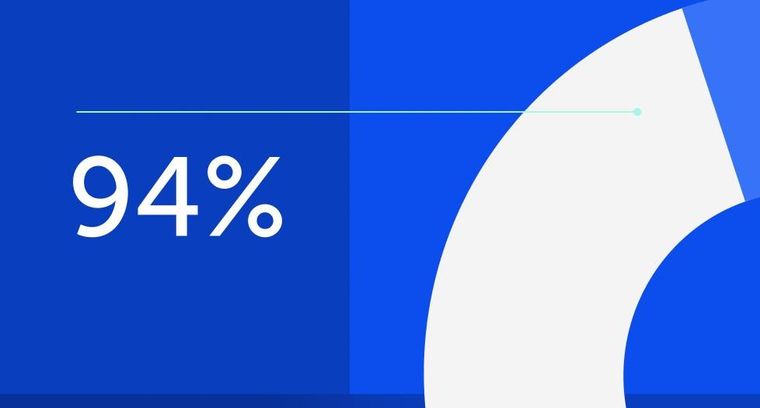
94% of researchers rate our articles as excellent or good
Learn more about the work of our research integrity team to safeguard the quality of each article we publish.
Find out more
SYSTEMATIC REVIEW article
Front. Neurosci., 09 March 2022
Sec. Perception Science
Volume 16 - 2022 | https://doi.org/10.3389/fnins.2022.790130
This article is part of the Research TopicAccessory Cells of Sensory SystemsView all 5 articles
Sensory corpuscles, or cutaneous end-organ complexes, are complex structures localized at the periphery of Aβ-axon terminals from primary sensory neurons that primarily work as low-threshold mechanoreceptors. Structurally, they consist, in addition to the axons, of non-myelinating Schwann-like cells (terminal glial cells) and endoneurial- and perineurial-related cells. The terminal glial cells are the so-called lamellar cells in Meissner and Pacinian corpuscles. Lamellar cells are variably arranged in sensory corpuscles as a “coin stack” in the Meissner corpuscles or as an “onion bulb” in the Pacinian ones. Nevertheless, the origin and protein profile of the lamellar cells in both morphotypes of sensory corpuscles is quite similar, although it differs in the expression of mechano-gated ion channels as well as in the composition of the extracellular matrix between the cells. The lamellar cells have been regarded as supportive cells playing a passive role in the process of genesis of the action potential, i.e., the mechanotransduction process. However, they express ion channels related to the mechano–electric transduction and show a synapse-like mechanism that suggest neurotransmission at the genesis of the electrical action potential. This review updates the current knowledge about the embryonic origin, development modifications, spatial arrangement, ultrastructural characteristics, and protein profile of the lamellar cells of cutaneous end-organ complexes focusing on Meissner and Pacinian morphotypes.
A subset of peripheral axon terminals from primary sensory nerve fibers reaches the vertebrate dermis and contacts with distinct differentiated cells to form microscopic sensory organs referred to as sensory corpuscles (Zimmerman et al., 2014; Cobo et al., 2021). Recently, Handler and Ginty (2021) used the term cutaneous end-organ complexes (CEOCs) to refer to these structures, which is surely more appropriate. CEOCs are continuous with nerve fibers whose axons originate from intermediate- or large-sized mechanosensory neurons that work as low threshold mechanoreceptors (LTMRs) [see for a review (Abraira and Ginty, 2013)]. LTMRs can be divided into two categories: rapidly adapting (RA) and slowly adapting (SA) mechanoreceptors. Both, in turn, have type I and type II variants (Zimmerman et al., 2014; Olson et al., 2016; Cobo et al., 2021). RA type I are Meissner corpuscles, RA type II correspond to Pacinian corpuscles, SA type I are the Merkel cell–neurite complexes, and SA type II are the dermal Ruffini’s corpuscles. They are widely distributed throughout the body but are especially abundant in the skin where there is high sensibility to touch and transduce different qualities of mechanosensitivity (Zimmerman et al., 2014; Cobo et al., 2021).
The cutaneous cells contacting the axon terminal of LTMRs, especially the Aβ ones (Abraira and Ginty, 2013), are specialized epithelial cells or glial Schwann-like cells: the axon–epithelial cell associations form the Merkel cell–neurite complexes or touch discs; the sets of axon and glial Schwann-like cells (terminal glial cells) form the core of CEOCs, i.e., Meissner corpuscles, Ruffini’s corpuscles, and Pacinian corpuscles (Munger and Ide, 1988; Zelená, 1994; Zimmerman et al., 2014; Cobo et al., 2021). Therefore, CEOC structurally consists of the axon terminal (also called dendritic zone) of one LTMR and non-myelinating terminal glial cells variably arranged depending on the morphotype of the corpuscle, and both are surrounded by a capsule of endoneurial/perineurial cells (Vega et al., 1996, 2009; García-Piqueras et al., 2017, 2020a; Cobo et al., 2021). So, the periaxonal cells within sensory corpuscles are continuous with the periaxonal cells on the nerve fibers [see (Cobo et al., 2021)].
The glial cells forming a part of CEOCs are a special subpopulation of peripheral glial cells named terminal glial cells or skin end-organ glia (Kastriti and Adameyko, 2017). These cells are habitually neglected in neurohistology books and reviews [see (Reed et al., 2021)] and were classically regarded as supportive and inert, and passive in the genesis of the action potential since they are non-excitable cells. However, emerging data support that terminal glial cells of CEOCs are fundamental in the process of mechanotransduction, but the putative role of the terminal glia on somatosensation remains largely unknown.
Most of the data collected for this review come from humans, although other vertebrates, especially mice, are also mentioned. To avoid confusion about which cells are reviewed here, it must be clarified that laminar cells are understood, exclusively, as the terminal glial cells, which form the lamellae arranged between or around the axon terminal. In some morphotypes of CEOCs there are other cells that also adopt a laminar morphology or arrangement but are not glial cells, for example, those that form the intermediate layer, the outer core, and the capsule in Pacinian corpuscles, or the capsule in Meissner corpuscles, but those cells are not the objective of this review. Here, we review and update the embryonic origin, development, morphology, ultrastructure, immunohistochemical profile, and putative functions of the terminal glial cells within the CEOCs, especially Meissner and Pacinian corpuscles.
Meissner corpuscles (Figure 1A) are exclusive in humans and primates, although similar structures, i.e., Meissner-like corpuscles, are also present in other mammals (Zelená, 1994). They are typical of the glabrous skin but concentrate in areas of fine touch discriminative capacities (fingertips, palm, sole of the feet, lips, and male and female genital skin) and occasionally on the tongue and palate (Vega et al., 1996, 2009).
Figure 1. (A) Schematic representation of human digital Meisser. a, axon; c, capsule; lc, lamellar cells, Sc, Schwann cells. Lamellar cells in Meissner corpuscles are closely related to the axon and form a “coin stack.” (B) Schematic representation of human Pacinian corpuscle. a, axon; c, capsule; ic, inner core; il, intermediate layer; lc, lamellar cells; oc, outer core; Sc, Schwann cells. (C) Arrangement of the terminal glial cells in the terminal and ultraterminal zones of the inner core.
Meissner corpuscles are found in the dermal papillae, just below the basal lamina of the epidermis. They have a typical oval shape with the major axis perpendicular to the surface of the skin, but its morphology and size are largely variable. Structurally, they consist of one single axon, terminal glial cells, and a capsule. In humans, the axon is usually unique, whereas in Macaca fascicularis and Macaca mulata, multiple axons can be detected, the main one being of Aβ type, and the accessories are the ones of C or Aδ type (Paré et al., 2001). Very recently, Neubarth and coworkers (Neubarth et al., 2020) observed that murine Meissner-like corpuscles contain two mechanoreceptor axon subtypes with distinct responses to tactile stimuli. The myelin sheath that envelops the axon of the nerve fiber supplying the corpuscle is lost upon entering the corpuscle (García-Suárez et al., 2009), and the axon is coated by Schwann-related cell-denominated laminar cells. They are arranged as stacks of flattened sheets (classically described as in a “coin stack”) parallel to the skin surface. Typically, the lamellar cells possess a basal lamina (Vega et al., 1995), and the interlamellar space is filled with a chemically complex extracellular matrix. Delimiting the corpuscle, adapted at the external side of the lamellar cells, there is a capsule of CD34 + positive endoneurial cells (García-Piqueras et al., 2020a).
Functionally, Meissner’s corpuscles are RA type I-LTMRs that detect fine touch, vibration, and fine movements on the skin (Johnson et al., 2000). Furthermore, Meissner’s corpuscles in monkeys could also work as nociceptors since accessory axons contain nociceptive neuropeptides (Paré et al., 2001).
Pacini’s corpuscles (Figure 1B) are large ovoid formations distributed in most organs and tissues, including the deep dermis and hypodermis (Bell et al., 1994; Zelená, 1994). Cells forming Pacinian corpuscles are typically arranged forming hemilamellae and lamellae around the axon to show a typical appearance of an “onion bulb.”
