- 1Psychiatric and Neurodevelopmental Genetics Unit, Center for Genomic Medicine, Massachusetts General Hospital, Boston, MA, United States
- 2Department of Psychiatry, Harvard Medical School, Boston, MA, United States
- 3Stanley Center for Psychiatric Research, Broad Institute of MIT and Harvard, Cambridge, MA, United States
- 4Department of Cellular and Physiological Sciences, Faculty of Medicine, The University of British Columbia, Vancouver, BC, Canada
Prenatal alcohol exposure can impact virtually all body systems, resulting in a host of structural, neurocognitive, and behavioral abnormalities. Among the adverse impacts associated with prenatal alcohol exposure are alterations in immune function, including an increased incidence of infections and alterations in immune/neuroimmune parameters that last throughout the life-course. Epigenetic patterns are also highly sensitive to prenatal alcohol exposure, with widespread alcohol-related alterations to epigenetic profiles, including changes in DNA methylation, histone modifications, and miRNA expression. Importantly, epigenetic programs are crucial for immune system development, impacting key processes such as immune cell fate, differentiation, and activation. In addition to their role in development, epigenetic mechanisms are emerging as attractive candidates for the biological embedding of environmental factors on immune function and as mediators between early-life exposures and long-term health. Here, following an overview of the impact of prenatal alcohol exposure on immune function and epigenetic patterns, we discuss the potential role for epigenetic mechanisms in reprogramming of immune function and the consequences for health and development. We highlight a range of both clinical and animal studies to provide insights into the array of immune genes impacted by alcohol-related epigenetic reprogramming. Finally, we discuss potential consequences of alcohol-related reprogramming of immune/neuroimmune functions and their effects on the increased susceptibility to mental health disorders. Overall, the collective findings from animal models and clinical studies highlight a compelling relationship between the immune system and epigenetic pathways. These findings have important implications for our understanding of the biological mechanisms underlying the long-term and multisystem effects of prenatal alcohol exposure, laying the groundwork for possible novel interventions and therapeutic strategies to treat individuals prenatally exposed to alcohol.
Reprogramming of Physiological Systems by Prenatal Alcohol Exposure
Alcohol (ethanol) exposure in utero can have numerous adverse effects on a developing fetus. In humans, prenatal alcohol exposure (PAE) can result in Fetal Alcohol Spectrum Disorder (FASD), which refers to the broad spectrum of structural, neurocognitive, and behavioral abnormalities or deficits that can occur following PAE (Astley and Clarren, 2000). The magnitude of these effects is variable and depends on factors such as timing and level of maternal alcohol use, physiological and genetic background, and a host of environmental factors including overall maternal health and nutrition (Pollard, 2007). Despite the innate variability of these moderating factors, children across the entire spectrum of FASD display long-term cognitive and neurobehavioral alterations, including neurocognitive impairment (i.e., cognition, learning, memory, and executive function), impaired self-regulation (i.e., attention, impulsivity, behavioral regulation, stress responsiveness, mood/affect, and sleep), and deficits in adaptive functioning (i.e., communication, social behavior, and activities of daily living) (Streissguth and O’Malley, 2000; Astley et al., 2009; Doyle and Mattson, 2015; Lynch et al., 2015; Carter et al., 2016; Panczakiewicz et al., 2016). Taken together, these findings highlight the complex reprogramming effects of PAE on neurobehavioral, neurobiological, and physiological systems. However, the mechanisms underlying the pervasive and multisystem impacts of alcohol are not yet fully understood.
The Developmental Origins of Health and Disease (DOHaD) hypothesis provides an important framework to interpret both the transient and long-term effects of PAE (Hellemans et al., 2010). This hypothesis developed through mounting evidence indicating that early-life exposures or events can have a long-lasting impact on adult health outcomes (Barker and Osmond, 1986; Barker et al., 1989, 1993; Wadhwa et al., 2009). Importantly, the DOHaD hypothesis suggests that early environments can exert their influence on long-term health through the mechanism of fetal programming, by which early life experiences shape development of neurobiological and physiological systems, altering their function over the lifespan. However, additional research is needed to fully uncover the mechanisms that drive the reprogramming of physiological systems.
Disruption of immune development has been recognized as a “pathway to pathology”, impacting risk for both childhood and adult diseases (Dietert, 2014). Specifically, the field of developmental immunotoxicity has identified a wide range of agents capable of immune disruption or programming of the immune system with long-term health consequences, which include diet, environmental, chemical, and physical factors such as UV radiation, and notably, alcohol/drug exposure, as well as psychological factors (Dietert, 2014). While the molecular mechanisms underlying these effects have yet to be firmly established, epigenetic mechanisms are emerging as attractive candidates for the biological embedding of environmental factors on immune function, as they may link external stimuli and physiological systems to influence health and behavior into later life (Kobor and Weinberg, 2011; Yuen et al., 2011; Shulha et al., 2013; Fujii et al., 2021). In the present review, we address the impact of PAE on immune function and epigenetic patterns, highlighting a potential role for epigenetic mechanisms in the reprogramming of immune function and risk for mental health disorders. These links are conceptually summarized in Figure 1.
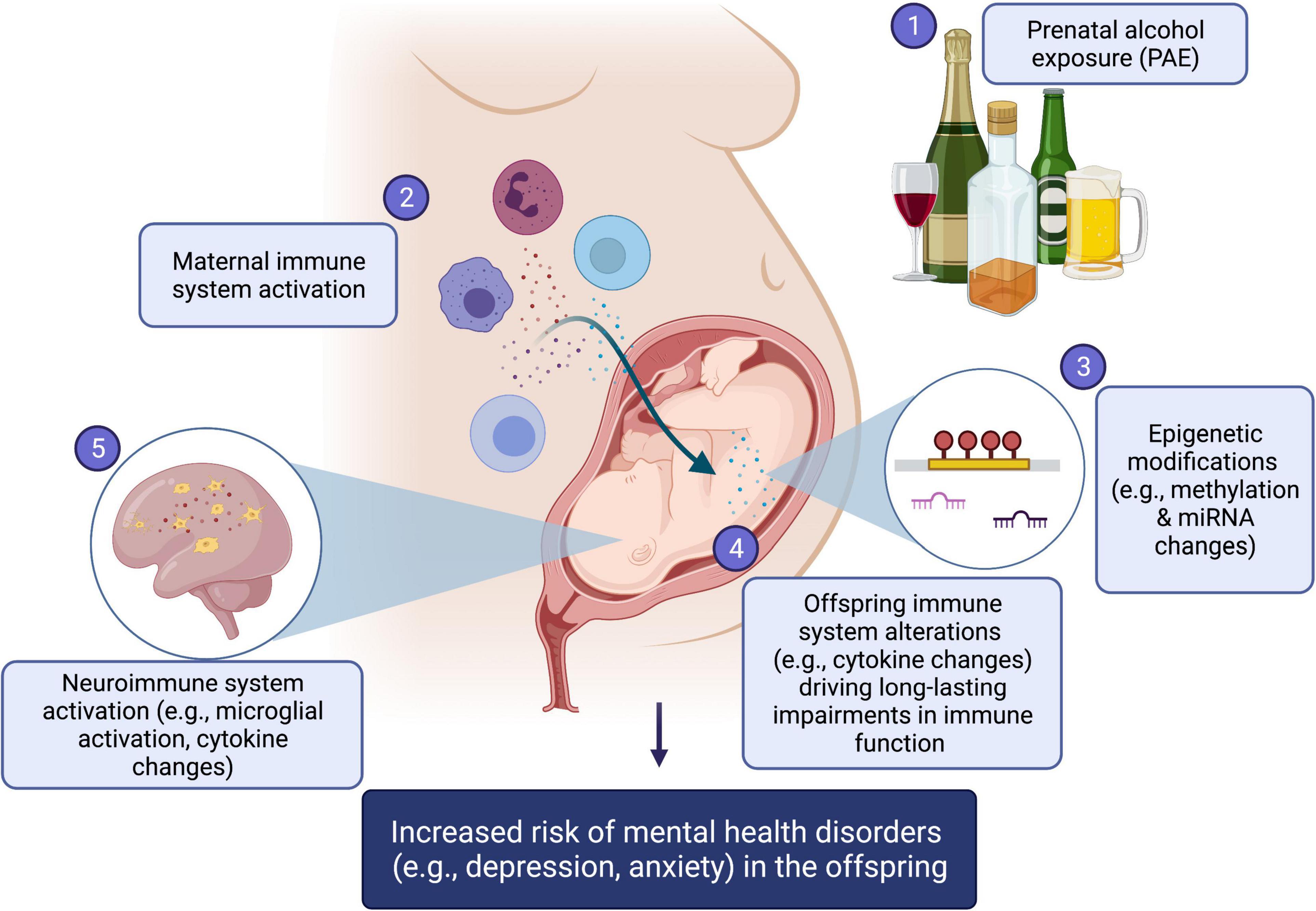
Figure 1. Summary figure. (1) Prenatal alcohol exposure (PAE) can result in (2) maternal immune system activation, altering the fine cytokine balance during pregnancy, which in turn impacts the developing immune system of the fetus. In turn, both direct effects of PAE and alcohol-induced maternal immune activation may result changes in (3) epigenetic mechanisms, which include alterations to DNA methylation levels, histone modification patterns, and miRNAs expression levels. These epigenetic changes are likely important mechanistic drivers of (4) life-long impairments in offspring immune function and (5) neuroimmune system alterations, including microglial activation and central cytokine changes. Together, offspring central and peripheral immune system activation, by way of epigenetic changes, are hypothesized as driving, at least in part, the increased risk of mental health conditions, such as depression and anxiety, in alcohol-exposed offspring. Created with BioRender.com.
Impact of Prenatal Alcohol Exposure on Immune and Neuroimmune Function
Clinical data examining alcohol-induced alterations in immune competence in children and adults with FASD remain somewhat limited [reviewed in Bodnar and Weinberg (2013), Reid et al. (2019)]. However, one of the earliest and most consistently clinically demonstrated effects of PAE is increased infection rates. Specifically, early investigations identified that children with Fetal Alcohol Syndrome (FAS), at the more severe end of the FASD spectrum, have a higher incidence of major and minor infections, including recurrent otitis media, upper respiratory tract infections, urinary tract infections, sepsis, pneumonia, and acute gastroenteritis (Johnson et al., 1981; Ammann et al., 1982; Church and Gerkin, 1988). In addition, decreased eosinophil and neutrophil cell counts in alcohol-exposed compared to unexposed children, and decreased leukocyte response to mitogens [(Johnson et al., 1981); reviewed in Gottesfeld and Abel (1991)] were observed. Maternal alcohol consumption has also been shown to result in a 5–7-fold increase in the rates of chorioamnionitis (inflammation of the chorion, amnion, and placenta) (Rickert et al., 1998; Aly et al., 2008), which is a common cause of preterm birth (Galinsky et al., 2013), and associated with higher rates of poor neurological outcomes due to inflammation and placental perfusion defects (Pappas et al., 2014). Recently, Gauthier et al. (2004) also reported that very low birth weight newborns exposed to alcohol in utero have a 15-fold higher incidence of early-onset sepsis compared to matched controls. Furthermore, high levels of maternal drinking (binge drinking), specifically during the second trimester, has also been shown to increase the risk of infection by approximately 4-fold, compared to that of unexposed newborns (Gauthier et al., 2005). Similarly, Libster et al. (2015) have reported increased rates of adverse infection outcomes in young children (<2 years of age) with PAE who were hospitalized for acute respiratory infections.
