excitatory, projecting across cortical and subcortical areas and promoting cortical signal propagation (DeFelipe et al., 2002). The remaining 15–20% are highly heterogeneous cortical inhibitory interneurons (INs) that project onto local excitatory neurons (Wonders and Anderson, 2006; Benarroch, 2013). Generally, the neocortical neural network consists of the excitatory system and the inhibitory system; the two major neurotransmitters are glutamate and GABA (Benarroch, 2013). The structure of the neocortex has been evidenced by numerous cytoarchitectonic and myeloarchitectonic research on human and other mammals; a “six-layered laminar structure” with large neuron taxonomy is reported (Brodmann, 1909; Amunts and Zilles, 2015; Palomero-Gallagher and Zilles, 2019). The resolution of the neuron landscape was further increased by neurochemical tracing of specific protein and neuropeptide expression, from which the neural response to intracellular and extracellular environmental alteration was also unveiled (Banasr et al., 2017).
The excitatory–inhibitory balance in the human primary motor cortex (M1) can also be assessed and modulated with transcranial magnetic stimulation (TMS) (Barker et al., 1985). Although the macroscopic TMS response represents the collective activity of massive neurons and neurocircuitries (Sanchez-Vives et al., 2017), the investigation of specific circuits can be achieved by varying the TMS pulse parameter and paradigm. For example, the intracortical and interhemispheric excitatory (facilitatory) and inhibitory phenomena mediated by glutamatergic and GABAergic circuitries have been widely investigated using the paired-pulse TMS paradigm (Ferbert et al., 1992; Kujirai et al., 1993). Meanwhile, epidural recordings of TMS-evoked corticospinal descending volleys, as the most direct demonstration of the TMS-evoked aggregative cortical activities, have also provided valuable evidence indicating the properties of the cortical neural circuits involved in TMS (Di Lazzaro et al., 1999; Kallioniemi et al., 2018; Higashihara et al., 2020).
Experimental evidence from TMS studies has indicated a close relationship between TMS outcomes and the cortical neural network (including the neurotransmitters glutamate and GABA) (Premoli et al., 2014; Du et al., 2018). However, as molecular and neurobiological studies mainly focus on the cytoarchitecture (Boyle et al., 2017; Llorca et al., 2019) and cytochemistry (Adotevi and Leitch, 2017) of the neocortical neurons, it remains to be elucidated how the macroscopic electrophysiological response connects and interacts with the microscopic neural circuitry. Specifically, what subsets of cortical network participate in the TMS-evoked response and contribute to the electrophysiological phenomena? Where are the TMS-related circuits and neurons located in the neocortex and how do they interact? Through what neuron population and connection does the TMS-related neural network function? Ultimately, is there a perspective able to connect the macroscopic and microscopic evidence between M1-TMS and M1 cortical neurons? To answer these key problems, an explicit model connecting the evidence from brain stimulation and cortical neural architecture is required. Indeed, establishing a micro–macro connection model between cortical neural circuits and TMS, despite the considerable difficulties so far encountered, is not only essential for exploring the TMS mechanisms in the resolution of neurons, but is also a key issue to be addressed in deciphering the topology and wiring of the normal human cortical network. As TMS is a promising neuromodulation technology applied worldwide, elucidating its underlying mechanisms is also of vital importance for optimizing the outcomes of its use in neurorehabilitation.
In the present integrative review, we focus particularly on the micro–macro connection between cortical neurons in the human motor network (with an emphasis on M1) and TMS to first address the aforementioned questions. By reviewing a large body of literature in the disciplines of neocortical neurocytology and brain stimulation, we seek to establish a model regarding the underlying neural mechanisms of TMS, which is able to bridge the TMS-related electrophysiological and cellular mechanisms while fitting the major experimental evidence. In particular, we reviewed a wider range of neurocytologic literature that seems to have less relationship with TMS for two reasons. First, concerning the shared common research target of the two disciplines—the cortical neurons, we believe that with the knowledge of the microstructure of the cortex, a great leap forward in neuroscience can be expected if we can connect the micro-macro evidence regarding the cortical neurons. Second, by reviewing the developmental and taxonomical evidence, we intend to generate a wider fundament for the micro-macro connection at the preliminary stage, as there may be further involvement of the neuron types other than those included in our preliminary model that has not been identified.
Methods
Search Strategy
Based on the integrative review strategy, the author DT searched the electronic databases of PubMed, PubMed Central (PMC), Science Direct, Scopus, Google Scholar, and Web of Science in December 2021. The search terms included the combinations of variants of: “GABA,” “glutamate,” “cortical neurons,” “cortical layers,” “transcranial magnetic stimulation,” “TMS,” “paired-pulse TMS,” “motor cortex,” “I-wave,” “interneurons,” and “cortical neuron hierarchy.” The returned articles were then screened according to the criteria for inclusion and exclusion, duplicates or articles that disqualify the inclusion criteria were excluded.
Inclusion and Exclusion Criteria
The general inclusion criteria required: (1) peer-reviewed publications (or preprints) with full-text available; (2) publications in English and Japanese; (3) published between January 1, 1993 and November 30, 2021. Criteria for inclusion of neurocytologic articles included (1) human or animal studies with clear ethical statements; (2) study on healthy human or animals (non-pathological studies); and (3) study on the cortical neurons and interneurons. The inclusion criteria of TMS studies required (1) study in healthy adults; (2) TMS of the motor cortex (M1 hand area and the motor-related areas); (3) study with no involvement of neuroplasticity (which can possibly affect the original properties of TMS protocols); and (4) original experimental research (not systematic reviews, dissertations or letters to the editor).
Cortical Neurons
Development, Taxonomy, and Morphology
Evidence from mammalian studies has identified that cortical neurons are originally generated at subcortical regions. For excitatory pyramidal neurons (PNs), the developmental origin is known as the radial glia progenitor cells in cortical proliferative pool, located in the proliferative zones (ventricular and subventricular zone, VZ, and SVZ) in primates (Charvet et al., 2017). The newborn neurons migrate under the guidance of the radial fiber through four stages of mitosis and morphological changes and finally arrive at the target neocortical plates (Kriegstein, 2005). For inhibitory INs, a generally accepted origin is the ganglionic eminence (GE, consisting of lateral, medial, and caudal parts: LGE, MGE, and CGE, respectively) in VZ and SVZ. Neuroblasts generated in the GE migrate to the different layers of the neocortex until postnatal stages (Scarabello et al., 2021). In the prenatal and postnatal human brain, neuroblasts migrate from the GE to the neocortex following two main migrating streams, known as the rostral migratory stream (RMS) and the medial migratory stream (MMS) (Sanai et al., 2011; van Strien et al., 2011), and finally differentiate into INs (Faux et al., 2012). The MMS then diverges from the main stream of RMS (heading toward the olfactory bulb) at the anterior horn of the lateral ventricle and heads toward the ventromedial prefrontal cortex, comprising the inhibitory INs in the neocortex (Kubo and Deguchi, 2020).
Regarding the taxonomy of motor cortex neurons in developed rodents and primates, a transcriptome-based taxonomy was recently proposed (Callaway et al., 2021). This hierarchy has emerged from numerous visualization and tracing methodologies for neocortical neurons, including immunocytochemistry (using whole-tissue sections) (Gu et al., 2017), immunohistochemistry (using isolated cells) (Ding et al., 2021), electron (fluorescence) microscopy (Tiveron et al., 2016; Glausier et al., 2021), and single-nucleus RNA sequencing (Krienen et al., 2020). Neocortical neurons are generally categorized by the main neurotransmitter: glutamate for the excitatory PNs and GABA for the inhibitory INs. GABAergic INs are further divided by the expression of specific proteins or neuropeptides, whereas glutamatergic neurons are classified by their laminal distribution and projection patterns (Tremblay et al., 2016; Callaway et al., 2021; Langseth et al., 2021; Figure 1). While the laminar layout and neuron taxonomy are similar across neocortical areas (Douglas and Martin, 2004; Kast and Levitt, 2019), the human primary motor cortex (Brodmann area 4), in particular, distinguishes itself from other cortical areas by the unique presence of giant PNs in layer 5b (L5b, Betz cells), in which the descending axons constitute the core of the corticospinal tract (CST) (Betz, 1874). As further detailed discussions of the development, genetic properties, and pathological alterations of neocortical neurons are beyond the scope of the present review, interested readers are redirected to the comprehensive reviews in these fields (Hu et al., 2017; Tasic et al., 2018; Bakken et al., 2021; Callaway et al., 2021; Matho et al., 2021).
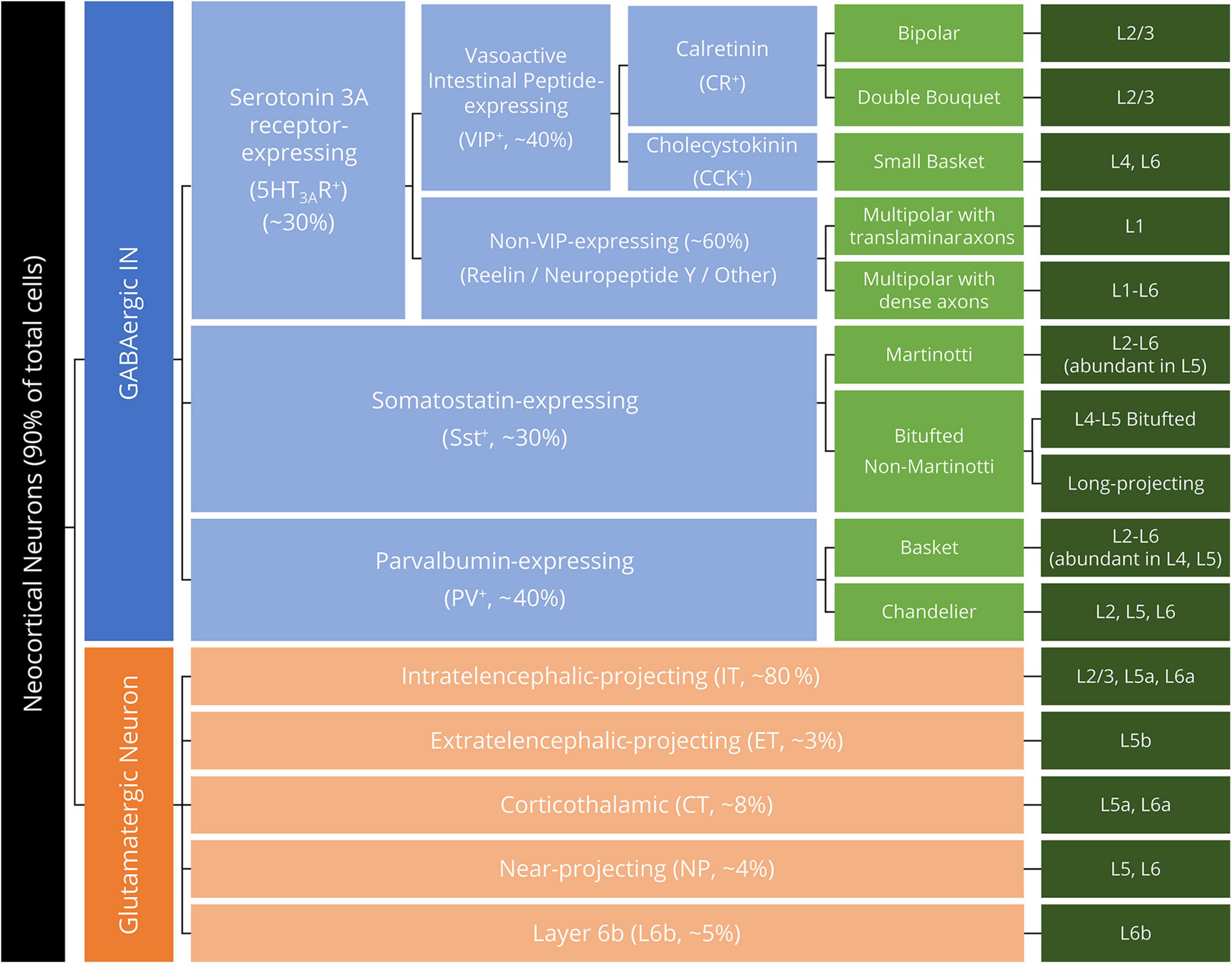
Figure 1. Hierarchical neuron taxonomy of the human motor cortex. All neurons are classified by the neurotransmitter glutamate and GABA as glutamatergic (excitatory) neurons (orange boxes) and GABAergic interneurons (INs, blue boxes). For the morphologically diversified INs, the morphological feature of the IN is specified in light green boxes. Dark green boxes denote the laminar distribution (layer, L) of the listed neuron subclasses.
Neurotransmitter: Glutamate
Intratelencephalic Projecting Neurons
Located mainly in L2/3, L5a, and L6a, the IT neurons constitute approximately 80% of the PN population (Bakken et al., 2021; Zhang et al., 2021). In the human motor cortex, the relative proportion of IT neurons is significantly larger than that in mice and marmosets (Bakken et al., 2021), suggesting the essential contribution of IT neurons in the control of human movement. IT neurons can be divided according to the projection area into corticofugal projection neurons (projecting to subcortical structures in both hemispheres), associative projection neurons (projecting to other cortical areas in the ipsilateral hemisphere), and commissural projection neurons (projecting to homologous cortical area in the contralateral hemisphere) (Fame et al., 2011; Greig et al., 2013; Sohur et al., 2014). The L2/3 cortical IT neurons in the motor cortex are mostly commissural and associative projection neurons, which interconnect by synapses, receive input from other cortical areas, and project to the bilateral cortex and striatum or target the L5 extratelencephalic projecting (ET) and IT neurons as a pivot in the sensorimotor integration (Mao et al., 2011; Hooks et al., 2013; Lin et al., 2018). IT neurons in L5a and L6a are mostly corticofugal projecting, with different developmental and functional properties from L2/3 IT neurons (Greig et al., 2013). In the human temporal cortex, the L5 IT neurons display tuftless dendritic morphology and nearby projection property (Kalmbach et al., 2021), whereas the projection of L6 IT neurons in the human motor cortex remains largely unknown. Together, L2–6 IT neurons send their projections within the telencephalon, targeting the bilateral neocortex, striatum, and subcortical structures and, in particular, the contralateral neocortex (Brown and Hestrin, 2009). L2/3 commissural projecting IT neurons of the motor cortex project through the corpus callosum and other white matter (WM) commissures (e.g., the anterior commissure), forming axodendritic synapses at all cortical layers (yet dominating in L2/3) of the contralateral hemisphere (Lin et al., 2018; Tasic et al., 2018).
Extratelencephalic Projecting Neurons
Extratelencephalic projecting neurons, also known as the pyramidal tract neurons (PTN) in the M1, concentrate in L5b and contribute to the CST. In primates, the quantity of ET neurons is approximately eightfold lower than that of L2/3 IT neurons. With a more diffuse projection than the IT neurons, ET neurons target not only the telencephalon but also the ipsilateral subthalamic nucleus, brainstem, and spinal cord (Kita and Kita, 2012; Callaway et al., 2021). Importantly, ET neurons contain no interhemispheric projection (projection to the contralateral telencephalon), as proven by the retrograde tracing study by Reiner et al. (2003). However, in the rodent motor cortex, ET neurons received interhemispheric monosynaptic excitatory input from contralateral motor-related areas (with weak input from somatosensory areas), as reported in a retrograde labeling study (Mao et al., 2011; Hooks et al., 2013). While the input to L5 ET neurons in the human motor cortex is not clearly addressed, it is shown that ET neurons can be excited by receiving integrated signal from multiple IT neurons in both hemispheres, although the signal propagation is irreversible due to the nature of synapse connection. That is, whereas IT neurons can excite ET neurons, ET neurons cannot excite IT neurons in reverse. As a subset of L5 ET neurons, the anatomically and physiologically specialized Betz cells were also found in the premotor cortex (PM) and supplementary motor area (SMA) of the human brain with similar transcriptomic definition (Rivara et al., 2003; Bakken et al., 2021; Takata et al., 2021), consistent with the reported origin from the premotor cortex and the supplementary motor area of the CST (Rouiller et al., 1996; Seo and Jang, 2013). Collectively, L5 ET neurons in the motor cortex receive multiple inputs from both hemispheres and have a wide-range projection to cortical and subcortical areas. Additionally, L5 ET neurons in the motor-related areas (M1, PM, and SMA) share cellular similarity and may have close internal interactions so as to function as the cortical motor network.
