- 1Department of Occupational Therapy, College of Medicine, and Healthy Ageing Research Center, Chang Gung University, Taoyuan, Taiwan
- 2Department of Psychiatry, Chang Gung Memorial Hospital at Linkou, Taoyuan, Taiwan
- 3Department of Occupational Therapy, Rocky Mountain University of Health Professions, Provo, UT, United States
- 4Laboratory of Brain Imaging and Neural Dynamics (BIND Lab), Chang Gung University, Taoyuan, Taiwan
- 5Research Group Health Psychology, University of Leuven, Leuven, Belgium
- 6Bachelor Program in Artificial Intelligence, Chang Gung University, Taoyuan, Taiwan
- 7Graduate Institute of Mind, Brain and Consciousness, Taipei Medical University, Taipei, Taiwan
- 8Brain and Consciousness Research Center, Shuang-Ho Hospital, Taipei Medical University, Taipei, Taiwan
Psychological challenges have been found to impact respiratory symptom perception in healthy individuals as well as in patients with various neurological disorders. Human respiratory sensory gating is an objective measure to examine respiratory sensory information processing of repetitive respiratory mechanical stimuli in the central nervous system. With this electrophysiological method, patients with higher anxiety levels showed reduced respiratory sensory gating function in the cortex, and increased symptom perception. In addition, positive emotional contexts were found to increase the respiratory sensory gating function using RREPs. However, neural substrates related to emotional impacts on respiratory sensory gating remain still unclear. In the present study, we examined the emotion processing of respiratory sensory gating using functional magnetic resonance imaging. We hypothesized that positive compared with neutral stimuli would result in reduced brain activations in cortical areas with the paired occlusion paradigm. Thirty-five healthy adults participated in this event-designed fMRI experiment. Paired inspiratory occlusions (two transient occlusions with a 500 ms inter-stimulus-interval are delivered during one inspiration) were provided using an external trigger outside of the scanner. At least 40 paired inspiratory occlusions were collected for each trial. The experiment contained three runs during which participants underwent 12 min for the paired inspiratory occlusion paradigm while watching a fixation cross (the control condition), neutral and positive emotional picture series. The order of emotional picture series was randomized across the participants. Our results revealed an overall trend of reduction of brain activity from the neutral (minus fixation) condition, to the pleasant (minus fixation) condition. For bilateral thalamus and primary visual cortices, there was no significant difference in neural activation between the two contrasts of pleasant (ContrastP–F) and neutral condition (ContrastN–F). The activation of the mid-cingulate and the orbitofrontal cortex was lower in ContrastP–F compared to ContrastN–F. In conclusion, our results suggest that emotional context, especially positive valence, modulates neural correlates in middle cingulate cortex and orbitofrontal cortex in terms of respiratory sensory gating. Future studies are recommended to test emotional impacts on respiratory sensations in patients with neurological disorders.
Introduction
Previous research has shown that emotions affect mental and physical health and wellbeing (DeSteno et al., 2013). Unhealthy or negative emotions can deplete vital energy and increase the propensity for chronic diseases (Locke et al., 2004; Roest et al., 2010; Hartono et al., 2012). Specifically, the adverse impact of psychological distress on disease exacerbation, mortality, and lung function is well documented (Felker et al., 2001; McCathie et al., 2002; Yohannes et al., 2005; Ng et al., 2007). For example, patients with chronic diseases such as chronic obstructive pulmonary disease (COPD) patients with significant impairments of lung function often suffer from negative emotions including feelings of worry, fear, and sadness (Strang et al., 2014) as well as elevated levels of depression and anxiety (Yohannes and Alexopoulos, 2014). Similar findings have been observed in patients with neurological disorders such as Parkinson’s disease (Baille et al., 2019a,b; Das et al., 2019). Moreover, previous psychophysiological research has demonstrated increased respiratory sensations in patients with COPD during experimentally induced short-lasting negative affective states (von Leupoldt et al., 2010c) or in those patients with higher trait-like long-term forms of negative affectivity (Livermore et al., 2008; von Leupoldt et al., 2011; von Leupoldt and Denutte, 2020). Similarly, patients with Parkinson’s disease with high levels of depression and anxiety showed stronger symptoms of breathlessness (Baille et al., 2019a). In addition, Masaoka and Homma (2001) revealed that unpleasant emotions changed breathing patterns, and especially respiratory rate was positively correlated with individual state and trait anxiety levels (Masaoka and Homma, 2001). Leander et al. (2014) also found a connection between respiratory symptoms and psychological status and suggested that improving psychological status may be a valuable method to decrease respiratory symptoms in patients suffering from pulmonary diseases, such as COPD (Leander et al., 2014).
Psychophysiological studies further confirmed the relationship between emotions and the neural processing of respiratory sensations (Bogaerts et al., 2005; Chan et al., 2014, 2016), which is significant for human survival and can be measured by the respiratory-related evoked potential (RREP) in the electroencephalogram (Chan et al., 2015a; Herzog et al., 2021). With the single-obstruction (odd-ball) paradigm, von Leupoldt et al. (2010a) found that the amplitude of the RREP P3 component decreased in pleasant and unpleasant emotional contexts compared to a neutral emotional context, indicating that the processing of respiratory sensory input was modulated by emotions (von Leupoldt et al., 2010a). Two recent studies confirmed the observation that experimentally induced negative emotional states modulate the perception of respiratory sensations and their neural processing as evidenced by significant amplitude modulations of RREP components P2 and P3 (Herzog et al., 2019a,b). Another RREP study showed that the P3 amplitude of a high trait-like anxious group was smaller in a neutral emotional context compared to an unpleasant emotional context (von Leupoldt et al., 2011). In contrast, the P3 amplitude of a low anxious group was higher in the neutral emotional context compared to the unpleasant emotional context, which the authors interpreted such that respiratory sensations in participants with higher anxiety levels might demand more attentional and neural resources in an unpleasant affective context (von Leupoldt et al., 2011). Also in patients with COPD, a recent study showed increased RREP amplitudes compared to healthy controls, which were related to increased perception of respiratory sensations, presumably due to the higher relevance and attentional as well as neural demand in the patients (Reijnders et al., 2020).
The paired-obstruction RREP paradigm (i.e., obstructing twice within a single inspiration), another RREP method, is used to quantify a neural filter mechanism for respiratory sensations called “respiratory sensory gating,” i.e., the ability of the brain to suppress the processing of repetitive respiratory sensory stimuli (Chan and Davenport, 2008; von Leupoldt et al., 2011). This paradigm employs paired identical inspiratory obstructions of 150 milliseconds (ms) each (first stimulus/S1 and second stimulus/S2) with an inter-stimulus-interval (ISI) of 500 ms, resulting in a RREP N1 peak amplitude of S2/S1 ratio, which is an index of the amount of neural information filtered “in” vs. filtered “out” (Chan and Davenport, 2010a). Previous studies have shown that stress and anxiety are related to an altered respiratory sensory gating function (Chan and Davenport, 2010b; Chan et al., 2012, 2014, 2015b; Herzog et al., 2018). For instance, Chan et al. (2012) found that the neural throughput of the second stimulus may have been disinhibited at the subcortical level in anxious individuals, causing over-perception (Chan et al., 2012). Chan et al. (2015b) further showed that individuals with generalized anxiety disorder exhibited a decreased respiratory sensory gating due to a smaller response to the novel stimulus than the healthy controls (Chan et al., 2015b). Moreover, Chenivesse et al. (2014) demonstrated that short-lasting negative emotional states as induced by affective picture viewing reduced the respiratory sensory gating function when compared to a neutral emotional state (Chenivesse et al., 2014). Besides the effect of negative affect and anxiety on respiratory sensory gating, positive emotional contexts also gained attention in previous research (Tsai et al., 2013; Chan et al., 2016). For example, Chan et al. (2016) showed that a positive emotional context, relative to a neutral emotional context, was related to a better respiratory sensory gating function as evidenced by a reduced N1 peak S2/S1 ratio (Chan et al., 2016).
