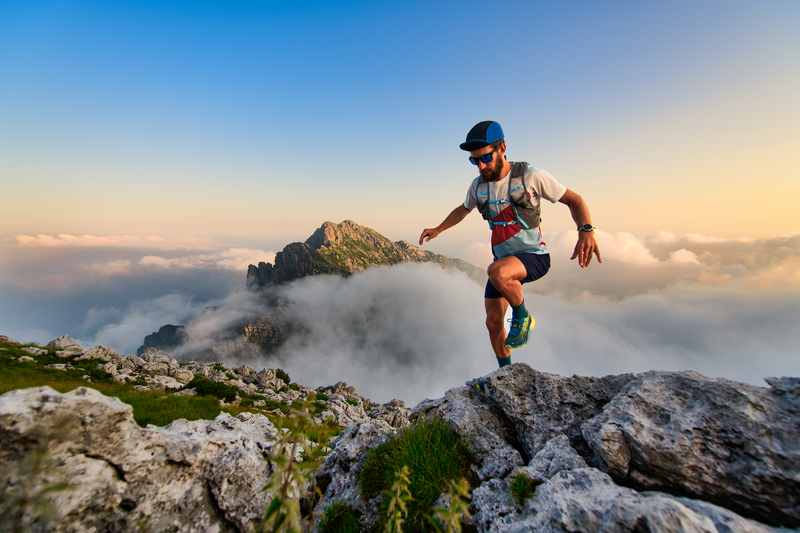
95% of researchers rate our articles as excellent or good
Learn more about the work of our research integrity team to safeguard the quality of each article we publish.
Find out more
EDITORIAL article
Front. Neurosci. , 04 January 2022
Sec. Neurodegeneration
Volume 15 - 2021 | https://doi.org/10.3389/fnins.2021.824145
This article is part of the Research Topic Metabolism in Alzheimer’s Disease View all 11 articles
Editorial on the Research Topic
Metabolism in Alzheimer's Disease
Alzheimer's disease (AD) pathology begins decades before clinical onset of dementia. Amyloid beta (Aβ) generally accumulates first in cognitively normal (CN) individuals, with tau and cognitive abnormalities following (Jack et al., 2013). AD pathologies have been found to correlate and interact with metabolic outcomes in studies spanning numerous experimental paradigms (Mosconi et al., 2009, 2010a,b,c; Mosconi, 2013; Morris et al., 2014a; Wilkins et al., 2014; Swerdlow et al., 2017; Weidling et al., 2020; Wilkins and Swerdlow, 2021).
Metabolic changes are prominent in AD. Fluorodeoxyglucose positron emission tomography (FDG-PET) comparing AD and CN individuals reveals lower glucose levels in the brains of AD patients (Herholz et al., 2002; Mosconi et al., 2010a; Marcus et al., 2014; Suppiah et al., 2019). These findings have led to overwhelming evidence of metabolic deficiencies in AD. Beyond reductions in brain glucose metabolism, mitochondrial dysfunction is observed not only within the brain but also systemically (Parker, 1991; Kish et al., 1992; Cardoso et al., 2004a,b; Morris et al., 2014b; Fisar et al., 2016; Guo et al., 2017; Swerdlow, 2018; Baloyannis, 2019; Chakravorty et al., 2019). More recent genome wide association studies (GWAS) identified risk-associated single nucleotide polymorphisms (SNPs) in genes which function in mitochondrial and metabolic pathways (Lakatos et al., 2010; Swerdlow et al., 2020; Harwood et al., 2021; Wightman et al., 2021). Apolipoprotein E (APOE), the strongest genetic risk factor for sporadic AD, is both central to lipid metabolism and has been found to interact with inherited mitochondrial genes to amplify risk for AD (Carrieri et al., 2001; Andrews et al., 2020; Swerdlow et al., 2020). Moreover, molecular studies of AD brain show an overall reduction in the number of intact mitochondria and mitochondrial DNA (Swerdlow, 2018; Wilkins and Swerdlow, 2021). Thus, mitochondrial function/dysfunction plays a role in protein aggregation, inflammation, and cell death; all events observed in AD. Overall, metabolism and mitochondrial function/dysfunction are strongly associated with AD.