In the central part of the corpuscle is the axon of an Aβ mechanosensory neuron. The periaxonal cells of Pacinian corpuscles form three distinct compartments denominated as the inner core, outer core, and capsule. The inner core consists of hemilamellae of non-myelinating terminal glial cells, while the outer core and the capsule are composed of concentrically arranged flattened lamellae of perineurial fibroblast-like cells; the interlamellar spaces of the capsule typically contains capillaries and macrophages. Between the inner and the outer core, there is an intermediate cell stratum, called intermediate layer of growth layer, whose cellular elements are modified endoneurial CD34 + fibroblasts (García-Piqueras et al., 2017). Among the lamellae of the inner core, outer core, and capsule, there is a fluid-filled cellular space containing a molecularly complex extracellular matrix, including collagen (Vega et al., 1995; Dubový and Bednárová, 1999; Sames et al., 2001; García-Piqueras et al., 2019a,b, 2020b).
Functionally, Pacini’s corpuscles are RA type II-LTMRs and respond to pressure and vibratory stimuli between 20 and 1,500 Hz, with a maximum sensitivity at 200–400 Hz (Jones and Smith, 2014; Olson et al., 2016).
The spatial arrangement of the cells forming sensory corpuscles, i.e., axon, terminal glial cells, endoneurial-, and perineurial-like cells, varies according to the morphotype of the CEOCs. In particular, the terminal glial cells can be disposed within the corpuscle irregularly (Krause and Ruffini corpuscles), parallel (Meissner corpuscles), or concentric (Pacinian corpuscles).
The terminal glial cells of Meissner corpuscles are usually denominated lamellar cells because their typical horizontal flattened appearance that form stacks of lamellae (“coin stack”) between the lamellar cells are the axon branches (Munger and Ide, 1988; Vega et al., 2012). The lamellae are divided by relatively wide interspaces that contain the extracellular matrix components, and septal partitions may subdivide the corpuscle into two or more compartments. The lamellar cell bodies, containing the nuclei, are localized at the periphery of the corpuscle and stretch their flat cytoplasmic processes across. Their lamellae contain only a few mitochondria and other organelles but are rich in pinocytotic vesicles related to the mechanism of endocytosis/exocytosis (Munger and Ide, 1988; Zelená, 1994).
In murine digital Meisser-like corpuscles, using freeze–fracture techniques, it has been observed that the membranes of the axon terminals and lamellar cells contain intramembranous particles about 10 nm in diameter. The density of these particles in the axon membranes was somewhat lower than that of the lamellar cell membranes, and presumably, they have specific physiological properties in mechanoreceptive functions including mechano–electric transduction [see (Ide et al., 1985; Munger and Ide, 1988)].
The terminal glial cells of Pacinian corpuscles form the inner core, which lies between the axon and the intermediate layer. The inner core starts where the myelin ends, and it consists of tightly packed lamellae that originate from the lamellar cells whose bodies are disposed at the periphery of the core.
Within the inner core, the lamellae are diversely arranged in the preterminal, terminal, and ultraterminal zones of the corpuscle (Bell et al., 1994; Zelená, 1994). In the preterminal zone, they fully encircle the initial intracorpuscular segment of the terminal axon and are myelinating cells. In the terminal zone, the lamellae adopt a bilateral symmetric organization: the inner core is formed by two bilaterally symmetric hemilamellae systems in which the ends are opposite each other that corresponds to the longer axis of the axon. So, in the terminal zone, the inner core, the hemilamellae, forms dual gaps of radial clefts set 180° apart; processes of the axon terminal, called axonal spines, protrude into the radial clefts. Finally, the lamellae of the ultraterminal zone loses bilaterally, and small portions of their cytoplasm are disposed irregularly to surround the bulbous terminal of the axon (Figure 1C).
The lamellae of the inner core are very thin and contain mitochondria, concentric arrays of rough endoplasmic reticulum, and other membranous structures, as well as pinocytotic vesicles. The lamellar tips that abut the axon terminal are sometimes filled with dense cored vesicles and tubules. On the other hand, the lamellae are connected with many tight junctions. They are further joined with numerous gap junctions, which could allow a free flow of ions between individual inner core cells. Attachment plaques characterized by dense undercoating frequently connect successive lamellae and lamellar tips, but true desmosomes have been, so far, not encountered in freeze–fracture replicas. These attachment plaques probably ensure mechanical cell-to-cell attachment and, thus, contribute to the mechanical stability of the inner core together with tight junctions [see for a review (Munger and Ide, 1988; Bell et al., 1994; Zelená, 1994)].
The elegant studies carried out by review in Saxod (1978, 1996) demonstrated that the development of cutaneous sensory corpuscles results from complex morphogenetic interactions between the dermal mesenchyme, the somatosensory nerve, and neural crest-derived cells. These cells, considered originally as specialized Schwann cells (Idé, 1977), are the origin of the lamellar cells in the cutaneous sensory corpuscles in higher vertebrates, including humans.
The progenitors of the peripheral glial cells originate mainly from neural crest cells (NCCs) or from cap boundary cells (BCs). These cells contact and attach to the surface of developing axons and differentiate into Schwann cell precursors (S) and then into immature Schwann cells (ISCs) under the action of different molecules, especially neuregulins (NRGs). The ISCs give rise to a pleiad of peripheral glia including myelinating and non-myelinating Schwann cells, the satellite glial cells of the peripheral sensory and vegetative ganglia, the enteric glia, the terminal glial cells of the neuromuscular junctions and CEOCs (also denominated end-organ glia), and the sensory nerve fiber-associated glia (SNF-AG). Most of those cells originate directly from NCCs, and only the glial cells associated with cutaneous sensory nerves derivate from BCs (Maro et al., 2004; Aquino et al., 2006; Kastriti and Adameyko, 2017; Petersen and Adameyko, 2017; Furlan and Adameyko, 2018; Figure 2).
Figure 2. Schematic representation of development and differentiation of peripheral glial cells subtypes. BC, boundary cells; BDNF, brain-derived neurotrophic factor; DRG, dorsal root ganglia (dr, dorsa root; vr, ventral root); erbBs, neuregulin receptors; NCCs, neural crest cells; NRGs, neuregulins; NT, neural tube; SCs, Schwann cells; SGCs, satellite glial cells; TGCs, terminal glial cells; TrkB, high affinity receptor for BDNF. Imitated from Kastriti and Adameyko (2017).
Neural crest cells and boundary cells migrating toward the skin attach the growing sensory axons together with other nerve-associated cells, especially fibroblasts, and in the dermis, they give rise to highly specialized glial cells denominated SNF-AGs, which are associated to intraepidermal nerve endings (i.e., the adult intraepidermal free nerve endings). Nevertheless, SNF-AGs are different from the terminal glial cells of the sensory corpuscles since, whereas SNF-AGs are in contact with C-o Aδ fibers, the terminal glial cells are in relation to Aβ fibers (Gresset et al., 2015). Therefore, NCCs and BCs originate from at least three cutaneous glial populations: one type of Schwann cells (mainly non-myelinating) in relation with subcutaneous and dermal nerves and the CEOCs connected to them, and two types of terminal Schwann cells associated with lanceolate endings (in the hairy skin) and free nerve endings (in the glabrous and hairy skin). At present, the precise origin of the terminal glial cells within sensory corpuscles is still unknown, but probably, they derivate from NCCs (Etxaniz et al., 2014). The final phenotype of the cutaneous glial cells is probably a consequence of both specific molecular and local factors (Kastriti and Adameyko, 2017; Jessen and Mirsky, 2019). Interestingly, some SCPs remain undifferentiated postnatally in the dermis and peripheral nerves, and retain embryonic properties, that is, they do not completely lose multipotentiality (Widera et al., 2011; Calavia et al., 2012; Dyachuk et al., 2014).
Sensory axons are critical for the development of cutaneous CEOCs. Bidirectional interactions between sensory axons and SCPs, mediated by NRGs, initiate their morphogenesis (Saxod, 1996). In fact, the interaction of SCPs with growing axons (Jessen and Mirsky, 2005; Nave and Salzer, 2006) through NRG-1/ErbB2/ErbB3 receptors induce the comigration (Birchmeier and Nave, 2008), and a RET-ER81-NRG-1 signaling pathway promotes reciprocal interactions between axon and non-myelinating Schwann cells (Fleming et al., 2016). The terminal glial cells forming the inner core of murine Pacinian corpuscles display erbB2, erbB3, and erbB4 NRG receptors, whereas the central axon contains NRG-1, and deletions of Ret and Nrg1 in mechanosensory neurons results in the absence of Pacinian corpuscles, while Meissner corpuscles are unaffected (González-Martínez et al., 2007; Luo et al., 2009). Conversely, the functional complex of brain-derived neurotrophic factor and TrkB (BDNF/TrkB), control the development of Meissner corpuscles without effect on Pacinian corpuscles indicating a role in the development of specific mechanosensory neurons (LeMaster et al., 1999; Ichikawa et al., 2000; González-Martínez et al., 2004, 2005; Krimm et al., 2006; Pérez-Piñera et al., 2008). Nevertheless, other members of the NT family could also participate in the development of Pacinian corpuscles (Sedý et al., 2004). On the other hand, ER81, a member of the E26 transformation-specific family of transcription factors, is expressed in the inner core cells of Pacinian corpuscles, and the Er81-null mice lack of Pacini corpuscles is presumably because the sensory neurons subserving Pacinian corpuscles do not survive (Sedý et al., 2006).