However, although emotional modulations of the RREP were previously demonstrated (von Leupoldt et al., 2013), studying the precise brain areas involved in the cortical and subcortical processing of respiratory sensations as reflected by the RREP method remains challenging and lacks precision. Past source analyses and animal studies suggested that the somatosensory cortex, somatosensory association cortex (SII), prefrontal, premotor, and supplementary motor cortices, as well as parietal areas, thalamus, and brainstem, were activated during short respiratory occlusions (Yates et al., 1994; Davenport et al., 1996; Logie et al., 1998; von Leupoldt et al., 2010b). More recent studies have used functional magnetic resonance imaging (fMRI) to compensate for the poor spatial resolution of the RREP method. These fMRI studies revealed activation patterns of neural substrates of respiratory sensations elicited by short single inspiratory occlusions, including cortical (sensorimotor cortices, frontal lobes, supramarginal gyrus, cingulate cortex, insular cortex) and subcortical (thalamus, hippocampus, and caudate) areas (Jack et al., 2010; Chan et al., 2018, 2019, 2022b). In addition, more prolonged respiratory obstructions demonstrated additional neural activation of the amygdala, cingulate cortex, and insular cortex under conditions of resistive loaded breathing-induced dyspnea (Peiffer et al., 2001; von Leupoldt et al., 2008, 2009a, 2010b; Faull et al., 2017; Vlemincx et al., 2021), which were also areas associated with elevated levels of negative affect such as anxiety or increased unpleasantness of breathlessness (von Leupoldt et al., 2008, 2009a,b, 2010b). In addition, Chan et al. (2019) found an association between anxiety levels and activation of the precuneus and inferior parietal gyrus in the right hemisphere in response to short inspiratory occlusions (Chan et al., 2019).
However, the specific cortical activation patterns underlying the modulation of respiratory sensory gating during positive emotional contexts remain unclear. Therefore, the present fMRI study aimed to determine the neural substrates of paired inspiratory occlusions while viewing positive emotional pictures in healthy volunteers. Specifically, we expected to observe different levels of neural activation in emotion-related brain areas, including the cingulate cortex and insula as well as further cortical regions, such as sensorimotor cortices, frontal lobes, and supramarginal gyrus.
Materials and methods
Study participants
Thirty-five healthy adults of at least 20 years of age participated in the study. All participants self-reported to have no respiratory, cardiovascular, neurological, or psychiatric diseases. In order to comply with the fMRI room requirements, those with metal implants including denture/braces or with a pacemaker were excluded from the study. In addition, potential participants with a head circumference over 60 cm and/or with claustrophobia were also not complying with the current fMRI setup and were therefore excluded. All study procedures were approved by the Institutional Review Board of the Chang Gung Medical Foundation.
Experiment protocol
The participants were required to perform a spirometry pulmonary function test (Cardinal Health Inc., Dublin, OH, USA) based on the American Thoracic Society and European Respiratory Society guidelines (Miller et al., 2005). All participants passed the minimum requirement of the Forced Expiratory Volume in 1 s (FEV1) of at least 70% of predicted normative values to be eligible to participate in the study. Upon completion, the participants were instructed to lie supine in the fMRI scanner. During the experiment, the participants were instructed to breathe through the facemask as normally as possible regardless of the occasional obstructed breaths.
For the details of respiratory apparatus, please refer to a previous study of Chan et al. (2019). Briefly, the inspiratory port of the two-way non-rebreathing valve at the facemask was connected via tubing to a customized occlusion valve placed approximately 3 m away from the fMRI scanner (Hans Rudolph Inc., Kansas City, USA). The occlusion valve was connected to a solenoid of a customized trigger and a pressure tank outside of the scanner. Occasional inspiratory occlusions were elicited by the closing of the occlusion valve (triggered manually by the experimenter). Two 150-ms inspiratory occlusions with a 500-ms inter-stimulus-interval were provided during the inspiratory phase every 2–4 breaths. At least 40 successful paired inspiratory occlusions per condition were collected for data analysis. Every participant underwent three paired inspiratory occlusion conditions, each lasting approximately 12 min. The first condition was always the control condition. For this control condition, the participant was instructed to focus on the fixation on the screen. The control condition was followed by the neutral and positive emotional context conditions with the sequence of these emotional conditions being randomized across participants. For the emotional context conditions, participants were instructed to watch a series of neutral emotional pictures during the neutral condition and a series of pleasant emotional pictures during the positive condition.
The emotional pictures used to create the positive and neutral emotional context were selected from the International Affective Picture Series (Lang et al., 2008). The IAPS is a standardized and validated instrument used in various studies to induce different short-lasting emotional states in study participants (Lang et al., 2008). The positive and neutral conditions were composed of 120 pictures selected from each category. Every picture was presented on the screen for 6 s. The participants viewed the picture series via a mirror mounted on the head coil. After each scan, the participants rated the perceived level of breathlessness using a visual analog scale (VAS), ranging from 0 to 100 (0 = no breathlessness and 100 = maximal level of breathlessness), as well as the subjective level of valence and arousal using the Self-Assessment Manikin (SAM) scale, ranging from 1 to 9 (1 = no arousal or not pleasant at all, and 9 = maximal arousal or pleasantness).
Image acquisition
The fMRI study was performed using a 3-T Magnetom scanner (Siemens MAGNETOM Prisma, Erlangen, Germany) in the National Taiwan University, Taipei, Taiwan. During the scan, the participants laid supine and their heads were comfortably positioned inside a standard head coil, which was padded with sponges for head immobilization. The participants were wearing earplugs in order to minimize interferences with the scanner noise. During the fMRI experiments, thirty-two continuous axial slices (3-mm thickness) were acquired by using a gradient echo, echo-planar imaging sequence to acquire blood oxygen level-dependent (BOLD) data (TR = 2000 ms, TE = 30 ms; flip angle = 90 degrees; matrix = 64 × 64; field of view = 220 × 220 mm). The total acquisition time was 12 min for each condition (360 scans).
Data analysis
The fMRI data was pre-processed using SPM8 (Welcome Department of Cognitive Neurology, Institute of Neurology, London, UK) on the MATLAB platform (The MathWorks, Inc., Natick, MA, USA) and AFNI (Cox, 1996). All images were motion corrected and realigned to the first imaging volume, spatially normalized into standard Montreal Neurologic Institute space and smoothed with an isotropic Gaussian kernel of 6-mm full-width at half-maximum.
At the first-level, the statistical analysis was performed with the general linear model (GLM) across the three experimental conditions: The fixation condition, the neutral emotional context, and the positive emotional context. A high-pass filter with a cut-off frequency of 128 s per wave was used to eliminate baseline drifts. Specifically, 12 regressors were considered in the GLM: one for onset timing of the corresponding respiratory occlusion condition convolved with the canonical hemodynamic response function, two for time and dispersion derivatives of the first regressor, one for baseline intensity, six for motion parameters, one for the voxel time series with highest temporal standard deviation, and the last one for the averaged time series within a cerebrospinal fluid (CSF) mask. The last two regressors were used as nuisance regressors to minimize the impact of physiological noise caused by baseline respiration (Birn et al., 2006). The CSF mask was generated by the labeled bilateral lateral ventricles provided by Neuromorphometrics, Inc.,1 with single-voxel erosion. After model estimation, the linear contrasts of interest were generated on the basis of the ensuing parameter estimates for each participant. The contrasts compared the averaged neuronal activity (BOLD response) across the baseline conditions (without occlusions) with the averaged neuronal activity for every condition to examine the main effect of respiratory sensation induced by the paired-occlusion paradigm in the fixation control, neutral, and positive contexts. Next, beta estimates were used in the second-level (group-level) analysis in SPM to identify the brain regions that were significantly activated in each condition and in the between-condition contrasts. The one-sample group activation maps were calculated separately for each condition with the multiple comparison correction of the family-wise error rate (FWE) corrected p < 0.05 and a cluster threshold of 20 voxels. Additionally, the contrast maps, i.e., the contrast between neutral context and fixation condition (ContrastN–F) as well as the contrast between positive context and fixation condition (ContrastP–F), were conducted using AFNI 3dClustSim correction method with autocorrelation function (corrected p < 0.05), where the parameter was set with uncorrected p < 0.001 and a cluster threshold of 65 voxels.
