- Sorbonne Université, INSERM, CNRS, Institut de la Vision, Paris, France
Bone morphogenetic proteins (BMPs) are secreted factors that contribute to many aspects of the formation of the vertebrate central nervous system (CNS), from the initial shaping of the neural primordium to the maturation of the brain and spinal cord. In particular, the canonical (SMAD1/5/8-dependent) BMP pathway appears to play a key role during neurogenesis, its activity dictating neural stem cell fate decisions and thereby regulating the growth and homeostasis of the CNS. In this mini-review, I summarize accumulating evidence demonstrating how the canonical BMP activity promotes the amplification and/or maintenance of neural stem cells at different times and in diverse regions of the vertebrate CNS, and highlight findings suggesting that this function is evolutionarily conserved.
Introduction
Bone morphogenetic proteins (BMPs) are a subgroup of secreted molecules belonging to the transforming growth factor β (TGF-β) superfamily (Feng and Derynck, 2005; Schmierer and Hill, 2007). BMPs act as homo- or hetero-dimers to induce the formation of a tetrameric complex of pairs of type-1 and type-2 transmembrane serine/threonine kinase receptors, in which constitutively active type-2 receptors phosphorylate type-1 receptors (Schmierer and Hill, 2007). Once activated, type-1 receptors propagate the signal intracellularly through either a canonical or a non-canonical path (Zhang, 2009; Le Dréau and Martí, 2013). In the so-called canonical BMP pathway, they phosphorylate serine residues in the carboxy-terminal tail of SMAD transcription factors (TFs), enabling them to interact with their co-partner SMAD4 to form a heterotrimeric complex with enhanced nuclear stability (Feng and Derynck, 2005; Schmierer and Hill, 2007). This activated SMAD complex thereby recruits co-factors and modulates the transcription of its target genes (Figure 1A), hence regulating different aspects of cell behavior, in particular cell fate decisions (Feng and Derynck, 2005; Schmierer and Hill, 2007).
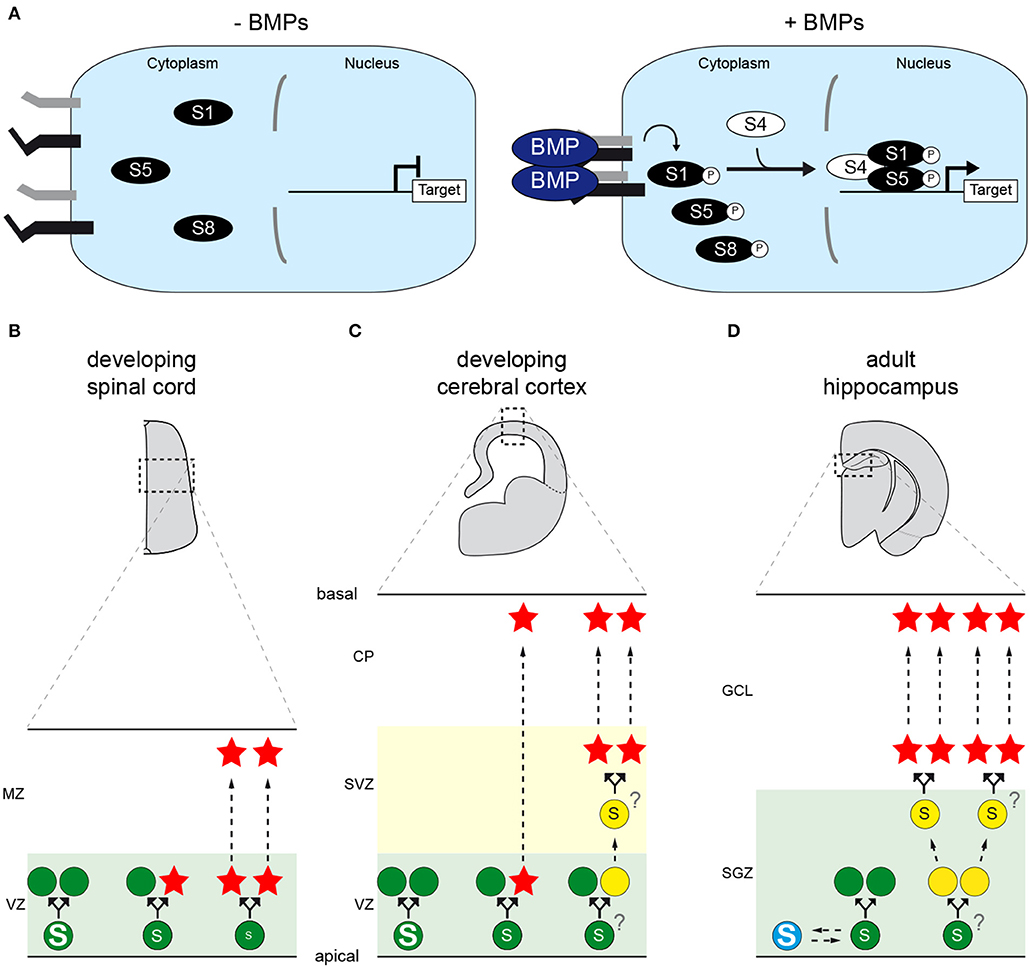
Figure 1. The canonical BMP pathway and its role(s) during vertebrate CNS neurogenesis. (A) Representation of the canonical BMP pathway. Dimers of extracellular BMP ligands induce the formation of a tetrameric complex of transmembrane serine/threonine kinase receptors, in which constitutively active type-2 receptors (black) activate type-1 receptors (gray) through phosphorylation. Type-1 receptors in turn phosphorylate serine residues in the carboxy-terminal tail of SMAD transcription factors (SMAD1, SMAD5 or SMAD8/9), enabling them to interact with their co-partner SMAD4. This activated SMAD complex thereby enters the nucleus, recruits co-factors and regulates the transcription of target genes, such as members of the ID family. The duration of exposure to BMPs and their concentration are therefore converted into different levels and/or durations of transcriptional activity. (B–D) The canonical BMP activity dictates neural stem cell (NSC) divisions during neurogenesis in the vertebrate CNS. (B) During spinal neurogenesis, high levels of SMAD1/5 activity instruct NSCs (green disks) located in the ventricular zone (VZ) to self-amplify, whereas low levels force NSCs to undergo self-consuming divisions, hence depleting their pool and producing neurons (red stars) that will delaminate and migrate toward the mantle zone (MZ). Intermediate levels are proposed to instruct self-renewing neurogenic divisions. (C) During cortical neurogenesis, high levels of SMAD1/5 activity stimulate NSC self-amplification, whereas lower SMAD1/5 levels force them to produce neurons that will migrate to the cortical plate (CP). In this region, neurogenesis can occur either directly or indirectly, the latter case giving rise to transit-amplifying basal progenitors (yellow disks) that undergo self-consuming divisions in the subventricular zone (SVZ). Whether the modes of direct and indirect neurogenesis are instructed by comparable or distinct thresholds of SMAD1/5 activity remains unknown. (D) In the adult mammalian hippocampus, NSCs located in the sub-granular zone (SGZ) of the dentate gyrus are instructed to remain quiescent (blue disk) in response to canonical BMP signaling. Reducing its activity forces the activation of NSCs, which might self-amplify for several rounds before undergoing self-consuming divisions that produce transit-amplifying progenitors. These in turn produce adult-born neurons that will populate the granule cell layer (GCL). Whether the distinct modes of division that activated NSCs undergo are instructed by comparable or distinct thresholds of SMAD1/5 activity remains unknown.