Corticothalamic Neurons
Corticothalamic neurons project to the thalamus exclusively from L5a/L6a of the neocortex while connecting with IT neurons in the nearby lamina (i.e., L5a and L6a IT neurons) (Behrens et al., 2003; Kim et al., 2014; Yamawaki and Shepherd, 2015; Callaway et al., 2021). In the multiple circuit-analysis research by Yamawaki and Shepherd (2015), it was observed that the CT–IT connection presented only in L6, but not in L2/3. Although CT may interact with PTN through disynaptic circuitry involving an inhibitory IN, the direct connection of CT-PTN is almost absent, despite the vicinity of L6a, L5a, and L5b. However, in the mouse barrel cortex, CT neurons are reported to receive strong and focused innervation from L4 PN (Tanaka et al., 2011; Qi and Feldmeyer, 2016); however, whether this innervation exists in the motor cortex remains unknown. Moreover, despite the thalamus acting as a pivot of both the ascending and descending pathways and processing both afferent and efferent information (McFarland and Haber, 2002; Behrens et al., 2003), thalamocortical projections have almost no interaction with the projections of CT neurons at the ventrolateral nucleus (Yamawaki and Shepherd, 2015), consistent with the structural model of the cortico-thalamo-cortical loop (for a recent review, see Shepherd and Yamawaki, 2021). In terms of interhemispheric connectivity, while Molinari et al. (1985) reported bilateral components of the CT fibers from the motor cortex in rats and cats, a recent cell census proposed by the Brain Initiative Cell Census Network (BICCN) suggested that CT projections contain no interhemispheric components (Callaway et al., 2021). For interaction with INs, L6 CT neurons innervate local parvalbumin-expressing (PV+) and somatostatin-expressing (Sst+) INs, resulting in general inhibition in superficial layers (Baker et al., 2018). With a lower amount than IT neurons, the CT neurons with distinct neural properties are considered the third major subclass of neocortical principal neurons after IT and ET.
Near-Projecting and L6b Neurons
The L5/6 NP neurons and L6b neurons are mostly defined by transcriptomic signatures (Tasic et al., 2018; Langseth et al., 2021); their physiological properties are largely unknown. The L5/6 NP neurons, populating about 4% of the entire neocortical PNs, have no long-distance projections like the IT, ET, and CT neurons (Tasic et al., 2018; Bakken et al., 2021). Moreover, the L6b neurons (comprising 5% of total PN population) in the mouse visual cortex have been observed to send projections to the thalamus or anterior cingulate cortex (Tasic et al., 2018). However, there is no evidence reporting the physiology and function of the two subsets of principal neurons in the human neocortex owing to the difficulty in assessing activities in deep cortical layers in vivo. A viable investigation method analyzing L6b and NP neuron activity is needed to unveil their connectivity and physiological roles in the neocortical neural circuits.
Neurotransmitter: γ-Aminobutyric Acid (GABA)
Parvalbumin-Expressing (PV+) Inhibitory Interneuron
The fast-spiking PV+ IN is the most common and well-studied neocortical inhibitory IN, distributed in L2–L6 of the neocortex (Bakken et al., 2021). PV+ INs stem from MGE, and can be morphologically divided into two subtypes: the basket cell (BC, greater quantity) and the chandelier cell (ChC, smaller quantity). Both BCs and ChCs have fast-spiking electrophysiological properties, yet the firing pattern (latency, frequency, etc.) differed between the two subtypes (Povysheva et al., 2013). For innervation target on the PN, it was reported that BCs targeted the cell soma and proximal dendrites, whereas ChCs innervated the axon initial segment (AIS) of the PN (Szentagothai and Arbib, 1974; Inan and Anderson, 2014).
Contrary to the common inhibitory effects elicited by BC, the postsynaptic effects elicited by ChCs are more intriguing. A series of rodent and human studies reported that ChCs received input from the PN of the ipsilateral and contralateral hemispheres (Lu et al., 2017). Woodruff et al. (2011) performed a patch-clamp study and reported that L2/3 ChCs were activated by the electrical stimulation of L1 (where most of the ChC dendrites reside), with different timing-dependent feedforward effects of both inhibition (ChC activation 5 ms prior to PN activation) and facilitation (ChC activation 15–30 ms prior to PN activation). Consistent with this study (Woodruff et al., 2011), ChCs were reported to excite postsynaptic PNs by depolarizing the AIS under certain postsynaptic membrane potential states (Szabadics et al., 2006; Woodruff et al., 2009, 2011), despite its classification as “GABAergic inhibitory IN.” In the developed neocortex, the existence of axo-axonic ChC provides an important strategy to optimize PN output via GABAA-receptor-induced excitation, as a specific GABAA receptor (GABAAR-α2) is selectively enriched in the AIS of neocortical PNs (Gulledge and Stuart, 2003; Howard et al., 2005; Szabadics et al., 2006; Gao and Heldt, 2016).
Nevertheless, it should be noted that neocortical BCs stem from two different origins of CGE and MGE (Marin-Padilla, 1972; Butt et al., 2005; Gelman et al., 2011), with those from CGE expressing cholecystokinin (CCK), a neuropeptide of serotonin 3A Receptor (5HT3AR) family. PV+ BCs exceed CCK+ BCs in both quantity and size (Wang et al., 2002), and BCs are further classified as nest basket cells (mainly PV+), large basket cells (mainly PV+), and small basket cells (mainly CCK+) (for reviews, see Druga, 2009; Armstrong and Soltesz, 2012). Further description of CCK+ basket cells can be found in Section “Serotonin 3A Receptor-Expressing (5HT3AR+) Inhibitory Interneuron.”
Somatostatin-Expressing (Sst+) Inhibitory Interneuron
The second major GABAergic IN subclass (∼30% of the entire IN population) in the human motor cortex resides in L2–L6, expressing the neuropeptide somatostatin (Kowall and Beal, 1988; Wonders and Anderson, 2006; Bakken et al., 2021). Sst+ INs stem from MGE and predominantly consist of non-fast-spiking Martinotti cells and a minor proportion of non-Martinotti cells, with the latter demonstrating diverse morphological properties such as bitufted cell soma and long-range axonal projections (Rudy et al., 2011; Jiang et al., 2015; Tremblay et al., 2016; Urban-Ciecko and Barth, 2016).
The majority of Martinotti cells concentrates in L5 and L6, receiving excitatory synaptic input from L5 PN axon collaterals and forming synapses with other PN dendrites, and forming disynaptic inhibitory circuits between neighboring L5 PNs and translaminar recurrent inhibitory circuits targeting L2/3 IT neurons (Wang et al., 2004; Silberberg and Markram, 2007; Kätzel et al., 2011; Zolnik et al., 2020). In frequency-dependent disynaptic inhibition, it has been reported that fast (immediate) disynaptic inhibition was mediated by fast-spiking PV+ basket cells, whereas non-fast-spiking Martinotti cells mediated delayed disynaptic inhibition between L5 ET neurons (Silberberg and Markram, 2007; Berger et al., 2009; Obermayer et al., 2018).
In contrast to Sst+ Martinotti cells targeting L1, the quasi-fast spiking (fast-spiking pattern intermitted by random silences) Sst+ non-Martinotti cells targeting L4 were discovered over a century later than Martinotti cells (Ma et al., 2006; Xu et al., 2013). Of note, although the human M1 has been canonically characterized by the lack of the specific Layer 4, the existence of L4 neuron phenotypes in M1 (as in the middle temporal gyrus) was proven by the recent transcriptomic profiling study by BICCN (Bakken et al., 2021), aligning with the histological evidence from animals and humans (Cajal, 1899; Yamawaki et al., 2014; Barbas and García-Cabezas, 2015). Morphologically, non-Martinotti cells have ramified axons inside L4, and their dendritic projections do not extend to L1 (Muñoz et al., 2017; Scala et al., 2019). Thus, it is inferable that this type of non-Martinotti cells may coexist with L4-phenotype neurons scattered in L2/3 and L5 of the human M1. For synaptic connectivity, Sst+ non-Martinotti cells in L4 of mouse somatosensory cortex made more connections with PV+ INs than with PNs and this connection caused disinhibition of PV+ INs, controlling the processing of afferent input from the thalamus (Xu et al., 2013; Nigro et al., 2018). However, the specific role of non-Martinotti cells in the human motor cortex is not clear, as the presence of L4 in the motor cortex has not yet been firmly defined.
Serotonin 3A Receptor-Expressing (5HT3AR+) Inhibitory Interneuron
Apart from PV+ and Sst+ INs, almost all of the remaining 30% of cortical GABAergic INs express 5HT3AR, reside in the supragranular layers (L1–L3, especially abundant in L1) and specifically target PV+ and Sst+ INs in the neocortex (Chameau and van Hooft, 2006; Lee et al., 2010; Rudy et al., 2011; Bakken et al., 2021). 5HT3AR+ INs originate from CGE, and correspondingly, CGE-derived neurons specifically express 5HT3AR as the molecular marker (Wester and McBain, 2014; Wester et al., 2019). Similar to the heterogeneity of PV+ and Sst+ INs from MGE, CGE-derived INs also have highly diverse morphology and electrophysiological profiles (Lee et al., 2010; Miyoshi et al., 2010; Vucurovic et al., 2010). For example, in a genetic fate mapping study of CGE-derived INs by Miyoshi et al. (2010), nine types of morphologically and physiologically different INs were identified in the mice somatosensory cortex. In the same study (Miyoshi et al., 2010), it was also reported that around 75% of CGE-derived INs expressed reelin and vasoactive intestinal peptides (including calretinin and CCK, under the category of 5HT3AR), and had bipolar, single/double-bouquet, or multipolar morphology. The reelin-expressing INs were found to be late-spiking neurogliaform cells and INs with single bouquet or multipolar morphology, whereas INs expressing calretinin and CCK were burst-spiking double-bouquet or bipolar INs. While the double-bouquet cells mainly target fast-spiking PV+ BCs and ChCs (Hioki et al., 2018), single bouquet cells were reported to form synapses that selectively inhibited the activity of L2/3 INs, thus disinhibiting L5 ET neurons (Jiang et al., 2013). In particular, the multipolar CCK-expressing INs were classified as small basket cells, for its morphological and target resemblance with PV+ BCs (Armstrong and Soltesz, 2012; Goff and Goldberg, 2019). However, CCK+ BCs significantly differed from PV+ BCs by their high response sensitivity to acetylcholine, serotonin, or cannabinoids (Földy et al., 2007; Lee et al., 2010). Furthermore, CCK+ BCs had a non-fast-spiking profile with asynchronous GABA release onto the postsynaptic neurons, whereas PV+ BCs are fast-spiking cells with high GABA release synchronicity (Daw et al., 2009; Bartos and Elgueta, 2012).
Hypothetical Neuron Landscape of Human M1
Based on the reviewed literature of human and animal studies, we have summarized the neurocytological evidence into a schematic figure of the hypothetical neuronal landscape in the human motor cortex (Figure 2). As research evidence regarding the human motor cortex remains low in quantity, we therefore integrated the collective evidence from both animal and human studies that is included in the literature review of section 3 Cortical Neurons into Figure 2. The figure summarizes the motor cortex neuron subtypes, basic morphology, distribution in the six-layer structure, the intracortical and interhemispheric synaptic connection between neuron subtypes, and the evidenced communication pathways (input and output) with other cortical areas. All the displayed elements and relevant citations can be found in the text of section 3 Cortical Neurons.
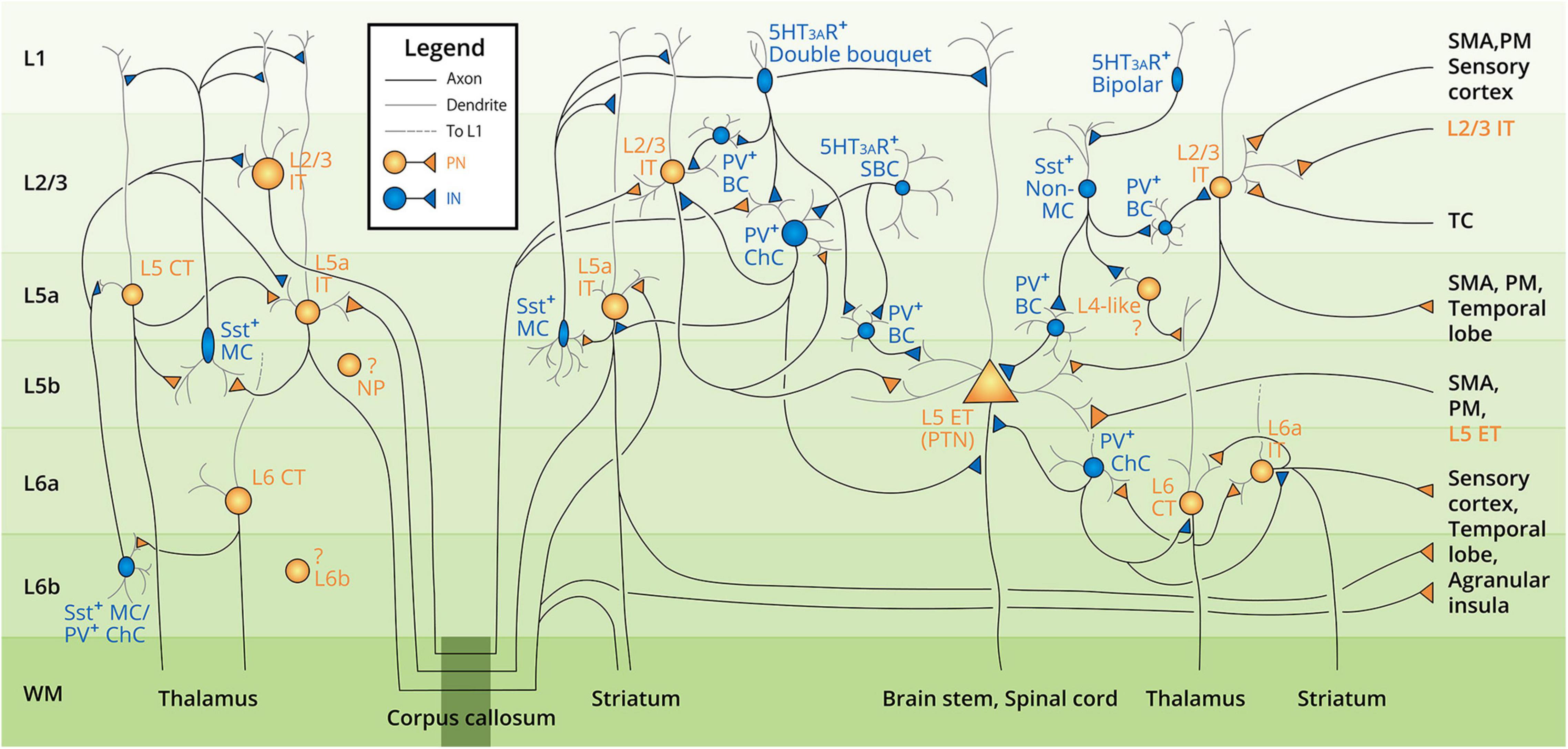
Figure 2. Summarized diagram of motor cortex neocortical neuron landscape and circuit connectivity. Neuron subtypes are labeled next to the cell soma from layer 1 to layer 6b (L1–L6b, labeled at the left side of the diagram), with the text color corresponding to the neuron type (orange–excitatory pyramidal neuron, PN; blue–inhibitory interneuron, IN). Gray lines denote the dendrites; black lines denote the axons. The target of the descending axons is indicated in the white matter (WM) area. Intracortical and interhemispheric connections are denoted by the division of the corpus callosum (dark green box at the bottom of the diagram). Note that all the neurons and connections are presented in both hemispheres. The question mark denotes the unclarified connectivity of the corresponding neurons. Abbreviations of the neuron subtypes can be found in Figure 1. Other abbreviations: MC, Martinotti cell; ChC, chandelier cell; BC, basket cell; SBC, small basket cell; PTN, pyramidal tract neuron; SMA, supplementary motor cortex; PM, premotor cortex; TC, thalamocortical axon.
Neural Activities Involved in Transcranial Magnetic Stimulation
Assessment of Transcranial Magnetic Stimulation-Related Neural Activities
The TMS-related neural activities can be measured at different levels along the descending of the efferent motor pathways (predominantly the CST). The neurophysiological measurements in most TMS and other non-invasive brain stimulation (NIBS) studies, as sorted by descending order along the CST, include (1) spinal cord-evoked potential (SCEP) at the cervical spinal cord level, (2) peristimulus time histogram (PSTH) from the single motor unit (SMU) recordings, and (3) motor-evoked potential (MEP) at the collective muscle output level.
Measurements along the descending pathways have revealed the different properties of TMS-related neural activities. Firstly, the transcranial electric stimulation (TES)-/TMS-evoked descending volleys recorded by epidural electrodes as the SCEP provide direct and valuable evidence of TMS-evoked general output from the brain (Patton and Amassian, 1954; Burke et al., 1993). Despite the invasiveness and subject limitation (patients with implanted epidural electrodes only) of the SCEP recordings, the discovery of direct and indirect waves (D-waves and I-waves) have illustrated the cortical network underlying TES/TMS in a robust dimension, inspiring numerous hypotheses and insights into the neural circuitries related to TMS and TES [for related SCEP reviews see Izumi (2001), Di Lazzaro et al. (2007, 2012, 2017), Di Lazzaro and Ziemann (2013), Di Lazzaro and Rothwell (2014), Ziemann, 2020]. Secondly, TMS-evoked SMU activity measured from a needle electrode inserted into the target muscle revealed the time-course spiking patterns in the measured motor unit as the PSTH. Compared with SCEP, less invasive SMU recordings can be obtained from normal subjects. Similarly, PSTH also clearly showed the arrival of D-wave and I-waves in a spatiotemporal order, similar to SCEP (Day et al., 1989). Finally, based on surface electromyography (EMG), MEP reflected the integrity and excitability of the entire motor cortex and pathways. Up to now, the majority of TMS outcomes have been measured by MEP, both in studies unveiling the mechanisms of TMS or estimating NIBS treatment effects in neurology patients. Overall, the measurement at all levels along the CST has offered complementary experimental evidence regarding the property and pattern of TMS- and TES-induced physiological process, which is largely reflected in the selectivity of different TMS pulse properties and paradigms.