In addition to the molecules that are involved int the axon–glial cells interactions, or those that control indirectly the CEOC development by regulating neuronal survival, local molecules also seems to be involved in their development. Among them are target-derived growth factors (Schecterson and Bothwell, 1992; Dontchev and Letourneau, 2003), β-arrestin-1 (Komori et al., 2003), semaphorins (Dontchev and Letourneau, 2003; Curley et al., 2014; McCormick et al., 2015), ankyrin-B (Engelhardt et al., 2013), and even mechanical signals (Koser et al., 2016).
To study the temporal pattern of CEOC development, specific markers for the different constituents (axon, terminal cells, endoneurial, and perineurial cells) are currently used in immunohistochemical studies. Interestingly, these studies are facilitated by the fact that NCCs, SCPs, ISCs, and mature Schwann cells have common markers, including nestin, vimentin, and S100β protein (Jessen and Mirsky, 2005; Engelhardt et al., 2013). Using antibodies against these proteins, we established the timetable of the development of human digital CEOCs (Feito et al., 2018).
Meissner’s corpuscles, which start differentiation at around 20 weeks of estimated gestational age (WEGA), show basic morphology by 36 WEGA, and acquire the definitive aspect and immunohistochemical profile postnatally. Nevertheless, the density of Meissner’s corpuscles is not stable throughout life, and its number and size are reduced with aging (McCormick et al., 2015). By 23 WEGA, axons with different morphologies are identified in the dermal papillae and occasionally penetrating the epidermis. However, for 10 WEGA, the axons remain “naked” since the S100 protein + precursors of the glial terminal cells, of rounded morphology, do not reach the dermal papillae until 33 WEGA. By 36–40 weeks S100 protein + cells also become vimentin + and progressively flattened. However, the typical morphology and arrangement of the lamellar cells of digital Meissner corpuscles are not observed until around 8 months of postnatal life (Feito et al., 2018).
Pacinian corpuscles start development and structural differentiation in human digital skin by 13WEGA. At this time, and until 16 WEGA, the S100 protein + cells are organized in one or two layers of rounded cells, which progressively become smaller and flattened without evidence of lamellar organization (16 to 18 WEGA). These cells are the primigenial inner core. In parallel, the cells of the dermal surrounding mesenchyme organize to form the outer core and the capsule. Thereafter, between 20 and 24 WEGA, the S100 protein + cells emitted expansions that form a network and penetrate the outermost zone of the corpuscle, which later retract toward the central part of the corpuscle. Simultaneously, and extending between 24 and 36 WEGA, the edge of the inner core acquires a laminar arrangement, the lamellae becoming strongly packed and the inner core clefts being clearly distinguished. Nevertheless, the full maturation of Pacinian corpuscles in both morphology structure and expression of specific cell antigens is not totally defined until the fourth month of life. During the development of Pacinian corpuscles, expression of vimentin started shortly later than that of S100P and did not vary along the lifespan (Feito et al., 2018). In addition, Pacinian corpuscles generally showed no relevant age-related alterations (García-Piqueras et al., 2019).
On the other hand, Albuerne et al. (2000) analyzed the development of Meissner-like and Pacinian corpuscles in murine glabrous skin from the forepaw and hindpaw, as well as from the group of Pacinian corpuscles present in the interosseus membrane of the hindlimb. Although both kinds of sensory corpuscles start to develop prenatally, they become mature around postnatal day (Pd) 19–Pd28 for the Meissner-like corpuscles, and by Pd20 for the Pacinian ones. The lamellar cells in Meissner-like corpuscles expressed first the S100 protein (Pd7), then vimentin IR (Pd12), and transitory p75LNGFR (Pd7 to Pd19–20). In the Pacinian corpuscles, the lamellar cells forming the inner core displayed S100 protein by Pd7 and vimentin by Pd19 but lack p75LNGFR.
Cutaneous end-organ complexes have a very complex and heterogeneous protein content, as demonstrated in numerous immunohistochemical studies performed for more than 40 years. The references for papers reporting each of those proteins in the lamellar cells are included in the reviews by Vega’s lab (Vega et al., 1996, 2009; Cobo et al., 2021), Johansson’s lab (Johansson et al., 1999), and Pawson’s lab (Pawson et al., 2000).
Different cytoskeletal or cytosolic proteins have been detected in lamellar cells of sensory corpuscles. Some of them could be regarded as specific to those cells, but most of them are present in other cells where presumably they fulfill identical or similar functions. The intermediate filament protein filling the cytoplasm of the lamellar cells is vimentin instead of glial fibrillary acidic protein. However, differences seem to occur between species or anatomical localization since Pacinian corpuscles from human and cat peritoneum contain glial fibrillary acidic protein in addition to vimentin. Nevertheless, the coexpression of both cytoskeletal proteins was never observed; it is absent in human cutaneous sensory corpuscles. Interestingly, a subpopulation of lamellar cells in human digital Meissner and Pacinian corpuscles also display immunoreactivity for nestin, even in the adult life.
Several calcium-binding proteins, which presumably participate in the Ca2+ homeostasis and mechanoreceptor electrogenesis, are also detectable in the terminal glial cells of sensory corpuscles. They include S-100β, calbindin D28, calretinin, parvalbumin, and neurocalcin.
Also, some growth factors belonging to the family of the neurotrophins (BDNF) and their cognate receptors (p75NTR, TrkA, and TrkB), and epidermal growth factor receptor as well, are expressed by the terminal glial cells of cutaneous sensory corpuscles.
On the other hand, filling in spaces between lamellar cells, there is a chemically complex extracellular matrix formed by both fibrillary proteins and glycosaminoglycans, some of them organized as the basal lamina. In Meissner corpuscles, heparan sulfate proteoglycans were colocalized with type IV collagen, thus, suggesting that they are a part of the basal membrane, whereas chondroitin sulfate was absent. Regarding Pacinian corpuscles, the inner core contains decorin, biglycan, lumican, fibromodulin, and osteoadherin; the intermediate layer displays immunoreactivity for chondroitin sulfate and osteoadherin; was detected in the outer core lamellae and capsule, in the intermediate layer, and the inner core of Pacinian corpuscles expressing decorin, biglycan, lumican, fibromodulin, and osteoadherin (Vega et al., 1995; Dubový and Bednárová, 1999; García-Piqueras et al., 2019a,b, 2020b).
The axons and terminal glial cells of CEOCs are associated at multiple subcellular points, which can be observed using electron microscopy, in the so-called neuron receptive endings (NREs). NREs are specialized subcellular structures on sensory cells or neurons that receive inputs from either the environment or other neurons (Singhvi and Shaham, 2019). The sensory organs, including sensory corpuscles, have NREs exquisitely tuned to the sensory modality it transduces. Probably, NREs in sensory axons of CEOCs, and maybe also in the lamellar cells, could be related to the membrane regions where ion channels concentrate (see later). Classically, it is accepted that a key function of many glial cell subtypes is to modulate the NRE ionic microenvironment; for the terminal glia in CEOCs, it can be suggested that there is a modulation of the extracellular levels of K+, Na+, and Cl–, and probably also Ca2+ ions [for a review, see (Singhvi and Shaham, 2019; Reed et al., 2021)].
In this section, we review the putative role of lamellar cells in mechanosensing, updating the occurrence of mechano-gated ion channels and their possible involvement in mechanotransduction, as well as the data supporting neurotransmission in sensory corpuscles.
Touch is not only required for detection of objects and discrimination of shape, size, and texture but also for vibration. For touch perception, the first step is the conversion of mechanical stimuli into electrical activity, i.e., the receptor potential, and it occurs in cutaneous CEOCs. At present, the characteristics of the action potential generated in each CEOC subtype are rather well known, but the molecular events of this for mechanoelectrical transduction have not been fully identified.