In vertebrates, the BMP family consists of numerous ligands (BMP2/4, BMP5/6/7/8, BMP9/10 and the Growth and differentiation factors GDF5/6/7), as is also the case for type-1 receptors (ALK1, ALK2/ACVR1, ALK3/BMPR1A, and ALK6/BMPR1B), type-2 receptors (BMPR2 and ACTR2A/2B, the latter two being shared with the Activin/TGF-β subfamily), and SMADs (SMAD1, SMAD5, and SMAD8/9) (Schmierer and Hill, 2007). These factors are often expressed in overlapping patterns, show some versatility in their molecular interactions and some degree of functional redundancy (Feng and Derynck, 2005; Miyazono et al., 2010).
The canonical BMP pathway plays multiple roles during development of the vertebrate central nervous system (CNS), ranging from the initial specification of the neural tissue to the maturation its cell types (Le Dréau and Martí, 2013; Hart and Karimi-Abdolrezaee, 2020). Recent evidences suggest that BMPs also participate in regulating homeostasis and repair in the adult CNS (Hart and Karimi-Abdolrezaee, 2020). Here, I specifically review their contribution to CNS neurogenesis, focusing mostly on findings obtained in vivo in amniote models.
Neurogenesis, a Matter of Fate
To function, the vertebrate CNS relies on the coordinated activity of billions of neurons, which are produced through a complex process called neurogenesis. Generated from multipotent neural stem cells (NSCs) or from fate-restricted progenitors, new-born neurons exit the cell cycle and progressively differentiate as they delaminate from the germinal zones and migrate basally, plus tangentially in some regions, toward their final location (Götz and Huttner, 2005). This process occurs mainly during embryonic development but is still on-going in discrete regions of the postnatal and adult CNS (Götz and Huttner, 2005; Grandel and Brand, 2013). The production of the appropriate numbers and subtypes of neurons found in the mature vertebrate CNS is regulated both spatially and temporally and is put in balance with the amplification and/or maintenance of the NSC pool (Götz and Huttner, 2005; Obernier and Alvarez-Buylla, 2019; Fischer and Morin, 2021). This equilibrium is achieved via a tight control of the distinct modes of division that NSCs and neural progenitors can adopt during neurogenesis.
The neural tissue initially consists of a pseudo-stratified epithelial layer (the ventricular zone, VZ) formed by neuroepithelial cells. These primary NSCs contact both the ventricle and the basal lamina, they undergo mitosis near the apical surface and expand their pool through self-amplifying (symmetric proliferative) divisions that produce two daughter NSCs retaining, as far as we know, the full lineage potential of their mother cell (Götz and Huttner, 2005; Cárdenas and Borrell, 2020). This self-amplification drives the tangential growth of the developing CNS and is the only mode of division at play before neurogenesis.
Developmental neurogenesis is initiated when some NSCs switch from self-amplifying to neurogenic divisions, which come in different flavors. NSCs can undergo self-consuming (symmetric/terminal neurogenic) divisions that directly generate two neurons while depleting the NSC pool, as seen in the developing spinal cord (Saade et al., 2013; Le Dréau et al., 2014). They can otherwise undergo two types of self-renewing divisions that maintain the NSC pool intact by producing a new NSC and another daughter cell with a distinct (asymmetric) identity (Cárdenas and Borrell, 2020). During direct neurogenesis, a NSC produces one neuron per division, hence increasing neuron production at a slow pace. During indirect neurogenesis, a NSC instead gives rise to a transient-amplifying progenitor (called basal progenitor, BP) that delaminates from the VZ and divides basally, possibly self-amplifying for several rounds before producing neurons through self-consuming divisions. This mode of indirect neurogenesis is observed in CNS regions undergoing a remarkable radial growth, such as the developing cerebral cortex (Cárdenas and Borrell, 2020). The numbers and subtypes of BPs (intermediate progenitor cells, IPCs; and basal radial glial cells, bRGCs) produced by cortical NSCs (called apical radial glial cells, aRGCs) are strongly correlated to the thickness of the cerebral cortex and to the radial expansion and morphological changes it underwent during evolution (Cárdenas and Borrell, 2020).
In contrast to embryonic NSCs that are permanently cycling, adult NSCs can be found in a reversible state of cell cycle arrest called quiescence. Once activated, adult NSCs appear to divide mostly symmetrically, undergoing either self-amplifying divisions or self-consuming divisions that give rise to transit-amplifying progenitors (called TAPs, TACs or C cells), which in turn produce neurons through self-consuming divisions (Obernier et al., 2018; Obernier and Alvarez-Buylla, 2019).
Therefore, neurogenesis appears to be a matter of fate, whereby the decision of a NSC to undergo self-amplifying, self-consuming, direct or indirect neurogenic divisions has a huge impact on the final neuron output. Throughout the years, many families of intracellular and extracellular actors have been shown to regulate these cell fate decisions during developmental and/or adult neurogenesis (Martynoga et al., 2012; Tiberi et al., 2012; Saade et al., 2018; Obernier and Alvarez-Buylla, 2019; Urbán et al., 2019). One such family corresponds to BMPs.
