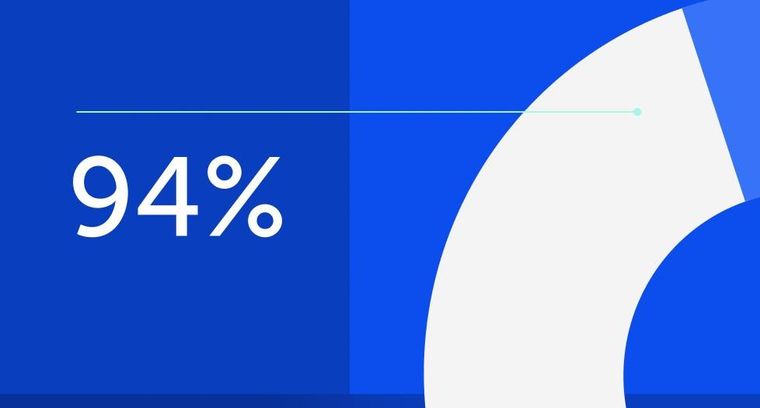
94% of researchers rate our articles as excellent or good
Learn more about the work of our research integrity team to safeguard the quality of each article we publish.
Find out more
REVIEW article
Front. Neurosci., 16 December 2022
Sec. Neurodevelopment
Volume 16 - 2022 | https://doi.org/10.3389/fnins.2022.1021721
This article is part of the Research TopicNeurodevelopment: Parental Influences, In Utero Exposures, and GeneticsView all 10 articles
Pregnant women constitute one of the most vulnerable populations to be affected by severe acute respiratory syndrome coronavirus 2 (SARS-CoV-2) infection, the cause of coronavirus disease 2019. SARS-CoV-2 infection during pregnancy could negatively impact fetal brain development via multiple mechanisms. Accumulating evidence indicates that mother to fetus transmission of SARS-CoV-2 does occur, albeit rarely. When it does occur, there is a potential for neuroinvasion via immune cells, retrograde axonal transport, and olfactory bulb and lymphatic pathways. In the absence of maternal to fetal transmission, there is still the potential for negative neurodevelopmental outcomes as a consequence of disrupted placental development and function leading to preeclampsia, preterm birth, and intrauterine growth restriction. In addition, maternal immune activation may lead to hypomyelination, microglial activation, white matter damage, and reduced neurogenesis in the developing fetus. Moreover, maternal immune activation can disrupt the maternal or fetal hypothalamic-pituitary-adrenal (HPA) axis leading to altered neurodevelopment. Finally, pro-inflammatory cytokines can potentially alter epigenetic processes within the developing brain. In this review, we address each of these potential mechanisms. We propose that SARS-CoV-2 could lead to neurodevelopmental disorders in a subset of pregnant women and that long-term studies are warranted.
In December of 2019 a novel virus, severe acute respiratory syndrome coronavirus 2 (SARS-CoV-2), caused a pneumonia outbreak in Wuhan City, Hubei Province in China. The disease caused by the virus was designated coronavirus disease 19 (COVID-19). SARS-CoV-2 expanded rapidly across the globe, and on March 11, 2020 the World Health Organization [WHO] (2022) declared COVID-19 a pandemic and global public health emergency. As of November 22, 2022 [WHO Coronavirus (COVID-19) Dashboard | WHO Coronavirus (COVID-19) Dashboard with Vaccination Data], 634 million cases have been detected worldwide. SARS-CoV-2 has already posed a great threat not only to the health of the people but also to the economy and healthcare system.
Severe acute respiratory syndrome coronavirus 2 (SARS-CoV-2) is an enveloped, positive stranded ribonucleic acid (RNA) virus of the family of Coronaviridae that causes respiratory and gastrointestinal infections ranging from mild, self-limiting conditions to more serious disorders such as viral pneumonia with systemic impairment (di Mascio et al., 2020). Unfortunately, pregnant women constitute one of the most vulnerable groups to be affected by this viral infection, due to anatomical, reproductive, endocrine, and immune changes (Zhao et al., 2020). With specific regard to the latter, immunological changes in pregnancy result in suppressed cell mediated immunity which would increase susceptibility to SARS-CoV-2 (Liu et al., 2020). In addition, pregnant women are more likely to have severe disease and ICU admissions compared to their non-pregnant counterparts after adjusting for age, underlying medical conditions, race, and ethnicity (Ellington et al., 2020; Sutton et al., 2020). As per a systematic review of over 11,000 pregnant women with suspected or confirmed COVID-19, the disease commonly manifests as fever (40%), cough (39%), dyspnea (19%), loss of taste (15%), myalgia (10%) and diarrhea (7%) (Allotey et al., 2020).
The novel SARS-CoV-2 infection could negatively impact fetal brain development in both direct and indirect ways (Figure 1) (Ellul et al., 2020). Regarding the direct route, an increasing number of case studies provide evidence for transplacental transmission of SARS-CoV-2, which could invade the central nervous system and disrupt brain development. Regarding indirect routes, SARS-CoV-2 could produce placental dysfunction, preeclampsia, and preterm birth, and trigger immune responses in the mother, which could, in turn affect the developing fetus. Interestingly, many of these routes involve the action of pro-inflammatory cytokines. Preclinical studies have revealed that inducing inflammation during the perinatal period produces long-term alterations in brain structure and function and a wealth of epidemiological studies have documented associations between infection-induced immune activation and offspring neuropsychiatric risk. In this review, we address each of these potential mechanisms and propose that SARS-CoV-2 could lead to neurodevelopmental disorders in a subset of pregnant women. We also review emerging empirical evidence supporting this hypothesis. This manuscript builds upon prior review articles on this topic such as (Shook et al., 2022), and (Figueiredo et al., 2021), which focused primarily on emerging evidence for transplacental transmission and the role of maternal immune activation (MIA), and (Kleeman et al., 2022), which focused primarily on epigenetic mechanisms in MIA. The current manuscript aims to be both comprehensive and concise. It provides a more detailed discussion of how altered levels of glucocorticoids in the context of SARS-CoV-2 could affect fetal neurodevelopment, and is the first, to our knowledge, to raise the possibility that SARS-CoV-2 induced hypocortisolism could be a risk factor for adverse neurodevelopmental outcomes. Finally, this review includes the most recent empirical studies on neurodevelopmental consequences of in utero exposure to SARS-CoV-2 including (Aldrete-Cortez et al., 2022; Hessami et al., 2022; Shuffrey et al., 2022).
Several viruses are known to be transmitted from pregnant women to their children and subsequently disrupt neurodevelopment. These are the TORCH pathogens. TORCH is an acronym standing for Toxoplasma gondii, Other infections, Rubella, human Cytomegalovirus (HCMV), and Herpes simplex viruses 1 and 2 (HSV-1 and HSV-2, respectively). “Other infections” include human immunodeficiency virus (HIV), syphilis, parvovirus B19 (fifth disease), varicella (chickenpox) and Zika (reviewed in Schwartz and Hyg, 2017). HCMV and HSV infections are the most common causes of neonatal morbidity worldwide (Looker et al., 2017; Marsico and Kimberlin, 2017) and in recent years Zika virus remains a threat for pregnant women (reviewed in Spitz, 2019). These TORCH pathogens can induce brain calcifications, major brain malformations including microcephaly, and neurodevelopmental disorders (Chen et al., 2021; Krenn et al., 2021). Consequently, one of the first questions we ought to ask when considering the adverse neurodevelopmental potential of SARS-CoV-2 is whether there is evidence of maternal transmission to the fetus or neonate.
Transmission of SARS-CoV-2 from mother to child could occur transplacentally, during labor and delivery, or in the early post-partum period. The possibility of vertical transmission of SARS-CoV-2 from mother to fetus in utero is currently a topic of widespread debate. The published literature is both sparse and contradictory, with some reports supporting direct in utero transmission (Bahadur et al., 2020; Dong et al., 2020; Patanè et al., 2020), and others suggesting little or no vertical transmission (Dashraath et al., 2020; Khan et al., 2020; Xiong et al., 2020; Zhu et al., 2020). The presence of SARS-CoV-2 either in amniotic fluid, placental samples, or infant nasopharyngeal swabs collected shortly after birth, represents compelling evidence for an in utero infection. Several case studies have been published documenting such evidence. Sisman et al. (2020) reported a case of congenital SARS-CoV-2 in an infant born through vaginal delivery to a COVID-19 positive mother. This report confirmed the intrauterine transmission of SARS-CoV-2 via presence of SARS-CoV-2 nucleocapsid protein and viral particles in placental syncytiotrophoblastic cells and nasopharyngeal samples of the infant (Sisman et al., 2020). Similarly, Patanè et al. (2020), demonstrated vertical transmission of SARS-CoV-2 from mother to fetus in utero as evidenced by the presence of SARS-CoV-2 RNA on the fetal side of placental tissues (Patanè et al., 2020). Transplacental transmission of SARS-CoV-2 is also supported by reports of caesarean delivery where strict neonatal isolation was implemented immediately after birth without delayed cord clamping or skin to skin contact. In one such case study, neonatal nasopharyngeal swabs were positive for SARS-CoV-2 RT-PCR test within 16 h of birth despite these precautions (Alzamora et al., 2020) and within 24 h in the other (Kirtsman et al., 2020). Perhaps the strongest early evidence supporting congenital infection was reported by Kirtsman et al. (2020). In this case, a woman with active SARS-CoV-2 delivered via caesarean delivery. The neonate had no contact with vaginal secretions or maternal skin. Artificial rupture of membranes was performed at operation, which was conducted with airborne, droplet, and contact precautions. The infant was immediately removed from the operative field, in a sterile fashion, to a resuscitator 2 m away in the same room. Never-the-less, neonatal nasopharyngeal swabs were SARS-CoV-2 positive by RT-PCR test on the day of birth as well as day 2 and day 7. Furthermore, placental micrographs revealed multiple areas of infiltration by inflammatory cells and extensive early infarction (Kirtsman et al., 2020). While, a number of researchers cautioned against treating early data as conclusive (Kimberlin and Stagno, 2020), evidence for transplacental transmission has continued to accumulate, though it appears to be a very rare event. In a study of 427 pregnant women from the UK admitted to hospital with SARS-CoV-2 infection, 12 of 265 infants tested positive, a rate of 5%, though only 6 of those did so within the first 12 h after birth (Knight et al., 2020). A recent systematic review and meta-analysis including data up to 3 August 2021 and including over 14,000 babies born to mothers with SARS-CoV-2 infection found about 2% of babies tested positive with 14 confirmed mother-to-child vertical transmission, seven of which occurred in utero (Allotey et al., 2022). A slightly smaller systematic review of 47 studies and over 900 neonates reported that slightly less than 1% had a confirmed or probable vertical transmission of infection (Jeganathan and Paul, 2022). Similarly, a recent “systemic review of systematic reviews” suggested that mother to child transmission was relatively rare with about 70% of cases attributable to environmental exposure and about 20% related to potential vertical transmission (Musa et al., 2021). Overall, it appears that transplacental transmission is possible, but rare.
Severe acute respiratory syndrome coronavirus 2 (SARS-CoV-2) is similar in many ways to SARS-CoV, a virus identified in 2003, which is known have neuroinvasive potential (Ding et al., 2004; Xu et al., 2005), as evidenced by its presence in neural tissue from SARS autopsies (Gu et al., 2005). Studies indicate that the genomic sequence is similar between SARS-CoV-2 and SARS-CoV (Lu et al., 2020; Yu et al., 2020). It is particularly notable that the receptor-binding domain of SARS-CoV is structurally similar to SARS-CoV-2 (Lu et al., 2020). Hence, it is possible that SARS-CoV-2 follows the same path of neuroinvasiveness as SARS-CoV using the ACE2 receptor for cellular entry into the human brain. Several hypotheses have been put forth regarding possible mechanisms of SARS-CoV-2 mediated neural invasion including: (1) Transplacental transmission could induce viremia which would promote viral binding to the endothelial ACE2 receptors of the blood brain barrier (BBB) and subsequently entry into the central nervous system (CNS). Electron micrography on post-mortem brain biopsies revealed viral particles in the frontal cortex of a SARS-CoV-2 infected adult. The presence of particles in brain capillary endothelium and blebbing of viral-like particles coming in/out of the endothelial wall strongly suggested neuroinvasion through the BBB (Paniz-Mondolfi et al., 2020). (2) Cells of the immune system (macrophages and monocytes), which may express the ACE2 receptor, could act as a reservoir for dissemination into the CNS (Desforges et al., 2014). Further, infected immune cells (monocytes neutrophils and T cells) may disseminate into brain via various entry points including meninges, vasculatures, and the choroid plexus (Iadecola et al., 2020). (3) Neurons in the gut could carry the virus into the CNS via retrograde axonal transport (Esposito et al., 2020). (4) The virus could enter the CNS through the olfactory bulb. This possibility is strengthened by SARS-CoV-2 induced anosmia being a notable symptom during viral infection. Studies have shown expression of ACE2 receptors and other receptors that can facilitate SARS-CoV-2 binding in the olfactory epithelium (Fodoulian et al., 2020). This could play a role in neonatal infection during delivery through contact with vaginal secretions or soon after delivery through other means (physical or airborne). (5) Finally, the lymphatic pathway represents another possible route for neuroinvasion by the SARS-CoV-2 virus. The virus may directly enter the brain via olfactory/cervical lymphatic vessels (Bostancıklıoğlu, 2020).
One key question when considering the neuroinvasive potential of SARS-CoV-2 in utero is whether the fetal brain expresses cellular components that interact with the spike protein of SARS-CoV-2. Using publicly available RNA sequencing datasets, Varma et al. (2021) revealed that while ACE2 mRNA is expressed at relatively low levels in the fetal brain, other spike protein interactors including FURIN, ZDHHC5, GOLGA7, and ATP1A1 are highly expressed, especially in neurons. These proteins may play key roles in SARS-CoV-2 fetal brain pathogenesis, especially during the 2nd and 3rd trimesters of pregnancy (Varma et al., 2021).
Even in the absence of direct transmission of a pathogen from mother to child, infections can disrupt neurodevelopment in indirect ways. For example, the H1N1 influenza virus is not teratogenic, but severe infections were associated with elevated risks for adverse infant outcomes, such as preterm birth, which have neurodevelopmental consequences [Maternal and Infant Outcomes Among Severely Ill Pregnant and Postpartum Women with 2009 Pandemic Influenza A (H1N1) — United States, April 2009–August 2010; Newsome et al., 2019]. In this section we discuss emerging evidence that SARS-CoV2 impacts placental functioning and how this could lead to altered neurodevelopment.
Severe acute respiratory syndrome coronavirus 2 (SARS-CoV-2) binding ACE2 receptors are highly expressed in placental tissues. SARS-CoV-2 infected pregnant women have placental inflammatory signs along with systemic maternal inflammation (Vivanti et al., 2020), which can lead to placental microvascular dysfunction. This may present clinically as preeclampsia or preeclampsia-like features, fetal distress, intrauterine growth restriction, and/or or preterm labor depending on gestational age at time of SARS-CoV-2 infection (Mulvey et al., 2020).
To understand the impact of COVID-19 infection on the placenta, a brief review of the salient aspects of the renin–angiotensin system (RAS) axis in the formation of a well perfused placental vascular bed may be helpful. In the maternal portion of the human placenta, which is derived from the maternal stromal cells, ACE2 is highly expressed in the invading and intravascular trophoblast and in decidual cells. ACE2 is also found in arterial and venous endothelium and smooth muscle of the umbilical cord (Valdés et al., 2006). Levels of ACE2 vary temporally depending on gestational age in both humans and rodents (Valdés et al., 2006; Ghadhanfar et al., 2017). The various components of RAS-Ang II, ACE2, and Ang-(1-7) function mainly to regulate blood pressure and fetal development. Ang II stimulates trophoblast invasion in rat and human cells (Hering et al., 2010). Ang-(1-7) and ACE2 may act as local autocrine/paracrine regulators in the early (angiogenesis, apoptosis, and growth) and late (uteroplacental blood flow) events of pregnancy (Neves et al., 2008). ACE2 hydrolyzes Ang II into Ang-(1-7), and Ang I into Ang-(1-9), which is quickly converted to Ang-(1-7), thereby controlling the blood pressure and hydro-salinity balance of pregnant women (Pringle et al., 2011).