The genesis of the receptor potential in the CEOCs of LTMRs was accepted that it depended on the mechanical properties of the periaxonal cells, especially the fibroblast of the outer core and the capsule, together with the characteristics of the axon membrane. In this mechanical theory, all the periaxonal cells are included in the term “capsule,” although the inner core, the intermediate layer, and the outer core capsule are completely different in their origin and cell composition. Thus, according to this theory, the mechanotransduction was regarded as a physical mechanical process. However, the discovery that mechanical forces can gate some ion channels present in the membranes of cutaneous, the somatosensory neurons including CEOCs, opened a new conception about the mechanisms of mechanotransduction (Sharif-Naeini, 2015).
A recent research has identified proteins essential for mechanotransduction and some others that can be required for some events of the mechanotransduction. Most of these proteins are related to ion channels (Martinac and Poole, 2018; Douguet and Honoré, 2019; Jin et al., 2020; Kefauver et al., 2020). Both voltage-gated and voltage-independent ion channels have been proposed to initiate mechanotransduction, and all have been localized in Meissner and/or Pacinian corpuscles primarily in the membrane of the axon but also in the terminal glial cells. Importantly, it must be underlined that the effect of a mechanical force on a cell depends on the site of incidence with the membrane. Cell membranes contain “protein corrals” (lipid-protein spatial domains), and the incidence of mechanical forces inside any domain is different from that in the surrounding membrane. So, the mechanosensitive ion channels can be gated or not by inclusion or exclusion from a domain (Sukharev and Sachs, 2012). Furthermore, it remains to be studied whether mechanosensitive ion channels are grouped on or in the vicinity of NREs (Singhvi and Shaham, 2019).
One of voltage-dependent K+ channels detected in a subpopulation of LTMRs is KCNQ4 (Kv7.4), which is crucial for setting the velocity and frequency preference in both mice and humans (Heidenreich et al., 2011). Moreover, the α-subunit of type I and type II voltage-gated Na+ channels are present in the axon and the non-neural inner and outer lamellae of rabbit Pacinian corpuscles. Those localizations suggest that they are involved in both transduction and action potential generation making it available to the axon via transport from the lamellae (Pawson and Bolanowski, 2002). Nevertheless, in this study, it is not clear whether the occurrence of voltage-gated Na+ channels occur in the inner core, the outer core–capsule, or both.
The voltage-independent mechano-gated ion channels fall into two main categories that respond to membrane tension or membrane stretch (Delmas and Coste, 2013). The opening of these channels allows the passage of ions into the axon to trigger the mechanotransduction (Gu and Gu, 2014; Ranade et al., 2015; Cobo et al., 2020). Thus, deformations in the membrane of the cells that form the mechanoreceptors open mechanosensitive ion channels that transduce mechanical energy into electrical activity. Therefore, if mechanotransduction starts in CEOCs, ion channels activated by force or displacement that act as mechanosensors and/or mechanotransducers should be expressed in the cells forming CEOCs. It should be clear that mechanical forces never directly reach the axon, which are ultimately responsible for triggering the potential for action. On the contrary, forces act on layers of cells, sometimes very numerous and of different natures, which in conjunction with the extracellular matrix and the cytoskeleton deform the axon membrane, opening the ion channels. In addition, the lamellae of terminal glial cells in CEOCs also express putative mechano-gated channels (Figure 3).
Figure 3. (A) Schematic representation of a Pacinian corpuscle showing the corpuscular structures that are modified by a force to generate the action potential. a, axon; c, capsule; ic, inner core; il, intermediate layer; lc, lamellar cells; oc, outer core; Sc, Schwann cells. (B) Schematic transversal section of a Pacinian corpuscle showing all cell structures potentially involved in mechanotransduction.
Channel complexes of the family of the degenerin/epithelial Na+ channels (DEG/ENa+C) might be involved in mechanotransduction. Ion channel subunits and family branches of DEG/ENa+C [like acid-sensing ion channels (ASIC)] are gated by mechanical forces and, for this reason, have been proposed as mechanotransducers [see for a review, (Ruan et al., 2021)]. DEG/ENa+C subunits and ASIC2 have been detected in the lamellar cells of murine and human Pacinian corpuscles (Montaño et al., 2009; Calavia et al., 2010) as well as in a subpopulation of human Meissner corpuscles (Cabo et al., 2015). ASIC2-knockout mice exhibit a decreased sensitivity of rapidly adapting cutaneous LTMRs, and disruption of ASIC3 reduces responses of cutaneous high-threshold mechanoreceptors to noxious stimuli (Drew et al., 2004). Nevertheless, the role of ASIC in cutaneous mechanosensitivity is doubted (Page et al., 2004).
Nearly all TRP (transient receptor potential) families of ion channels have members with potential mechanosensory capacities (Clapham et al., 2005; Samanta et al., 2018). Nevertheless, it has not been established whether these ion channels are mechanosensors or participate only when required for mechanosensation since some of them are insensitive to membrane stretch [see (Nikolaev et al., 2020)]. As far as we know, TRPV4 (vanilloid 4) is the only member of the TRP superfamily that has been detected in the lamellar cells of human digital Meissner (Alonso-González et al., 2016). Nevertheless, a role for this protein within the lamellar cells of sensory corpuscles remains to be demonstrated, although disruption of trpv4 in mice leads to insensitivity to pressure sensation in the mouse tail (Suzuki et al., 2003).
The occurrence of the members of the other two families of ion channels involved in mechanotransduction, i.e., the family of two pore domain channels and Piezo have been never detected in the terminal glial cells of CEOCs. More recently, the proteins codified by the Piezo gene, PIEZO1 and PIEZO2, have proven to have true mechanosensory ability and are directly involved in mechanotransduction (Coste et al., 2010; Ranade et al., 2014). PIEZO2 function as a transducer in LTMRs and Merkel cells, such as the lamellar cells, can be regarded as sensing cells in Merkel cell–neurite complexes and are associated with the axon terminal of Aβ mechanosensory fibers. In the murine skin, PIEZO2 is expressed and has functional expression present in Merkel discs and isolated Merkel cells (Ikeda et al., 2014; Maksimovic et al., 2014; Woo et al., 2014, 2015a,b), Meissner-like corpuscles, and lanceolate nerve endings (Ikeda et al., 2014). PIEZO2 has been also detected in human Merkel cells and Meissner’s corpuscle axon, in an age-dependent manner (Sedý et al., 2004; García-Mesa et al., 2017). Consistently with those localizations, PIEZO2-deficient mice show an almost complete deficit in light-touch sensation and proprioception with preserved function in other somatosensory modalities (Ranade et al., 2014). PIEZO2 mutations in human patients lead to selective loss of touch perception and heavily decreased proprioception (Chesler et al., 2016; Mahmud et al., 2017).
The most overwhelming putative role of lamellar cells in mechanosensation was provided recently by Nikolaev et al. (2020). They observed that the lamellar cells of Grandry’s corpuscles (assimilated to the lamellar cells within Meissner corpuscles) from duck bill skin detect tactile stimuli, thus, suggesting that also lamellar cells are touch sensors. The authors observed that Grandry’s cells act as non-neuronal mechanosensors that contain mechanically gated ion channels and can generate robust Ca2+-dependent action potentials via R-type Cav channels. Furthermore, they also showed that outer-core lamellar cells from Herbtst corpuscles (equivalent to the mammalian Pacinian corpuscles) are mechanosensitive, but unlike lamellar cells in Grandry’s corpuscles, they are not excitable. Consistently, these propose that Meissner and Pacinian corpuscles use neuronal and non-neuronal mechanoreception to detect mechanical signals. It may be worth to note that even the structures are not identical; there is striking similarity between microanatomy, function, and electrophysiology of the avian Grandry’s and Herbtst corpuscles vs. Meissner and Pacinian corpuscles, respectively.
The extracellular matrix and cytoskeletal proteins anchored to the extra- or intracytoplasmic domains, respectively, of the cell membrane in the vicinity of mechano-gated ion channels could play important roles in mechanotransduction. As mentioned in previous paragraphs, the force exerted on the skin does not act directly on the axon that generates the action potential. Among the skin surface and the axon membrane are the different layers of the epidermis, the epidermal–dermic connections, and the transmission from the dermis through a greater or lesser number of cell layers (capsule and laminar cells in the Meissner corpuscles; capsule, outer core, intermediate lamina, and inner core in the Pacini corpuscles) until the axon. Therefore, we consider that the role of the extracellular matrix, the cytoskeletal proteins, and the cell-to-cell junctions in CEOCs are of capital importance in the process of mechanosensing. Integrins (Dubovy et al., 1999) and other linking extracellular matrix proteins present in sensory corpuscles could participate in mechanosensing and/or mechanotransduction. For example, integrin α1β1 is necessary for the function of TRPV4 ion channel in chondrocytes (Jablonski et al., 2014) and influence the density of Meissner-like corpuscles in murine footpads (Wai et al., 2021). Recently, Schwaller et al. (2021) found in the lamellar cells of Meissner-like corpuscles USH2A, a transmembrane protein with a very large extracellular domain, and in the absence of this protein, the RA type I LTMRs showed large reductions in vibration sensitivity.