BuMPing Into Neurogenesis
Modulating canonical BMP signaling has been reported to alter neuron production in numerous contexts. But in most instances, it is still unclear whether these alterations reflect their involvement in instructing progenitor cell specification at early stages, or if they effectively demonstrate a proper role during neurogenesis per se. I thus chose to focus on three tangible examples describing a discrete function of the canonical BMP pathway in regulating NSC fate decisions during neurogenesis: in the spinal cord and cerebral cortex during development, and in the adult hippocampus.
Promoting Stem Cell Amplification During Spinal Neurogenesis
Emerging from the caudal neural tube, the spinal cord represents the most evolutionarily conserved region of the vertebrate CNS. Before neurogenesis, spinal NSCs are progressively patterned into discrete progenitor domains arrayed along the anterior-posterior (AP) and dorsal-ventral (DV) axes (from dorsal to ventral: roof plate, dorsal progenitor domains dP1-dP6; ventral progenitor domains p0-p2, pMN, p3 and floor plate). Their identity and lineage potential are defined by discrete combinations of patterning homeodomain proteins and proneural basic helix-loop-helix (bHLH) TFs (Le Dréau and Martí, 2012; Sagner and Briscoe, 2019). Secreted from dorsal sources (the roof plate and the surrounding ectoderm), BMPs act as morphogens instructing spinal progenitors to adopt dorsal identities through their canonical pathway (Le Dréau and Martí, 2013; Tozer et al., 2013; Zagorski et al., 2017). Beyond its early role in establishing DV patterning, the canonical BMP pathway also plays a discrete function in regulating neurogenesis later on.
Around the onset of neurogenesis in the developing chick spinal cord, SMAD1/5/8 activity is re-deployed along most of the DV axis, coinciding with the emerging expression of several BMP ligands, in particular BMP7, in intermediate and ventral progenitor domains (Le Dréau et al., 2012). High, intermediate and low levels of nuclear SMAD1/5 activity correlate, respectively, to self-amplifying, direct self-renewing and self-consuming divisions (Figure 1B). Gain- and loss-of-functions experiments revealed that SMAD1/5 activity promotes self-amplifying divisions while restraining neurogenic ones. In particular, inhibiting SMAD1/5 activity first causes a premature increase in neurogenic divisions (especially self-consuming ones) at the expense of self-amplifying ones, subsequently depleting the progenitor pool and ultimately reducing the global production of spinal neurons (Le Dréau et al., 2014). In agreement with these findings, reducing the expression of either BMP7, SMAD1, SMAD5 or overexpressing the inhibitory SMAD6 and SMAD7 impairs the generation of spinal neurons in chick (Hazen et al., 2011; Le Dréau et al., 2012, 2014, 2018). The canonical BMP activity is thus crucial to properly balance progenitor expansion and neuron production during chick spinal neurogenesis. Similar phenotypes are observed in mutant mice lacking either BMP7, SMAD1 or SMAD5 (Hazen et al., 2012; Le Dréau et al., 2012), suggesting that this role is evolutionarily conserved in amniotes.
However, this stem cell-promoting role appears to be context-dependent. Indeed, the generation of spinal neurons deriving from progenitors expressing either the proneural bHLH TF ATOH1 (dP1) or high levels of ASCL1 (dP3, dP5, and p2) is highly sensitive to variations in canonical BMP activity. Conversely, the production of spinal neurons deriving from progenitors expressing PTF1a (dP4), NEUROG1 (dP2, dP6-p1), NEUROG2 (pMN) and possibly NEUROG3 (p3) is much less affected by such variations (Hazen et al., 2011, 2012; Le Dréau et al., 2012, 2018; Andrews et al., 2017). A mechanistic explanation for this context-dependent requirement was recently proposed (Le Dréau et al., 2018). Once activated, SMAD1/5 positively regulate the expression of ID2 (Inhibitor of DNA-binding 2), and possibly of other ID members. IDs physically sequester the bHLH E proteins TCF3 and TCF12, hence blocking their ability to dimerize with the proneural TFs. Interestingly, E proteins share the preferential binding of ATOH1 and ASCL1 for CAGSTG DNA motifs (Lin et al., 2010; Castro et al., 2011; Klisch et al., 2011; Lai et al., 2011; Borromeo et al., 2014; Pfurr et al., 2017). By restraining the availability of E proteins, high SMAD1/5 activity thus indirectly impedes ASCL1/ATOH1 from triggering neurogenic divisions, hence promoting self-amplifying divisions. When SMAD1/5 activity is instead reduced, E proteins are released and heterodimerize with ASCL1/ATOH1, forcing spinal progenitors to undergo neurogenic divisions (Le Dréau et al., 2018). Inversely, E proteins show lower affinity for the CADATG motifs preferentially bound by NEUROG1/2/3 (Seo et al., 2007; Madelaine and Blader, 2011; Borromeo et al., 2014). The ability of NEUROG TFs to trigger neurogenic divisions appears to be less dependent on, and even somewhat restrained by, E proteins, making them less sensitive to variations in canonical BMP signaling (Le Dréau et al., 2018). Therefore, the molecular machinery instructing the identity and lineage potential of spinal progenitors dictates their specific requirement on canonical BMP signaling to pace neurogenesis.
Promoting Stem Cell Amplification During Cortical Neurogenesis
Emerging from the pallium, which itself represents the dorsal part of the telencephalon, the cerebral cortex represents the most evolutionarily divergent region of the mammalian CNS, with comparable structures being only found in the amniote clade (Goffinet, 2017; Briscoe and Ragsdale, 2018; García-Moreno and Molnár, 2020). During early mammalian brain development, various BMP ligands (BMP2/4/5/6/7) are expressed and secreted by discrete regions of the dorsal telencephalon, the cortical hem and the choroid plaque, from where they act as morphogens to pattern the dorsal telencephalic midline (Furuta et al., 1997; Grove et al., 1998; Hebert et al., 2002; Hébert and Fishell, 2008). Accordingly, most of these ligands are detected in the developing cerebrospinal fluid known to nurture the survival and proliferation of cortical aRGCs (Lehtinen et al., 2011).
