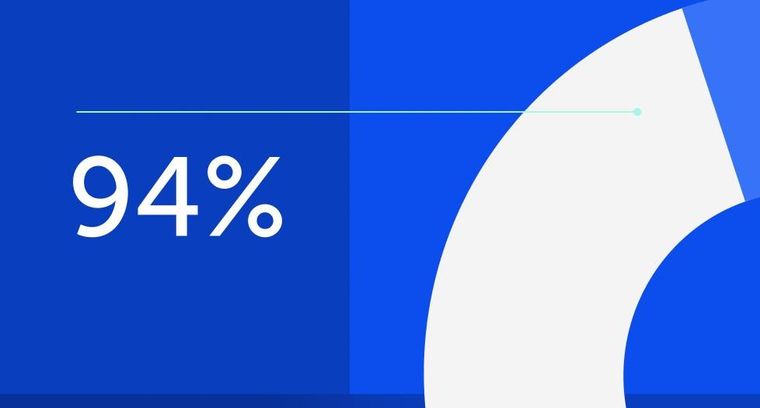
94% of researchers rate our articles as excellent or good
Learn more about the work of our research integrity team to safeguard the quality of each article we publish.
Find out more
REVIEW article
Front. Neurosci., 17 September 2021
Sec. Neurodegeneration
Volume 15 - 2021 | https://doi.org/10.3389/fnins.2021.741503
This article is part of the Research TopicModulating the Immune Response in Neurological DisordersView all 5 articles
Multiple sclerosis (MS) is an autoimmune disease characterized by chronic inflammation, neuronal degeneration and demyelinating lesions within the central nervous system. The mechanisms that underlie the pathogenesis and progression of MS are not fully known and current therapies have limited efficacy. Preclinical investigations using the murine experimental autoimmune encephalomyelitis (EAE) model of MS, as well as clinical observations in patients with MS, provide converging lines of evidence implicating the endogenous opioid system in the pathogenesis of this disease. In recent years, it has become increasingly clear that endogenous opioid peptides, binding μ- (MOR), κ- (KOR) and Γ-opioid receptors (DOR), function as immunomodulatory molecules within both the immune and nervous systems. The endogenous opioid system is also well known to play a role in the development of chronic pain and negative affect, both of which are common comorbidities in MS. As such, dysregulation of the opioid system may be a mechanism that contributes to the pathogenesis of MS and associated symptoms. Here, we review the evidence for a connection between the endogenous opioid system and MS. We further explore the mechanisms by which opioidergic signaling might contribute to the pathophysiology and symptomatology of MS.
Multiple sclerosis (MS) is a neuroinflammatory disease characterized by chronic inflammation, demyelinating lesions, and neurodegeneration within the central nervous system (CNS) (Compston and Coles, 2008; Polman et al., 2011). MS is a highly prevalent chronic condition and a leading cause of disability in North America (Browne et al., 2014). Despite decades of research, the complex pathogenesis of MS remains incompletely understood. While its exact etiology is unknown, it is generally believed that symptoms of MS result from damage to the myelin sheath and interruption of myelinated tracts in the CNS. As such, the diagnosis of MS is limited to the recurrent presentation of clinical symptoms that indicate CNS demyelination or the identification of radiologically observable demyelinated lesions within the CNS (Karussis, 2014). More recently, the presence of oligoclonal immunoglobulin bands specifically within the cerebrospinal fluid has been offered as an alternative diagnostic criterion to a secondary clinical or radiological event (Link and Huang, 2006; Carroll, 2018). Given that different neuroanatomical locations within the CNS can be involved in disease pathophysiology, MS can present with a wide range of symptoms. Clinical symptoms of the disease include motor, cognitive, sensory, and autonomic disturbances in most patients with MS. These can manifest as loss of coordination and balance, deficits in executive functioning, vision impairment, chronic pain, and mood disorders (Compston and Coles, 2008).
There is currently no cure for MS, although various forms of pharmaceutical and rehabilitation therapies are available for treating acute attacks, improving symptoms, and modifying the disease course (Gilmour et al., 2018). Given the chronic and heterogeneous nature of the disease, treatment with multiple concurrent therapies is frequent in clinical practice. Disease-modifying therapies interfere with the course of MS through modulation or suppression of the immune system. The disease-modifying therapies that are widely used in the clinic primarily inhibit lymphocyte access to the CNS, sequester lymphocytes in primary lymphoid organs, or deplete B cells (Vargas and Tyor, 2017; Greenfield and Hauser, 2018; Hauser and Cree, 2020). However, many of these therapies have considerable undesirable side effects and confer only partial protection against disease progression and symptomatology. Thus, there is an unmet clinical need to understand the complex pathophysiology of MS and identify novel drug targets.
A growing body of evidence suggests that the opioid system may contribute to the pathogenesis of MS and the development of comorbid symptoms. Endogenous opioid signaling is seemingly altered in people with MS (Gironi et al., 2000, 2008; Ludwig et al., 2017) and treatment with opioid therapies in the clinic is largely ineffective for pain management (Kalman et al., 2002). The role of endogenous opioid peptides and their receptors in the modulation of the immune system, nociceptive processes, and mood states has been well characterized. The interaction between these systems is complex and likely contributes to the MS disease course. The goal of this review is to discuss the role of the endogenous opioid system in MS. It will highlight pathophysiological mechanisms by which dysregulated opioid signaling may contribute to MS progression and symptomatology, with a focus on pain and affective disorders.
The endogenous opioid system plays a critical role in modulating nociception, affective states, motivational and mood processes, neuroendocrine function, respiratory activity, and autonomic stress and immunological responses. Opioid receptors and their ligands are widely distributed throughout the central and peripheral nervous systems, the immune system, and the gastrointestinal tract. Human (Kuhar et al., 1973; Peckys and Landwehrmeyer, 1999; Peng et al., 2012) and rodent studies (Mansour et al., 1987, 1994) have characterized the widespread but distinct expression of the opioid subsystems in various tissues and cell types. In the CNS, the opioid system is classically implicated in pain signaling and antinociception. Opioid receptors are highly expressed at all levels of the central pain control network. Activation of opioid receptors within the descending pain modulatory system, which consists of the periaqueductal gray, rostral ventromedial medulla, and dorsal horn of the spinal cord (Basbaum and Fields, 1984), suppresses spinal cord nociceptive transmission and contributes to opioid-induced antinociception (Tortorici et al., 2001; Wang and Wessendorf, 2002; Lueptow et al., 2018; Wang et al., 2018a). Opioid receptors modulate a diverse range of additional functions, such as mood and the stress response, which can be attributed to their expression throughout cortical, limbic and midbrain structures (Mansour et al., 1987; Likhtik et al., 2008; Peng et al., 2012; Vanāt Veer and Carlezon, 2013; Blaesse et al., 2015). Opioid receptors and their ligands are also found in neuronal and non-neuronal tissues, including cells of the immune (Wybran et al., 1979; Chuang et al., 1995; Bidlack, 2000) and enteric systems (Bagnol et al., 1997; Wood and Galligan, 2004; Poole et al., 2011).
The opioid system is comprised of three genetically distinct families of endogenous opioid peptides, including β-endorphin (derived from the precursor pro-opiomelanocortin), dynorphins (derived from pre-prodynorphin), and methionine (met)- and leucine (leu)-enkephalins (derived from pre-proenkephalin). All opioid peptides have a conserved NH2-terminal Tyr-Gly-Gly-Phe signature sequence that interacts with the classical opioid receptors: μ- (MOR), κ- (KOR), and Γ-opioid receptors (DOR). Each receptor is encoded by a unique gene (OPRM1, OPRK1, OPRD1, respectively). The opioid receptors are all seven-transmembrane spanning proteins that couple to inhibitory G proteins (Simon, 1991; Al-Hasani and Bruchas, 2011; Benarroch, 2012) to modulate intracellular signaling cascades involving the cyclic adenosine monophosphate pathway (Vigano et al., 2003). In general, β-endorphin binds to MOR and DOR, dynorphin preferentially binds KOR, and met- and leu-enkephalin bind DOR and MOR (Benarroch, 2012). Additional opioid peptides, such as endomorphin and nociceptin/orphanin FQ (N/OFQ), which have respective affinities for MOR and nociceptin/orphanin FQ receptor (NOP), have also been described (Meunier, 1997; Zadina et al., 1997; Horvath, 2000).
A connection between the opioid and immune systems is well established and has been detailed in several excellent reviews (Salzet et al., 2000; Vallejo et al., 2004; Al-Hashimi et al., 2013; Liang et al., 2016; Plein and Rittner, 2018; Eisenstein, 2019). The immunomodulatory properties of opioids were identified over 30 years ago, when Wybran and colleagues first reported the presence of opioid receptors in normal human T lymphocytes (Wybran et al., 1979). Subsequent studies detected the presence of transcripts for all three opioid receptor subtypes (MOR, DOR, and KOR) in cells of the immune system, including T cells, B cells, and macrophages (Chuang et al., 1995; Wick et al., 1996; Sharp et al., 1997; Bidlack, 2000; Ninkovic and Roy, 2013). Several immune cell types can stimulate the release or enhance the synthesis of endogenous opioid peptides. For example, the mRNA for β-endorphin, its precursor pro-opiomelanocortin, and proenkephalin are expressed by macrophages, monocytes, granulocytes, and T and B lymphocytes(Mousa et al., 2004; Pomorska et al., 2014). Under pathological pain and inflammatory conditions, leukocytes actively synthesize and secrete opioid peptides that interact with opioid receptors within inflamed tissue to produce analgesia (Stein et al., 1990; Przewlocki et al., 1992; Cabot et al., 1997). In addition, leukocyte-derived opioid peptides suppress neuropathy-induced mechanical allodynia in mice via opioid receptors expressed in nociceptors at the site of nerve injury (Labuz et al., 2009).
Endomorphin 1 and 2, two endogenous opioid peptides with high specificity and affinity for MOR, were originally detected in the CNS (Zadina et al., 1997) and later identified in cells and tissues of the immune system (Jessop et al., 2000; Mousa et al., 2002; Labuz et al., 2006). Accumulating evidence suggests that endomorphins, particularly endomorphin-1, possess potent antinociceptive and anti-inflammatory properties (PrzewÅocka et al., 1999; Jessop et al., 2010; Zhang et al., 2018). Endomorphin-1 increases the secretion of the anti-inflammatory cytokine interleukin (IL)-10 and suppresses the secretion of proinflammatory cytokines IL-12 and IL-23 in lipopolysaccharide-activated dendritic cells in vitro (Li et al., 2009). Investigations involving in vivo rodent models of acute inflammation have shown that local or intrathecal administration of endomorphin-1 improves peripheral inflammatory pain and reduces a localized inflammatory response (Khalil et al., 1999; McDougall et al., 2004; Zhang et al., 2018). Furthermore, the addition of endomorphin-2 in vitro inhibits the release of inflammatory mediators, such as tumor necrosis factor (TNF)-α and IL-12 by stimulated macrophage cells (Azuma and Ohura, 2002). Endomorphin-2 also attenuates macrophage chemotaxis and phagocytosis, suggesting that this peptide alters macrophage functions related to innate host defense (Azuma and Ohura, 2002).
β-endorphin and met-enkephalin have received significant attention for their influence on T lymphocyte function. The effects of these peptides on T lymphocytes have been explored by numerous investigators with conflicting results. Early in vitro investigations report that β-endorphin modifies T lymphocyte function by either enhancing (Gilman et al., 1982; Gilmore and Weiner, 1989; Hemmick and Bidlack, 1990; Van Den Bergh et al., 1991; Navolotskaya et al., 2002) or inhibiting proliferation and cytokine secretion (Hough et al., 1990; Garcia et al., 1992; Marchini et al., 1995; Panerai et al., 1995). More recently, it has been demonstrated that β-endorphin suppresses IL-2 transcription (Börner et al., 2009) and potentiates IL-4 expression in a human T lymphocyte cell line (Börner et al., 2013). Met-enkephalin is implicated in the regulation of neural and non-neural cell proliferation (Zagon and McLaughlin, 1991; Zagon et al., 2002; Donahue et al., 2009). In a similar manner to β-endorphin, treatment with met-enkephalin has been shown to increase (Hucklebridge et al., 1989; Bajpai et al., 1995; Kowalski, 1998; Zagon et al., 2011; Hua et al., 2012), suppress (Brown and Van Epps, 1985; Ye et al., 1989; Ohmori et al., 2009) or have no overall effect on T lymphocyte activity or proliferation (Gilman et al., 1982; Ye et al., 1989; Kamphuis et al., 1998). Dose-dependent effects of β-endorphin (Van Den Bergh et al., 1993) and met-enkephalin (Fóris et al., 1986; Piva et al., 2005) on T lymphocyte function have been reported, which may account for inconsistencies in the literature. For instance, Piva et al. (2005) found that low doses of met-enkephalin and its metabolic derivatives stimulated the production of several cytokines by splenocytes in vitro, whereas higher doses were suppressive (Piva et al., 2005). The discrepancies between investigations may also be due to differences in methodologies, including the concentration of the peptide in question, whether the peptides were natural or synthetic, whether the cells were stimulated or homeostatic, the presence or absence of serum in culture, and the types of assays used to assess cell proliferation. Nevertheless, it is clear that endogenous opioid peptides can influence immune cell function and may therefore contribute to immune system pathology as seen in MS.
