- 1Beijing Stomatological Hospital, Capital Medical University, Beijing, China
- 2Key Laboratory of Molecular Medicine and Biotherapy, Department of Biology, School of Life Sciences, Beijing Institute of Technology, Beijing, China
Background: Both animal studies and prospective observational studies on patients with neurodegenerative disease have reported a positive link between oral diseases and cognitive function. However, the effect of early tooth loss on hippocampal morphology remains unknown.
Methods: In this study, 6-week-old, male, juvenile Sprague–Dawley (SD) rats were randomized into the control (C) and tooth loss (TL) groups. In the TL group, all right maxillary molars of SD rats were extracted, while in the C group, no teeth were extracted. After 3 months, the learning and memory behavior were examined by Morris Water Maze (MWM), and the protein expression and mechanic signaling pathways were analyzed by real-time polymerase chain reaction, and cresyl violet staining.
Results: Two days after the operation, the body weight of both groups recovered and gradually returned to the level before operation. Three months after tooth extraction, the completion time of the C group in the MWM was significantly shorter than the TL group. The mRNA expression of BDNF, TrkB, AKT1, and NR2B in the C group were significantly higher than in the TL group. The pyramidal neurons in the TL group was fewer than in the C group.
Conclusion: Tooth loss in the juvenile SD rats will reduce the number of pyramidal neurons in the hippocampus, inhibit the expression of BDNF, TrkB, AKT1, and NR2B, and eventually lead to cognitive dysfunction.
Introduction
The population of aging people in the world has considerably increased, which is related to an increasing number of Alzheimer’s disease (AD) cases in the world. According to research, it is predicted that there will be about 75 million dementia patients in the world by 2030 (Katano et al., 2020). Therefore, clarifying the pathogenesis of dementia will help to develop new treatments. A large number of studies have shown that there are many associations between loss of occlusal support and cognitive dysfunction (Bruno et al., 2014; Chen et al., 2014; He et al., 2014; Pang et al., 2015). The incidence of tooth loss increases with age. And the impairment of masticatory function caused by tooth loss can lead to corresponding cognitive dysfunction diseases such as dementia and AD (Weijenberg et al., 2011; Lexomboon et al., 2012).
Several studies have shown that the loss of molars in aged rats will lead to the decline of masticatory function and subsequent learning and memory impairment. This leads to neuronal damage in brain regions related to learning and memory, resulting in the occurrence of AD and other diseases (Onozuka et al., 2000; Kubo et al., 2005, 2017; Pang et al., 2020). However, the effect of early tooth loss on hippocampal morphology remains unknown. If the elderly is understood to suffer from AD due to tooth loss, it remains to be seen to what extent tooth loss affects the younger population. This is of great significance for the prevention and treatment of cognitive dysfunction-related diseases.
Therefore, this study hypothesized that tooth loss in youth will lead to hippocampal injury and eventually to cognitive dysfunction in juvenile rats. The right maxillary molars of 6-week-old Sprague–Dawley (SD) rats were extracted. Three months after tooth extraction, the Morris Water Maze test was used to detect learning and memory behavior, and real-time polymerase chain reaction and cresol violet staining analysis, to determine whether tooth loss in juvenile rats could cause cognitive dysfunction.
Materials and Methods
Animals
Twenty juvenile, SD rats aged 6 weeks were randomly divided into two groups: tooth loss (TL) group and the control (C) group (n = 10, each group) (weight: 185–230 g). The animals were housed under standard conditions and exposed to a 12-h light-dark cycle and had ad libitum access to food and water. The experiment was approved by the Animal Experiment Committee of Capital Medical University. Each rat was anesthetized using a compound anesthetic (0.15 mg/kg medetomidine, 2.0 mg/kg midazolam, and 2.5 mg/kg butorphanol). All molars in the right maxillary region were removed in the TL group. No teeth were extracted in the C group. Finally, each rat was injected with buprenorphine (0.05 mg/kg) intramuscularly to alleviate suffering during surgery.
Morris Water Maze Test
The device consists of a circular water tank (120 cm in diameter) coated with black paint. The water tank is filled with water (24 ± 1°C). The labyrinth is divided into four quadrants. In one quadrant, place a transparent escape platform (10 cm in diameter); this platform is located 1 cm below the water surface. All rats received 5-day MWM training, 3 months after the extraction. During the training, each rat was placed into the water from the four quadrants to find an escape platform within 90 s. For rats that found the escape platform within 90 s, the time (in seconds) when they first found the platform was recorded; for rats that did not find it in 90 s, the time was recorded as 90 s. Rats that did not find the platform within 90 s during the training were artificially guided. They find the escape platform and stay on the platform for 20 s. After 5 days of training, a formal test was conducted. During the formal test, the escape platform was taken away. Each rat was placed into the water at a position opposite the platform, and they found the platform within 90 s. The time of the rats first passing the platform and the frequency of passing the platform were recorded. A CCD video camera took the test video by linking to a computer system.
Hippocampus Collection
The rats were anesthetized with intraperitoneal injection of 3% chloral hydrate (13 mL/kg) and killed. The brain was removed and fixed in 4% paraformaldehyde for 4–6 h, and then soaked in 20% sucrose overnight. The brain tissue was cut with a cryostat (Leica, Nußloch, Germany), to create 20 μM-thick coronal sections.
Cresol Violet Staining Analysis
After the frozen sections were washed three times with distilled water, the slides were immersed in 0.25% cresol violet (Sigma, St. Louis, MO, United States) for 30 s, washed with distilled water again, and passed through a graded series of alcohol (immersed in 70, 80, 90, and twice in 100% alcohol for 30 s each). The images were observed under an optical microscope (Zeiss, Axioskop 40, Germany) and processed using Axio vision 4.5 imaging software (Carl Zeiss).
Immunohistochemistry
After the frozen sections were washed three times with PBS for 5 min each time, blocked with immunol staining blocking buffer (Beyotime Institute of Biotechnology, China) for 30 min, and were then incubated with the primary antibody overnight at 4°C. The sections were washed three times with PBS for 5 min each time, incubated with secondary antibodies for 1 h at room temperature, and mounted with antifade medium containing 4′,6-diamidino-2-phenylindole (DAPI). The primary antibodies used in this study were as follows: glial fibrillary acidic protein (GFAP) (1:1000, Millipore, cat #MAB360). Goat anti rabbit IgG (1:100, Alexa Fluor® 488, ab150077) was used as the secondary antibody. Images of the sections were captured using a fluorescence microscope (Olympus/BX51, Japan).