Region of interest analysis
In the region of interest (ROI) analysis, β-values were extracted and averaged over a whole ROI. One set of ROIs were defined based on the between-condition contrast maps (ContrastN–F compared with ContrastP–F). In addition, ROIs from the automated anatomical labeling (AAL) templates were chosen on the basis of previous studies to test the effects of emotional contexts on paired-occlusion elicited brain areas, which included bilateral anterior cingulate cortex, insula, middle cingulate cortex, thalamus, precentral cortex, postcentral cortex, and calcarine fissure (Tzourio-Mazoyer et al., 2002; Chan et al., 2016, 2019, 2022b,a).
Statistical analysis
Descriptive statistics were performed for analyzing personal characteristics, including gender, age, and pulmonary function data. Self-reported ratings of valence and arousal were compared between the neutral condition and pleasant emotional condition, and levels of breathlessness were compared between the three conditions using a one-way repeated measures analysis of variance (RMANOVA) with R software (ezANOVA version 4.4.0). The ContrastN–F was compared with theContrastP–F using their β contrasts with the paired t-test or Wilcoxon signed-rank test, depending on the results of the normality test. The statistical significance level was set as p < 0.05.
Results
A total of 35 participants (22 females; mean age = 24.11 ± 3.95 years) completed the experiments. Table 1 shows the participants’ baseline characteristics. The average FEV1 was 3.20 ± 0.57 L (82.44 ± 9.00% of predicted values), and the FEV1/FVC ratio was 107.42 ± 6.63%. The average levels of perceived breathlessness reported for the fixation, neutral, and positive condition were 27.63 ± 21.42 vs. 30.32 ± 23.66 vs. 29.66 ± 25.16, respectively (p > 0.1). The perceived level of valence was significantly lower in the neutral compared to the positive context (4.91 + 1.70 vs. 6.51 ± 1.42, p < 0.01), whereas the level of arousal was not different between the two emotional contexts (3.53 ± 1.85 vs. 2.74 ± 1.68, p > 0.1).
Figure 1 shows the average brain activation maps for the study participants in the control and experimental conditions (FWE-corrected, p < 0.05 and cluster size > 20 voxels). Overall, paired inspiratory occlusions elicited various brain activities in various brain regions including bilateral thalamus, sensorimotor cortices, parietal cortices, cingulate cortices, and frontal gyrus. The level of activation was observed to be higher in the fixation (control) condition, followed by the neutral emotional condition, and the positive emotional condition. In addition, ContrastN–F as well as ContrastP–F were conducted using the AFNI 3dClustSim correction method with autocorrelation function. The brain areas above the 3dClustSim threshold are shown in Table 2. The two ROIs showing different activation levels between ContrastN–F and ContrastP–F, the middle cingulate cortex (MCC) and the orbitofrontal cortex (OFC), were chosen from Table 2 for further ROI analysis.
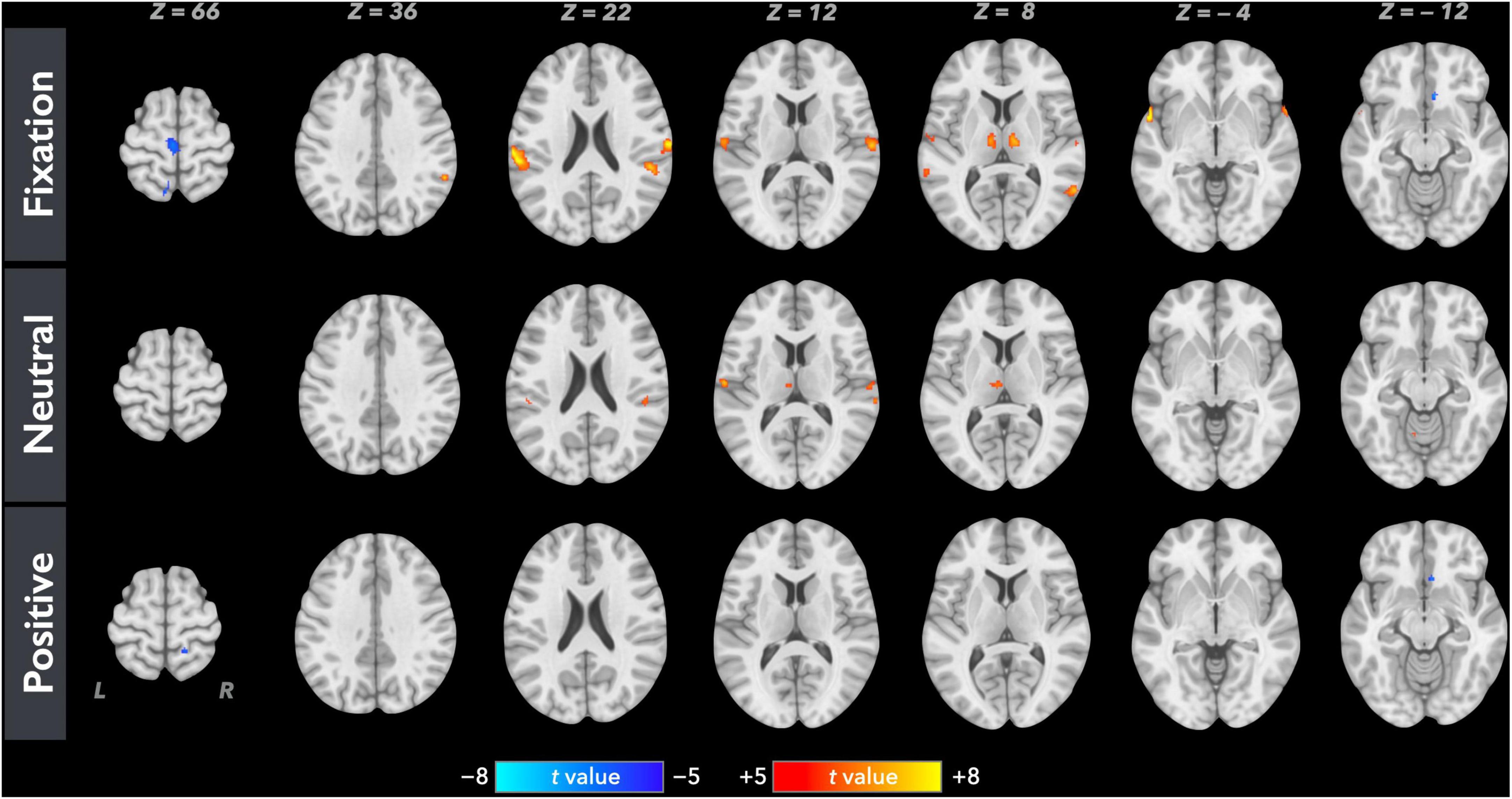
Figure 1. Averaged neural activation maps for the study participants (N = 35) in the fixation, neutral, and positive conditions (FWE-corrected, p < 0.05 and cluster size > 20 voxels).
For the ROI analysis, the paired t-test for the bilateral thalamus revealed that occlusion-induced neural activation (beta values) was comparable between the two contrasts (ContrastN–F vs. ContrastP–F, p = 0.47). For the primary visual area (V1), the analysis also showed no significant difference between ContrastN–F and ContrastP–F (p = 0.45). Figure 2 shows the bar graphs for the ContrastN–F and ContrastP–F in bilateral thalamus as well as the V1 cortices. Similarly, no significant difference was detected between the two contrasts ContrastN–F vs. ContrastP–F for cortical neural activation level in the inferior frontal gyrus (IFG) and supramarginal gyrus (p = 0.12 and 0.22, respectively). Regarding the sensorimotor areas, the analysis on the beta values revealed a trend for decreased activation in the positive context, but without statistically significant difference between the two contrasts (p = 0.34 and 0.25 for the precentral gyrus and postcentral gyrus, respectively). For the orbitofrontal cortex and MCC, the analysis showed a significant decrease of beta values in the positive compared to the neutral context (ContrastN-F vs. ContrastP-F, p < 0.01 and p < 0.03 for orbitofrontal cortex and MCC, respectively). Figure 3 showed the bar graphs for the ContrastN–F and ContrastP–F in the sensorimotor areas, OFC and MCC.