Preclinical and clinical studies have demonstrated that exogenously administered opioids exert robust immunomodulatory effects, which are highly dependent on the type of opioid and the duration of exposure (Sacerdote et al., 2000; Martucci et al., 2004; Al-Hashimi et al., 2013; Franchi et al., 2019). For instance, chronic morphine treatment appears to have potent modulatory effects on the immune system, whereas codeine and hydromorphone do not (Sacerdote et al., 1997; Ninkovic and Roy, 2013). The modulatory effects of clinically used opioids on peripheral immune cells have been most extensively studied in vitro and in vivo (Vallejo et al., 2004; Ninkovic and Roy, 2013). The majority of experiments that involved the in vivo administration of opiates, such as morphine and heroin, or the addition of MOR, KOR, and DOR agonists to cell cultures in vitro, indicate significant suppression of the immune system. Immunosuppression was reported as reduced natural killer cell activity (Shavit et al., 1986b, a; Weber and Pert, 1989; Yeager et al., 1995; Sacerdote et al., 1997; GavƩriaux-Ruff et al., 1998), cytokine and chemokine production by peripheral blood mononuclear cells (Bussiere et al., 1993; Chao et al., 1993; Bonnet et al., 2008) and monocytes (Bussiere et al., 1993; Bian et al., 1995; Roy et al., 1998), T and B cell reactivity (Sacerdote et al., 1997; Govitrapong et al., 1998), phagocytic activity (Tubaro et al., 1985; Casellas et al., 1991; Rojavin et al., 1993; Szabo et al., 1993; Tomassini et al., 2004), as well the induction of macrophage apoptosis (Bhat et al., 2004; Lin et al., 2021). Additional evidence supporting the immunosuppressive role of opioid analgesics emerges from epidemiological studies showing increased prevalence of infections such as HIV, pneumonia, hepatitis and tuberculosis among opioid users (Nath et al., 2002; Quaglio et al., 2002; Roy et al., 2011; Wiese et al., 2018).
Multiple sclerosis immunopathology is generally thought to be mediated by myelin-reactive CD4+ T helper (Th) cells. Autoreactive effector CD4+ T cells can differentiate into Th1 or Th2 effector cells based upon their functions and cytokine profile. Disruption to the Th cell balance, especially the decrement of the Th1/Th2 ratio, is implicated in the development of several autoimmune diseases, including MS (van Langelaar et al., 2018; Kunkl et al., 2020). Similarly, several studies indicate that Th17 cells, a subset of CD4+ T-cells that produces IL-17, play a key role in the pathogenesis of various inflammatory and autoimmune diseases (Waite and Skokos, 2012; Yasuda et al., 2019; Moser et al., 2020). Modulation of T cell differentiation and function by opioids has been well documented and may therefore be relevant in MS immunopathology. Morphine has been shown to selectively direct T cells toward Th2 differentiation in vitro and in vivo, resulting in a shift in the Th1/Th2 balance (Roy et al., 2004; Han et al., 2020). Gao et al. (2012) demonstrated that the effects of morphine on CD4+ T lymphocytes isolated from healthy volunteers include altered cytokine expression, suppression of T cell apoptosis and Th cell differentiation, as well as an imbalance in the ratio of Th1/Th2 cells (Gao et al., 2012). Morphine dose-dependently suppresses the proliferative activity of phytohemagglutinin-stimulated T lymphocytes isolated from opioid-naive subjects in vitro (Govitrapong et al., 1998). In line with these findings, heroin users show reduced CD4+ T cell proliferative activity upon stimulation in vitro and an altered Th1/Th2 balance when compared with healthy controls and individuals on opioid maintenance therapy (Sacerdote et al., 2008; RiĆ et al., 2012). In rats, acute morphine exposure (Peng et al., 2020) and moderate doses of naltrexone (Xu et al., 2020) have been shown to suppress Th17 cell expression and function, as well as disrupt the balance between Th1 and Th2 cells (Xu et al., 2020). Moreover, treatment with chronic morphine enhances Th17 cell functional activity in peripheral blood mononuclear cells isolated from non-human primates (Cornwell et al., 2013).
Glial cells, consisting primarily of microglia, astrocytes, and oligodendrocytes, represent immune cells of the CNS. Several laboratories have demonstrated that microglia and astrocytes become activated in response to chronic morphine exposure, inducing the upregulation of proinflammatory cytokines IL-1, IL-6, and TNF-α, microglial and astrocytic activation markers, and purinergic receptors P2Ć4 and P2Ć7 (Raghavendra et al., 2002; Tawfik et al., 2005; Cui et al., 2006; Horvath and Deleo, 2009; Hutchinson et al., 2009; Watkins et al., 2009). Interfering with glial function reduces opioid tolerance and opioid-induced hyperalgesia, providing further evidence for the modulatory role of opioids on glial cells (Raghavendra et al., 2002, 2003; Eidson and Murphy, 2013).
Overwhelming evidence indicates that endogenous opioid peptides and clinically used opioids have significant influence on innate and adaptive immunity. While the etiology of MS remains incompletely understood, it is recognized that the pathogenesis and progression of this disease are mediated by the immune system. Thus, it is important to elucidate the relationship between the opioid and immune systems in the context of MS to gain mechanistic insight into pathophysiological processes associated with this disease.
Human and animal studies provide converging lines of evidence indicating that perturbations to the endogenous opioid system contribute to the pathogenesis of several autoimmune disorders, including MS. Patients with MS show decreased concentrations of endogenous opioid peptides β-endorphin and enkephalin in peripheral blood mononuclear cells and cerebrospinal fluid samples compared with healthy controls (Panerai et al., 1994; Gironi et al., 2000, 2008; Ludwig et al., 2017). Mice with experimental autoimmune encephalomyelitis (EAE), the most commonly used preclinical murine model of MS, also show a marked reduction in serum concentrations of met-enkephalin compared with baseline levels and with controls prior to the onset of clinical behavioral signs of disease (Ludwig et al., 2017; Patel et al., 2020). Studies assessing changes in endogenous opioid peptide and receptor expression in MS patients and animal models are summarized in Table 1. EAE is a CD4+ T lymphocyte-mediated demyelinating autoimmune disease of the CNS, characterized by widespread central inflammation and infiltration of T cells and monocytes into the CNS (Robinson et al., 2014). The EAE model shares many pathological features with MS, including neuroinflammation, demyelination, neurodegeneration, axonopathy and pain (Olechowski et al., 2009; Kipp et al., 2012; Potter et al., 2016; Catuneanu et al., 2019). Given the role of met-enkephalin in modulating adaptive immune cell reactivity (Zagon and McLaughlin, 1991; Zagon et al., 2002; Malendowicz et al., 2005; Donahue et al., 2009), reduced serum enkephalin levels in MS patients may promote immune cell proliferation and drive immune-mediated flares. Indeed, a series of investigations reveal that increasing levels of met-enkephalin confer a neuroprotective effect in mice with EAE and people with MS (Gironi et al., 2008; Zagon et al., 2009, 2010; Ludwig et al., 2017). Jankovic and Maric (1987) demonstrated that injections of met-enkephalin to rats with EAE prevents or delays paralysis. Daily administration of met-enkephalin to mice with EAE at the time of disease induction prevents the onset and progression of disease, and decreases overall disease severity, areas of demyelination, and activated glia in the spinal cord relative to saline-treated controls (Zagon et al., 2010; Rahn et al., 2011; Patel et al., 2020). In mice with established EAE, treatment with met-enkephalin halts the progression of disease, improves the clinical behavioral scores, and reduces the number of activated glia, T cells, and demyelinated areas in the spinal cord (Campbell et al., 2012, 2013). Moreover, treatment with met-enkephalin in mice with relapse-remitting EAE results in less severe clinical disease scores, fewer and shorter relapses, and diminished glial activation and spinal cord pathology compared to controls (Hammer et al., 2013). In summary, results from studies involving exogenous therapy with enkephalins in the EAE model indicate beneficial effects of modulating endogenous opioid levels and suggest that the opioid system plays an integral role in the underlying disease process.
Table 1. Changes in endogenous opioid peptide and receptor expression in MS patients and animal models.
Naltrexone is a non-selective opioid receptor antagonist that is primarily prescribed for the treatment of opioid addiction in daily doses of at least 50 mg (Minozzi et al., 2011). When prescribed at the lowest dosage levels (1ā4.5 mg), it acts as an immune modulator by reducing the inflammatory glial response (Mattioli et al., 2010; Younger et al., 2014), in addition to systemically upregulating endogenous opioid signaling by transient opioid receptor blockade (Gironi et al., 2008; Ludwig et al., 2017). Treatment with low dose naltrexone has been demonstrated to improve symptoms in a variety of chronic inflammatory conditions, including Crohnās disease (Lie et al., 2018), fibromyalgia (Younger and Mackey, 2009; Younger et al., 2013), complex regional pain syndrome (Chopra and Cooper, 2013; Weinstock et al., 2016), and MS (Gironi et al., 2008; Cree et al., 2010; Sharafaddinzadeh et al., 2010; Ludwig et al., 2017).
Preclinical studies wherein mice were immunized with EAE report beneficial effects of low dose naltrexone treatment in modulating disease processes (Zagon et al., 2009; Hammer et al., 2013, 2015; Ludwig et al., 2017). Therapy with low dose naltrexone, but not high dose naltrexone, prevents neurological signs of disease, suppresses disease onset and progression, and reduces the number of activated astrocytes in the spinal cord of EAE mice (Zagon et al., 2009). In addition, mice with chronic EAE receiving low dose naltrexone show reduced sensitivity to heat relative to saline-treated EAE mice (Ludwig et al., 2017). In studies with mice immunized with relapsing-remitting EAE, treatment with low dose naltrexone initiated at the time of established disease significantly diminishes behavioral scores and increases the incidence and lengthens the time of remissions compared with EAE mice treated with saline (Hammer et al., 2015). Low dose naltrexone therapy also reduces numbers of inflammatory cells, such as microglia, CD3+ T cells, and activated astrocytes, as well as areas of demyelination in the lumbar spinal cord (Hammer et al., 2015). Recent work extended these findings and demonstrated that treatment with low dose naltrexone preserves myelin basic protein expression and the number of oligodendrocytes within the spinal cord in EAE mice relative to control animals (Patel et al., 2020).
Results from clinical trials suggest that low dose naltrexone treatment enhances the quality of life of patients with MS (Gironi et al., 2008; Cree et al., 2010) and that treatment is well tolerated (Gironi et al., 2008; Cree et al., 2010; Sharafaddinzadeh et al., 2010). The first multi-center open-label pilot study involving 40 patients with primary progressive MS reported that spasticity was significantly reduced following 6 months of treatment with low dose naltrexone (Gironi et al., 2008). Levels of β-endorphin in patientsā peripheral blood mononuclear cells increased concurrently with low dose naltrexone administration, providing support for a potential mechanism of action. The interpretation of these results, however, is limited by the uncontrolled design of the study and the small sample size. More recently, low dose naltrexone therapy was shown to restore depressed serum enkephalin levels of MS patients to non-MS patient concentrations (Ludwig et al., 2017). An additional randomized, placebo-controlled study comprised of 60 MS patients found that 8 weeks of therapy with low dose naltrexone was associated with significant improvement in self-reported mental health outcome measures (Cree et al., 2010). By contrast, a 17-week randomized, double-blind, placebo-controlled clinical trial involving 96 MS patients found no statistically significant improvements in self-reported quality of life following low dose naltrexone treatment between groups (Sharafaddinzadeh et al., 2010). The authors noted that low dose naltrexone therapy was relatively safe and that longer trials are needed to conclude that there is no beneficial effect.