Western Blot Analysis
Proteins were extracted with Applygen total protein extraction kit. Protein concentration was normalized using Coomassie brilliant blue G-250 staining. Equal amounts of proteins were separated by SDS-PAGE on 10% polyacrylamide gel, and the proteins were transferred to PVDF membrane. After blocking with 0.1% TBST containing 5% non-fat milk at room temperature for 2 h. The membranes were blocked with 5% non-fat milk at room temperature for 1 h and were then incubated overnight at 4°C with primary antibodies, including rabbit Anti-BDNF antibody (1:1000, Abcam ab108319), rabbit Anti-AKT1 + AKT2 + AKT3 antibody (1:1000, Abcam ab179463), rabbit Anti-PAX6 antibody (1:1000, Abcam, ab195045). Rabbit Anti-GAPDH (1:1000, Abcam ab128915) was used as an internal reference. The membranes were then incubated for 20 min at 37°C with goat anti-rabbit IgG (TDY bio, S004, China) and goat anti-mouse IgG (TDY bio, S001, China) for 1 h at room temperature. The images of the bands were captured using Tanon Image software (version 4100, Shanghai, China). The color was developed using the ECL kit. Images were acquired using the Fuji Digital Science Imager and analyzed with Gel-Pro Analyzer (Version 4.0) to measure the integrated optimal density (IOD) values of specific bands. The blots were analyzed by ImageJ software (Version 1.52t).
Real-Time Polymerase Chain Reaction
Rats were sacrificed, and brain tissue was isolated from the hippocampus on ice. Then, the hippocampi were frozen in liquid nitrogen and stored at −80°C. According to the manufacturer’s instructions, total RNA was extracted with RNAiso Plus (Takara, Japan). After the initial denaturation step at 95°C for 5 min, 40 cycles of denaturation at 95°C for 5 s, annealing at 60°C for 10 s, and extension for 30 s were carried out. Rotor gene SYBR Green RT-PCR kit (Qiagen, Japan) with Corbett rotor gene RG-3000a real-time PCR system (Rotor-Gene 6.1.93, Corbett) was used. The data were evaluated using the RG-3000a software program (rotor gene version 6.1.93, Corbett). All RT-PCR experiments were repeated thrice. The primer sequences used were as follows: rat Brain-derived neurotrophic factor (BDNF): 5′-CCATAAGGACGCGGACTTGT-3′ and 5′-GAGGCTCCAAAGGCACTTGA-3′; rat Tyrosine Kinase receptor B (TrkB): 5′-ATTGACCCAGAGAACATCAC-3′ and 5′-CAGGAAATGGTCACAGACTT-3′; rat Protein kinase B-1 (AKT1): 5′-GTGGCAAGATGTGTATGAG-3′ and 5′-CTGGCTGAGTAGGAGAAC-3′; rat N-methyl-D-aspartate receptor 2B (NR2B): CGCATCTGTCCACCATT-3′ and 5′-GCATCAGGAAAGCCTCG-3′.
Statistical Analyses
SPSS 23.0 (IBM, Armonk, NY, United States) was used for data analyses. All experiments were repeated independently thrice. Data are expressed as mean ± standard deviation. The experimental data of each group showed normal distribution and homogeneity of variance. One-way analysis of variance with t-test was performed. P < 0.05 was considered to indicate statistical significance.
Results
Weight Change
As shown in Figure 1, the body weight of the two groups decreased to varying degrees after the extraction. From 2 days after the extraction, the body weight of both groups recovered and gradually returned to the level before operation. After weight recovery, the weight of the two groups increased gradually in a similar trend.
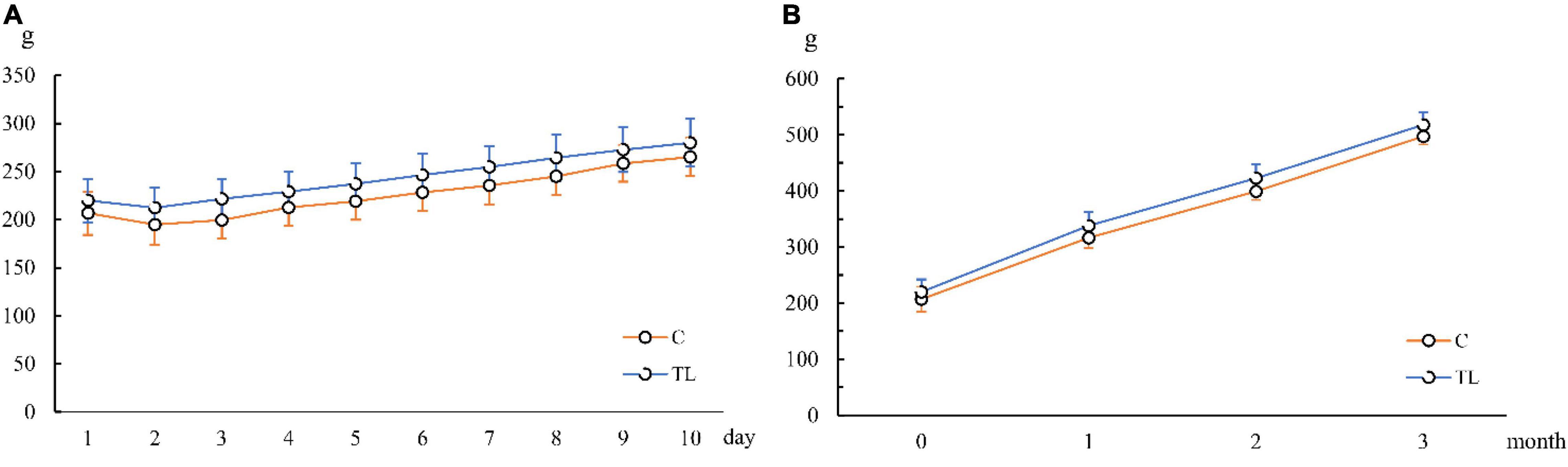
Figure 1. Changes of average body weight of juvenile SD rats. (A) Changes of average body weight in 10 days after tooth extraction. (B) Changes of average body weight in 3 months after tooth extraction. The orange line represents the C group and the blue line represents the TL group.