Several studies have also explored the link between PAE and atopic disorders (mainly dermatitis, eczema and asthma). Whereas PAE is associated with an increased risk of dermatitis (Linneberg et al., 2004; Carson et al., 2012), the risk of eczema is less clear, as there are conflicting reports of PAE increasing risk of eczema (Wada et al., 2016) and having no associations with eczema (Apfelbacher et al., 2011; Shaheen et al., 2014). By contrast, there are consistent reports of a lack of association between PAE and asthma, with studies including a range of developmental time points and alcohol exposure levels (Oleson et al., 1998; Yuan et al., 2004; Magnus et al., 2014; Shaheen et al., 2014; Wada et al., 2016).
Although the mechanisms underlying the immune-teratogenic effects of PAE remain unclear, alcohol consumption in adulthood has been shown to increase circulating cytokine levels (Crews et al., 2006; He and Crews, 2008), with chronic alcohol consumption during pregnancy increasing levels of key cytokines in both the fetus and mother (Ahluwalia et al., 2000). Importantly, as maternal cytokine induction can have a considerable impact on fetal development (Deverman and Patterson, 2009), alcohol-induced maternal immune system activation may drive some of the adverse developmental outcomes that occur following PAE. Indeed, our work has shown that alcohol consumption during pregnancy alters the maternal cytokine milieu through activation and/or inhibition of key cytokine networks (Bodnar et al., 2018). Importantly, these distinct maternal immune profiles predict neurodevelopmental status, distinguishing children with high risk or resilience to alcohol-induced neurodevelopmental delay1 from typically developing children (Bodnar et al., 2018). We have also shown that child cytokine networks are themselves disrupted following PAE and that network activity patterns again differ based on neurodevelopmental status of the child (i.e., typical development vs. neurodevelopmental delay) (Bodnar et al., 2020), further linking cytokine disruptions to altered developmental outcomes. Finally, while studies investigating immune outcomes of PAE in older individuals is limited, there is evidence for increased rates of atopic conditions and elevated lymphocyte counts in adolescents with PAE (Oleson et al., 1998). However, despite clear evidence of immune alterations and associated developmental alterations following PAE, further studies are needed to elucidate the mechanism(s) underlying the impact of alcohol exposure on immune function. Importantly, in utero alcohol exposure appears to induce long-lasting changes in immune function; however, most immune cells themselves are not long-lived, and as such, mechanistic investigations will be required to fill this gap.
Animal model experiments have allowed for more in-depth explorations of the immune disturbances associated with PAE, and in particular, have allowed for investigations into the impact of PAE on the neuroimmune system. Work from a range of animal models has shown that alcohol exposure generally increases cytokine production within the brain, a marker of neuroimmune activation (reviewed in Table 1). In third trimester equivalent exposure models, alcohol increased cytokine levels in the cerebellum, cortex, and hippocampus (Drew et al., 2015; Topper et al., 2015). Our laboratory has identified alterations in cytokine levels in the brain following alcohol exposure throughout gestation (i.e., first and second trimester equivalent), with increased cytokine levels observed in the hippocampus, and prefrontal cortex but decreased cytokine levels observed in the hypothalamus (Bodnar et al., 2016). Despite inherent differences among the various models, such as method and timing of alcohol administration, species, and cytokine detection method, the overall concordance of these findings highlights that neuroinflammation may be a cross-cutting feature in both FASD and animal models of PAE.
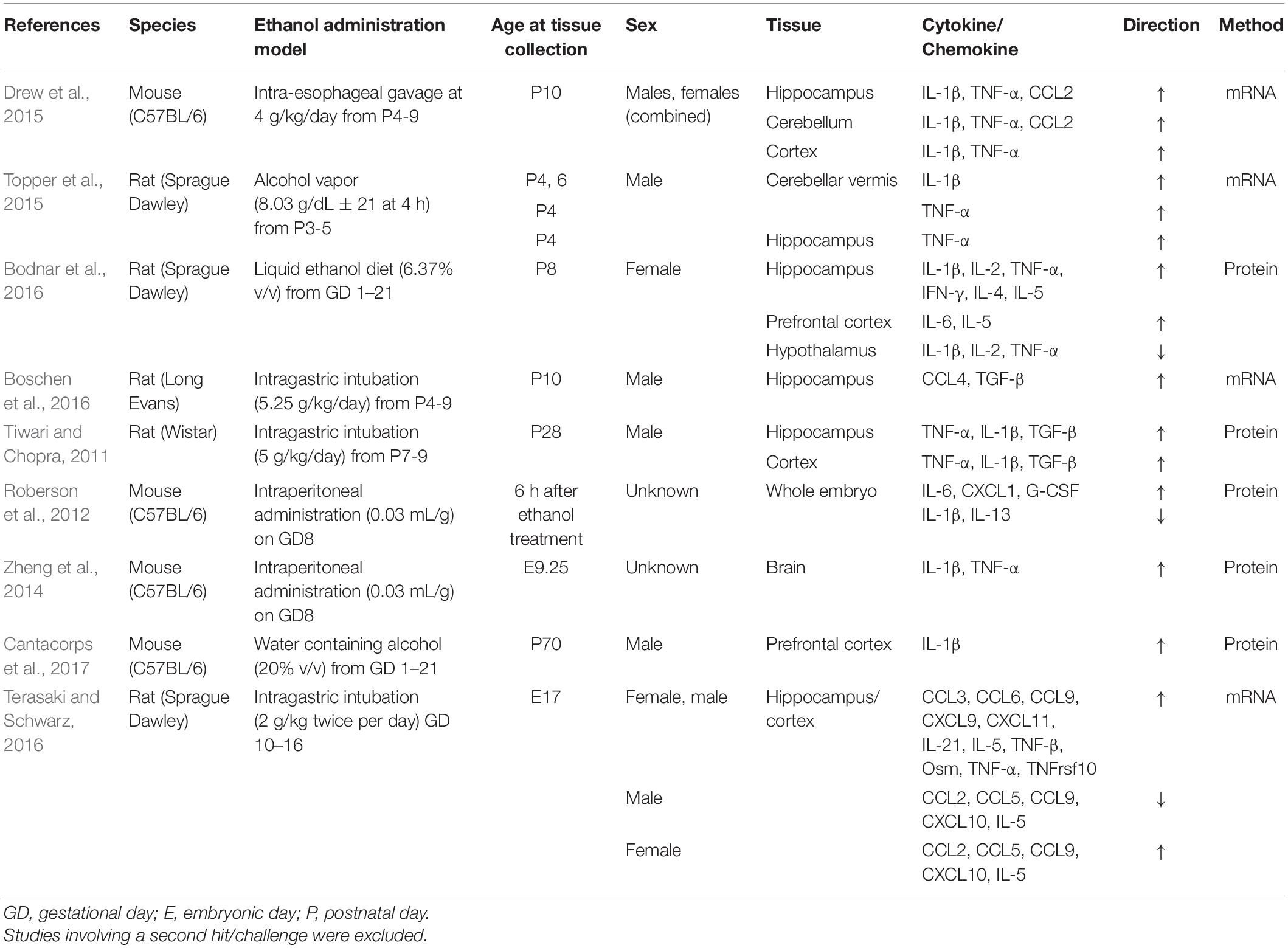
Table 1. Studies showing the impact of prenatal/early postnatal (third trimester equivalent) alcohol exposure on central cytokine levels.
In addition to alterations in cytokine levels, alcohol exposure can alter microglial levels and/or activational status (Fernandez-Lizarbe et al., 2009; Kane et al., 2011; Drew et al., 2015; Topper et al., 2015; Boschen et al., 2016; Ruggiero et al., 2018; Chastain et al., 2019; Gursky et al., 2020) [reviewed in Wilhelm and Guizzetti (2015), Mahnke et al. (2019), Kane and Drew (2021)]. During early development, microglia, resident macrophages of the CNS, exist in an activated state. In this state, microglia produce cytokines (Fujita et al., 1981; Ling and Wong, 1993) and contribute to brain development through their important roles in neurobiological processes, including phagocytosis of newborn neurons (Marin-Teva et al., 2004), synaptic pruning and maturation (Paolicelli et al., 2011), remodeling of synaptic circuits (Tremblay et al., 2011), and synaptic plasticity (Xavier et al., 2014). As a result, alterations in microglial populations and activational status may be an important mechanism through which alcohol exposure impacts early brain development. By weaning, microglia transition to a quiescent state and remain relatively inactive throughout adulthood, unless activated by injury or immune challenge (Ling and Wong, 1993). However, alcohol exposure may impair/delay the transition of microglia to a quiescent state (Drew et al., 2015) and as such, may result in heightened responses to challenges such as infection, with potential consequences for behavior and cognition [reviewed in Bilbo and Schwarz (2012)]. Thus, microglia are uniquely poised to retain an immunological memory of early-life insults, such as exposure to alcohol, due the long-lived nature of these cells amid a more ephemeral immune cell background. Nevertheless, the mechanisms underlying the impact of alcohol exposure on cytokine levels and microglial activation are not fully known. Based on evidence of fetal programming by PAE and from other models where epigenetic alterations of microglia are associated with neuroinflammation (Jovicic et al., 2013; Chauhan et al., 2015), we propose that epigenetic influences may be a critical link, tying together alcohol exposure, long-term impacts on immune function and subsequent health outcomes.