Transcranial Magnetic Stimulation Pulse Property Selectivity
Altering the intrinsic properties (phasic pattern, pulse width, pulse intensity, induced current direction, etc.) of a TMS pulse resulted in outcome difference, indicating the selectivity of the underlying neural circuits to the pulse. For phasic patterns, the resting and active motor thresholds (RMT and AMT) were higher when assessed with monophasic than biphasic TMS pulses (all with a figure-of-eight coil, unless otherwise specified) [Di Lazzaro et al., 2001a; Kammer et al., 2001; Sommer et al., 2006, 2018; see also Casula et al. (2018) for contradictory results]. Notably, the optimal coil orientations contradicted each other in the monophasic and biphasic TMS pulses. In monophasic TMS, MT was lower when the induced current followed the posterior–anterior (PA) direction than the anterior–posterior (AP) direction, whereas in biphasic TMS, the MT was lower when the current flow was in the AP–PA direction (Di Lazzaro et al., 2001a; Sommer et al., 2006, 2018). Along with lower MT, biphasic TMS also yielded shorter MEP latency than monophasic TMS (Sommer et al., 2006), indicating the higher efficacy of biphasic TMS pulse in depolarizing neurons. The difference between monophasic and biphasic TMS was further supported by SCEP and EEG studies showing that the cortical origin of this difference was the neural components with different excitation threshold resulted in the response difference between monophasic and biphasic TMS (Di Lazzaro et al., 2001a; Casula et al., 2018). For pulse width, it has been shown that lengthening the pulse width decreased MT and MEP latency (Peterchev et al., 2008; D’Ostilio et al., 2016; Casula et al., 2018). Combined with coil orientation, D’Ostilio et al. (2016) and Hannah and Rothwell (2017) reported that AP-TMS with shorter pulse width (30 μs, 60 μs) resulted in longer onset latency in both MEP and SMU (PSTH) responses in comparison to a 120-μs pulse, yet no specific difference was observed in PA-TMS. For pulse intensity, SCEP recordings indicated that subthreshold pulses may have an impact on the intracortical circuits (Di Lazzaro et al., 1998b), which in subsequently became the theoretical basis of the well-known short interval intracortical inhibition (SICI) paradigm (Kujirai et al., 1993; Izumi, 2001; see Section “M1 Paired-Pulse Transcranial Magnetic Stimulation”). Moreover, given that the TMS stimulation depth in the cortex is correlated with pulse intensity, we speculate that the difference between AMT and RMT-TMS may not merely result from motor drive but also subject to the stimulation depth of the pulse. However, as it remains difficult to investigate the influences of the motor drive independently, we therefore do not make further speculations on the impact of AMT-TMS. Finally, in terms of current direction, the reason that the TMS-evoked descending volleys differed significantly when altering current direction remains enigmatic [for recent reviews, see Ziemann (2020), Opie and Semmler (2021)]. Until now, studies measuring SCEP, PSTH, and MEP (Day et al., 1989; Sakai et al., 1997; Di Lazzaro et al., 1998a,b, 2001a,2001b,2004; Ni et al., 2011a) have demonstrated that (1) lateromedial (LM)-TMS recruited D-waves at the lowest intensity and had the shortest MEP/PSTH onset latency among all directions; (2) PA-TMS recruited both early and late I-waves at a lower intensity while D-waves emerged as the intensity increased, with a 1-ms MEP/PSTH onset delay compared to LM-TMS; and (3) AP-TMS showed distinct recruitment of late I-waves in SCEP (which may differ from that evoked by PA-TMS in neural origin), had the highest threshold, the longest MEP/PSTH onset latency, and more functional relevance. Overall, the most agreed opinion on the TMS current direction is that varying coil orientation can induce a remarkable change in the recruited neural components (neurons, synapses, axons), and can thus alter all aspects of the outcome. However, it should be noted that the I-waves recruited by different TMS forms (phasic pattern, pulse width, pulse intensity, and current direction) may originate from different neuro populations of the motor cortex, as evidence exists that the SCEP recordings of monophasic PA- and AP-TMS, as well as by biphasic PA-AP pulse show difference in latency and frequency of the I-waves (Di Lazzaro et al., 2017). Specifically, monophasic PA-TMS preferentially evokes an I1-wave at threshold intensity, while AP-TMS preferentially recruits I-waves with lower frequency and longer latency. Although PA-TMS at high intensity also evokes later I-waves, the neural population activated by the two pulses is thought to be different, despite the possibility that these circuits may overlap.
Transcranial Magnetic Stimulation Paradigm Selectivity
Based on the experimental evidence, we present a comprehensive schematic of TMS paradigm selectivity in Figure 3, showing the phenomenal properties, mediating neurotransmitter, and interactions of the common TMS paradigms. As the paradigm selectivity is mostly reflected in paired-pulse TMS, we have divided the paired-pulse TMS experimental evidence according to the stimulation site and discussed the selectivity of M1 paired-pulse TMS and motor network dual-site TMS, respectively.
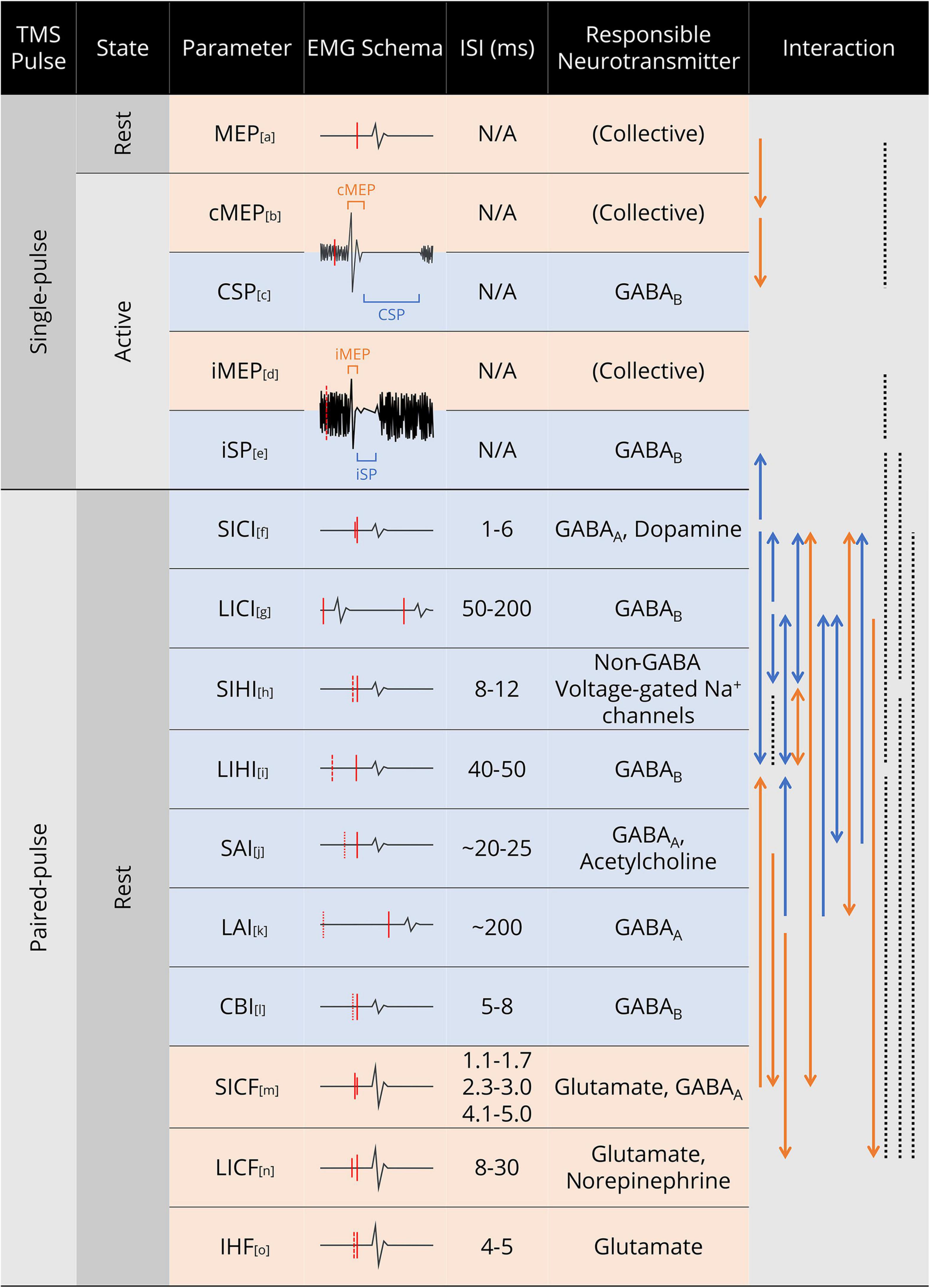
Figure 3. Summary of common TMS paradigms and parameters. In the EMG Schema column, red solid line denotes TMS pulse; red dashed line denotes TMS pulse in the contralateral hemisphere, with the line length indicating stimulus intensity; red dotted line denotes median nerve electric stimulus in SAI and long interval afferent inhibition (LAI), and cerebellar stimulus in CBI. In the Interaction column, solid line denotes the relationship between the two parameters at both ends; dotted line denotes no interaction/correlation was observed between the two parameters; arrow denotes the direction of the interaction (facilitation/inhibition); for line colors, orange denotes facilitation and blue denotes inhibition. Abbreviations: cMEP, contralateral MEP; CSP, cortical silent period; iMEP, ipsilateral MEP; iSP, ipsilateral silent period; SICI, short interval intracortical inhibition; LICI, long interval intracortical inhibition; SIHI, short interval interhemispheric inhibition; LIHI, long interval interhemispheric inhibition; SAI, short latency afferent inhibition; LAI, long latency afferent inhibition; CBI, cerebellar inhibition; SICF, short interval intracortical facilitation; LICF, long interval intracortical facilitation; IHF, interhemispheric facilitation. References for the paradigms and interactions: a. (Izumi, 2001; Rossini et al., 2015); b. (Ngomo et al., 2012); c. (Orth and Rothwell, 2004); d. (Wassermann et al., 1994; Tazoe and Perez, 2014); e. (Chen et al., 2003; Jung et al., 2006); f. (Chen, 2004; Trompetto et al., 2004; Lee et al., 2007; Ni et al., 2007); g. (Daskalakis et al., 2002; Lee et al., 2007; Udupa et al., 2009); h. (Daskalakis et al., 2002; Irlbacher et al., 2007; Ghosh et al., 2013); i. (Chen, 2004; Lee et al., 2007; Udupa et al., 2010); j. (Udupa et al., 2009; Chen, 2017; Saravanamuttu et al., 2021); k. (Trompetto et al., 2001; Sailer et al., 2002; Kobayashi et al., 2003); l. (Fernandez et al., 2018; Hassan et al., 2019); m. (Avanzino et al., 2007; Chen, 2017; Qasem et al., 2020); n. (Kobayashi et al., 2003; Chen, 2004; Lee et al., 2007; Udupa et al., 2010); o. (Hanajima et al., 2001; Baumer et al., 2006; Belyk et al., 2021).
M1 Paired-Pulse Transcranial Magnetic Stimulation
M1 paired-pulse TMS paradigms have been widely used to investigate cortical facilitation and inhibition. Although studies using multipulse paradigms have also been proposed, the basic strategy of multipulse protocols can be regarded as an integration of two or more single- and paired-pulse paradigms. Supported by pharmacological evidence [for reviews, see Ziemann et al. (2015), Darmani and Ziemann (2019)], it has been widely accepted that different TMS paradigms preferentially activate different excitatory/inhibitory circuits (Figure 3). As cortical inhibition has been extensively investigated and the results are highly systematic, in the present review, we summarize the patterns and interaction of cortical inhibition in Figure 3, and we specifically focus the discussion on cortical facilitation phenomena, which, at present, remain poorly understood.
As presented in Figure 3, cortical inhibition has been extensively investigated and evidenced to have relationship with cortical GABA activity, which can alternatively be assessed non-invasively using magnetic resonance spectroscopy (MRS). Specifically, the prior study that connected TMS and MRS measurements have revealed that SICI at 1-ms ISI was negatively correlated with GABA level in the sensorimotor cortex (SMC GABA level) while SICI at 2.5-ms ISI did not show specific correlation (Stagg et al., 2011). Accordingly, the authors speculated that the 1-ms SICI and SMC GABA level correlation may reflect extra-synaptic GABA tone, which is different from those involved in 2.5-ms SICI. A subsequent study that investigated the comprehensive relationship between TMS-measured SICI, LICI, and CSP supported the previous results, showing no relationship between 3-ms SICI, 100-ms LICI, and SMC GABA level (Tremblay et al., 2012). Tremblay et al. also reported a moderate positive correlation between CSP duration and SMC glutamate + glutamine level, as well as a positive correlation of SMC GABA and glutamate levels. Based on the prior pharmaceutical evidence that the 2–4-ms SICI may reflect low-threshold GABAA receptor activity (Ilic et al., 2002), it can therefore be inferred that the mechanisms of 1-ms SICI and 2–4-ms SICI may also come from different ways that GABA acts upon the neurons (i.e., extra-synaptic tone or GABA receptor activity), even though the targeting neurons may be the same. However, contrasting results emerged from recent studies investigating the effect of aging. Hermans et al. (2018) reported reduction of 1- and 3-ms SICI in the group of older adults with no alteration of SMC GABA level compared with younger adults, along with no correlation observed between SICI and SMC GABA level in both groups. Additionally, another study reported reduced SMC GABA level in older adults compared with younger population, along with the task-related SICI (3-ms ISI) reduction of the dominant hemisphere. Similarly, no correlation was found between SICI and MRS outcomes (Cuypers et al., 2020). Regarding the disconnection between MRS-measured SMC GABA level and 2–4-ms SICI outcomes, two reasons can be considered. Firstly, as the MRS measures the general GABA level of the sensorimotor cortex due to the minimum of a 3 × 3 × 3-cm voxel size to acquire reliable outcome (Mullins et al., 2014), possibility exists that the GABA level from the somatosensory cortex (included in MRS outcomes) may affect the MRS-TMS correlation while the paired-pulse TMS reflects mainly the GABA activity in the M1. Secondly, since TMS is an external magnetic stimulation measured through the CST output (MEP, SCEP, etc., reflecting merely the CST-related pathway GABA activity) and MRS outcomes reflect a general GABA level within the SMC, the correlation can be blurred by the possibility that MRS outcomes may come from circuits and neural populations that are not involved in TMS.