Morris Water Maze
As shown in Figure 2, the MWM results showed that the C group performed better in finding the escape platform and had lower crossing times than the TL group (P < 0.01). In the C group, the average time of crossing the platform for the first time was 9.6 ± 4.27 s, and the average crossing times for the platform was 4.9 ± 0.83, and the motion trajectory was regular. The average first crossing time of the TL group was 24.6 ± 10.16 s, and the average crossing time was 2.8 ± 1.08; the motion trajectory was disordered and irregular.
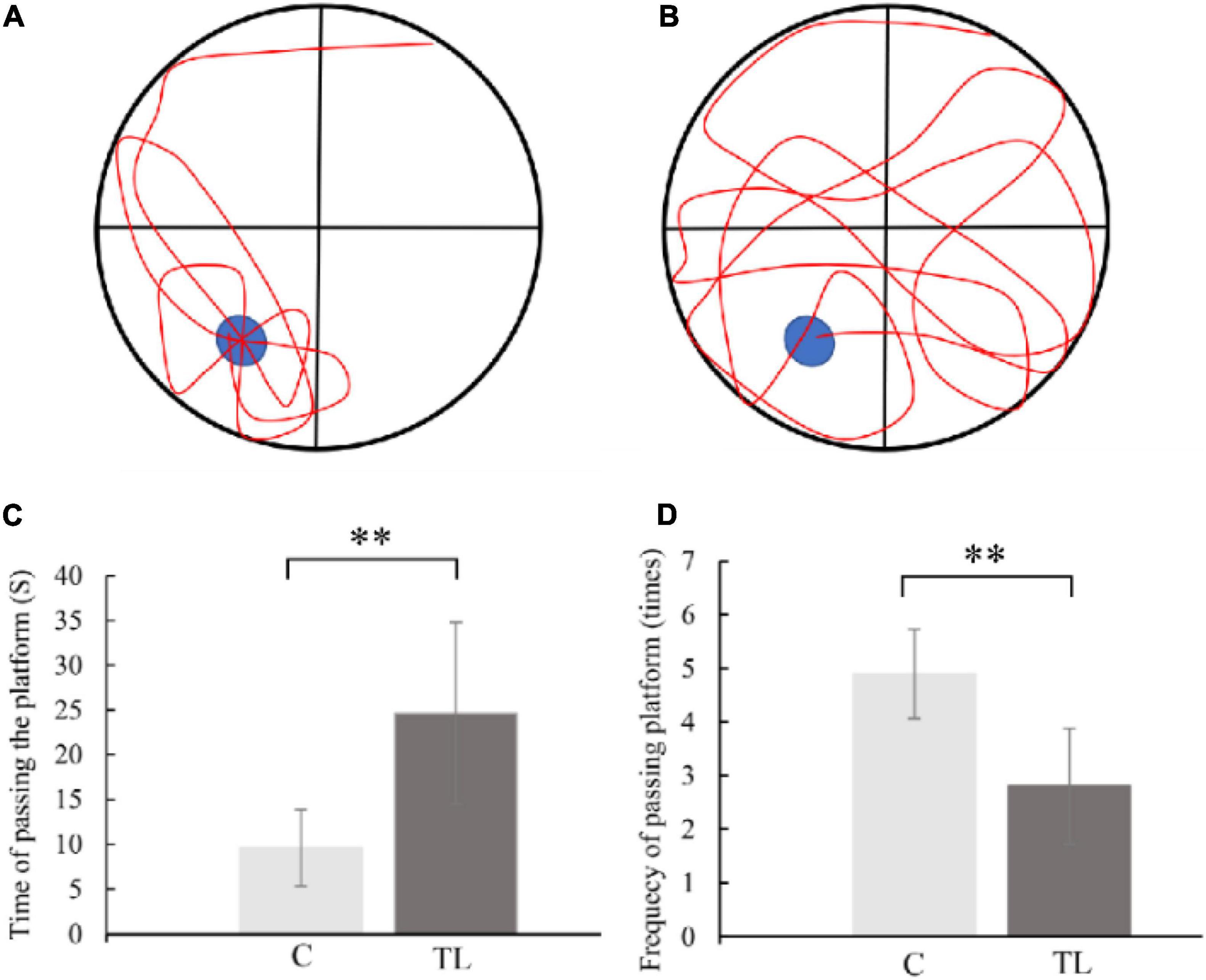
Figure 2. (A) Schematic diagram of C group looking for platform trajectory. The blue circle represents the position of life-saving platform and red represents the trajectory of movement of rats. (B) Schematic diagram of TL group looking for platform trajectory. The blue circle represents the position of life-saving platform and red represents the trajectory of movement of rats. (C) The data are expressed as the mean ± SD, **indicates that there is a statistical difference between the two groups (t-test, **P < 0.01). (D) The data are expressed as the mean ± SD, **indicates that there is a statistical difference between the two groups (t-test, **P < 0.01).
Pyramidal Cell
Pyramidal cell layers of different thickness were seen in hippocampal CA1 and CA3 regions of SD rats in each group (Figure 3). Further, layers of pyramidal neurons could be seen in the CA1 region were 4.8 ± 0.75 in C group, 3.0 ± 0.89 in TL group which was significantly less than C group (P < 0.01) and the cells were arranged in a disorderly manner. Layers of pyramidal neurons in the CA3 region were 5.0 ± 0.63 in C group, 3.6 ± 0.66 in TL group which was significantly less than C group (P < 0.01).