Nevertheless, high quality clinical studies evaluating the therapeutic effects of low dose naltrexone in treating MS are lacking. Of the completed studies, results indicate that low dose naltrexone is generally safe, compatible with currently recommended MS treatments and well tolerated, but do not show significant changes in symptoms beyond quality-of-life improvements. Low dose naltrexone may be a promising alternative or adjunct therapy for MS treatment; however, additional research is necessary to determine the clinical potential of low dose naltrexone use in MS.
There is emerging evidence indicating that targeting the kappa opioid system may be a promising therapeutic target for attenuating the progression of MS via remyelination (Wang and Mei, 2019). An in vitro myelination assay has shown that KOR agonism promotes differentiation of oligodendrocyte precursor cells (OPCs) into mature oligodendrocytes and subsequent myelination (Mei et al., 2016). This beneficial effect on myelination is abolished in mice that have KOR conditionally knocked out in OPCs. This study provides support that KOR ligands are directly acting on KORs expressed on OPCs and suggests that future studies should consider targeting this receptor for remyelination therapy (Mei et al., 2016). In line with these results, treatment with a selective KOR agonist, U50,488H, has been shown to enhance remyelination in lysolecithin-, hypoxia-, and cuprizone-induced demyelination models (Mei et al., 2016; Wang et al., 2018b), and has since been replicated in the EAE model using a delayed treatment schedule (Denny et al., 2021). Furthermore, Du et al. (2016) found that administration of U50,488H reduced the severity of motor impairments in EAE mice through promoting oligodendrocyte differentiation and remyelination. The authors also induced EAE in opioid receptor knockout mice. MOR-deficient mice did not show any changes in the severity or progression of EAE, DOR knockout mice only displayed a small increase in peak disease severity, and genetic deletion of KOR worsened disease severity compared with wild-type (Du et al., 2016). These data indicate that KOR contributes to remyelinating processes and that targeting the kappa opioid system is an intriguing avenue for developing novel therapeutics for the treatment of MS.
Chronic pain is one of the most frequent and debilitating symptoms of MS, affecting between 50ā80% of patients over the course of their disease (Ćsterberg et al., 2005). MS-related pain is characterized by hyperalgesia (enhanced pain responses to noxious input) and allodynia (perception of innocuous stimuli as painful). MS patients often describe their pain as constant, bilateral aching, burning, and pricking sensations in both the lower and upper extremities (Ćsterberg et al., 2005). Classical pain treatments, such as opioid therapy, are typically ineffective in treating MS-related pain, with only a minority of patients receiving significant relief (Kalman et al., 2002). Chronic pain associated with MS represents a significant clinical and societal burden. As the general population ages, it can be expected that the rates of MS will only increase, thus it is becoming increasingly imperative that adequate treatments for pain in this disease are developed. A more thorough understanding of the basic mechanisms driving pain in MS is necessary for the development of novel therapies to improve pain management for this patient population.
There are a large number of autoimmune and inflammatory diseases with different etiologies and symptomatologies, including rheumatoid arthritis, irritable bowel syndrome, complex regional pain syndrome, and MS, and pain appears to be a common factor in most of these conditions (Mifflin and Kerr, 2017). Activation of the endogenous opioid system is evidenced in a variety of these conditions that are associated with the development of pathological pain. Human positron emission tomography studies show that compared with controls, patients with rheumatoid arthritis (Jones et al., 1994), complex regional pain syndrome (Klega et al., 2010), and central neuropathic pain following stroke (Willoch et al., 2004) have reduced opioid receptor binding potential at several neural loci involved in the central pain matrix and emotional regulation. This may indicate increased occupancy of receptors by endogenous opioid peptides or a reduction in available receptors for binding. Work from animal studies further corroborate the release of endogenous peptides in chronic pain states. For example, experimental hindpaw inflammation induces a rapid increase in pre-prodynorphin mRNA and a prolonged increase in a dynorphin peptide in the spinal cord (Iadarola et al., 1988). These data collectively suggest that several inflammatory pain states are associated with the release and binding of endogenous opioids to their cognate receptors. However, studies that directly investigate the contribution of the opioid system to pain hypersensitivity in MS and EAE are limited.
As discussed above, the role of endogenous opioids in MS has primarily been evaluated in the context of immunity and disease progression. Although the contribution of opioidergic neurotransmission to MS-related pain remains relatively unexplored, there is evidence to indicate that dysfunction of the opioid system may be implicated in the development and maintenance of pain in this disease (summarized in Table 2). Similar to that observed in other chronic pain conditions (ArnĆ©r and Meyerson, 1988; Zurek et al., 2001; Luger et al., 2002; Rowbotham et al., 2003; Chen et al., 2013; Kissin, 2013), opioid analgesics often provide inadequate relief for MS patients, except at high doses that might enhance the risk for adverse side effects (Kalman et al., 2002). Animal models of MS-related pain also show reduced opioid analgesia compared with controls (Lynch et al., 2008; Dworsky-Fried et al., 2021). We previously reported that morphine lacks potent analgesic efficacy in female mice induced with EAE at a time point that was associated with peak pain hypersensitivity and inflammation in the brain (Dworsky-Fried et al., 2021). Consistent with these findings, male and female mice infected with Theilerās murine encephalomyelitis virus (TMEV) as a model for MS display a loss of morphine analgesia compared to uninfected control mice (Lynch et al., 2008). Mice infected with TMEV also show reductions in spinal cord mRNA levels of all three opioid receptors (MOR, DOR, and KOR), which correlated with the development of thermal and mechanical hyperalgesia (Lynch et al., 2008). While this study did not investigate the causal relations between receptor changes and pain behaviors, decreases in spinal opioid receptors may explain the increased central neuropathic pain commonly observed in MS patients (OāConnor et al., 2008; Khan and Smith, 2014). From a clinical perspective, dysregulation of the opioid system might also help to explain the poor patient response to this class of analgesics (Kalman et al., 2002). Taken together, these findings provide support for the hypothesis that loss of endogenous antinociceptive processes mediated by the opioid system contribute to MS- and EAE-related pain. As such, restoring opioid system function may be a viable target for novel analgesic drugs and therapeutics to manage pain in this disease. Additional investigations are needed to understand the contribution of endogenous opioids and receptors to pathological pain in MS.
Mental health comorbidities are highly prevalent among individuals with MS. Depression is the most common of these comorbidities, affecting approximately 50% of people with MS (Marrie et al., 2009). This is nearly three times higher than the current rate of depression in the general United States population (Villarroel and Terlizzi, 2020). People living with MS are twice as likely to commit suicide as someone without MS, with suicidal ideation showing a similar increase (Turner et al., 2006; Feinstein and Pavisian, 2017). Studies using functional magnetic resonance imaging reveal that MS patients with chronic pain show structural and functional alterations in brain regions involved in reward processing, which are associated with impaired reward responsiveness and depression (Pardini et al., 2013; Seixas et al., 2016; Heitmann et al., 2020). Pharmacological treatment of depression is not often pursued in people with MS because there is limited evidence to support a beneficial effect in this patient population (Minden et al., 2014; Patten et al., 2017). In addition, there are reports of a possible harmful interaction between some antidepressants and the initiation of fingolimod useāa common immunomodulatory drug prescribed for managing MS (Patten et al., 2017). This undertreatment of negative affect in people with MS poses a significant problem and has profound impacts on quality of life. As such, understanding whether dysfunction of the opioid system is involved in the etiology of mood disorders in MS is critical for effective management of this condition.
In recent years, dysfunction of the endogenous opioid system has garnered significant attention as a key component in depressive symptomatology and pathophysiology (Hegadoren et al., 2009; Peciña et al., 2019). Preclinical and clinical studies provide evidence of opioid system involvement in negative affective states. For instance, individuals with a history of depression who commit suicide have increased MOR density in the brain, specifically in the prefrontal cortex, temporal cortex, and basal ganglia (Gross-Isseroff et al., 1990; Gabilondo et al., 1995). Women with major depressive disorder exhibit increased MOR system activation compared with control subjects (Kennedy et al., 2006). Indeed, increases in KOR and MOR protein in the blood serum have become targets for biomarker identification of major depressive disorder in humans (Al-Hakeim et al., 2019, 2020). Clinical reports have described the effectiveness of MOR agonists, including oxycodone, tramadol, oxymorphone, and buprenorphine, as well as β-endorphin, in patients suffering from depression (Darko et al., 1992; Bodkin et al., 1995; Stoll and Rueter, 1999; Shapira and DeGraw, 2001). Preclinical studies using animal paradigms of depression corroborate these findings (Rojas-Corrales et al., 1998, 2004). The evidence of opioid system dysfunction as an important mechanism driving negative affect suggests that altered opioidergic mechanisms may also play a role in the development of comorbid mood disorders in MS.
The EAE mouse model is a useful tool for modeling the affective symptoms of MS (Pollak et al., 2002). Affective disturbances, such as depressive- and anxiety-like behaviors, and cognitive and memory dysfunction have been noted early in the EAE disease course, similar to the clinical population (Pollak et al., 2002; Peruga et al., 2011; Acharjee et al., 2013; Olechowski et al., 2013). Several studies report that mice with EAE show higher levels of anxious and depressive behaviors than control mice in a variety of experimental paradigms including the elevated plus maze, open field test, forced swim test, tail suspension test, and social interaction test (Acharjee et al., 2013; Olechowski et al., 2013). Using the conditioned place preference assay to assess drug reinforcement, our group has recently demonstrated that morphine reward is blunted in mice with EAE (Dworsky-Fried et al., 2021). This finding indicates that affective and reward processing is disrupted in the EAE model, and is consistent with other reports indicating dysregulated reward processing in chronic pain states (Ozaki et al., 2002, 2003; Martin et al., 2007; Petraschka et al., 2007; Niikura et al., 2008). Although literature that focuses on the involvement of the endogenous opioid system in MS-related mood and affective disorders is limited, existing evidence warrants further exploration into this research avenue. Understanding whether disruptions to endogenous opioid signaling contribute to impaired mood and reward regulation in MS is paramount for future treatment of this comorbidity.
Opioid peptides and their receptors are intimately involved in regulating various aspects of immune function, nociceptive processing, and affective states. Dysregulation of the opioid system may be an important mechanism to help explain the pathophysiology of MS, as well as the pathological pain and disordered mood commonly observed in this disease. Therefore, it is of interest to further investigate and consider the opioid system as a potentially attractive therapeutic target for MS and its symptoms.
Although MS is a highly prevalent autoimmune disorder, a comprehensive understanding of the pathogenesis and symptomatology of the disease is still lacking. Accumulating data imply functional association between endogenous opioid systems and MS. Patients with MS and animal models show decreased levels of endogenous opioid peptides compared with healthy controls (Panerai et al., 1994; Gironi et al., 2000, 2008; Ludwig et al., 2017; Patel et al., 2020) and some clinical trials have shown beneficial effects of therapies that enhance endogenous opioid concentrations (Gironi et al., 2008; Ludwig et al., 2017). Moreover, opioid analgesics often provide inadequate pain relief for patients with MS. Given the complex interactions between the opioid and immune systems, nociceptive processing, and mood regulation as discussed in this review, targeting opioidergic mechanisms may provide an effective measure to interfere with the development and progression of MS and improve disabling symptoms.
ZD-F and AT conceptualized the work. ZD-F drafted the initial manuscript and edited the text. ZD-F and CC reviewed the literature and contributed to the final manuscript text. CC created the tables. BK and AT provided the editorial comments. All authors contributed to the manuscript revision and approved the submitted version.
This work was supported by a Project Grant from the Canadian Institutes for Health Research (CIHR) FRN:162434.
The authors declare that the research was conducted in the absence of any commercial or financial relationships that could be construed as a potential conflict of interest.
All claims expressed in this article are solely those of the authors and do not necessarily represent those of their affiliated organizations, or those of the publisher, the editors and the reviewers. Any product that may be evaluated in this article, or claim that may be made by its manufacturer, is not guaranteed or endorsed by the publisher.