Preeclampsia is a serious pregnancy complication which typically begins after the 20th week of gestation and manifests as high blood pressure and proteinuria. The exact cause of preeclampsia is not known, but it is generally believed to arise due to improper functioning of the placenta. Placental vascular anomalies and inflammation are often observed in women affected by preeclampsia (Harmon et al., 2016; Ramos et al., 2017). Brosnihan et al. (2004), reported that pre-eclamptic women presented with suppressed plasma Ang-(1-7) levels when compared with normal pregnancy subjects (Brosnihan et al., 2004). Furthermore, high expression of Ang II in the placental villus during preeclampsia can cause decreased blood flow and nutrition supply to the fetus (Shibata et al., 2006; Anton and Brosnihan, 2008; Anton et al., 2009). An observational study published early in the pandemic suggested that prevalence of preeclampsia is remarkably higher in SARS-CoV-2 infected pregnant women, with five out of eight infected pregnant women admitted to the intensive care unit having preeclampsia like syndrome (Mendoza et al., 2020), which may lead to higher occurrence of preterm birth (Yan et al., 2020). A case report by Hosier and colleagues might be an example of placental infection with SARS-CoV-2 or manifestation of SARS-CoV-2 induced cytokine release or both presenting as severe early-onset preeclampsia. The patient, who had a history of gestational hypertension in a prior pregnancy, but normal blood pressure during early pregnancy and normal baseline preeclampsia evaluation, presented acutely at 22-weeks’ gestation with features mimicking preeclampsia with Disseminated Intravascular Coagulation (DIC) and fever. SARS-CoV-2 RNA was positive in a nasopharyngeal swab. Patient’s thrombocytopenia and hypofibrinogenemia were more severe than what would have been expected from SARS-CoV-2 alone. Placental pathology post termination of the pregnancy revealed SARS-CoV-2 localized to the synciotiotrophoblast layer and the intervillous invasion of macrophages (intervillositis) (Hosier et al., 2020). A study by Mulvey et al. (2020), on placenta (after term delivery) of COVID-19 infected pregnant women found fetal vascular malperfusion due to focal avascular villi and thrombi in large fetal vessels (Mulvey et al., 2020). Vivanti et al. (2020) also reported a case of delivery at 35 weeks through cesarean section following fetal distress indicated by category 3 fetal heart rate tracing, and RT-PCR in the placenta was positive for SARS-CoV-2. The mother who was having an uneventful pregnancy until diagnosis of COVID-19, without any severe or critical presentation of the infection, had thrombocytopenia, lymphopenia, elevated acute phase reactants, and abnormalities in coagulation cascade on admission. Three days after hospitalization, without any deterioration of maternal status, a category 3 fetal heart tracing was observed, representing fetal compromise likely due to uteroplacental insufficiency for which delivery through cesarean section was performed (Vivanti et al., 2020). This strongly indicates that SARS-CoV-2 can cause uteroplacental dysfunction and can induce a preeclampsia like picture either due to direct placental invasion or through induction of excess cytokine release in the mother or both. However, one must acknowledge that much of the empirical evidence for this hypothesis comes from case studies, which may not generalize to all SARS-CoV-2 infected mothers. Case studies may also be subject to researcher bias and do not allow the production of quantifiable risk estimates. A recent systematic review of cardiovascular complications among pregnant women with COVID-19 found substantial variance in estimates across studies with some reporting rates of preeclampsia as high as 69% and others as low as 0.5% (Yaghoobpoor et al., 2022).
A potential mechanism by which SARS-CoV-2 could induce placental dysfunction and preeclampsia is illustrated in Figure 2. The viral spike protein of SARS-CoV-2 facilitates binding to the ACE2 receptor. When viremia occurs in the mother during severe SARS-CoV-2 infection, the virus may invade the placenta. The viral spike protein of SARS-CoV-2 after binding with ACE2 receptor, enters the placental trophoblast with the help of protease-mediated cleavage (TMPRSS2 or cathepsin L) of the S protein subunit- S2. This internalization of the virus along with the ACE2 receptor into the placental trophoblast may increase the activity of ADAM17 (a matrix metalloproteinase) (reviewed in Schreiber et al., 2021; Jackson et al., 2022), as seen in previous SARS-CoV infection (Haga et al., 2008). ADAM17 up-regulation leads to proteolytic cleavage of the ACE2 ectodomain (Lambert et al., 2005; Heurich et al., 2014), which results in reduced membrane ACE2. The resulting imbalance in AngII/ACE2 interaction may result in hypertension of pregnancy, pre-eclampsia, or eclampsia in susceptible mothers. Of note, systemic inflammation and oxidative stress induced by SARS-CoV-2 infection may also lead to increased ADAM17 expression in the placental trophoblasts promoting the development of preeclampsia in susceptible women even in the absence of direct placental infection by SARS-CoV-2 (Gooz, 2010) (Figure 2).
Even in the absence of preeclampsia, SARS-CoV-2 infection in the early stages of pregnancy can cause fetal growth restriction (FGR)/intrauterine growth restriction (IUGR) (Allotey et al., 2020), which is itself a risk factor for abnormal postnatal neurodevelopment in babies (Adams Waldorf and McAdams, 2013; Dang et al., 2020). IUGR influences the overall growth of fetus and is accompanied by reduced total brain volume, which may cause cognitive (Hartkopf et al., 2018; reviewed in Miller et al., 2016) and motor regulation deficits (Dubois et al., 2008; Miller et al., 2016; Hartkopf et al., 2018; reviewed in Miller et al., 2016), and as well as some neurodevelopmental disorders. More specifically, reduced brain volumes have been observed in schizophrenia and bipolar disorder (Wright et al., 2000; McDonald et al., 2004; Arnone et al., 2009; Ellison-Wright and Bullmore, 2010; Haijma et al., 2013; Hibar et al., 2016, 2018; van Erp et al., 2016, 2018) and in ADHD (Boedhoe et al., 2020).
Epidemiological studies indicate a strong correlation between maternal viral infection and neuropsychiatric disorders in offspring, especially for ASD and schizophrenia (reviewed in Estes and McAllister, 2016). For ASD, this includes a large-scale registry-based study in Denmark which revealed that severe viral infections (requiring hospitalization) during the first trimester of pregnancy are associated with increased risk of ASDs in offspring (Atladóttir et al., 2010) and a cohort study conducted in Finland which suggested that early stage increases in gestational CRP due to prenatal infection increases risk of ASD in children by 43% (Brown et al., 2014). A meta-analysis of 15 studies, conducted in 2016 and including over 40,000 ASD cases, reported an OR of 1.13 for the association between maternal infection during pregnancy and increased risk of ASD in offspring [95% confidence interval (CI): 1.03–1.23], with risk being moderated by the severity of infection, type of infectious agent, time of infectious exposure, and site of infection. Greater severity of infection (indexed by hospitalization) was associated with higher risk. Bacterial infections appeared to confer greater risk than viral infections and genitourinary and skin infections appeared to confer higher risk than gastrointestinal or respiratory infections. With specific regard to infection timing, second trimester exposures conferred the greatest risk, followed by first trimester exposures, and third trimester exposures had minimal effects (Jiang et al., 2016). This differs from a recent meta-analysis evaluating the impact of maternal fever on neurodevelopmental disorders in general (includes ASD, ADHD, Developmental Delay, and Developmental Coordination Disorder) which suggested first trimester exposures were most detrimental. Regarding schizophrenia, since Mednick et al. (1988) published their seminal, ecological study revealing increased risk of schizophrenia in pregnant women exposed to the 1957 influenza A epidemic in Helsinki, evidence linking schizophrenia to maternal infection has accumulated and includes (Mednick et al., 1988; Barr et al., 1990; Susser et al., 2000; Brown et al., 2001, 2004a,b,2005; Buka et al., 2001; Mortensen et al., 2007). A recent meta-analysis of seven cohort studies reported that maternal infection during gestation increased the risk of non-affective psychosis with a relative risk (RR) of 1.28 (95% CI:1.05-1.57) (Saatci et al., 2021). Relative risk was even high for schizophrenia (1.63) with the strongest effects observed in the second trimester. Several studies have highlighted the second trimester as the time of greatest risk (Brown et al., 2000; Nielsen et al., 2013), which may indicate an impact of infection on gestational neurogenesis, which peaks during this period (Stiles and Jernigan, 2010). However, another study reported that the risk of schizophrenia was increased 7-fold for influenza exposure during the first trimester with no increased risk of schizophrenia for exposure during the second or third trimester (Brown et al., 2004a). Another study indicating that first trimester prenatal exposures may increase risk for schizophrenia in offspring is (Clarke et al., 2009). Despite these inconsistencies regarding timing of exposure, the general idea that gestational infection predisposes individuals to schizophrenia is widely accepted. This is not to say there are no controversies in the literature. For example, Selten et al. (2010) published a meta-analysis challenging earlier studies linking the 1957 influenza pandemic to schizophrenia (Selten et al., 2010). In addition, even large population-based studies can suffer from methodological problems such as misclassification of exposure and genetic confounding. Karlsson and Dalman (2020) argue that infections in general appear to have a much smaller effect on schizophrenia risk compared to specific exposures such as Toxoplasma gondii (Karlsson and Dalman, 2020). Of particular relevance to SARS-CoV-2, research suggests that second trimester respiratory infections are a risk factor for schizophrenia spectrum disorders (Brown et al., 2000). There are also epidemiological studies linking maternal infections to ADHD (Pineda et al., 2007; Mann and McDermott, 2011; Silva et al., 2014) and mood disorders (see Simanek and Meier, 2015 for review), but as the evidence is more limited (for the former) and ambiguous (for the latter), we do not provide additional details here. Activation of the pregnant mother’s immune system in response to infection is thought to be the primary mechanism responsible for these associations, a hypothesis that is supported by a substantial body of preclinical research (reviewed in Boksa, 2010; Careaga et al., 2017).
Like other viral infections, SARS-CoV-2 can trigger systemic inflammation during pregnancy in both mother and fetus. ACE2 receptors are expressed widely in the mouth, tongue, respiratory tract, lung, heart, kidney, gut, endothelium, and in other tissues like placental tissues (Deverman and Patterson, 2009). Binding of ACE2 located on the surface of the target cells with the receptor-binding domain of SARS-CoV-2 results in endocytosis and translocation of both viruses and ACE2 into the endosomes located in the cell. Inside the cell, it replicates and induces cytotoxicity. The damaged host cell undergoes pyroptosis and releases damage-associated molecular patterns resulting in the initial inflammatory response.
As noted in the introduction, pregnant women are more likely to experience severe SARS-CoV-2 infection and ICU admissions compared to their non-pregnant counterparts, and COVID-19 can induce a systemic inflammatory disorder. With increasing severity of the infection, higher levels of circulating cytokines and other inflammatory biomarkers like IL6, IL-1β, TNFα, C-reactive protein (CRP) and D-dimer occurs (Smith et al., 2007; Deverman and Patterson, 2009; Wu et al., 2017; Zupan et al., 2017). These proteins attract monocytes, macrophages, and T-cells to the site of infection, promoting further inflammation and establishing a pro-inflammatory feedback loop. In addition, non-neutralizing antibodies produced by B-cells may enhance SARS-CoV-2 infection through antibody-dependent enhancement, further exacerbating organ damage (Deverman and Patterson, 2009). The resulting cytokine storm circulates to other organs, leading to multi-organ damage.
Even in the absence of a cytokine storm, maternal immune activation during COVID-19 along with proinflammatory changes in the placental vascular bed could potentially activate interleukin (IL-6) signaling in the syncitiotrophoblast layer. Furthermore, increased cytokines and complement factors in the maternal environment can bleed over into the fetus, altering neurodevelopment. Pro-inflammatory cytokines including IL-6, IL-1β, and TNF-α have a molecular mass of about 50kDa and can easily cross the placental barrier, passing from mother to fetus (Zaretsky et al., 2004; Aaltonen et al., 2005; Smith et al., 2007; Deverman and Patterson, 2009; Ratnayake et al., 2013; Wu et al., 2017; Zupan et al., 2017). All neural and non-neural cell types within the developing CNS use cytokines for paracrine and autocrine signaling. Thus, maternal immune activation secondary to maternal infection can disrupt brain development in multiple ways, which we review briefly in the following paragraphs and summarize in Figure 3. Most of the studies discussed in the ensuing paragraphs used rodent models of maternal immune infection (MIA). Multiple models of MIA exist including prenatal administration of immunogenic liposaccharides (LPS), transmembrane protein toll-like receptor (TLR) 4, or polyriboinosinic–polyribocytidilic acid [Poly(I:C)]. The specific models used are noted throughout.
Figure 3. Maternal SARS-CoV-2 infection, inflammation, and subsequent fetal/neonatal brain development.
First, maternal immune activation can trigger periventricular white matter damage. White matter damage has been observed in the context of many different prenatal infections in human newborns and in animal models [Yoon et al., 1997 (rabbit, E. coli infection); Dammann et al., 1999; reviewed in Malaeb and Dammann, 2009], and in a newborn baby following transplacental transmission of SARS-CoV2 infection (Vivanti et al., 2020). These observations partly reflect associations between prenatal infection and preterm delivery, which is a well-established risk factor for intraventricular hemorrhage, neonatal white matter damage, and subsequent cerebral palsy. However, rodent studies confirm that white matter injury in offspring can be induced by intrauterine maternal infection in the absence of preterm birth [Wang et al., 2007; Zhan et al., 2021 (LPS)]. Mechanisms of injury may include both direct effects of pro-inflammatory cytokines on oligodendrocytes and axons and indirect effects via activation of microglia (reviewed in Robinson, 2005; Burd et al., 2012). Microglial cells enter the human brain as early as 4 weeks of gestation and accumulate in the prospective white matter of the corona radiata between weeks 19 and 24 (Monier et al., 2007). When activated, microglia cause localized neuroinflammation and injury [ Monier et al., 2007; reviewed in Burd et al., 2012]. Once activated, the microglia may continue to remain activated into infancy or early childhood, resulting in sustained production of pro-inflammatory cytokines, oxidative and nitrosative products, and excitotoxic metabolites such as glutamate and quinolinic acid, all of which can injure oligodendrocytes. Specific pro-inflammatory cytokines that have been linked to oligodendrocyte injury include IL-1β which impairs myelination by reducing the number of developing oligodendrocytes when injected into the cerebrum of rat pups (Cai et al., 2004) and TNF-α which induces death of human oligodendrocyte cells by activation of apoptosis-inducing factor (Yoon et al., 1997; Saliba and Henrot, 2001), With regard to axonal development, Makinodan et al. (2008) report that juvenile mice had reduced axonal diameters in the hippocampus following maternal immune activation [poly(I:C)], a phenotype that normalized by adulthood.
Second, maternal immune activation can influence developmental neurogenesis and neurodifferentiation. In general, hyperactivation of the immune response is thought to impair survival and differentiation of neural progenitors (Borsini et al., 2015; Kim et al., 2016) by attenuating the production of neurotrophic factors including brain-derived neurotrophic factor (BDNF), nerve growth factor (NGF), platelet-derived growth factors (PDGF), and neurotrophins (NT-3,4). Much of this evidence is based on in vitro models, which are though to best model human midgestational neurogenesis. However, in vitro models cannot fully capture the impact of infection on a pregnant mother. Furthermore, different cytokines induce different effects in in vitro models – some positive and some negative. For example, IL-1β and TNF-α reduce neurogenesis of fetal hippocampal neural progenitor cells (NPCs) (Johansson et al., 2008; Zunszain et al., 2012; Chen et al., 2013), while IL-6 was reported to increase neurogenesis in human hippocampal NPCs (Johansson et al., 2008). Very few animal studies have directly assessed the impact of MIA on developmental neurogenesis. One recent study examined Ki67 + /Nestin + and Tbr2 + neural progenitor cells in the subventricular zone (SVZ) of neonatal mice following mid-gestation MIA (LPS) and reported robust increases (Loayza et al., 2022). In a similar manner, significant increases in the proportion of Pax6-positive neural progenitor cells and Pax6/Tbr2 double-positive cells have been observed in mouse fetal brains 24 h after poly(I:C) injection (Tsukada et al., 2021). This contrasts with the findings of Canales et al. (2021) who reported evidence of overall decreased neurogenesis by E17.5, following poly(I:C) injection at E12.5 (Canales et al., 2021) and with Tsukada et al. (2015) who reported that mid-gestatational MI (Poly:I:C) impairs neurogenesis in the cerebellum. There is a rich body of literature demonstrating that pro-inflammatory cytokines disrupt neurogenesis in the adult rodent hippocampus [see (Kim et al., 2016) for review] and several MIA studies examined adult hippocampal neurogenesis. Mid-gestation MIA (LPS) suppressed hippocampal neurogenesis in adult rat (Okano et al., 2022), as did late gestation treatment with Poly(I:C) (Zhao et al., 2019), while suppression of maternal IL-6 enhanced it (Mouihate and Kalakh, 2021). Finally, defective neurogenesis in the subventricular zone (SVZ)-olfactory bulb (OB) pathway has been reported following early gestational exposure to Poly:I:C in mouse (Liu et al., 2013). The mechanisms by which MIA primes dysfunction in the unique hippocampal pool of neural stem/progenitor cells in adulthood remains to be fully elucidated (Couch et al., 2021). Furthermore, effects of specific cytokines on neurogenesis and differentiation may vary based on brain region, species, and developmental stage.
Finally, pro-inflammatory cytokines can promote cytoskeletal damage and neural apoptosis. Astrocytes may play a key role in this process. MIA (Poly:I:C) induces a hypertrophied morphology and intense GFAP immunoreactivity in astrocytes in the hippocampus that persist at least until weaning (Patro et al., 2013), with upregulation of GFAP detectable in adulthood following LPS (Berkiks et al., 2019). Hypertrophied morphology and upregulation of GFAP indicate astrocytic activation and astrocytes produce reactive oxygen species (ROS) including nitric oxide (NO), which are neurotoxic. Activated microglia also produce ROS, as discussed previously, which could damage neurons as well as glia. Increased oxidative stress has been observed in the hippocampus and cerebral cortex of adult rats exposed to LPS MIA (Cieślik et al., 2020, 2021). In Cieślik et al. (2021), oxidative stress did not appear to result from activated microglia but was accompanied by evidence of mitochondrial dysfunction (Cieślik et al., 2021), which has also been linked to oxidative damage in mouse models of autism spectrum disorder (Yui et al., 2015). Cieślik et al. (2021) also observed abnormal phosphorylation and dysfunction of MAPT, which is involved in assembling and stabilizing microtubules, which make up the cytoskeleton (Cieślik et al., 2021). Increased neural apoptosis appears to be linked to late-gestational MIA, rather than mid-gestation MIA (Meyer et al., 2006) [poly(I:C), mice] and appears to arise due to the interactive effect of multiple cytokines (Matelski et al., 2021) (in vitro model).