Epigenetic Mechanisms Bridge Early-Life Environments and Long-Term Health
Epigenetics refers to modifications of DNA and/or its regulatory factors that mediate the accessibility of DNA, which can, in turn, modulate gene expression and cellular functions without changes to underlying genomic sequences (Bird, 2007). These regulatory factors include histone modifications, non-coding RNA (ncRNA), and direct DNA modifications, such as methylation and hydroxymethylation. In general, epigenetic patterns are closely associated with cellular specification and differentiation, highlighting their role in the regulation of cellular functions (Ziller et al., 2013). As each cellular subtype is closely associated with a characteristic epigenomic landscape that provides long-term stability to its identity, cell type is the main driver of stable epigenetic patterns. However, environmental stimuli can also influence epigenetic patterns throughout the genome, albeit with subtler effects than ontogenic profiles. These mechanisms rely on an apparent paradox between the stability of cell-specific profiles and plasticity in response to external cues to modulate both short- and long-term epigenetic regulation (Boyce and Kobor, 2015; Aristizabal et al., 2020). Overall, epigenetic patterns act in concert to fine-tune the cellular response to external stimuli and regulate cellular functions [reviewed in Allis and Jenuwein (2016)]. Importantly, emerging evidence suggests that epigenetic patterns, such as DNA methylation, may mediate the relationship between environmental insults and chronic disease, highlighting a potentially crucial role in our understanding of the biological embedding of early-life exposures (Fujii et al., 2021).
Prenatal Alcohol Exposure Alters Epigenetic Programs
The initial evidence that epigenetic mechanisms might be involved in programming of physiological function by PAE originated from studies of gene expression. Genome-wide alterations to gene expression patterns occur in the brains of fetal, neonatal, and adult animals following PAE, highlighting the possibility that the effects of alcohol may shift early developmental trajectories and lead to persistent alterations in adulthood (Hard et al., 2005; Green et al., 2007; Zhou et al., 2011b). One recent example comes from a study identifying large-scale alterations to neuroimmune gene networks of the olfactory system (Gano et al., 2020). Our work has shown that PAE animals show changes in the brain’s transcriptome both under basal conditions and in response to an immune challenge, supporting the reprogramming of neuroendocrine and neuroimmune function by alcohol (Lussier et al., 2015). Studies investigating possible epigenetic mechanisms of developmental alcohol exposure followed closely behind the initial gene expression studies, identifying widespread alterations to epigenetic profiles in both central nervous system and peripheral tissues, including DNA methylation, histone modifications, and miRNA expression [reviewed in Lussier et al. (2017)]. These findings have highlighted a potential role for epigenetic factors in the reprogramming of neurobiological functions by PAE in both animal models of PAE and clinical cohorts of individuals with FASD. Although relatively few epigenome-wide studies have been performed to date, several have identified alcohol-induced alterations to genes involved in immune function (Liu et al., 2009; Zhou et al., 2011a; Khalid et al., 2014; Laufer et al., 2015; Marjonen et al., 2015; Chater-Diehl et al., 2016; Portales-Casamar et al., 2016; Frey et al., 2018; Lussier et al., 2018a,b; Sharp et al., 2018; Cobben et al., 2019; Table 2). These findings provide further evidence that the immune deficits observed following PAE may be linked to changes in epigenetic patterns.
Epigenetic Programs Play a Key Role in Immune System Development and Function
Epigenetic programs are crucial to the broader development and function of the immune system, playing important roles in the regulation of immune cell development and identity as well as neuroinflammatory processes (Garden, 2013). Given the vital role of epigenetic mechanisms in the regulation of cell fate, it is perhaps not surprising that epigenetic mechanisms play an important part in the developmental cascades associated with immune cell differentiation (Obata et al., 2015). In particular, epigenetic processes regulate stem-cell properties of progenitor cells and become increasingly specialized as immune cells progress through lineage commitment. The vital importance of these patterns is exemplified by the deficits in immune development and function in animals lacking components of the epigenetic machinery, including DNA methyltransferase (DNMTs) and histone deacetylases (HDAC) (Lee et al., 2001; Akimova et al., 2012; Dovey et al., 2013; Obata et al., 2015). miRNAs also play a key role in immune system development, displaying unique expression signatures in different cellular subtypes, including microglia, granulocytes, and monocytes, which likely help modulate their specific functions and developmental trajectories (Taganov et al., 2006; Johnnidis et al., 2008; Hashimi et al., 2009; Schmidl et al., 2018). Moreover, the activation state of immune effectors relies on epigenetic mechanisms, particularly histone modifications, to induce the phenotypic alterations necessary for their rapid response to pathogens (Aung et al., 2006; Nicodeme et al., 2010; Satoh et al., 2010; Obata et al., 2015; Zhang and Cao, 2019). Of particular relevance to the current review, the epigenetic profiles of microglia are closely linked to neuroinflammatory processes, reflective of their role in the immune response of neural tissues (Jovicic et al., 2013; Chauhan et al., 2015; Kaminska et al., 2016). Importantly, the epigenetic responsivity of microglia to both external and internal signals may play a crucial role in modulating the inflammatory status of the brain, which has important ramifications for neurobiological functions (Cheray and Joseph, 2018). Overall, immune system development occurs in parallel with epigenetic changes in immune cells, which are responsive to both environmental and biological cues.
Several lines of evidence also suggest that developmental exposures can influence epigenetic patterns within the developing organism to potentially alter immune function and susceptibility to neurobiological deficits later in life (Hinz et al., 2012). For instance, animal model studies suggest that increased maternal care can alter IL-10 expression and DNA methylation in microglial cells to diminish morphine-induced addictive behavior (Schwarz et al., 2011; Wang et al., 2018). Such findings highlight the role of early life experiences in shaping developmental trajectories within the immune system and suggest that epigenetic mechanisms could play an integral role in the reprogramming of immune functions by PAE.
Epigenetic Mechanisms May Influence the Immune Alterations Associated With Prenatal Alcohol Exposure
Studies investigating epigenetic mechanisms involved in PAE effects have also identified alterations to cytokines, chemokines, and signaling pathways involved in the cellular response to immune molecules. Table 2 outlines the findings from genome-wide studies of PAE, highlighting epigenetic alterations to genes involved in immune response and regulation (immune gene annotations obtained from immport.org, May 2021) (Bhattacharya et al., 2018). Of particular note, results from work on a cohort of children with FASD reported that DNA methylation levels in buccal epithelial cells showed alterations in HLA-DPB1, a component of the major histocompatibility complex previously associated with rheumatoid arthritis (Raychaudhuri et al., 2012; Liu et al., 2013; Portales-Casamar et al., 2016; Lussier et al., 2018b). Importantly, both evidence from animal models (Zhang X. et al., 2012) and reports from a recent informal health survey in adults with FASD (Himmelreich et al., 2020) indicate that the incidence of rheumatoid arthritis is higher following PAE. While the findings of altered HLA-DPB1 were identified in a peripheral tissue not involved in immune modulation, they may provide insight into changes in global epigenetic patterns associated with altered immune profiles in children with FASD. Members of the complement system, a key immune pathway that promotes inflammatory responses to combat infection (Sarma and Ward, 2011), also appear across multiple studies, from animal models to clinical cohorts of individuals with FASD. For instance, CFP displays alterations in both mouse and human embryonic cells exposed to alcohol, while C1R and C1S show alterations in the peripheral tissue of individuals with FASD (Khalid et al., 2014; Portales-Casamar et al., 2016).
Through the use of animal models, where one can assess the brain directly, several candidate genes involved in the reprogramming of neuroimmune functions have been identified as being altered following PAE, particularly genes within the TNF receptor (TNFRSF1A, TNFRSF8, TNFRSF10D, TNFRSF11B, and TNFRSF19) and CXC/CC chemokine receptor families (CCR3, CCRL2, and CXCR7) (Liu et al., 2009; Khalid et al., 2014; Portales-Casamar et al., 2016). Several studies also reported alterations to epigenetic patterns in members of the TNF (TNF, TNFSF10, and TNFSF14) and CXC/CC chemokine (CCBP2, CCL11, CXCL2, and CXCL12) families themselves (Table 2). Together, almost every study of epigenetic patterns following PAE identified alterations to genes involved in cytokine/chemokine/interleukin expression and signaling, showing clear parallels and links to changes in circulating levels of these markers described earlier. Importantly, several immune system genes were common across model organisms and human populations, including CFP (Liu et al., 2009; Khalid et al., 2014), CRH7 (Liu et al., 2009; Hellemans et al., 2010), CSF1 (Khalid et al., 2014; Frey et al., 2018), FGFR2 (Khalid et al., 2014; Lussier et al., 2018b), ITGAL (Khalid et al., 2014; Portales-Casamar et al., 2016), PGFRA (Liu et al., 2009; Sharp et al., 2018), PTHLH (Liu et al., 2009; Laufer et al., 2015), and VIPR2 (Liu et al., 2009; Laufer et al., 2015), which may point to potential pathways for mechanistic follow-up studies to assess whether they may be suitable targets for intervention.
Beyond genes directly involved in immune system functioning, several immune-related transcription factors also show differential epigenetic profiles following PAE and could play a role in the altered genomic response to immune signals. Of note, PPARG (PPAR-γ), a transcription factor that promotes anti-inflammatory processes (Le Menn and Neels, 2018), shows differential DNA methylation and expression in the brain following PAE and has been previously implicated in the prevention of alcohol-induced cell death (Kane et al., 2011; Khalid et al., 2014). The polycomb group proteins are also altered by PAE and have been implicated in the deficits caused by PAE, as they play a key role in modulating the properties of neural stem cells and immune cell progenitors (Aloia et al., 2013; Veazey et al., 2013). Taken together, epigenetic alterations to immune genes provides a potential mechanistic link between PAE and long-lasting immune system dysfunction.
In addition to DNA and protein-based epigenetic alterations, several differentially expressed miRNAs known to be critical regulators of neuroimmune function have also been identified in PAE models, including miR-9, -21, -153, -155, and -335 (Sathyan et al., 2007; Wang et al., 2009; Balaraman et al., 2012; Jovicic et al., 2013; Ignacio et al., 2014; Qi et al., 2014). For example, alcohol exposure increases miR-155 expression, which typically promotes the secretion of pro-inflammatory cytokines by microglial cells following Toll-like receptor activation (Cardoso et al., 2012; Ignacio et al., 2014). By contrast, alcohol decreases miR-21 expression, a neuroprotectant that suppresses Fas ligand levels, which may lead to greater vulnerability to microglial-induced cell death (Sathyan et al., 2007; Balaraman et al., 2012; Zhang L. et al., 2012). These data suggest that developmental alcohol exposure may shift the balance of different neuroimmune cell types in the brain, as well as the cytokines they produce, setting the stage for more robust neuroinflammatory responses. This possibility represents an important consideration for epigenetic studies, as cell type proportions are the major drivers of epigenetic patterns and must be taken into consideration when analyzing these types of data. To this point, a recent single-cell RNA-sequencing study of GD14.5 mice exposed to binge-levels of alcohol showed that PAE altered the cell cycle status of microglia in the ventricular zone, suggesting that alcohol may shift the developmental trajectories of neuroimmune pathways and mechanisms (Salem et al., 2021). These results also highlight the importance of timing in the study of PAE, as identifying the developmental trajectories of key neurobiological pathways may provide profound insight into the mechanisms that drive the effects of PAE on neurodevelopmental and physiological outcomes. Taken together, these findings suggest a complex interplay between the immune system and epigenomic profiles, which may, at least partially, influence the neurobiological and neuroinflammatory profiles observed following PAE, and, in the future, may enable us to describe unique immune and neuroinflammatory signatures in FASD.