The goal of this Research Topic was to further understand topics in the AD field that broadly focus on metabolic changes in AD and the interaction between metabolism, AD risk factors, and pathologies. These include: the role of genetic risk factors for sporadic AD (such as APOE) in non-cell autonomous functions, the intersection between metabolism and inflammation, the role of metabolism in protein aggregation, how current therapies target metabolism, inflammation, and protein aggregation, the role of novel metabolism/mitochondrial genes identified by GWAS in pathological mechanisms, the role of metabolism in the communication between neurons and glia in AD, how we can leverage existing model systems and develop better models to address questions of brain metabolism in the context of AD.
The articles of this Research Topic highlight a variety of reviews and original research which discuss and address topics of metabolism in AD (Figure 1). Several articles focus on potential AD therapeutics. These include a thorough review on the use of pioglitazone for AD treatment. Pioglitazone is a peroxisome proliferator-activated receptor gamma and alpha (PPAR-γ; PPAR-α) activator. PPARs are transcription factors critical for regulation of many pathways implicated in AD including insulin and glucose metabolism, lipid homeostasis, inflammation, tau and Aβ homeostasis, and mitochondrial function. The review by Saunders et al. discusses pre-clinical and clinical data with longitudinal observational studies revealing a positive impact of pioglitazone in AD and dementia onset in those at risk. The authors also discuss the dose-dependent effects and the caveats revealing future needs for further study into discrepancies found with placebo controlled blinded studies. Norowitz and Querfurth discusses mTOR regulation and drug targeting in AD. The authors focus on nuances for targeting mTOR in therapies including specificity for disease/region/and timing, pleiotropy, personalized therapy with relation to the effects of genetic factors, and the role of lifestyle factors and interventions.
Figure 1. Metabolism in Alzheimer's Disease. Research and review articles discuss the role of hormone response to meals, lifestyle factors on brain function with respect to mTOR, PPAR, PS1, ABAC7, and APOE. All of which regulate glycolysis/glucose and lipid metabolism, mitophagy/autophagy, and mitochondrial function. Created with BioRender.com.
Several other articles discuss the role of specific metabolic pathways in AD. Zhang et al. reviewed the role of glycolytic metabolism in brain resilience in AD. The authors highlight the correlation between glycolytic flux, Aβ, and tau accumulation in humans, where decreased glycolytic function is associated with higher pathologies. In a separate review article, Kyrtata et al. discuss glucose transport in AD with particular focus on glucose transporter (GLUT) deficiencies in AD. The authors discuss the timing of changes to GLUT expression and glucose uptake in brain through rodent studies and how this relates to the timing of onset of Aβ pathology.
An additional review presents the effect of sialometabolism on brain health and AD. Rawal and Zhao discuss the role of sialic acids in brain function and neuroinflammation. The novelty of this pathway in AD is the identification of sialic acid binding Ig-like lectin 3 (CD33) as a genetic risk factor for AD through GWAS.
A separate AD genetic risk factor, ATP binding cassette subfamily A member 7 (ABCA7) was examined. Aikawa et al. used mice with haplodeficiency of ABCA7 to determine the response to immune modulation with lipopolysaccharide (LPS). The authors report that mice deficient in ABCA7 had activated lipid metabolism pathways. This study again highlights the relationship between metabolism and neuroinflammation. Morris et al. describes the role of meal stimulated hormone response through the incretin pathway in cognitive function and brain volume. The authors report that in human AD subjects, a higher meal-stimulated response of insulin, glucose, and peptide tyrosine was observed. Brain volume significantly correlated negatively with insulin, C-peptide, and glucose-dependent insulinotropic polypeptide (GIP). These articles highlight the role of diverse metabolic pathways in brain health, aging, and AD.