We end this section by noting that animal models of MIA can also help address questions about how timing of infection relates to neurodevelopmental sequelae. For example, Nakamura et al. (2022) recently reported that early gestational exposure to MIA [poly(I:C)] disrupted working memory and reduced perseverative behavior in female offspring while late gestational exposure induced male-specific deficits in working memory and reversal learning (Nakamura et al., 2022). Guma et al. (2022) have reported that early gestational exposure to MIA [poly(I:C)] induces profound reductions in certain regions of the embryonic brain, likely through increased apoptosis, while late gestational exposure induced volume expansions, possibly due to acute inflammatory responses (Guma et al., 2022). The same group has also presented timing of exposure specific effects on neonatal mice brain volumes in regions of the amygdala, hippocampus, entorhinal cortex, striatum, and periaqueductal gray matter and reported that neonatal communication abilities, indexed by ultrasonic vocalizations, are reduced following early, but not late exposure (Guma et al., 2021b). Early exposure also appears to produce more profound effects on anxiety-like, stereotypic, and sensorimotor gating behaviors, measured in adolescence, than late exposure, changes that are accompanied by transcriptional alteration in genes linked to inflammation and autistic behaviors (Guma et al., 2021a).
Maternal immune activation (MIA) is accompanied by increased levels of multiple pro-inflammatory cytokines. However, IL-6 appears to play an especially important role in mediating the impact of maternal infection on offspring neurodevelopment (Smith et al., 2007). This was demonstrated by Smith et al. (2007) via an elegant series of rodent experiments. First, they showed that administration of IL-6 during pregnancy was sufficient to induce prepulse inhibition (PPI) and latent inhibition (LI) deficits in adult offspring, while administration of IFNγ was not. Second, they demonstrated that administration of an IL-6-neutralizing antibody during MIA [poly(I:C)] rescued deficits in PPI and IL and normalized exploratory and social behavior. Next, they showed that IL-6 knock-out mice failed to exhibit deficit in PPI, social interaction, or exploratory behavior following MIA. Finally, they demonstrated that administration of an IL-6-neutralizing antibody during MIA also normalized gene expression differences in the brains of offspring (Smith et al., 2007). Hence, IL-6 emerged as the main driving factor through which MIA causes long term behavioral changes in offspring (Smith et al., 2007).
The importance of IL-6 in human brain development has been demonstrated via neuroimaging studies of infants and children. Specifically, Rudolph et al. (2018) reported associations between maternal IL-6 levels and neonatal functional brain connectivity, with the salience, dorsal attention, and subcortical networks being most extensively involved. Furthermore, these associations may explain associations between maternal IL-6 and offspring working memory performance at 2 years of age in the same sample (Rudolph et al., 2018). Effects of IL-6 on the salience network were also reported by Spann et al. (2018). Also, MRI data of infants (n = 30) shows that higher levels of IL-6 during pregnancy may lead to disruption in frontolimbic white matter and cognitive development (Rasmussen et al., 2019). Furthermore, children born to women (n = 86) with high IL-6 levels during early pregnancy showed larger right amygdala volumes and stronger bilateral amygdala connectivity to other parts of brain including fusiform, somatosensory cortex and thalamus (for sensory processing and integration), anterior insula (for salience detection), caudate and parahippocampal gyrus (for learning and memory) at 24 months age. Moreover, volume of the right amygdala and stronger left amygdala connectivity mediated associations between maternal IL-6 and compromised impulse control in offspring (Graham et al., 2018). While these studies are relevant to the issue at hand, it is important to note that the sample sizes are relatively small. A recent study by Marek et al. (2022), suggests that rigorous and reproducible associations between brain structure or function and complex cognitive or behavioral data may require thousands of individuals (Marek et al., 2022). In addition to being insufficiently powered, small sample sizes are vulnerable to sampling variability, inflated effect sizes, high statistical error rates, and poor reproducibility.
Various mechanisms have been proposed to explain how IL-6 induces abnormal neurodevelopment (Boulanger-Bertolus et al., 2018). Many of these mechanisms highlight the placenta as a key organ in this pathophysiological process. Knockout of the trophoblastic IL-6 receptor in mice prevents cerebellar neuropathology and behavioral impairments following MIA [poly(I:C)] and attenuates immune responses in the fetal brain (Wu et al., 2017). This suggests that placental IL-6 signaling, specifically in the trophoblast, is required for MIA-induced acute immune activation in the fetal brain and subsequent detrimental effects on offspring neurodevelopment, at least in rodents. The authors of this study proposed three different ways placental IL-6 signaling might impact the fetal brain. First, they proposed that the placenta may initiate a feed-forward cycle of IL-6 induction in the embryo. Second, they proposed effects of placental IL-6 signaling on the fetal brain might be mediated by changes in placental hormones including prolactin and corticotrophin-releasing factor (CRF). Finally, they suggested that IL-6 might induce trophoblasts to produce factors that increase vascular permeability in the placenta, thereby altering the metabolic and nutritional environment of the fetus. An earlier study by the same group revealed another placental hormone system disrupted by poly(I:C) MIA – the growth hormone-insulin-like growth factor (GH-IGF) axis. Levels of growth hormone (GH), insulin like growth factor 1 (IGF1), and insulin like growth factor binding protein 3 (IGFBP3) levels were all reduced following MIA (Hsiao and Patterson, 2011). More recently, Monteiro et al. (2022) reported that mid-pregnancy MIA [poly(I:C)] alters expression of placental ATP-Binding Cassette (ABC) efflux transporters, which transport a variety of substances including cholesterol, drugs, xenobiotics, and cytokines across the placental barrier (Monteiro et al., 2022). While this data is clearly of interest, it should be noted that there are substantial differences between the most frequently used rodent models and human placentas, which make it difficult to extrapolate directly from mice and rats to human. Schmidt et al. (2015) and Carter (2020) review several key differences in anatomy including (1) in human placenta maternal blood perfuses the intervillous space, while in mice and rats exchange of material is between fetal and maternal capillaries, (2) humans do not have an inverted yolk sac placenta in addition to the chorioallantoic placenta, while mice and rats do, (3) mice and rats have trichorial placentas while humans have monochorial placentas, and (4) in humans there is deep interstitial and endovascular invasion of trophoblast cells into the inner third of the human myometrium, while in mice the invasion is restricted to the decidua basalis (Schmidt et al., 2015; Carter, 2020). The latter difference is particularly problematic when studying preeclampsia. There are also important species differences in placental endocrinology, molecular features, and immune responses. For example, the human placenta can actively transport protective immunoglobulin IgG antibodies to the fetus during gestation, while rodents do not transport IgG as efficiently and mice acquire maternal IgG antibodies via yolk sac–derived cells and after birth via suckling (reviewed in Ander et al., 2019). In addition, the human placenta secrets primate-specific antiviral microRNAs (miRNAs) from a cluster on chromosome 19 (C19MC) from syncytiotrophoblast layer that broadly restrict viral infections while mouse placenta uses interferons to restrict viral infections (Ander et al., 2019). To overcome this translational challenge, additional studies using in vitro models and alternative animal models are needed (Schmidt et al., 2015; Ander et al., 2019; Carter, 2020).
It is well established that viral infection increases the production of proinflammatory cytokines which in turn activate the HPA axis, resulting in increased glucocorticoid production (reviewed in Silverman et al., 2005; Raony et al., 2020). When the maternal HPA axis is activated, levels of glucocorticoids in maternal blood increase. Glucocorticoids can cross the placental barrier thereby increasing fetal glucocorticoid levels. Also, maternal cytokines can cross the placenta and activate the fetal HPA axis and stimulate the release of corticotrophin releasing hormone (CRH). This subsequently would stimulate secretion of adrenocorticotrophic hormone (ACTH) from the fetal anterior pituitary and glucocorticoids from the fetal adrenals (reviewed in Seckl, 2004; Ratnayake et al., 2013). Glucocorticoids play a central role in the fetal programming of HPA function (reviewed in Kapoor et al., 2006). Exposure to high levels of glucocorticoids in utero can manifest as disrupted HPA axis reactivity in later life and may underlie cognitive deficits and addictive behaviors in childhood and adulthood (reviewed in Waffarn and Davis, 2012; Moisiadis and Matthews, 2014; Granja et al., 2021).
In a recent review article, Granja et al. (2021) proposed a possible mechanism by which SARS-CoV-2 infection in a pregnant woman may disrupt fetal brain development via interference with the HPA axis. They explain that during a normal pregnancy, levels of 11β-HSD2 (glucocorticoid inactivating hormone) increase to ensure the appropriate exposure of glucocorticoids to the fetus. At the same time progesterone levels are also increasing to counter the cytokine balance toward an anti-inflammatory profile at the maternal-fetal interface (Granja et al., 2021). They hypothesize that viral infection (e.g., SARS-CoV-2 infection) may disrupt placental 11β-HSD2 expression resulting in increased exposure of the fetus to glucocorticoids (Granja et al., 2021). We are unaware of any studies directly testing Granja et al’s hypothesis, but there is a growing body of literature on the immune environment of the human placenta during COVID-19 infection. Lu-Culligan et al. (2021) reported robust inflammatory responses in placenta tissue from third trimester COVID-19 infections including increased expression of pro-inflammatory genes and chemokines, revealed by single-cell transcriptomic profiling (Lu-Culligan et al., 2021). In contrast, Juttukonda et al. (2022) have reported that while decidual tissue from individuals with third trimester COVID-19 infections have increased macrophages, NK cells, and T cells, levels of IL-8 are reduced compared to controls and levels of IFN-γ, IL-1β, IL-6, IL-10, and TNF do not differ (Juttukonda et al., 2022). In the same study, decidual tissue from individuals with second trimester infections showed a significant decrease in IL-6, IL-8, IL-10, and TNF-α and no change in abundance for IL-1β or IFN-γ (Juttukonda et al., 2022). Bordt et al. (2021) reported increased levels of IFN-α, IFN-γ, and IL-10 in placentas from individuals with third trimester infections, but only in males (Bordt et al., 2021). Thus, there is still much to be done in terms of understanding how maternal COVID-19 impacts inflammatory profiles of the placenta and a dearth of studies on how this might impact glucocorticoids.
Maternal glucocorticoids can reach fetal brain and bind with glucocorticoid receptors (GR) to exert detrimental effects (reviewed in Miranda and Sousa, 2018). In fact, disrupted placental 11β-HSD2 expression, may lead to abnormal glucocorticoid receptor (GR) expression in hippocampus and amygdala, leading to a hyperreactive HPA axis and increased anxiety-like behaviors in adult rat offspring (Welberg et al., 2000). Furthermore, higher glucocorticoid exposure to the fetus increases inducible nerve growth factor A and activates transcriptional activity thereby disrupting fetal brain development (Andrews et al., 2004) (guinea pig). In addition to these molecular changes, fetal exposure to high levels of glucocorticoids also impacts neurogenesis in both rodent and human in vitro models and changes hippocampal structure (Gould et al., 1992; Lemaire et al., 2000; Provençal et al., 2020). Similarly, higher levels of glucocorticoid may alter microglial function (reviewed in Walker et al., 2013), disrupting synaptogenesis, neurogenesis, synaptic pruning, axonal growth, myelination and astrocyte maturation (reviewed in Harry and Kraft, 2012; Schafer and Stevens, 2015).
It is reasonable to hypothesize that MIA in the context of SARS-CoV-2 would produce similar effects on the HPA axis which may increase risk for behavioral problems in offspring as shown in Figure 4A. Indeed, SARS-CoV-2 infected patients may experience a ‘cytokine storm’ leading to excessive glucocorticoids that may have deleterious effects on the host (Figure 4A) (Silverman et al., 2005; Raony et al., 2020). On the other hand, there is also evidence to suggest that SARS-associated coronaviruses can produce hypocortisolism (Figure 4B) (Leow et al., 2005). In a prospective cohort study of SARS-CoV survivors, 24 of 61 patients developed HPA axis dysfunction resulting in reduced blood cortisol levels during the 3-month follow-up period (Leow et al., 2005). A published case report suggests that SARS-CoV-2 infection can also produce hypocortisolism; a 69-year-old Iranian man, admitted to the ICU with SARS-CoV-2, developed adrenal insufficiency and reduced serum cortisol level (Heidarpour et al., 2020). Two mechanisms have been proposed for the association of SARS-associated coronaviruses with hypocortisolism: (1) destruction of ACTH due to infection and (2) damage to the hypothalamus. Regarding the first potential mechanism, in 2004, Wheatland proposed the molecular mimicry theory of ACTH in SARS. This theory is based on the observation that SARS-CoV expresses certain amino acid sequences that mimic the adrenocorticotropic hormone (ACTH). Thus, antibodies produced by the host in response to SARS-CoV may also destroy host ACTH thereby reducing the patient’s cortisol level (Wheatland, 2004). Regarding the second potential mechanism, the authors of the prospective cohort study of SARS-CoV survivors discussed above proposed in their discussion that SARS-CoV-2 infection could damage the hypothalamus and/or pituitary via the ACE2 receptor or CD209L/L-SIGN, a C-type lectin surface glycoprotein implicated in viral pathogenesis, leading to HPA axis dysfunction and hypocortisolism (Leow et al., 2005). Support for this mechanism has recently been provided by a French group, who reported that ACE2 and the transmembrane proteinase, serine 2 (TMPRSS2), which cleaves the SARS-CoV-2 spike protein, are expressed in the adult human hypothalamus, with the paraventricular nucleus showing the highest expression among hypothalamic nuclei. Interestingly, a KEGG pathway enrichment analysis suggested that both ACE2 and TMPRSS2 play important roles in the “neuroactive ligand-receptor interaction” pathway supporting an impact of SARS-CoV-2 on neuroendocrine function, including interactions between corticotropin releasing hormone and its receptor. Furthermore, they report that viral markers for SARS-CoV-2 were abundant in the hypothalamus of a 63-year-old male patient who died of COVID, but absent from the hypothalamus of controls (Nampoothiri et al., 2020). Very little work has been done on the possible neurodevelopmental consequences of low cortisol levels during pregnancy, but pregnancy is typically accompanied by substantial increases in cortisol (Mastorakos and Ilias, 2003; Jensen et al., 2011; Guardino et al., 2016). Several studies, conducted in sheep, indicate that lowering maternal cortisol during pregnancy alters placental morphology and reduces placental and uterine blood flow, which could result in restricted fetal growth (Jensen et al., 2005, 2007) and altered neurodevelopment, as previously discussed. Overall, depending on the timing of SARS-CoV-2 infection, the developing fetus may be exposed to both abnormally high and abnormally low levels of cortisol with potential consequences for neurodevelopment.
Figure 4. The relationship between maternal SARS-CoV-2 infection and Fetal HPA axis modulation, (A) SARS-CoV-2 infection and hypercortisolism, (B) SARS-CoV-2 infection and hypocortisolism.
Accumulating evidence suggests that maternal infection during gestation may affect intergenerational and transgenerational offspring neurodevelopmental process via epigenetic modifications (reviewed in Kleeman et al., 2022). Epigenetic processes produce long term and heritable modifications in gene expression without changing the DNA sequence (reviewed in Bale, 2015). These processes include DNA methylation, histone modification, and expression of microRNA (miRNA) (reviewed in Bale, 2015; Szyf, 2015; Weber-Stadlbauer, 2017). Epigenetic processes play a critical role in linking early environmental experiences to long-term changes in brains structure and function and are likely to play a key role in explaining the impact of maternal infection on brain development as described in subsequent paragraphs (reviewed in Bale, 2015; Dubey et al., 2018; Bergdolt and Dunaevsky, 2019).
DNA methylation involves the attachment of a methyl (CH3) group to cytosines within the DNA sequence, a reaction that requires both methyltransferases, such as DNA cytosine-5-methyltransferase 1 (DNMT1), and methyl donors, which are derived from nutrients such as folate. Methylated DNA attracts methyl binding proteins, such as methyl CpG binding protein 2 (MeCP2), that condense the structure of the nucleosome, thereby preventing transcription. A growing body of research suggests that infection during pregnancy alters DNA methylation in the offspring brain in ways that are both complex and region-specific (Richetto et al., 2017b). Richetto and colleagues observed that MIA following treatment with the viral mimetic Poly(I:C) altered DNA methylation in the medial prefrontal cortex of adult offspring. Adult offspring of immune activated mothers showed hyper or hypomethylation of CpGs at various loci including loci influencing GABAergic differentiation and signaling (Dlx1, Lhx5, Lhx8), Wnt signaling (Wnt3, Wnt7b, Wnt8a), neural development (Efnb3, Mid1, Nlgn1, Nrxn2) (Richetto et al., 2017b), and myelination related gene α-myelin-associated oligodendrocytic basic protein (mobp) in prefrontal cortex and nucleus accumbens of adult offspring (Richetto et al., 2017a), suggesting that epigenetic modification might mediate the impact of prenatal infections on offspring behavior (Richetto et al., 2017a,b). Labouesse and colleagues have also investigated Poly(I:C) induced MIA impacts on prefrontal cortex in mice and found increased methylation of promotors for GAD1 and GAD2, key enzymes in GABA synthesis (Labouesse et al., 2015). Poly(I:C) induced MIA also induces significant global DNA hypomethylation in the hippocampus, including in the promotor of Mecp2, but not in the mice striatum (Basil et al., 2014), as well as increased expression of DNase I hypersensitivity sites (DHSs) and MECP2 binding sites genes, namely Abat and Gnas9 in mice hypothalamus (Basil et al., 2018). In another study, poly(I:C) induced MIA increased methylation of the promoter region of tyrosine hydroxylase (Th) gene in the dopaminergic neurons of ventral midbrain in adult mice (Weber-Stadlbauer et al., 2021). All the above-mentioned studies used Poly (I:C) induced MIA model, because it is widely applied to study neurodevelopment (reviewed in Bao et al., 2022). Further, Poly (I:C) induced MIA are mainly driven via IL-6 activity (reviewed in Bao et al., 2022). IL-6 may provide a mechanistic link between infection and altered DNA methylation as it promotes nuclear translocation of DNMT1, the major enzyme responsible for maintaining methylation patterns following DNA replication (Hodge et al., 2007). Further, alterated or defective DNA replication may lead to impaired neuronal development (Kalogeropoulou et al., 2019).