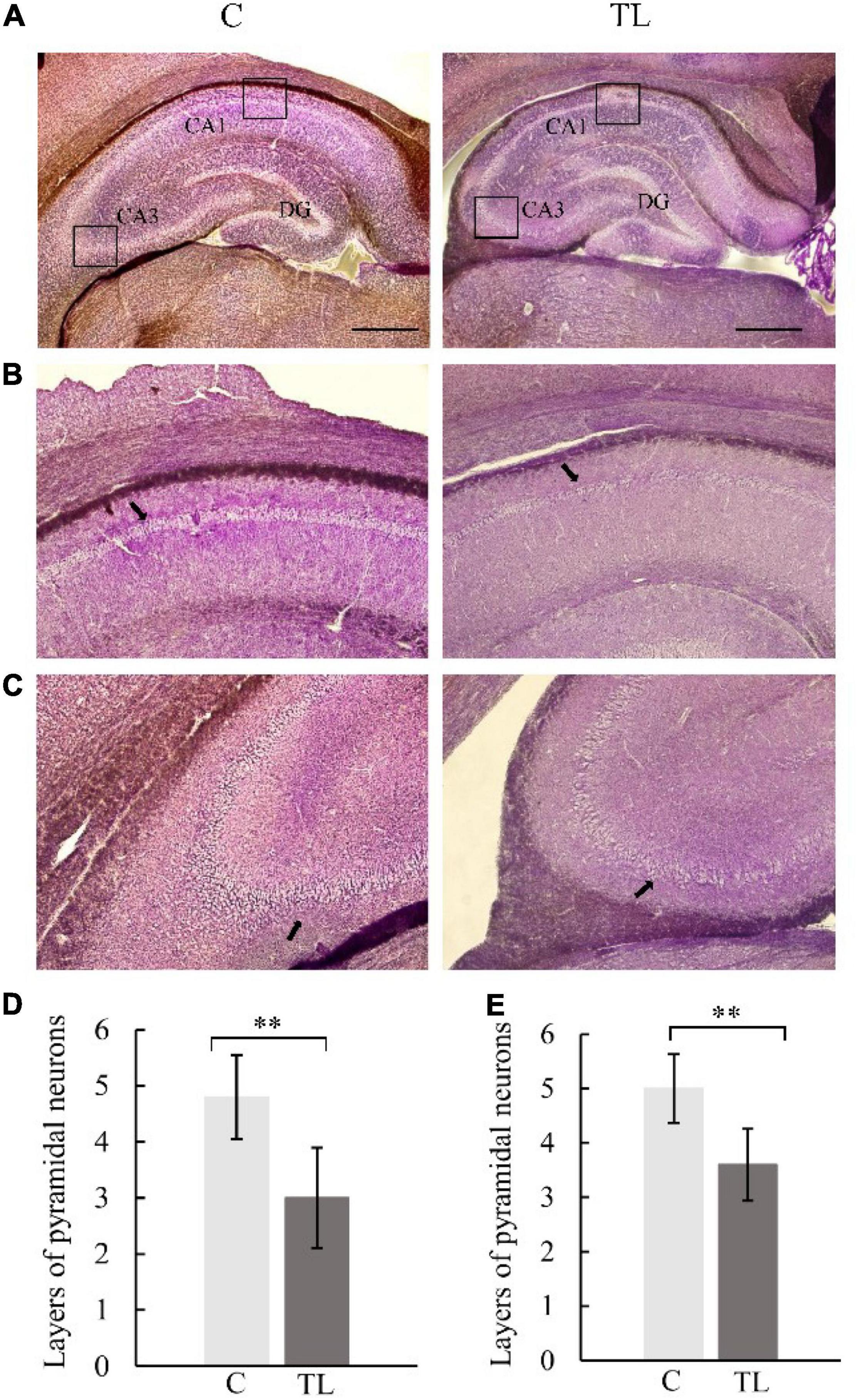
Figure 3. Optical micrograph of rat hippocampus. (A) The morphology of hippocampus in the C group and TL group was similar, with a scale of 1 mm. (B) Vertebral cell layer in CA1 region of the C and TL groups, with black arrow identifying the pyramidal cells. (C) Vertebral cell layer in the CA3 region of the C and TL groups, with black arrow identifying the pyramidal cells. (D) The layers number of pyramidal neurons in CA1 region in two groups. The data are expressed as the mean ± SD, **indicates that there is a statistical difference between the two groups (t-test, **P < 0.01). (E) The layers number of pyramidal neurons in CA3 region in two groups. The data are expressed as the mean ± SD, **indicates that there is a statistical difference between the two groups (t-test, **P < 0.01).
Astrocyte
The activation of astrocytes in the CA1 of hippocampus (Figure 4B). A fluorescence detection of glial fibrillary acidic protein [GFAP (green)], a marker of activated astrocytes in hippocampus. The immunohistochemistry result showed that the number of GFAP-positive cells in CA1 of hippocampus was significantly higher in TL group than in C group.