In Pawson et al. (2007, 2009) published a series of elegant studies that suggested that in cats, Pacinian corpuscle classical neurotransmission cannot be excluded for the genesis of the action potential, involving both the axons and the periaxonal cells. This is in addition to the opening of voltage-gated and non-voltage-gated channels as responsible, or at least necessary, for mechanotransduction. The authors specifically speak about the lamellar cells of the terminal glial but only of periaxonal cells (“modified Schwann cells of the inner core”).
The studies of Pawson and coworkers postulated that “action potentials in response to dynamic stimuli are due to depolarization of the axon by cations entering mechano-gated channels that are opened due to mechanical motion; however, action potentials in the static portion of the Pacinian corpuscle rapidly adapting response are due to glutamatergic excitation, which are then inhibited by GABA released from the modified Schwann cells of the inner core.” This synaptic-like activity is based on the following observations: (a) lamellar cells contains the machinery to synthesize, store (express immunoreactivity for the synaptic proteins synaptobrevin VAMP2 and SNAP-23), and release neurotransmitters (glutamate, GABA); (b) lamellar cells release neurotransmitters when they are stimulated by glutamate, ATP, or even by mechanical motion; and (c) the lamellar cells express glutamate receptors. Thus, the sequence for mechanotransduction based on the synaptic-like theory is as follows: After initial opening of mechano-gated channels by mechanical stimulus, the entry of Ca2+ in the axon and the subsequent depolarization that originates the receptor potential induce the release of glutamate from clear-core vesicles of the axon. Glutamate can act either on the lamellar cells, the axon, or both the lamellar cells and the axon, which express glutamate receptors. Glutamate acting on lamellar cells induces GABA release, which, acting on GABA-receptors of the axon, inhibits glutamate excitation. In addition, the lamellar cells contain vesicular-glutamate transporters, and SNARE proteins, that consent glutamate release by the lamellar cells. Importantly, the mechanical stimulus alone may be responsible for the release of either the glutamate or the GABA from the lamellar cells (Figure 4).
Figure 4. Schematic representation of the synaptic-like coupling of the axon and lamellar cells in Pacinian corpuscles. Opening of mechano-gated channels (1) causes the entry of Ca2+ in the axon (red arrow) and release of glutamate from clear-core vesicles of the axon (green arrow), which can act on the lamellar cells or the axon (2). Glutamate acting on lamellar cells induces GABA release (brown arrow), which activates GABA receptors in the axon (3) inhibiting glutamate excitation. In addition, the lamellar cells contain vesicular-glutamate transporters, and SNARE proteins, that consent glutamate release by the lamellar cells (4). Modified from Pawson et al. (2009).
These findings argue for a chemical, possibly bidirectional, interaction between the lamellar cells and the axon. This “mechanochemical” theory for the genesis of mechanotransduction has been proposed only in Pacinian corpuscles and remains to be demonstrated that similar mechanisms of neurotransmission occur in other kinds of sensory corpuscles. Nevertheless, it is well documented that mechanosensory axon terminals, but not the associated terminal glial cells, have “an apparently ubiquitous glutamate secretory system” of unknown functional significance [see for review (Bewick, 2015)]. The only cells associated with Aβ afferents are Merkel cells that establish synapsis-like contact at Merkel cell–neurite complexes. Consistently, Merkel cells, regarded as the presynaptic cells, contain all the components of the presynaptic machinery (active-zone molecules and synaptic vesicle proteins) and release neurotransmitters (Haeberle et al., 2004; Maksimovic et al., 2013; Nakatani et al., 2015).
The terminal glial cells of CEOCs are a subpopulation of peripheral glial cells, highly differentiated, with putative roles in mechanosensing and/or mechanotransduction. In the last decade, some mechano-gated ion channels have been discovered in terminal glial cells. This fact, associated with the presence of some components of a GABA-ergic/glutamatergic neurotransmission system in the Pacinian corpuscles, suggests that the glial cells of sensory corpuscles are not passive supporting cells but could have an active role in the mechanotransduction process and the genesis of the action potential.
The raw data supporting the conclusions of this article will be made available by the authors, without undue reservation.
All authors listed have made a substantial, direct, and intellectual contribution to the work, and approved it for publication.
YG-M was supported by a grant “Severo Ochoa” from the Government of the Principality of Asturias (Ref. BP17-044).
The authors declare that the research was conducted in the absence of any commercial or financial relationships that could be construed as a potential conflict of interest.
All claims expressed in this article are solely those of the authors and do not necessarily represent those of their affiliated organizations, or those of the publisher, the editors and the reviewers. Any product that may be evaluated in this article, or claim that may be made by its manufacturer, is not guaranteed or endorsed by the publisher.
Abraira, V. E., and Ginty, D. D. (2013). The sensory neurons of touch. Neuron 79, 618–639. doi: 10.1016/j.neuron.2013.07.051
Albuerne, M., De Lavallina, J., Esteban, I., Naves, F. J., Silos-Santiago, I., and Vega, J. A. (2000). Development of Meissner-like and Pacinian sensory corpuscles in the mouse demonstrated with specific markers for corpuscular constituents. Anat. Rec. 258, 235–242.
Alonso-González, P., Cabo, R., San José, I., Gago, A., Suazo, I. C., García-Suárez, O., et al. (2016). Human digital Meissner corpuscles display immunoreactivity for the multifunctional ion channels Trpc6 and Trpv4. Anat. Rec. 300, 1022–1031. doi: 10.1002/ar.23522
Aquino, J. B., Hjerling-Leffler, J., Koltzenburg, M., Edlund, T., Villar, M. J., and Ernfors, P. (2006). In vitro and in vivo differentiation of boundary cap neural crest stem cells into mature Schwann cells. Exp. Neurol. 198, 438–449. doi: 10.1016/j.expneurol.2005.12.015
Bell, J., Bolanowski, S., and Holmes, M. H. (1994). The structure and function of Pacinian corpuscles: a review. Prog. Neurobiol. 42, 79–128. doi: 10.1016/0301-0082(94)90022-1
Bewick, G. S. (2015). Synaptic-like vesicles and candidate transduction channels in mechanosensory terminals. J. Anat. 227, 194–213. doi: 10.1111/joa.12337
Birchmeier, C., and Nave, K. A. (2008). Neuregulin-1, a key axonal signal that drives Schwann cell growth and differentiation. Glia 56, 1491–1497. doi: 10.1093/brain/aww039
Cabo, R., Alonso, P., Viña, E., Vázquez, G., Gago, A., Feito, J., et al. (2015). ASIC2 is present in human mechanosensory neurons of the dorsal root ganglia and in mechanoreceptors of the glabrous skin. Histochem. Cell. Biol. 143, 267–276. doi: 10.1007/s00418-014-1278-y
Calavia, M., Viña, E., Menéndez-González, M., López-Muñiz, A., Alonso-Guervós, M., Cobo, J., et al. (2012). Evidence of nestin-positive cells in the human cutaneous Meissner and Pacinian corpuscles. CNS. Neurol. Disord. Drug. Targets 11, 869–877. doi: 10.2174/1871527311201070869
Calavia, M. G., Montaño, J. A., García-Suárez, O., Feito, J., Guervós, M. A., Germanà, A., et al. (2010). Differential localization of acid-sensing ion channels 1 and 2 in human cutaneous pacinian corpuscles. Cell. Mol. Neurobiol. 30, 841–848. doi: 10.1007/s10571-010-9511-2
Chesler, A. T., Szczot, M., Bharucha-Goebel, D., Čeko, M., Donkervoort, S., Laubacher, C., et al. (2016). The Role of PIEZO2 in Human Mechanosensation. N. Engl. J. Med. 375, 1355–1364. doi: 10.1056/NEJMoa1602812
Clapham, D. E., Julius, D., Montell, C., and Schultz, G. (2005). International Union of Pharmacology. XLIX. Nomenclature and structure-function relationships of transient receptor potential channels. Pharmacol. Rev. 57, 427–450. doi: 10.1124/pr.57.4.6
Cobo, R., García-Piqueras, J., Cobo, J., and Vega, J. A. (2021). The human cutaneous sensory corpuscles: an update. J. Clin. Med. 10:227. doi: 10.3390/jcm10020227
Cobo, R., García-Piqueras, J., García-Mesa, Y., Feito, J., García-Suárez, O., and Vega, J. A. (2020). Peripheral mechanobiology of touch-studies on vertebrate cutaneous sensory corpuscles. Int. J. Mol. Sci. 21:6221. doi: 10.3390/ijms21176221
Coste, B., Mathur, J., Schmidt, M., Earley, T. J., Ranade, S., Petrus, M. J., et al. (2010). Piezo1 and Piezo2 are essential components of distinct mechanically activated cation channels. Science 330, 55–60. doi: 10.1126/science.1193270
Curley, J. L., Catig, G. C., Horn-Ranney, E. L., and Moore, M. J. (2014). Sensory axon guidance with semaphorin 6A and nerve growth factor in a biomimetic choice point model. Biofabrication 6:035026. doi: 10.1088/1758-5082/6/3/035026
Delmas, P., and Coste, B. (2013). Mechano-gated ion channels in sensory systems. Cell 155, 278–284. doi: 10.1016/j.cell.2013.09.026
Dontchev, V. D., and Letourneau, P. C. (2003). Growth cones integrate signaling from multiple guidance. J. Histochem. Cytochem. 51, 435–444. doi: 10.1177/002215540305100405
Douguet, D., and Honoré, E. (2019). Mammalian mechanoelectrical transduction: structure and function of force-gated ion channels. Cell 179, 340–354. doi: 10.1016/j.cell.2019.08.049
Drew, L. J., Rohrer, D. K., Price, M. P., Blaver, K. E., Cockayne, D. A., Cesare, P., et al. (2004). Acid-sensing ion channels ASIC2 and ASIC3 do not contribute to mechanically activated currents in mammalian sensory neurons. J. Physiol. 556, 691–710. doi: 10.1113/jphysiol.2003.058693
Dubový, P., and Bednárová, J. (1999). The extracellular matrix of rat pacinian corpuscles: an analysis of its fine structure. Anat. Embryol. 200, 615–623. doi: 10.1007/s004290050309
Dubovy, P., Svłzenska, I., Jancalek, R., and Klusakova, I. (1999). Immunohistochemical localization of some extracellular molecules and their integrin receptors in the rat Pacinian corpuscles. Gen. Physiol. Biophys. 18, 66–68.