Early in vitro studies performed on explants and dissociated cortical progenitors suggested that BMPs inhibit their proliferation and stimulate neuronal differentiation (Li et al., 1998; Mabie et al., 1999). The phenotypes of brain over-proliferation and premature differentiation, respectively, reported for transgenic mice expressing constitutively active forms of ALK3 or ALK6, suggested a more complex contribution (Panchision et al., 2001). In most instances the phenotypes obtained after deleting one BMP family member were poorly informative, causing either early embryonic lethality (BMP2/4, SMAD1/5) (Winnier et al., 1995; Zhang and Bradley, 1996; Yang et al., 1999; Tremblay et al., 2001), no obvious brain defects (BMP5/6/9, GDF5/6 or FoxG1Cre; BMP4fl/fl) (Kingsley et al., 1992; Storm et al., 1994; Solloway et al., 1998; Hebert et al., 2003; Settle et al., 2003; Ricard et al., 2012), or only mild ones limited to the dorsal midline (GDF7, SMAD8/9, FOXG1Cre;ALK3fl/fl) (Lee et al., 1998; Hebert et al., 2002; Hester et al., 2005).
The first conclusive evidence of the physiological implication of BMP signaling in cortical neurogenesis came from a study revealing that BMP7 null mice are microcephalic (Segklia et al., 2012). The cortices of BMP7 null embryos show a normal organization and layering but are thinner and contain lower numbers of neurons. At mid-corticogenesis, the amount of cortical aRGCs is also reduced, as are their abilities to proliferate and to sustain neurosphere formation in vitro (Segklia et al., 2012). Remarkably, reducing the expression of both SMAD1 and SMAD5 in mouse neural progenitors, using a Nestin:Cre driver triggering recombination at early stages, also causes microcephaly (Najas et al., 2020). These SmadNes mice moreover show an increased production of early-born cortical projection neurons at the expense of late-born ones, which correlates with the premature differentiation and depletion of the pools of cortical progenitors, including both aRGCs and IPCs (Najas et al., 2020). This phenotype, combined with the detection of higher SMAD1/5 activity in mitotic aRGCs than in IPCs, suggested that these two canonical BMP effectors stimulate stem cell maintenance during mammalian corticogenesis.
This idea was further tested in the chick hyperpallium. Whether this dorsal pallial derivative specific of birds should be considered homologous to the mammalian neocortex remains debated (Goffinet, 2017; Briscoe and Ragsdale, 2018; García-Moreno and Molnár, 2020). Nevertheless, neurogenesis in the chick hyperpallium involves progenitor cell types, cellular events and a temporal sequence similar to those described in mammals (Cárdenas and Borrell, 2020). In the developing chick hyperpallium, higher levels of SMAD1/5 activity correlate with aRGC self-amplifying potential (Najas et al., 2020). Inhibiting their expression during early neurogenesis reduces self-amplifying divisions in favor of neurogenic ones, resulting in premature neuronal differentiation as observed in mouse. Conversely, enhancing SMAD1/5 activity stimulates aRGC self-amplifying divisions and restrains their neuronal commitment (Figure 1C). Mechanistically, SMAD1/5 appear to stimulate aRGC amplification during both chick and mouse corticogenesis by positively regulating and recruiting YAP (Najas et al., 2020), a key transcriptional co-factor of the Hippo pathway known to regulate organ size (Yu et al., 2015). Thus, the canonical BMP pathway also promotes stem cell amplification during corticogenesis, and this function is likely conserved throughout the amniote lineage, at least between birds and mammals.
Sustaining Stem Cell Quiescence During Adult Neurogenesis
The ability of the brain to produce new neurons during adult life is apparently conserved throughout the vertebrate lineage, being more widespread in amphibians and fish than in amniotes (Grandel and Brand, 2013). In the mammalian brain, adult NSCs are found in two neurogenic niches: the subgranular zone (SGZ) of the hippocampal dentate gyrus and the ventricular-subventricular zone (V-SVZ) of the lateral telencephalic ventricle walls (Obernier and Alvarez-Buylla, 2019; Urbán et al., 2019). Various BMPs (BMP2/4/5/6/7) and extracellular antagonists (such as NOGGIN and CHORDIN) are found in these two adult neurogenic niches, being expressed by adult NSCs themselves or secreted by their microenvironment, including the choroid plexus, ependymal cells and blood vessels (Urbán and Guillemot, 2014; Obernier and Alvarez-Buylla, 2019). Adult NSCs express various BMP receptors and show nuclear SMAD1/5/8 activity, which demonstrates their responsiveness to BMPs.
The ability of BMPs to regulate adult neurogenesis has been well-characterized in the SGZ of the dentate gyrus, where hippocampal NSCs reside (Urbán et al., 2019). There, inhibiting BMP signaling using intracerebral injections of NOGGIN or through the selective deletion of ALK3 or SMAD4, transiently enhances NSC self-amplification but subsequently causes their depletion, thereby impairing the long-term production of newborn granule neurons (Bonaguidi et al., 2008; Mira et al., 2010). Therefore, BMP signaling actively supports hippocampal NSC quiescence. Apparently, its activity can stimulate a return to quiescence at multiple stages of this neurogenic sequence (Bond et al., 2014).
Mechanistically, maintaining adult hippocampal NSCs in quiescence requires the continuous degradation of ASCL1 protein levels (Urbán et al., 2016). This process is triggered when its dimerizing co-factor E47 is sequestered by IDs, at least ID4 (Blomfield et al., 2019). In agreement with the fact that IDs are direct transcriptional targets of SMAD1/5 in numerous contexts, treatment of hippocampal NSCs in vitro with BMP4 increases the transcript levels of all four ID members, triggering ASCL1 protein degradation (Blomfield et al., 2019). Altogether, these findings support the notion that BMPs promote adult NSC quiescence through their canonical pathway (Figure 1D).
This function might be conserved throughout the vertebrate lineage. Indeed, in the adult zebrafish telencephalon, ID1 is mostly expressed by quiescent NSCs and its overexpression stimulates NSC quiescence in vivo, while its knockdown increases neurogenesis (Rodriguez Viales et al., 2015). The expression of ID1 in these adult zebrafish NSCs depends on an evolutionarily conserved cis-regulatory DNA motif that is controlled by BMPs in a SMAD-dependent manner (Zhang et al., 2020).
Conclusions
There is accumulating evidence that the canonical BMP pathway is a master regulator of neurogenesis in vertebrates, orchestrating this process in the CNS throughout space, time and possibly throughout evolution. As highlighted above, this pathway stimulates the expansion of the NSC pool in both the cerebral cortex and spinal cord during amniote development. In the adult mammalian hippocampus and zebrafish telencephalon, it instead limits the numbers of NSCs by promoting their quiescence. One might thus reason that the canonical BMP pathway plays different roles in the adult CNS or during its development. It might however be argued that this pathway has the same function in all these contexts, considering that its activity always restrains NSCs from progressing into the neurogenic lineage.