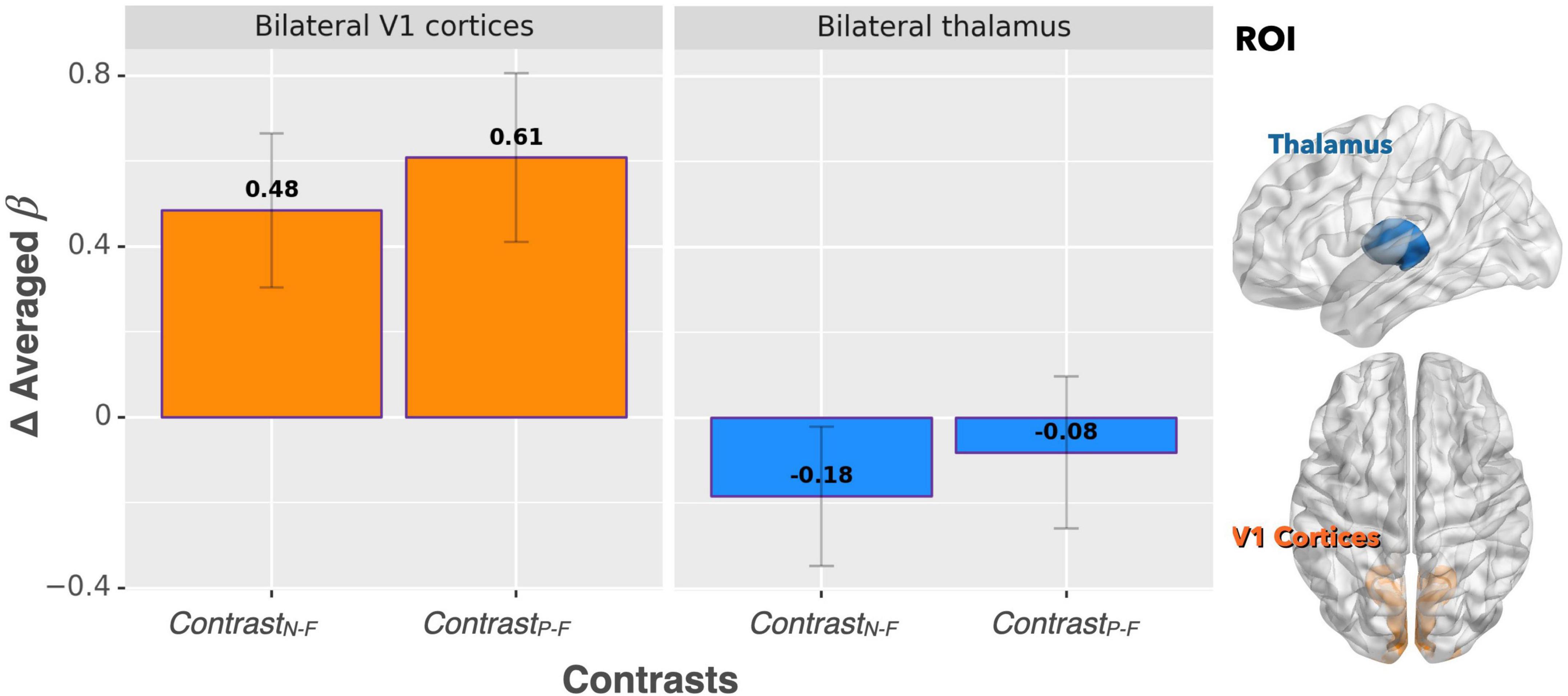
Figure 2. Beta value (averaged over the whole ROIs) comparisons between ContrastN–F and ContrastP–F for bilateral thalamus and V1 cortices. No significant difference was detected between the two contrasts in terms of activation in either ROI.
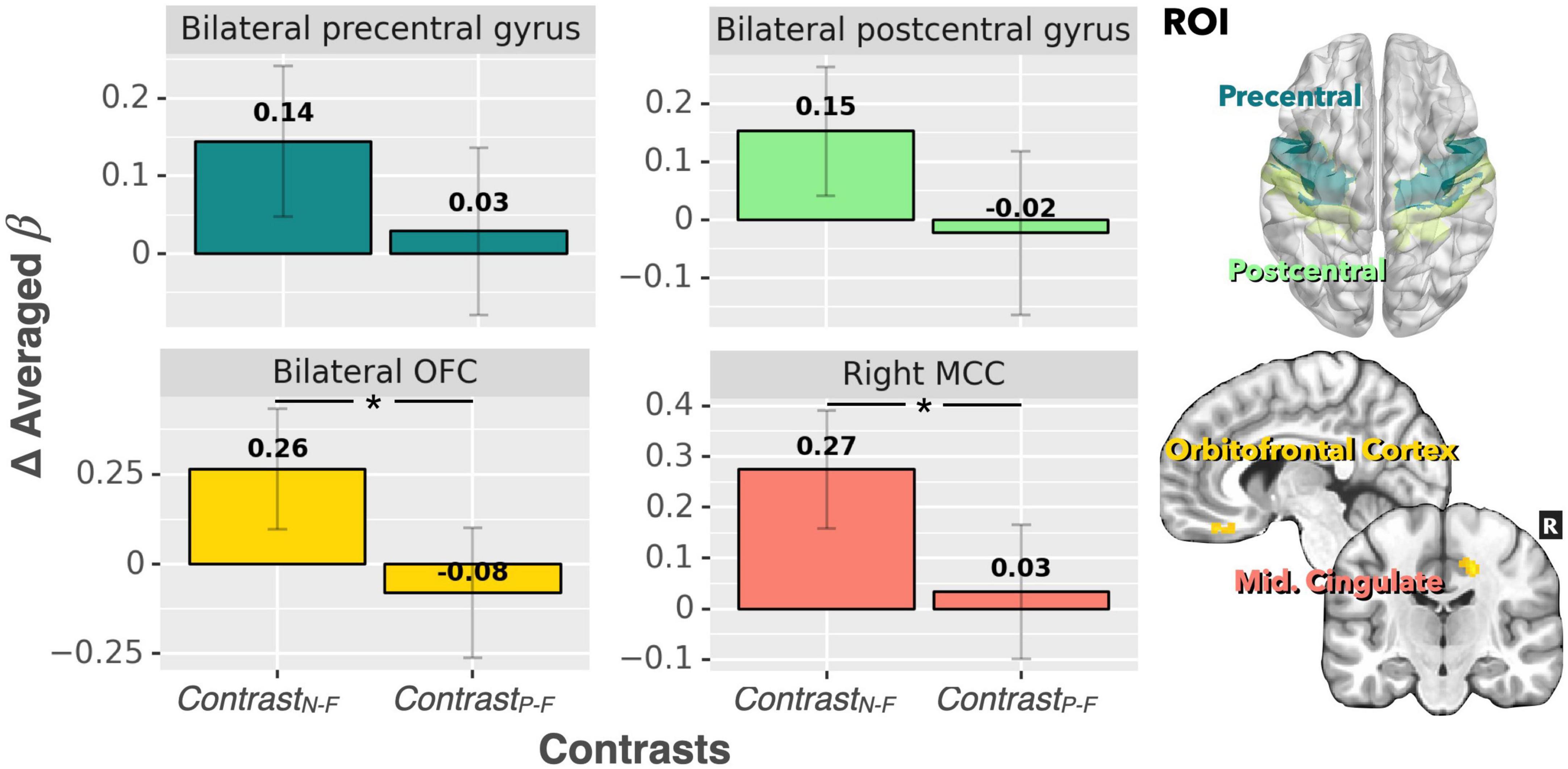
Figure 3. Beta value (averaged over the whole ROIs) comparisons between ContrastN–F and ContrastP–F for bilateral precentral gyrus, postcentral gyrus, bilateral OFC, and right MCC. There was a significant difference in neural activation between the two contrasts for both the MCC and OFC. A trend of decreased activation in ContrastP–F (compared to ContrastN–F) was observed for the precentral gyrus and postcentral gyrus (p < 0.1). *p < 0.001.