Dyachuk, V., Furlan, A., Shahidi, M. K., Giovenco, M., Kaukua, N., Konstantinidou, C., et al. (2014). Neurodevelopment. Parasympathetic neurons originate from nerve-associated peripheral glial progenitors. Science 345, 82–87. doi: 10.1126/science.1253281
Engelhardt, M., Vorwald, S., Sobotzik, J. M., Bennett, V., and Schultz, C. (2013). Ankyrin-B structurally defines terminal microdomains of peripheral somatosensory axons. Brain Struct. Funct. 218, 1005–1016. doi: 10.1007/s00429-012-0443-0
Etxaniz, U., Pérez-San Vicente, A., Gago-López, N., García-Dominguez, M., Iribar, H., Aduriz, A., et al. (2014). Neural-competent cells of adult human dermis belong to the Schwann lineage. Stem. Cell. Rep. 3, 774–788. doi: 10.1016/j.stemcr.2014.09.009
Feito, J., García-Suárez, O., García-Piqueras, J., García-Mesa, Y., Pérez-Sánchez, A., Suazo, I., et al. (2018). The development of human digital Meissner’s and Pacinian corpuscles. Ann. Anat. 219, 8–24. doi: 10.1016/j.aanat.2018.05.001
Fleming, M. S., Li, J. J., Ramos, D., Li, T., Talmage, D. A., Abe, S. I., et al. (2016). A RET-ER81-NRG1 signaling pathway drives the development of pacinian corpuscles. J. Neurosci. 36, 10337–10355. doi: 10.1523/jneurosci.2160-16.2016
Furlan, A., and Adameyko, I. (2018). Schwann cell precursor: a neural crest cell in disguise? Dev. Biol. 444, S25–S35. doi: 10.1016/j.ydbio.2018.02.008
García-Mesa, Y., García-Piqueras, J., García, B., Feito, J., Cabo, R., Cobo, J., et al. (2017). Merkel cells and Meissner’s corpuscles in human digital skin display Piezo2 immunoreactivity. J. Anat. 213, 978–989. doi: 10.1111/joa.12688
García-Piqueras, J., García-Mesa, Y., Cárcaba, L., Feito, J., Torres-Parejo, I., Martín-Biedma, B., et al. (2019). Ageing of the somatosensory system at the periphery: age-related changes in cutaneous mechanoreceptors. J. Anat. 234, 839–852. doi: 10.1111/joa.12983
García-Piqueras, J., Carcaba, L., García-Mesa, Y., Feito, J., García, B., Viña, E., et al. (2019a). Chondroitin Sulfate in Human Cutaneous Meissner and Pacinian Sensory Corpuscles. Anat. Rec. 302, 325–331. doi: 10.1002/ar.23951
García-Piqueras, J., García-Mesa, Y., Feito, J., García, B., Quiros, L. M., Martín-Biedma, B., et al. (2019b). Class I and Class II small leucine-rich proteoglycans in human cutaneous pacinian corpuscles. Ann. Anat. 224, 62–72. doi: 10.1016/j.aanat.2019.02.007
García-Piqueras, J., Cobo, R., Cárcaba, L., García-Mesa, Y., Feito, J., Cobo, J., et al. (2020a). The capsule of human Meissner corpuscles: immunohistochemical evidence. J. Anat. 236, 854–861. doi: 10.1111/joa.13139
García-Piqueras, J., García-Suárez, O., García-Mesa, Y., García-Fernandez, B., Quirós, L. M., Cobo, R., et al. (2020b). Heparan sulfate in human cutaneous Meissner’s and Pacinian corpuscles. Anat. Rec. 2020:24328. doi: 10.1002/ar.24328
García-Piqueras, J., García-Suárez, O., Rodríguez-González, M. C., Cobo, J. L., Cabo, R., Vega, J. A., et al. (2017). Endoneurial-CD34 positive cells define an intermediate layer in human digital Pacinian corpuscles. Ann. Anat. 211, 55–60. doi: 10.1016/j.aanat.2017.01.006
García-Suárez, O., Montaño, J. A., Esteban, I., González-Martínez, T., Alvarez-Abad, C., López-Arranz, E., et al. (2009). Myelin basic protein-positive nerve fibres in human Meissner corpuscles. J. Anat. 214, 888–893. doi: 10.1111/j.1469-7580.2009.01078.x
González-Martínez, T., Fariñas, I., Del Valle, M. E., Feito, J., Germanà, G., Cobo, J., et al. (2005). BDNF, but not NT-4, is necessary for normal development of Meissner corpuscles. Neurosci. Lett. 377, 12–15. doi: 10.1016/j.neulet.2004.11.078
González-Martínez, T., Germana, A., Catania, S., Cobo, T., Ochoa-Erena, F. J., de Carlos, F., et al. (2007). Postnatal developmental changes in the expression of ErbB receptors in murine Pacinian corpuscles. Neurosci. Lett. 420, 90–95. doi: 10.1016/j.neulet.2007.04.061
González-Martínez, T., Germanà, G. P., Monjil, D. F., Silos-Santiago, I., de Carlos, F., Germanà, G., et al. (2004). Absence of Meissner corpuscles in the digital pads of mice lacking functional TrkB. Brain. Res. 1002, 120–128. doi: 10.1016/j.brainres.2004.01.003
Gresset, A., Coulpier, F., Gerschenfeld, G., Jourdon, A., Matesic, G., Richard, L., et al. (2015). Boundary Caps Give Rise to Neurogenic Stem Cells and Terminal Glia in the Skin. Stem. Cell. Rep. 5, 278–290. doi: 10.1016/j.stemcr.2015.06.005
Gu, Y., and Gu, C. (2014). Physiological and pathological functions of mechanosensitive ion channels. Mol. Neurobiol. 50, 339–347. doi: 10.1007/s12035-014-8654-4
Haeberle, H., Fujiwara, M., Chuang, J., Medina, M. M., Panditrao, M. V., Bechstedt, S., et al. (2004). Molecular profiling reveals synaptic release machinery in Merkel cells. Proc. Natl. Acad. Sci. USA 101, 14503–14508. doi: 10.1073/pnas.0406308101
Handler, A., and Ginty, D. D. (2021). The mechanosensory neurons of touch and their mechanisms of activation. Nat. Rev. Neurosci. 22, 521–537. doi: 10.1038/s41583-021-00489-x
Heidenreich, M., Lechner, S. G., Vardanyan, V., Wetzel, C., Cremers, C. W., De Leenheer, E. M., et al. (2011). KCNQ4 K+ channels tune mechanoreceptors for normal touch sensation in mouse and man. Nat. Neurosci. 15, 138–145. doi: 10.1038/nn.2985
Ichikawa, H., Matsuo, S., Silos-Santiago, I., and Sugimoto, T. (2000). Developmental dependency of Meissner corpuscles on trkB but not trkA or trkC. Neuroreport 11, 259–262. doi: 10.1097/00001756-200002070-00007
Idé, C. (1977). Development of Meissner corpuscle of mouse toe pad. Anat. Rec. 188, 49–67. doi: 10.1002/ar.1091880107
Ide, C., Kumagai, K., and Hayashi, S. (1985). Freeze-fracture study of the mechanoreceptive digital corpuscles of mice. J. Neurocytol. 14, 1037–1052. doi: 10.1007/BF01224811
Ikeda, R., Cha, M., Ling, J., Jia, Z., Coyle, D., and Gu, J. (2014). Merkel cells transduce and encode tactile stimuli to drive Aβ-afferent impulses. Cell 157, 664–675. doi: 10.1016/j.cell.2014.02.026
Jablonski, C. L., Ferguson, S., Pozzi, A., and Clark, A. L. (2014). Integrin α1β1 participates in chondrocyte transduction of osmotic stress. Biochem. Biophys. Res. Commun. 445, 184–190. doi: 10.1016/j.bbrc.2014.01.157
Jessen, K. R., and Mirsky, R. (2005). The origin and development of glial cells in peripheral nerves. Nat. Rev. Neurosci. 6, 671–682. doi: 10.1038/nrn1746
Jessen, K. R., and Mirsky, R. (2019). Schwann cell precursors; multipotent glial cells in embryonic nerves. Front. Mol. Neurosci. 12:69. doi: 10.3389/fnmol.2019.00069
Jin, P., Jan, L. Y., and Jan, Y. N. (2020). Mechanosensitive ion channels: structural features relevant to mechanotransduction mechanisms. Annu. Rev. Neurosci. 43, 207–229. doi: 10.1146/annurev-neuro-070918-050509