Histone modifications are post translational modifications of histone protein (Szyf, 2015). There can be hundreds of modifications on a given histone producing a cumulative effect on how DNA around that histone is packaged. The addition or removal of acetyl groups is one biochemical process known to be important for transcriptional regulation and is accomplished by two types of enzymes: histone acetyltransferases (HATs) and histone deacetylases (HDACs). HATs promote DNA histone acetylation, which loosens the chromatin and facilitates gene transcription, while HDACs remove acetyl groups, which condenses the chromatin and reduces gene transcription (reviewed in Dubey et al., 2018). LPS-induced inflammation increases expression of HDAC2 and HDAC5 in the brain, an effect that can be attenuated by treating with an HDAC inhibitor prior to administering LPS. Moreover, pre-treatment with an HDAC inhibitor diminished LPS-induced anhedonia, anorexia, and microglia activation, supporting histone deacetylation as a key mechanism linking systemic inflammation to cognitive dysfunction in adult and juvenile animals (Hsing et al., 2015). Another study in mice suggests that MIA [Poly(I:C)] regulates hippocampal serotonin transporter (SERT) levels via modulation of histone acetylation which results in anhedonic behavior in offspring (Reisinger et al., 2016). Specific cytokines linked to histone modification include TNF-alpha, which increases histone acetylation in human alveolar epithelial cells (Rahman et al., 2002) and IL-17a, which reduces HDAC2 activity via the PI3K pathway in human bronchial epithelial cells (Zijlstra et al., 2012). Whether these cytokines have similar effects in the developing brain is currently unknown. In one study, MIA [Poly(I:C)] during pregnancy did not appear to alter histone modification in cerebral cortex of adult offspring, although activation of cytokine signaling in primary cultures from fetal forebrain influenced trimethylated histone H3-lysine 4 (H3K4me3) marks in a limited set of genes (Connor et al., 2012). In contrast, Tang et al. (2013), observed that MIA [Poly(I:C)] leads to global hypoacetylation of histone H3 at H3K9K14 and H4K8 in the cortex of juvenile mice. This was accompanied by reduced expression of genes involved in neuronal development, synaptic transmission, and immune signaling. Specific genes exhibiting hypoacetylation included Robo1, which is involved in axon guidance and neuronal precursor cell migration, arhgap18, and Ntrk3, which is likely involved with cell survival and differentiation in the nervous system. Hyperacetylation was also observed at specific loci in the hippocampus of juvenile mice including Disc1, which is involved in many aspects of nervous system development, Nr2f1, Ntrk3, which is a transcriptional regulator, and Gria1 and Gria2, which are both subunits of AMPA-type ionotropic glutamate receptors (Tang et al., 2013). Further, glutamate activities may modulate the early brain developmental process (Tanaka, 2013). Another, mouse study suggested that prenatal poly (I:C) exposure at late gestation (embryonic day 17) leads to deficits in working memory of the adult animal due to altered histone H3K4me3 methylation in approx. 30 genes, including Disc1 (Connor et al., 2012).
MicroRNA (miRNA) are small endogenous non-coding RNAs involved in post-transcriptional gene regulation (Ha and Kim, 2014; Weber-Stadlbauer, 2017). They target most protein coding transcription and prevent the production of specific proteins by binding to and destroying messenger RNA (mRNA). miRNA are highly expressed in brain and essential for brain development and neuronal function (Petri et al., 2014). Several recent studies demonstrate an impact of MIA on miRNA expression in offspring brain. Sunwoo et al. (2018) have reported that MIA [Poly(I:C)] alters brain miRNA expression in offspring at 3 weeks of age, a time when both synaptogenesis and myelination are ongoing. 8 miRNA were upregulated and 21 were downregulated. Furthermore, target genes of 18 downregulated and 3 upregulated miRNA were found to be significantly enriched among differentially expressed genes, confirming that MIA induced alterations in miRNA have functional consequences, at least at the level of gene expression. Offspring exhibited behavioral changes typical of MIA including lack of a preference for social novelty and reduced prepulse inhibition, but the study design did not address whether miRNA alterations played a causal role in the behavioral abnormalities (Sunwoo et al., 2018). Berger et al. examined how MIA [Poly(I:C)] affected 13 specific miRNA in offspring hippocampus and observed increased levels of miR-15b-2, miR-98-1, miR-103-2, and miR-124-1. None of these overlap with the differentially expressed miRNA in Sunwoo et al., indicating that additional studies are needed to clarify which miRNA are most robustly associated with MIA. Interestingly, MIA also influenced MiRNA expression in F2 generations of offspring, along the paternal line. The specific miRNA involved differed from observations in the F1 generation. This and the modest magnitude of changes led the authors to conclude that miRNA did not play a substantial role in the behavioral impacts of MIA (Berger et al., 2018).
With specific regard to SARS-CoV-2, no studies have examined miRNA levels in the brains of exposed fetuses. However, a recently published cohort study indicated that various miRNAs are upregulated in both plasma and placental tissues of pregnant mothers infected with SARS-CoV-2 (Saulle et al., 2021). 35 miRNA were differentially expressed in human plasma including seven antiviral miRNAs (miR-21, miR-23b, miR-28, miR-29a, miR-29c, miR-98 and miR-326) and six immunomodulatory miRNAs (miR-17, miR-92, miR-146, miR-150, miR-155, miR-223), all upregulated in infected mothers. In placenta, eight miRNA were upregulated in the context of maternal infection including ones with direct effects on viral replication (miR-21b, miR-29c, miR-98) and ones influencing viral replication by indirect mechanisms (miR-146, miR-155, miR-190, miR-346, and miR-326) (Saulle et al., 2021). Further, an in silico study showed that miR-21, miR-16 and miR-146a have high affinity to the SARS-CoV-2 virus (Jafarinejad-Farsangi et al., 2020).
Interestingly, several of these miRNAs have also been implicated in neurodevelopment. miR-146a is one of the most commonly dysregulated miRNAs in neurodevelopmental disorders (Fregeac et al., 2016; Nguyen et al., 2016, 2018; Schepici et al., 2019). In H9 human neural stem cells, miR-146a overexpression enhances neurite outgrowth and branching and favors differentiation into neuronal like cells (Nguyen et al., 2018). In mouse primary cell cultures, miR-146a overexpression leads to impaired neuronal dendritic arborization and increased astrocyte glutamate uptake capacities (Nguyen et al., 2016). miR-146a is highly expressed in mouse hippocampus, amygdala and entorhinal cortex, areas with important roles in social cognition, memory, spatial navigation, and the perception of time, and targets genes with known roles in neurodevelopment including MAP1B, FMR1, and KCNK2 (Nguyen et al., 2016). Furthermore, miR-146a overexpression promotes oligodendrocyte differentiation and myelination in the context of neurological injury (Santra et al., 2014; Liu et al., 2017; Zhang et al., 2017, 2019), raising the possibility that it also effects these processes during neurodevelopment. In addition, downregulation of miRNA-146a expression in mice leads to impaired neurogenesis, abnormal brain anatomy, along with deficits in working and spatial memory (Fregeac et al., 2020). Apart from miR-146a, miR-21, miR-146b, miR-23a, miR-23b, miR-92(a1-a2) and miR-23a-3p have been reported to differentiate between controls and individuals with ASD in peripheral tissues, e.g., lymphoblastoid cell lines and saliva (Talebizadeh et al., 2008; Sehovic et al., 2020; Frye et al., 2021), while miR-21-3p is overexpressed in the cortex of post-mortem ASD patients (Wu et al., 2016). These miRNAs have been less thoroughly studied than MiR-146a in terms of their roles in neurodevelopment, but miR-21 expression in the placenta is associated with fetal growth (Maccani et al., 2011), which could explain the association of maternal SARS-CoV-2 infection with intrauterine growth restriction, a known risk factor for altered neurodevelopment. miR-23 regulates progenitor fate decisions by inhibiting cyclin D1 mRNA. Inhibition of miR-23 increases cyclin D1 protein in mouse progenitor cells leading to reduced neuronal differentiation during cortical neurogenesis (Ghosh et al., 2014). miR-21-3p downregulates multiple genes in a specific gene co-expression module enriched for ASD risk genes in post-mortem human brain (Wu et al., 2016). This module is upregulated in early cortical development and is enriched for genes implicated in neural development and synaptic function (Parikshak et al., 2013). In addition, miR-21-3p over-expression led to a pronounced decrease in the PCDH19 gene (Wu et al., 2016), which encodes a cell-adhesion protein primarily expressed in the brain. Mutations in PCDH19 are associated with both epilepsy and ASD (Redies et al., 2012).
Overall, maternal immune activation can affect multiple epigenetic processes in the developing brain leading to long-lasting behavioral changes in offspring as summarized in Figure 5. Readers interested in additional details may find the following reviews of interest: (Woods et al., 2021; Kleeman et al., 2022). The latter provides a systematic review of MIA-induced changes in gene expression and epigenetic features. Additional research is needed to study these relationships in the context of maternal SARS-CoV-2.
Figure 5. Potential epigenetic mechanisms linking prenatal SARS-CoV-2 infection to offspring brain development.
The information presented thus far strongly suggests that gestational SARS-CoV-2 infection could alter fetal neurodevelopment. Empirical evidence supporting this hypothesis is currently sparse and somewhat inconsistent, but intriguing. A preliminary study conducted in 2020 (N = 57) reported that a substantial proportion of children born to mothers infected with SARS-CoV-2 during gestation were identified as high risk for social and emotional problems at 3 months of age using the Ages and Stages Questionnaire: Social-Emotional, second edition (ASQ:SE-2) (63.6%) (Wang et al., 2020). In contrast, the proportion of children identified as high risk using the Ages and Stages Questionnaires, third edition (ASQ-3) was lower – 0% for communication and gross motor skills, 5.8% for fine motor skills and problem solving, and 9.6% for personal-social skills. No control group was included for comparison. Interestingly, gross motor, problem solving, personal–social, and social–emotional were negatively linked with the amount of time mothers and babies were separated after birth. More recent studies with larger sample sizes provide additional insights (Aldrete-Cortez et al., 2022; Ayed et al., 2022; Edlow et al., 2022). A prospective cohort study conducted in Kuwait (N = 298) reported developmental delays in around 10% of infants whose mothers had COVID-19 during pregnancy using the ASQ-3 (Ayed et al., 2022), which is similar to rates of developmental delay in healthy children in a similar geographical and cultural setting (Charafeddine et al., 2019). The ASQ:SE-2 was not included in this study. Risk of developmental delay was significantly higher in infants born to mothers infected during the first and second trimester than mothers infected in the third trimester, suggesting that adverse neurodevelopmental consequences of SARS-CoV-2 may be time-specific (Ayed et al., 2022). Key neurodevelopmental events occurring in the first and second trimesters include formation of the neural tube and neurogenesis (Stiles and Jernigan, 2010). A study of 254 infants born in New York City during the pandemic (114 exposed to COVID-19 in utero and 141 unexposed) and 62 infants born before the pandemic, revealed that birth during the pandemic was associated with significantly lower scores on gross motor, fine motor, and personal-social skills, with no significant differences between the exposed and unexposed groups (Shuffrey et al., 2022). This suggests that COVID-19-related stress may have a stronger impact on neurodevelopment than gestational exposure to SARS-CoV-2. Relatively few women had confirmed exposures in early pregnancy, but post hoc analyses suggested exposure in the first trimester might have adverse neurodevelopmental consequences, similar to the earlier Ayed study. The above studies all relied on the ASQ-3, a parent-response instrument used to screen for developmental delays, as an index of early neurodevelopment. Aldrete-Cortez et al. took a different approach using observations of early motor repertoires as their outcome. They showed that at 3-5 months of age, a motor optimality score was significantly lower in infants prenatally exposed to SARS-CoV-2 than unexposed controls, suggesting they are at higher risk for later neurological disorders (Aldrete-Cortez et al., 2022). Finally, Edlow et al. used electronic health records and a retrospective cohort design to test associations between SARS-CoV-2 exposure in utero and risk for neurodevelopmental disorders in the first year of offspring life (Edlow et al., 2022). This cohort included 7772 live births of which 222 were to SARS-Cov-2 positive mothers. Maternal SARS-CoV-2 positivity during pregnancy was associated with greater rate of neurodevelopmental diagnoses in both unadjusted models and models adjusted for race, ethnicity, insurance status, offspring sex, maternal age, and preterm status. In contrast to studies using the ASQ-3, third-trimester exposures appeared to confer greater risk. Finally, a recent meta-analysis suggested that infants born during the SARS-CoV-2 pandemic had similar rates of neurodevelopmental issues compared to those born before the pandemic, regardless of whether they were exposed in utero. However, communication impairments were more common in children born during the pandemic and fine motor deficits were more common in those with in utero exposure (Hessami et al., 2022). Overall, emerging evidence suggests maternal SARS-CoV-2 infection may impact neurodevelopment but is subject to several limitations. Sample sizes are relatively small, follow-up has been limited to the first year of life, and most studies have relied on a single parent-report instrument designed to screen for developmental delays. Thus, there is an urgent need for long term follow up in infants born during the pandemic.
Infection of pregnant women with SARS-CoV-2 could influence fetal brain development, potentially increasing risk for later cognitive and behavioral problems. SARS-CoV-2 infection can be transferred transplacentally from an infected mother to her fetus and the virus does have neuroinvasive potential. However, this appears to be a relatively rare event. Consequently, we anticipate that long-term neurodevelopmental effects, if they occur, are more likely to reflect indirect mechanisms. Like other viruses, SARS-CoV-2 infection may trigger maternal immune activation, which may disrupt fetal neurodevelopmental and lead to long term cognitive and motor deficits, behavioral abnormalities, and, potentially, psychiatric illness in children. SARS-CoV-2 may also trigger preeclampsia, preterm birth, and/or intrauterine growth restriction, which are known risk factors for later neurodevelopmental issues. Key finding related to SARS-CoV-2 infection are summarized in Table 1. Given the high numbers of pregnant women that have been, and will be, exposed during this pandemic, the long-term impact of SARS-CoV-2 on fetal brain development needs to be investigated.
HD conceived the idea for the manuscript and wrote the first draft. RS, SK, and RK contributed to manuscript revision. All authors read and approved the submitted version.
The authors declare that this review was conducted in the absence of any commercial or financial relationships that could be construed as a potential conflict of interest.
All claims expressed in this article are solely those of the authors and do not necessarily represent those of their affiliated organizations, or those of the publisher, the editors and the reviewers. Any product that may be evaluated in this article, or claim that may be made by its manufacturer, is not guaranteed or endorsed by the publisher.