Discussion
The present study examined the brain activation patterns associated with respiratory sensory gating in response to paired inspiratory occlusions while viewing neutral and positive emotional pictures. We hypothesized that the positive emotional stimuli would result in lower brain activation than the neutral emotional stimuli as reflected by BOLD responses. Our results showed that overall, across the three conditions (fixation, neutral, and positive picture series), the positive emotional condition elicited the least neural activation in response to paired inspiratory occlusions. The level of brain activations elicited in the positive emotional condition (relative to fixation) was comparable with the neutral condition (relative to fixation) in several areas including bilateral primary visual cortices, thalamus, the IFG and supramarginal gyrus, whereas bilateral sensorimotor areas showed a trend for decreased neural activation in the positive emotional condition (ContrastP–F compared ContrastN–F). In addition, neural activations were significantly lower in the positive emotional condition (ContrastP–F compared ContrastN–F) for both the MCC and the OFC.
Based on the activated areas elicited by the paired inspiratory occlusion paradigm in the three conditions, it can be inferred that these brain regions, including the thalamus, hippocampus, sensorimotor cortex, temporal gyrus, frontal gyrus, and cingulate cortex, are involved in mediating respiratory gating functions. This view is consistent with our previous study (Chan et al., 2022a) and is further supported by another study examining voluntary breathing (McKay et al., 2003). Both previous studies demonstrated comparable brain activation patterns than the present study. Interestingly, the ContrastP–F and ContrastN–F showed comparable neural activations in the bilateral thalamus in response to the paired occlusion paradigm in the current study. The important role of the thalamus for neural gating functions was confirmed earlier by an animal lesion study where the thalamic reticular nucleus was crucial in mediating auditory sensory gating function in rats (Krause et al., 2003). However, our present results showed that the bilateral thalamus did not emerge as a brain area, which significantly differentiates between the neutral and positive emotional conditions. This finding suggests the following interpretations: first, the thalamus remains in charge of the sensory level of respiratory neural gating regardless of the emotional context; secondly, emotional context can modulate stimulus-related respiratory sensory inputs to the extent that brain energies or neural resources were allocated into regions other than thalamus.
For bilateral V1 cortex, the effect of increased visual processing was observed during both positive and neutral emotional conditions where the activation pattern of V1 was higher, while being the lowest in the fixation control condition. This confirms that the participants did voluntarily attend to the picture series and processed the stimuli during the experiment. The result also proves that, as can be expected, emotional effects on respiratory sensory gating were not processed by the occipital lobe as no difference between the ContrastP–F and ContrastN–F in the V1 cortices were observed. It has been suggested that emotion-related activity could reflect a general enhancement of visual processing (McAlonan et al., 2008), and perceptual representations in the visual cortex could mirror the content of emotional situations (Miskovic and Anderson, 2018). Therefore, it was anticipated that a comparable activation of V1 during both positive and neutral emotional conditions can be observed.
The present study revealed decreased levels of respiratory gating-induced brain activation under the positive emotional context in the orbitofrontal cortex and the middle cingulate cortex. Two possible mechanisms are proposed: First, the positive emotion context promotes attentional resource re-distribution when processing respiratory sensory information. This notion is supported by von Leupoldt et al. (2010a), where they showed emotional influences on the perception of respiratory sensation by decreased P3 amplitudes of the RREP when watching pleasant and unpleasant emotional picture series compared to neutral picture series (von Leupoldt et al., 2010d). Second, it is possible that the positive emotional context improves sensory gating function. As such, almost all respiratory sensory information is gated, which is supported by a previous RREP study showing a better respiratory sensory gating function in a positive emotional context compared to a neutral emotional context (Chan et al., 2016). Another study found that respiratory sensory gating was decreased in the presence of negative emotional visual stimuli (Chenivesse et al., 2014). In the present study, significantly lower levels of activations in the orbitofrontal cortex and middle cingulate cortex suggest that the need to process emotional aspects of respiratory sensation is much lower in a positive emotional context. However, our results showed that the precentral gyrus was activated in the positive emotional condition, which may be related to sensomotoric aspects related to the stimulus intensity, which means that inspiratory occlusions may involve consciously controlling breathing and putting more effort into breathing during the occluded phase. Nevertheless, the present results still showed a non-significant trend for decreased activation in the positive context for the precentral and postcentral gyrus, which suggests that the effect of occlusion stimuli could still be potentially modulated by emotional context at both sensation and motor action levels. Future research is recommended to test this aspect further by examining the relationships between individuals’ respiratory effort and emotional context during respiratory occlusions.
The middle cingulate cortex and the orbitofrontal cortex were found activated to a lesser extent in the positive emotional context (as shown in ContrastP–F compared to ContrastN–F) in response to paired inspiratory occlusions. This finding converges with several previous studies. For example, the cingulate cortex has been identified as a brain area that is directly associated with emotional aspects of dyspnea (Vogt and Laureys, 2005; von Leupoldt et al., 2009a; Herigstad et al., 2017; Stoeckel et al., 2018; Chan et al., 2019). The present finding of the MCC activation is in line with the study of Chan et al. (2019), where the MCC was found to be more activated in high anxious individuals compared to low anxious individuals, while the present study found the MCC less activated in the positive emotional context (Chan et al., 2019). The present result regarding the orbitofrontal cortex converges with several previous studies in which the prefrontal cortex was found activated during dyspneic sensations (Parsons et al., 2001; Evans et al., 2002; Peiffer et al., 2008). Notably, previous studies have more consistently suggested the anterior cingulate cortex (ACC) to be associated with emotional aspects of respiratory perception, whereas fewer studies found the MCC as the neural substrate that was directly associated with these emotional aspects (Chan et al., 2019). The discrepancy may be due to different study designs and methodologies, as the present study used inspiratory occlusion stimuli with the BOLD fMRI technique, whereas most of the previous studies used different stimulus methods including resistive loading, hypercapnia, and mechanical ventilation with PET or fMRI technique. Interestingly, MCC was consistently found activated in previous studies that examined brain substrates induced by hypercapnia, although these studies were not directly testing the effect of affective processing of dyspnea (Banzett et al., 2000; Parsons et al., 2001; Evans et al., 2002), which calls for future neuroimaging studies examining the effects of emotional modulations of hypercapnia-induced respiratory sensations.
Moreover, future studies are required in patients with neurological disorders, who can also suffer from increased respiratory symptoms such as dyspnea (Baille et al., 2019a,b; Das et al., 2019). For example, Baille et al. (2019a) recently demonstrated high levels of dyspnea in patients with Parkinson’s disease, which was related to higher levels of depression and anxiety (Baille et al., 2019a). It may be suspected that these associations are related to disturbed emotional modulations of the neural respiratory sensory gating mechanism, which is further supported by previous findings showing abnormal sensory gating patterns for oropharyngeal stimuli in patients with Parkinson’s disease (Pitts et al., 2016). If found to be true, such disturbances in the interrelationships between emotions and respiratory sensory gating may offer a target for therapeutic interventions in these patients. In addition, our study did not perform resting-state fMRI recordings because the current focus was on brain activities induced by respiratory sensory gating across different emotional contexts. However, functional connectivities between ROIs such as the OFC and MCC may also be examined in future investigations, so that clinical implications in terms of neural bases of emotions and respiratory sensation can be informed for diseases including neurological disorders.