Whether the canonical BMP pathway plays the same role in other neurogenic contexts remains subject to debate. For instance, BMP signaling was initially proposed to promote stem cell maintenance in the adult V-SVZ (Lim et al., 2000), but later studies led to divergent conclusions (Bonaguidi et al., 2008; Colak et al., 2008; Silva-Vargas et al., 2016). There are also a few regions of the developing CNS in which BMPs appear to restrain or instead stimulate neurogenesis in a more complex, stage-dependent manner (Alder et al., 1999; Angley et al., 2003; Rios et al., 2004; Krizhanovsky and Ben-Arie, 2006; Jovanovic et al., 2018). These context-dependent effects appear to depend on the identity of the molecular actors involved, both at the level of the BMP pathway and downstream. For instance, the type-1 BMP receptors ALK2/3/6 often show complementary expression patterns and non-redundant effects on neurogenesis, both during development and in adult neurogenic niches (Panchision et al., 2001; Caronia et al., 2010; Mira et al., 2010; Choe et al., 2013). This could imply that the distinct BMP ligands and receptors differentially regulate SMAD1/5/8 activity. On the other hand, NSCs and neural progenitors seem to differentially respond to SMAD1/5/8 activity on the basis of intrinsic molecular differences, such as the identity of the proneural bHLH TFs that they express (Le Dréau et al., 2018).
Therefore, fully understanding the role(s) played by the canonical BMP pathway during neurogenesis will require addressing the discrete contribution of each ligand and downstream effector. Given the diversity of members existing at all levels of the BMP signaling cascade, this represents a laborious task. Such hard work might however offer the possibility to harness the ability of the canonical BMP pathway to regulate neurogenesis for regenerative medicine.
Author Contributions
The author confirms being the sole contributor of this work and has approved it for publication.
Funding
This work was supported by Grants from IHU FOReSIGHT (ANR-18-IAHU-01) and LabEx LIFESENSES (ANR-10-LABX-65).
Conflict of Interest
The author declares that the research was conducted in the absence of any commercial or financial relationships that could be construed as a potential conflict of interest.
Publisher's Note
All claims expressed in this article are solely those of the authors and do not necessarily represent those of their affiliated organizations, or those of the publisher, the editors and the reviewers. Any product that may be evaluated in this article, or claim that may be made by its manufacturer, is not guaranteed or endorsed by the publisher.
Acknowledgments
I wish to thank Jean Livet and the members of the lab for insightful discussions. I apologize to those whose work could not be cited due to space constraints.
References
Alder, J., Lee, K. J., Jessell, T. M., and Hatten, M. E. (1999). Generation of cerebellar granule neurons in vivo by transplantation of BMP-treated neural progenitor cells. Nat. Neurosci. 2, 535–540. doi: 10.1038/9189
Andrews, M. G., del Castillo, L. M., Ochoa-Bolton, E., Yamauchi, K., Smogorzewski, J., and Butler, S. J. (2017). BMPs direct sensory interneuron identity in the developing spinal cord using signal-specific not morphogenic activities. Elife 6:e30647. doi: 10.7554/eLife.30647.033
Angley, C., Kumar, M., Dinsio, K. J., Hall, A. K., and Siegel, R. E. (2003). Signaling by bone morphogenetic proteins and Smad1 modulates the postnatal differentiation of cerebellar cells. J. Neurosci. 23, 260–268. doi: 10.1523/JNEUROSCI.23-01-00260.2003
Blomfield, I. M., Rocamonde, B., del Mar Masdeu, M., Mulugeta, E., Vaga, S., van den Berg, D. L. C., et al. (2019). Id4 promotes the elimination of the pro-activation factor ascl1 to maintain quiescence of adult hippocampal stem cells. Elife 8, 1–26. doi: 10.7554/eLife.48561
Bonaguidi, M. A., Peng, C. Y., McGuire, T., Falciglia, G., Gobeske, K. T., Czeisler, C., et al. (2008). Noggin expands neural stem cells in the adult hippocampus. J. Neurosci. 28, 9194–9204. doi: 10.1523/JNEUROSCI.3314-07.2008
Bond, A. M., Peng, C. Y., Meyers, E. A., Mcguire, T., Ewaleifoh, O., and Kessler, J. A. (2014). BMP signaling regulates the tempo of adult hippocampal progenitor maturation at multiple stages of the lineage. Stem Cells 32, 2201–2214. doi: 10.1002/stem.1688
Borromeo, M. D., Meredith, D. M., Castro, D. S., Chang, J. C., Tung, K. C., Guillemot, F., et al. (2014). A transcription factor network specifying inhibitory versus excitatory neurons in the dorsal spinal cord. Development 141, 2803–2812. doi: 10.1242/dev.105866
Briscoe, S. D., and Ragsdale, C. W. (2018). Homology, neocortex, and the evolution of developmental mechanisms. Science. 362, 190–193. doi: 10.1126/science.aau3711
Cárdenas, A., and Borrell, V. (2020). Molecular and cellular evolution of corticogenesis in amniotes. Cell. Mol. Life Sci. 77, 1435–1460. doi: 10.1007/s00018-019-03315-x[10.1007/s00018-019-03315-x]
Caronia, G., Wilcoxon, J., Feldman, P., and Grove, E. A. (2010). Bone morphogenetic protein signaling in the developing telencephalon controls formation of the hippocampal dentate gyrus and modifies fear-related behavior. J. Neurosci. 30, 6291–6301. doi: 10.1523/JNEUROSCI.0550-10.2010