Aaltonen, R., Heikkinen, T., Hakala, K., Laine, K., and Alanen, A. (2005). Transfer of proinflammatory cytokines across term placenta. Obstet. Gynecol. 106:84837. doi: 10.1097/01.AOG.0000178750.84837.ed
Adams Waldorf, K. M., and McAdams, R. M. (2013). Influence of infection during pregnancy on fetal development. Reproduction 146:232. doi: 10.1530/REP-13-0232
Aldrete-Cortez, V. I., Bobadilla, L., Tafoya, I. S. A., Gonzalez-Carpinteiro, A., Nava, F., Viñals, C., et al. (2022). Infants prenatally exposed to SARS-CoV-2 show the absence of fidgety movements and are at higher risk for neurological disorders: a comparative study. PLoS One 17:e0267575. doi: 10.1371/journal.pone.0267575
Allotey, J., Chatterjee, S., Kew, T., Gaetano, A., Stallings, E., Fernández-García, S., et al. (2022). SARS-CoV-2 positivity in offspring and timing of mother-to-child transmission: living systematic review and meta-analysis. BMJ 376:67686. doi: 10.1136/BMJ-2021-067696
Allotey, J., Stallings, E., Bonet, M., Yap, M., Chatterjee, S., Kew, T., et al. (2020). Clinical manifestations, risk factors, and maternal and perinatal outcomes of coronavirus disease 2019 in pregnancy: living systematic review and meta-analysis. BMJ 370:3320. doi: 10.1136/bmj.m3320
Alzamora, M. C., Paredes, T., Caceres, D., Webb, C. M., Webb, C. M., Valdez, L. M., et al. (2020). Severe COVID-19 during pregnancy and possible vertical transmission. Am. J. Perinatol. 37:10050. doi: 10.1055/s-0040-1710050
Ander, S. E., Diamond, M. S., and Coyne, C. B. (2019). Immune responses at the maternal-fetal interface. Sci. Immunol. 4:6114. doi: 10.1126/sciimmunol.aat6114
Andrews, M. H., Kostaki, A., Setiawan, E., McCabe, L., Owen, D., Banjanin, S., et al. (2004). Developmental regulation of the 5-HT7 serotonin receptor and transcription factor NGFI-A in the fetal guinea-pig limbic system: influence of GCs. J. Physiol. 555:56705. doi: 10.1113/jphysiol.2003.056705
Anton, L., and Brosnihan, K. B. (2008). Systemic and uteroplacental renin—angiotensin system in normal and pre-eclamptic pregnancies. Ther. Adv. Cardiovasc. Dis. 2:529. doi: 10.1177/1753944708094529
Anton, L., Merrill, D. C., Neves, L. A. A., Diz, D. I., Corthorn, J., Valdes, G., et al. (2009). The uterine placental bed renin-angiotensin system in normal and preeclamptic pregnancy. Endocrinology 150:76. doi: 10.1210/en.2009-0076
Arnone, D., Cavanagh, J., Gerber, D., Lawrie, S. M., Ebmeier, K. P., and McIntosh, A. M. (2009). Magnetic resonance imaging studies in bipolar disorder and schizophrenia: meta-analysis. Br. J. of Psychiatry 195:9717. doi: 10.1192/bjp.bp.108.059717
Atladóttir, H., Thorsen, P., Østergaard, L., Schendel, D. E., Lemcke, S., Abdallah, M., et al. (2010). Maternal infection requiring hospitalization during pregnancy and autism spectrum disorders. J. Autism Dev. Dis. 40:1006. doi: 10.1007/s10803-010-1006-y
Ayed, M., Embaireeg, A., Kartam, M., More, K., Alqallaf, M., Alnafisi, A., et al. (2022). Neurodevelopmental outcomes of infants born to mothers with SARS-CoV-2 infections during pregnancy: a national prospective study in Kuwait. BMC Pediatr. 22:319. doi: 10.1186/s12887-022-03359-2
Bahadur, G., Homburg, R., Yoong, W., Singh, C., Bhat, M., Kotabagi, P., et al. (2020). Adverse outcomes in SAR-CoV-2 (COVID-19) and SARS virus related pregnancies with probable vertical transmission. J. Bras Reprod Assist 24:57. doi: 10.5935/1518-0557.20200057
Bale, T. L. (2015). Epigenetic and transgenerational reprogramming of brain development. Nat. Rev. Neurosci. 16:3818. doi: 10.1038/nrn3818
Bao, M., Hofsink, N., and Plösch, T. (2022). LPS vs. poly I:C model: comparison of long-term effects of bacterial and viral maternal immune activation (MIA) on the offspring. Am. J. Physiol. Regul. Int. Comput. Physiol. 322:2021. doi: 10.1152/AJPREGU.00087.2021
Barr, C. E., Mednick, S. A., and Munk Jorgensen, P. (1990). Exposure to influenza epidemics during gestation and adult schizophrenia: a 40-year study. Arch. Gen. Psychiatry 47:7012. doi: 10.1001/archpsyc.1990.01810210077012
Basil, P., Li, Q., Dempster, E. L., Mill, J., Sham, P. C., Wong, C. C. Y., et al. (2014). Prenatal maternal immune activation causes epigenetic differences in adolescent mouse brain. Trans. Psychiatry 4:80. doi: 10.1038/tp.2014.80
Basil, P., Li, Q., Gui, H., Hui, T. C. K., Ling, V. H. M., Wong, C. C. Y., et al. (2018). Prenatal immune activation alters the adult neural epigenome but can be partly stabilised by a n-3 polyunsaturated fatty acid diet. Trans. Psychiatry 8:167. doi: 10.1038/s41398-018-0167-x
Bergdolt, L., and Dunaevsky, A. (2019). Brain changes in a maternal immune activation model of neurodevelopmental brain disorders. Prog. Neurobiol. 175:2. doi: 10.1016/j.pneurobio.2018.12.002
Berger, S., Ronovsky, M., Horvath, O., Berger, A., and Pollak, D. D. (2018). Impact of maternal immune activation on maternal care behavior, offspring emotionality and intergenerational transmission in C3H/He mice. Brain Behav. Immun. 70:8. doi: 10.1016/j.bbi.2018.02.008
Berkiks, I., Garcia-Segura, L. M., Nassiri, A., Mesfioui, A., Ouichou, A., Boulbaroud, S., et al. (2019). The sex differences of the behavior response to early Life immune stimulation: Microglia and astrocytes involvement. Physiol Behav 199, 37. doi: 10.1016/j.physbeh.2018.11.037
Boedhoe, P. S. W., van Rooij, D., Hoogman, M., Twisk, J. W. R., Schmaal, L., Abe, Y., et al. (2020). Subcortical brain volume, regional cortical thickness, and cortical surface area across disorders: findings from the ENIGMA ADHD, ASD, and OCD working groups. Am. J. Psychiatry 177:331. doi: 10.1176/appi.ajp.2020.19030331
Boksa, P. (2010). Effects of prenatal infection on brain development and behavior: a review of findings from animal models. Brain Behav. Immun. 24:5. doi: 10.1016/j.bbi.2010.03.005
Bordt, E. A., Shook, L. L., Atyeo, C., Pullen, K. M., de Guzman, R. M., Meinsohn, M. C., et al. (2021). Maternal SARS-CoV-2 infection elicits sexually dimorphic placental immune responses. Sci. Trans. Med. 13:428. doi: 10.1126/SCITRANSLMED.ABI7428
Borsini, A., Zunszain, P. A., Thuret, S., and Pariante, C. M. (2015). The role of inflammatory cytokines as key modulators of neurogenesis. Trends Neurosci. 38:6. doi: 10.1016/j.tins.2014.12.006
Bostancıklıoğlu, M. (2020). SARS-CoV2 entry and spread in the lymphatic drainage system of the brain. Brain Behav. Immun. 87:80. doi: 10.1016/j.bbi.2020.04.080
Boulanger-Bertolus, J., Pancaro, C., and Mashour, G. A. (2018). Increasing role of maternal immune activation in neurodevelopmental disorders. Front. Behav. Neurosci. 12:230. doi: 10.3389/fnbeh.2018.00230
Brosnihan, K. B., Neves, L. A. A., Anton, L., Joyner, J., Valdes, G., and Merrill, D. C. (2004). Enhanced expression of Ang-(1-7) during pregnancy. Brazili. J. Med. Biol. Res. 37:17. doi: 10.1590/S0100-879X2004000800017
Brown, A. S., Begg, M. D., Gravenstein, S., Schaefer, C. A., Wyatt, R. J., Bresnahan, M., et al. (2004a). Serologic evidence of prenatal influenza in the etiology of schizophrenia. Arch. Gen. Psychiatry 61:774. doi: 10.1001/archpsyc.61.8.774
Brown, A. S., Hooton, J., Schaefer, C. A., Zhang, H., Petkova, E., Babulas, V., et al. (2004b). Elevated maternal interleukin-8 levels and risk of schizophrenia in adult offspring. Am. J. Psychiatry 161:889. doi: 10.1176/appi.ajp.161.5.889
Brown, A. S., Cohen, P., Harkavy-Friedman, J., Babulas, V., Malaspina, D., Gorman, J. M., et al. (2001). Prenatal rubella, premorbid abnormalities, and adult schizophrenia. Biol. Psychiatry 49:1068. doi: 10.1016/S0006-3223(01)01068-X
Brown, A. S., Schaefer, C. A., Quesenberry, C. P., Liu, L., Babulas, V. P., and Susser, E. S. (2005). Maternal exposure to toxoplasmosis and risk of schizophrenia in adult offspring. Am. J. Psychiatry 162:767. doi: 10.1176/appi.ajp.162.4.767
Brown, A. S., Schaefer, C. A., Wyatt, R. J., Goetz, R., Begg, M. D., Gorman, J. M., et al. (2000). Maternal exposure to respiratory infections and adult schizophrenia spectrum disorders: a prospective birth cohort study. Schizophr Bull. 26:33453. doi: 10.1093/oxfordjournals.schbul.a033453
Brown, A. S., Sourander, A., Hinkka-Yli-Salomäki, S., McKeague, I. W., Sundvall, J., and Surcel, H. M. (2014). Elevated maternal C-reactive protein and autism in a national birth cohort. Mol. Psychiatry 19:197. doi: 10.1038/mp.2012.197
Buka, S. L., Tsuang, M. T., Torrey, E. F., Klebanoff, M. A., Wagner, R. L., and Yolken, R. H. (2001). Maternal cytokine levels during pregnancy and adult psychosis. Brain Behav. Immun. 15:644. doi: 10.1006/brbi.2001.0644
Burd, I., Balakrishnan, B., and Kannan, S. (2012). Models of fetal brain injury, intrauterine inflammation, and preterm birth. Am. J. Reproduct. Immunol. 67:1110. doi: 10.1111/j.1600-0897.2012.01110.x
Cai, Z., Lin, S., Pang, Y., and Rhodes, P. G. (2004). Brain injury induced by intracerebral injection of interleukin-1beta and tumor necrosis factor-alpha in the neonatal rat. Pediatr Res. 56:14. doi: 10.1203/01.PDR.0000134249.92944.14
Canales, C. P., Estes, M. L., Cichewicz, K., Angara, K., Aboubechara, J. P., Cameron, S., et al. (2021). Sequential perturbations to mouse corticogenesis following in utero maternal immune activation. Elife 10:60100. doi: 10.7554/eLife.60100
Careaga, M., Murai, T., and Bauman, M. D. (2017). Maternal immune activation and autism spectrum disorder: from rodents to nonhuman and human primates. Biol. Psychiatry 81:20. doi: 10.1016/j.biopsych.2016.10.020
Carter, A. M. (2020). Animal models of human pregnancy and placentation: alternatives to the mouse. Reproduction 160:354. doi: 10.1530/REP-20-0354
Charafeddine, L., Dani, A., Badr, L. K., Sinno, D., Tamim, H., Khoury, J., et al. (2019). The psychometric properties of the ages and stages questionnaires-3 in arabic: cross-sectional observational study. Early Hum. Dev. 136:10. doi: 10.1016/j.earlhumdev.2019.06.010
Chen, E., Xu, D., Lan, X., Jia, B., Sun, L., Zheng, J., et al. (2013). A novel role of the STAT3 pathway in brain inflammation-induced human neural progenitor cell differentiation. Curr. Mol. Med. 13:76. doi: 10.2174/15665240113139990076
Chen, W., Foo, S. S., Hong, E., Wu, C., Lee, W. S., Lee, S. A., et al. (2021). Zika virus NS3 protease induces bone morphogenetic protein-dependent brain calcification in human fetuses. Nat. Microbiol. 6:455. doi: 10.1038/S41564-020-00850-3
Cieślik, M., Gąssowska-Dobrowolska, M., Jêśko, H., Czapski, G. A., Wilkaniec, A., Zawadzka, A., et al. (2020). Maternal immune activation induces neuroinflammation and cortical synaptic deficits in the adolescent rat offspring. Int. J. Mol. Sci. 21:4097. doi: 10.3390/ijms21114097
Cieślik, M., Gassowska-Dobrowolska, M., Zawadzka, A., Frontczak-Baniewicz, M., Gewartowska, M., Dominiak, A., et al. (2021). The synaptic dysregulation in adolescent rats exposed to maternal immune activation. Front. Mol. Neurosci. 13:555290. doi: 10.3389/fnmol.2020.555290
Clarke, M. C., Tanskanen, A., Huttunen, M., Whittaker, J. C., and Cannon, M. (2009). Evidence for an interaction between familial liability and prenatal exposure to infection in the causation of schizophrenia. Am. J. Psychiatry 166:10031. doi: 10.1176/appi.ajp.2009.08010031
Connor, C. M., Dincer, A., Straubhaar, J., Galler, J. R., Houston, I. B., and Akbarian, S. (2012). Maternal immune activation alters behavior in adult offspring, with subtle changes in the cortical transcriptome and epigenome. Schizophr Res. 140:37. doi: 10.1016/j.schres.2012.06.037
Couch, A. C. M., Berger, T., Hanger, B., Matuleviciute, R., Srivastava, D. P., Thuret, S., et al. (2021). Maternal immune activation primes deficiencies in adult hippocampal neurogenesis. Brain Behav. Immun. 97:21. doi: 10.1016/j.bbi.2021.07.021
Dammann, O., Durum, S. K., and Leviton, A. (1999). Modification of the infection-associated risks of preterm birth and white matter damage in the preterm newborn by polymorphisms in the tumor necrosis factor-locus? Pathogenesis 1999:1.