One major limitation in the present study was the lack of independently recorded physiological signals, e.g., participants’ heart beats and respiratory end-tidal PCO2, for subsequent noise correction. Although the in-scanner respiration data was recorded by the physiological monitor unit (PMU in PRISMA, Siemens) in this study, the data was not analyzable due to a synchronization failure between fMRI and PMU. Therefore, we adopted the signal extraction method from fMRI data as a remedy for physiological noise correction. Future studies with similar experimental designs are encouraged to include the independently recorded PMU signals during fMRI scanning and to carefully confirm the functionality of PMU with the MRI technical support. In addition, although the present study did not test cognitive processing of respiratory perception, a few studies in the literature supported the notion that emotional context could potentially modulate cognitive awareness of respiratory sensory information processing. For example, Bogaerts et al. (2005) examined the effect of different emotional contexts in modulating respiratory interoceptive accuracy. They discovered that people with negative affective states showed less accuracy in an emotionally distressing context compared to a pleasant context (Bogaerts et al., 2005). In addition, von Leupoldt et al. (2011) tested the impact of unpleasant, neutral and pleasant emotional picture series on the RREP. They showed that the RREP P3 peak (indicative of attentional processing of respiratory sensory stimuli) amplitude was smaller in the unpleasant experimental conditions compared to the neutral condition in lower anxious individuals; however, higher anxious individuals processed more respiratory sensory information cognitively as evidenced by higher RREP P3 peak amplitudes (von Leupoldt et al., 2011). Therefore, future investigations are warranted to examine the role of emotional context in cognitive processing of respiratory interceptive information.
In summary, the present study shows that the emotional context modulates the neural substrates of respiratory sensory gating in a paired inspiratory-occlusion paradigm. Especially a positive emotional context may facilitate a top-down modulation of the respiratory sensory gating function. Imaging results in the present study showed that the MCC and the OFC are the two most prominent brain regions involved in both positive and neutral emotional conditions. Our current results further support the concept that respiratory information processing indeed includes both sensory and affective components and can each inform the future development in clinical rehabilitation strategies. Future studies are recommended to test the emotional impact of clinical interventions on respiratory sensations for managing respiratory symptoms.
Data availability statement
The raw data supporting the conclusions of this article will be made available by the authors, without undue reservation.
Ethics statement
The studies involving human participants were reviewed and approved by the Institutional Review Board, Chang Gung Medical Foundation. The patients/participants provided their written informed consent to participate in this study.
Author contributions
P-YC contributed to the conception and design of the study, organized the database, and wrote the first draft of the manuscript. A-LH and CW performed the statistical analysis. W-PC, C-HC, C-YL, and AvL wrote sections of the manuscript. All authors contributed to the manuscript revision, read, and approved the submitted version.
Funding
This study was supported by MOST-109-2320-B-182-008-MY3, MOST-105-2420-H-182-002-MY3, and MOST 111-2222-E-182-001-MY3 from the Ministry of Science & Technology, CMRPD1K0081 as well as BMRPB96 from the Linkou Chang Gung Memorial Hospital in Taiwan. AvL was supported by research grants from the Research Foundation—Flanders (FWO), Belgium (G0C1921N; GOA3718N), an infrastructure grant from the FWO and the Research Fund KU Leuven, Belgium (AKUL/19/06; I011320N), and by the “Asthenes” long-term structural funding Methusalem grant (METH/15/011) by the Flemish Government, Belgium. A-LH was supported by the MOST111-2222-E-182-001-MY3 from the Ministry of Science and Technology as well as BMRPK39 from the Chang Gung Memorial Hospital in Taiwan.
Acknowledgments
We thank Ms Yu-Ting Wu and Dr. Chia-Wei Li for assisting with fMRI data collection and data reduction. The gratitude is extended to the Imaging Center for Integrated Body, Mind and Culture Research at the National Taiwan University for the fMRI equipment support.
Conflict of interest
The authors declare that the research was conducted in the absence of any commercial or financial relationships that could be construed as a potential conflict of interest.
Publisher’s note
All claims expressed in this article are solely those of the authors and do not necessarily represent those of their affiliated organizations, or those of the publisher, the editors and the reviewers. Any product that may be evaluated in this article, or claim that may be made by its manufacturer, is not guaranteed or endorsed by the publisher.
Footnotes
References
Baille, G., Perez, T., Devos, D., Machuron, F., Dujardin, K., Chenivesse, C., et al. (2019b). Dyspnea is a specific symptom in Parkinson’s disease. J. Parkinsons Dis. 9, 785–791. doi: 10.3233/JPD-191713
Baille, G., Chenivesse, C., Perez, T., Machuron, F., Dujardin, K., Devos, D., et al. (2019a). Dyspnea: An underestimated symptom in Parkinson’s disease. Parkinsonism Relat. Disord. 60, 162–166. doi: 10.1016/j.parkreldis.2018.09.001
Banzett, R. B., Mulnier, H. E., Murphy, K., Rosen, S. D., Wise, R. J., and Adams, L. (2000). Breathlessness in humans activates insular cortex. Neuroreport 11, 2117–2120. doi: 10.1097/00001756-200007140-00012
Bogaerts, K., Notebaert, K., Van Diest, I., Devriese, S., De Peuter, S., and Van den Bergh, O. (2005). Accuracy of respiratory symptom perception in different affective contexts. J. Psychosom. Res. 58, 537–543. doi: 10.1016/j.jpsychores.2004.12.005
Birn, R. M., Diamond, J. B., Smith, M. A., and Bandettini, P. A. (2006). Separating respiratory-variation-related fluctuations from neuronal-activity-related fluctuations in fMRI. NeuroImage 31, 1536–1548. doi: 10.1016/j.neuroimage.2006.02.048
Chan, P. S., Cheng, C. H., Wu, Y. T., Wu, C. W., Hsu, A. L., Liu, C. Y., et al. (2022b). Neural substrates of respiratory sensory gating: A human fMRI study. Biol. Psychol. 169:108277. doi: 10.1016/j.biopsycho.2022.108277
Chan, P. S., Cheng, C. H., Liu, C. Y., and Davenport, P. W. (2022a). cortical sources of respiratory mechanosensation, laterality, and emotion: An MEG study. Brain Sci. 12:249. doi: 10.3390/brainsci12020249
Chan, P. S., Wu, Y. T., Hsu, A. L., Li, C. W., Wu, C. W., von Leupoldt, A., et al. (2019). The effect of anxiety on brain activation patterns in response to inspiratory occlusions: an fMRI study. Sci. Rep. 9:15045. doi: 10.1038/s41598-019-51396-2
Chan, P. Y., and Davenport, P. W. (2008). Respiratory-related evoked potential measures of respiratory sensory gating. J. Appl. Physiol. 105, 1106–1113. doi: 10.1152/japplphysiol.90722.2008
Chan, P. Y., and Davenport, P. W. (2010a). Respiratory related evoked potential measures of cerebral cortical respiratory information processing. Biol. Psychol. 84, 4–12. doi: 10.1016/j.biopsycho.2010.02.009