Johansson, O., Fantini, F., and Hu, H. (1999). Neuronal structural proteins, transmitters, transmitter enzymes and neuropeptides in human Meissner’s corpuscles: a reappraisal using immunohistochemistry. Arch. Dermatol. Res. 291, 419–424. doi: 10.1007/s004030050432
Johnson, K. O., Yoshioka, T., and Vega-Bermudez, F. (2000). Tactile functions of mechanoreceptive afferents innervating the hand. J. Clin. Neurophysiol. 17, 539–558. doi: 10.1097/00004691-200011000-00002
Jones, L. A., and Smith, A. M. (2014). Tactile sensory system: encoding from the periphery to the cortex. Wiley Interdiscip. Rev. Syst. Biol. Med. 6, 279–287. doi: 10.1002/wsbm.1267
Kastriti, M. E., and Adameyko, I. (2017). Specification, plasticity and evolutionary origin of peripheral glial cells. Curr. Opin. Neurobiol. 47, 196–202. doi: 10.1016/j.conb.2017.11.004
Kefauver, J. M., Ward, A. B., and Patapoutian, A. (2020). Discoveries in structure and physiology of mechanically activated ion channels. Nature 587, 567–576. doi: 10.1038/s41586-020-2933-1
Komori, N., Neal, J., Cain, S. D., Logan, J., Wirsig, C., and Miller, K. E. (2003). Presence of beta-arrestin-1 immunoreactivity in the cutaneous nerve fibers of rat glabrous skin. Brain. Res. 988, 121–129. doi: 10.1016/s0006-8993(03)03356-0
Koser, D. E., Thompson, A. J., Foster, S. K., Dwivedy, A., Pillai, E. K., Sheridan, G. K., et al. (2016). Mechanosensing is critical for axon growth in the developing brain. Nat. Neurosci. 19, 1592–1598. doi: 10.1038/nn.4394
Krimm, R. F., Davis, B. M., Noel, T., and Albers, K. M. (2006). Overexpression of neurotrophin 4 in skin enhances myelinated sensory endings but does not influence sensory neuron number. J. Comp. Neurol. 498, 455–465. doi: 10.1002/cne.21074
LeMaster, A. M., Krimm, R. F., Davis, B. M., Noel, T., Forbes, M. E., Johnson, J. E., et al. (1999). Overexpression of brain-derived neurotrophic factor enhances sensory innervation and selectively increases neuron number. J. Neurosci. 19, 5919–5931. doi: 10.1523/jneurosci.19-14-05919.1999
Luo, W., Enomoto, H., Rice, F. L., Milbrandt, J., and Ginty, D. D. (2009). Molecular identification of rapidly adapting mechanoreceptors and their developmental dependence on ret signaling. Neuron 64, 841–856. doi: 10.1016/j.neuron.2009.11.003
Mahmud, A. A., Nahid, N. A., Nassif, C., Sayeed, M. S., Ahmed, M. U., Parveen, M., et al. (2017). Loss of the proprioception and touch sensation channel PIEZO2 in siblings with a progressive form of contractures. Clin. Genet. 91, 470–475. doi: 10.1111/cge.12850
Maksimovic, S., Baba, Y., and Lumpkin, E. A. (2013). Neurotransmitters and synaptic components in the Merkel cell-neurite complex, a gentle-touch receptor. Ann. NY Acad. Sci. 1279, 13–21. doi: 10.1111/nyas.12057
Maksimovic, S., Nakatani, M., Baba, Y., Nelson, A. M., Marshall, K. L., Wellnitz, S. A., et al. (2014). Epidermal Merkel cells are mechanosensory cells that tune mammalian touch receptors. Nature 509, 617–621. doi: 10.1038/nature13250
Maro, G. S., Vermeren, M., Voiculescu, O., Melton, L., Cohen, J., Charnay, P., et al. (2004). Neural crest boundary cap cells constitute a source of neuronal and glial cells of the PNS. Nat. Neurosci. 7, 930–938. doi: 10.1038/nn1299
Martinac, B., and Poole, K. (2018). Mechanically activated ion channels. Int. J. Biochem. Cell. Biol. 97, 104–107. doi: 10.1016/j.biocel.2018.02.011
McCormick, A. M., Jarmusik, N. A., and Leipzig, N. D. (2015). Co-immobilization of semaphorin3A and nerve growth factor to guide and pattern axons. Acta. Biomater. 28, 33–44. doi: 10.1016/j.actbio.2015.09.022
Montaño, J. A., Calavia, M. G., García-Suárez, O., Suarez-Quintanilla, J. A., Gálvez, A., Pérez-Piñera, P., et al. (2009). The expression of ENa(+)C and ASIC2 proteins in Pacinian corpuscles is differently regulated by TrkB and its ligands BDNF and NT-4. Neurosci. Lett. 463, 114–118. doi: 10.1016/j.neulet.2009.07.073
Munger, B. L., and Ide, C. (1988). The structure and function of cutaneous sensory receptors. Arch. Histol. Cytol. 51, 1–34. doi: 10.1679/aohc.51.1
Nakatani, M., Maksimovic, S., Baba, Y., and Lumpkin, E. A. (2015). Mechanotransduction in epidermal Merkel cells. Pflug. Arch. 467, 101–108. doi: 10.1007/s00424-014-1569-0
Nave, K. A., and Salzer, J. L. (2006). Axonal regulation of myelination by neuregulin 1. Curr. Opin. Neurobiol. 16, 492–500. doi: 10.1016/j.conb.2006.08.008
Neubarth, N. L., Emanuel, A. J., Liu, Y., Springel, M. W., Handler, A., Zhang, Q., et al. (2020). Meissner corpuscles and their spatially intermingled afferents underlie gentle touch perception. Science 368:eabb2751. doi: 10.1126/science.abb2751
Nikolaev, Y. A., Feketa, V. V., Anderson, E. O., Gracheva, E. O., and Bagriantsev, S. N. (2020). Lamellar cells in Pacinian and Meissner corpuscles are touch sensors. Sci. Adv. 6:eabe6393. doi: 10.1126/sciadv.abe6393
Olson, W., Dong, P., Fleming, M., and Luo, W. (2016). The specification and wiring of mammalian cutaneous low-threshold mechanoreceptors. Wiley Interdiscip. Rev. Dev. Biol. 5, 389–404. doi: 10.1002/wdev.229
Page, A. J., Brierley, S. M., Martin, C. M., Martinez-Salgado, C., Wemmie, J. A., Brennan, T. J., et al. (2004). The ion channel ASIC1 contributes to visceral but not cutaneous mechanoreceptor function. Gastroenterology 127, 1739–1747. doi: 10.1136/gut.2005.071084
Paré, M., Elde, R., Mazurkiewicz, J. E., Smith, A. M., and Rice, F. L. (2001). The Meissner corpuscle revised: a multiafferented mechanoreceptor with nociceptor immunochemical properties. J. Neurosci. 21, 7236–7246. doi: 10.1523/JNEUROSCI.21-18-07236.2001
Pawson, L., and Bolanowski, S. J. (2002). Voltage-gated sodium channels are present on both the neural and capsular structures of Pacinian corpuscles. Somatosens. Mot. Res. 19, 231–237. doi: 10.1080/0899022021000009152
Pawson, L., Pack, A. K., and Bolanowski, S. J. (2007). Possible glutaminergic interaction between the capsule and neurite of Pacinian corpuscles. Somatosens. Mot. Res. 24, 85–95. doi: 10.1080/0899022070138836
Pawson, L., Prestia, L. T., Mahoney, G. K., Güçlü, B., Cox, P. J., and Pack, A. K. (2009). GABAergic/glutamatergic-glial/neuronal interaction contributes to rapid adaptation in pacinian corpuscles. J. Neurosci. 29, 2695–2705. doi: 10.1523/jneurosci.5974-08.2009
Pawson, L., Slepecky, N. B., and Bolanowski, S. J. (2000). Immunocytochemical identification of proteins within the Pacinian corpuscle. Somatosens. Mot. Res. 17, 159–170. doi: 10.1080/08990220050020571
Pérez-Piñera, P., García-Suarez, O., Germanà, A., Díaz-Esnal, B., de Carlos, F., Silos-Santiago, I., et al. (2008). Characterization of sensory deficits in TrkB knockout mice. Neurosci. Lett. 433, 43–47. doi: 10.1016/j.neulet.2007.12.035