Apart from the inhibitory neural circuits, excitatory circuits give rise to paired-pulse cortical facilitation phenomena, which can be subdivided regarding the paired-pulse interstimulus interval (ISI) into (1) short interval intracortical facilitation (SICF), (2) long interval intracortical facilitation (LICF), and (3) interhemispheric facilitation (IHF) (Figure 3). For SICF, it is reported that the ISI with peak MEP facilitation was consistent with the timings of the I-wave; thus, SICF has been used widely as a non-invasive method to evaluate I-wave recruitment, acting as a substitute for invasive SCEP and SMU recordings (Tokimura et al., 1996; Ziemann et al., 1998; Hannah and Rothwell, 2017; Van den Bos et al., 2018; Ziemann, 2020). However, the controversial results from the SICF recruitment curve (the curve plot of SICF-MEP amplitude in different ISIs) in the AP and PA directions shed brought to light some uncertainty in the correspondence between I-waves and the SICF curve. Specifically, the SICF curve assessed by AP-TMS showed enhanced I1-wave-latency facilitation, whereas PA-TMS yielded comparable facilitation for all I-wave latencies (Delvendahl et al., 2014), contradictory to the SCEP evidence showing preferential late I-wave recruitment by AP-TMS. Meanwhile, a recent study reported the facilitation of SICF by the presence of short interval afferent inhibition (SAI) (Saravanamuttu et al., 2021), which also contradicted the PSTH evidence showing inhibition of late I-waves (especially late I-waves in PA-TMS) by SAI (Ni et al., 2011a). Consequently, it may be problematic to analogize SICF to I-waves, because the causal link between SICF and I-wave recruitment is merely the timing consistency, which is inadequate for equalizing the two physiological processes. For LICF, the origin and underlying circuitry remains unknown, despite its early discovery in 1993 (Kujirai et al., 1993) and broad application in basic and clinical TMS studies. One of the complex conundrums regarding LICF is that almost all paradigm-interaction studies found no interaction of LICF with other TMS paradigms (Chen, 2004; Lee et al., 2007; Reis et al., 2008; Udupa et al., 2010; Ni et al., 2011c), not even corticospinal descending volleys in SCEP recordings (Ni et al., 2011b). However, an early study (Nakamura et al., 1997) reported late I-wave facilitation in the LICF paradigm, and another two (Kobayashi et al., 2003; Ni et al., 2007) reported LICF facilitation by long interval intracortical inhibition (LICI) and long interval afferent inhibition (LAI). As the cortical origin of LICF has long been known to be different from those generating the high-frequency I-waves (reflected in the SCEP) (Kujirai et al., 1993; Nakamura et al., 1997; Di Lazzaro et al., 2006), it is therefore inferable that LICF was facilitated by LICI mechanisms (upon which LICI and LAI act), possibly due to selective disinhibition of the silenced PNs by LICI. For IHF, little evidence has been provided since the report of M1-IHF (hand motor area) existence by paired-pulse TMS at an ISI of 4–5 ms (Ferbert et al., 1992; Ugawa et al., 1993; Hanajima et al., 2001). Although Sommer et al. (2012) observed inconsistent M1-IHF at 2 ms ISI paired-pulse TMS that was diminished by sodium channel blocker carbamazepine, the 2 ms ISI brought uncertainty about the observed IHF as it was significantly lower than common M1–M1 interhemispheric transfer time by TMS (8.8–12.2 ms) (Cracco et al., 1989). As IHF is thought to be masked by massive cortical inhibitory mechanisms (especially the powerful IHI), the investigation into IHF appears unviable due to its inconsistency and vulnerability to IHI in paired-pulse TMS paradigms. However, it should be noted that IHF is a phenomenon actually present in cortical activities, as the excitatory attribute of callosal fibers has long been proved (Kawaguchi, 1992; Conti and Manzoni, 1994). That is, excitatory callosal axons project to local inhibitory INs, generating IHI, which, as an alternative can be interpreted as a structure with “superficial IHI above deeper callosal excitation.” Accordingly, if the “superficial” inhibitory effects could be reduced or eliminated, IHF presence may therefore be expected and observed. This insight has gradually gathered interest, and researches attempting to measure online and offline IHF emerged in recent years. In a recent study by Belyk et al. (2021) using paired-pulse IHF with 10 and 50 ms ISI, the authors reported paradoxical facilitation presenting with increased conditioning stimulus (CS) intensity; therefore, it was suggested that when CS is sufficiently intensive, it may overwhelm the contralateral local inhibitory circuits and eventually demonstrate IHF in the common IHI paradigm. Together, we suggest that the previous consideration of “difficult to investigate” for IHF has now reached the prime stage for investigation, to determine the expected clinical application perspectives of IHF in stroke, brain injury, neurodegenerative diseases, and compensation of impaired brain function.
Motor Network Dual-Site Transcranial Magnetic Stimulation
In humans, the cortical motor network consists of M1, dorsal and ventral PM (PMd and PMv), SMA, cingulate motor area, somatosensory cortex (S1), and the inferior parietal lobule (IPL) (Volz et al., 2015; Ruddy et al., 2017). Unlike the M1 paired-pulse paradigm selectivity, dual-site TMS targeting M1 and non-M1 motor areas yielded highly stimulus intensity- and ISI-dependent results. For the PM-M1 interaction, the first report by Civardi et al. (2001) using dual-site TMS and PSTH demonstrated that the motor response of M1-test stimulus (TS) was inhibited by a preceding PMd-CS (90% AMT) at 6 ms ISI, and that when the CS intensity was increased to 110–120% AMT, the effect became facilitatory. Subsequently, MEP of M1-TS was also reported to be facilitated by a delayed ipsilateral suprathreshold PMd-CS at 1.2 and 4.4 ms ISI in humans, yet the facilitation at 1.2 ms ISI was abolished during muscle activation (note that the CS intensity, ISI timing, and discrimination in muscle activation resembled that of M1-SICF) (Ortu et al., 2008; Groppa et al., 2012a,b). For interhemispheric interaction, Baumer et al. (2006) reported IHF effects by conditioning the contralateral PMd with a subthreshold pulse of 60/80% AMT at 6/8 ms ISI, respectively. Similar to the ipsilateral PMd-M1 interaction, the IHF effect also turned into IHI when the CS intensity was set to 90–110% RMT and applied prior to the TS by an 8–10 ms ISI (Mochizuki et al., 2004). Based on this basal intracortical facilitatory and interhemispheric inhibitory effect of suprathreshold PMd-TMS, a further triple-pulse TMS study by Koch et al. (2007) revealed that conditioning the left PMd with paired-pulse TMS also altered the outcome of this basal facilitation and inhibition that PMd exerted on ipsilateral and contralateral M1. Specifically, the pairing of an 80–100% (but not 70%) RMT PMd-CS1 and a 110% PMd-CS2 by a 5 ms ISI canceled the 6 ms PMd-ipsilateral M1 facilitation (i.e., the M1-TS was applied 6 ms after CS2), as well as the 8 ms PMd-contralateral M1 inhibition at both 1 ms and 5 ms CS1-CS2 ISI. Contrary to the basal intracortical facilitatory and interhemispheric inhibitory effects that PMd exerts on M1, PMv tends to elicit more intracortical inhibitory and interhemispheric facilitatory effects on M1 than PMd, as proven by intracortical microstimulation (ICMS) study (Tokuno and Nambu, 2000; Côté et al., 2017), which suggests a substantial functional difference between PMv and PMd. Correspondingly, as reported in rest-state dual-site TMS studies, conditioning ipsilateral PMv 8 ms (80% RMT) or contralateral PMv 40 ms (90 and 110% RMT) before M1-TS led to MEP inhibition (Buch et al., 2011; Côté et al., 2017; Fiori et al., 2017). For the SMA-M1 interaction, the glutamatergic connectivity between M1 and SMA has long been proven in primate and human studies (Tokuno and Nambu, 2000). In humans, this excitatory connectivity typically underpins bimanual coordination, action preparation, and motor imagery (Al-Wasity et al., 2021; Neige et al., 2021). Using dual-site TMS, the SMA-M1 facilitation was confirmed that SMA-CS (140% AMT) applied 6/7/8 ms prior to M1-TMS induced stable rest MEP facilitation at all ISIs in younger adults but not in older adults (Rurak et al., 2021a). However, another study also adopted 140% AMT SMA-CS and a subsequent M1-TS by 6/8 ms ISI, but the MEP facilitation was observed only in younger adults with 6 ms ISI; older adults showed no facilitation at any ISIs (Green et al., 2018). Overall, the experimental evidence for motor network dual-site TMS suggests a close circuit wiring inside the motor network, although the results depend highly on the dual-site TMS paradigm even when assessed in the rest state. As the task-related motor network connectivity and interaction may be much more complicated due to the huge diversity of task types, we do not perform further review of the task-dependent motor network connectivity. Nevertheless, possible reasons for this varying evidence can be summarized as (1) TMS coil size limitation and (2) results contamination by direct CST projections from the non-M1 motor areas. In (1), except for two PMd-M1 dual-site TMS studies (Groppa et al., 2012a,b) in which a customized high-focal TMS coil was used and one S1-M1 dual-site TMS study (Brown et al., 2019) that used figure-of-eight coils with an inner diameter of 25 mm, all other aforementioned dual-site TMS studies targeting M1/PM/SMA adopted a figure-of-eight coil with ≥50 mm loop diameter to perform the non-M1 TMS. Therefore, it is technically difficult to simultaneously aim M1 and ipsilateral nearby areas with two 50/70 mm coils, because the PM-M1 and SMA-M1 distances are both less than 30 mm, and even less than 20 mm in the case of ipsilateral M1-S1 (Mochizuki et al., 2004; Holmes and Tamè, 2018; Brown et al., 2019; Rurak et al., 2021b). For reason (2), while the fibers with largest diameter and fastest conducting velocity in CST come from M1, it was also reported that the CST contained direct projections from SMA, PMd, parietal cortex (mainly terminated in the brain stem), S1, and PMv, presented in descending order of the axon conduction velocity (Firmin et al., 2014; Innocenti et al., 2019). Accordingly, we may expect the change in M1-MEP to be a result of CST output change from other cortical areas instead of M1 excitability. As the axon conducting velocities from different cortical areas vary from 6.01 to 15.49 m/s in the monkey CST (Innocenti et al., 2019) and this variability may result in the iteration of corticospinal descending volleys, future studies are expected to examine the descending volley modulation under motor network dual-site TMS protocols.
Discussion: Connecting System and Cellular Process Underlying Transcranial Magnetic Stimulation
In this chapter, we integrate the neurophysiological evidence based on the presented neuron landscape (Figure 2) and propose our model of the underlying neural mechanisms of motor cortex TMS. Based on our literature review, we connect the TMS results and cellular/anatomic evidence from the perspectives of (1) stimulus intensity, (2) paired-pulse timing, and (3) TMS-induced current direction.
Paired-Pulse Timing
The effect of intracortical and interhemispheric paired-pulse TMS (see Section “Transcranial Magnetic Stimulation Paradigm Selectivity” and Figure 3) can be expected to reflect certain neuron connectivity through precise ISI and pulse intensity. In chemical synapses, the latency from the peak of presynaptic action potential (AP) to the initiation of postsynaptic EPSP between PNs was approximately 1.5 ms, and the same latency was preserved in both IN–IN and IN–PN synaptic connections (Di Lazzaro et al., 1999; Guo et al., 2012). From the consistency of synaptic latency and SICF timing, the contribution of a translaminar PN–PN synaptic chain in SICF can be expected. That is, whereas the first suprathreshold pulse generates EPSPs in most PNs, only a part of them fires, in which the generated EPSP is strong enough to depolarize the membrane to the AP threshold. The “residual” EPSPs in the unfired PNs can then be raised above the threshold by the upcoming subthreshold stimulus, generating additional APs, which is reflected as MEP facilitation and late I-wave facilitation in SCEP recordings (Di Lazzaro et al., 1999; Ziemann, 2020). The facilitation in SICF may circumvent the inhibitory INs, as there is abundant evidence to show that with ISI at the SICF curve troughs (indicating the refractory period of the fired PN) and even under pharmaceutical control, MEP remained facilitated in the SICF paradigm (Ziemann et al., 1998; Delvendahl et al., 2014; Van den Bos et al., 2018; Opie and Semmler, 2021). The facilitation of SICF may come from both M1 and PMd through direct synaptic connection with L2/3 IT neurons targeting L5 PN. However, as discussed in Section “M1 Paired-Pulse Transcranial Magnetic Stimulation,” it should be noted that as SICF and I-wave are not completely identical, the suppression of I-waves by inhibitory protocols (Di Lazzaro et al., 1998b; Ni et al., 2011b) can therefore involve an IN component, which is different from that of SICF. Specifically, the generation of I-waves [for a comprehensive review, see Ziemann (2020)] represents an aggregative output of all cortical neurons excited by a TMS pulse, whereas SICF may selectively recruit the EPSPs in the PNs by the paired-pulse (Figure 4). In paired-pulse inhibitory protocols, such as SICI, LICI, and IHI, the involvement of cortical INs can also be analyzed in the pulse timing. SICI and LICF were first reported by Kujirai et al. (1993) with constant CS and TS intensity and varied ISI, in which a consecutive evolution from inhibition to disinhibition was revealed as having ISI as the sole variable. By analogy of the timing and pulse intensity, we speculated that the two phenomena may be related to similar neural circuits involving cortical BC and ChC function [see Section “Parvalbumin-Expressing (PV+) Inhibitory Interneuron”]. There are three reasons for this speculation. First, in both SICI and LICF, the first pulse is subthreshold (usually 60–90% AMT), which cannot reach the deeper layers as threshold pulse does. Accordingly, the subthreshold pulse results in the excitation of the supragranular PV+ BC and ChC, which can elicit powerful and extensive feedforward influence onto the incoming second suprathreshold pulse. Second, in addition to the demonstrated contribution of fast-spiking BC in SICI generation (Aberra et al., 2020; Fong et al., 2021), the timings of SICI and LICF (1–6 ms and 8–30 ms) were also consistent with the time window of ChC inhibition and disinhibition in patch-clamp studies (Woodruff et al., 2011). Third, it has been proved that ChCs mainly innervate the AIS of PN through GABAAR-α2 (Gulledge and Stuart, 2003; Howard et al., 2005; Szabadics et al., 2006; Gao and Heldt, 2016). As pharmaceutical studies have reported the effect of GABAAR (especially α2 or α3 subunits) in SICI (Kujirai et al., 1993; Di Lazzaro et al., 2007), there is the possibility that SICI and LICF are related to supragranular ChC activity. For LICI (CSP) and LAI that have an extremely long latency of 50–200 ms, we propose that the asynchronous GABA release from a different subset of INs may be a contributing factor (Hefft and Jonas, 2005). In the neocortex, this asynchrony was mainly produced by Sst+ Martinotti cells [the regulator of neocortical surround inhibition, see Section “Somatostatin-Expressing (Sst+) Inhibitory Interneuron”], causing persisting inhibition lasting 42.2–210.3 ms after presynaptic PN firing (Deng et al., 2020). Additionally, in the amygdala, neocortical CCK+ BCs were also reported to delay PN firing by up to 40 ms (Veres et al., 2017). Although whether this long-time delay in the amygdala is preserved in the neocortex remains unclear, neocortical Sst+ and 5HT3AR+ INs that cause GABA release asynchrony and delay may be related to the mechanisms of the long latency paired-pulse TMS paradigms with ISI > 50 ms.
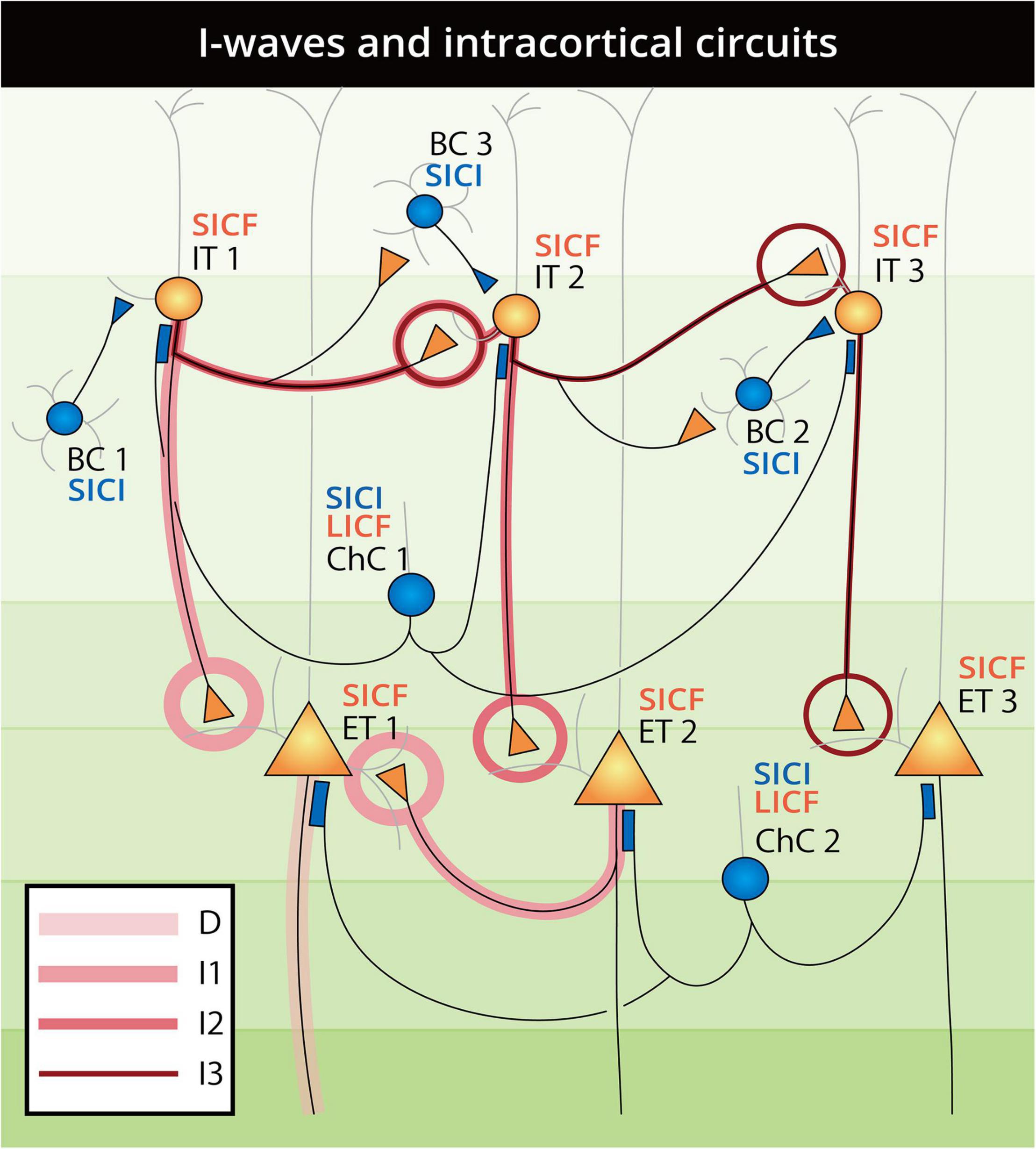
Figure 4. Schematic landscape of D-wave and I-wave generation and paired-pulse SICI, SICF, and LICF intracortical circuits. Neurons are constructed and labeled as in Figure 2 and are numbered to illustrate the pathways of D-waves and I-waves. D-waves arise from the direct firing of ET neurons, as lined from the axon of ET1. The I1-wave is generated through the IT1-ET1 and ET2-ET1 types of circuit with one synaptic connection, which accounts for the ∼1.5 ms lag after the D-wave. Accordingly, the I2-wave is generated through the IT1-IT2-ET2 type of circuit with two synaptic connections, and the I3-wave is generated from the IT1-IT2-IT3-ET3 pathway with three synaptic connections. As the suprathreshold TMS pulse activates all neurons at the same time, the arrival of BC1 inhibition to the IT1 neuron lags one synaptic delay after the first firing of the IT1 neuron (generating the I1-wave), and can therefore explain the SCEP evidence of no inhibition of the I1-wave in the inhibitory protocols. Subsequently, with the regulation of INs, the I2- and later I-waves can be selectively suppressed by a timing-matched subthreshold pulse and displayed as SICI. When the influence of ChC at the AIS turned from inhibition to disinhibition (excitation) in the 8–30 ms time window, the outcome shifts consecutively from inhibition (SICI) to facilitation (LICF). As SICF selectively recruits the residual EPSPs from the unfired IT and ET neurons at a short latency, the regulation of INs may be eliminated by the second pulse arriving at the firing interval of the highly synchronous fast-spiking BCs and ChCs.