Epigenetic Dysregulation of Immune Function – The Missing Link Between Prenatal Alcohol Exposure and Mental Health Disorders?
Individuals with FASD experience high rates of mental health problems. In the general population, approximately 20% of individuals experience a mental health disorder (Nestler et al., 2002), whereas 90% of individuals with FASD have a mental health disorder, with anxiety and depression among the most common (Famy et al., 1998; O’Connor et al., 2002; Pei et al., 2011). Although the molecular mechanisms underlying this increased vulnerability in alcohol-exposed individuals remains unclear, alterations in the epigenetic regulation of immune genes resulting in abnormal immune/neuroimmune functioning has been implicated in the pathophysiology of a number of mental health disorders (Alam et al., 2017). For instance, individuals diagnosed with major depressive disorder (MDD) show increases in circulating leukocytes and proinflammatory cytokine production [reviewed in Hodes et al. (2015)], with higher childhood levels of IL-6 and C-reactive protein (CRP) potentially predating the onset of depression (Khandaker et al., 2014). Importantly, these differences are linked to epigenetic alterations, as blood cells from individuals with a lifetime history of depression show alterations to DNA methylation in IL-6 and CRP (Uddin et al., 2011). Beyond these gene-specific epigenetic alterations, recent evidence from human studies shows that epigenetic risk scores for higher inflammatory status, measured through CRP levels, are associated with increased internalizing and externalizing behaviors in children (Barker et al., 2018).
Taken together, these findings suggest a correlation between alterations in immune function and increased risk of mental health disorders, which may be mediated, at least in part, through epigenetic alterations. While this connection has yet to be specifically evaluated following PAE, the high prevalence of mental disorders in individuals with FASD concomitant with lasting alterations to immune function and epigenetic programs highlight a need for future mechanistic studies that explore this complex bidirectional relationship.
Conclusion and Future Directions
As a whole, it is becoming increasingly apparent that a multisystem approach is needed to gain a better understand of mechanisms underlying the teratogenic effects of alcohol. To that end, we propose that immune disturbances arising as a result of in utero alcohol exposure may have long-term consequences extending beyond direct immune functions (e.g., protection from pathogens) to include an impact on mental health and that this may be occurring through the mechanism of epigenetics. However, the findings from epigenetic studies must be interpreted with caution, as the vast majority are correlative rather than causative in nature. As such, they do not provide a direct link between molecular mechanisms and disease and further studies are needed prior to making inferences as to causality. Nevertheless, the findings from epigenetic studies have provided important insights into potential regulatory mechanisms of immune reprogramming and may represent future targets to investigate the molecular underpinnings of alcohol-induced deficits.
Interactions between the gut microbiome, immune system, and brain are now emerging as potential moderators of neural function and potentially disease, although their connection to neuroepigenetics and neuroinflammation remain mostly unknown (Alenghat et al., 2013; Stilling et al., 2014; Carabotti et al., 2015; Petra et al., 2015; Mohajeri et al., 2018; Morais et al., 2021). Moving forward, and with this multisystem approach in mind, it will be important that future research also consider the impact of PAE on the gut-brain-immune axis (Louwies et al., 2019), as to date, there is no research in this area. It is, however, known that chronic alcohol consumption results in compromised gut-barrier function and increased rates of dysbiosis (Keshavarzian et al., 2001; Bull-Otterson et al., 2013) and as a result, in utero alcohol exposure would be expected to have an impact on the immature, developing gut. Moreover, dysbiosis during early life is linked to a proinflammatory state and an increased incidence of inflammatory-related diseases in adulthood (Yan et al., 2011; Hooper et al., 2012; Tamburini et al., 2016). Alterations in the microbiome may also confer increased risk of disease by altering immune system development and potentially inducing long-term epigenetic changes in immune regulators (Tamburini et al., 2016; Alam et al., 2017). Importantly, the establishment of the gut microbiome appears to rely partially on epigenetic mechanisms to establish microbe - T-cell mutualism, suggesting a complex interplay between physiological systems to dynamically regulate interactions between the microbiome and immune system (Obata et al., 2015). Thus, in the context of the present overview, future work to investigate the impact of in utero alcohol exposure on the gut microbiome and the gut-brain-immune axis will complement the growing body of work on immune and epigenetic alterations in preclinical PAE models and clinical studies of individuals with FASD.
Finally, a better understanding of mechanisms underlying the teratogenic effects of PAE will also pave the way for the development of more informed, targeted intervention strategies for individuals with FASD. Unlike other neurodevelopmental disorders where the underlying cause(s) are still under investigation, such as autism spectrum disorder (Won et al., 2013) or schizophrenia (Fatemi and Folsom, 2009), alcohol is a known teratogen and intervention is the key to better long-term outcomes. Due to the pervasiveness of immune disturbances across PAE models (Drew et al., 2015; Topper et al., 2015), and the link between immune function and overall physical and mental health (Raison et al., 2006), the immune system may be an ideal pharmacological target for individuals with FASD. Moreover, immune activation/cytokines play a key role in brain development, and increasing evidence demonstrates that altered immune activation may underlie altered cognition, attention, behavior, self-regulation, and adaptive functioning. Thus research on immune-based interventions will have broad implications for improving overall function of individuals with FASD [reviewed in Drew and Kane (2014)]. As such, future investigations examining the safety and utility of anti-inflammatory agents applied during early postnatal life will be important. This is particularly urgent in that currently, with the exception of ongoing work to evaluate the therapeutic potential of choline2 supplementation (Thomas et al., 2000, 2007; Wozniak et al., 2015, 2020; Nguyen et al., 2016) and evaluation of pioglitazone3 in animal models (Kane et al., 2011; Drew et al., 2015) there are relatively few available drugs specifically shown to significantly improve the outcomes of PAE.
As a whole, the collective findings from animal models and clinical studies of FASD point to a compelling relationship between the immune system and epigenetic pathways, which may have important causal links to the long-term and multisystem effects of PAE. Ultimately, additional research in this area will not only provide deeper insight into the molecular mechanisms that influence mental health processes, but also help identify novel interventions and therapeutic strategies that may alleviate the adverse health consequences arising from alcohol exposure.
Author’s Note
Data for Table 2 were obtained from https://www.immport.org/shared/genelists.
Author Contributions
AL and TB conceptualized and wrote the manuscript. All authors contributed to manuscript revision and read and approved the submitted version.
Funding
This research was supported by grants from the Collaborative Initiative on Fetal Alcohol Spectrum Disorders (CIFASD) (NIH/NIAAA U01 AA026101), NIH/NIAAA R37 AA007789, and a Kids Brain Health Network grant to JW, as well as NIH/NIAAA R01 AA022460 to JW and TB. AL was supported by a Developmental Neurosciences Research Training Award from Brain Canada and NeuroDevNet.
Conflict of Interest
The authors declare that the research was conducted in the absence of any commercial or financial relationships that could be construed as a potential conflict of interest.
Publisher’s Note
All claims expressed in this article are solely those of the authors and do not necessarily represent those of their affiliated organizations, or those of the publisher, the editors and the reviewers. Any product that may be evaluated in this article, or claim that may be made by its manufacturer, is not guaranteed or endorsed by the publisher.
- ^ Neurodevelopment was assessed using the Bayley Scales of Infant Development, Second Edition (BSID-II) administered at 6 and 12 months. Children were considered to have neurodevelopmental delay if they received a score of <85 on either the Mental Development Index (MDI) or the Psychomotor Development Index (PDI) at 6 and/or 12 months.
- ^ Choline is an essential nutrient that, in pre-clinical models, has been shown to decrease the learning and memory deficits associated with PAE. In clinical studies, choline supplementation has been shown to result in higher non-verbal intelligence, visual-spatial skills, and working and verbal memory, as well as fewer attention deficit hyperactivity disorder symptoms.
- ^ Pioglitazone is a PPAR-γ agonist and PPAR-γ agonists have been shown to prevent ethanol-induced neuronal and microglial loss, as well as dampen microglial activation.
References
Ahluwalia, B., Wesley, B., Adeyiga, O., Smith, D. M., Da-Silva, A., and Rajguru, S. (2000). Alcohol modulates cytokine secretion and synthesis in human fetus: an in vivo and in vitro study. Alcohol 21, 207–213. doi: 10.1016/s0741-8329(00)00076-8
Akimova, T., Beier, U. H., Liu, Y., Wang, L., and Hancock, W. W. (2012). Histone/protein deacetylases and T-cell immune responses. Blood 119, 2443–2451. doi: 10.1182/blood-2011-10-292003
Alam, R., Abdolmaleky, H. M., and Zhou, J. R. (2017). Microbiome, inflammation, epigenetic alterations, and mental diseases. Am. J. Med. Genet. B Neuropsychiatr. Genet. 174, 651–660. doi: 10.1002/ajmg.b.32567
Alenghat, T., Osborne, L. C., Saenz, S. A., Kobuley, D., Ziegler, C. G., Mullican, S. E., et al. (2013). Histone deacetylase 3 coordinates commensal-bacteria-dependent intestinal homeostasis. Nature 504, 153–157. doi: 10.1038/nature12687
Allis, C. D., and Jenuwein, T. (2016). The molecular hallmarks of epigenetic control. Nat. Rev. Genet. 17, 487–500. doi: 10.1038/nrg.2016.59
Aloia, L., Di Stefano, B., and Di Croce, L. (2013). Polycomb complexes in stem cells and embryonic development. Development 140, 2525–2534. doi: 10.1242/dev.091553
Aly, H., Alhabashi, G., Hammad, T. A., Owusu-Ansah, S., Bathgate, S., and Mohamed, M. (2008). ABO phenotype and other risk factors associated with chorioamnionitis. J Pediatr. 153, 16–18. doi: 10.1016/j.jpeds.2008.02.008
Ammann, A. J., Wara, D. W., Cowan, M. J., Barrett, D. J., and Stiehm, E. R. (1982). The DiGeorge syndrome and the fetal alcohol syndrome. Am. J. Dis. Child. 136, 906–908. doi: 10.1001/archpedi.1982.03970460036008
Apfelbacher, C. J., Diepgen, T. L., and Schmitt, J. (2011). Determinants of eczema: population-based cross-sectional study in Germany. Allergy 66, 206–213.