A focus on genetic risk factors and metabolism was discussed through a review of APOE in AD by Husain et al. The authors focused on the role of APOE in lipid transport and interactions with AD pathologies (such as tau and Aβ). Other genetic components of AD include mutations in presenilin (PS) in familial AD, and PS has a role in mitochondrial function. Contino et al. examined the role of PS deficiency on neurons and astrocytes derived from mice. Their prior studies showed mitochondrial deficits in mouse embryonic fibroblasts, but in the current study no effects were observed on similar endpoints. This study highlights the importance of model systems used for study.
Mitophagy and autophagy are implicated in AD and are the focus of many therapeutic initiatives. Tran and Reddy discuss deficiencies in autophagy and mitophagy in AD. The authors focus on metabolic drivers of autophagy/mitophagy deficiencies, the influence of aging, and how these pathways influence AD pathologies.
Collectively, the articles in this Research Topic emphasize that the field of brain metabolism in AD is emerging and generating large interest from a therapeutic standpoint. Progress in filling our gap in knowledge on the role of metabolism in AD will advance new therapeutic avenues for this devastating disease.
JM, LW, and HW contributed to the recruitment and editing of the special Research Topic. All authors contributed to writing and editing the editorial.
Support for this project includes the Peg McLaughlin fund (JM and HW), R00AG050490 and R01AG062548 (JM), R01AG075820 (LW), R00AG056600 (HW), and P30AG072973.
The authors declare that the research was conducted in the absence of any commercial or financial relationships that could be construed as a potential conflict of interest.
All claims expressed in this article are solely those of the authors and do not necessarily represent those of their affiliated organizations, or those of the publisher, the editors and the reviewers. Any product that may be evaluated in this article, or claim that may be made by its manufacturer, is not guaranteed or endorsed by the publisher.
Andrews, S. J., Fulton-Howard, B., Patterson, C., McFall, G. P., Gross, A., Michaelis, E. K., et al. (2020). Alzheimer's disease neuroimaging, mitonuclear interactions influence Alzheimer's disease risk. Neurobiol. Aging 87, 138 e7–138 e14. doi: 10.1016/j.neurobiolaging.2019.09.007
Baloyannis, S. J. (2019). Mitochondria in Alzheimer's Disease: An Electron Microscopy Study. Retreived from: https://www.intechopen.com/chapters/67355 (accessed September 25, 2019).
Cardoso, S. M., Proenca, M. T., Santos, S., Santana, I., and Oliveira, C. R. (2004a). Cytochrome c oxidase is decreased in Alzheimer's disease platelets. Neurobiol. Aging 25, 105–110. doi: 10.1016/S0197-4580(03)00033-2
Cardoso, S. M., Santana, I., Swerdlow, R. H., and Oliveira, C. R. (2004b). Mitochondria dysfunction of Alzheimer's disease cybrids enhances Abeta toxicity. J. Neurochem. 89, 1417–1426. doi: 10.1111/j.1471-4159.2004.02438.x
Carrieri, G., Bonafe, M., De Luca, M., Rose, G., Varcasia, O., Bruni, A., et al. (2001). Mitochondrial DNA haplogroups and APOE4 allele are non-independent variables in sporadic Alzheimer's disease. Hum. Genet. 108, 194–198. doi: 10.1007/s004390100463