Acharjee, S., Nayani, N., Tsutsui, M., Hill, M. N., Ousman, S. S., and Pittman, Q. J. (2013). Altered cognitive-emotional behavior in early experimental autoimmune encephalitis - cytokine and hormonal correlates. Brain Behav. Immun. 33, 164ā172. doi: 10.1016/j.bbi.2013.07.003
Al-Hakeim, H. K., Al-Fadhel, S. Z., Al-Dujaili, A. H., Carvalho, A., Sriswasdi, S., and Maes, M. (2019). Development of a novel neuro-immune and opioid-associated fingerprint with a cross-validated ability to identify and authenticate unknown patients with major depression: far beyond differentiation, discrimination, and classification. Mol. Neurobiol. 56, 7822ā7835. doi: 10.1007/s12035-019-01647-1640
Al-Hakeim, H. K., Zeki Al-Fadhel, S., Al-Dujaili, A. H., and Maes, M. (2020). In major depression, increased kappa and mu opioid receptor levels are associated with immune activation. Acta Neuropsychiatr. 32, 99ā108. doi: 10.1017/neu.2019.47
Al-Hasani, R., and Bruchas, M. R. (2011). Molecular mechanisms of opioid receptor-dependent signaling and behavior. Anesthesiology 115, 1363ā1381. doi: 10.1097/ALN.0b013e318238bba6
Al-Hashimi, M., Scott, S. W. M., Thompson, J. P., and Lambert, D. G. (2013). Opioids and immune modulation: more questions than answers. Br. J. Anaesth. 111, 80ā88. doi: 10.1093/bja/aet153
ArnĆ©r, S., and Meyerson, B. A. (1988). Lack of analgesic effect of opioids on neuropathic and idiopathic forms of pain. Pain 33, 11ā23. doi: 10.1016/0304-3959(88)90198-90194
Azuma, Y., and Ohura, K. (2002). Endomorphins 1 and 2 inhibit IL-10 and IL-12 production and innate immune functions, and potentiate NF-ĪŗB DNA binding in THP-1 differentiated to macrophage-like cells. Scand. J. Immunol. 56, 260ā269. doi: 10.1046/j.1365-3083.2002.01128.x
Bagnol, D., Mansour, A., Akil, H., and Watson, S. J. (1997). Cellular localization and distribution of the cloned mu and kappa opioid receptors in rat gastrointestinal tract. Neuroscience 81, 579ā591. doi: 10.1016/S0306-4522(97)00227-223
Bajpai, K., Singh, V. K., Agarwal, S. S., Dhawan, V. C., Naqvi, T., Haq, W., et al. (1995). Immunomodulatory activity of met-enkephalin and its two potent analogs. Int. J. Immunopharmacol. 17, 207ā212. doi: 10.1016/0192-0561(94)00080-88
Basbaum, A. I., and Fields, H. L. (1984). Endogenous pain control systems: brainstem spinal pathways and endorphin circuitry. Annu. Rev. Neurosci. 7, 309ā338. doi: 10.1146/annurev.ne.07.030184.001521
Benarroch, E. E. (2012). Endogenous opioid systems: current concepts and clinical correlations. Neurology 79, 807ā814. doi: 10.1212/WNL.0b013e3182662098
Bhat, R. S., Bhaskaran, M., Mongia, A., Hitosugi, N., and Singhal, P. C. (2004). Morphine-induced macrophage apoptosis: oxidative stress and strategies for modulation. J. Leukoc. Biol. 75, 1131ā1138. doi: 10.1189/jlb.1203639
Bian, T. H., Wang, X. F., and Li, X. Y. (1995). Effect of morphine on interleukin-1 and tumor necrosis factor α production from mouse peritoneal macrophages in vitro. Acta Pharmacol. Sin. 16, 449ā451.
Bidlack, J. M. (2000). Detection and function of opioid receptors on cells from the immune system. Clin. Diagn. Lab. Immunol. 7, 719ā723. doi: 10.1128/cdli.7.5.719-723.200010.1128/cdli.7.5.719-723.2000
Blaesse, P., Goedecke, L., Bazelot, M., Capogna, M., Pape, H. C., and Jüngling, K. (2015). μ-Opioid receptor-mediated inhibition of intercalated neurons and effect on synaptic transmission to the central amygdala. J. Neurosci. 35, 7317ā7325. doi: 10.1523/JNEUROSCI.0204-15.2015
Bodkin, A. J., Zornberg, G. L., Lukas, S. E., and Cole, J. O. (1995). Buprenorphine treatment of refractory depression. J. Clin. Psychopharmacol. 15, 49ā57. doi: 10.1097/00004714-199502000-199502008
Bonnet, M. P., Beloeil, H., Benhamou, D., Mazoit, J. X., and Asehnoune, K. (2008). The μ opioid receptor mediates morphine-induced tumor necrosis factor and interleukin-6 inhibition in toll-like receptor 2-stimulated monocytes. Anesth. Analg. 106, 1142ā1149. doi: 10.1213/ane.0b013e318165de89
Bƶrner, C., Lanciotti, S., Koch, T., Hƶllt, V., and Kraus, J. (2013). Mu opioid receptor agonist-selective regulation of interleukin-4 in T lymphocytes. J. Neuroimmunol. 263, 35ā42. doi: 10.1016/j.jneuroim.2013.07.012
Bƶrner, C., Warnick, B., Smida, M., Hartig, R., Lindquist, J. A., Schraven, B., et al. (2009). Mechanisms of opioid-mediated inhibition of human T cell receptor signaling. J. Immunol. 183, 882ā889. doi: 10.4049/jimmunol.0802763
Brown, S. L., and Van Epps, D. E. (1985). Suppression of T lymphocyte chemotactic factor production by the opioid peptides beta-endorphin and met-enkephalin. J. Immunol. 134, 3384ā3390.
Browne, P., Chandraratna, D., Angood, C., Tremlett, H., Baker, C., Taylor, B. V., et al. (2014). Atlas of multiple sclerosis 2013: a growing global problem with widespread inequity. Neurology 83, 1022ā1024. doi: 10.1212/WNL.0000000000000768
Bussiere, J. L., Adler, M. W., Rogers, T. J., and Eisenstein, T. K. (1993). Cytokine reversal of morphine-induced suppression of the antibody response 1. J. Pharmacol. Exp. Ther. 264, 591ā597.
Cabot, P. J., Carter, L., Gaiddon, C., Zhang, Q., SchƤfer, M., Loeffler, J. P., et al. (1997). Immune Cell-derived beta-endorphin: production, release, and control of inflammatory pain in rats. J. Clin. Invest. 100, 142ā148. doi: 10.1172/JCI119506
Campbell, A. M., Zagon, I. S., and McLaughlin, P. J. (2012). Opioid growth factor arrests the progression of clinical disease and spinal cord pathology in established experimental autoimmune encephalomyelitis. Brain Res. 1472, 138ā148. doi: 10.1016/j.brainres.2012.07.006
Campbell, A. M., Zagon, I. S., and Mclaughlin, P. J. (2013). Astrocyte proliferation is regulated by the OGF-OGFr axis in vitro and in experimental autoimmune encephalomyelitis. Brain Res. Bull. 90, 43ā51. doi: 10.1016/j.brainresbull.2012.09.001
Carroll, W. M. (2018). 2017 mcdonald MS diagnostic criteria: evidence-based revisions. Mult. Scler. 24, 92ā95. doi: 10.1177/1352458517751861
Casellas, A. M., Guardiola, H., and Renaud, F. L. (1991). Inhibition by opioids of phagocytosis in peritoneal macrophages. Neuropeptides 18, 35ā40. doi: 10.1016/0143-4179(91)90161-B
Catuneanu, A., Paylor, J. W., Winship, I., Colbourne, F., and Kerr, B. J. (2019). Sex differences in central nervous system plasticity and pain in experimental autoimmune encephalomyelitis. Pain 160, 1037ā1049. doi: 10.1097/j.pain.0000000000001483
Chao, C. C., Molitor, T. W., Close, K., Hu, S., and Peterson, P. K. (1993). Morphine inhibits the release of tumor necrosis factor in human peripheral blood mononuclear cell cultures. Int. J. Immunopharmacol. 15, 447ā453. doi: 10.1016/0192-0561(93)90057-90056
Chen, L., Vo, T., Seefeld, L., Malarick, C., Houghton, M., Ahmed, S., et al. (2013). Lack of correlation between opioid dose adjustment and pain score change in a group of chronic pain patients. J. Pain 14, 384ā392. doi: 10.1016/j.jpain.2012.12.012
Chopra, P., and Cooper, M. S. (2013). Treatment of complex regional pain syndrome (CRPS) using low dose naltrexone (LDN). J. Neuroimmune Pharmacol. 8, 470ā476. doi: 10.1007/s11481-013-9451-y
Chuang, T. K., Killam, K. F., Chuang, L. F., Kung, H. F., Sheng, W. S., Chao, C. C., et al. (1995). Mu opioid receptor gene expression in immune cells. Biochem. Biophys. Res. Commun. 216, 922ā930. doi: 10.1006/bbrc.1995.2709
Compston, A., and Coles, A. (2008). Multiple sclerosis. Lancet 372, 1502ā1517. doi: 10.1016/S0140-6736(08)61620-61627
Cornwell, W. D., Lewis, M. G., Fan, X., Rappaport, J., and Rogers, T. J. (2013). Effect of chronic morphine administration on circulating T cell population dynamics in rhesus macaques. J. Neuroimmunol. 265, 43ā50. doi: 10.1016/j.jneuroim.2013.09.013
Cree, B. A. C., Kornyeyeva, E., and Goodin, D. S. (2010). Pilot trial of low-dose naltrexone and quality of life in multiple sclerosis. Ann. Neurol. 68, 145ā150. doi: 10.1002/ana.22006
Cui, Y., Chen, Y., Zhi, J.-L., Guo, R.-X., Feng, J.-Q., and Chen, P.-X. (2006). Activation of p38 mitogen-activated protein kinase in spinal microglia mediates morphine antinociceptive tolerance. Brain Res. 1069, 235ā243. doi: 10.1016/j.brainres.2005.11.066
Darko, D. F., Risch, S. C., Gillin, J. C., and Golshan, S. (1992). Association of β-endorphin with specific clinical symptoms of depression. Am. J. Psychiatry 149, 1162ā1167. doi: 10.1176/ajp.149.9.1162
Denny, L., Al Abadey, A., Robichon, K., Templeton, N., Prisinzano, T. E., Kivell, B. M., et al. (2021). Nalfurafine reduces neuroinflammation and drives remyelination in models of CNS demyelinating disease. Clin. Transl. Immunol. 10:e1234. doi: 10.1002/cti2.1234
Donahue, R. N., McLaughlin, P. J., and Zagon, I. S. (2009). Cell proliferation of human ovarian cancer is regulated by the opioid growth factor-opioid growth factor receptor axis. Am. J. Physiol. Regul. Integr. Comp. Physiol. 296, 1716ā1725. doi: 10.1152/ajpregu.00075.2009
Du, C., Duan, Y., Wei, W., Cai, Y., Chai, H., Lv, J., et al. (2016). Kappa opioid receptor activation alleviates experimental autoimmune encephalomyelitis and promotes oligodendrocyte-mediated remyelination. Nat. Commun. 7:11120. doi: 10.1038/ncomms11120
Dworsky-Fried, Z., Faig, C. A., Vogel, H. A., Kerr, B. J., and Taylor, A. M. W. (2021). Central amygdala inflammation drives pain hypersensitivity and attenuates morphine analgesia in experimental autoimmune encephalomyelitis. Pain doi: 10.1097/j.pain.0000000000002307 [Online ahead of print].
Eidson, L. N., and Murphy, A. Z. (2013). Blockade of toll-like receptor 4 attenuates morphine tolerance and facilitates the pain relieving properties of morphine. J. Neurosci. 33, 15952ā15963. doi: 10.1523/JNEUROSCI.1609-13.2013
Eisenstein, T. K. (2019). The role of opioid receptors in immune system function. Front. Immunol. 10:2904. doi: 10.3389/fimmu.2019.02904
Feinstein, A., and Pavisian, B. (2017). Multiple sclerosis and suicide. Mult. Scler. 23, 923ā927. doi: 10.1177/1352458517702553
Fóris, G., Medgyesi, G. A., and Hauck, M. (1986). Bidirectional effect of met-enkephalin on macrophage effector functions. Mol. Cell. Biochem. 69, 127ā137.
Franchi, S., Moschetti, G., Amodeo, G., and Sacerdote, P. (2019). Do all opioid drugs share the same immunomodulatory properties? A review from animal and human studies. Front. Immunol. 10:2914. doi: 10.3389/fimmu.2019.02914
Gabilondo, A. M., Javier Meana, J., and GarcĆa-Sevilla, J. A. (1995). Increased density of μ-opioid receptors in the postmortem brain of suicide victims. Brain Res. 682, 245ā250. doi: 10.1016/0006-8993(95)00333-L
Gao, M., Sun, J., Jin, W., and Qian, Y. (2012). Morphine, but not ketamine, decreases the ratio of Th1/Th2 in CD4-positive cells through T-bet and GATA3. Inflammation 35, 1069ā1077. doi: 10.1007/s10753-011-9413-9416
Garcia, I., Perez-Castillo, A., Moreno, J., and Alemany, S. (1992). β-Endorphin inhibits interleukin-2 release and expression of interleukin- 2 receptors in concanavalin A-stimulated splenic lymphocytes. Lymphokine Cytokine Res. 11, 339ā345.