However, as there is evidence that dopamine, acetylcholine, and norepinephrine can also affect the activity of INs and PNs apart from the main neurotransmitter of glutamate and GABA, and that regulating those neurotransmitters actually alters the TMS outcome (Ziemann et al., 2015; Turco et al., 2018), questions regarding the neural substrates of TMS remain unanswered. It is also necessary to take other neurotransmitters into account when explaining the TMS mechanisms in the future.
Transcranial Magnetic Stimulation-Induced Current Direction
For TMS current directions, we believe that further insight can be obtained by zooming out from the cellular structure to the gross anatomy of the precentral gyrus. Even with the “focal” figure-of-eight coil, the excitation area of M1-TMS can cover both the anterior and posterior banks of the precentral gyrus, with strongest electric field presenting at the gyral crown (Aberra et al., 2020). In this case, the side in which the axon direction follows the induced current can be preferentially excited compared with the opposite side. That is, AP- and PA-TMS can result in preferential excitation of the anterior and posterior bank of precentral gyrus, respectively. This preference was partly reflected in computation models, in which the threshold of the anterior bank was lower than the posterior bank in AP-TMS than in PA-TMS, and vice versa (Aberra et al., 2020; Wendt et al., 2021). In addition, the most extensively investigated M1 area—the “hand knob,” has been strongly evidenced as an area mainly located in the posterior bank of the precentral gyrus, with the opposing anterior bank being largely PMd, which has a high neuroanatomical variability across individuals (Yousry et al., 1997; Siebner, 2020; Eichert et al., 2021; Simone et al., 2021). We highlight this evidence because it implies a potential explanation for D-wave and I-wave recruitment that can fit almost all experimental evidence (I-wave recruitment, MEP latency, inter-individual differences etc.; for details, see Sections “Transcranial Magnetic Stimulation Pulse Property Selectivity,” “Motor Network Dual-Site Transcranial Magnetic Stimulation”). Specifically, we propose the hypothesis that AP- and PA-TMS can represent the anterior–posterior or posterior–anterior bank preferential excitation order of the precentral gyrus, whereas LM-TMS excites both the banks of the precentral gyrus to the same extent, resulting in lowest threshold and shortest MEP latency. For I-wave preferential induction by PA- and AP-TMS, we speculate that the late I-wave selectivity of AP-TMS may be related to the IT neurons located in the anterior bank of the precentral gyrus, which can be priorly excited by AP-TMS. These IT neurons may receive the regulation of INs by disynaptic inhibition during the synaptic relay from the anterior bank to the “hand knob” M1 ET neurons, resulting in the late I-wave inhibition in the SAI, SICI, and LICI (Di Lazzaro et al., 1999; Ilic et al., 2002; Ni et al., 2011b) paradigms (see Section “M1 Paired-Pulse Transcranial Magnetic Stimulation”).
Implications and Future Directions
Efforts to establish the connection of the microstructure and macro-outcome of the human brain are in a preliminary stage, not only for TMS but in all aspects of neurocytology. Based on our literature review, we identified a number of research gaps underlying TMS, both in cellular and system neurocytology. First, we noticed that the major cellular evidence on neocortical neurons comes from animal studies of various cortical areas, which is influenced by the differences in species and cerebral areas when we seek to explain the specific mechanisms of human motor cortex TMS. However, we acknowledge the technical and ethical conundrums of conducting human cellular experiments (even on postmortem tissue). Consequently, alternative methods for analyzing the interspecies differences have emerged [e.g., the BICCN studies on the motor cortex (Bakken et al., 2021; Kalmbach et al., 2021)], which is a helpful solution to the understanding of the human brain from animal experiments. Second, even though TMS has been practiced for over 35 years, a large potential for development remains. To perform more precise, detailed investigations, as well more efficient neuromodulations, practical modifications such as a more focal TMS coil design, a narrower TMS pulse width, and a reduction in TMS coil size are expected in the future. Third, since the final goal is to “decipher and optimize the human brain,” it is ultimately inevitable that we should link the highly developed cellular neuroscience knowledge with system neuroscience outcomes, so that precise and efficient neuromodulation based on this connection can be achieved in clinical practice.
Consequently, at the preliminary stage, we begin with the most unified, most fundamental, and simplest analysis in M1-TMS at rest or with simple movement. Based on this analysis, future investigations can be expected that integrate complex movements, a combination of brain areas, and neuroplasticity protocols. While we acknowledge that we cannot fully answer the questions we posed in the introduction, which are important research gaps we have identified, we intend to leave these questions open, in the hope that further investigators can be inspired and make novel discoveries based on the existing evidence and hypothetical models.
Conclusion
In this integrative review, we have reviewed the existing literature related to motor cortex neuron biology and TMS; on this basis, we have proposed a preliminary model for the micro–macro connections between neocortical neurons and TMS in the human motor cortex. Additionally, for the unanswered questions underlying certain TMS paradigms (such as the mechanisms of LICF, IHF, and the TMS-induced current selectivity on neural circuits), we provided hypotheses based on the reviewed literature. As a preliminary endeavor to bridge the microscopic and macroscopic facts regarding TMS, the present integrative review provides a foundation for future investigations, as well as a platform for technical advances and applications of TMS.
Author Contributions
DT organized the literature database, performed the review, and wrote the first draft of the manuscript. S-II provided revision of the manuscript. Both authors contributed to conception and design of the study, manuscript revision, read, and approved the submitted version.
Funding
This work was supported by JST SPRING, grant number JPMJSP2114.
Conflict of Interest
The authors declare that the research was conducted in the absence of any commercial or financial relationships that could be construed as a potential conflict of interest.
Publisher’s Note
All claims expressed in this article are solely those of the authors and do not necessarily represent those of their affiliated organizations, or those of the publisher, the editors and the reviewers. Any product that may be evaluated in this article, or claim that may be made by its manufacturer, is not guaranteed or endorsed by the publisher.
Acknowledgments
We would like to acknowledge Fuping Han for technical assistance on the literature collection and preliminary summary.
References
Aberra, A. S., Wang, B., Grill, W. M., and Peterchev, A. V. (2020). Simulation of transcranial magnetic stimulation in head model with morphologically-realistic cortical neurons. Brain Stimul. 13, 175–189. doi: 10.1016/j.brs.2019.10.002
Adotevi, N. K., and Leitch, B. (2017). Synaptic changes in AMPA receptor subunit expression in cortical parvalbumin interneurons in the stargazer model of absence epilepsy. Front. Mol. Neurosci. 10:434. doi: 10.3389/fnmol.2017.00434
Al-Wasity, S., Vogt, S., Vuckovic, A., and Pollick, F. E. (2021). Upregulation of supplementary motor area activation with fMRI neurofeedback during motor imagery. Eneuro 8, ENEURO.377–ENEURO.318. doi: 10.1523/ENEURO.0377-18.2020
Amunts, K., and Zilles, K. (2015). Architectonic mapping of the human brain beyond brodmann. Neuron 88, 1086–1107. doi: 10.1016/j.neuron.2015.12.001
Armstrong, C., and Soltesz, I. (2012). Basket cell dichotomy in microcircuit function. J. Physiol. 590, 683–694. doi: 10.1113/jphysiol.2011.223669
Avanzino, L., Teo, J. T., and Rothwell, J. C. (2007). Intracortical circuits modulate transcallosal inhibition in humans. J. Physiol. 583(Pt 1), 99–114. doi: 10.1113/jphysiol.2007.134510
Baker, A., Kalmbach, B., Morishima, M., Kim, J., Juavinett, A., Li, N., et al. (2018). Specialized subpopulations of deep-layer pyramidal neurons in the neocortex: bridging cellular properties to functional consequences. J. Neurosci. 38:5441. doi: 10.1523/JNEUROSCI.0150-18.2018
Bakken, T. E., Jorstad, N. L., Hu, Q., Lake, B. B., Tian, W., Kalmbach, B. E., et al. (2021). Comparative cellular analysis of motor cortex in human, marmoset and mouse. Nature 598, 111–119. doi: 10.1038/s41586-021-03465-8
Banasr, M., Lepack, A., Fee, C., Duric, V., Maldonado-Aviles, J., DiLeone, R., et al. (2017). Characterization of GABAergic marker expression in the chronic unpredictable stress model of depression. Chronic Stress 1:2470547017720459. doi: 10.1177/2470547017720459
Barbas, H., and García-Cabezas, M. A. (2015). Motor cortex layer 4: less is more. Trends Neurosci. 38, 259–261. doi: 10.1016/j.tins.2015.03.005
Barker, A. T., Jalinous, R., and Freeston, I. L. (1985). Non-invasive magnetic stimulation of human motor cortex. Lancet 325, 1106–1107. doi: 10.1016/s0140-6736(85)92413-4
Bartos, M., and Elgueta, C. (2012). Functional characteristics of parvalbumin- and cholecystokinin-expressing basket cells. J. Physiol. 590, 669–681. doi: 10.1113/jphysiol.2011.226175
Baumer, T., Bock, F., Koch, G., Lange, R., Rothwell, J. C., Siebner, H. R., et al. (2006). Magnetic stimulation of human premotor or motor cortex produces interhemispheric facilitation through distinct pathways. J. Physiol. 572(Pt 3), 857–868. doi: 10.1113/jphysiol.2006.104901
Behrens, T. E. J., Johansen-Berg, H., Woolrich, M. W., Smith, S. M., Wheeler-Kingshott, C. A. M., Boulby, P. A., et al. (2003). Non-invasive mapping of connections between human thalamus and cortex using diffusion imaging. Nat. Neurosci. 6, 750–757. doi: 10.1038/nn1075
Belyk, M., Banks, R., Tendera, A., Chen, R., and Beal, D. S. (2021). Paradoxical facilitation alongside interhemispheric inhibition. Exp. Brain Res. 239, 3303–3313. doi: 10.1007/s00221-021-06183-9
Benarroch, E. E. (2013). Neocortical interneurons: functional diversity and clinical correlations. Neurology 81, 273–280. doi: 10.1212/WNL.0b013e31829c002f
Berger, T. K., Perin, R., Silberberg, G., and Markram, H. (2009). Frequency-dependent disynaptic inhibition in the pyramidal network: a ubiquitous pathway in the developing rat neocortex. J. Physiol. 587, 5411–5425. doi: 10.1113/jphysiol.2009.176552
Boyle, K. A., Gutierrez-Mecinas, M., Polgár, E., Mooney, N., O’Connor, E., Furuta, T., et al. (2017). A quantitative study of neurochemically defined populations of inhibitory interneurons in the superficial dorsal horn of the mouse spinal cord. Neuroscience 363, 120–133. doi: 10.1016/j.neuroscience.2017.08.044
Brodmann, K. (1909). Vergleichende Lokalisationslehre der Grosshirnrinde in ihren Prinzipien Dargestellt auf Grund des Zellenbaues. Leipzig: Barth.
Brown, M. J. N., Weissbach, A., Pauly, M. G., Vesia, M., Gunraj, C., Baarbé, J., et al. (2019). Somatosensory-motor cortex interactions measured using dual-site transcranial magnetic stimulation. Brain Stimul. 12, 1229–1243. doi: 10.1016/j.brs.2019.04.009
Brown, S. P., and Hestrin, S. (2009). Intracortical circuits of pyramidal neurons reflect their long-range axonal targets. Nature 457, 1133–1136. doi: 10.1038/nature07658
Buch, E. R., Johnen, V. M., Nelissen, N., Shea, J., and Rushworth, M. F. S. (2011). Noninvasive associative plasticity induction in a corticocortical pathway of the human brain. J. Neurosci. 31:17669. doi: 10.1523/JNEUROSCI.1513-11.2011
Burke, D., Hicks, R., Gandevia, S. C., Stephen, J., Woodforth, I., and Crawford, M. (1993). Direct comparison of corticospinal volleys in human subjects to transcranial magnetic and electrical stimulation. J. Physiol. 470, 383–393. doi: 10.1113/jphysiol.1993.sp019864
Butt, S. J. B., Fuccillo, M., Nery, S., Noctor, S., Kriegstein, A., Corbin, J. G., et al. (2005). The temporal and spatial origins of cortical interneurons predict their physiological subtype. Neuron 48, 591–604. doi: 10.1016/j.neuron.2005.09.034
Cajal, S. R. Y. (1899). Estudios sobre la corteza cerebral humana. II. Estructura de la corteza motriz del hombre y mamíferos superiores. Revista Trimestral Microgáfica 4, 117–200.