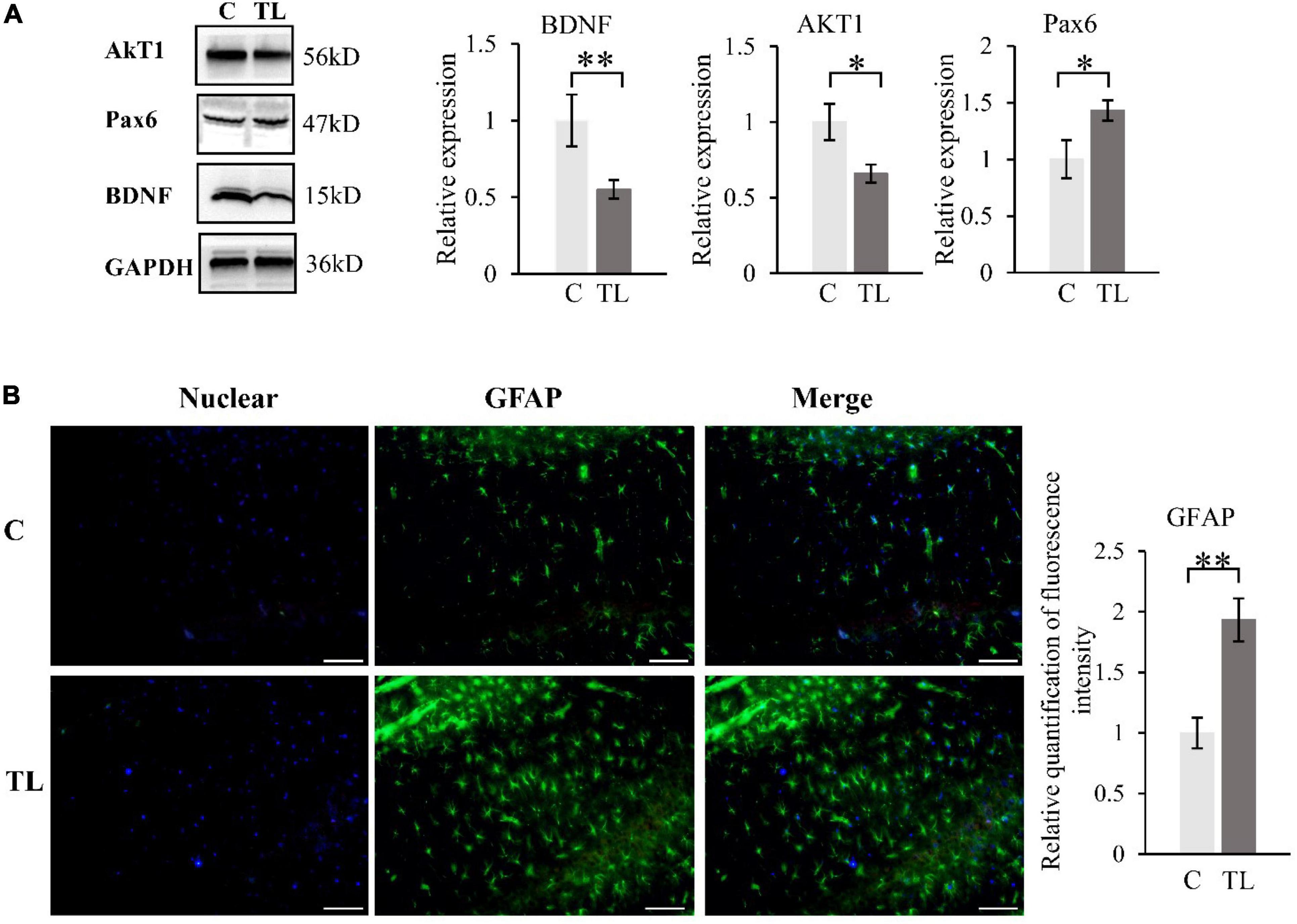
Figure 4. (A) WB results about BDNF, AKT1 and PAX6. The data are expressed as the mean ± SD, *indicates that there is a statistical difference between the two groups (t-test, *P < 0.05, **P < 0.01). (B) The activation of astrocytes in the CA1 of hippocampus. A Fluorescence detection of GFAP (green), Nuclear was labelled with DAPI (shown in blue). Quantification of fluorescence intensity of Astrocyte in two groups (t-test, **P < 0.01). The scale bar is 50 μm.
Western Blot Analysis
We measured and analyzed the expression of BDNF, AKT1, and Pax6 by WB (Figure 4A). Compared to the C group, the BDNF and AKT1 in the TL group decreased, while the Pax6 increased (t-test, *P < 0.05, **P < 0.01).
Real-Time Polymerase Chain Reaction Analysis
We measured and analyzed the expression of BDNF, TrkB, AKT1, and NR2B by RT-PCR (Figure 5). Compared with the C group, the expression of BDNF, TrkB, AKT1, and NR2B in the TL group decreased by varying degrees (P < 0.05). t-test, *P < 0.05, **P < 0.01.
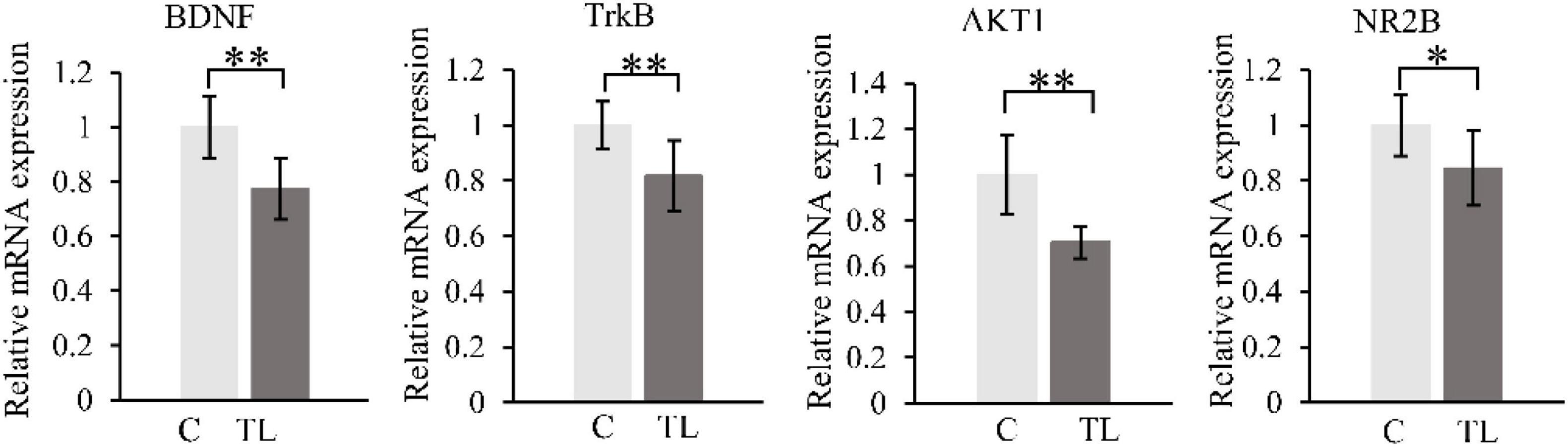
Figure 5. RT-PCR results. BDNF (A), TrkB (B), AKT1 (C), and NR2B (D). The data are expressed as the mean ± SD, *indicates that there is a statistical difference between the two groups (t-test, *P < 0.05, **P < 0.01).
Discussion
Several studies have shown that the loss of teeth in elderly rats will lead to the decline of masticatory function, learning and memory impairment, and then lead to occurrence of AD and other diseases (Luo et al., 2019; Goto et al., 2020). However, whether early tooth loss in juvenile rats can affect cognitive function is still unknown. The purpose of this study was to observe the changes of hippocampal structure caused by early tooth loss in juvenile SD rats, and the subsequent impact regarding cognitive dysfunction.