Dang, D., Wang, L., Zhang, C., Li, Z., and Wu, H. (2020). Potential effects of SARS-CoV-2 infection during pregnancy on fetuses and newborns are worthy of attention. J. Obstet. Gynaecol. Res. 46:14406. doi: 10.1111/jog.14406
Dashraath, P., Wong, J. L. J., Lim, M. X. K., Lim, L. M., Li, S., Biswas, A., et al. (2020). Coronavirus disease 2019 (COVID-19) pandemic and pregnancy. Am. J. Obstet. Gynecol. 222:21. doi: 10.1016/j.ajog.2020.03.021
Desforges, M., Coupanec, A., Stodola, J. K., Meessen-Pinard, M., and Talbot, P. J. (2014). Human coronaviruses: viral and cellular factors involved in neuroinvasiveness and neuropathogenesis. Virus Res. 194:11. doi: 10.1016/j.virusres.2014.09.011
Deverman, B. E., and Patterson, P. H. (2009). Cytokines and CNS development. Neuron 64:2. doi: 10.1016/j.neuron.2009.09.002
di Mascio, D., Khalil, A., Saccone, G., Rizzo, G., Buca, D., Liberati, M., et al. (2020). Outcome of coronavirus spectrum infections (SARS, MERS, COVID-19) during pregnancy: a systematic review and meta-analysis. Am. J. Obstet. Gynecol. MFM 2:100107. doi: 10.1016/j.ajogmf.2020.100107
Ding, Y., He, L., Zhang, Q., Huang, Z., Che, X., Hou, J., et al. (2004). Organ distribution of severe acute respiratory syndrome (SARS) associated coronavirus (SARS-CoV) in SARS patients: implications for pathogenesis virus transmission pathways. J. Pathol. 203:1560. doi: 10.1002/path.1560
Dong, L., Tian, J., He, S., Zhu, C., Wang, J., Liu, C., et al. (2020). Possible vertical transmission of SARS-CoV-2 from an infected mother to her newborn. JAMA J. Am. Med. Assoc. 323:4621. doi: 10.1001/jama.2020.4621
Dubey, H., Gulati, K., and Ray, A. (2018). Recent studies on cellular and molecular mechanisms in Alzheimer’s disease: focus on epigenetic factors and histone deacetylase. Rev. Neurosci. 29, 241–260. doi: 10.1515/revneuro-2017-0049
Dubois, J., Benders, M., Borradori-Tolsa, C., Cachia, A., Lazeyras, F., Ha-Vinh Leuchter, R., et al. (2008). Primary cortical folding in the human newborn: an early marker of later functional development. Brain 131:137. doi: 10.1093/brain/awn137
Edlow, A. G., Castro, V. M., Shook, L. L., Kaimal, A. J., and Perlis, R. H. (2022). Neurodevelopmental outcomes at 1 year in infants of mothers who tested positive for SARS-CoV-2 during pregnancy key points. JAMA Netw Open. 5:2215787. doi: 10.1001/jamanetworkopen.2022.15787
Ellington, S., Strid, P., Tong, V. T., Woodworth, K., Galang, R. R., Zambrano, L. D., et al. (2020). Characteristics of women of reproductive age with laboratory-confirmed SARS-CoV-2 infection by pregnancy status — united states, january 22–june 7, 2020. MMWR Morb. Mortal. Wkly Rep. 69:6925. doi: 10.15585/mmwr.mm6925a1
Ellison-Wright, I., and Bullmore, E. (2010). Anatomy of bipolar disorder and schizophrenia: a meta-analysis. Schizophr Res. 117:22. doi: 10.1016/j.schres.2009.12.022
Ellul, M. A., Benjamin, L., Singh, B., Lant, S., Michael, B. D., Easton, A., et al. (2020). Neurological associations of COVID-19. Lancet Neurol. 19:30221. doi: 10.1016/S1474-4422(20)30221-0
Esposito, G., Pesce, M., Seguella, L., Sanseverino, W., Lu, J., and Sarnelli, G. (2020). Can the enteric nervous system be an alternative entrance door in SARS-CoV2 neuroinvasion? Brain Behav. Immun. 87:60. doi: 10.1016/j.bbi.2020.04.060
Estes, M. L., and McAllister, A. K. (2016). Maternal immune activation: implications for neuropsychiatric disorders. Science 1979:353. doi: 10.1126/science.aag3194
Figueiredo, C. P., Fontes-Dantas, F. L., da Poian, A. T., and Clarke, J. R. (2021). SARS-CoV-2-associated cytokine storm during pregnancy as a possible risk factor for neuropsychiatric disorder development in post-pandemic infants. Neuropharmacology 201:108841. doi: 10.1016/j.neuropharm.2021.108841
Fodoulian, L., Tuberosa, J., Rossier, D., Boillat, M., Kan, C., Pauli, V., et al. (2020). SARS-CoV-2 receptors and entry genes are expressed in the human olfactory neuroepithelium and brain. iScience 23:101839. doi: 10.1016/j.isci.2020.101839
Fregeac, J., Colleaux, L., and Nguyen, L. S. (2016). The emerging roles of MicroRNAs in autism spectrum disorders. Neurosci. Biobehav. Rev. 71:18. doi: 10.1016/j.neubiorev.2016.10.018
Fregeac, J., Moriceau, S., Poli, A., Nguyen, L. S., Oury, F., and Colleaux, L. (2020). Loss of the neurodevelopmental disease-associated gene miR-146a impairs neural progenitor differentiation and causes learning and memory deficits. Mol. Autism 11:328. doi: 10.1186/s13229-020-00328-3
Frye, R. E., Rose, S., McCullough, S., Bennuri, S. C., Porter-Gill, P. A., Dweep, H., et al. (2021). Microrna expression profiles in autism spectrum disorder: Role for mir-181 in immunomodulation. J. Pers. Med. 11:922. doi: 10.3390/jpm11090922
Ghadhanfar, E., Alsalem, A., Al-Kandari, S., Naser, J., Babiker, F., and Al-Bader, M. (2017). The role of ACE2, angiotensin-(1-7) and Mas1 receptor axis in glucocorticoid-induced intrauterine growth restriction. Reproduct. Biol. Endocrinol. 15:8. doi: 10.1186/s12958-017-0316-8
Ghosh, T., Aprea, J., Nardelli, J., Engel, H., Selinger, C., Mombereau, C., et al. (2014). MicroRNAs establish robustness and adaptability of a critical gene network to regulate progenitor fate decisions during cortical neurogenesis. Cell Rep. 7:29. doi: 10.1016/j.celrep.2014.05.029
Gooz, M. (2010). ADAM-17: the enzyme that does it all. Crit. Rev. Biochem. Mol. Biol. 45:15. doi: 10.3109/10409231003628015
Gould, E., Cameron, H. A., Daniels, D. C., Woolley, C. S., and McEwen, B. S. (1992). Adrenal hormones suppress cell division in the adult rat dentate gyrus. J. Neurosci. 12:1992. doi: 10.1523/jneurosci.12-09-03642.1992
Graham, A. M., Rasmussen, J. M., Rudolph, M. D., Heim, C. M., Gilmore, J. H., Styner, M., et al. (2018). Maternal systemic interleukin-6 during pregnancy is associated with newborn amygdala phenotypes and subsequent behavior at 2 years of age. Biol. Psychiatry 83:27. doi: 10.1016/j.biopsych.2017.05.027
Granja, M. G., Oliveira, A. C. D. R., de Figueiredo, C. S., Gomes, A. P., Ferreira, E. C., Giestal-De-Araujo, E., et al. (2021). SARS-CoV-2 infection in pregnant women: neuroimmune-endocrine changes at the maternal-fetal interface. Neuroimmunomodulation 28:556. doi: 10.1159/000515556
Gu, J., Gong, E., Zhang, B., Zheng, J., Gao, Z., Zhong, Y., et al. (2005). Multiple organ infection and the pathogenesis of SARS. J. Exp. Med. 202:828. doi: 10.1084/jem.20050828
Guardino, C. M., Schetter, C. D., Saxbe, D. E., Adam, E. K., Ramey, S. L., and Shalowitz, M. U. (2016). Diurnal salivary cortisol patterns prior to pregnancy predict infant birth weight. Health Psychol. 35:313. doi: 10.1037/hea0000313
Guma, E., Bordeleau, M., Ibañez, F. G., Picard, K., Snook, E., Desrosiers-Gregoire, G., et al. (2022). Differential effects of early or late exposure to prenatal maternal immune activation on mouse embryonic neurodevelopment. Proc. Natl. Acad. Sci. U.S.A. 119:5119. doi: 10.1073/pnas.2114545119
Guma, E., Bordignon, P., do, C., Devenyi, G. A., Gallino, D., Anastassiadis, C., et al. (2021a). Early or late gestational exposure to maternal immune activation alters neurodevelopmental trajectories in mice: an integrated neuroimaging, behavioral, and transcriptional study. Biol. Psychiatry 90:17. doi: 10.1016/j.biopsych.2021.03.017
Guma, E., Snook, E., Spring, S., Lerch, J. P., Nieman, B. J., Devenyi, G. A., et al. (2021b). Subtle alterations in neonatal neurodevelopment following early or late exposure to prenatal maternal immune activation in mice. Neuroimage Clin. 32:102868. doi: 10.1016/j.nicl.2021.102868
Ha, M., and Kim, V. N. (2014). Regulation of microRNA biogenesis. Nat. Rev. Mol. Cell Biol. 15:3838. doi: 10.1038/nrm3838
Haga, S., Yamamoto, N., Nakai-Murakami, C., Osawa, Y., Tokunaga, K., Sata, T., et al. (2008). Modulation of TNF-α-converting enzyme by the spike protein of SARS-CoV and ACE2 induces TNF-α production and facilitates viral entry. Proc. Natl. Acad. Sci. U.S.A. 105:1105. doi: 10.1073/pnas.0711241105
Haijma, S., van Haren, N., Cahn, W., Koolschijn, P. C. M., Hulshoff Pol, H. E., and Kahn, R. S. (2013). Brain volumes in schizophrenia: a meta-analysis in over 18 000 subjects. Schizophr Bull. 39:118. doi: 10.1093/schbul/sbs118
Harmon, A. C., Cornelius, D. C., Amaral, L. M., Faulkner, J. L., Cunningham, M. W., Wallace, K., et al. (2016). The role of inflammation in the pathology of preeclampsia. Clin. Sci. 130:702. doi: 10.1042/CS20150702
Harry, G. J., and Kraft, A. D. (2012). Microglia in the developing brain: a potential target with lifetime effects. Neurotoxicology 33:12. doi: 10.1016/j.neuro.2012.01.012
Hartkopf, J., Schleger, F., Keune, J., Wiechers, C., Pauluschke-Froehlich, J., Weiss, M., et al. (2018). Impact of intrauterine growth restriction on cognitive and motor development at 2 years of age. Front. Physiol. 9:1278. doi: 10.3389/fphys.2018.01278
Heidarpour, M., Vakhshoori, M., Abbasi, S., Shafie, D., and Rezaei, N. (2020). Adrenal insufficiency in coronavirus disease 2019: a case report. J. Med. Case Rep. 14:2461. doi: 10.1186/s13256-020-02461-2
Hering, L., Herse, F., Geusens, N., Verlohren, S., Wenzel, K., Staff, A. C., et al. (2010). Effects of circulating and local uteroplacental angiotensin ii in rat pregnancy. Hypertension 56:150961. doi: 10.1161/HYPERTENSIONAHA.110.150961
Hessami, K., Norooznezhad, A. H., Monteiro, S., Barrozo, E. R., Abdolmaleki, A. S., Arian, S. E., et al. (2022). COVID-19 pandemic and infant neurodevelopmental impairment: a systematic review and meta-analysis. JAMA Netw Open. 5:e2238941. doi: 10.1001/JAMANETWORKOPEN.2022.38941
Heurich, A., Hofmann-Winkler, H., Gierer, S., Liepold, T., Jahn, O., and Pöhlmann, S. (2014). TMPRSS2 and ADAM17 cleave ACE2 differentially and only proteolysis by TMPRSS2 augments entry driven by the severe acute respiratory syndrome coronavirus spike protein. J. Virol. 88:13. doi: 10.1128/jvi.02202-13
Hibar, D. P., Westlye, L. T., Doan, N. T., Jahanshad, N., Cheung, J. W., Ching, C. R. K., et al. (2018). Cortical abnormalities in bipolar disorder: an MRI analysis of 6503 individuals from the ENIGMA bipolar disorder working group. Mol. Psychiatry 23:73. doi: 10.1038/mp.2017.73
Hibar, D. P., Westlye, L. T., van Erp, T. G. M., Rasmussen, J., Leonardo, C. D., Faskowitz, J., et al. (2016). Subcortical volumetric abnormalities in bipolar disorder. Mol. Psychiatry 21:227. doi: 10.1038/mp.2015.227
Hodge, D. R., Cho, E., Copeland, T. D., Guszczynski, T., Yang, E., Seth, A. K., et al. (2007). IL-6 enhances the nuclear translocation of DNA cytosine-5-methyltransferase 1 (DNMT1) via phosphorylation of the nuclear localization sequence by the AKT kinase. Cancer Genom Proteom. 2007:4.
Hosier, H., Farhadian, S. F., Morotti, R. A., Deshmukh, U., Lu-Culligan, A., Campbell, K. H., et al. (2020). SARS-CoV-2 infection of the placenta. J. Clin. Invest. 130:569. doi: 10.1172/JCI139569
Hsiao, E. Y., and Patterson, P. H. (2011). Activation of the maternal immune system induces endocrine changes in the placenta via IL-6. Brain Behav. Immun. 25:17. doi: 10.1016/j.bbi.2010.12.017
Hsing, C. H., Hung, S. K., Chen, Y. C., Wei, T. S., Sun, D. P., Wang, J. J., et al. (2015). Histone deacetylase inhibitor trichostatin a ameliorated endotoxin-induced neuroinflammation and cognitive dysfunction. Med. Inflamm. 2015:163140. doi: 10.1155/2015/163140
Iadecola, C., Anrather, J., and Kamel, H. (2020). Effects of COVID-19 on the nervous system. Cell 183:28. doi: 10.1016/j.cell.2020.08.028
Jackson, C. B., Farzan, M., Chen, B., and Choe, H. (2022). Mechanisms of SARS-CoV-2 entry into cells. Nat. Rev. Mol. Cell Biol. 23:3. doi: 10.1038/S41580-021-00418-X
Jafarinejad-Farsangi, S., Jazi, M. M., Rostamzadeh, F., and Hadizadeh, M. (2020). High affinity of host human microRNAs to SARS-CoV-2 genome: an in silico analysis. Noncoding RNA Res. 5:2020. doi: 10.1016/j.ncrna.2020.11.005
Jeganathan, K., and Paul, A. B. M. (2022). Vertical transmission of SARS-CoV-2: a systematic review. Obstet. Med. 15:91. doi: 10.1177/1753495X211038157
Jensen, E. C., Bennet, L., Wood, C., Vickers, M., Breier, B., Gunn, A. J., et al. (2011). Loss of the pregnancy-induced rise in cortisol concentrations in the ewe impairs the fetal insulin-like growth factor axis. Rep. Fertil Dev. 23:317. doi: 10.1071/RD10317
Jensen, E., Wood, C. E., and Keller-Wood, M. (2005). Chronic alterations in ovine maternal corticosteroid levels influence uterine blood flow and placental and fetal growth. Am. J. Physiol. Regul. Int. Comput. Physiol. 288:2004. doi: 10.1152/ajpregu.00149.2004
Jensen, E., Wood, C. E., and Keller-Wood, M. (2007). Reduction of maternal adrenal steroids results in increased VEGF protein without increased eNOS in the ovine placenta. Placenta 28:5. doi: 10.1016/j.placenta.2006.09.005
Jiang, H., Xu, L., Shao, L., Xia, R., Yu, Z., Ling, Z., et al. (2016). Maternal infection during pregnancy and risk of autism spectrum disorders: a systematic review and meta-analysis. Brain Behav. Immun. 58:5. doi: 10.1016/j.bbi.2016.06.005
Johansson, S., Price, J., and Modo, M. (2008). Effect of inflammatory cytokines on major histocompatibility complex expression and differentiation of human neural stem/progenitor cells. Stem. Cells 26:116. doi: 10.1634/stemcells.2008-0116
Juttukonda, L. J., Wachman, E. M., Boateng, J., Jain, M., Benarroch, Y., and Taglauer, E. S. (2022). Decidual immune response following COVID-19 during pregnancy varies by timing of maternal SARS-CoV-2 infection. J. Rep. Immunol. 151:103501. doi: 10.1016/j.jri.2022.103501
Kalogeropoulou, A., Lygerou, Z., and Taraviras, S. (2019). Cortical development and brain malformations: insights from the differential regulation of early events of DNA replication. Front. Cell Dev. Biol. 7:29. doi: 10.3389/fcell.2019.00029
Kapoor, A., Dunn, E., Kostaki, A., Andrews, M. H., and Matthews, S. G. (2006). Fetal programming of hypothalamo-pituitary-adrenal function: prenatal stress and glucocorticoids. J. Physiol. 572:105254. doi: 10.1113/jphysiol.2006.105254