Chan, P. Y., and Davenport, P. W. (2010b). The role of nicotine on respiratory sensory gating measured by respiratory-related evoked potentials. J. Appl. Physiol. 108, 662–669. doi: 10.1152/japplphysiol.00798.2009
Chan, P. Y., Cheng, C. H., Hsu, S. C., Liu, C. Y., Davenport, P. W., and von Leupoldt, A. (2015b). Respiratory sensory gating measured by respiratory-related evoked potentials in generalized anxiety disorder. Front. Psychol. 6:957. doi: 10.3389/fpsyg.2015.00957
Chan, P. Y., Cheng, C. H., and von Leupoldt, A. (2015a). The effect of development in respiratory sensory gating measured by electrocortical activations. Neural Plast. 2015:389142. doi: 10.1155/2015/389142
Chan, P. Y., Cheng, C. H., Jhu, Y. J., Chen, C. L., and von Leupoldt, A. (2016). Being anxious, thinking positively: The effect of emotional context on respiratory sensory gating. Front. Physiol. 7:19. doi: 10.3389/fphys.2016.00019
Chan, P. Y., Cheng, C. H., Wu, Y. T., Wu, C. W., Liu, H. A., Shaw, F. Z., et al. (2018). Cortical and subcortical neural correlates for respiratory sensation in response to transient inspiratory occlusions in humans. Front. Physiol. 9:1804. doi: 10.3389/fphys.2018.01804
Chan, P. Y., von Leupoldt, A., Bradley, M. M., Lang, P. J., and Davenport, P. W. (2012). The effect of anxiety on respiratory sensory gating measured by respiratory-related evoked potentials. Biol. Psychol. 91, 185–189. doi: 10.1016/j.biopsycho.2012.07.001
Chan, P. Y., von Leupoldt, A., Liu, C. Y., and Hsu, S. C. (2014). Respiratory perception measured by cortical neural activations in individuals with generalized anxiety disorder. Respir. Physiol. Neurobiol. 204, 36–40. doi: 10.1016/j.resp.2014.09.009
Chenivesse, C., Chan, P. Y., Tsai, H. W., Wheeler-Hegland, K., Silverman, E., von Leupoldt, A., et al. (2014). Negative emotional stimulation decreases respiratory sensory gating in healthy humans. Respir. Physiol. Neurobiol. 204, 50–57. doi: 10.1016/j.resp.2014.08.019
Cox, R. W. (1996). AFNI: Software for analysis and visualization of functional magnetic resonance neuroimages. Comput. Biomed. Res. 29, 162–173. doi: 10.1006/cbmr.1996.0014
Das, P., Naing, N. N., Wan-Arfah, N., Naing Noor Jan, K. O., Kueh, Y. C., and Rasalingam, K. (2019). Depression and quality of life in patients with neurological disorder in a Malaysian Hospital. East Asian Arch. Psychiatry 29, 66–70. doi: 10.12809/eaap1777
Davenport, P. W., Colrain, I. M., and Hill, P. M. (1996). Scalp topography of the short-latency components of the respiratory-related evoked potential in children. J. Appl. Physiol. 80, 1785–1791. doi: 10.1152/jappl.1996.80.5.1785
DeSteno, D., Gross, J. J., and Kubzansky, L. (2013). Affective science and health: the importance of emotion and emotion regulation. Health Psychol. 32, 474–486. doi: 10.1037/a0030259
Evans, K. C., Banzett, R. B., Adams, L., McKay, L., Frackowiak, R. S., and Corfield, D. R. (2002). BOLD fMRI identifies limbic, paralimbic, and cerebellar activation during air hunger. J. Neurophysiol. 88, 1500–1511. doi: 10.1152/jn.2002.88.3.1500
Faull, O. K., Hayen, A., and Pattinson, K. T. S. (2017). Breathlessness and the body: Neuroimaging clues for the inferential leap. Cortex 95, 211–221. doi: 10.1016/j.cortex.2017.07.019
Felker, B., Katon, W., Hedrick, S. C., Rasmussen, J., McKnight, K., McDonnell, M. B., et al. (2001). The association between depressive symptoms and health status in patients with chronic pulmonary disease. Gen. Hosp. Psychiatry 23, 56–61. doi: 10.1016/s0163-8343(01)00127-x
Hartono, J. L., Mahadeva, S., and Goh, K. L. (2012). Anxiety and depression in various functional gastrointestinal disorders: do differences exist? J. Dig. Dis. 13, 252–257. doi: 10.1111/j.1751-2980.2012.00581.x
Herigstad, M., Faull, O. K., Hayen, A., Evans, E., Hardinge, F. M., Wiech, K., et al. (2017). Treating breathlessness via the brain: changes in brain activity over a course of pulmonary rehabilitation. Eur. Respir. J. 50:1701029. doi: 10.1183/13993003.01029-2017
Herzog, M., Sucec, J., Jelincic, V., Van Diest, I., Van den Bergh, O., Chan, P. S., et al. (2021). The test-retest reliability of the respiratory-related evoked potential. Biol. Psychol. 163:108133. doi: 10.1016/j.biopsycho.2021.108133
Herzog, M., Sucec, J., Vukovic, M., Van Diest, I., Van den Bergh, O., and von Leupoldt, A. (2019b). Experimental social rejection increases dyspnoea perception and neural processing of respiratory sensations in healthy subjects. Eur. Respir. J. 53:1801409. doi: 10.1183/13993003.01409-2018
Herzog, M., Sucec, J., Van Diest, I., Van den Bergh, O., and von Leupoldt, A. (2019a). The presence of others reduces dyspnea and cortical neural processing of respiratory sensations. Biol. Psychol. 140, 48–54. doi: 10.1016/j.biopsycho.2018.11.004
Herzog, M., Sucec, J., Van Diest, I., Van den Bergh, O., Chan, P. S., Davenport, P., et al. (2018). Reduced neural gating of respiratory sensations is associated with increased dyspnoea perception. Eur. Respir. J. 52:1800559. doi: 10.1183/13993003.00559-2018
Jack, S., Kemp, G. J., Bimson, W. E., Calverley, P. M., and Corfield, D. R. (2010). Patterns of brain activity in response to respiratory stimulation in patients with idiopathic hyperventilation (IHV). Adv. Exp. Med. Biol. 669, 341–345. doi: 10.1007/978-1-4419-5692-7_70
Krause, M., Hoffmann, W. E., and Hajos, M. (2003). Auditory sensory gating in hippocampus and reticular thalamic neurons in anesthetized rats. Biol. Psychiatry 53, 244–253. doi: 10.1016/s0006-3223(02)01463-4
Lang, P. J., Bradley, M. M., and Cuthbert, B. N. (2008). International affective picture system (IAPS): Affective ratings of pictures and instruction manual. Gainesville, FL: University of Florida.
Leander, M., Lampa, E., Rask-Andersen, A., Franklin, K., Gislason, T., Oudin, A., et al. (2014). Impact of anxiety and depression on respiratory symptoms. Respir. Med. 108, 1594–1600. doi: 10.1016/j.rmed.2014.09.007
Livermore, N., Butler, J. E., Sharpe, L., McBain, R. A., Gandevia, S. C., and McKenzie, D. K. (2008). Panic attacks and perception of inspiratory resistive loads in chronic obstructive pulmonary disease. Am. J. Respir. Crit. Care Med. 178, 7–12. doi: 10.1164/rccm.200711-1700OC
Locke, G. R. III, Weaver, A. L., Melton, L. J. III, and Talley, N. J. (2004). Psychosocial factors are linked to functional gastrointestinal disorders: a population based nested case-control study. Am. J. Gastroenterol. 99, 350–357. doi: 10.1111/j.1572-0241.2004.04043.x
Logie, S. T., Colrain, I. M., and Webster, K. E. (1998). Source dipole analysis of the early components of the RREP. Brain Topogr. 11, 153–164. doi: 10.1023/A:1022210723257
Masaoka, Y., and Homma, I. (2001). The effect of anticipatory anxiety on breathing and metabolism in humans. Respir. Physiol. 128, 171–177. doi: 10.1016/S0034-5687(01)00278-X
McAlonan, K., Cavanaugh, J., and Wurtz, R. H. (2008). Guarding the gateway to cortex with attention in visual thalamus. Nature 456, 391–394. doi: 10.1038/nature07382
McCathie, H. C., Spence, S. H., and Tate, R. L. (2002). Adjustment to chronic obstructive pulmonary disease: the importance of psychological factors. Eur. Respir. J. 19, 47–53. doi: 10.1183/09031936.02.00240702
McKay, L. C., Evans, K. C., Frackowiak, R. S., and Corfield, D. R. (2003). Neural correlates of voluntary breathing in humans. J. Appl. Physiol. 95, 1170–1178. doi: 10.1152/japplphysiol.00641.2002
Miller, M. R., Hankinson, J., Brusasco, V., Burgos, F., Casaburi, R., Coates, A., et al. (2005). Standardisation of spirometry. Eur. Respir. J. 26, 319–338. doi: 10.1183/09031936.05.00034805