Chakravorty, A., Jetto, C. T., and Manjithaya, R. (2019). Dysfunctional mitochondria and mitophagy as drivers of Alzheimer's disease pathogenesis. Front. Aging Neurosci. 11:311. doi: 10.3389/fnagi.2019.00311
Fisar, Z., Hroudova, J., Hansikova, H., Spacilova, J., Lelkova, P., Wenchich, L., et al. (2016). Mitochondrial respiration in the platelets of patients with Alzheimer's disease. Curr. Alzheimer Res. 13, 930–941. doi: 10.2174/1567205013666160314150856
Guo, L., Tian, J., and Du, H. (2017). Mitochondrial dysfunction and synaptic transmission failure in Alzheimer's disease. J. Alzheimers. Dis. 57, 1071–1086. doi: 10.3233/JAD-160702
Harwood, J. C., Leonenko, G., Sims, R., Escott-Price, V., Williams, J., and Holmans, P. (2021). Defining functional variants associated with Alzheimer's disease in the induced immune response. Brain Commun. 3:fcab083. doi: 10.1093/braincomms/fcab083
Herholz, K., Salmon, E., Perani, D., Baron, J. C., Holthoff, V., Frolich, L., et al. (2002). Discrimination between Alzheimer dementia and controls by automated analysis of multicenter FDG PET. Neuroimage 17, 302–316. doi: 10.1006/nimg.2002.1208
Jack, C. R., Barrio, J. R., and Kepe, V. (2013). Cerebral amyloid PET imaging in Alzheimer's disease. Acta Neuropathol. 126, 643–657. doi: 10.1007/s00401-013-1185-7
Kish, S. J., Bergeron, C., Rajput, A., Dozic, S., Mastrogiacomo, F., Chang, L. J., et al. (1992). Brain cytochrome oxidase in Alzheimer's disease. J. Neurochem. 59, 776–779. doi: 10.1111/j.1471-4159.1992.tb09439.x
Lakatos, A., Derbeneva, O., Younes, D., Keator, D., Bakken, T., Lvova, M., et al. (2010). Alzheimer's Disease Neuroimaging, Association between mitochondrial DNA variations and Alzheimer's disease in the ADNI cohort. Neurobiol. Aging 31, 1355–1363. doi: 10.1016/j.neurobiolaging.2010.04.031
Marcus, C., Mena, E., and Subramaniam, R. M. (2014). Brain PET in the diagnosis of Alzheimer's disease. Clin. Nucl. Med. 39, e413–e422. doi: 10.1097/RLU.0000000000000547
Morris, J. K., Honea, R. A., Vidoni, E. D., Swerdlow, R. H., and Burns, J. M. (2014b). Is Alzheimer's disease a systemic disease? Biochim. Biophys. Acta 1842, 1340–1349. doi: 10.1016/j.bbadis.2014.04.012
Morris, J. K., Vidoni, E. D., Honea, R. A., and Burns, J. M. (2014a). Impaired glycemia and Alzheimer's disease. Neurobiol. Aging 35:e23. doi: 10.1016/j.neurobiolaging.2014.04.018
Mosconi, L. (2013). Glucose metabolism in normal aging and Alzheimer's disease: Methodological and physiological considerations for PET studies. Clin. Transl. Imaging 1:10. doi: 10.1007/s40336-013-0026-y
Mosconi, L., Berti, V., Glodzik, L., Pupi, A., De Santi, S., and de Leon, M. J. (2010a). Pre-clinical detection of Alzheimer's disease using FDG-PET, with or without amyloid imaging. J. Alzheimers. Dis. 20, 843–854. doi: 10.3233/JAD-2010-091504
Mosconi, L., Berti, V., Swerdlow, R. H., Pupi, A., Duara, R., and de Leon, M. (2010b). Maternal transmission of Alzheimer's disease: prodromal metabolic phenotype and the search for genes. Hum. Genomics 4, 170–193. doi: 10.1186/1479-7364-4-3-170
Mosconi, L., Glodzik, L., Mistur, R., McHugh, P., Rich, K. E., Javier, E., et al. (2010c). Oxidative stress and amyloid-beta pathology in normal individuals with a maternal history of Alzheimer's. Biol. Psychiatry 68, 913–921. doi: 10.1016/j.biopsych.2010.07.011