In this study, we first observed that the changes of daily food intake and body weight in the C and TL groups showed a similar trend: 1 day after the extraction, food intake decreased and body weight decreased; 2 days after the extraction, the body weight gradually increased and returned to the level before tooth extraction. Therefore, in the entire study, there was no significant difference in body weight between the two groups, excluding the interference of malnutrition on this experiment (Jiang et al., 2011).
The hippocampus is crucial for memory storage and conversion (Mujawar et al., 2021; Prince et al., 2021). It contains the CA1–4 region, which is mainly composed of pyramidal neurons. The CA1 region is responsible for memory information coding, and the CA3 region is responsible for spatial information coding (Aggleton, 2012; Kesner, 2013). This study found that teeth loss in juvenile rats can lead to the decrease of pyramidal cells in CA1 and CA3 regions and the decline of hippocampal ability to recognize familiar objects and read spatial information, i.e., the decline of spatial and learning and memory ability. This is consistent with the MWM experimental results. The C group could quickly and accurately find the corresponding platform position in the MWM test after 5 days of learning. The TL group showed chaotic trajectory and unclear direction. This result indicated that tooth loss could reduce the density of neurons in CA1 and CA3 regions, and the distribution of pyramidal neurons was irregular. The results shown that tooth loss in the early age could lead to the remodeling of the ultrastructure of the hippocampus and impaired memory storage, which is manifested as a decline in learning and memory ability. Astrocytes, a type of glial cells in the brain, support essential functions such as maintenance of neurotransmitter pools, trophic support, metabolism, synaptic formation and plasticity, myelin sheath formation, injury healing, and immune surveillance (Burda et al., 2016; Manninen et al., 2020). After exogenous chemicals or trauma to the central nervous system, astrocytes in the injured area can form a glial “scar” through proliferation. This proliferation is often accompanied by increased expression of GFAP (Onozuka et al., 2000). Reactive astrocytes release of numerous effector molecules such as cytokines, chemokines, and proteases. When the brain exhibits a pathological response, reactive astrocytes induce the release of a variety of multiple harmful factors, such as IL-1β, IL-6, and IL-17 (Araki et al., 2020). The release of these factors inhibits BDNF expression, reduces or cause synaptic dysfunction, impairs nerve growth and induces neuronal cell death (Cohen and Torres, 2019). We used GFAP immunostaining to determine reactive astrocyte numbers to estimate the effect of tooth loss conditions on the hippocampus. We found that the number of GFAP-positive astrocytes was significantly increased in the CA1 region in the TL group, suggesting that this region is particularly susceptible to Cognitive dysfunction due to decreased chewing ability, which is also consistent with the results in the MWM.
BDNF is a member of the neurotrophic factor family, which is widely distributed in the central nervous system, peripheral nervous system, endocrine system, and other regions. The BDNF concentration is the highest in the hippocampus (Canivet et al., 2015). It plays a role by binding with TrkB. The intracellular region of TrkB has intrinsic tyrosine kinase activity. After binding with TrkB, BDNF activates the intracellular region, resulting in enhanced self-phosphorylation of TrkB, which leads to promote the survival of nerve cells and increase synaptic plasticity and neurogenesis (Xiang et al., 2014; Peng et al., 2020; Liu et al., 2021; Wang et al., 2022). Increasing the synaptic plasticity can affect long-term potentiation and enhance the learning process and memory formation. This study shows that tooth loss reduces the expression levels of BDNF and TrkB, resulting in the weakening of learning ability and long-term memory ability of juvenile SD rats. However, BDNF can activate phosphatidylinositol 3-kinase (PI3K)-protein kinase B (Akt) pathway after binding to the TrkB receptor (Jeong et al., 2021; Li et al., 2021). This pathway can regulate the proliferation and differentiation of neural stem cells and repair neuronal injury (Lv et al., 2016; Fu et al., 2020). This study shows that tooth loss reduces the expression level of AKT1, thereby inhibiting the proliferation and differentiation of neural stem cells and the repair of neuronal damage, which also leads to the weakening of learning ability and long-term memory ability of juvenile SD rats. The same results also appeared in the expression of NR2B. NR2B has an important relationship of N-methyl-D-aspartic acid (NMDA) nerve endings in the brain, which is related to memory function (Faure et al., 2007; Melike et al., 2021). This study also showed that tooth loss reduces the expression of NR2B, and then reduces the learning ability of juvenile SD rats. Pax6 is an important transcription factor for eye, brain and olfactory system development, some researchers found that there are probably more targets and downstream pathways of Pax6 involved in Alzheimer’s disease pathogenesis (Dunckley et al., 2006; Zhang et al., 2021). And Pax6 expression is increased in the brains of APP transgenic mice and human Alzheimer’s disease patients. In our research, it was also found that tooth loss leads to increased expression of Pax6, which leads to cognitive impairment in rats.
We speculate that after tooth loss in juvenile SD rats, dental pulp- and periodontal membrane-derived neural signals are reduced, and afferent nerve fibers in the trigeminal nucleus are reduced. On the one hand, it affects BDNF/TrkB and PI3K/Akt pathways to inhibit the proliferation and differentiation of neural stem cells and the repair of neuronal damage. On the other hand, increasing the expression of Pax6 increased the amyloid-β-induced neuronal death and tau phosphorylation, eventually lead to the occurrence of AD and other diseases.
The limitation of this study is that we extracted all the right maxillary molars of juvenile SD rats, and set up different experimental groups for the number of lost teeth. Therefore, in future studies, we hope to explore whether the number of lost teeth has an impact on the learning and memory ability of juvenile SD rats.
Conclusion
Tooth loss in juvenile SD rats will reduce synaptic function, impairs nerve growth and induces neuronal cell death in the hippocampus, and inhibit the proliferation and differentiation of neural stem cells and the repair of neuronal damage, finally lead to cognitive dysfunction.
Data Availability Statement
The raw data supporting the conclusions of this article will be made available by the authors, and the generated datasets are available by request to the corresponding author.
Ethics Statement
The animal study was reviewed and approved by the Animal Experiment Committee of Capital Medical University.
Author Contributions
JH contributed to the design of the experiments, collection and assembly of all data, data analysis and interpretation, and manuscript writing. XW contributed to the design of the experiments and collection and assembly of all data. WK participated in the data collection and analysis. QJ conceived the idea and contributed to data interpretation. All authors have approved the final version of the manuscript.