GavĆ©riaux-Ruff, C., Matthes, H. W. D., Peluso, J., and Kieffer, B. L. (1998). Abolition of morphine-immunosuppression in mice lacking the μ-opioid receptor gene. Proc. Natl. Acad. Sci. U. S. A. 95, 6326ā6330. doi: 10.1073/pnas.95.11.6326
Gilman, S. C., Schwartzt, J. M., Milnert, R. J., Bloomt, F. E., and Feldman, J. D. (1982). β-Endorphin enhances lymphocyte proliferative responses (neuropeptides/mitogens/lymphocyte activation/opiates/stress). Proc. Natl. Acad. Sci. U. S. A. 79, 4226ā4230.
Gilmore, W., and Weiner, L. P. (1989). The opioid specificity of beta-endorphin enhancement of murine lymphocyte proliferation. Immunopharmacology 17, 19ā30. doi: 10.1016/0162-3109(89)90004-90000
Gilmour, H., Ramage-Morin, P. L., and Wong, S. L. (2018). Multiple sclerosis: prevalence and impact. Health Rep. 29, 3ā8.
Gironi, M., Martinelli, V., Brambilla, E., Furlan, R., Panerai, A. E., Comi, G., et al. (2000). β-Endorphin concentrations in peripheral blood mononuclear cells of patients with multiple sclerosis: effects of treatment with interferon beta. Arch. Neurol. 57, 1178ā1181. doi: 10.1001/archneur.57.8.1178
Gironi, M., Martinelli-Boneschi, F., Sacerdote, P., Solaro, C., Zaffaroni, M., Cavarretta, R., et al. (2008). A pilot trial of low-dose naltrexone in primary progressive multiple sclerosis. Mult. Scler. 14, 1076ā1083. doi: 10.1177/1352458508095828
Govitrapong, P., Suttitum, T., Kotchabhakdi, N., and Uneklabh, T. (1998). Alterations of immune functions in heroin addicts and heroin withdrawal subjects. J. Pharmacol. Exp. Ther. 286, 883ā889.
Greenfield, A. L., and Hauser, S. L. (2018). B-cell therapy for multiple sclerosis: entering an era. Ann. Neurol. 83, 13ā26. doi: 10.1002/ana.25119
Gross-Isseroff, R., Dillon, K. A., Israeli, M., and Biegon, A. (1990). Regionally selective increases in μ opioid receptor density in the brains of suicide victims. Brain Res. 530, 312ā316. doi: 10.1016/0006-8993(90)91301-V
Hammer, L. A., Zagon, I. S., and McLaughlin, P. J. (2013). Treatment of a relapse-remitting model of multiple sclerosis with opioid growth factor. Brain Res. Bull. 98, 122ā131. doi: 10.1016/j.brainresbull.2013.08.001
Hammer, L. A., Zagon, I. S., and McLaughlin, P. J. (2015). Improved clinical behavior of established relapsing-remitting experimental autoimmune encephalomyelitis following treatment with endogenous opioids: implications for the treatment of multiple sclerosis. Brain Res. Bull. 112, 42ā51. doi: 10.1016/j.brainresbull.2015.01.009
Hammer, L. A., Waldner, H., Zagon, I. S., and McLaughlin, P. J. (2016). Opioid growth factor and low-dose naltrexone impair central nervous system infiltration by CD4+ T lymphocytes in established experimental autoimmune encephalomyelitis, a model of multiple sclerosis. Exp. Biol. Med. 241, 71ā78. doi: 10.1177/1535370215596384
Han, C., Lei, D., Liu, L., Xie, S., He, L., Wen, S., et al. (2020). Morphine induces the differentiation of T helper cells to Th2 effector cells via the PKC-Īø-GATA3 pathway. Int. Immunopharmacol. 80:106133. doi: 10.1016/j.intimp.2019.106133
Hauser, S. L., and Cree, B. A. C. (2020). Treatment of multiple sclerosis: a review. Am. J. Med. 133, 1380ā1390.e2. doi: 10.1016/j.amjmed.2020.05.049
Hegadoren, K. M., OāDonnell, T., Lanius, R., Coupland, N. J., and Lacaze-Masmonteil, N. (2009). The role of β-endorphin in the pathophysiology of major depression. Neuropeptides 43, 341ā353. doi: 10.1016/J.NPEP.2009.06.004
Heitmann, H., Andlauer, T. F. M., Korn, T., Mühlau, M., Henningsen, P., Hemmer, B., et al. (2020). Fatigue, depression, and pain in multiple sclerosis: how neuroinflammation translates into dysfunctional reward processing and anhedonic symptoms. Mult. Scler. J. doi: 10.1177/1352458520972279 [Online ahead of print].
Hemmick, L. M., and Bidlack, J. M. (1990). β-Endorphin stimulates rat T lymphocyte proliferation. J. Neuroimmunol. 29, 239ā248. doi: 10.1016/0165-5728(90)90167-l
Horvath, G. (2000). Endomorphin-1 and endomorphin-2: pharmacology of the selective endogenous μ-opioid receptor agonists. Pharmacol. Ther. 88, 437ā463. doi: 10.1016/S0163-7258(00)00100-105
Horvath, R. J., and Deleo, J. A. (2009). Morphine enhances microglial migration through modulation of P2X4 receptor signaling. J. Neurosci. 29, 998ā1005. doi: 10.1523/JNEUROSCI.4595-08.2009
Hough, C. J., Halperin, J. I., Mazorow, D. L., Yeandle, S. L., and Millar, D. B. (1990). β-Endorphin modulates T-cell intracellular calcium flux and c-myc expression via a potassium channel. J. Neuroimmunol. 27, 163ā171. doi: 10.1016/0165-5728(90)90066-V
Hua, H., Lu, C., Li, W., Meng, J., Wang, D., Plotnikoff, N. P., et al. (2012). Comparison of stimulating effect on subpopulations of lymphocytes in human peripheral blood by methionine enkephalin with IL-2 and IFN-γ. Hum. Vaccines Immunother. 8, 1082ā1089. doi: 10.4161/hv.20759
Hucklebridge, F. H., Hudspith, J., Muhamed, B. N., Lydyard, P. M., and Brostoff, J. (1989). Methionine-Enkephalin stimulates in vitro proliferation of human peripheral lymphocytes via 6-Opioid receptors. Brain Behav. Immun. 3, 183ā189. doi: 10.1016/0889-1591(89)90019-90016
Hutchinson, M. R., Lewis, S. S., Coats, B. D., Skyba, D. A., Crysdale, N. Y., Berkelhammer, D. L., et al. (2009). Reduction of opioid withdrawal and potentiation of acute opioid analgesia by systemic AV411 (ibudilast). Brain Behav. Immun. 23, 240ā250. doi: 10.1016/j.bbi.2008.09.012
Iadarola, M. J., Brady, L. S., Draisci, G., and Dubner, R. (1988). Enhancement of dynorphin gene expression in spinal cord following experimental inflammation: stimulus specificity, behavioral parameters and opioid receptor binding. Pain 35, 313ā326. doi: 10.1016/0304-3959(88)90141-90148
Jankovic, B. D., and Maric, D. (1987). Enkephalins and autoimmunity: differential effect of methionine-enkephalin on experimental allergic encephalomyelitis in wistar and lewis rats. J. Neurosci. Res. 18, 88ā94. doi: 10.1002/jnr.490180115
Jessop, D. S., Fassold, A., Wolff, C., Hofbauer, R., Chover-Gonzalez, A., Richards, L. J., et al. (2010). Endomorphins in rheumatoid arthritis, osteoarthritis, and experimental arthritis: annals of the New York Academy of Sciences. Ann. N. Y. Acad. Sci. 1193, 117ā122. doi: 10.1111/j.1749-6632.2009.05294.x
Jessop, D. S., Major, G. N., Coventry, T. L., Kaye, S. J., Fulford, A. J., Harbuz, M. S., et al. (2000). Novel opioid peptides endomorphin-1 and endomorphin-2 are present in mammalian immune tissues. J. Neuroimmunol. 106, 53ā59. doi: 10.1016/s0165-5728(99)00216-217
Jones, A. K. P., Cunningham, V. J., Ha-Kawa, S., Fujiwara, T., Luthra, S. K., Silva, S., et al. (1994). Changes in central opioid receptor binding in relation to inflammation and pain in patients with rheumatoid arthritis. Br. J. Rheumatol. 33, 33ā42. doi: 10.1093/rheumatology/33.10.909
Kalman, S., Ćsterberg, A. O., Sƶrensen, J., Boivie, J., and Bertler, A. (2002). Morphine responsiveness in a group of well-defined multiple sclerosis patients: a study with i.v. morphine. Eur. J. Pain 6, 69ā80. doi: 10.1053/eujp.2001.0307
Kamphuis, S., Eriksson, F., Kavelaars, A., Zijlstra, J., Van De Pol, M., Kuis, W., et al. (1998). Role of endogenous pro-enkephalin A-derived peptides in human T cell proliferation and monocyte IL-6 production. J. Neuroimmunol. 84, 53ā60. doi: 10.1016/s0165-5728(97)00240-243
Karussis, D. (2014). The diagnosis of multiple sclerosis and the various related demyelinating syndromes: a critical review. J. Autoimmun. 4, 134ā142. doi: 10.1016/j.jaut.2014.01.022
Kennedy, S. E., Koeppe, R. A., Young, E. A., and Zubieta, J. K. (2006). Dysregulation of endogenous opioid emotion regulation circuitry in major depression in women. Arch. Gen. Psychiatry 63, 1199ā1208. doi: 10.1001/archpsyc.63.11.1199
Khalil, Z., Sanderson, K., Modig, M., and Nyberg, F. (1999). Modulation of peripheral inflammation by locally administered endomorphin-1. Inflamm. Res. 48, 550ā556. doi: 10.1007/s000110050502
Khan, N., and Smith, M. T. (2014). Multiple sclerosis-induced neuropathic pain: pharmacological management and pathophysiological insights from rodent EAE models. Inflammopharmacology 22, 1ā22. doi: 10.1007/s10787-013-0195-193
Kipp, M., van der Star, B., Vogel, D. Y., Puentes, F., van der Valk, P., Baker, D., et al. (2012). Experimental in vivo and in vitro models of multiple sclerosis: EAE and beyond. Mult. Scler. Relat. Disord. 1, 15ā28. doi: 10.1016/j.msard.2011.09.002
Kissin, I. (2013). Long-term opioid treatment of chronic nonmalignant pain: unproven efficacy and neglected safety? J. Pain Res. 6, 513ā529. doi: 10.2147/JPR.S47182
Klega, A., Eberle, T., Buchholz, H. G., Maus, S., Maihƶfner, C., Schreckenberger, M., et al. (2010). Central opioidergic neurotransmission in complex regional pain syndrome. Neurology 75, 129ā136. doi: 10.1212/WNL.0b013e3181e7ca2e
Kowalski, J. (1998). Immunologic action of Met enkephalin fragments. Eur. J. Pharmacol. 347, 95ā99. doi: 10.1016/s0014-2999(98)00079-x
Kuhar, M. J., Pert, C. B., and Snyder, S. H. (1973). Regional distribution of opiate receptor binding in monkey and human brain. Nature 245, 447ā450. doi: 10.1038/245447a0
Kunkl, M., Frascolla, S., Amormino, C., Volpe, E., and Tuosto, L. (2020). T Helper cells: the modulators of inflammation in multiple sclerosis. Cells 9:482. doi: 10.3390/cells9020482
Labuz, D., Berger, S., Mousa, S. A., Zƶllner, C., Rittner, H. L., Shaqura, M. A., et al. (2006). Peripheral antinociceptive effects of exogenous and immune cell-derived endomorphins in prolonged inflammatory pain. J. Neurosci. 26, 4350ā4358. doi: 10.1523/JNEUROSCI.4349-05.2006
Labuz, D., Schmidt, Y., Schreiter, A., Rittner, H. L., Mousa, S. A., and Machelska, H. (2009). Immune cell-derived opioids protect against neuropathic pain in mice. J. Clin. Invest. 119, 278ā286. doi: 10.1172/JCI36246
Li, Z. H., Chu, N., Shan, L. D., Gong, S., Yin, Q. Z., and Jiang, X. H. (2009). Inducible expression of functional mu opioid receptors in murine dendritic cells. J. Neuroimmune Pharmacol. 4, 359ā367. doi: 10.1007/s11481-009-9145-9147
Liang, X., Liu, R., Chen, C., Ji, F., and Li, T. (2016). Opioid system modulates the immune function: a review. Transl. Perioper Pain Med. 1, 5ā13.