Callaway, E. M., Dong, H.-W., Ecker, J. R., Hawrylycz, M. J., Huang, Z. J., Lein, E. S., et al. (2021). A multimodal cell census and atlas of the mammalian primary motor cortex. Nature 598, 86–102. doi: 10.1038/s41586-021-03950-0
Casula, E. P., Rocchi, L., Hannah, R., and Rothwell, J. C. (2018). Effects of pulse width, waveform and current direction in the cortex: a combined cTMS-EEG study. Brain Stimul. 11, 1063–1070. doi: 10.1016/j.brs.2018.04.015
Chameau, P., and van Hooft, J. A. (2006). Serotonin 5-HT3 receptors in the central nervous system. Cell Tissue Res. 326, 573–581. doi: 10.1007/s00441-006-0255-8
Charvet, C. J., Šimić, G., Kostović, I., Knezović, V., Vukšić, M., Babić Leko, M., et al. (2017). Coevolution in the timing of GABAergic and pyramidal neuron maturation in primates. Proc. R. Soc. B Biol. Sci. 284:20171169. doi: 10.1098/rspb.2017.1169
Chen, R. (2004). Interactions between inhibitory and excitatory circuits in the human motor cortex. Exp. Brain Res. 154, 1–10. doi: 10.1007/s00221-003-1684-1
Chen, R. (2017). Methods to examine I-wave interactions and effects of afferent input. Brain Stimul. 10:539. doi: 10.1016/j.brs.2017.01.566
Chen, R., Yung, D., and Li, J.-Y. (2003). Organization of ipsilateral excitatory and inhibitory pathways in the human motor cortex. J. Neurophysiol. 89, 1256–1264. doi: 10.1152/jn.00950.2002
Civardi, C., Cantello, R., Asselman, P., and Rothwell, J. C. (2001). Transcranial magnetic stimulation can be used to test connections to primary motor areas from frontal and medial cortex in humans. NeuroImage 14, 1444–1453. doi: 10.1006/nimg.2001.0918
Conti, F., and Manzoni, T. (1994). The neurotransmitters and postsynaptic actions of callosally projecting neurons. Behav. Brain Res. 64, 37–53. doi: 10.1016/0166-4328(94)90117-1
Côté, S. L., Hamadjida, A., Quessy, S., and Dancause, N. (2017). Contrasting modulatory effects from the dorsal and ventral premotor cortex on primary motor cortex outputs. J. Neurosci. 37:5960. doi: 10.1523/JNEUROSCI.0462-17.2017
Cracco, R. Q., Amassian, V. E., Maccabee, P. J., and Cracco, J. B. (1989). Comparison of human transcallosal responses evoked by magnetic coil and electrical stimulation. Electroencephalogr. Clin. Neurophysiol. Evoked Potentials Sect. 74, 417–424. doi: 10.1016/0168-5597(89)90030-0
Cuypers, K., Verstraelen, S., Maes, C., Hermans, L., Hehl, M., Heise, K. F., et al. (2020). Task-related measures of short-interval intracortical inhibition and GABA levels in healthy young and older adults: a multimodal TMS-MRS study. NeuroImage 208:116470. doi: 10.1016/j.neuroimage.2019.116470
Darmani, G., and Ziemann, U. (2019). Pharmacophysiology of TMS-evoked EEG potentials: a mini-review. Brain Stimul. 12, 829–831. doi: 10.1016/j.brs.2019.02.021
Daskalakis, Z. J., Christensen, B. K., Fitzgerald, P. B., Roshan, L., and Chen, R. (2002). The mechanisms of interhemispheric inhibition in the human motor cortex. J. Physiol. 543, 317–326. doi: 10.1113/jphysiol.2002.017673
Daw, M. I., Tricoire, L., Erdelyi, F., Szabo, G., and McBain, C. J. (2009). Asynchronous transmitter release from cholecystokinin-containing inhibitory interneurons is widespread and target-cell independent. J. Neurosci. 29:11112. doi: 10.1523/JNEUROSCI.5760-08.2009
Day, B. L., Dressler, D., Maertens de Noordhout, A., Marsden, C. D., Nakashima, K., Rothwell, J. C., et al. (1989). Electric and magnetic stimulation of human motor cortex: surface EMG and single motor unit responses. J. Physiol. 412, 449–473. doi: 10.1113/jphysiol.1989.sp017626
DeFelipe, J., Alonso-Nanclares, L., and Arellano, J. I. (2002). Microstructure of the neocortex: comparative aspects. J. Neurocytol. 31, 299–316. doi: 10.1023/A:1024130211265
Delvendahl, I., Lindemann, H., Jung, N. H., Pechmann, A., Siebner, H. R., and Mall, V. (2014). Influence of waveform and current direction on short-interval intracortical facilitation: a paired-pulse TMS study. Brain Stimul. 7, 49–58. doi: 10.1016/j.brs.2013.08.002
Deng, S., Li, J., He, Q., Zhang, X., Zhu, J., Li, L., et al. (2020). Regulation of recurrent inhibition by asynchronous glutamate release in neocortex. Neuron 105, 522.e524–533.e524. doi: 10.1016/j.neuron.2019.10.038
Di Lazzaro, V., Oliviero, A., Mazzone, P., Insola, A., Pilato, F., Saturno, E., et al. (2001a). Comparison of descending volleys evoked by monophasic and biphasic magnetic stimulation of the motor cortex in conscious humans. Exp. Brain Res. 141, 121–127. doi: 10.1007/s002210100863
Di Lazzaro, V., Oliviero, A., Saturno, E., Pilato, F., Insola, A., Mazzone, P., et al. (2001b). The effect on corticospinal volleys of reversing the direction of current induced in the motor cortex by transcranial magnetic stimulation. Exp. Brain Res. 138, 268–273. doi: 10.1007/s002210100722
Di Lazzaro, V., Oliviero, A., Pilato, F., Saturno, E., Dileone, M., Meglio, M., et al. (2004). Comparison of descending volleys evoked by transcranial and epidural motor cortex stimulation in a conscious patient with bulbar pain. Clin. Neurophysiol. 115, 834–838. doi: 10.1016/j.clinph.2003.11.026
Di Lazzaro, V., Restuccia, D., Oliviero, A., Profice, P., Ferrara, L., Insola, A., et al. (1998b). Magnetic transcranial stimulation at intensities below active motor threshold activates intracortical inhibitory circuits. Exp. Brain Res. 119, 265–268. doi: 10.1007/s002210050341
Di Lazzaro, V., Oliviero, A., Profice, P., Saturno, E., Pilato, F., Insola, A., et al. (1998a). Comparison of descending volleys evoked by transcranial magnetic and electric stimulation in conscious humans. Electroencephalogr. Clin. Neurophysiol. 109, 397–401. doi: 10.1016/s0924-980x(98)00038-1
Di Lazzaro, V., Pilato, F., Dileone, M., Profice, P., Ranieri, F., Ricci, V., et al. (2007). Segregating two inhibitory circuits in human motor cortex at the level of GABAA receptor subtypes: a TMS study. Clin. Neurophysiol. 118, 2207–2214. doi: 10.1016/j.clinph.2007.07.005
Di Lazzaro, V., Pilato, F., Oliviero, A., Dileone, M., Saturno, E., Mazzone, P., et al. (2006). Origin of facilitation of motor-evoked potentials after paired magnetic stimulation: direct recording of epidural activity in conscious humans. J. Neurophysiol. 96, 1765–1771. doi: 10.1152/jn.00360.2006
Di Lazzaro, V., Profice, P., Ranieri, F., Capone, F., Dileone, M., Oliviero, A., et al. (2012). I-wave origin and modulation. Brain Stimul. 5, 512–525. doi: 10.1016/j.brs.2011.07.008
Di Lazzaro, V., Rothwell, J., and Capogna, M. (2017). Noninvasive stimulation of the human brain: activation of multiple cortical circuits. Neuroscientist 24, 246–260. doi: 10.1177/1073858417717660
Di Lazzaro, V., and Rothwell, J. C. (2014). Corticospinal activity evoked and modulated by non-invasive stimulation of the intact human motor cortex. J. Physiol. 592, 4115–4128. doi: 10.1113/jphysiol.2014.274316
Di Lazzaro, V., Rothwell, J. C., Oliviero, A., Profice, P., Insola, A., Mazzone, P., et al. (1999). Intracortical origin of the short latency facilitation produced by pairs of threshold magnetic stimuli applied to human motor cortex. Exp. Brain Res. 129, 494–499. doi: 10.1007/s002210050919
Di Lazzaro, V., and Ziemann, U. (2013). The contribution of transcranial magnetic stimulation in the functional evaluation of microcircuits in human motor cortex. Front. Neural. Circuits 7:18. doi: 10.3389/fncir.2013.00018
Ding, C., Emmenegger, V., Schaffrath, K., and Feldmeyer, D. (2021). Layer-specific inhibitory microcircuits of layer 6 interneurons in rat prefrontal cortex. Cereb. Cortex 31, 32–47. doi: 10.1093/cercor/bhaa201
D’Ostilio, K., Goetz, S. M., Hannah, R., Ciocca, M., Chieffo, R., Chen, J.-C. A., et al. (2016). Effect of coil orientation on strength–duration time constant and I-wave activation with controllable pulse parameter transcranial magnetic stimulation. Clin. Neurophysiol. 127, 675–683. doi: 10.1016/j.clinph.2015.05.017
Douglas, R. J., and Martin, K. A. C. (2004). Neuronal circuits of the neocortex. Annu. Rev. Neurosci. 27, 419–451. doi: 10.1146/annurev.neuro.27.070203.144152
Du, X., Rowland, L. M., Summerfelt, A., Wijtenburg, A., Chiappelli, J., Wisner, K., et al. (2018). TMS evoked N100 reflects local GABA and glutamate balance. Brain Stimul. 11, 1071–1079. doi: 10.1016/j.brs.2018.05.002
Eichert, N., Watkins, K. E., Mars, R. B., and Petrides, M. (2021). Morphological and functional variability in central and subcentral motor cortex of the human brain. Brain Struct. Funct. 226, 263–279. doi: 10.1007/s00429-020-02180-w
Fame, R. M., MacDonald, J. L., and Macklis, J. D. (2011). Development, specification, and diversity of callosal projection neurons. Trends Neurosci. 34, 41–50. doi: 10.1016/j.tins.2010.10.002
Faux, C., Rakic, S., Andrews, W., and Britto, J. M. (2012). Neurons on the move: migration and lamination of cortical interneurons. Neurosignals 20, 168–189. doi: 10.1159/000334489
Ferbert, A., Priori, A., Rothwell, J. C., Day, B. L., Colebatch, J. G., and Marsden, C. D. (1992). Interhemispheric inhibition of the human motor cortex. J. Physiol. 453, 525–546. doi: 10.1113/jphysiol.1992.sp019243
Fernandez, L., Major, B. P., Teo, W.-P., Byrne, L. K., and Enticott, P. G. (2018). Assessing cerebellar brain inhibition (CBI) via transcranial magnetic stimulation (TMS): a systematic review. Neurosci. Biobehav. Rev. 86, 176–206. doi: 10.1016/j.neubiorev.2017.11.018
Fiori, F., Chiappini, E., Candidi, M., Romei, V., Borgomaneri, S., and Avenanti, A. (2017). Long-latency interhemispheric interactions between motor-related areas and the primary motor cortex: a dual site TMS study. Sci. Rep. 7:14936. doi: 10.1038/s41598-017-13708-2
Firmin, L., Field, P., Maier, M. A., Kraskov, A., Kirkwood, P. A., Nakajima, K., et al. (2014). Axon diameters and conduction velocities in the macaque pyramidal tract. J. Neurophysiol. 112, 1229–1240. doi: 10.1152/jn.00720.2013
Földy, C., Lee, S. Y., Szabadics, J., Neu, A., and Soltesz, I. (2007). Cell type–specific gating of perisomatic inhibition by cholecystokinin. Nat. Neurosci. 10, 1128–1130. doi: 10.1038/nn1952
Fong, P.-Y., Spampinato, D., Rocchi, L., Hannah, R., Teng, Y., Di Santo, A., et al. (2021). Two forms of short-interval intracortical inhibition in human motor cortex. Brain Stimul. 14, 1340–1352. doi: 10.1016/j.brs.2021.08.022
Gao, Y., and Heldt, S. A. (2016). Enrichment of GABAA receptor α-Subunits on the axonal initial segment shows regional differences. Front. Cell Neurosci. 10:39. doi: 10.3389/fncel.2016.00039
Gelman, D., Griveau, A., Dehorter, N., Teissier, A., Varela, C., Pla, R., et al. (2011). A wide diversity of cortical GABAergic interneurons derives from the embryonic preoptic area. J. Neurosci. 31:16570. doi: 10.1523/JNEUROSCI.4068-11.2011
Ghosh, S., Mehta, A. R., Huang, G., Gunraj, C., Hoque, T., Saha, U., et al. (2013). Short- and long-latency interhemispheric inhibitions are additive in human motor cortex. J. Neurophysiol. 109, 2955–2962. doi: 10.1152/jn.00960.2012
Glausier, J. R., Datta, D., Fish, K. N., Chung, D. W., Melchitzky, D. S., and Lewis, D. A. (2021). Laminar differences in the targeting of dendritic spines by cortical pyramidal neurons and interneurons in human dorsolateral prefrontal cortex. Neuroscience 452, 181–191. doi: 10.1016/j.neuroscience.2020.10.022
Goff, K. M., and Goldberg, E. M. (2019). Vasoactive intestinal peptide-expressing interneurons are impaired in a mouse model of Dravet syndrome. eLife 8:e46846. doi: 10.7554/eLife.46846
Green, P. E., Ridding, M. C., Hill, K. D., Semmler, J. G., Drummond, P. D., and Vallence, A.-M. (2018). Supplementary motor area—primary motor cortex facilitation in younger but not older adults. Neurobiol. Aging 64, 85–91. doi: 10.1016/j.neurobiolaging.2017.12.016
Greig, L. C., Woodworth, M. B., Galazo, M. J., Padmanabhan, H., and Macklis, J. D. (2013). Molecular logic of neocortical projection neuron specification, development and diversity. Nat. Rev. Neurosci. 14, 755–769. doi: 10.1038/nrn3586
Groppa, S., Schlaak, B. H., Munchau, A., Werner-Petroll, N., Dunnweber, J., Baumer, T., et al. (2012a). The human dorsal premotor cortex facilitates the excitability of ipsilateral primary motor cortex via a short latency cortico-cortical route. Hum. Brain Mapp. 33, 419–430. doi: 10.1002/hbm.21221
Groppa, S., Werner-Petroll, N., Munchau, A., Deuschl, G., Ruschworth, M. F., and Siebner, H. R. (2012b). A novel dual-site transcranial magnetic stimulation paradigm to probe fast facilitatory inputs from ipsilateral dorsal premotor cortex to primary motor cortex. Neuroimage 62, 500–509. doi: 10.1016/j.neuroimage.2012.05.023
Gu, F., Parada, I., Shen, F., Li, J., Bacci, A., Graber, K., et al. (2017). Structural alterations in fast-spiking GABAergic interneurons in a model of posttraumatic neocortical epileptogenesis. Neurobiol. Dis. 108, 100–114. doi: 10.1016/j.nbd.2017.08.008
Gulledge, A. T., and Stuart, G. J. (2003). Excitatory actions of GABA in the cortex. Neuron 37, 299–309. doi: 10.1016/S0896-6273(02)01146-7
Guo, D., Wang, Q., and Perc, M. (2012). Complex synchronous behavior in interneuronal networks with delayed inhibitory and fast electrical synapses. Phys. Rev. E 85:061905. doi: 10.1103/PhysRevE.85.061905
Hanajima, R., Ugawa, Y., Machii, K., Mochizuki, H., Terao, Y., Enomoto, H., et al. (2001). Interhemispheric facilitation of the hand motor area in humans. J. Physiol. 531(Pt 3), 849–859. doi: 10.1111/j.1469-7793.2001.0849h.x
Hannah, R., and Rothwell, J. C. (2017). Pulse duration as well as current direction determines the specificity of transcranial magnetic stimulation of motor cortex during contraction. Brain Stimul. 10, 106–115. doi: 10.1016/j.brs.2016.09.008
Hassan, S. S., Baumgarten, T. J., Ali, A. M., Füllenbach, N.-D., Jördens, M. S., Häussinger, D., et al. (2019). Cerebellar inhibition in hepatic encephalopathy. Clin. Neurophysiol. 130, 886–892. doi: 10.1016/j.clinph.2019.02.020
Hefft, S., and Jonas, P. (2005). Asynchronous GABA release generates long-lasting inhibition at a hippocampal interneuron–principal neuron synapse. Nat. Neurosci. 8, 1319–1328. doi: 10.1038/nn1542
Hermans, L., Levin, O., Maes, C., van Ruitenbeek, P., Heise, K.-F., Edden, R. A. E., et al. (2018). GABA levels and measures of intracortical and interhemispheric excitability in healthy young and older adults: an MRS-TMS study. Neurobiol. Aging 65, 168–177. doi: 10.1016/j.neurobiolaging.2018.01.023
Higashihara, M., Van den Bos, M. A. J., Menon, P., Kiernan, M. C., and Vucic, S. (2020). Interneuronal networks mediate cortical inhibition and facilitation. Clin. Neurophysiol. 131, 1000–1010. doi: 10.1016/j.clinph.2020.02.012
Hioki, H., Sohn, J., Nakamura, H., Okamoto, S., Hwang, J., Ishida, Y., et al. (2018). Preferential inputs from cholecystokinin-positive neurons to the somatic compartment of parvalbumin-expressing neurons in the mouse primary somatosensory cortex. Brain Res. 1695, 18–30. doi: 10.1016/j.brainres.2018.05.029
Holmes, N. P., and Tamè, L. (2018). Locating primary somatosensory cortex in human brain stimulation studies: systematic review and meta-analytic evidence. J. Neurophysiol. 121, 152–162. doi: 10.1152/jn.00614.2018
Hooks, B. M., Mao, T., Gutnisky, D. A., Yamawaki, N., Svoboda, K., and Shepherd, G. M. G. (2013). Organization of cortical and thalamic input to pyramidal neurons in mouse motor cortex. J. Neurosci. 33:748. doi: 10.1523/JNEUROSCI.4338-12.2013