Funding
This work was supported by the National Natural Science Foundation of China (Grant Nos. 81771094 and 2170980) and Beijing Stomatological Hospital, Capital Medical University Young Scientist Program (No. YSP202003).
Conflict of Interest
The authors declare that the research was conducted in the absence of any commercial or financial relationships that could be construed as a potential conflict of interest.
Publisher’s Note
All claims expressed in this article are solely those of the authors and do not necessarily represent those of their affiliated organizations, or those of the publisher, the editors and the reviewers. Any product that may be evaluated in this article, or claim that may be made by its manufacturer, is not guaranteed or endorsed by the publisher.
References
Aggleton, J. P. (2012). Multiple anatomical systems embedded within the primate medial temporal lobe: implications for hippocampal function. Neurosci. Biobehav. Rev. 15, 1579–1596. doi: 10.1016/j.neubiorev.2011.09.005
Araki, T., Ikegaya, Y., and Koyama, R. (2020). The effects of microglia- and astrocyte-derived factors on neurogenesis in health and disease. Euro. J. Neurosci. 54, 5880–5901. doi: 10.1111/ejn.14969
Bruno, T. F., Luanna, D. M. P. F., Tavares, N. P. A., Raiol, D. S. M. A., Walace, G. L., Cristiane, D. S. F. M., et al. (2014). Masticatory deficiency as a risk factor for cognitive dysfunction. Int. J. Med. Sci. 11, 209–214. doi: 10.7150/ijms.6801
Burda, J. E., Bernstein, A. M., and Sofroniew, M. V. (2016). Astrocyte roles in traumatic brain injury. Exp. Neurol. 275, 305–315. doi: 10.1016/j.expneurol.2015.03.020
Canivet, A., Cédric, T., Albinet, André, N., Pylouster, J., Rodríguez-Ballesteros, M., et al. (2015). Effects of bdnf polymorphism and physical activity on episodic memory in the elderly: a cross sectional study. Euro. Rev. Aging Phys. Act. 12:15. doi: 10.1186/s11556-015-0159-2
Chen, H., Iinuma, M., Onozuka, M., and Kubo, K. Y. (2014). Chewing maintains hippocampus-dependent cognitive function. Int. J. Med. Sci. 12, 502-9. doi: 10.7150/ijms.11911
Cohen, J., and Torres, C. (2019). Astrocyte senescence: evidence and significance. Aging cell 18:e12937. doi: 10.1111/acel.12937
Dunckley, T., Beach, T. G., Ramsey, K. E., Grover, A., Mastroeni, D., Walker, D. G., et al. (2006). Gene expression correlates of neurofibrillary tangles in Alzheimer’s disease. Neurobiol Aging 27, 1359–1371. doi: 10.1016/j.neurobiolaging.2005.08.013
Faure, J., Uys, J., Marais, L., Dan, J. S., and Daniels, W. (2007). Early maternal separation alters the response to traumatization: resulting in increased levels of hippocampal neurotrophic factors. Metab. Brain Dis. 22, 183–195. doi: 10.1007/s11011-007-9048-3
Fu, S., Luo, X., Wu, X., Zhang, T., Gu, L., Wang, Y., et al. (2020). Activation of the melanocortin-1 receptor by Ndp-Msh attenuates oxidative stress and neuronal apoptosis through Pi3k/Akt/Nrf2 pathway after intracerebral hemorrhage in mice. Oxid. Med. Cell. longev. 2020:8864100. doi: 10.1155/2020/8864100.8864100
Goto, T., Kuramoto, E., Dhar, A., Wang, P. H., and Chang, C. C. (2020). Neurodegeneration of trigeminal mesencephalic neurons by the tooth loss triggers the progression of alzheimer’s disease in 3×tg-ad model mice. J. Alzheim. Dis. 76, 1443–1459. doi: 10.3233/JAD-200257
He, Y., Zhu, J., Huang, F., Liu, Q., Fan, W., and He, H. (2014). Age-dependent loss of cholinergic neurons in learning and memory-related brain regions and impaired learning in SAMP8 mice with trigeminal nerve damage. Neural Regen. Res. 9, 1985–1994. doi: 10.4103/1673-5374.145380
Jeong, Y. H., Kim, T. I., Oh, Y. C., and Ma, J. Y. (2021). Chrysanthemum indicum Prevents Hydrogen Peroxide-Induced Neurotoxicity by Activating the TrkB/Akt Signaling Pathway in Hippocampal Neuronal Cells. Nutrients. 13:3690. doi: 10.3390/nu13113690
Jiang, Q. S., Liang, Z. L., Wu, M. J., Feng, L., Liu, L. L., Zhang, J. J., et al. (2011). Reduced brain-derived neurotrophic factor expression in cortex and hippocampus involved in the learning and memory deficit in molarless SAMP8 mice. Chin. Med. J. 2011, 1540–1544.