Lie, M. R. K. L., Van Der Giessen, J., Fuhler, G. M., De Lima, A., Peppelenbosch, M. P., Van Der Ent, C., et al. (2018). Low dose naltrexone for induction of remission in inflammatory bowel disease patients. J. Transl. Med. 16:55. doi: 10.1186/s12967-018-1427-1425
Likhtik, E., Popa, D., Apergis-Schoute, J., Fidacaro, G. A., and ParĆ©, D. (2008). Amygdala intercalated neurons are required for expression of fear extinction. Nature 454, 642ā645. doi: 10.1038/nature07167
Lin, M., Deng, K., Li, Y., and Wan, J. (2021). Morphine enhances LPS-induced macrophage apoptosis through a PPARγ-dependent mechanism. Exp. Ther. Med. 22, 1ā8. doi: 10.3892/etm.2021.10146
Link, H., and Huang, Y. M. (2006). Oligoclonal bands in multiple sclerosis cerebrospinal fluid: an update on methodology and clinical usefulness. J. Neuroimmunol. 180, 17ā28. doi: 10.1016/j.jneuroim.2006.07.006
Ludwig, M. D., Zagon, I. S., and McLaughlin, P. J. (2017). Serum [Met5]-enkephalin levels are reduced in multiple sclerosis and restored by low-dose naltrexone. Exp. Biol. Med. 242, 1524ā1533. doi: 10.1177/1535370217724791
Lueptow, L. M., Fakira, A. K., and Bobeck, E. N. (2018). The contribution of the descending pain modulatory pathway in opioid tolerance. Front. Neurosci. 12:886. doi: 10.3389/fnins.2018.00886
Luger, N. M., Sabino, M. A. C., Schwei, M. J., Mach, D. B., Pomonis, J. D., Keyser, C. P., et al. (2002). Efficacy of systemic morphine suggests a fundamental difference in the mechanisms that generate bone cancer vs. inflammatory pain. Pain 99, 397ā406. doi: 10.1016/S0304-3959(02)00102-101
Lynch, J. L., Alley, J. F., Wellman, L., and Beitz, A. J. (2008). Decreased spinal cord opioid receptor mRNA expression and antinociception in a Theilerās murine encephalomyelitis virus model of multiple sclerosis. Brain Res. 1191, 180ā191. doi: 10.1016/j.brainres.2007.11.034
Malendowicz, L. K., Rebuffat, P., Tortorella, C., Nussdorfer, G. G., Ziolkowska, A., and Hochol, A. (2005). Effects of met-enkephalin on cell proliferation in different models of adrenocortical-cell growth. Int. J. Mol. Med. 15, 841ā845. doi: 10.3892/ijmm.15.5.841
Mansour, A., Fox, C. A., Burke, S., Meng, F., Thompson, R. C., Akil, H., et al. (1994). Mu, delta, and kappa opioid receptor mRNA expression in the rat CNS: an in situ hybridization study. J. Comp. Neurol. 350, 412ā438. doi: 10.1002/cne.903500307
Mansour, A., Khachaturian, H., Lewis, M. E., Akil, H., and Watson, S. J. (1987). Autoradiographic differentiation of Mu. delta, and kappa opioid receptors in the rat forebrain and midbrain. J. Neurosci. 7, 2445ā2464.
Marchini, M., Manfredi, B., Tozzi, L., Sacerdote, P., Panerai, A., and Fedele, L. (1995). Mitogen-induced lymphocyte proliferation and peripheral blood mononuclear cell β-endorphin concentrations in primary dysmenorrhoea. Hum. Reprod. 10, 815ā817. doi: 10.1093/oxfordjournals.humrep.a136045
Marrie, R. A., Horwitz, R., Cutter, G., Tyry, T., Campagnolo, D., and Vollmer, T. (2009). The burden of mental comorbidity in multiple sclerosis: frequent, underdiagnosed, and undertreated. Mult. Scler. 15, 385ā392. doi: 10.1177/1352458508099477
Martin, T. J., Kim, S. A., Buechler, N. L., Porreca, F., and Eisenach, J. C. (2007). Opioid self-administration in the nerve-injured rat: relevance of antiallodynic effects to drug consumption and effects of intrathecal analgesics. Anesthesiology 106, 312ā322. doi: 10.1097/00000542-200702000-200702020
Martucci, C., Panerai, A. E., and Sacerdote, P. (2004). Chronic fentanyl or buprenorphine infusion in the mouse: similar analgesic profile but different effects on immune responses. Pain 110, 385ā392. doi: 10.1016/j.pain.2004.04.020
Mattioli, T.-A. M., Milne, B., and Cahill, C. M. (2010). Ultra-low dose naltrexone attenuates chronic morphine-induced gliosis in rats. Mol. Pain 6:22. doi: 10.1186/1744-8069-6-22
McDougall, J. J., Baker, C. L., and Hermann, P. M. (2004). Attenuation of knee joint inflammation by peripherally administered endomorphin-1. J. Mol. Neurosci. 22, 125ā138.
McLaughlin, P. J., McHugh, D. P., Magister, M. J., and Zagon, I. S. (2015). Endogenous opioid inhibition of proliferation of T and B cell subpopulations in response to immunization for experimental autoimmune encephalomyelitis. BMC Immunol. 16:24. doi: 10.1186/s12865-015-0093-0
Mei, F., Mayoral, S. R., Nobuta, H., Wang, F., Desponts, C., Lorrain, D. S., et al. (2016). Identification of the kappa-opioid receptor as a therapeutic target for oligodendrocyte remyelination. J. Neurosci. 36, 7925ā7935. doi: 10.1523/JNEUROSCI.1493-16.2016
Meunier, J. C. (1997). Nociceptin/orphanin FQ and the opioid receptor-like ORL1 receptor. Eur. J. Pharmacol. 340, 1ā15. doi: 10.1016/S0014-2999(97)01411-1418
Mifflin, K. A., and Kerr, B. J. (2017). Pain in autoimmune disorders. J. Neurosci. Res. 95, 1282ā1294. doi: 10.1002/jnr.23844
Minden, S. L., Feinstein, A., Kalb, R. C., Miller, D., Mohr, D. C., Patten, S. B., et al. (2014). Evidence-based guideline: assessment and management of psychiatric disorders in individuals with MS. Neurology 82, 174ā181. doi: 10.1212/WNL.0000000000000013
Minozzi, S., Amato, L., Vecchi, S., Davoli, M., Kirchmayer, U., and Verster, A. (2011). Oral naltrexone maintenance treatment for opioid dependence. Cochrane Database Syst. Rev. 2011:CD001333. doi: 10.1002/14651858.cd001333.pub4
Moser, T., Akgün, K., Proschmann, U., Sellner, J., and Ziemssen, T. (2020). The role of TH17 cells in multiple sclerosis: therapeutic implications. Autoimmun. Rev. 19:102647. doi: 10.1016/j.autrev.2020.102647
Mousa, S. A., Machelska, H., SchƤfer, M., and Stein, C. (2002). Immunohistochemical localization of endomorphin-1 and endomorphin-2 in immune cells and spinal cord in a model of inf lammatory pain. J. Neuroimmunol. 126, 5ā15. doi: 10.1016/s0165-5728(02)00049-48
Mousa, S. A., Shakibaei, M., Sitte, N., SchƤfer, M., and Stein, C. (2004). Subcellular pathways of β-Endorphin synthesis, processing, and release from immunocytes in inflammatory pain. Endocrinology 145, 1331ā1341. doi: 10.1210/en.2003-1287
Nath, A., Hauser, K. F., Wojna, V., Booze, R. M., Maragos, W., Prendergast, M., et al. (2002). Molecular basis for interactions of HIV and drugs of abuse. J. Acquir. Immune Defic. Syndr. 31, S62āS69. doi: 10.1097/00126334-200210012-200210016
Navolotskaya, E. V., Malkova, N. V., Zargarova, T. A., Lepikhova, T. N., Krasnova, S. B., and Lipkin, V. M. (2002). Effect of synthetic β-endorphin-like peptide immunorphin on human T lymphocytes. Biochemistry 67, 357ā363. doi: 10.1023/A:1014844718799
Niikura, K., Narita, M., Narita, M., Nakamura, A., Okutsu, D., Ozeki, A., et al. (2008). Direct evidence for the involvement of endogenous β-endorphin in the suppression of the morphine-induced rewarding effect under a neuropathic pain-like state. Neurosci. Lett. 435, 257ā262. doi: 10.1016/j.neulet.2008.02.059
Ninkovic, J., and Roy, S. (2013). Role of the mu-opioid receptor in opioid modulation of immune function. Amino Acids 45, 9ā24. doi: 10.1007/s00726-011-1163-1160
OāConnor, A. B., Schwid, S. R., Herrmann, D. N., Markman, J. D., and Dworkin, R. H. (2008). Pain associated with multiple sclerosis: systematic review and proposed classification. Pain 137, 96ā111. doi: 10.1016/j.pain.2007.08.024
Ohmori, H., Fujii, K., Sasahira, T., Luo, Y., Isobe, M., Tatsumoto, N., et al. (2009). Methionine-enkephalin secreted by human colorectal cancer cells suppresses T lymphocytes. Cancer Sci. 100, 497ā502. doi: 10.1111/j.1349-7006.2008.01073.x
Olechowski, C. J., Tenorio, G., Sauve, Y., and Kerr, B. J. (2013). Changes in nociceptive sensitivity and object recognition in experimental autoimmune encephalomyelitis (EAE). Exp. Neurol. 241, 113ā121. doi: 10.1016/j.expneurol.2012.12.012
Olechowski, C. J., Truong, J. J., and Kerr, B. J. (2009). Neuropathic pain behaviours in a chronic-relapsing model of experimental autoimmune encephalomyelitis (EAE). Pain 141, 156ā164. doi: 10.1016/j.pain.2008.11.002
Ćsterberg, A., Boivie, J., and Thuomas, K. -Ć . (2005). Central pain in multiple sclerosis - prevalence and clinical characteristics. Eur. J. Pain 9, 531ā531. doi: 10.1016/j.ejpain.2004.11.005
Ozaki, S., Narita, M., Narita, M., Iino, M., Miyoshi, K., and Suzuki, T. (2003). Suppression of the morphine-induced rewarding effect and G-protein activation in the lower midbrain following nerve injury in the mouse: involvement of G-protein-coupled receptor kinase 2. Neuroscience 116, 89ā97. doi: 10.1016/S0306-4522(02)00699-691
Ozaki, S., Narita, M., Narita, M., Iino, M., Sugita, J., Matsumura, Y., et al. (2002). Suppression of the morphine-induced rewarding effect in the rat with neuropathic pain: implication of the reduction in μ-opioid receptor functions in the ventral tegmental area. J. Neurochem. 82, 1192ā1198. doi: 10.1046/j.1471-4159.2002.01071.x
Panerai, A. E., Manfredi, B., Granucci, F., and Sacerdote, P. (1995). The β-endorphin inhibition of mitogen-induced splenocytes proliferation is mediated by central and peripheral paracrine/autocrine effects of the opioid. J. Neuroimmunol. 58, 71ā76. doi: 10.1016/0165-5728(94)00189-U
Panerai, A. E., Monastra, G., Manfredi, B., Sacerdote, P., Radulovic, J., and Locatelli, L. (1994). β-Endorphin concentrations in brain areas and peritoneal macrophages in rats susceptible and resistant to experimental allergic encephalomyelitis: a possible relationship between tumor necrosis factor a and opioids in the disease. J. Neuroimmunol. 51, 169ā176. doi: 10.1016/0165-5728(94)90078-90077
Pardini, M., Capello, E., Krueger, F., Mancardi, G., and Uccelli, A. (2013). Reward responsiveness and fatigue in multiple sclerosis. Mult. Scler. J. 19, 233ā240. doi: 10.1177/1352458512451509
Patel, C., Meadowcroft, M. D., Zagon, I. S., and McLaughlin, P. J. (2020). [Met 5]-enkephalin preserves diffusion metrics in EAE mice. Brain Res. Bull. 165, 246ā252. doi: 10.1016/j.brainresbull.2020.10.015
Patten, S. B., Marrie, R. A., and Carta, M. G. (2017). Depression in multiple sclerosis. Int. Rev. Psychiatry 29, 463ā472. doi: 10.1080/09540261.2017.1322555
PeciƱa, M., Karp, J. F., Mathew, S., Todtenkopf, M. S., Ehrich, E. W., and Zubieta, J. K. (2019). Endogenous opioid system dysregulation in depression: implications for new therapeutic approaches. Mol. Psychiatry 24, 576ā587. doi: 10.1038/s41380-018-0117-112