Karlsson, H., and Dalman, C. (2020). Epidemiological studies of prenatal and childhood infection and schizophrenia. Curr. Top. Behav. Neurosci. 44:87. doi: 10.1007/7854_2018_87
Khan, S., Peng, L., Siddique, R., Nabi, G., and Xue, M. (2020). Impact of COVID-19 infection on pregnancy outcomes and the risk of maternal-to-neonatal intrapartum transmission of COVID-19 during natural birth. Infect. Control Hosp. Epidemiol. 41:84. doi: 10.1017/ice.2020.84
Kim, Y. K., Na, K. S., Myint, A. M., and Leonard, B. E. (2016). The role of pro-inflammatory cytokines in neuroinflammation, neurogenesis and the neuroendocrine system in major depression. Prog. Neuropsychopharmacol. Biol. Psychiatry 64:8. doi: 10.1016/j.pnpbp.2015.06.008
Kimberlin, D. W., and Stagno, S. (2020). Can SARS-CoV-2 infection be acquired in utero? more definitive evidence is needed. JAMA J. Am. Med. Assoc. 323:4868. doi: 10.1001/jama.2020.4868
Kirtsman, M., Diambomba, Y., Poutanen, S. M., Malinowski, A. K., Vlachodimitropoulou, E., Parks, W. T., et al. (2020). Probable congenital sars-cov-2 infection in a neonate born to a woman with active sars-cov-2 infection. CMAJ 192:200821. doi: 10.1503/cmaj.200821
Kleeman, E. A., Gubert, C., and Hannan, A. J. (2022). Transgenerational epigenetic impacts of parental infection on offspring health and disease susceptibility. Trends Genet. 38, 662–675. doi: 10.1016/J.TIG.2022.03.006
Knight, M., Bunch, K., Vousden, N., Morris, E., Simpson, N., Gale, C., et al. (2020). Characteristics and outcomes of pregnant women admitted to hospital with confirmed SARS-CoV-2 infection in UK: national population based cohort study. BMJ 369:2107. doi: 10.1136/bmj.m2107
Krenn, V., Bosone, C., Burkard, T. R., Spanier, J., Kalinke, U., Calistri, A., et al. (2021). Organoid modeling of Zika and herpes simplex virus 1 infections reveals virus-specific responses leading to microcephaly. Cell Stem. Cell. 28:1362. doi: 10.1016/J.STEM.2021.03.004
Labouesse, M. A., Dong, E., Grayson, D. R., Guidotti, A., and Meyer, U. (2015). Maternal immune activation induces GAD1 and GAD2 promoter remodeling in the offspring prefrontal cortex. Epigenetics 10:4202. doi: 10.1080/15592294.2015.1114202
Lambert, D. W., Yarski, M., Warner, F. J., Thornhill, P., Parkin, E. T., Smith, A. I., et al. (2005). Tumor necrosis factor-α convertase (ADAM17) mediates regulated ectodomain shedding of the severe-acute respiratory syndrome-coronavirus (SARS-CoV) receptor, angiotensin-converting enzyme-2 (ACE2). J. Biol. Chem. 280, 30113–30119. doi: 10.1074/jbc.M505111200
Lemaire, V., Koehl, M., Moal, M., and Abrous, D. N. (2000). Prenatal stress produces learning deficits associated with an inhibition of neurogenesis in the hippocampus. Proc. Natl. Acad. Sci. U.S.A. 97:11032. doi: 10.1073/pnas.97.20.11032
Leow, M. K. S., Kwek, D. S. K., Ng, A. W. K., Ong, K. C., Kaw, G. J. L., and Lee, L. S. U. (2005). Hypocortisolism in survivors of severe acute respiratory syndrome (SARS). Clin. Endocrinol. 63:2325. doi: 10.1111/j.1365-2265.2005.02325.x
Liu, H., Wang, L. L., Zhao, S. J., Kwak-Kim, J., Mor, G., and Liao, A. H. (2020). Why are pregnant women susceptible to COVID-19? An immunological viewpoint. J. Rep. Immunol. 139:103122. doi: 10.1016/j.jri.2020.103122
Liu, X. S., Chopp, M., Pan, W. L., Wang, X. L., Fan, B. Y., Zhang, Y., et al. (2017). MicroRNA-146a promotes oligodendrogenesis in stroke. Mol. Neurobiol. 54:9655. doi: 10.1007/s12035-015-9655-7
Liu, Y. H., Lai, W. S., Tsay, H. J., Wang, T. W., and Yu, J. Y. (2013). Effects of maternal immune activation on adult neurogenesis in the subventricular zone-olfactory bulb pathway and olfactory discrimination. Schizophr Res. 151:7. doi: 10.1016/j.schres.2013.09.007
Loayza, M., Lin, S., Carter, K., Ojeda, N., Fan, L. W., Ramarao, S., et al. (2022). Maternal immune activation alters fetal and neonatal microglia phenotype and disrupts neurogenesis in mice. Pediat. Res. 2022, 1–10. doi: 10.1038/s41390-022-02239-w
Looker, K. J., Magaret, A. S., May, M. T., Turner, K. M. E., Vickerman, P., Newman, L. M., et al. (2017). First estimates of the global and regional incidence of neonatal herpes infection. Lancet Glob Health 5:e300. doi: 10.1016/S2214-109X(16)30362-X
Lu, R., Zhao, X., Li, J., Niu, P., Yang, B., Wu, H., et al. (2020). Genomic characterisation and epidemiology of 2019 novel coronavirus: implications for virus origins and receptor binding. Lancet 395:30251. doi: 10.1016/S0140-6736(20)30251-8
Lu-Culligan, A., Chavan, A. R., Vijayakumar, P., Irshaid, L., Courchaine, E. M., Milano, K. M., et al. (2021). Maternal respiratory SARS-CoV-2 infection in pregnancy is associated with a robust inflammatory response at the maternal-fetal interface. Medical 2:16. doi: 10.1016/j.medj.2021.04.016
Maccani, M. A., Padbury, J. F., and Marsit, C. J. (2011). miR-16 and miR-21 expression in the placenta is associated with fetal growth. PLoS One 6:210. doi: 10.1371/journal.pone.0021210
Makinodan, M., Tatsumi, K., Manabe, T., Yamauchi, T., Makinodan, E., Matsuyoshi, H., et al. (2008). Maternal immune activation in mice delays myelination and axonal development in the hippocampus of the offspring. J. Neurosci. Res. 86:216763. doi: 10.1002/jnr.21673
Malaeb, S., and Dammann, O. (2009). Fetal inflammatory response and brain injury in the preterm newborn. J. Child. Neurol. 24:8066. doi: 10.1177/0883073809338066
Mann, J. R., and McDermott, S. (2011). Are maternal genitourinary infection and pre-eclampsia associated with ADHD in school-aged children? J. Atten. Dis. 15:66. doi: 10.1177/1087054710370566
Marek, S., Tervo-Clemmens, B., Calabro, F. J., Montez, D. F., Kay, B. P., Hatoum, A. S., et al. (2022). Reproducible brain-wide association studies require thousands of individuals. Nature 603:4492. doi: 10.1038/s41586-022-04492-9
Marsico, C., and Kimberlin, D. W. (2017). Congenital cytomegalovirus infection: advances and challenges in diagnosis, prevention and treatment. Ital. J. Pediatr. 43:358. doi: 10.1186/S13052-017-0358-8
Mastorakos, G., and Ilias, I. (2003). Maternal and fetal hypothalamic-pituitary-adrenal axes during pregnancy and postpartum. Ann. N.Y. Acad. Sci. 1290:16. doi: 10.1196/annals.1290.016
Matelski, L., Morgan, R. K., Grodzki, A. C., van de Water, J., and Lein, P. J. (2021). Effects of cytokines on nuclear factor-kappa B, cell viability, and synaptic connectivity in a human neuronal cell line. Mol. Psychiatry 26:647. doi: 10.1038/s41380-020-0647-2
McDonald, C., Bullmore, E. T., Sham, P. C., Chitnis, X., Wickham, H., Bramon, E., et al. (2004). Association of genetic risks for schizophrenia and bipolar disorder with specific and generic brain structural endophenotypes. Arch. Gen. Psychiatry 61:974. doi: 10.1001/archpsyc.61.10.974
Mednick, S. A., Machon, R. A., Huttunen, M. O., and Bonett, D. (1988). Adult schizophrenia following prenatal exposure to an influenza epidemic. Arch. Gen. Psychiatry 45:9013. doi: 10.1001/archpsyc.1988.01800260109013
Mendoza, M., Garcia-Ruiz, I., Maiz, N., Rodo, C., Garcia-Manau, P., Serrano, B., et al. (2020). Pre-eclampsia-like syndrome induced by severe COVID-19: a prospective observational study. BJOG 127, 1374–1380. doi: 10.1111/1471-0528.16339
Meyer, U., Nyffeler, M., Engler, A., Urwyler, A., Schedlowski, M., Knuesel, I., et al. (2006). The time of prenatal immune challenge determines the specificity of inflammation-mediated brain and behavioral pathology. J. Neurosci. 26:2006. doi: 10.1523/JNEUROSCI.0099-06.2006
Miller, S. L., Huppi, P. S., and Mallard, C. (2016). The consequences of fetal growth restriction on brain structure and neurodevelopmental outcome. J. Physiol. 594:402. doi: 10.1113/JP271402
Miranda, A., and Sousa, N. (2018). Maternal hormonal milieu influence on fetal brain development. Brain Behav. 8:920. doi: 10.1002/brb3.920
Moisiadis, V. G., and Matthews, S. G. (2014). Glucocorticoids and fetal programming part 1: outcomes. Nat. Rev. Endocrinol. 10:73. doi: 10.1038/nrendo.2014.73
Monier, A., Adle-Biassette, H., Delezoide, A. L., Evrard, P., Gressens, P., and Verney, C. (2007). Entry and distribution of microglial cells in human embryonic and fetal cerebral cortex. J. Neuropathol. Exp. Neurol. 66:46. doi: 10.1097/nen.0b013e3180517b46
Monteiro, V. R. S., Andrade, C. B., Gomes, H. R., Reginatto, M. W., Império, G. E., Fontes, K. N., et al. (2022). Mid-pregnancy poly(I:C) viral mimic disrupts placental ABC transporter expression and leads to long-term offspring motor and cognitive dysfunction. Sci. Rep. 12:10262. doi: 10.1038/s41598-022-14248-0
Mortensen, P. B., Nørgaard-Pedersen, B., Waltoft, B. L., Sørensen, T. L., Hougaard, D., Torrey, E. F., et al. (2007). Toxoplasma gondii as a risk factor for early-onset schizophrenia: analysis of filter paper blood samples obtained at birth. Biol. Psychiatry 61:24. doi: 10.1016/j.biopsych.2006.05.024
Mouihate, A., and Kalakh, S. (2021). Maternal interleukin-6 hampers hippocampal neurogenesis in adult rat offspring in a sex-dependent manner. Dev. Neurosci. 43:16370. doi: 10.1159/000516370
Mulvey, J. J., Magro, C. M., Ma, L. X., Nuovo, G. J., and Baergen, R. N. (2020). Analysis of complement deposition and viral RNA in placentas of COVID-19 patients. Ann. Diagn. Pathol. 46:530. doi: 10.1016/j.anndiagpath.2020.151530
Musa, S. S., Bello, U. M., Zhao, S., Abdullahi, Z. U., Lawan, M. A., and He, D. (2021). Vertical transmission of sars-cov-2: A systematic review of systematic reviews. Viruses 13, 1877. doi: 10.3390/V13091877/S1
Nakamura, J. P., Schroeder, A., Gibbons, A., Sundram, S., and Hill, R. A. (2022). Timing of maternal immune activation and sex influence schizophrenia-relevant cognitive constructs and neuregulin and GABAergic pathways. Brain Behav. Immun. 100, 70–82. doi: 10.1016/j.bbi.2021.11.006
Nampoothiri, S., Sauve, F., Ternier, G., Fernandois, D., Coelho, C., Imbernon, M., et al. (2020). The hypothalamus as a hub for SARS-CoV-2 brain infection and pathogenesis. bioRxiv [preprint]. doi: 10.1101/2020.06.08.139329
Neves, L. A. A., Stovall, K., Joyner, J. N., Valdés, G., Gallagher, P. E., Ferrario, C. M., et al. (2008). ACE2 and ANG-(1-7) in the rat uterus during early and late gestation. Am. J. Physiol. Regul. Int. Comput. Physiol. 294:2007. doi: 10.1152/ajpregu.00514.2007
Newsome, K., Alverson, C. J., Williams, J., McIntyre, A. F., Fine, A. D., Wasserman, C., et al. (2019). Outcomes of infants born to women with influenza a (H1N1)pdm09. Birth Defects Res. 111:88. doi: 10.1002/BDR2.1445
Nguyen, L. S., Fregeac, J., Bole-Feysot, C., Cagnard, N., Iyer, A., Anink, J., et al. (2018). Role of miR-146a in neural stem cell differentiation and neural lineage determination: relevance for neurodevelopmental disorders. Mol. Autism 9:219. doi: 10.1186/s13229-018-0219-3
Nguyen, L. S., Lepleux, M., Makhlouf, M., Martin, C., Fregeac, J., Siquier-Pernet, K., et al. (2016). Profiling olfactory stem cells from living patients identifies miRNAs relevant for autism pathophysiology. Mol. Autism 7:64. doi: 10.1186/s13229-015-0064-6
Nielsen, P. R., Laursen, T. M., and Mortensen, P. B. (2013). Association between parental hospital-treated infection and the risk of schizophrenia in adolescence and early adulthood. Schizophr Bull. 39, 149. doi: 10.1093/schbul/sbr149
Okano, H., Takashima, K., Takahashi, Y., Ojiro, R., Tang, Q., Ozawa, S., et al. (2022). Progressive disruption of neurodevelopment by mid-gestation exposure to lipopolysaccharides and the ameliorating effect of continuous alpha-glycosyl isoquercitrin treatment. Environ. Toxicol. [Epub ahead of print]. doi: 10.1002/TOX.23661
Paniz-Mondolfi, A., Bryce, C., Grimes, Z., Gordon, R. E., Reidy, J., Lednicky, J., et al. (2020). Central nervous system involvement by severe acute respiratory syndrome coronavirus-2 (SARS-CoV-2). J. Med. Virol. 92:25915. doi: 10.1002/jmv.25915
Parikshak, N. N., Luo, R., Zhang, A., Won, H., Lowe, J. K., Chandran, V., et al. (2013). XIntegrative functional genomic analyses implicate specific molecular pathways and circuits in autism. Cell 155:31. doi: 10.1016/j.cell.2013.10.031
Patanè, L., Morotti, D., Giunta, M. R., Sigismondi, C., Piccoli, M. G., Frigerio, L., et al. (2020). Vertical transmission of coronavirus disease 2019: severe acute respiratory syndrome coronavirus 2 RNA on the fetal side of the placenta in pregnancies with coronavirus disease 2019–positive mothers and neonates at birth. Am. J. Obstet. Gynecol. MFM 2:100145. doi: 10.1016/j.ajogmf.2020.100145
Patro, N., Singh, K., and Patro, I. (2013). Differential microglial and astrocytic response to bacterial and viral infection in the developing hippocampus of neonatal rats. Indian J. Exp. Biol. 51, 606–614.
Petri, R., Malmevik, J., Fasching, L., Åkerblom, M., and Jakobsson, J. (2014). MiRNAs in brain development. Exp. Cell Res. 321:22. doi: 10.1016/j.yexcr.2013.09.022
Pineda, D. A., Palacio, L. G., Puerta, I. C., Merchán, V., Arango, C. P., Galvis, A. Y., et al. (2007). Environmental influences that affect attention deficit/hyperactivity disorder: study of a genetic isolate. Eur. Child Adolesc. Psychiatry 16:605. doi: 10.1007/s00787-007-0605-4
Pringle, K. G., Tadros, M. A., Callister, R. J., and Lumbers, E. R. (2011). The expression and localization of the human placental prorenin/renin- angiotensin system throughout pregnancy: roles in trophoblast invasion and angiogenesis? Placenta 32:20. doi: 10.1016/j.placenta.2011.09.020
Provençal, N., Arloth, J., Cattaneo, A., Anacker, C., Cattane, N., Wiechmann, T., et al. (2020). Glucocorticoid exposure during hippocampal neurogenesis primes future stress response by inducing changes in DNA methylation. Proc. Natl. Acad. Sci. U.S.A. 117:2116. doi: 10.1073/pnas.1820842116
Rahman, I., Gilmour, P. S., Jimenez, L. A., and MacNee, W. (2002). Oxidative stress and TNF-alpha induce histone acetylation and NF-kappaB/AP-1 activation in alveolar epithelial cells: potential mechanism in gene transcription in lung inflammation. Mol. Cell. Biochem. 235, 239–248.