Aristizabal, M. J., Anreiter, I., Halldorsdottir, T., Odgers, C. L., McDade, T. W., Goldenberg, A., et al. (2020). Biological embedding of experience: a primer on epigenetics. Proc. Natl. Acad. Sci. U.S.A. 117, 23261–23269. doi: 10.1073/pnas.1820838116
Astley, S. J., and Clarren, S. K. (2000). Diagnosing the full spectrum of fetal alcohol-exposed individuals: introducing the 4-digit diagnostic code. Alcohol Alcohol. 35, 400–410. doi: 10.1093/alcalc/35.4.400
Astley, S. J., Olson, H. C., Kerns, K., Brooks, A., Aylward, E. H., Coggins, T. E., et al. (2009). Neuropyschological and behavioral outcomes from a comprehensive magnetic resonance study of children with fetal alcohol spectrum disorders. Can. J. Clin. Pharmacol. 16, e178–201.
Aung, H. T., Schroder, K., Himes, S. R., Brion, K., van Zuylen, W., Trieu, A., et al. (2006). LPS regulates proinflammatory gene expression in macrophages by altering histone deacetylase expression. FASEB J. 20, 1315–1327. doi: 10.1096/fj.05-5360com
Balaraman, S., Winzer-Serhan, U. H., and Miranda, R. C. (2012). Opposing actions of ethanol and nicotine on microRNAs are mediated by nicotinic acetylcholine receptors in fetal cerebral cortical-derived neural progenitor cells. Alcohol Clin. Exp. Res. 36, 1669–1677. doi: 10.1111/j.1530-0277.2012.01793.x
Barker, D. J., Gluckman, P. D., Godfrey, K. M., Harding, J. E., Owens, J. A., and Robinson, J. S. (1993). Fetal nutrition and cardiovascular disease in adult life. Lancet 341, 938–941. doi: 10.1016/0140-6736(93)91224-a
Barker, D. J., and Osmond, C. (1986). Infant mortality, childhood nutrition, and ischaemic heart disease in England and Wales. Lancet 1, 1077–1081.
Barker, D. J., Winter, P. D., Osmond, C., Margetts, B., and Simmonds, S. J. (1989). Weight in infancy and death from ischaemic heart disease. Lancet 2, 577–580.
Barker, E. D., Cecil, C. A. M., Walton, E., Houtepen, L. C., O’Connor, T. G., Danese, A., et al. (2018). Inflammation-related epigenetic risk and child and adolescent mental health: a prospective study from pregnancy to middle adolescence. Dev. Psychopathol. 30, 1145–1156. doi: 10.1017/S0954579418000330
Bhattacharya, S., Dunn, P., Thomas, C. G., Smith, B., Schaefer, H., Chen, J., et al. (2018). ImmPort, toward repurposing of open access immunological assay data for translational and clinical research. Sci. Data 5:180015. doi: 10.1038/sdata.2018.15
Bilbo, S. D., and Schwarz, J. M. (2012). The immune system and developmental programming of brain and behavior. Front. Neuroendocrinol. 33, 267–286. doi: 10.1016/j.yfrne.2012.08.006
Bodnar, T., and Weinberg, J. (2013). “Prenatal alcohol exposure: impact on neuroendocrine-neuroimmune networks,” in Neural-Immune Interactions in Brain Function and Alcohol Related Disorders, eds C. Cui, L. Grandison, and A. Noronha (New York, NY: Springer), 312–362.
Bodnar, T. S., Hill, L. A., and Weinberg, J. (2016). Evidence for an immune signature of prenatal alcohol exposure in female rats. Brain Behav. Immun. 58, 130–141.
Bodnar, T. S., Raineki, C., Wertelecki, W., Yevtushok, L., Plotka, L., Granovska, I., et al. (2020). Immune network dysregulation associated with child neurodevelopmental delay: modulatory role of prenatal alcohol exposure. J. Neuroinflamm. 17:39. doi: 10.1186/s12974-020-1717-8
Bodnar, T. S., Raineki, C., Wertelecki, W., Yevtushok, L., Plotka, L., Zymak-Zakutnya, N., et al. (2018). Altered maternal immune networks are associated with adverse child neurodevelopment: impact of alcohol consumption during pregnancy. Brain Behav. Immun. 73, 205–215. doi: 10.1016/j.bbi.2018.05.004
Boschen, K. E., Ruggiero, M. J., and Klintsova, A. Y. (2016). Neonatal binge alcohol exposure increases microglial activation in the developing rat hippocampus. Neuroscience 324, 355–366. doi: 10.1016/j.neuroscience.2016.03.033
Boyce, W. T., and Kobor, M. S. (2015). Development and the epigenome: the ‘synapse’ of gene-environment interplay. Dev. Sci. 18, 1–23. doi: 10.1111/desc.12282
Bull-Otterson, L., Feng, W., Kirpich, I., Wang, Y., Qin, X., Liu, Y., et al. (2013). Metagenomic analyses of alcohol induced pathogenic alterations in the intestinal microbiome and the effect of Lactobacillus rhamnosus GG treatment. PLoS One 8:e53028. doi: 10.1371/journal.pone.0053028
Cantacorps, L., Alfonso-Loeches, S., Moscoso-Castro, M., Cuitavi, J., Gracia-Rubio, I., Lopez-Arnau, R., et al. (2017). Maternal alcohol binge drinking induces persistent neuroinflammation associated with myelin damage and behavioural dysfunctions in offspring mice. Neuropharmacology 123, 368–384. doi: 10.1016/j.neuropharm.2017.05.034
Carabotti, M., Scirocco, A., Maselli, M. A., and Severi, C. (2015). The gut-brain axis: interactions between enteric microbiota, central and enteric nervous systems. Ann. Gastroenterol. 28, 203–209.
Cardoso, A. L., Guedes, J. R., Pereira de Almeida, L., and Pedroso de Lima, M. C. (2012). miR-155 modulates microglia-mediated immune response by down-regulating SOCS-1 and promoting cytokine and nitric oxide production. Immunology 135, 73–88. doi: 10.1111/j.1365-2567.2011.03514.x
Carson, C. G., Halkjaer, L. B., Jensen, S. M., and Bisgaard, H. (2012). Alcohol intake in pregnancy increases the child’s risk of atopic dermatitis. the COPSAC prospective birth cohort study of a high risk population. PLoS One 7:e42710. doi: 10.1371/journal.pone.0042710
Carter, R. C., Jacobson, J. L., Molteno, C. D., Dodge, N. C., Meintjes, E. M., and Jacobson, S. W. (2016). Fetal alcohol growth restriction and cognitive impairment. Pediatrics 138:e20160775.
Chastain, L. G., Franklin, T., Gangisetty, O., Cabrera, M. A., Mukherjee, S., Shrivastava, P., et al. (2019). Early life alcohol exposure primes hypothalamic microglia to later-life hypersensitivity to immune stress: possible epigenetic mechanism. Neuropsychopharmacology 44, 1579–1588. doi: 10.1038/s41386-019-0326-7
Chater-Diehl, E. J., Laufer, B. I., Castellani, C. A., Alberry, B. L., and Singh, S. M. (2016). Alteration of Gene Expression, DNA Methylation, and Histone Methylation in Free Radical Scavenging Networks in Adult Mouse Hippocampus following Fetal Alcohol Exposure. PLoS One 11:e0154836. doi: 10.1371/journal.pone.0154836
Chauhan, A., Quenum, F. Z., Abbas, A., Bradley, D. S., Nechaev, S., Singh, B. B., et al. (2015). Epigenetic modulation of microglial inflammatory gene loci in helminth-induced immune suppression: implications for immune regulation in neurocysticercosis. ASN Neuro. 7:1759091415592126. doi: 10.1177/1759091415592126
Cheray, M., and Joseph, B. (2018). Epigenetics control microglia plasticity. Front. Cell Neurosci. 12:243. doi: 10.3389/fncel.2018.00243
Church, M. W., and Gerkin, K. P. (1988). Hearing disorders in children with fetal alcohol syndrome: findings from case reports. Pediatrics 82, 147–154.
Cobben, J. M., Krzyzewska, I. M., Venema, A., Mul, A. N., Polstra, A., Postma, A. V., et al. (2019). DNA methylation abundantly associates with fetal alcohol spectrum disorder and its subphenotypes. Epigenomics 11, 767–785. doi: 10.2217/epi-2018-0221
Crews, F. T., Bechara, R., Brown, L. A., Guidot, D. M., Mandrekar, P., Oak, S., et al. (2006). Cytokines and alcohol. Alcohol Clin. Exp. Res. 30, 720–730.
Dovey, O. M., Foster, C. T., Conte, N., Edwards, S. A., Edwards, J. M., Singh, R., et al. (2013). Histone deacetylase 1 and 2 are essential for normal T-cell development and genomic stability in mice. Blood 121, 1335–1344. doi: 10.1182/blood-2012-07-441949
Doyle, L. R., and Mattson, S. N. (2015). Neurobehavioral Disorder Associated with Prenatal Alcohol Exposure (ND-PAE): review of evidence and guidelines for assessment. Curr. Dev. Disord. Rep. 2, 175–186. doi: 10.1007/s40474-015-0054-6
Drew, P. D., Johnson, J. W., Douglas, J. C., Phelan, K. D., and Kane, C. J. (2015). Pioglitazone blocks ethanol induction of microglial activation and immune responses in the hippocampus, cerebellum, and cerebral cortex in a mouse model of fetal alcohol spectrum disorders. Alcohol Clin. Exp. Res. 39, 445–454. doi: 10.1111/acer.12639
Drew, P. D., and Kane, C. J. (2014). Fetal alcohol spectrum disorders and neuroimmune changes. Int. Rev. Neurobiol. 118, 41–80. doi: 10.1016/b978-0-12-801284-0.00003-8
Famy, C., Streissguth, A. P., and Unis, A. S. (1998). Mental illness in adults with fetal alcohol syndrome or fetal alcohol effects. Am. J. Psychiatry 155, 552–554. doi: 10.1176/ajp.155.4.552
Fatemi, S. H., and Folsom, T. D. (2009). The neurodevelopmental hypothesis of schizophrenia, revisited. Schizophr. Bull. 35, 528–548. doi: 10.1093/schbul/sbn187
Fernandez-Lizarbe, S., Pascual, M., and Guerri, C. (2009). Critical role of TLR4 response in the activation of microglia induced by ethanol. J. Immunol. 183, 4733–4744. doi: 10.4049/jimmunol.0803590
Frey, S., Eichler, A., Stonawski, V., Kriebel, J., Wahl, S., Gallati, S., et al. (2018). Prenatal alcohol exposure is associated with adverse cognitive effects and distinct whole-genome DNA methylation patterns in primary school children. Front. Behav. Neurosci. 12:125. doi: 10.3389/fnbeh.2018.00125
Fujii, R., Sato, S., Tsuboi, Y., Cardenas, A., and Suzuki, K. (2021). DNA methylation as a mediator of associations between the environment and chronic diseases: a scoping review on application of mediation analysis. Epigenetics doi: 10.1080/15592294.2021.1959736 [Epub ahead of print].