Peckys, D., and Landwehrmeyer, G. B. (1999). Expression of mu, kappa, and delta opioid receptor messenger RNA in the human CNS: a 33P in situ hybridization study. Neuroscience 88, 1093ā1135. doi: 10.1016/s0306-4522(98)00251-256
Peng, J., Sarkar, S., and Chang, S. L. (2012). Opioid receptor expression in human brain and peripheral tissues using absolute quantitative real-time RT-PCR. Drug Alcohol Depend 124, 223ā228. doi: 10.1016/j.drugalcdep.2012.01.013
Peng, Y., Yang, J., Guo, D., Zheng, C., Sun, H., Zhang, Q., et al. (2020). Sufentanil postoperative analgesia reduce the increase of T helper 17 (Th17) cells and FoxP3+regulatory T (Treg) cells in rat hepatocellular carcinoma surgical model: a randomised animal study. BMC Anesthesiol. 20:212. doi: 10.1186/s12871-020-01129-1120
Peruga, I., Hartwig, S., Thƶne, J., Hovemann, B., Gold, R., Juckel, G., et al. (2011). Inflammation modulates anxiety in an animal model of multiple sclerosis. Behav. Brain Res. 220, 20ā29. doi: 10.1016/j.bbr.2011.01.018
Petraschka, M., Li, S., Gilbert, T. L., Westenbroek, R. E., Bruchas, M. R., Schreiber, S., et al. (2007). The absence of endogenous β-endorphin selectively blocks phosphorylation and desensitization of mu opioid receptors following partial sciatic nerve ligation. Neuroscience 146, 1795ā1807. doi: 10.1016/j.neuroscience.2007.03.029
Piva, M., Moreno, J. I., Montgomery, C. O., and Wilson, C. (2005). In vitro modulation of cytokine expression by enkephalin-derived peptides. Neuroimmunomodulation 12, 339ā347. doi: 10.1159/000091127
Plein, L. M., and Rittner, H. L. (2018). Opioids and the immune system-friend or foe. Br. J. Pharmacol. 175, 2717ā2725. doi: 10.1111/bph.13750
Pollak, Y., Orion, E., Goshen, I., Ovadia, H., and Yirmiya, R. (2002). Experimental autoimmune encephalomyelitis-associated behavioral syndrome as a model of ādepression due to multiple sclerosisā. Brain Behav. Immun. 16, 533ā543. doi: 10.1016/S0889-1591(02)00010-17
Polman, C. H., Reingold, S. C., Banwell, B., Clanet, M., Cohen, J. A., Filippi, M., et al. (2011). Diagnostic criteria for multiple sclerosis: 2010 revisions to the McDonald criteria. Ann. Neurol. 69, 292ā302. doi: 10.1002/ana.22366
Pomorska, D. K., Gach, K., and Janecka, A. (2014). Immunomodulatory effects of endogenous and synthetic peptides activating opioid receptors. Med. Chem. (Los Angeles) 14, 1148ā1155. doi: 10.2174/1389557515666150101095237
Poole, D. P., Pelayo, J., Scherrer, G., Evans, C. J., Kieffer, B. L., and Bunnett, N. W. (2011). Localization and regulation of fluorescently labeled delta opioid receptor, expressed in enteric neurons of mice. Gastroenterology 141, 982ā991.e18. doi: 10.1053/j.gastro.2011.05.042
Potter, L. E., Paylor, J. W., Suh, J. S., Tenorio, G., Caliaperumal, J., Colbourne, F., et al. (2016). Altered excitatory-inhibitory balance within somatosensory cortex is associated with enhanced plasticity and pain sensitivity in a mouse model of multiple sclerosis. J. Neuroinflammation 13, 1ā20. doi: 10.1186/s12974-016-0609-604
PrzewÅocka, B., Mika, J., Åabuz, D., Toth, G., and PrzewÅocki, R. (1999). Spinal analgesic action of endomorphins in acute, inflammatory and neuropathic pain in rats. Eur. J. Pharmacol. 367, 189ā196. doi: 10.1016/S0014-2999(98)00956-X
Przewlocki, R., Hassan, A. H. S., Lason, W., Epplen, C., Herz, A., and Stein, C. (1992). Gene expression and localization of opioid peptides in immune cells of inflamed tissue: functional role in antinociception. Neuroscience 48, 491ā500.
Quaglio, G., Talamini, G., Lechi, A., Mezzelani, P., Lugoboni, F., and Mezzelani, P. (2002). Prevalence of tuberculosis infection and comparison of multiple-puncture liquid tuberculin test and mantoux test among drug users. Scand. J. Infect. Dis. 34, 574ā576. doi: 10.1080/00365540110080791
Raghavendra, V., Rutkowski, M. D., and Deleo, J. A. (2002). The role of spinal neuroimmune activation in morphine tolerance/hyperalgesia in neuropathic pain and sham-operated rats. J. Neurosci. 22, 9980ā9989. doi: 10.1523/jneurosci.22-22-09980.2002
Raghavendra, V., Tanga, F., and DeLeo, J. A. (2003). Inhibition of microglial activation attenuates the development but not existing hypersensitivity in a rat model of neuropathy. J. Pharmacol. Exp. Ther. 306, 624ā630. doi: 10.1124/jpet.103.052407
Rahn, K. A., Mclaughlin, P. J., and Zagon, I. S. (2011). Prevention and diminished expression of experimental autoimmune encephalomyelitis by low dose naltrexone (LDN) or opioid growth factor (OGF) for an extended period: therapeutic implications for multiple sclerosis. Brain Res. 1381, 243ā253. doi: 10.1016/j.brainres.2011.01.036
RiĆ, G. L., Chang, D. I., Wevers, C., Westendorf, A. M., Buer, J., Scherbaum, N., et al. (2012). Opioid maintenance therapy restores CD4+ T cell function by normalizing CD4+CD25high regulatory T cell frequencies in heroin user. Brain. Behav. Immun. 26, 972ā978. doi: 10.1016/j.bbi.2012.05.008
Robinson, A. P., Harp, C. T., Noronha, A., and Miller, S. D. (2014). The experimental autoimmune encephalomyelitis (EAE) model of MS. utility for understanding disease pathophysiology and treatment. Handb. Clin. Neurol. 122, 173ā189. doi: 10.1016/B978-0-444-52001-2.00008-X
Rojas-Corrales, M. O., Berrocoso, E., Gibert-Rahola, J., and Micó, J. A. (2004). Antidepressant-like effect of tramadol and its enantiomers in reserpinized mice: comparative study with fesipramine, fluvoxamine, venlafaxine and opiates. J. Psychopharmacol. 18, 404ā411. doi: 10.1177/026988110401800305
Rojas-Corrales, M. O., Gibert-Rahola, J., and Micó, J. A. (1998). Tramadol induces antidepressant-type effects in mice. Life Sci. 63, L175āL180. doi: 10.1016/S0024-3205(98)00369-365
Rojavin, M., Szabo, I., Bussiere, J. L., Rogers, T. J., Adler, M. W., and Eisenstein, T. K. (1993). Morphine treatment in vitro or in vivo decreases phagocytic functions of murine macrophages. Life Sci. 53, 997ā1006. doi: 10.1016/0024-3205(93)90122-J
Rowbotham, M. C., Twilling, L., Davies, P. S., Reisner, L., Taylor, K., and Mohr, D. (2003). Oral opioid therapy for chronic peripheral and central neuropathic pain. N. Engl. J. Med. 348, 1223ā1232. doi: 10.1056/nejmoa021420
Roy, S., Barke, R. A., and Loh, H. H. (1998). MU-opioid receptor-knockout mice: role of μ-opioid receptor in morphine mediated immune functions. Mol. Brain Res. 61, 190ā194. doi: 10.1016/s0169-328x(98)00212-215
Roy, S., Ninkovic, J., Banerjee, S., Charboneau, R. G., Das, S., Dutta, R., et al. (2011). Opioid drug abuse and modulation of immune function: consequences in the susceptibility to opportunistic infections. J. Neuroimmune Pharmacol. 6, 442ā465. doi: 10.1007/s11481-011-9292-9295
Roy, S., Wang, J., Gupta, S., Charboneau, R., Loh, H. H., and Barke, R. A. (2004). Chronic morphine treatment differentiates T helper cells to Th2 effector cells by modulating transcription factors GATA 3 and T-bet. J. Neuroimmunol. 147, 78ā81. doi: 10.1016/j.jneuroim.2003.10.016
Sacerdote, P., Bianchi, M., Gaspani, L., Manfredi, B., Maucione, A., Terno, G., et al. (2000). The effects of tramadol and morphine on immune responses and pain after surgery in cancer patients. Anesth. Analg. 90, 1411ā1414. doi: 10.1097/00000539-200006000-200006028
Sacerdote, P., Franchi, S., Gerra, G., Leccese, V., Panerai, A. E., and Somaini, L. (2008). Buprenorphine and methadone maintenance treatment of heroin addicts preserves immune function. Brain Behav. Immun. 22, 606ā613. doi: 10.1016/j.bbi.2007.12.013
Sacerdote, P., Manfredi, B., Mantegazza, P., and Panerai, A. E. (1997). Antinociceptive and immunosuppressive effects of opiate drugs: a structure-related activity study. Br. J. Pharmacol. 121, 834ā840. doi: 10.1038/sj.bjp.0701138
Salzet, M., Vieau, D., and Day, R. (2000). Crosstalk between nervous and immune systems through the animal kingdom: focus on opioids. Trends Neurosci. 23, 550ā555. doi: 10.1016/s0166-2236(00)01642-1648
Seixas, D., Palace, J., and Tracey, I. (2016). Chronic pain disrupts the reward circuitry in multiple sclerosis. Eur. J. Neurosci. 44, 1928ā1934. doi: 10.1111/ejn.13272
Shapira, N. A., and DeGraw, J. D. (2001). Treatment of refractory major depression with tramadol monotherapy. J. Clin. Psychiatry 62, 205ā206. doi: 10.4088/jcp.v62n0312b
Sharafaddinzadeh, N., Moghtaderi, A., Kashipazha, D., Majdinasab, N., and Shalbafan, B. (2010). The effect of low-dose naltrexone on quality of life of patients with multiple sclerosis: a randomized placebo-controlled trial. Mult. Scler. 16, 964ā969. doi: 10.1177/1352458510366857
Sharp, B. M., Shahabi, N., McKean, D., Li, M. D., and McAllen, K. (1997). Detection of basal levels and induction of delta opioid receptor mRNA in murine splenocytes. J. Neuroimmunol. 78, 198ā202. doi: 10.1016/S0165-5728(97)00101-X
Shavit, Y., Depaulis, A., Martint, F. C., Terman, G. W., Pechnick, R. N., Zane, C. J., et al. (1986a). Involvement of brain opiate receptors in the immune-suppressive effect of morphine. Proc. Natl. Acad. Sci. U. S. A. 83, 7114ā7117. doi: 10.1073/pnas.83.18.7114
Shavit, Y., Terman, G. W., Lewis, J. W., Zane, C. J., Gale, R. P., and Liebeskind, J. C. (1986b). Effects of footshock stress and morphine on natural killer lymphocytes in rats: studies of tolerance and cross-tolerance. Brain Res. 372, 382ā385. doi: 10.1016/0006-8993(86)91149-91142
Simon, E. J. (1991). Opioid receptors and endogenous opioid peptides. Med. Res. Rev. 11, 357ā374. doi: 10.1002/med.2610110402
Stein, C., Hassan, A. H. S., Przewlocki, R., Gramsch, C., Peter, K., and Herz, A. (1990). Opioids from immunocytes interact with receptors on sensory nerves to inhibit nociception in inflammation. Proc. Natl. Acad. Sci. U. S. A. 87, 5935ā5939. doi: 10.1073/pnas.87.15.5935
Stoll, A. L., and Rueter, S. (1999). Treatment augmentation with opiates in severe and refractory major depression. Am. J. Psychiatry 156:2017. doi: 10.1176/AJP.156.12.2017
Szabo, I., Rojavin, M., Bussiere, J. L., Eisenstein, T. K., Adler, M. W., and Rogers, T. J. (1993). Suppression of peritoneal macrophage phagocytosis of candida albicans by opioids. J. Pharmacol. Exp. Ther. 267, 703ā706.