Ramos, J. G. L., Sass, N., and Costa, S. H. M. (2017). Préeclâmpsia. Rev Brasil. Ginecol. Obstet. 39, 496–512. doi: 10.1055/s-0037-1604471
Raony, Í, de Figueiredo, C. S., Pandolfo, P., Giestal-de-Araujo, E., Oliveira-Silva Bomfim, P., and Savino, W. (2020). Psycho-neuroendocrine-immune interactions in COVID-19: potential impacts on mental health. Front. Immunol. 11:1170. doi: 10.3389/fimmu.2020.01170
Rasmussen, J. M., Graham, A. M., Entringer, S., Gilmore, J. H., Styner, M., Fair, D. A., et al. (2019). Maternal interleukin-6 concentration during pregnancy is associated with variation in frontolimbic white matter and cognitive development in early life. Neuroimage 185:20. doi: 10.1016/j.neuroimage.2018.04.020
Ratnayake, U., Quinn, T., Walker, D. W., and Dickinson, H. (2013). Cytokines and the neurodevelopmental basis of mental illness. Front. Neurosci. 2013:180. doi: 10.3389/fnins.2013.00180
Redies, C., Hertel, N., and Hübner, C. A. (2012). Cadherins and neuropsychiatric disorders. Brain Res. 1470:20. doi: 10.1016/j.brainres.2012.06.020
Reisinger, S. N., Kong, E., Khan, D., Schulz, S., Ronovsky, M., Berger, S., et al. (2016). Maternal immune activation epigenetically regulates hippocampal serotonin transporter levels. Neurobiol. Stress 4:7. doi: 10.1016/j.ynstr.2016.02.007
Richetto, J., Chesters, R., Cattaneo, A., Labouesse, M. A., Gutierrez, A. M. C., Wood, T. C., et al. (2017a). Genome-wide transcriptional profiling and structural magnetic resonance imaging in the maternal immune activation model of neurodevelopmental disorders. Cerebral Cortex 27:320. doi: 10.1093/cercor/bhw320
Richetto, J., Massart, R., Weber-Stadlbauer, U., Szyf, M., Riva, M. A., and Meyer, U. (2017b). Genome-wide DNA methylation changes in a mouse model of infection-mediated neurodevelopmental disorders. Biol. Psychiatry 81:10. doi: 10.1016/j.biopsych.2016.08.010
Robinson, S. (2005). Systemic prenatal insults disrupt telencephalon development: implications for potential interventions. Epile. Behav. 7:5. doi: 10.1016/j.yebeh.2005.06.005
Rudolph, M. D., Graham, A. M., Feczko, E., Miranda-Dominguez, O., Rasmussen, J. M., Nardos, R., et al. (2018). Maternal IL-6 during pregnancy can be estimated from newborn brain connectivity and predicts future working memory in offspring. Nat. Neurosci. 21:128. doi: 10.1038/s41593-018-0128-y
Saatci, D., van Nieuwenhuizen, A., and Handunnetthi, L. (2021). Maternal infection in gestation increases the risk of non-affective psychosis in offspring: a meta-analysis. J. Psychiatr. Res. 139:39. doi: 10.1016/j.jpsychires.2021.05.039
Saliba, E., and Henrot, A. (2001). Inflammatory mediators and neonatal brain damage. Biol. Neonate 2001:7096. doi: 10.1159/000047096
Santra, M., Zhang, Z. G., Yang, J., Santra, S., Santra, S., Chopp, M., et al. (2014). Thymosin β4 up-regulation of MicroRNA-146a promotes oligodendrocyte differentiation and suppression of the toll-like proinflammatory pathway. J. Biol. Chem. 289:9966. doi: 10.1074/jbc.M113.529966
Saulle, I., Garziano, M., Fenizia, C., Cappelletti, G., Parisi, F., Clerici, M., et al. (2021). MiRNA profiling in plasma and placenta of SARS-CoV-2-infected pregnant women. Cells 10:1788. doi: 10.3390/cells10071788
Schafer, D. P., and Stevens, B. (2015). Microglia function in central nervous system development and plasticity. Cold Spring Harb Perspect Biol. 7:545. doi: 10.1101/cshperspect.a020545
Schepici, G., Cavalli, E., Bramanti, P., and Mazzon, E. (2019). Autism spectrum disorder and mirna: an overview of experimental models. Brain Sci. 9:265. doi: 10.3390/brainsci9100265
Schmidt, A., Morales-Prieto, D. M., Pastuschek, J., Fröhlich, K., and Markert, U. R. (2015). Only humans have human placentas: molecular differences between mice and humans. J. Rep. Immunol. 108, 65–71. doi: 10.1016/j.jri.2015.03.001
Schreiber, B., Patel, A., and Verma, A. (2021). Shedding light on COVID-19: ADAM17 the missing link? Am. J. Ther. 28:1226. doi: 10.1097/MJT.0000000000001226
Schwartz, D. A., and Hyg, M. (2017). The origins and emergence of zika virus, the newest TORCH infection: what’s old is new again. Arch. Pathol. Lab. Med. 141, 18–25. doi: 10.5858/ARPA.2016-0429-ED
Seckl, J. R. (2004). Prenatal glucocorticoids and long-term programming. Eur. J. Endocrinol. Suppl. 2004:49. doi: 10.1530/eje.0.151u049
Sehovic, E., Spahic, L., Smajlovic-Skenderagic, L., Pistoljevic, N., Dzanko, E., and Hajdarpasic, A. (2020). Identification of developmental disorders including autism spectrum disorder using salivary miRNAs in children from bosnia and herzegovina. PLoS One 15:351. doi: 10.1371/journal.pone.0232351
Selten, J. P., Frissen, A., Lensvelt-Mulders, G., and Morgan, V. A. (2010). Schizophrenia and 1957 pandemic of influenza: meta-analysis. Schizophr. Bull. 36:147. doi: 10.1093/schbul/sbp147
Shibata, E., Powers, R. W., Rajakumar, A., von Versen-Höynck, F., Gallaher, M. J., Lykins, D. L., et al. (2006). Angiotensin II decreases system a amino acid transporter activity in human placental villous fragments through AT1 receptor activation. Am. J. Physiol. Endocrinol. Metab. 291:2006. doi: 10.1152/ajpendo.00134.2006
Shook, L. L., Sullivan, E. L., Lo, J. O., Perlis, R. H., and Edlow, A. G. (2022). COVID-19 in pregnancy: implications for fetal brain development. Trends Mol. Med. 28:4. doi: 10.1016/j.molmed.2022.02.004
Shuffrey, L. C., Firestein, M. R., Kyle, M. H., Fields, A., Alcántara, C., Amso, D., et al. (2022). Association of birth during the COVID-19 pandemic with neurodevelopmental status at 6 months in infants with and without in utero exposure to maternal SARS-CoV-2 infection. JAMA Pediatr. 176:5563. doi: 10.1001/jamapediatrics.2021.5563
Silva, D., Colvin, L., Hagemann, E., and Bower, C. (2014). Environmental risk factors by gender associated with attention-deficit/hyperactivity disorder. Pediatrics 133:1434. doi: 10.1542/peds.2013-1434
Silverman, M. N., Pearce, B. D., Biron, C. A., and Miller, A. H. (2005). Immune modulation of the hypothalamic-pituitary-adrenal (HPA) axis during viral infection. Viral Immunol. 18:41. doi: 10.1089/vim.2005.18.41
Simanek, A. M., and Meier, H. C. S. (2015). Association between prenatal exposure to maternal infection and offspring mood disorders: a review of the literature. Curr. Probl Pediatr. Adolesc. Health Care 45:8. doi: 10.1016/j.cppeds.2015.06.008
Sisman, J., Jaleel, M. A., Moreno, W., Rajaram, V., Collins, R. R. J., Savani, R. C., et al. (2020). Intrauterine transmission of SARS-COV-2 infection in a preterm infant. Pediatric Infect. Dis. J. 2020:2815. doi: 10.1097/INF.0000000000002815
Smith, S. E. P., Li, J., Garbett, K., Mirnics, K., and Patterson, P. H. (2007). Maternal immune activation alters fetal brain development through interleukin-6. J. Neurosci. 27:2007. doi: 10.1523/JNEUROSCI.2178-07.2007
Spann, M. N., Monk, C., Scheinost, D., and Peterson, B. S. (2018). Maternal immune activation during the third trimester is associated with neonatal functional connectivity of the salience network and fetal to toddler behavior. J. Neurosci. 38:2018. doi: 10.1523/JNEUROSCI.2272-17.2018
Spitz, D. (2019). Zika: the continuing threat. Bull. World Health Organ. 97:6. doi: 10.2471/BLT.19.020119
Stiles, J., and Jernigan, T. L. (2010). The basics of brain development. Neuropsychol. Rev. 20:9148. doi: 10.1007/s11065-010-9148-4
Sunwoo, J. S., Jeon, D., Lee, S. T., Moon, J., Yu, J. S., Park, D. K., et al. (2018). Maternal immune activation alters brain microRNA expression in mouse offspring. Ann. Clin. Transl Neurol. 5:652. doi: 10.1002/acn3.652
Susser, E. S., Schaefer, C. A., Brown, A. S., Begg, M. D., and Wyatt, R. J. (2000). The design of the prenatal determinants of schizophrenia study. Schizophr. Bull. 26:3451. doi: 10.1093/oxfordjournals.schbul.a033451
Sutton, D., Fuchs, K., D’Alton, M., and Goffman, D. (2020). Universal screening for SARS-CoV-2 in women admitted for delivery. New England J. Med. 382:316. doi: 10.1056/nejmc2009316
Szyf, M. (2015). Prospects for the development of epigenetic drugs for CNS conditions. Nat. Rev. Drug Discov 14, 4580. doi: 10.1038/nrd4580
Talebizadeh, Z., Butler, M. G., and Theodoro, M. F. (2008). Feasibility and relevance of examining lymphoblastoid cell lines to study role of microRNAs in autism. Autism Res. 1:33. doi: 10.1002/aur.33
Tang, B., Jia, H., Kast, R. J., and Thomas, E. A. (2013). Epigenetic changes at gene promoters in response to immune activation in utero. Brain Behav. Immun 30:86. doi: 10.1016/j.bbi.2013.01.086
Tsukada, T., Sakata-Haga, H., Shimada, H., Shoji, H., and Hatta, T. (2021). Mid-pregnancy maternal immune activation increases Pax6-positive and Tbr2-positive neural progenitor cells and causes integrated stress response in the fetal brain in a mouse model of maternal viral infection. IBRO Neurosci. Rep. 11:3. doi: 10.1016/j.ibneur.2021.07.003
Tsukada, T., Simamura, E., Shimada, H., Arai, T., Higashi, N., Akai, T., et al. (2015). The suppression of maternal-fetal leukemia inhibitory factor signal relay pathway by maternal immune activation impairs brain development in mice. PLoS One 10:29011. doi: 10.1371/journal.pone.0129011
Valdés, G., Neves, L. A. A., Anton, L., Corthorn, J., Chacón, C., Germain, A. M., et al. (2006). Distribution of angiotensin-(1-7) and ACE2 in human placentas of normal and pathological pregnancies. Placenta 27:15. doi: 10.1016/j.placenta.2005.02.015
van Erp, T. G. M., Hibar, D. P., Rasmussen, J. M., Glahn, D. C., Pearlson, G. D., Andreassen, O. A., et al. (2016). Subcortical brain volume abnormalities in 2028 individuals with schizophrenia and 2540 healthy controls via the ENIGMA consortium. Mol. Psychiatry 21:63. doi: 10.1038/mp.2015.63
van Erp, T. G. M., Walton, E., Hibar, D. P., Schmaal, L., Jiang, W., Glahn, D. C., et al. (2018). Cortical brain abnormalities in 4474 individuals with schizophrenia and 5098 control subjects via the enhancing neuro imaging genetics through meta analysis (ENIGMA) consortium. Biol. Psychiatry 84:23. doi: 10.1016/j.biopsych.2018.04.023
Varma, P., Lybrand, Z. R., Antopia, M. C., and Hsieh, J. (2021). Novel targets of SARS-CoV-2 spike protein in human fetal brain development suggest early pregnancy vulnerability. Front. Neurosci. 14:614680. doi: 10.3389/fnins.2020.614680
Vivanti, A. J., Vauloup-Fellous, C., Prevot, S., Zupan, V., Suffee, C., do Cao, J., et al. (2020). Transplacental transmission of SARS-CoV-2 infection. Nat. Commun. 11:17436. doi: 10.1038/s41467-020-17436-6
Waffarn, F., and Davis, E. P. (2012). Effects of antenatal corticosteroids on the hypothalamic-pituitary-adrenocortical axis of the fetus and newborn: experimental findings and clinical considerations. Am. J. Obstet. Gynecol. 207:12. doi: 10.1016/j.ajog.2012.06.012
Walker, F., Nilsson, M., and Jones, K. (2013). Acute and chronic stress-induced disturbances of microglial plasticity, phenotype and function. Curr. Drug. Targets 14:208. doi: 10.2174/13894501113149990208
Wang, X., Hagberg, H., Zhu, C., Jacobsson, B., and Mallard, C. (2007). Effects of intrauterine inflammation on the developing mouse brain. Brain Res. 1144:83. doi: 10.1016/j.brainres.2007.01.083
Wang, Y., Chen, L., Wu, T., Shi, H., Li, Q., Jiang, H., et al. (2020). Impact of Covid-19 in pregnancy on mother’s psychological status and infant’s neurobehavioral development: a longitudinal cohort study in China. BMC Med. 18:1825. doi: 10.1186/s12916-020-01825-1
Weber-Stadlbauer, U. (2017). Epigenetic and transgenerational mechanisms in infection-mediated neurodevelopmental disorders. Trans. Psychiatry 7:78. doi: 10.1038/tp.2017.78
Weber-Stadlbauer, U., Richetto, J., Zwamborn, R. A. J., Slieker, R. C., and Meyer, U. (2021). Transgenerational modification of dopaminergic dysfunctions induced by maternal immune activation. Neuropsychopharmacology 46:855. doi: 10.1038/s41386-020-00855-w
Welberg, L. A. M., Seckl, J. R., and Holmes, M. C. (2000). Inhibition of 11β-hydroxysteroid dehydrogenase, the foetoplacental barrier to maternal glucocorticoids, permanently programs amygdala GR mRNA expression and anxiety-like behaviour in the offspring. Eur. J. Neurosci. 12:958. doi: 10.1046/j.1460-9568.2000.00958.x
Wheatland, R. (2004). Molecular mimicry of ACTH in SARS-implications for corticosteroid treatment and prophylaxis. Med. Hypotheses 63:9. doi: 10.1016/j.mehy.2004.04.009
World Health Organization [WHO] (2022). WHO coronavirus (COVID-19) dashboard with vaccination data. Available online at: https://covid19.who.int/ (accessed November 21, 2022).
Woods, R. M., Lorusso, J. M., Potter, H. G., Neill, J. C., Glazier, J. D., and Hager, R. (2021). Maternal immune activation in rodent models: a systematic review of neurodevelopmental changes in gene expression and epigenetic modulation in the offspring brain. Neurosci. Biobehav. Rev. 129:15. doi: 10.1016/j.neubiorev.2021.07.015
Wright, I. C., Rabe-Hesketh, S., Woodruff, P. W. R., David, A. S., Murray, R. M., and Bullmore, E. T. (2000). Meta-analysis of regional brain volumes in schizophrenia. Am. J. Psychiatry 157:16. doi: 10.1176/ajp.157.1.16
Wu, W. L., Hsiao, E. Y., Yan, Z., Mazmanian, S. K., and Patterson, P. H. (2017). The placental interleukin-6 signaling controls fetal brain development and behavior. Brain Behav. Immun. 62:7. doi: 10.1016/j.bbi.2016.11.007
Wu, Y. E., Parikshak, N. N., Belgard, T. G., and Geschwind, D. H. (2016). Genome-wide, integrative analysis implicates microRNA dysregulation in autism spectrum disorder. Nat. Neurosci. 19:4373. doi: 10.1038/nn.4373
Xiong, X., Wei, H., Zhang, Z., Chang, J., Ma, X., Gao, X., et al. (2020). Vaginal delivery report of a healthy neonate born to a convalescent mother with COVID-19. J. Med. Virol. 92:25857. doi: 10.1002/jmv.25857
Xu, J., Zhong, S., Liu, J., Li, L., Li, Y., Wu, X., et al. (2005). Detection of severe acute respiratory syndrome coronavirus in the brain: potential role of the chemokine mig in pathogenesis. Clin. Infect. Dis. 41:461. doi: 10.1086/444461
Yaghoobpoor, S., Fathi, M., Vakili, K., Tutunchian, Z., Dehghani, M., Bahrami, A., et al. (2022). Cardiovascular complications of COVID-19 among pregnant women and their fetuses: a systematic review. J. Clin. Med. 11:6194. doi: 10.3390/JCM11206194/S1
Yan, J., Guo, J., Fan, C., Juan, J., Yu, X., Li, J., et al. (2020). Coronavirus disease 2019 in pregnant women: a report based on 116 cases. Am. J. Obstet. Gynecol. 223:14. doi: 10.1016/j.ajog.2020.04.014
Yoon, B., Jun, J., Romero, R., Park, K., Gomez, R., Choi, J., et al. (1997). Amniotic fluid inflammatory cytokines (interleukin-6, interleukin-1(beta), and tumor necrosis factor-(alpha)), neonatal brain white matter lesions, and cerebral palsy. Am. J. Obstet. Gynecol. 177, 19–26. doi: 10.1016/s0002-9378(97)70432-0
Yu, F., Du, L., Ojcius, D. M., Pan, C., and Jiang, S. (2020). Measures for diagnosing and treating infections by a novel coronavirus responsible for a pneumonia outbreak originating in Wuhan, China. Microbes Infect. 22:3. doi: 10.1016/j.micinf.2020.01.003
Yui, K., Sato, A., and Imataka, G. (2015). Mitochondrial dysfunction and its relationship with mtor signaling and oxidative damage in autism spectrum disorders. Mini Rev. Med. Chem. 15:930. doi: 10.2174/1389557515666150324122930
Zaretsky, M., Alexander, J. M., Byrd, W., and Bawdon, R. E. (2004). Transfer of inflammatory cytokines across the placenta. Obstetr. Gynecol. 103:83. doi: 10.1097/01.AOG.0000114980.40445.83
Zhan, D., Zhang, C., Long, W., Wei, L., Jin, S., Du, C., et al. (2021). Intrauterine inflammation induced white matter injury protection by fibrinogen-like protein 2 deficiency in perinatal mice. Pediatr. Res. 89:1211. doi: 10.1038/s41390-020-01211-w
Zhang, J., Zhang, Z. G., Lu, M., Wang, X., Shang, X., Elias, S. B., et al. (2017). MiR-146a promotes remyelination in a cuprizone model of demyelinating injury. Neuroscience 348:29. doi: 10.1016/j.neuroscience.2017.02.029
Zhang, J., Zhang, Z. G., Lu, M., Zhang, Y., Shang, X., and Chopp, M. (2019). MiR-146a promotes oligodendrocyte progenitor cell differentiation and enhances remyelination in a model of experimental autoimmune encephalomyelitis. Neurobiol. Dis. 125:19. doi: 10.1016/j.nbd.2019.01.019
Zhao, Q., Wang, Q., Wang, J., Tang, M., Huang, S., Peng, K., et al. (2019). Maternal immune activation-induced PPARγ-dependent dysfunction of microglia associated with neurogenic impairment and aberrant postnatal behaviors in offspring. Neurobiol. Dis. 125:5. doi: 10.1016/j.nbd.2019.01.005
Zhao, X., Jiang, Y., Zhao, Y., Xi, H., Liu, C., Qu, F., et al. (2020). Analysis of the susceptibility to COVID-19 in pregnancy and recommendations on potential drug screening. Eur. J. Clin. Microbiol. Infect. Dis. 39:3897. doi: 10.1007/s10096-020-03897-6
Zhu, H., Wang, L., Fang, C., Peng, S., Zhang, L., Chang, G., et al. (2020). Clinical analysis of 10 neonates born to mothers with 2019-nCoV pneumonia. Trans. Pediatr. 9:6. doi: 10.21037/tp.2020.02.06
Zijlstra, G. J., ten Hacken, N. H. T., Hoffmann, R. F., van Oosterhout, A. J. M., and Heijink, I. H. (2012). Interleukin-17A induces glucocorticoid insensitivity in human bronchial epithelial cells. Eur. Res. J. 39:7911. doi: 10.1183/09031936.00017911
Zunszain, P. A., Anacker, C., Cattaneo, A., Choudhury, S., Musaelyan, K., Myint, A. M., et al. (2012). Interleukin-1β: a new regulator of the kynurenine pathway affecting human hippocampal neurogenesis. Neuropsychopharmacology 37:277. doi: 10.1038/npp.2011.277
Keywords: SARS-CoV-2, COVID-19, HPA axis, preeclampsia, brain development, inflammation, pregnancy
Citation: Dubey H, Sharma RK, Krishnan S and Knickmeyer R (2022) SARS-CoV-2 (COVID-19) as a possible risk factor for neurodevelopmental disorders. Front. Neurosci. 16:1021721. doi: 10.3389/fnins.2022.1021721
Received: 17 August 2022; Accepted: 28 November 2022;
Published: 16 December 2022.
Edited by:
Saulo Gantes Tractenberg, Pontifical Catholic University of Rio Grande do Sul, BrazilReviewed by:
Anthony Hannan, The University of Melbourne, AustraliaCopyright © 2022 Dubey, Sharma, Krishnan and Knickmeyer. This is an open-access article distributed under the terms of the Creative Commons Attribution License (CC BY). The use, distribution or reproduction in other forums is permitted, provided the original author(s) and the copyright owner(s) are credited and that the original publication in this journal is cited, in accordance with accepted academic practice. No use, distribution or reproduction is permitted which does not comply with these terms.
*Correspondence: Rebecca Knickmeyer, a25pY2ttZXlAbXN1LmVkdQ==
Disclaimer: All claims expressed in this article are solely those of the authors and do not necessarily represent those of their affiliated organizations, or those of the publisher, the editors and the reviewers. Any product that may be evaluated in this article or claim that may be made by its manufacturer is not guaranteed or endorsed by the publisher.
Research integrity at Frontiers
Learn more about the work of our research integrity team to safeguard the quality of each article we publish.