Fujita, S., Tsuchihashi, Y., and Kitamura, T. (1981). Origin, morphology and function of the microglia. Prog Clin Biol Res. 59A, 141–169.
Galinsky, R., Polglase, G. R., Hooper, S. B., Black, M. J., and Moss, T. J. (2013). The consequences of chorioamnionitis: preterm birth and effects on development. J. Pregnancy 2013:412831.
Gano, A., Prestia, L., Middleton, F. A., Youngentob, S. L., Ignacio, C., and Deak, T. (2020). Gene expression profiling reveals a lingering effect of prenatal alcohol exposure on inflammatory-related genes during adolescence and adulthood. Cytokine 133:155126. doi: 10.1016/j.cyto.2020.155126
Garden, G. A. (2013). Epigenetics and the modulation of neuroinflammation. Neurotherapeutics 10, 782–788. doi: 10.1007/s13311-013-0207-4
Gauthier, T. W., Drews-Botsch, C., Falek, A., Coles, C., and Brown, L. A. (2005). Maternal alcohol abuse and neonatal infection. Alcohol Clin. Exp. Res. 29, 1035–1043.
Gauthier, T. W., Manar, M. H., and Brown, L. A. (2004). Is maternal alcohol use a risk factor for early-onset sepsis in premature newborns? Alcohol 33, 139–145. doi: 10.1016/j.alcohol.2004.06.003
Gottesfeld, Z., and Abel, E. L. (1991). Maternal and paternal alcohol use: effects on the immune system of the offspring. Life Sci. 48, 1–8. doi: 10.1016/0024-3205(91)90419-c
Green, M. L., Singh, A. V., Zhang, Y., Nemeth, K. A., Sulik, K. K., and Knudsen, T. B. (2007). Reprogramming of genetic networks during initiation of the Fetal Alcohol Syndrome. Dev. Dyn. 236, 613–631. doi: 10.1002/dvdy.21048
Gursky, Z. H., Johansson, J. R., and Klintsova, A. Y. (2020). Postnatal alcohol exposure and adolescent exercise have opposite effects on cerebellar microglia in rat. Int. J. Dev. Neurosci. 80, 558–571. doi: 10.1002/jdn.10051
Hard, M. L., Abdolell, M., Robinson, B. H., and Koren, G. (2005). Gene-expression analysis after alcohol exposure in the developing mouse. J. Lab. Clin. Med. 145, 47–54. doi: 10.1016/j.lab.2004.11.011
Hashimi, S. T., Fulcher, J. A., Chang, M. H., Gov, L., Wang, S., and Lee, B. (2009). MicroRNA profiling identifies miR-34a and miR-21 and their target genes JAG1 and WNT1 in the coordinate regulation of dendritic cell differentiation. Blood 114, 404–414. doi: 10.1182/blood-2008-09-179150
He, J., and Crews, F. T. (2008). Increased MCP-1 and microglia in various regions of the human alcoholic brain. Exp. Neurol. 210, 349–358. doi: 10.1016/j.expneurol.2007.11.017
Hellemans, K. G., Sliwowska, J. H., Verma, P., and Weinberg, J. (2010). Prenatal alcohol exposure: fetal programming and later life vulnerability to stress, depression and anxiety disorders. Neurosci. Biobehav. Rev. 34, 791–807. doi: 10.1016/j.neubiorev.2009.06.004
Hicks, S. D., Middleton, F. A., and Miller, M. W. (2010). Ethanol-induced methylation of cell cycle genes in neural stem cells. J. Neurochem. 114, 1767–1780. doi: 10.1111/j.1471-4159.2010.06886.x
Himmelreich, M., Lutke, C., and Travis, E. (2020). “The lay of the land: Fetal Alcohol Spectrum Disorder (FASD) as a whole-body diagnosis,” in The Routledge Handbook of Social Work and Addictive Behaviors, eds A. Begun and M. M. Murray (Abingdon: Routledge), 191–215.
Hinz, D., Bauer, M., Roder, S., Olek, S., Huehn, J., Sack, U., et al. (2012). Cord blood Tregs with stable FOXP3 expression are influenced by prenatal environment and associated with atopic dermatitis at the age of one year. Allergy 67, 380–389. doi: 10.1111/j.1398-9995.2011.02767.x
Hodes, G. E., Kana, V., Menard, C., Merad, M., and Russo, S. J. (2015). Neuroimmune mechanisms of depression. Nat. Neurosci. 18, 1386–1393. doi: 10.1038/nn.4113
Hooper, L. V., Littman, D. R., and Macpherson, A. J. (2012). Interactions between the microbiota and the immune system. Science 336, 1268–1273.
Ignacio, C., Mooney, S. M., and Middleton, F. A. (2014). Effects of acute prenatal exposure to ethanol on microRNA expression are ameliorated by social enrichment. Front. Pediatr. 2:103. doi: 10.3389/fped.2014.00103
Johnnidis, J. B., Harris, M. H., Wheeler, R. T., Stehling-Sun, S., Lam, M. H., Kirak, O., et al. (2008). Regulation of progenitor cell proliferation and granulocyte function by microRNA-223. Nature 451, 1125–1129. doi: 10.1038/nature06607
Johnson, S., Knight, R., Marmer, D. J., and Steele, R. W. (1981). Immune deficiency in fetal alcohol syndrome. Pediatr. Res. 15, 908–911.
Jovicic, A., Roshan, R., Moisoi, N., Pradervand, S., Moser, R., Pillai, B., et al. (2013). Comprehensive expression analyses of neural cell-type-specific miRNAs identify new determinants of the specification and maintenance of neuronal phenotypes. J. Neurosci. 33, 5127–5137. doi: 10.1523/JNEUROSCI.0600-12.2013
Kaminska, B., Mota, M., and Pizzi, M. (2016). Signal transduction and epigenetic mechanisms in the control of microglia activation during neuroinflammation. Biochim. Biophys. Acta. 1862, 339–351. doi: 10.1016/j.bbadis.2015.10.026
Kane, C. J., Phelan, K. D., Han, L., Smith, R. R., Xie, J., Douglas, J. C., et al. (2011). Protection of neurons and microglia against ethanol in a mouse model of fetal alcohol spectrum disorders by peroxisome proliferator-activated receptor-gamma agonists. Brain Behav. Immun. 25, S137–S145. doi: 10.1016/j.bbi.2011.02.016
Kane, C. J. M., and Drew, P. D. (2021). Neuroinflammatory contribution of microglia and astrocytes in fetal alcohol spectrum disorders. J. Neurosci. Res. 99, 1973–1985. doi: 10.1002/jnr.24735
Keshavarzian, A., Choudhary, S., Holmes, E. W., Yong, S., Banan, A., Jakate, S., et al. (2001). Preventing gut leakiness by oats supplementation ameliorates alcohol-induced liver damage in rats. J. Pharmacol. Exp. Ther. 299, 442–448.
Khalid, O., Kim, J. J., Kim, H. S., Hoang, M., Tu, T. G., Elie, O., et al. (2014). Gene expression signatures affected by alcohol-induced DNA methylomic deregulation in human embryonic stem cells. Stem Cell Res. 12, 791–806. doi: 10.1016/j.scr.2014.03.009
Khandaker, G. M., Pearson, R. M., Zammit, S., Lewis, G., and Jones, P. B. (2014). Association of serum interleukin 6 and C-reactive protein in childhood with depression and psychosis in young adult life: a population-based longitudinal study. JAMA Psychiatry 71, 1121–1128. doi: 10.1001/jamapsychiatry.2014.1332
Kobor, M. S., and Weinberg, J. (2011). Focus on: epigenetics and fetal alcohol spectrum disorders. Alcohol Res. Health 34, 29–37.
Krishnamoorthy, M., Gerwe, B. A., Scharer, C. D., Sahasranaman, V., Eilertson, C. D., Nash, R. J., et al. (2013). Ethanol alters proliferation and differentiation of normal and chromosomally abnormal human embryonic stem cell-derived neurospheres. Birth Defects Res. B Dev. Reprod. Toxicol. 98, 283–295. doi: 10.1002/bdrb.21063
Laufer, B. I., Kapalanga, J., Castellani, C. A., Diehl, E. J., Yan, L., and Singh, S. M. (2015). Associative DNA methylation changes in children with prenatal alcohol exposure. Epigenomics 7, 1259–1274. doi: 10.2217/epi.15.60
Laufer, B. I., Mantha, K., Kleiber, M. L., Diehl, E. J., Addison, S. M. F., and Singh, S. M. (2013). Long-lasting alterations to DNA methylation and ncRNAs could underlie the effects of fetal alcohol exposure in mice. Dis. Model Mech. 6, 977–992.
Le Menn, G., and Neels, J. G. (2018). Regulation of immune cell function by PPARs and the connection with metabolic and neurodegenerative diseases. Int. J. Mol. Sci. 19:1575. doi: 10.3390/ijms19061575
Lee, P. P., Fitzpatrick, D. R., Beard, C., Jessup, H. K., Lehar, S., Makar, K. W., et al. (2001). A critical role for Dnmt1 and DNA methylation in T cell development, function, and survival. Immunity 15, 763–774. doi: 10.1016/s1074-7613(01)00227-8
Libster, R., Ferolla, F. M., Hijano, D. R., Acosta, P. L., Erviti, A., Polack, F. P., et al. (2015). Alcohol during pregnancy worsens acute respiratory infections in children. Acta Paediatr. 104, e494–e499. doi: 10.1111/apa.13148
Ling, E. A., and Wong, W. C. (1993). The origin and nature of ramified and amoeboid microglia: a historical review and current concepts. Glia 7, 9–18. doi: 10.1002/glia.440070105
Linneberg, A., Petersen, J., Gronbaek, M., and Benn, C. S. (2004). Alcohol during pregnancy and atopic dermatitis in the offspring. Clin. Exp. Allergy 34, 1678–1683. doi: 10.1111/j.1365-2222.2004.02101.x
Liu, Y., Aryee, M. J., Padyukov, L., Fallin, M. D., Hesselberg, E., Runarsson, A., et al. (2013). Epigenome-wide association data implicate DNA methylation as an intermediary of genetic risk in rheumatoid arthritis. Nat. Biotechnol. 31, 142–147. doi: 10.1038/nbt.2487
Liu, Y., Balaraman, Y., Wang, G., Nephew, K. P., and Zhou, F. C. (2009). Alcohol exposure alters DNA methylation profiles in mouse embryos at early neurulation. Epigenetics 4, 500–511.