Tangherlini, G., Kalinin, D. V., Schepmann, D., Che, T., Mykicki, N., StƤnder, S., et al. (2019). Development of novel quinoxaline-based Īŗ-opioid receptor agonists for the treatment of neuroinflammation. J. Med. Chem. 62, 893ā907. doi: 10.1021/acs.jmedchem.8b01609
Tawfik, V. L., LaCroix-Fralish, M. L., Nutile-McMenemy, N., and DeLeo, J. A. (2005). Transcriptional and translational regulation of glial activation by morphine in a rodent model of neuropathic pain. J. Pharmacol. Exp. Ther. 313, 1239ā1247. doi: 10.1124/jpet.104.082420
Tomassini, N., Renaud, F., Roy, S., and Loh, H. H. (2004). Morphine inhibits Fc-mediated phagocytosis through mu and delta opioid receptors. J. Neuroimmunol. 147, 131ā133. doi: 10.1016/j.jneuroim.2003.10.028
Tortorici, V., Morgan, M. M., and Vanegas, H. (2001). Tolerance to repeated microinjection of morphine into the periaqueductal gray is associated with changes in the behavior of off- and on-cells in the rostral ventromedial medulla of rats. Pain 89, 237ā244. doi: 10.1016/S0304-3959(00)00367-365
Tubaro, E., Avico, U., Santiangeli, C., Zuccaro, P., Cavallo, G., Pacifici, R., et al. (1985). Morphine and methadone impact on human phagocytic physiology. Int. J. Immunopharmacol. 7, 865ā874. doi: 10.1016/0192-0561(85)90049-90049
Turner, A. P., Williams, R. M., Bowen, J. D., Kivlahan, D. R., and Haselkorn, J. K. (2006). Suicidal ideation in multiple sclerosis. Arch. Phys. Med. Rehabil. 87, 1073ā1078. doi: 10.1016/j.apmr.2006.04.021
Vallejo, R., de Leon-Casasola, O., and Benyamin, R. (2004). Opioid therapy and immunosuppression. Am. J. Ther. 11, 354ā365. doi: 10.1097/01.mjt.0000132250.95650.85
Van Den Bergh, P., Rozing, J., and Nagelkerken, L. (1991). Two opposing modes of action of beta-endorphin on lymphocyte function. Immunology 72, 537ā543.
Van Den Bergh, P., Rozing, J., and Nagelkerken, L. (1993). Identification of two moieties of β-endorphin with opposing effects on rat T-cell proliferation. Immunology 79, 18ā23.
van Langelaar, J., van der Vuurst, de Vries, R. M., Janssen, M., Wierenga-Wolf, A. F., Spilt, I. M., et al. (2018). T helper 17.1 cells associate with multiple sclerosis disease activity: perspectives for early intervention. Brain 141, 1334ā1349. doi: 10.1093/brain/awy069
Vanāt Veer, A., and Carlezon, W. A. (2013). Role of kappa-opioid receptors in stress and anxiety-related behavior. Psychopharmacology (Berl) 229, 435ā452. doi: 10.1007/s00213-013-3195-3195
Vargas, D. L., and Tyor, W. R. (2017). Update on disease-modifying therapies for multiple sclerosis. J. Investig. Med. 65, 883ā891. doi: 10.1136/jim-2016-000339
Vigano, D., Rubino, T., Di Chiara, G., Ascari, I., Massi, P., and Parolaro, D. (2003). Mu opioid receptor signaling in morphine sensitization. Neuroscience 117, 921ā929. doi: 10.1016/S0306-4522(02)00825-824
Villarroel, M. A., and Terlizzi, E. P. (2020). Symptoms of Depression Among Adults: United States, 2019. Hyattsville, MD: National Center for Health Statistics. NCHS Data Brief, no 379.
Waite, J. C., and Skokos, D. (2012). Th17 response and inflammatory autoimmune diseases. Int. J. Inflam. 2012:819467. doi: 10.1155/2012/819467
Wang, D., Tawfik, V. L., Corder, G., Low, S. A., FranƧois, A., Basbaum, A. I., et al. (2018a). Functional divergence of delta and Mu opioid receptor organization in CNS pain circuits. Neuron 98, 90ā108. doi: 10.1016/j.neuron.2018.03.002
Wang, F., and Mei, F. (2019). Kappa opioid receptor and oligodendrocyte remyelination. Vitam. Horm. 111, 281ā297. doi: 10.1016/bs.vh.2019.05.004
Wang, F., Yang, Y. J., Yang, N., Chen, X. J., Huang, N. X., Zhang, J., et al. (2018b). Enhancing oligodendrocyte myelination rescues synaptic loss and improves functional recovery after chronic hypoxia. Neuron 99, 689ā701. doi: 10.1016/j.neuron.2018.07.017
Wang, H., and Wessendorf, M. W. (2002). Mu- and delta-opioid receptor mRNAs are expressed in periaqueductal gray neurons projecting to the rostral ventromedial medulla. Neuroscience 109, 619ā634. doi: 10.1016/S0306-4522(01)00328-321
Watkins, L. R., Hutchinson, M. R., Rice, K. C., and Maier, S. F. (2009). The āTollā of opioid-induced glial activation: improving the clinical efficacy of opioids by targeting glia. Trends Pharmacol. Sci. 30, 581ā591. doi: 10.1016/j.tips.2009.08.002
Weber, R. J., and Pert, A. (1989). The periaqueductal gray matter mediates opiate-induced immunosuppression. Science 245, 188ā190. doi: 10.1126/science.2749256
Weinstock, L. B., Myers, T. L., Walters, A. S., Schwartz, O. A., Younger, J. W., Chopra, P. J., et al. (2016). Identification and treatment of new inflammatory triggers for complex regional pain syndrome: small intestinal bacterial overgrowth and obstructive sleep apnea. A A Case Reports 6, 272ā276. doi: 10.1213/XAA.0000000000000292
Wick, M. J., Minnerath, S. R., Roy, S., Ramakrishnan, S., and Loh, H. H. (1996). Differential expression of opioid receptor genes in human lymphoid cell lines and peripheral blood lymphocytes. J. Neuroimmunol. 64, 29ā36. doi: 10.1016/0165-5728(95)00144-141
Wiese, A. D., Griffin, M. R., Schaffner, W., Michael Stein, C., Greevy, R. A., and Mitchel, E. F. Jr., et al. (2018). Opioid analgesic use and risk for invasive pneumococcal diseases a nested case-control study. Ann. Intern. Med. 168, 396ā404. doi: 10.7326/M17-1907
Willoch, F., Schindler, F., Wester, H. J., Empl, M., Straube, A., Schwaiger, M., et al. (2004). Central poststroke pain and reduced opioid receptor binding within pain processing circuitries: a [11C]diprenorphine PET study. Pain 108, 213ā220. doi: 10.1016/j.pain.2003.08.014
Wood, J. D., and Galligan, J. J. (2004). Function of opioids in the enteric nervous system. Neurogastroenterol. Motil. 16, 17ā28. doi: 10.1111/j.1743-3150.2004.00554.x
Wybran, J., Appelboom, T., Famaey, J.-P., and Govaerts, A. (1979). Suggestive evidence for receptors for morphine and methionine-enkephalin on normal human blood T lymphocytes. J. Immunol. 123, 1068ā1070.
Xu, N., Wang, Y., Zhao, S., Jiao, T., Xue, H., Shan, F., et al. (2020). Naltrexone (NTX) relieves inflammation in the collagen-induced- arthritis (CIA) rat models through regulating TLR4/NFĪŗB signaling pathway. Int. Immunopharmacol. 79:106056. doi: 10.1016/j.intimp.2019.106056
Yasuda, K., Takeuchi, Y., and Hirota, K. (2019). The pathogenicity of Th17 cells in autoimmune diseases. Seminars 41, 283ā297. doi: 10.1007/s00281-019-00733-738
Ye, S., Applegren, R. R., Davis, J. M., and Tak Cheung, H. (1989). Modulation of lymphocyte motility by β-endorphin and met-enkephalin. Immunopharmacology 17, 81ā89. doi: 10.1016/0162-3109(89)90053-90052
Yeager, M. P., Colacchio, T. A., Yu, C. T., Hildebrandt, L., Howell, A. L., Weiss, J., et al. (1995). Morphine inhibits spontaneous and cytokine-enhanced natural killer cell cytotoxicity in volunteers. Anesthesiology 83, 500ā508. doi: 10.1097/00000542-199509000-199509008
Younger, J., and Mackey, S. (2009). Fibromyalgia symptoms are reduced by low-dose naltrexone: a pilot study. Pain Med. 10, 663ā672. doi: 10.1111/j.1526-4637.2009.00613.x
Younger, J., Noor, N., McCue, R., and MacKey, S. (2013). Low-dose naltrexone for the treatment of fibromyalgia: findings of a small, randomized, double-blind, placebo-controlled, counterbalanced, crossover trial assessing daily pain levels. Arthritis Rheum. 65, 529ā538. doi: 10.1002/art.37734
Younger, J., Parkitny, L., and McLain, D. (2014). The use of low-dose naltrexone (LDN) as a novel anti-inflammatory treatment for chronic pain. Clin. Rheumatol. 33, 451ā459. doi: 10.1007/s10067-014-2517-2512
Zadina, J. E., Hackler, L., Ge, L.-J., and Kastln, A. J. (1997). A potent and selective endogenous agonist for the μ-opiate receptor. Nature 386, 499ā502. doi: 10.1038/386499a0
Zagon, I. S., Donahue, R. N., Bonneau, R. H., and McLaughlin, P. J. (2011). T lymphocyte proliferation is suppressed by the opioid growth factor ([Met5]-enkephalin)āopioid growth factor receptor axis: implication for the treatment of autoimmune diseases. Immunobiology 216, 579ā590. doi: 10.1016/j.imbio.2010.09.014
Zagon, I. S., and McLaughlin, P. J. (1991). Identification of opioid peptides regulating proliferation of neurons and glia in the developing nervous system. Brain Res. 542, 318ā323. doi: 10.1016/0006-8993(91)91585-O
Zagon, I. S., Rahn, K. A., Bonneau, R. H., Turel, A. P., and McLaughlin, P. J. (2010). Opioid growth factor suppresses expression of experimental autoimmune encephalomyelitis. Brain Res. 1310, 154ā161. doi: 10.1016/j.brainres.2009.11.026
Zagon, I. S., Rahn, K. A., Turel, A. P., and McLaughlin, P. J. (2009). Endogenous opioids regulate expression of experimental autoimmune encephalomyelitis: a new paradigm for the treatment of multiple sclerosis. Exp. Biol. Med. 234, 1383ā1392. doi: 10.3181/0906-RM-189
Zagon, I. S., Verderame, M. F., and McLaughlin, P. J. (2002). The biology of the opioid growth factor receptor (OGFr). Brain Res. Rev. 38, 351ā376. doi: 10.1016/S0165-0173(01)00160-166
Zhang, T., Zhang, N., Zhang, R., Zhao, W., Chen, Y., Wang, Z., et al. (2018). Preemptive intrathecal administration of endomorphins relieves inflammatory pain in male mice via inhibition of p38 MAPK signaling and regulation of inflammatory cytokines. J. Neuroinflammation 15:320. doi: 10.1186/s12974-018-1358-1353
Keywords: multiple sclerosis, opioid, inflammation, pain, affect, mood, immune system
Citation: Dworsky-Fried Z, Chadwick CI, Kerr BJ and Taylor AMW (2021) Multiple Sclerosis and the Endogenous Opioid System. Front. Neurosci. 15:741503. doi: 10.3389/fnins.2021.741503
Received: 14 July 2021; Accepted: 26 August 2021;
Published: 17 September 2021.
Edited by:
John R. Bethea, Drexel University, United StatesReviewed by:
Cinzia Severini, National Research Council (CNR), ItalyCopyright Ā© 2021 Dworsky-Fried, Chadwick, Kerr and Taylor. This is an open-access article distributed under the terms of the Creative Commons Attribution License (CC BY). The use, distribution or reproduction in other forums is permitted, provided the original author(s) and the copyright owner(s) are credited and that the original publication in this journal is cited, in accordance with accepted academic practice. No use, distribution or reproduction is permitted which does not comply with these terms.
*Correspondence: Anna M. W. Taylor, ataylor1@ualberta.ca
Disclaimer: All claims expressed in this article are solely those of the authors and do not necessarily represent those of their affiliated organizations, or those of the publisher, the editors and the reviewers. Any product that may be evaluated in this article or claim that may be made by its manufacturer is not guaranteed or endorsed by the publisher.
Research integrity at Frontiers
Learn more about the work of our research integrity team to safeguard the quality of each article we publish.