Howard, A., Tamas, G., and Soltesz, I. (2005). Lighting the chandelier: new vistas for axo-axonic cells. Trends Neurosci. 28, 310–316. doi: 10.1016/j.tins.2005.04.004
Hu, J. S., Vogt, D., Sandberg, M., and Rubenstein, J. L. (2017). Cortical interneuron development: a tale of time and space. Development 144, 3867–3878. doi: 10.1242/dev.132852
Ilic, T. V., Meintzschel, F., Cleff, U., Ruge, D., Kessler, K. R., and Ziemann, U. (2002). Short-interval paired-pulse inhibition and facilitation of human motor cortex: the dimension of stimulus intensity. J. Physiol. 545, 153–167. doi: 10.1113/jphysiol.2002.030122
Inan, M., and Anderson, S. A. (2014). The chandelier cell, form and function. Curr. Opin. Neurobiol. 26, 142–148. doi: 10.1016/j.conb.2014.01.009
Innocenti, G. M., Caminiti, R., Rouiller, E. M., Knott, G., Dyrby, T. B., Descoteaux, M., et al. (2019). Diversity of Cortico-descending projections: histological and diffusion MRI characterization in the monkey. Cereb. Cortex 29, 788–801. doi: 10.1093/cercor/bhx363
Irlbacher, K., Brocke, J., Mechow, J. V., and Brandt, S. A. (2007). Effects of GABAA and GABAB agonists on interhemispheric inhibition in man. Clin. Neurophysiol. 118, 308–316. doi: 10.1016/j.clinph.2006.09.023
Izumi, S.-I. (2001). Investigation of human motor control using transcranial magnetic stimulation. Japanese J. Rehabil. Med. 38, 671–681. doi: 10.2490/jjrm1963.38.671
Jiang, X., Shen, S., Cadwell Cathryn, R., Berens, P., Sinz, F., Ecker Alexander, S., et al. (2015). Principles of connectivity among morphologically defined cell types in adult neocortex. Science 350:aac9462. doi: 10.1126/science.aac9462
Jiang, X., Wang, G., Lee, A. J., Stornetta, R. L., and Zhu, J. J. (2013). The organization of two new cortical interneuronal circuits. Nat. Neurosci. 16, 210–218. doi: 10.1038/nn.3305
Jung, P., Beyerle, A., Humpich, M., Neumann-Haefelin, T., Lanfermann, H., and Ziemann, U. (2006). Ipsilateral silent period: a marker of callosal conduction abnormality in early relapsing-remitting multiple sclerosis? J. Neurol. Sci. 250, 133–139. doi: 10.1016/j.jns.2006.08.008
Kallioniemi, E., Savolainen, P., Jarnefelt, G., Koskenkorva, P., Karhu, J., and Julkunen, P. (2018). Transcranial magnetic stimulation modulation of corticospinal excitability by targeting cortical I-waves with biphasic paired-pulses. Brain Stimul. 11, 322–326. doi: 10.1016/j.brs.2017.10.014
Kalmbach, B. E., Hodge, R. D., Jorstad, N. L., Owen, S., de Frates, R., Yanny, A. M., et al. (2021). Signature morpho-electric, transcriptomic, and dendritic properties of human layer 5 neocortical pyramidal neurons. Neuron 109, 2914.e2915–2927.e2915. doi: 10.1016/j.neuron.2021.08.030
Kammer, T., Beck, S., Thielscher, A., Laubis-Herrmann, U., and Topka, H. (2001). Motor thresholds in humans: a transcranial magnetic stimulation study comparing different pulse waveforms, current directions and stimulator types. Clin. Neurophysiol. 112, 250–258. doi: 10.1016/S1388-2457(00)00513-7
Kast, R. J., and Levitt, P. (2019). Precision in the development of neocortical architecture: from progenitors to cortical networks. Progr. Neurobiol. 175, 77–95. doi: 10.1016/j.pneurobio.2019.01.003
Kätzel, D., Zemelman, B. V., Buetfering, C., Wölfel, M., and Miesenböck, G. (2011). The columnar and laminar organization of inhibitory connections to neocortical excitatory cells. Nat. Neurosci. 14, 100–107. doi: 10.1038/nn.2687
Kawaguchi, Y. (1992). Receptor subtypes involved in callosally-induced postsynaptic potentials in rat frontal agranular cortex in vitro. Exp. Brain Res. 88, 33–40. doi: 10.1007/BF02259126
Kim, J., Matney, C. J., Blankenship, A., Hestrin, S., and Brown, S. P. (2014). Layer 6 corticothalamic neurons activate a cortical output layer, Layer 5a. J. Neurosci. 34:9656. doi: 10.1523/JNEUROSCI.1325-14.2014
Kita, T., and Kita, H. (2012). The subthalamic nucleus is one of multiple innervation sites for long-range corticofugal axons: a single-axon tracing study in the rat. J. Neurosci. 32, 5990–5999. doi: 10.1523/JNEUROSCI.5717-11.2012
Kobayashi, M., Ng, J., Théoret, H., and Pascual-Leone, A. (2003). Modulation of intracortical neuronal circuits in human hand motor area by digit stimulation. Exp. Brain Res. 149, 1–8. doi: 10.1007/s00221-002-1329-9
Koch, G., Franca, M., Mochizuki, H., Marconi, B., Caltagirone, C., and Rothwell, J. C. (2007). Interactions between pairs of transcranial magnetic stimuli over the human left dorsal premotor cortex differ from those seen in primary motor cortex. J. Physiol. 578, 551–562. doi: 10.1113/jphysiol.2006.123562
Kowall, N. W., and Beal, M. F. (1988). Cortical somatostatin, neuropeptide Y, and NADPH diaphorase neurons: normal anatomy and alterations in alzheimer’s disease. Ann. Neurol. 23, 105–114. doi: 10.1002/ana.410230202
Kriegstein, A. R. (2005). Constructing circuits: neurogenesis and migration in the developing neocortex. Epilepsia 46, 15–21. doi: 10.1111/j.1528-1167.2005.00304.x
Krienen, F. M., Goldman, M., Zhang, Q., del Rosario, R. C. H., Florio, M., Machold, R., et al. (2020). Innovations present in the primate interneuron repertoire. Nature 586, 262–269. doi: 10.1038/s41586-020-2781-z
Kubo, K.-I., and Deguchi, K. (2020). Human neocortical development as a basis to understand mechanisms underlying neurodevelopmental disabilities in extremely preterm infants. J. Obstetrics Gynaecol. Res. 46, 2242–2250. doi: 10.1111/jog.14468
Kujirai, T., Caramia, M. D., Rothwell, J. C., Day, B. L., Thompson, P. D., Ferbert, A., et al. (1993). Corticocortical inhibition in human motor cortex. J. Phys. 471, 501–519. doi: 10.1113/jphysiol.1993.sp019912
Langseth, C. M., Gyllborg, D., Miller, J. A., Close, J. L., Long, B., Lein, E. S., et al. (2021). Comprehensive in situ mapping of human cortical transcriptomic cell types. Commun. Biol. 4:998. doi: 10.1038/s42003-021-02517-z
Lee, H., Gunraj, C., and Chen, R. (2007). The effects of inhibitory and facilitatory intracortical circuits on interhemispheric inhibition in the human motor cortex. J. Physiol. 580(Pt 3), 1021–1032. doi: 10.1113/jphysiol.2006.126011
Lee, S., Hjerling-Leffler, J., Zagha, E., Fishell, G., and Rudy, B. (2010). The largest group of superficial neocortical GABAergic interneurons expresses ionotropic serotonin receptors. J. Neurosci. 30:16796. doi: 10.1523/JNEUROSCI.1869-10.2010
Lin, H.-M., Kuang, J.-X., Sun, P., Li, N., Lv, X., and Zhang, Y.-H. (2018). Reconstruction of intratelencephalic neurons in the mouse secondary motor cortex reveals the diverse projection patterns of single neurons. Front. Neuroanat. 12:86. doi: 10.3389/fnana.2018.00086
Llorca, A., Ciceri, G., Beattie, R., Wong, F. K., Diana, G., Serafeimidou-Pouliou, E., et al. (2019). A stochastic framework of neurogenesis underlies the assembly of neocortical cytoarchitecture. eLife 8:e51381. doi: 10.7554/eLife.51381
Lu, J., Tucciarone, J., Padilla-Coreano, N., He, M., Gordon, J. A., and Huang, Z. J. (2017). Selective inhibitory control of pyramidal neuron ensembles and cortical subnetworks by chandelier cells. Nat. Neurosci. 20, 1377–1383. doi: 10.1038/nn.4624
Ma, Y., Hu, H., Berrebi, A. S., Mathers, P. H., and Agmon, A. (2006). Distinct subtypes of somatostatin-containing neocortical interneurons revealed in transgenic mice. J. Neurosci. 26:5069. doi: 10.1523/JNEUROSCI.0661-06.2006
Mao, T., Kusefoglu, D., Hooks, B. M., Huber, D., Petreanu, L., and Svoboda, K. (2011). Long-range neuronal circuits underlying the interaction between sensory and motor cortex. Neuron 72, 111–123. doi: 10.1016/j.neuron.2011.07.029
Marin-Padilla, M. (1972). Double origin of the pericellular baskets of the pyramidal cells of the human motor cortex: a Golgi study. Brain Res. 38, 1–12. doi: 10.1016/0006-8993(72)90585-9
Matho, K. S., Huilgol, D., Galbavy, W., He, M., Kim, G., An, X., et al. (2021). Genetic dissection of the glutamatergic neuron system in cerebral cortex. Nature 598, 182–187. doi: 10.1038/s41586-021-03955-9
McFarland, N. R., and Haber, S. N. (2002). Thalamic relay nuclei of the basal ganglia form both reciprocal and nonreciprocal cortical connections, linking multiple frontal cortical areas. J. Neurosci. 22:8117. doi: 10.1523/JNEUROSCI.22-18-08117.2002
Miyoshi, G., Hjerling-Leffler, J., Karayannis, T., Sousa, V. H., Butt, S. J. B., Battiste, J., et al. (2010). Genetic fate mapping reveals that the caudal ganglionic eminence produces a large and diverse population of superficial cortical interneurons. J. Neurosci. 30:1582. doi: 10.1523/JNEUROSCI.4515-09.2010
Mochizuki, H., Huang, Y.-Z., and Rothwell, J. C. (2004). Interhemispheric interaction between human dorsal premotor and contralateral primary motor cortex. J. Physiol. 561, 331–338. doi: 10.1113/jphysiol.2004.072843
Molinari, M., Minciacchi, D., Bentivoglio, M., and Macchi, G. (1985). Efferent fibers from the motor cortex terminate bilaterally in the thalamus of rats and cats. Exp. Brain Res. 57, 305–312. doi: 10.1007/BF00236536
Mullins, P. G., McGonigle, D. J., O’Gorman, R. L., Puts, N. A. J., Vidyasagar, R., Evans, C. J., et al. (2014). Current practice in the use of MEGA-PRESS spectroscopy for the detection of GABA. NeuroImage 86, 43–52. doi: 10.1016/j.neuroimage.2012.12.004
Muñoz, W., Tremblay, R., Levenstein, D., and Rudy, B. (2017). Layer-specific modulation of neocortical dendritic inhibition during active wakefulness. Science 355, 954–959. doi: 10.1126/science.aag2599
Nakamura, H., Kitagawa, H., Kawaguchi, Y., and Tsuji, H. (1997). Intracortical facilitation and inhibition after transcranial magnetic stimulation in conscious humans. J. Physiol. 498, 817–823. doi: 10.1113/jphysiol.1997.sp021905
Neige, C., Rannaud Monany, D., and Lebon, F. (2021). Exploring cortico-cortical interactions during action preparation by means of dual-coil transcranial magnetic stimulation: a systematic review. Neurosci. Biobehav. Rev. 128, 678–692. doi: 10.1016/j.neubiorev.2021.07.018
Ngomo, S., Leonard, G., Moffet, H., and Mercier, C. (2012). Comparison of transcranial magnetic stimulation measures obtained at rest and under active conditions and their reliability. J. Neurosci. Methods 205, 65–71. doi: 10.1016/j.jneumeth.2011.12.012
Ni, Z., Charab, S., Gunraj, C., Nelson, A. J., Udupa, K., Yeh, I. J., et al. (2011a). Transcranial magnetic stimulation in different current directions activates separate cortical circuits. J. Neurophysiol. 105, 749–756. doi: 10.1152/jn.00640.2010
Ni, Z., Muller-Dahlhaus, F., Chen, R., and Ziemann, U. (2011c). Triple-pulse TMS to study interactions between neural circuits in human cortex. Brain Stimul. 4, 281–293. doi: 10.1016/j.brs.2011.01.002
Ni, Z., Gunraj, C., Wagle-Shukla, A., Udupa, K., Mazzella, F., Lozano, A. M., et al. (2011b). Direct demonstration of inhibitory interactions between long interval intracortical inhibition and short interval intracortical inhibition. J. Physiol. 589(Pt 12), 2955–2962. doi: 10.1113/jphysiol.2011.207928
Ni, Z., Gunraj, C., and Chen, R. (2007). Short interval intracortical inhibition and facilitation during the silent period in human. J. Physiol. 583(Pt 3), 971–982. doi: 10.1113/jphysiol.2007.135749
Nigro, M. J., Hashikawa-Yamasaki, Y., and Rudy, B. (2018). Diversity and connectivity of layer 5 somatostatin-expressing interneurons in the mouse barrel cortex. J. Neurosci. 38:1622. doi: 10.1523/JNEUROSCI.2415-17.2017
Obermayer, J., Heistek, T. S., Kerkhofs, A., Goriounova, N. A., Kroon, T., Baayen, J. C., et al. (2018). Lateral inhibition by Martinotti interneurons is facilitated by cholinergic inputs in human and mouse neocortex. Nat. Commun. 9:4101. doi: 10.1038/s41467-018-06628-w
Opie, G. M., and Semmler, J. G. (2021). Preferential activation of unique motor cortical networks with transcranial magnetic stimulation: a review of the physiological, functional, and clinical evidence. Neuromodulat. Technol. Neural Interface 24, 813–828. doi: 10.1111/ner.13314
Orth, M., and Rothwell, J. C. (2004). The cortical silent period: intrinsic variability and relation to the waveform of the transcranial magnetic stimulation pulse. Clin. Neurophysiol. 115, 1076–1082. doi: 10.1016/j.clinph.2003.12.025
Ortu, E., Deriu, F., Suppa, A., Tolu, E., and Rothwell, J. C. (2008). Effects of volitional contraction on intracortical inhibition and facilitation in the human motor cortex. J. Physiol. 586, 5147–5159. doi: 10.1113/jphysiol.2008.158956
Palomero-Gallagher, N., and Zilles, K. (2019). Cortical layers: cyto-, myelo-, receptor- and synaptic architecture in human cortical areas. NeuroImage 197, 716–741. doi: 10.1016/j.neuroimage.2017.08.035
Patton, H. D., and Amassian, V. E. (1954). Single- and multiple-unit analysis of cortical stage of pyramidal tract activation. J. Neurophysiol. 17, 345–363. doi: 10.1152/jn.1954.17.4.345
Peterchev, A. V., Jalinous, R., and Lisanby, S. H. (2008). A transcranial magnetic stimulator inducing near-rectangular pulses with controllable pulse width (cTMS). IEEE Trans. Biomed. Eng. 55, 257–266. doi: 10.1109/TBME.2007.900540
Povysheva, N. V., Zaitsev, A. V., Gonzalez-Burgos, G., and Lewis, D. A. (2013). Electrophysiological heterogeneity of fast-spiking interneurons: chandelier versus basket cells. PLoS One 8:e70553. doi: 10.1371/journal.pone.0070553
Premoli, I., Rivolta, D., Espenhahn, S., Castellanos, N., Belardinelli, P., Ziemann, U., et al. (2014). Characterization of GABAB-receptor mediated neurotransmission in the human cortex by paired-pulse TMS-EEG. Neuroimage 103, 152–162. doi: 10.1016/j.neuroimage.2014.09.028
Qasem, H., Fujiyama, H., Rurak, B. K., and Vallence, A.-M. (2020). Good test–retest reliability of a paired-pulse transcranial magnetic stimulation protocol to measure short-interval intracortical facilitation. Exp. Brain Res. 238, 2711–2723. doi: 10.1007/s00221-020-05926-4
Qi, G., and Feldmeyer, D. (2016). Dendritic target region-specific formation of synapses between excitatory layer 4 neurons and layer 6 pyramidal cells. Cereb. Cortex 26, 1569–1579. doi: 10.1093/cercor/bhu334
Reiner, A., Jiao, Y., Del Mar, N., Laverghetta, A. V., and Lei, W. L. (2003). Differential morphology of pyramidal tract-type and intratelencephalically projecting-type corticostriatal neurons and their intrastriatal terminals in rats. J. Comparat. Neurol. 457, 420–440. doi: 10.1002/cne.10541
Reis, J., Swayne, O. B., Vandermeeren, Y., Camus, M., Dimyan, M. A., Harris-Love, M., et al. (2008). Contribution of transcranial magnetic stimulation to the understanding of cortical mechanisms involved in motor control. J. Physiol. 586, 325–351. doi: 10.1113/jphysiol.2007.144824
Rivara, C.-B., Sherwood, C. C., Bouras, C., and Hof, P. R. (2003). Stereologic characterization and spatial distribution patterns of Betz cells in the human primary motor cortex. Anatomical Record Part A Discov. Mol. Cell. Evol. Biol. 270A, 137–151. doi: 10.1002/ar.a.10015
Rossini, P. M., Burke, D., Chen, R., Cohen, L. G., Daskalakis, Z., Di Iorio, R., et al. (2015). Non-invasive electrical and magnetic stimulation of the brain, spinal cord, roots and peripheral nerves: Basic principles and procedures for routine clinical and research application. An updated report from an IFCN Committee. Clin. Neurophysiol. 126, 1071–1107. doi: 10.1016/j.clinph.2015.02.001