Mosconi, L., Mistur, R., Switalski, R., Brys, M., Glodzik, L., Rich, K., et al. (2009). Declining brain glucose metabolism in normal individuals with a maternal history of Alzheimer disease. Neurology 72, 513–520. doi: 10.1212/01.wnl.0000333247.51383.43
Parker, W. D. (1991). Cytochrome oxidase deficiency in Alzheimer's disease. Ann. N. Y. Acad. Sci. 640, 59–64. doi: 10.1111/j.1749-6632.1991.tb00191.x
Suppiah, S., Didier, M. A., and Vinjamuri, S. (2019). The who, when, why, and how of PET amyloid imaging in management of Alzheimer's disease-review of literature and interesting images. Diagnostics 9:20065. doi: 10.3390/diagnostics9020065
Swerdlow, R. H. (2018). Mitochondria and mitochondrial cascades in Alzheimer's disease. J. Alzheimers. Dis. 62, 1403–1416. doi: 10.3233/JAD-170585
Swerdlow, R. H., Hui, D., Chalise, P., Sharma, P., Wang, X., Andrews, S. J., et al. (2020). Alzheimer's disease neuroimaging, exploratory analysis of mtDNA haplogroups in two Alzheimer's longitudinal cohorts. Alzheimers. Dement. 16, 1164–1172. doi: 10.1002/alz.12119
Swerdlow, R. H., Koppel, S., Weidling, I., Hayley, C., Ji, Y., and Wilkins, H. (2017). Mitochondria cybrids, aging, Alzheimer's disease. Prog. Mol. Biol. Transl. Sci. 146, 259–302. doi: 10.1016/bs.pmbts.2016.12.017
Weidling, I. W., Wilkins, H. M., Koppel, S. J., Hutfles, L., Wang, X., Kalani, A., et al. (2020). Mitochondrial DNA manipulations affect tau oligomerization. J. Alzheimers. Dis. 77, 149–163. doi: 10.3233/JAD-200286
Wightman, D. P., Jansen, I. E., Savage, J. E., Shadrin, A. A., Bahrami, S., Holland, D., et al. (2021). A genome-wide association study with 1,126,563 individuals identifies new risk loci for Alzheimer's disease. Nat. Genet. 53, 1276–1282. doi: 10.1038/s41588-021-00921-z
Wilkins, H. M., Carl, S. M., and Swerdlow, R. H. (2014). Cytoplasmic hybrid (cybrid) cell lines as a practical model for mitochondriopathies. Redox Biol. 2, 619–631. doi: 10.1016/j.redox.2014.03.006
Keywords: metabolism, mitochondria, Alzheimer's disease, mitophagy, inflammation
Citation: Morris JK, Wood LB and Wilkins HM (2022) Editorial: Metabolism in Alzheimer's Disease. Front. Neurosci. 15:824145. doi: 10.3389/fnins.2021.824145
Received: 29 November 2021; Accepted: 09 December 2021;
Published: 04 January 2022.
Edited and reviewed by: Mark P. Burns, Georgetown University, United States
Copyright © 2022 Morris, Wood and Wilkins. This is an open-access article distributed under the terms of the Creative Commons Attribution License (CC BY). The use, distribution or reproduction in other forums is permitted, provided the original author(s) and the copyright owner(s) are credited and that the original publication in this journal is cited, in accordance with accepted academic practice. No use, distribution or reproduction is permitted which does not comply with these terms.
*Correspondence: Heather M. Wilkins, aHdpbGtpbnNAa3VtYy5lZHU=
Disclaimer: All claims expressed in this article are solely those of the authors and do not necessarily represent those of their affiliated organizations, or those of the publisher, the editors and the reviewers. Any product that may be evaluated in this article or claim that may be made by its manufacturer is not guaranteed or endorsed by the publisher.
Research integrity at Frontiers
Learn more about the work of our research integrity team to safeguard the quality of each article we publish.