Louwies, T., Johnson, A. C., Orock, A., Yuan, T., and Greenwood-Van Meerveld, B. (2019). The microbiota-gut-brain axis: an emerging role for the epigenome. Exp. Biol. Med. 245, 138–145. doi: 10.1177/1535370219891690
Lussier, A. A., Bodnar, T. S., Mingay, M., Morin, A. M., Hirst, M., Kobor, M. S., et al. (2018a). Prenatal alcohol exposure: profiling developmental DNA methylation patterns in central and peripheral tissues. Front. Genet. 9:610. doi: 10.3389/fgene.2018.00610
Lussier, A. A., Morin, A. M., MacIsaac, J. L., Salmon, J., Weinberg, J., Reynolds, J. N., et al. (2018b). DNA methylation as a predictor of fetal alcohol spectrum disorder. Clin. Epigenetics 10:5. doi: 10.1186/s13148-018-0439-6
Lussier, A. A., Stepien, K. A., Neumann, S. M., Pavlidis, P., Kobor, M. S., and Weinberg, J. (2015). Prenatal alcohol exposure alters steady-state and activated gene expression in the adult rat brain. Alcohol Clin. Exp. Res. 39, 251–261. doi: 10.1111/acer.12622
Lussier, A. A., Weinberg, J., and Kobor, M. S. (2017). Epigenetics studies of fetal alcohol spectrum disorder: where are we now? Epigenomics 9, 291–311. doi: 10.2217/epi-2016-0163
Lynch, M. E., Kable, J. A., and Coles, C. D. (2015). Prenatal alcohol exposure, adaptive function, and entry into adult roles in a prospective study of young adults. Neurotoxicol. Teratol. 51, 52–60. doi: 10.1016/j.ntt.2015.07.008
Magnus, M. C., DeRoo, L. A., Haberg, S. E., Magnus, P., Nafstad, P., Nystad, W., et al. (2014). Prospective study of maternal alcohol intake during pregnancy or lactation and risk of childhood asthma: the Norwegian mother and child cohort study. Alcohol Clin. Exp. Res. 38, 1002–1011. doi: 10.1111/acer.12348
Mahnke, A. H., Adams, A. M., Wang, A. Z., and Miranda, R. C. (2019). Toxicant and teratogenic effects of prenatal alcohol. Curr. Opin. Toxicol. 14, 29–34. doi: 10.1016/j.cotox.2019.08.002
Marin-Teva, J. L., Dusart, I., Colin, C., Gervais, A., van Rooijen, N., and Mallat, M. (2004). Microglia promote the death of developing Purkinje cells. Neuron 41, 535–547. doi: 10.1016/s0896-6273(04)00069-8
Marjonen, H., Sierra, A., Nyman, A., Rogojin, V., Grohn, O., Linden, A. M., et al. (2015). Early maternal alcohol consumption alters hippocampal DNA methylation, gene expression and volume in a mouse model. PLoS One 10:e0124931. doi: 10.1371/journal.pone.0124931
Mohajeri, M. H., La Fata, G., Steinert, R. E., and Weber, P. (2018). Relationship between the gut microbiome and brain function. Nutr. Rev. 76, 481–496. doi: 10.1093/nutrit/nuy009
Morais, L. H., Schreiber, H. L., and Mazmanian, S. K. (2021). The gut microbiota–brain axis in behaviour and brain disorders. Nat. Rev. Microbiol. 19, 241–255.
Nestler, E. J., Barrot, M., DiLeone, R. J., Eisch, A. J., Gold, S. J., and Monteggia, L. M. (2002). Neurobiology of depression. Neuron 34, 13–25.
Nguyen, T. T., Risbud, R. D., Mattson, S. N., Chambers, C. D., and Thomas, J. D. (2016). Randomized, double-blind, placebo-controlled clinical trial of choline supplementation in school-aged children with fetal alcohol spectrum disorders. Am. J. Clin. Nutr. 104, 1683–1692. doi: 10.3945/ajcn.116.142075
Nicodeme, E., Jeffrey, K. L., Schaefer, U., Beinke, S., Dewell, S., Chung, C. W., et al. (2010). Suppression of inflammation by a synthetic histone mimic. Nature 468, 1119–1123. doi: 10.1038/nature09589
Obata, Y., Furusawa, Y., and Hase, K. (2015). Epigenetic modifications of the immune system in health and disease. Immunol. Cell Biol. 93, 226–232.
O’Connor, M. J., Shah, B., Whaley, S., Cronin, P., Gunderson, B., and Graham, J. (2002). Psychiatric illness in a clinical sample of children with prenatal alcohol exposure. Am. J. Drug Alcohol. Abuse 28, 743–754.
Oleson, D. R., Magee, R. M., Donahoe, R. M., Falek, A., and Coles, C. D. (1998). Immunity and prenatal alcohol exposure. A pilot study in human adolescents. Adv. Exp. Med. Biol. 437, 255–264.
Panczakiewicz, A. L., Glass, L., Coles, C. D., Kable, J. A., Sowell, E. R., Wozniak, J. R., et al. (2016). Neurobehavioral Deficits Consistent Across Age and Sex in Youth with Prenatal Alcohol Exposure. Alcohol Clin. Exp. Res. 40, 1971–1981. doi: 10.1111/acer.13153
Paolicelli, R. C., Bolasco, G., Pagani, F., Maggi, L., Scianni, M., Panzanelli, P., et al. (2011). Synaptic pruning by microglia is necessary for normal brain development. Science 333, 1456–1458. doi: 10.1126/science.1202529
Pappas, A., Kendrick, D. E., Shankaran, S., Stoll, B. J., Bell, E. F., Laptook, A. R., et al. (2014). Chorioamnionitis and early childhood outcomes among extremely low-gestational-age neonates. JAMA Pediatr. 168, 137–147. doi: 10.1001/jamapediatrics.2013.4248
Pei, J., Denys, K., Hughes, J., and Rasmussen, C. (2011). Mental health issues in fetal alcohol spectrum disorder. J. Ment. Health 20, 438–448.
Petra, A. I., Panagiotidou, S., Hatziagelaki, E., Stewart, J. M., Conti, P., and Theoharides, T. C. (2015). Gut-microbiota-brain axis and its effect on neuropsychiatric disorders with suspected immune dysregulation. Clin. Ther. 37, 984–995. doi: 10.1016/j.clinthera.2015.04.002
Pollard, I. (2007). Neuropharmacology of drugs and alcohol in mother and fetus. Semin Fetal. Neonatal Med. 12, 106–113. doi: 10.1016/j.siny.2006.12.001
Portales-Casamar, E., Lussier, A. A., Jones, M. J., MacIsaac, J. L., Edgar, R. D., Mah, S. M., et al. (2016). DNA methylation signature of human fetal alcohol spectrum disorder. Epigenet, Chromat. 9:25.
Qi, Y., Zhang, M., Li, H., Frank, J. A., Dai, L., Liu, H., et al. (2014). MicroRNA-29b regulates ethanol-induced neuronal apoptosis in the developing cerebellum through SP1/RAX/PKR cascade. J. Biol. Chem. 289, 10201–10210. doi: 10.1074/jbc.M113.535195
Raison, C. L., Capuron, L., and Miller, A. H. (2006). Cytokines sing the blues: inflammation and the pathogenesis of depression. Trends Immunol. 27, 24–31. doi: 10.1016/j.it.2005.11.006
Raychaudhuri, S., Sandor, C., Stahl, E. A., Freudenberg, J., Lee, H. S., Jia, X., et al. (2012). Five amino acids in three HLA proteins explain most of the association between MHC and seropositive rheumatoid arthritis. Nat. Genet. 44, 291–296. doi: 10.1038/ng.1076
Reid, N., Moritz, K. M., and Akison, L. K. (2019). Adverse health outcomes associated with fetal alcohol exposure: a systematic review focused on immune-related outcomes. Pediatr. Allergy Immunol. 30, 698–707. doi: 10.1111/pai.13099
Rickert, V. I., Wiemann, C. M., Hankins, G. D., McKee, J. M., and Berenson, A. B. (1998). Prevalence and risk factors of chorioamnionitis among adolescents. Obstet. Gynecol. 92, 254–257. doi: 10.1016/s0029-7844(98)00135-5
Roberson, R., Kuddo, T., Benassou, I., Abebe, D., and Spong, C. Y. (2012). Neuroprotective peptides influence cytokine and chemokine alterations in a model of fetal alcohol syndrome. Am. J. Obstet. Gynecol. 207, 499.e1–499.e5. doi: 10.1016/j.ajog.2012.10.005.
Ruggiero, M. J., Boschen, K. E., Roth, T. L., and Klintsova, A. Y. (2018). Sex differences in early postnatal microglial colonization of the developing rat hippocampus following a single-day alcohol exposure. J. Neuroimmune Pharmacol. 13, 189–203. doi: 10.1007/s11481-017-9774-1
Salem, N. A., Mahnke, A. H., Konganti, K., Hillhouse, A. E., and Miranda, R. C. (2021). Cell-type and fetal-sex-specific targets of prenatal alcohol exposure in developing mouse cerebral cortex. iScience 24:102439. doi: 10.1016/j.isci.2021.102439
Sathyan, P., Golden, H. B., and Miranda, R. C. (2007). Competing interactions between micro-RNAs determine neural progenitor survival and proliferation after ethanol exposure: evidence from an ex vivo model of the fetal cerebral cortical neuroepithelium. J. Neurosci. 27, 8546–8557. doi: 10.1523/JNEUROSCI.1269-07.2007
Satoh, T., Takeuchi, O., Vandenbon, A., Yasuda, K., Tanaka, Y., Kumagai, Y., et al. (2010). The Jmjd3-Irf4 axis regulates M2 macrophage polarization and host responses against helminth infection. Nat. Immunol. 11, 936–944. doi: 10.1038/ni.1920
Schmidl, C., Delacher, M., Huehn, J., and Feuerer, M. (2018). Epigenetic mechanisms regulating T-cell responses. J. Allergy Clin. Immunol. 142, 728–743.