Rouiller, E. M., Moret, V., Tanné, J., and Boussaoud, D. (1996). Evidence for direct connections between the hand region of the supplementary motor area and cervical motoneurons in the macaque monkey. Eur. J. Neurosci. 8, 1055–1059. doi: 10.1111/j.1460-9568.1996.tb01592.x
Ruddy, K. L., Leemans, A., and Carson, R. G. (2017). Transcallosal connectivity of the human cortical motor network. Brain Struct. Funct. 222, 1243–1252. doi: 10.1007/s00429-016-1274-1
Rudy, B., Fishell, G., Lee, S., and Hjerling-Leffler, J. (2011). Three groups of interneurons account for nearly 100% of neocortical GABAergic neurons. Dev. Neurobiol. 71, 45–61. doi: 10.1002/dneu.20853
Rurak, B. K., Rodrigues, J. P., Power, B. D., Drummond, P. D., and Vallence, A.-M. (2021a). Reduced SMA-M1 connectivity in older than younger adults measured using dual-site TMS. Eur. J. Neurosci. 54, 6533–6552. doi: 10.1111/ejn.15438
Rurak, B. K., Rodrigues, J. P., Power, B. D., Drummond, P. D., and Vallence, A. M. (2021b). Test Re-test reliability of dual-site TMS measures of SMA-M1 connectivity differs across inter-stimulus intervals in younger and older adults. Neuroscience 472, 11–24. doi: 10.1016/j.neuroscience.2021.07.023
Sailer, A., Molnar, G. F., Cunic, D. I., and Chen, R. (2002). Effects of peripheral sensory input on cortical inhibition in humans. J. Physiol. 544, 617–629. doi: 10.1113/jphysiol.2002.028670
Sakai, K., Ugawa, Y., Terao, Y., Hanajima, R., Furubayashi, T., and Kanazawa, I. (1997). Preferential activation of different I waves by transcranial magnetic stimulation with a figure-of-eight-shaped coil. Exp. Brain Res. 113, 24–32. doi: 10.1007/BF02454139
Sanai, N., Nguyen, T., Ihrie, R. A., Mirzadeh, Z., Tsai, H.-H., Wong, M., et al. (2011). Corridors of migrating neurons in the human brain and their decline during infancy. Nature 478, 382–386. doi: 10.1038/nature10487
Sanchez-Vives, M. V., Massimini, M., and Mattia, M. (2017). Shaping the default activity pattern of the cortical network. Neuron 94, 993–1001. doi: 10.1016/j.neuron.2017.05.015
Saravanamuttu, J., Radhu, N., Udupa, K., Baarbé, J., Gunraj, C., and Chen, R. (2021). Impaired motor cortical facilitatory-inhibitory circuit interaction in Parkinson’s disease. Clin. Neurophysiol. 132, 2685–2692. doi: 10.1016/j.clinph.2021.05.032
Scala, F., Kobak, D., Shan, S., Bernaerts, Y., Laturnus, S., Cadwell, C. R., et al. (2019). Layer 4 of mouse neocortex differs in cell types and circuit organization between sensory areas. Nat. Commun. 10:4174. doi: 10.1038/s41467-019-12058-z
Scarabello, M., Righini, A., Severino, M., Pinelli, L., Parazzini, C., Scola, E., et al. (2021). Ganglionic eminence anomalies and coexisting cerebral developmental anomalies on fetal MR imaging: multicenter-based review of 60 cases. Am. J. Neuroradiol. 42:1151. doi: 10.3174/ajnr.A7062
Seo, J. P., and Jang, S. H. (2013). Different characteristics of the corticospinal tract according to the cerebral origin: DTI study. Am. J. Neuroradiol. 34:1359. doi: 10.3174/ajnr.A3389
Shepherd, G. M. G., and Yamawaki, N. (2021). Untangling the cortico-thalamo-cortical loop: cellular pieces of a knotty circuit puzzle. Nat. Rev. Neurosci. 22, 389–406. doi: 10.1038/s41583-021-00459-3
Siebner, H. R. (2020). Does TMS of the precentral motor hand knob primarily stimulate the dorsal premotor cortex or the primary motor hand area? Brain Stimul. 13, 517–518. doi: 10.1016/j.brs.2019.12.015
Silberberg, G., and Markram, H. (2007). Disynaptic inhibition between neocortical pyramidal cells mediated by martinotti cells. Neuron 53, 735–746. doi: 10.1016/j.neuron.2007.02.012
Simone, L., Viganò, L., Fornia, L., Howells, H., Leonetti, A., Puglisi, G., et al. (2021). Distinct functional and structural connectivity of the human hand-knob supported by intraoperative findings. J. Neurosci. 41:4223. doi: 10.1523/JNEUROSCI.1574-20.2021
Sohur, U. S., Padmanabhan, H. K., Kotchetkov, I. S., Menezes, J. R. L., and Macklis, J. D. (2014). Anatomic and molecular development of corticostriatal projection neurons in mice. Cereb. Cortex 24, 293–303. doi: 10.1093/cercor/bhs342
Sommer, M., Alfaro, A., Rummel, M., Speck, S., Lang, N., Tings, T., et al. (2006). Half sine, monophasic and biphasic transcranial magnetic stimulation of the human motor cortex. Clin. Neurophysiol. 117, 838–844. doi: 10.1016/j.clinph.2005.10.029
Sommer, M., Ciocca, M., Chieffo, R., Hammond, P., Neef, A., Paulus, W., et al. (2018). TMS of primary motor cortex with a biphasic pulse activates two independent sets of excitable neurones. Brain Stimul. 11, 558–565. doi: 10.1016/j.brs.2018.01.001
Sommer, M., Gileles, E., Knappmeyer, K., Rothkegel, H., Polania, R., and Paulus, W. (2012). Carbamazepine reduces short-interval interhemispheric inhibition in healthy humans. Clin. Neurophysiol. 123, 351–357. doi: 10.1016/j.clinph.2011.07.027
Stagg, C. J., Bestmann, S., Constantinescu, A. O., Moreno Moreno, L., Allman, C., Mekle, R., et al. (2011). Relationship between physiological measures of excitability and levels of glutamate and GABA in the human motor cortex. J. Physiol. 589, 5845–5855. doi: 10.1113/jphysiol.2011.216978
Szabadics, J., Varga, C., Molnár, G., Oláh, S., Barzó, P., and Tamás, G. (2006). Excitatory effect of GABAergic axo-axonic cells in cortical microcircuits. Science 311, 233–235. doi: 10.1126/science.1121325
Szentagothai, J., and Arbib, M. A. (1974). Conceptual models of neural organization. Neurosci. Res. Progr. Bull. 12, 510–510.
Takata, Y., Nakagawa, H., Ninomiya, T., Yamanaka, H., and Takada, M. (2021). Morphological features of large layer V pyramidal neurons in cortical motor-related areas of macaque monkeys: analysis of basal dendrites. Sci. Rep. 11:4171. doi: 10.1038/s41598-021-83680-5
Tanaka, Y. R., Tanaka, Y. H., Konno, M., Fujiyama, F., Sonomura, T., Okamoto-Furuta, K., et al. (2011). Local connections of excitatory neurons to corticothalamic neurons in the rat barrel cortex. J. Neurosci. 31:18223. doi: 10.1523/JNEUROSCI.3139-11.2011
Tasic, B., Yao, Z., Graybuck, L. T., Smith, K. A., Nguyen, T. N., Bertagnolli, D., et al. (2018). Shared and distinct transcriptomic cell types across neocortical areas. Nature 563, 72–78. doi: 10.1038/s41586-018-0654-5
Tazoe, T., and Perez, M. A. (2014). Selective activation of ipsilateral motor pathways in intact humans. J. Neurosci. 34:13924. doi: 10.1523/JNEUROSCI.1648-14.2014
Tiveron, M.-C., Beurrier, C., Céni, C., Andriambao, N., Combes, A., Koehl, M., et al. (2016). LAMP5 Fine-Tunes GABAergic synaptic transmission in defined circuits of the mouse brain. PLoS One 11:e0157052. doi: 10.1371/journal.pone.0157052
Tokimura, H., Ridding, M. C., Tokimura, Y., Amassian, V. E., and Rothwell, J. C. (1996). Short latency facilitation between pairs of threshold magnetic stimuli applied to human motor cortex. Electroencephalogr. Clin. Neurophysiol. Electromyogr. Motor Control 101, 263–272. doi: 10.1016/0924-980x(96)95664-7
Tokuno, H., and Nambu, A. (2000). Organization of nonprimary motor cortical inputs on pyramidal and nonpyramidal tract neurons of primary motor cortex: an electrophysiological study in the macaque monkey. Cereb. Cortex 10, 58–68. doi: 10.1093/cercor/10.1.58
Tremblay, R., Lee, S., and Rudy, B. (2016). GABAergic interneurons in the neocortex: from cellular properties to circuits. Neuron 91, 260–292. doi: 10.1016/j.neuron.2016.06.033
Tremblay, S., Beaulé, V., Proulx, S., de Beaumont, L., Marjañska, M., Doyon, J., et al. (2012). Relationship between transcranial magnetic stimulation measures of intracortical inhibition and spectroscopy measures of GABA and glutamate+glutamine. J. Neurophysiol. 109, 1343–1349. doi: 10.1152/jn.00704.2012
Trompetto, C., Bove, M., Marinelli, L., Avanzino, L., Buccolieri, A., and Abbruzzese, G. (2004). Suppression of the transcallosal motor output: a transcranial magnetic stimulation study in healthy subjects. Exp. Brain Res. 158, 133–140. doi: 10.1007/s00221-004-1881-6
Trompetto, C., Buccolieri, A., and Abbruzzese, G. (2001). Intracortical inhibitory circuits and sensory input: a study with transcranial magnetic stimulation in humans. Neurosci. Lett. 297, 17–20. doi: 10.1016/S0304-3940(00)01648-7
Turco, C. V., El-Sayes, J., Savoie, M. J., Fassett, H. J., Locke, M. B., and Nelson, A. J. (2018). Short- and long-latency afferent inhibition; uses, mechanisms and influencing factors. Brain Stimul. 11, 59–74. doi: 10.1016/j.brs.2017.09.009
Udupa, K., Ni, Z., Gunraj, C., and Chen, R. (2009). Interactions between short latency afferent inhibition and long interval intracortical inhibition. Exp. Brain Res. 199:177. doi: 10.1007/s00221-009-1997-9
Udupa, K., Ni, Z., Gunraj, C., and Chen, R. (2010). Effect of long interval interhemispheric inhibition on intracortical inhibitory and facilitatory circuits. J. Physiol. 588, 2633–2641. doi: 10.1113/jphysiol.2010.189548
Ugawa, Y., Hanajima, R., and Kanazawa, I. (1993). Interhemispheric facilitation of the hand area of the human motor cortex. Neurosci. Lett. 160, 153–155. doi: 10.1016/0304-3940(93)90401-6
Urban-Ciecko, J., and Barth, A. L. (2016). Somatostatin-expressing neurons in cortical networks. Nat. Rev. Neurosci. 17, 401–409. doi: 10.1038/nrn.2016.53
Van den Bos, M. A. J., Menon, P., Howells, J., Geevasinga, N., Kiernan, M. C., and Vucic, S. (2018). Physiological processes underlying short interval intracortical facilitation in the human motor cortex. Front. Neurosci. 12:240. doi: 10.3389/fnins.2018.00240
van Strien, M. E., van den Berge, S. A., and Hol, E. M. (2011). Migrating neuroblasts in the adult human brain: a stream reduced to a trickle. Cell Res. 21, 1523–1525. doi: 10.1038/cr.2011.101
Veres, J. M., Nagy, G. A., and Hájos, N. (2017). Perisomatic GABAergic synapses of basket cells effectively control principal neuron activity in amygdala networks. eLife 6:e20721. doi: 10.7554/eLife.20721
Volz, L. J., Eickhoff, S. B., Pool, E.-M., Fink, G. R., and Grefkes, C. (2015). Differential modulation of motor network connectivity during movements of the upper and lower limbs. NeuroImage 119, 44–53. doi: 10.1016/j.neuroimage.2015.05.101
Vucurovic, K., Gallopin, T., Ferezou, I., Rancillac, A., Chameau, P., van Hooft, J. A., et al. (2010). Serotonin 3A receptor subtype as an early and protracted marker of cortical interneuron subpopulations. Cereb. Cortex 20, 2333–2347. doi: 10.1093/cercor/bhp310
Wang, Y., Gupta, A., Toledo-Rodriguez, M., Wu, C. Z., and Markram, H. (2002). Anatomical, physiological, molecular and circuit properties of nest basket cells in the developing somatosensory cortex. Cereb. Cortex 12, 395–410. doi: 10.1093/cercor/12.4.395
Wang, Y., Toledo-Rodriguez, M., Gupta, A., Wu, C., Silberberg, G., Luo, J., et al. (2004). Anatomical, physiological and molecular properties of Martinotti cells in the somatosensory cortex of the juvenile rat. J. Physiol. 561, 65–90. doi: 10.1113/jphysiol.2004.073353
Wassermann, E. M., Pascual-Leone, A., and Hallett, M. (1994). Cortical motor representation of the ipsilateral hand and arm. Exp. Brain Res. 100, 121–132. doi: 10.1007/BF00227284
Wendt, K., Sorkhabi, M. M., O’Shea, J., Cagnan, H., and Denison, T. (2021). “Comparison between the modelled response of primary motor cortex neurons to pulse-width modulated and conventional TMS stimuli,” in Proceeding of the 2021 43rd Annual International Conference of the IEEE Engineering in Medicine & Biology Society (EMBC)), (Piscataway, NJ: IEEE), 6058–6061. doi: 10.1109/EMBC46164.2021.9629605
Wester, J. C., Mahadevan, V., Rhodes, C. T., Calvigioni, D., Venkatesh, S., Maric, D., et al. (2019). Neocortical projection neurons instruct inhibitory interneuron circuit development in a lineage-dependent manner. Neuron 102, 960.e966–975.e966. doi: 10.1016/j.neuron.2019.03.036
Wester, J. C., and McBain, C. J. (2014). Behavioral state-dependent modulation of distinct interneuron subtypes and consequences for circuit function. Curr. Opin. Neurobiol. 29, 118–125. doi: 10.1016/j.conb.2014.07.007
Wonders, C. P., and Anderson, S. A. (2006). The origin and specification of cortical interneurons. Nat. Rev. Neurosci. 7, 687–696. doi: 10.1038/nrn1954
Woodruff, A., Xu, Q., Anderson, S. A., and Yuste, R. (2009). Depolarizing effect of neocortical chandelier neurons. Front. Neural Circ. 3:15. doi: 10.3389/neuro.04.015.2009
Woodruff, A. R., McGarry, L. M., Vogels, T. P., Inan, M., Anderson, S. A., and Yuste, R. (2011). State-dependent function of neocortical chandelier cells. J. Neurosci. 31:17872. doi: 10.1523/JNEUROSCI.3894-11.2011
Xu, H., Jeong, H.-Y., Tremblay, R., and Rudy, B. (2013). Neocortical somatostatin-expressing GABAergic interneurons disinhibit the thalamorecipient layer 4. Neuron 77, 155–167. doi: 10.1016/j.neuron.2012.11.004
Yamawaki, N., Borges, K., Suter, B. A., Harris, K. D., and Shepherd, G. M. G. (2014). A genuine layer 4 in motor cortex with prototypical synaptic circuit connectivity. eLife 3:e05422. doi: 10.7554/eLife.05422
Yamawaki, N., and Shepherd, G. M. G. (2015). Synaptic circuit organization of motor corticothalamic neurons. J. Neurosci. 35:2293. doi: 10.1523/JNEUROSCI.4023-14.2015
Yousry, T. A., Schmid, U. D., Alkadhi, H., Schmidt, D., Peraud, A., Buettner, A., et al. (1997). Localization of the motor hand area to a knob on the precentral gyrus. A new landmark. Brain 120, 141–157. doi: 10.1093/brain/120.1.141
Zhang, M., Eichhorn, S. W., Zingg, B., Yao, Z., Cotter, K., Zeng, H., et al. (2021). Spatially resolved cell atlas of the mouse primary motor cortex by MERFISH. Nature 598, 137–143. doi: 10.1038/s41586-021-03705-x
Ziemann, U. (2020). I-waves in motor cortex revisited. Exp. Brain Res. 238:1601. doi: 10.1007/s00221-020-05764-4
Ziemann, U., Reis, J., Schwenkreis, P., Rosanova, M., Strafella, A., Badawy, R., et al. (2015). TMS and drugs revisited 2014. Clin. Neurophysiol. 126, 1847–1868. doi: 10.1016/j.clinph.2014.08.028
Ziemann, U., Tergau, F., Wischer, S., Hildebrandt, J., and Paulus, W. (1998). Pharmacological control of facilitatory I-wave interaction in the human motor cortex. A paired transcranial magnetic stimulation study. Electroencephalogr. Clin. Neurophysiol. Electromyogr. Motor Control 109, 321–330. doi: 10.1016/S0924-980X(98)00023-X
Keywords: motor cortex, neurocytology, neurophysiology, glutamate, GABA, transcranial magnetic stimulation (TMS), neurons
Citation: Tian D and Izumi S-I (2022) Transcranial Magnetic Stimulation and Neocortical Neurons: The Micro-Macro Connection. Front. Neurosci. 16:866245. doi: 10.3389/fnins.2022.866245
Received: 31 January 2022; Accepted: 28 February 2022;
Published: 12 April 2022.
Edited by:
Takashi Hanakawa, Kyoto University, JapanReviewed by:
Kazumasa Uehara, National Institute for Physiological Sciences (NIPS), JapanVincenzo Di Lazzaro, Campus Bio-Medico University, Italy
Copyright © 2022 Tian and Izumi. This is an open-access article distributed under the terms of the Creative Commons Attribution License (CC BY). The use, distribution or reproduction in other forums is permitted, provided the original author(s) and the copyright owner(s) are credited and that the original publication in this journal is cited, in accordance with accepted academic practice. No use, distribution or reproduction is permitted which does not comply with these terms.
*Correspondence: Dongting Tian, aXptdGVuQG1lZC50b2hva3UuYWMuanA=; Shin-Ichi Izumi, aXp1bWlzQG1lZC50b2hva3UuYWMuanA=