Katano, M., Kajimoto, K., Iinuma, M., Azuma, K., and Kubo, K. Y. (2020). Tooth loss early in life induces hippocampal morphology remodeling in senescence-accelerated mouse prone 8 (samp8) mice. Int. J. Med. Sci. 17, 517–524. doi: 10.7150/ijms.40241
Kesner, P. (2013). A process analysis of the CA3 subregion of the hippocampus. Front. Cell Neurosci. 7:78. doi: 10.3389/fncel.2013.00078
Kubo, K. Y., Iwaku, F., Watanabe, K., Fujita, M., and Onozuka, M. (2005). Molarless-induced changes of spines in hippocampal region of SAMP8 mice. Brain Res. 15, 191–195. doi: 10.1016/j.brainres.2005.07.038
Kubo, K. Y., Murabayashi, C., Kotachi, M., Suzuki, A., Mori, D., Sato, Y., et al. (2017). Tooth loss early in life suppresses neurogenesis and synaptophysin expression in the hippocampus and impairs learning in mice. Arch. Oral. Biol. 2017, 21–27. doi: 10.1016/j.archoralbio.2016.11.005
Lexomboon, D., Trulsson, M., Wårdh, I., and Parker, M. G. (2012). Chewing ability and tooth loss: association with cognitive impairment in an elderly population study. J. Am. Geriatr. Soc. 60, 1951–1956. doi: 10.1111/j.1532-5415.2012.04154.x
Li, C. X., Sui, C. L., Wang, W., Yan, J. T., Deng, N., Du, X., et al. (2021). Baicalin attenuates oxygen-glucose deprivation/reoxygenation-induced injury by modulating the BDNF-TrkB/PI3K/Akt and MAPK/Erk1/2 signaling axes in neuron-astrocyte cocultures. Front. Pharmacol. 12:599543. doi: 10.3389/fphar.2021.599543
Liu, B. P., Zhang, Y. P., Yang, Z. Y., Liu, M. J., Zhang, C., Zhao, Y. T., et al. (2021). ω-3 DPA Protected Neurons from Neuroinflammation by Balancing Microglia M1/M2 Polarizations through Inhibiting NF-κB/MAPK p38 Signaling and Activating Neuron-BDNF-PI3K/AKT Pathways. Mar Drugs 19:87. doi: 10.3390/md19110587
Luo, B., Pang, Q., and Jiang, Q. (2019). Tooth loss causes spatial cognitive impairment in rats through decreased cerebral blood flow and increased glutamate. Arch. Oral Biol. 102, 225–230. doi: 10.1016/j.archoralbio.2019.05.004
Lv, B., Feng, L., Fang, J., Xu, L., Sun, C., Han, J., et al. (2016). Activated microglia induce bone marrow mesenchymal stem cells to produce glial cell-derived neurotrophic factor and protect neurons against oxygen-glucose deprivation injury. Front. Cell. Neurosci. 2016:283. doi: 10.3389/fncel.2016.00283
Manninen, T., Saudargiene, A., and Linne, M. L. (2020). Astrocyte-mediated spike-timing-dependent long-term depression modulates synaptic properties in the developing cortex. PLoS Comput. Biol. 16:e1008360. doi: 10.1371/journal.pcbi.1008360
Melike, K., Aslıhan, D., Selçuk, C., Aruz, B., Elif, A., Özlem, G., et al. (2021). Behavioral and Neurodevelopmental Effects of Early Interventions in Adult Wistar Rats. Noro Psikiyatr. Ars. 58, 137–145. doi: 10.29399/npa.24943
Mujawar, S., Patil, J., Chaudhari, B., and Saldanha, D. (2021). Memory: Neurobiological mechanisms and assessment. Ind. Psychiatry J. 30(Suppl. 1), S311–S314. doi: 10.4103/0972-6748.328839
Onozuka, M., Watanabe, K., Nagasaki, S., Jiang, Y., and Nagatsu, I. (2000). Impairment of spatial memory and changes in astroglial responsiveness following loss of molar teeth in aged samp8 mice. Behav. Brain Res. 108, 145–155. doi: 10.1016/s0166-4328(99)00145-x
Pang, Q., Hu, X., Li, X., Zhang, J., and Jiang, Q. (2015). Behavioral impairments and changes of nitric oxide and inducible nitric oxide synthase in the brains of molarless km mice. Behav. Brain Res. 278, 411–416. doi: 10.1016/j.bbr.2014.10.020
Pang, Q., Wu, Q., Hu, X., Zhang, J., and Jiang, Q. (2020). Tooth loss, cognitive impairment and chronic cerebral ischemia. J. Dental Sci. 15, 84–91. doi: 10.1016/j.jds.2019.09.001
Peng, Z. L., Zhang, C., Yan, L., Zhang, Y. P., Yang, Z. Y., Wang, J. J., et al. (2020). EPA is more effective than DHA to improve depression-like behavior, Glia cell dysfunction and Hippcampal Apoptosis signaling in a chronic stress-induced rat model of depression. Int. J. Mol. Sci. 2020:1769. doi: 10.3390/ijms21051769
Prince, L. Y., Travis, B., Rachel, H., Krasimira, T. A., Claudia, C., and Mellor, J. R. (2021). Separable actions of acetylcholine and noradrenaline on neuronal ensemble formation in hippocampal ca3 circuits. PLoS Comput Biol. 17:e1009435. doi: 10.1371/journal.pcbi.1009435
Wang, W. Z., Liu, X., Yang, Z. Y., Wang, Y. Z., and Lu, H. T. (2022). Diffusion tensor imaging of the hippocampus reflects the severity of hippocampal injury induced by global cerebral ischemia/reperfusion injury. Neural Regen Res. 17, 838–844. doi: 10.4103/1673-5374.322468
Weijenberg, R., Scherder, E., and Lobbezoo, F. (2011). Mastication for the mind—the relationship between mastication and cognition in ageing and dementia. Neurosci. Biobehav. Rev. 35, 483–497. doi: 10.1016/j.neubiorev.2010.06.002
Xiang, J., Pan, J., Chen, F. J., Zheng, L. L., Chen, Y., Zhang, S. T., et al. (2014). L-3-n-butylphthalide improves cognitive impairment of APP/PS1 mice by BDNF/TrkB/PI3K/AKT pathway. Int. J. Clin. Exp. Med. 2014, 1706–1713.
Keywords: tooth loss, cognitive dysfunction, Alzheimer’s disease (AD), hippocampus, brain-derived neurotrophic factor (BDNF)
Citation: Hu J, Wang X, Kong W and Jiang Q (2022) Tooth Loss Suppresses Hippocampal Neurogenesis and Leads to Cognitive Dysfunction in Juvenile Sprague–Dawley Rats. Front. Neurosci. 16:839622. doi: 10.3389/fnins.2022.839622
Received: 20 December 2021; Accepted: 15 March 2022;
Published: 28 April 2022.
Edited by:
Zhou Wu, Kyushu University, JapanReviewed by:
Junjun Ni, Beijing Institute of Technology, ChinaHongchen Liu, First Medical Center of PLA General Hospital, China
Ting Jiang, Peking University School of Stomatology, China
Copyright © 2022 Hu, Wang, Kong and Jiang. This is an open-access article distributed under the terms of the Creative Commons Attribution License (CC BY). The use, distribution or reproduction in other forums is permitted, provided the original author(s) and the copyright owner(s) are credited and that the original publication in this journal is cited, in accordance with accepted academic practice. No use, distribution or reproduction is permitted which does not comply with these terms.
*Correspondence: Qingsong Jiang, cXNqaWFuZzc0QGhvdG1haWwuY29t