Castro, D. S., Martynoga, B., Parras, C., Ramesh, V., Pacary, E., Johnston, C., et al. (2011). A novel function of the proneural factor Ascl1 in progenitor proliferation identified by genome-wide characterization of its targets. Genes Dev. 25, 930–945. doi: 10.1101/gad.627811
Choe, Y., Kozlova, A., Graf, D., and Pleasure, S. J. (2013). Bone morphogenic protein signaling is a major determinant of dentate development. J. Neurosci. 33, 6766–6775. doi: 10.1523/JNEUROSCI.0128-13.2013
Colak, D., Mori, T., Brill, M. S., Pfeifer, A., Falk, S., Deng, C., et al. (2008). Adult neurogenesis requires Smad4-mediated bone morphogenic protein signaling in stem cells. J. Neurosci. 28, 434–446. doi: 10.1523/JNEUROSCI.4374-07.2008
Feng, X. H., and Derynck, R. (2005). Specificity and versatility in tgf-beta signaling through Smads. Annu. Rev. Cell. Dev. Biol. 21, 659–693. doi: 10.1146/annurev.cellbio.21.022404.142018
Fischer, E., and Morin, X. (2021). Fate restrictions in embryonic neural progenitors. Curr. Opin. Neurobiol. 66, 178–185. doi: 10.1016/j.conb.2020.10.014
Furuta, Y., Piston, D. W., and Hogan, B. L. M. (1997). Bone morphogenetic proteins (BMPs) as regulators of dorsal forebrain development. Development 124, 2203–2212. doi: 10.1242/dev.124.11.2203
García-Moreno, F., and Molnár, Z. (2020). Variations of telencephalic development that paved the way for neocortical evolution. Prog. Neurobiol. 194:e101865. doi: 10.1016/j.pneurobio.2020.101865
Goffinet, A. M.. (2017). The evolution of cortical development: the synapsid-diapsid divergence. Development 144, 4061–4077. doi: 10.1242/dev.153908
Götz, M., and Huttner, W. B. (2005). The cell biology of neurogenesis. Nat. Rev. Mol. Cell Biol. 6, 777–788. doi: 10.1038/nrm1739
Grandel, H., and Brand, M. (2013). Comparative aspects of adult neural stem cell activity in vertebrates. Dev. Genes Evol. 223, 131–147. doi: 10.1007/s00427-012-0425-5
Grove, E. A., Tole, S., Limon, J., Yip, L. W., and Ragsdale, C. W. (1998). The hem of the embryonic cerebral cortex is defined by the expression of multiple Wnt genes and is compromised in Gli3-deficient mice. Development 125, 2315–2325. doi: 10.1242/dev.125.12.2315
Hart, C. G., and Karimi-Abdolrezaee, S. (2020). Bone morphogenetic proteins: new insights into their roles and mechanisms in CNS development, pathology and repair. Exp. Neurol. 334:e113455. doi: 10.1016/j.expneurol.2020.113455
Hazen, V. M., Andrews, M. G., Umans, L., Crenshaw 3rd, E. B., Zwijsen, A., and Butler, S. J. (2012). BMP receptor-activated Smads confer diverse functions during the development of the dorsal spinal cord. Dev Biol. 367, 216–27. doi: 10.1016/j.ydbio.2012.05.014
Hazen, V. M., Phan, K. D., Hudiburgh, S., and Butler, S. J. (2011). Inhibitory Smads differentially regulate cell fate specification and axon dynamics in the dorsal spinal cord. Dev. Biol. 356, 566–575. doi: 10.1016/j.ydbio.2011.06.017
Hébert, J. M., and Fishell, G. (2008). The genetics of early telencephalon patterning: some assembly required. Nat. Rev. Neurosci. 9, 678–685. doi: 10.1038/nrn2463
Hebert, J. M., Hayhurst, M., Marks, M. E., Kulessa, H., Hogan, B. L., and McConnell, S. K. (2003). BMP ligands act redundantly to pattern the dorsal telencephalic midline. Genesis 35, 214–219. doi: 10.1002/gene.10183
Hebert, J. M., Mishina, Y., and McConnell, S. K. (2002). BMP signaling is required locally to pattern the dorsal telencephalic midline. Neuron 35, 1029–1041. doi: 10.1016/S0896-6273(02)00900-5
Hester, M., Thompson, J. C., Mills, J., Liu, Y., El-Hodiri, H. M., and Weinstein, M. (2005). Smad1 and Smad8 function similarly in mammalian central nervous system development. Mol. Cell. Biol. 25, 4683–4692. doi: 10.1128/MCB.25.11.4683-4692.2005
Jovanovic, V. M., Salti, A., Tilleman, H., Zega, K., Jukic, M. M., Zou, H., et al. (2018). BMP/SMAD pathway promotes neurogenesis of midbrain dopaminergic neurons in vivo and in human induced pluripotent and neural stem cells. J. Neurosci. 38, 1662–1676. doi: 10.1523/JNEUROSCI.1540-17.2018
Kingsley, D. M., Bland, A. E., Grubber, J. M., Marker, P. C., Russell, L. B., Copeland, N. G., et al. (1992). The mouse short ear skeletal morphogenesis locus is associated with defects in a bone morphogenetic member of the TGF beta superfamily. Cell 71, 399–410. doi: 10.1016/0092-8674(92)90510-J
Klisch, T. J., Xi, Y., Flora, A., Wang, L., Li, W., and Zoghbi, H. Y. (2011). In vivo Atoh1 targetome reveals how a proneural transcription factor regulates cerebellar development. Proc. Natl. Acad. Sci. USA 108, 3288–3293. doi: 10.1073/pnas.1100230108
Krizhanovsky, V., and Ben-Arie, N. (2006). A novel role for the choroid plexus in BMP-mediated inhibition of differentiation of cerebellar neural progenitors. Mech. Dev. 123, 67–75. doi: 10.1016/j.mod.2005.09.005
Lai, H. C., Klisch, T. J., Roberts, R., Zoghbi, H. Y., and Johnson, J. E. (2011). In vivo neuronal subtype-specific targets of Atoh1 (Math1) in dorsal spinal cord. J. Neurosci. 31, 10859–10871. doi: 10.1523/JNEUROSCI.0445-11.2011
Le Dréau, G., Escalona, R., Fueyo, R., Herrera, A., Martínez, J. D., Usieto, S., et al. (2018). E proteins sharpen neurogenesis by modulating proneural bHLH transcription factors' activity in an E-box-dependent manner. Elife 7, 1–29. doi: 10.7554/eLife.37267
Le Dréau, G., Garcia-Campmany, L., Rabadan, M. A., Ferronha, T., Tozer, S., Briscoe, J., et al. (2012). Canonical BMP7 activity is required for the generation of discrete neuronal populations in the dorsal spinal cord. Development 139, 259–268. doi: 10.1242/dev.074948