Miskovic, V., and Anderson, A. K. (2018). Modality general and modality specific coding of hedonic valence. Curr. Opin. Behav. Sci. 19, 91–97. doi: 10.1016/j.cobeha.2017.12.012
Ng, T. P., Niti, M., Tan, W. C., Cao, Z., Ong, K. C., and Eng, P. (2007). Depressive symptoms and chronic obstructive pulmonary disease: Effect on mortality, hospital readmission, symptom burden, functional status, and quality of life. Arch. Intern. Med. 167, 60–67. doi: 10.1001/archinte.167.1.60
Parsons, L. M., Egan, G., Liotti, M., Brannan, S., Denton, D., Shade, R., et al. (2001). Neuroimaging evidence implicating cerebellum in the experience of hypercapnia and hunger for air. Proc. Natl. Acad. Sci. U.S.A. 98, 2041–2046. doi: 10.1073/pnas.98.4.2041
Peiffer, C., Costes, N., Herve, P., and Garcia-Larrea, L. (2008). Relief of dyspnea involves a characteristic brain activation and a specific quality of sensation. Am. J. Respir. Crit. Care Med. 177, 440–449. doi: 10.1164/rccm.200612-1774OC
Peiffer, C., Poline, J. B., Thivard, L., Aubier, M., and Samson, Y. (2001). Neural substrates for the perception of acutely induced dyspnea. Am. J. Respir. Crit. Care Med. 163, 951–957. doi: 10.1164/ajrccm.163.4.2005057
Pitts, T., Hegland, K. W., Sapienza, C. M., Bolser, D. C., and Davenport, P. W. (2016). Alterations in oropharyngeal sensory evoked potentials (PSEP). with Parkinson’s disease. Respir. Physiol. Neurobiol. 229, 11–16. doi: 10.1016/j.resp.2016.04.004
Reijnders, T., Troosters, T., Janssens, W., Gosselink, R., Langer, D., Davenport, P. W., et al. (2020). Brain Activations to Dyspnea in Patients With COPD. Front. Physiol. 11:7. doi: 10.3389/fphys.2020.00007
Roest, A. M., Martens, E. J., de Jonge, P., and Denollet, J. (2010). Anxiety and risk of incident coronary heart disease: a meta-analysis. J. Am. Coll. Cardiol. 56, 38–46. doi: 10.1016/j.jacc.2010.03.034
Stoeckel, M. C., Esser, R. W., Gamer, M., Buchel, C., and von Leupoldt, A. (2018). Dyspnea catastrophizing and neural activations during the anticipation and perception of dyspnea. Psychophysiology 55:e13004. doi: 10.1111/psyp.13004
Strang, S., Ekberg-Jansson, A., and Henoch, I. (2014). Experience of anxiety among patients with severe COPD: A qualitative, in-depth interview study. Palliat. Support. Care 12, 465–472. doi: 10.1017/S1478951513000369
Tsai, H. W., Chan, P. Y., von Leupoldt, A., and Davenport, P. W. (2013). The impact of emotion on the perception of graded magnitudes of respiratory resistive loads. Biol. Psychol. 93, 220–224. doi: 10.1016/j.biopsycho.2013.02.008
Tzourio-Mazoyer, N., Landeau, B., Papathanassiou, D., Crivello, F., Etard, O., Delcroix, N., et al. (2002). Automated anatomical labeling of activations in SPM using a macroscopic anatomical parcellation of the MNI MRI single-subject brain. NeuroImage 15, 273–289. doi: 10.1006/nimg.2001.0978
Vlemincx, E., Sprenger, C., and Buchel, C. (2021). Expectation and dyspnoea: the neurobiological basis of respiratory nocebo effects. Eur. Respir. J. 58:2003008. doi: 10.1183/13993003.03008-2020
Vogt, B. A., and Laureys, S. (2005). Posterior cingulate, precuneal and retrosplenial cortices: cytology and components of the neural network correlates of consciousness. Prog. Brain Res. 150, 205–217. doi: 10.1016/S0079-6123(05)50015-3
von Leupoldt, A., and Denutte, Y. (2020). Affective traits, states, and breathlessness. Curr. Opin. Support. Palliat. Care 14, 182–189. doi: 10.1097/SPC.0000000000000506
von Leupoldt, A., Bradley, M. M., Lang, P. J., and Davenport, P. W. (2010a). Neural processing of respiratory sensations when breathing becomes more difficult and unpleasant. Front. Physiol. 1:144. doi: 10.3389/fphys.2010.00144
von Leupoldt, A., Keil, A., Chan, P. Y., Bradley, M. M., Lang, P. J., and Davenport, P. W. (2010b). Cortical sources of the respiratory-related evoked potential. Respir. Physiol. Neurobiol. 170, 198–201. doi: 10.1016/j.resp.2009.12.006
von Leupoldt, A., Taube, K., Henkhus, M., Dahme, B., and Magnussen, H. (2010c). The impact of affective states on the perception of dyspnea in patients with chronic obstructive pulmonary disease. Biol. Psychol. 84, 129–134. doi: 10.1016/j.biopsycho.2009.07.010
von Leupoldt, A., Vovk, A., Bradley, M. M., Keil, A., Lang, P. J., and Davenport, P. W. (2010d). The impact of emotion on respiratory-related evoked potentials. Psychophysiology 47, 579–586. doi: 10.1111/j.1469-8986.2009.00956.x
von Leupoldt, A., Chan, P. Y., Bradley, M. M., Lang, P. J., and Davenport, P. W. (2011). The impact of anxiety on the neural processing of respiratory sensations. NeuroImage 55, 247–252. doi: 10.1016/j.neuroimage.2010.11.050
von Leupoldt, A., Chan, P. Y., Esser, R. W., and Davenport, P. W. (2013). Emotions and neural processing of respiratory sensations investigated with respiratory-related evoked potentials. Psychosom. Med. 75, 244–252. doi: 10.1097/PSY.0b013e31828251cf
von Leupoldt, A., Sommer, T., Kegat, S., Baumann, H. J., Klose, H., Dahme, B., et al. (2008). The unpleasantness of perceived dyspnea is processed in the anterior insula and amygdala. Am. J. Respir. Crit. Care Med. 177, 1026–1032. doi: 10.1164/rccm.200712-1821OC
von Leupoldt, A., Sommer, T., Kegat, S., Baumann, H. J., Klose, H., Dahme, B., et al. (2009a). Dyspnea and pain share emotion-related brain network. NeuroImage 48, 200–206. doi: 10.1016/j.neuroimage.2009.06.015
von Leupoldt, A., Sommer, T., Kegat, S., Eippert, F., Baumann, H. J., Klose, H., et al. (2009b). Down-regulation of insular cortex responses to dyspnea and pain in asthma. Am. J. Respir. Crit. Care Med. 180, 232–238. doi: 10.1164/rccm.200902-0300OC
Yates, J. S., Davenport, P. W., and Reep, R. L. (1994). Thalamocortical projections activated by phrenic nerve afferents in the cat. Neurosci. Lett. 180, 114–118. doi: 10.1016/0304-3940(94)90500-2
Yohannes, A. M., and Alexopoulos, G. S. (2014). Pharmacological treatment of depression in older patients with chronic obstructive pulmonary disease: impact on the course of the disease and health outcomes. Drugs Aging 31, 483–492. doi: 10.1007/s40266-014-0186-0
Keywords: respiratory sensations, functional magnetic resonance imaging, human respiratory sensory gating, positive emotional context, cortical neural substrates
Citation: Chan P-YS, Chang W-P, Cheng C-H, Liu C-Y, von Leupoldt A, Hsu A-L and Wu CW (2022) The impact of emotional context on neural substrates of respiratory sensory gating. Front. Neurosci. 16:1004271. doi: 10.3389/fnins.2022.1004271
Received: 27 July 2022; Accepted: 21 September 2022;
Published: 20 October 2022.
Edited by:
Shakeeb Hassan Moosavi, Oxford Brookes University, United KingdomReviewed by:
Olivia Kate Harrison, University of Otago, New ZealandJianghai Ruan, The Affiliated Hospital of Southwest Medical University, China
Copyright © 2022 Chan, Chang, Cheng, Liu, von Leupoldt, Hsu and Wu. This is an open-access article distributed under the terms of the Creative Commons Attribution License (CC BY). The use, distribution or reproduction in other forums is permitted, provided the original author(s) and the copyright owner(s) are credited and that the original publication in this journal is cited, in accordance with accepted academic practice. No use, distribution or reproduction is permitted which does not comply with these terms.
*Correspondence: Ai-Ling Hsu, YWlsaW5naHN1MTExMEBnbWFpbC5jb20=; Changwei W. Wu, c2xlZXBicmFpbkB0bXUuZWR1LnR3