Petersen, J., and Adameyko, I. (2017). Nerve-associated neural crest: peripheral glial cells generate multiple fates in the body. Curr. Opin. Genet. Dev. 45, 10–14. doi: 10.1016/j.gde.2017.02.006
Ranade, S. S., Syeda, R., and Patapoutian, A. (2015). Mechanically Activated Ion Channels. Neuron 87, 1162–1179. doi: 10.1016/j.neuron.2015.08.032
Ranade, S. S., Woo, S. H., Dubin, A. E., Moshourab, R. A., Wetzel, C., Petrus, M., et al. (2014). Piezo2 is the major transducer of mechanical forces for touch sensation in mice. Nature 516, 121–125. doi: 10.1038/nature13980
Reed, C. B., Feltri, M. L., and Wilson, E. R. (2021). Peripheral glia diversity. J. Anat. 2021:13484. doi: 10.1111/joa.13484
Ruan, N., Tribble, J., Peterson, A. M., Jiang, Q., Wang, J. Q., and Chu, X. P. (2021). Acid-Sensing Ion Channels and Mechanosensation. Int. J. Mol. Sci. 22:4810. doi: 10.3390/ijms22094810
Samanta, A., Hughes, T. E. T., and Moiseenkova-Bell, V. Y. (2018). Transient Receptor Potential (TRP) Channels. Subcell. Biochem. 87, 141–165. doi: 10.1007/978-981-10-7757-9_6
Sames, K., Halata, Z., Jojovic, M., van Damme, E. J., Peumans, W. J., Delpech, B., et al. (2001). Lectin and proteoglycan histochemistry of feline pacinian corpuscles. J Histochem. Cytochem. 49, 19–28. doi: 10.1177/002215540104900103
Saxod, R. (1978). “Development of cutaneous sensory receptors in birds,” in Development of Sensory Systems, ed. M. Jacobson (Belin-Heidelberg: Springer), 338–417.
Schecterson, L. C., and Bothwell, M. (1992). Novel roles for neurotrophins are suggested by BDNF and NT-3 mRNA expression in developing neurons. Neuron 9, 449–466. doi: 10.1016/0896-6273(92)90183-e
Schwaller, F., Bégay, V., García-García, G., Taberner, F. J., Moshourab, R., McDonald, B., et al. (2021). USH2A is a Meissner’s corpuscle protein necessary for normal vibration sensing in mice and humans. Nat. Neurosci. 24, 74–81. doi: 10.1038/s41593-020-00751-y
Sedý, J., Szeder, V., Walro, J. M., Ren, Z. G., Nanka, O., Tessarollo, L., et al. (2004). Pacinian corpuscle development involves multiple Trk signaling pathways. Dev. Dyn. 231, 551–563. doi: 10.1002/dvdy.20156
Sedý, J., Tseng, S., Walro, J. M., Grim, M., and Kucera, J. (2006). ETS transcription factor ER81 is required for the Pacinian corpuscle development. Dev. Dyn. 235, 1081–1089. doi: 10.1002/dvdy.20710
Sharif-Naeini, R. (2015). Contribution of mechanosensitive ion channels to somatosensation. Prog. Mol. Biol. Transl. Sci. 131, 53–71. doi: 10.1016/bs.pmbts.2014.11.011
Singhvi, A., and Shaham, S. (2019). Glia-Neuron Interactions in Caenorhabditis elegans. Annu. Rev. Neurosci. 42, 149–168. doi: 10.1146/annurev-neuro-070918-050314
Sukharev, S., and Sachs, F. (2012). Molecular force transduction by ion channels – diversity and unifying principles. J. Cell. Sci. 125, 3075–3083. doi: 10.1242/jcs.092353
Suzuki, M., Mizuno, A., Kodaira, K., and Imai, M. (2003). Impaired pressure sensation in mice lacking TRPV4. J. Biol. Chem. 278, 22664–22668. doi: 10.1074/jbc.M302561200
Vega, J. A., Esteban, I., Naves, F. J., del Valle, M. E., and Malinovsky, L. (1995). Immunohistochemical localization of laminin and type IV collagen in human cutaneous sensory nerve formations. Anat. Embryol. 191, 33–39. doi: 10.1007/bf00215295
Vega, J. A., García-Suárez, O., Montaño, J. A., Pardo, B., and Cobo, J. M. (2009). The Meissner and Pacinian sensory corpuscles revisited: new data from the last decade. Microsc. Res. Tech. 72, 299–309. doi: 10.1002/jemt.20651
Vega, J. A., Haro, J. J., and Del Valle, M. E. (1996). Immunohistochemistry of human cutaneous Meissner and Pacinian corpuscles. Micros. Res. Tech. 34, 351–361.
Vega, J. A., López-Muñiz, A., Calavia, M. G., García-Suárez, O., Cobo, J., Otero, J., et al. (2012). Clinical implication of Meissner‘s corpuscles. CNS Neurol. Disord. Drug Targets 11, 856–868. doi: 10.2174/1871527311201070856
Wai, V., Roberts, L., Michaud, J., Bent, L. R., and Clark, A. L. (2021). The anatomical distribution of mechanoreceptors in mouse hind paw skin and the influence of integrin α1β1 on meissner-like corpuscle density in the footpads. Front. Neuroanat. 15:628711. doi: 10.3389/fnana.2021.628711
Widera, D., Heimann, P., Zander, C., Imielski, Y., Heidbreder, M., Heilemann, M., et al. (2011). Schwann cells can be reprogrammed to multipotency by culture. Stem. Cells. Dev. 20, 2053–2064. doi: 10.1089/scd.2010.0525
Woo, S. H., Lukacs, V., de Nooij, J. C., Zaytseva, D., Criddle, C. R., Francisco, A., et al. (2015a). Piezo2 is the principal mechanotransduction channel for proprioception. Nat. Neurosci. 18, 1756–1762. doi: 10.1038/nn.4162
Woo, S. H., Lumpkin, E. A., and Patapoutian, A. (2015b). Merkel cells and neurons keep in touch. Trends Cell Biol. 25, 74–81. doi: 10.1016/j.tcb.2014.10.003
Woo, S. H., Ranade, S., Weyer, A. D., Dubin, A. E., Baba, Y., Qiu, Z., et al. (2014). Piezo2 is required for Merkel-cell mechanotransduction. Nature 509, 622–626. doi: 10.1038/nature13251
Zelená, J. (1994). Nerves and mechanoreceptors: the role of innervation in the development and maintenance of mammalian mechanoreceptors. New York, NY: Chapman & Hall.
Keywords: lamellar cells, sensory corpuscles, mechanoreceptors, ion channels, mechanotransduction
Citation: Suazo I, Vega JA, García-Mesa Y, García-Piqueras J, García-Suárez O and Cobo T (2022) The Lamellar Cells of Vertebrate Meissner and Pacinian Corpuscles: Development, Characterization, and Functions. Front. Neurosci. 16:790130. doi: 10.3389/fnins.2022.790130
Received: 06 October 2021; Accepted: 21 January 2022;
Published: 09 March 2022.
Edited by:
Laura Bianchi, University of Miami, United StatesReviewed by:
Slav Bagriantsev, Yale University, United StatesCopyright © 2022 Suazo, Vega, García-Mesa, García-Piqueras, García-Suárez and Cobo. This is an open-access article distributed under the terms of the Creative Commons Attribution License (CC BY). The use, distribution or reproduction in other forums is permitted, provided the original author(s) and the copyright owner(s) are credited and that the original publication in this journal is cited, in accordance with accepted academic practice. No use, distribution or reproduction is permitted which does not comply with these terms.
*Correspondence: José A. Vega, amF2ZWdhQHVuaW92aS5lcw==
Disclaimer: All claims expressed in this article are solely those of the authors and do not necessarily represent those of their affiliated organizations, or those of the publisher, the editors and the reviewers. Any product that may be evaluated in this article or claim that may be made by its manufacturer is not guaranteed or endorsed by the publisher.
Research integrity at Frontiers
Learn more about the work of our research integrity team to safeguard the quality of each article we publish.