Le Dréau, G., and Martí, E. (2012). Dorsal-ventral patterning of the neural tube: a tale of three signals. Dev. Neurobiol. 72, 1471–1481. doi: 10.1002/dneu.22015
Le Dréau, G., and Martí, E. (2013). The multiple activities of BMPs during spinal cord development. Cell. Mol. Life Sci. 70, 4293–4305. doi: 10.1007/s00018-013-1354-9
Le Dréau, G., Saade, M., Gutiérrez-Vallejo, I., and Martí, E. (2014). The strength of SMAD1/5 activity determines the mode of stem cell division in the developing spinal cord. J. Cell Biol. 204, 591–605. doi: 10.1083/jcb.201307031
Lee, K. J., Mendelsohn, M., and Jessell, T. M. (1998). Neuronal patterning by BMPs: a requirement for GDF7 in the generation of a discrete class of commissural interneurons in the mouse spinal cord. Genes Dev. 12, 3394–3407. doi: 10.1101/gad.12.21.3394
Lehtinen, M. K., Zappaterra, M. W., Chen, X., Yang, Y. J., Hill, A. D., Lun, M., et al. (2011). The cerebrospinal fluid provides a proliferative niche for neural progenitor cells. Neuron 69, 893–905. doi: 10.1016/j.neuron.2011.01.023
Li, W., Cogswell, C. A., and LoTurco, J. J. (1998). Neuronal differentiation of precursors in the neocortical ventricular zone is triggered by BMP. J. Neurosci. 18, 8853–8862. doi: 10.1523/JNEUROSCI.18-21-08853.1998
Lim, D. A., Tramontin, A. D., Trevejo, J. M., Herrera, D. G., Garcia-Verdugo, J. M., and Alvarez-Buylla, A. (2000). Noggin antagonizes BMP signaling to create a niche for adult neurogenesis. Neuron 28, 713–726. doi: 10.1016/S0896-6273(00)00148-3
Lin, Y. C., Jhunjhunwala, S., Benner, C., Heinz, S., Welinder, E., Mansson, R., et al. (2010). A global network of transcription factors, involving E2A, EBF1 and Foxo1, that orchestrates B cell fate. Nat. Immunol. 11, 635–643. doi: 10.1038/ni.1891
Mabie, P. C., Mehler, M. F., and Kessler, J. A. (1999). Multiple roles of bone morphogenetic protein signaling in the regulation of cortical cell number and phenotype. J. Neurosci. 19, 7077–7088. doi: 10.1523/JNEUROSCI.19-16-07077.1999
Madelaine, R., and Blader, P. (2011). A cluster of non-redundant Ngn1 binding sites is required for regulation of deltaA expression in zebrafish. Dev. Biol. 350, 198–207. doi: 10.1016/j.ydbio.2010.10.025
Martynoga, B., Drechsel, D., and Guillemot, F. (2012). Molecular control of neurogenesis: a view from the mammalian cerebral cortex. Cold Spring Harb. Perspect. Biol. 4:8359. doi: 10.1101/cshperspect.a008359
Mira, H., Andreu, Z., Suh, H., Lie, D. C., Jessberger, S., Consiglio, A., et al. (2010). Signaling through BMPR-IA regulates quiescence and long-term activity of neural stem cells in the adult hippocampus. Cell Stem Cell 7, 78–89. doi: 10.1016/j.stem.2010.04.016
Miyazono, K., Kamiya, Y., and Morikawa, M. (2010). Bone morphogenetic protein receptors and signal transduction. J. Biochem. 147, 35–51. doi: 10.1093/jb/mvp148
Najas, S., Pijuan, I., Esteve-Codina, A., Usieto, S., Martinez, J. D., Zwijsen, A., et al. (2020). A SMAD1/5-YAP signalling module drives radial glia self-amplification and growth of the developing cerebral cortex. Development. 147:dev.187005. doi: 10.1242/dev.187005
Obernier, K., and Alvarez-Buylla, A. (2019). Neural stem cells: Origin, heterogeneity and regulation in the adult mammalian brain. Development. 146:dev156059. doi: 10.1242/dev.156059
Obernier, K., Cebrian-Silla, A., Thomson, M., Parraguez, J. I., Anderson, R., Guinto, C., et al. (2018). Adult neurogenesis is sustained by symmetric self-renewal and differentiation. Cell Stem Cell 22, 221–234.e8. doi: 10.1016/j.stem.2018.01.003
Panchision, D. M., Pickel, J. M., Studer, L., Lee, S. H., Turner, P. A., Hazel, T. G., et al. (2001). Sequential actions of BMP receptors control neural precursor cell production and fate. Genes Dev. 15, 2094–2110. doi: 10.1101/gad.894701
Pfurr, S., Chu, Y. H., Bohrer, C., Greulich, F., Beattie, R., Mammadzada, K., et al. (2017). The E2A splice variant E47 regulates the differentiation of projection neurons via p57(KIP2) during cortical development. Development 144, 3917–3931. doi: 10.1242/dev.145698
Ricard, N., Ciais, D., Levet, S., Subileau, M., Mallet, C., Zimmers, T. A., et al. (2012). BMP9 and BMP10 are critical for postnatal retinal vascular remodeling. Blood 119, 6162–6171. doi: 10.1182/blood-2012-01-407593
Rios, I., Alvarez-Rodriguez, R., Marti, E., and Pons, S. (2004). Bmp2 antagonizes sonic hedgehog-mediated proliferation of cerebellar granule neurones through Smad5 signalling. Development 131, 3159–3168. doi: 10.1242/dev.01188
Rodriguez Viales, R., Diotel, N., Ferg, M., Armant, O., Eich, J., Alunni, A., et al. (2015). The helix-loop-helix protein Id1 controls stem cell proliferation during regenerative neurogenesis in the adult zebrafish telencephalon. Stem Cells 33, 892–903. doi: 10.1002/stem.1883
Saade, M., Blanco-Ameijeiras, J., Gonzalez-Gobartt, E., and Marti, E. (2018). A centrosomal view of CNS growth. Development 145:dev.170613. doi: 10.1242/dev.170613
Saade, M., Gutiérrez-Vallejo, I., LeDréau, G., Rabadán, M. A., Miguez, D. G., Buceta, J., et al. (2013). Sonic hedgehog signaling switches the mode of division in the developing nervous system. Cell Rep. 4, 492–503. doi: 10.1016/j.celrep.2013.06.038
Sagner, A., and Briscoe, J. (2019). Establishing neuronal diversity in the spinal cord: a time and a place. Development. 146:dev.182154. doi: 10.1242/dev.182154