Schwarz, J. M., Hutchinson, M. R., and Bilbo, S. D. (2011). Early-life experience decreases drug-induced reinstatement of morphine CPP in adulthood via microglial-specific epigenetic programming of anti-inflammatory IL-10 expression. J. Neurosci. 31, 17835–17847. doi: 10.1523/JNEUROSCI.3297-11.2011
Shaheen, S. O., Rutterford, C., Zuccolo, L., Ring, S. M., Davey Smith, G., Holloway, J. W., et al. (2014). Prenatal alcohol exposure and childhood atopic disease: a Mendelian randomization approach. J. Allergy Clin. Immunol. 133, 225–32.e1-5. doi: 10.1016/j.jaci.2013.04.051
Sharp, G. C., Arathimos, R., Reese, S. E., Page, C. M., Felix, J., Küpers, L. K., et al. (2018). Maternal alcohol consumption and offspring DNA methylation: findings from six general population-based birth cohorts. Epigenomics 10, 27–42. doi: 10.2217/epi-2017-0095
Shulha, H. P., Cheung, I., Guo, Y., Akbarian, S., and Weng, Z. (2013). Coordinated cell type-specific epigenetic remodeling in prefrontal cortex begins before birth and continues into early adulthood. PLoS Genet. 9:e1003433. doi: 10.1371/journal.pgen.1003433
Stilling, R. M., Dinan, T. G., and Cryan, J. F. (2014). Microbial genes, brain & behaviour - epigenetic regulation of the gut-brain axis. Genes Brain Behav. 13, 69–86.
Streissguth, A. P., and O’Malley, K. (2000). Neuropsychiatric implications and long-term consequences of fetal alcohol spectrum disorders. Semin. Clin. Neuropsychiatry 5, 177–190. doi: 10.1053/scnp.2000.6729
Taganov, K. D., Boldin, M. P., Chang, K. J., and Baltimore, D. (2006). NF-kappaB-dependent induction of microRNA miR-146, an inhibitor targeted to signaling proteins of innate immune responses. Proc. Natl. Acad. Sci. U. S. A. 103, 12481–12486. doi: 10.1073/pnas.0605298103
Tamburini, S., Shen, N., Wu, H. C., and Clemente, J. C. (2016). The microbiome in early life: implications for health outcomes. Nat. Med. 22, 713–722.
Terasaki, L. S., and Schwarz, J. M. (2016). Effects of moderate prenatal alcohol exposure during early gestation in rats on inflammation across the maternal-fetal-immune interface and later-life immune function in the offspring. J. Neuroimmune Pharmacol. 11, 680–692. doi: 10.1007/s11481-016-9691-8
Thomas, J. D., Biane, J. S., O’Bryan, K. A., O’Neill, T. M., and Dominguez, H. D. (2007). Choline supplementation following third-trimester-equivalent alcohol exposure attenuates behavioral alterations in rats. Behav. Neurosci. 121, 120–130. doi: 10.1037/0735-7044.121.1.120
Thomas, J. D., La Fiette, M. H., Quinn, V. R., and Riley, E. P. (2000). Neonatal choline supplementation ameliorates the effects of prenatal alcohol exposure on a discrimination learning task in rats. Neurotoxicol. Teratol. 22, 703–711. doi: 10.1016/s0892-0362(00)00097-0
Tiwari, V., and Chopra, K. (2011). Resveratrol prevents alcohol-induced cognitive deficits and brain damage by blocking inflammatory signaling and cell death cascade in neonatal rat brain. J. Neurochem. 117, 678–690. doi: 10.1111/j.1471-4159.2011.07236.x
Topper, L. A., Baculis, B. C., and Valenzuela, C. F. (2015). Exposure of neonatal rats to alcohol has differential effects on neuroinflammation and neuronal survival in the cerebellum and hippocampus. J. Neuroinflamm. 12:160. doi: 10.1186/s12974-015-0382-9
Tremblay, M. E., Stevens, B., Sierra, A., Wake, H., Bessis, A., and Nimmerjahn, A. (2011). The role of microglia in the healthy brain. J. Neurosci. 31, 16064–16069. doi: 10.1523/jneurosci.4158-11.2011
Uddin, M., Koenen, K. C., Aiello, A. E., Wildman, D. E., de los Santos, R., and Galea, S. (2011). Epigenetic and inflammatory marker profiles associated with depression in a community-based epidemiologic sample. Psychol. Med. 41, 997–1007. doi: 10.1017/S0033291710001674
Veazey, K. J., Muller, D., and Golding, M. C. (2013). Prenatal alcohol exposure and cellular differentiation: a role for Polycomb and Trithorax group proteins in FAS phenotypes? Alcohol Res. 35, 77–85.
Wada, K., Konishi, K., Tamura, T., Shiraki, M., Iwasa, S., and Nagata, C. (2016). Alcohol intake during pregnancy and offspring’s atopic eczema risk. Alcohol Clin. Exp. Res. 40, 1037–1043. doi: 10.1111/acer.13048
Wadhwa, P. D., Buss, C., Entringer, S., and Swanson, J. M. (2009). Developmental origins of health and disease: brief history of the approach and current focus on epigenetic mechanisms. Semin. Reprod. Med. 27, 358–368. doi: 10.1055/s-0029-1237424
Wang, J., Hodes, G. E., Zhang, H., Zhang, S., Zhao, W., Golden, S. A., et al. (2018). Epigenetic modulation of inflammation and synaptic plasticity promotes resilience against stress in mice. Nat. Commun. 9:477. doi: 10.1038/s41467-017-02794-5
Wang, L. L., Zhang, Z., Li, Q., Yang, R., Pei, X., Xu, Y., et al. (2009). Ethanol exposure induces differential microRNA and target gene expression and teratogenic effects which can be suppressed by folic acid supplementation. Hum. Reprod. 24, 562–579. doi: 10.1093/humrep/den439
Wilhelm, C. J., and Guizzetti, M. (2015). Fetal alcohol spectrum disorders: an overview from the Glia perspective. Front. Integr. Neurosci. 9:65. doi: 10.3389/fnint.2015.00065
Won, H., Mah, W., and Kim, E. (2013). Autism spectrum disorder causes, mechanisms, and treatments: focus on neuronal synapses. Front. Mol. Neurosci. 6:19. doi: 10.3389/fnmol.2013.00019
Wozniak, J. R., Fink, B. A., Fuglestad, A. J., Eckerle, J. K., Boys, C. J., Sandness, K. E., et al. (2020). Four-year follow-up of a randomized controlled trial of choline for neurodevelopment in fetal alcohol spectrum disorder. J. Neurodev. Disord. 12:9. doi: 10.1186/s11689-020-09312-7
Wozniak, J. R., Fuglestad, A. J., Eckerle, J. K., Fink, B. A., Hoecker, H. L., Boys, C. J., et al. (2015). Choline supplementation in children with fetal alcohol spectrum disorders: a randomized, double-blind, placebo-controlled trial. Am. J. Clin. Nutr. 102, 1113–1125. doi: 10.3945/ajcn.114.099168
Xavier, A. L., Menezes, J. R., Goldman, S. A., and Nedergaard, M. (2014). Fine-tuning the central nervous system: microglial modelling of cells and synapses. Philos. Trans. R. Soc. Lond. B. Biol. Sci. 369:20130593. doi: 10.1098/rstb.2013.0593
Yan, A. W., Fouts, D. E., Brandl, J., Starkel, P., Torralba, M., Schott, E., et al. (2011). Enteric dysbiosis associated with a mouse model of alcoholic liver disease. Hepatology 53, 96–105. doi: 10.1002/hep.24018
Yuan, W., Sorensen, H. T., Basso, O., and Olsen, J. (2004). Prenatal maternal alcohol consumption and hospitalization with asthma in childhood: a population-based follow-up study. Alcohol Clin. Exp. Res. 28, 765–768. doi: 10.1097/01.alc.0000125348.23133.88
Yuen, R. K., Neumann, S. M., Fok, A. K., Penaherrera, M. S., McFadden, D. E., Robinson, W. P., et al. (2011). Extensive epigenetic reprogramming in human somatic tissues between fetus and adult. Epigenet. Chrom. 4:7. doi: 10.1186/1756-8935-4-7
Zhang, L., Dong, L. Y., Li, Y. J., Hong, Z., and Wei, W. S. (2012). miR-21 represses FasL in microglia and protects against microglia-mediated neuronal cell death following hypoxia/ischemia. Glia 60, 1888–1895. doi: 10.1002/glia.22404
Zhang, Q., and Cao, X. (2019). Epigenetic regulation of the innate immune response to infection. Nat. Rev. Immunol. 19, 417–432. doi: 10.1038/s41577-019-0151-6
Zhang, X., Lan, N., Bach, P., Nordstokke, D., Yu, W., Ellis, L., et al. (2012). Prenatal alcohol exposure alters the course and severity of adjuvant-induced arthritis in female rats. Brain Behav. Immun. 26, 439–450. doi: 10.1016/j.bbi.2011.11.005
Zheng, D., Li, Y., He, L., Tang, Y., Li, X., Shen, Q., et al. (2014). The protective effect of astaxanthin on fetal alcohol spectrum disorder in mice. Neuropharmacology 84, 13–18.
Zhou, F. C., Zhao, Q., Liu, Y., Goodlett, C. R., Liang, T., McClintick, J. N., et al. (2011b). Alteration of gene expression by alcohol exposure at early neurulation. BMC Genomics 12:124. doi: 10.1186/1471-2164-12-124
Zhou, F. C., Balaraman, Y., Teng, M., Liu, Y., Singh, R. P., and Nephew, K. P. (2011a). Alcohol alters DNA methylation patterns and inhibits neural stem cell differentiation. Alcohol Clin. Exp. Res. 35, 735–746. doi: 10.1111/j.1530-0277.2010.01391.x
Keywords: prenatal alcohol exposure (PAE), Fetal Alcohol Spectrum Disorder (FASD), development, immune function, epigenetics
Citation: Lussier AA, Bodnar TS and Weinberg J (2021) Intersection of Epigenetic and Immune Alterations: Implications for Fetal Alcohol Spectrum Disorder and Mental Health. Front. Neurosci. 15:788630. doi: 10.3389/fnins.2021.788630
Received: 03 October 2021; Accepted: 02 November 2021;
Published: 03 December 2021.
Edited by:
Yasir Ahmed Syed, Cardiff University, United KingdomReviewed by:
Lauren Jantzie, Johns Hopkins University, United StatesDanielle Burgess, Torrens University Australia, Australia
Copyright © 2021 Lussier, Bodnar and Weinberg. This is an open-access article distributed under the terms of the Creative Commons Attribution License (CC BY). The use, distribution or reproduction in other forums is permitted, provided the original author(s) and the copyright owner(s) are credited and that the original publication in this journal is cited, in accordance with accepted academic practice. No use, distribution or reproduction is permitted which does not comply with these terms.
*Correspondence: Tamara S. Bodnar, dGFtYXJhLmJvZG5hckB1YmMuY2E=
†These authors have contributed equally to this work