Schmierer, B., and Hill, C. S. (2007). TGFbeta-SMAD signal transduction: molecular specificity and functional flexibility. Nat. Rev. Mol. Cell Biol. 8, 970–982. doi: 10.1038/nrm2297
Segklia, A., Seuntjens, E., Elkouris, M., Tsalavos, S., Stappers, E., Mitsiadis, T. A., et al. (2012). Bmp7 regulates the survival, proliferation, and neurogenic properties of neural progenitor cells during corticogenesis in the mouse. PLoS ONE. 7:e34088. doi: 10.1371/journal.pone.0034088
Seo, S., Lim, J. W., Yellajoshyula, D., Chang, L. W., and Kroll, K. L. (2007). Neurogenin and NeuroD direct transcriptional targets and their regulatory enhancers. EMBO J. 26, 5093–5108. doi: 10.1038/sj.emboj.7601923
Settle, S. H., Rountree, R. B., Sinha, A., Thacker, A., Higgins, K., and Kingsley, D. M. (2003). Multiple joint and skeletal patterning defects caused by single and double mutations in the mouse Gdf6 and Gdf5 genes. Dev. Biol. 254, 116–130. doi: 10.1016/S0012-1606(02)00022-2
Silva-Vargas, V., Maldonado-Soto, A. R., Mizrak, D., Codega, P., and Doetsch, F. (2016). Age-dependent niche signals from the choroid plexus regulate adult neural stem cells. Cell Stem Cell 19, 643–652. doi: 10.1016/j.stem.2016.06.013
Solloway, M. J., Dudley, A. T., Bikoff, E. K., Lyons, K. M., Hogan, B. L., and Robertson, E. J. (1998). Mice lacking Bmp6 function. Dev. Genet 22, 321–339. doi: 10.1002/(SICI)1520-6408(1998)22:4andlt;321::AID-DVG3andgt;3.0.CO;2-8
Storm, E. E., Huynh, T. V., Copeland, N. G., Jenkins, N. A., Kingsley, D. M., and Lee, S. J. (1994). Limb alterations in brachypodism mice due to mutations in a new member of the TGF beta-superfamily. Nature 368, 639–643. doi: 10.1038/368639a0
Tiberi, L., Vanderhaeghen, P., and van den Ameele, J. (2012). Cortical neurogenesis and morphogens: diversity of cues, sources and functions. Curr. Opin. Cell. Biol. 24, 269–276. doi: 10.1016/j.ceb.2012.01.010
Tozer, S., Le Dréau, G., Marti, E., and Briscoe, J. (2013). Temporal control of BMP signalling determines neuronal subtype identity in the dorsal neural tube. Development. 140, 1467–74. doi: 10.1242/dev.090118
Tremblay, K. D., Dunn, N. R., and Robertson, E. J. (2001). Mouse embryos lacking Smad1 signals display defects in extra-embryonic tissues and germ cell formation. Development 128, 3609–3621. doi: 10.1242/dev.128.18.3609
Urbán, N., Blomfield, I. M., and Guillemot, F. (2019). Quiescence of adult mammalian neural stem cells: a highly regulated rest. Neuron 104, 834–848. doi: 10.1016/j.neuron.2019.09.026
Urbán, N., and Guillemot, F. (2014). Neurogenesis in the embryonic and adult brain: same regulators, different roles. Front. Cell. Neurosci. 8:e00396. doi: 10.3389/fncel.2014.00396
Urbán, N., van den Berg, D. L. C., Forget, A., Andersen, J., Demmers, J. A. A., Hunt, C., et al. (2016). Return to Quiescence of mouse neural stem cells by degradation of a proactivation protein. Science. 353, 292–295. doi: 10.1126/science.aaf4802
Winnier, G., Blessing, M., Labosky, P. A., and Hogan, B. L. (1995). Bone morphogenetic protein-4 is required for mesoderm formation and patterning in the mouse. Genes Dev 9, 2105–2116. doi: 10.1101/gad.9.17.2105
Yang, X., Castilla, L. H., Xu, X., Li, C., Gotay, J., Weinstein, M., et al. (1999). Angiogenesis defects and mesenchymal apoptosis in mice lacking SMAD5. Development 126, 1571–1580. doi: 10.1242/dev.126.8.1571
Yu, F. X., Zhao, B., and Guan, K. L. (2015). Hippo pathway in organ size control, tissue homeostasis, and cancer. Cell 163, 811–828. doi: 10.1016/j.cell.2015.10.044
Zagorski, M., Tabata, Y., Brandenberg, N., Lutolf, M. P., Tkačik, G., Bollenbach, T., et al. (2017). Decoding of position in the developing neural tube from antiparallel morphogen gradients. Science. 356, 1379–1383. doi: 10.1126/science.aam5887
Zhang, G., Ferg, M., Lübke, L., Takamiya, M., Beil, T., Gourain, V., et al. (2020). Bone morphogenetic protein signaling regulates Id1-mediated neural stem cell quiescence in the adult zebrafish brain via a phylogenetically conserved enhancer module. Stem Cells 38, 875–889. doi: 10.1002/stem.3182
Zhang, H., and Bradley, A. (1996). Mice deficient for BMP2 are nonviable and have defects in amnion/chorion and cardiac development. Development 122, 2977–2986. doi: 10.1242/dev.122.10.2977
Keywords: bone morphogenetic proteins, SMAD transcription factors, neurogenesis, neural stem cells, self-amplifying divisions, neurogenic divisions
Citation: Le Dréau G (2022) BuMPing Into Neurogenesis: How the Canonical BMP Pathway Regulates Neural Stem Cell Divisions Throughout Space and Time. Front. Neurosci. 15:819990. doi: 10.3389/fnins.2021.819990
Received: 22 November 2021; Accepted: 28 December 2021;
Published: 27 January 2022.
Edited by:
Takuma Kumamoto, Tokyo Metropolitan Institute of Medical Science, JapanReviewed by:
Fernando Garcia-Moreno, Achucarro Basque Center for Neuroscience, SpainTadashi Nomura, Kyoto Prefectural University of Medicine, Japan
Copyright © 2022 Le Dréau. This is an open-access article distributed under the terms of the Creative Commons Attribution License (CC BY). The use, distribution or reproduction in other forums is permitted, provided the original author(s) and the copyright owner(s) are credited and that the original publication in this journal is cited, in accordance with accepted academic practice. No use, distribution or reproduction is permitted which does not comply with these terms.
*Correspondence: Gwenvael Le Dréau, Z3dlbnZhZWwubGUtZHJlYXUmI3gwMDA0MDtpbnNlcm0uZnI=