- 1Department of Health Sciences and Technology, Samsung Advanced Institute for Health Sciences and Technology, Sungkyunkwan University, Seoul, South Korea
- 2Samsung Medical Center, Samsung Biomedical Research Institute, Seoul, South Korea
Many neurodegenerative diseases have been associated with defects in primary cilia, which are cellular organelles involved in diverse cellular processes and homeostasis. Several types of glial cells in both the central and peripheral nervous systems not only support the development and function of neurons but also play significant roles in the mechanisms of neurological disease. Nevertheless, most studies have focused on investigating the role of primary cilia in neurons. Accordingly, the interest of recent studies has expanded to elucidate the role of primary cilia in glial cells. Correspondingly, several reports have added to the growing evidence that most glial cells have primary cilia and that impairment of cilia leads to neurodegenerative diseases. In this review, we aimed to understand the regulatory mechanisms of cilia formation and the disease-related functions of cilia, which are common or specific to each glial cell. Moreover, we have paid close attention to the signal transduction and pathological mechanisms mediated by glia cilia in representative neurodegenerative diseases. Finally, we expect that this field of research will clarify the mechanisms involved in the formation and function of glial cilia to provide novel insights and ideas for the treatment of neurodegenerative diseases in the future.
Introduction
Since the identification of primary cilium, a cellular organelle composed of microtubules, in a variety of cells, including rabbit kidney tubular epithelial cells, human pancreatic ductal epithelial cells, seminal vesicle epithelial cells, uterine fundus epithelial cells, and thyroid gland epithelial cells, about a century ago (Bloodgood, 2009), extensive research continues to elucidate its functional significance. Based on previous findings, primary cilia are believed to serve as major hubs of various signaling pathways such as Wnt, Shh, Notch, PDGF, and mTOR in most cells (Wheway et al., 2018). Given that these signal transductions are involved in pivotal developmental processes, including cell proliferation, migration, and differentiation (Wong et al., 2001; Basson, 2012; Duronio and Xiong, 2013), impairment of primary cilia is closely associated with many diseases (Wheway et al., 2018). In particular, several neurodevelopmental diseases, such as Joubert syndrome, Bardet–Biedl syndrome, and Meckel–Gruber syndrome are representative ciliopathies (Rooryck et al., 2007; Giordano et al., 2009; Hildebrandt et al., 2009).
The tight link between the primary cilia and nervous system-related diseases has prompted researchers to investigate the role of cilia in neurons. It is noteworthy that several previous reports have suggested that primary cilia of neurons are important for Shh signaling-mediated cell proliferation in postnatal development of the hippocampus and the cerebellum (Breunig et al., 2008; Han et al., 2008; Spassky et al., 2008). However, most studies have been limited to adult neuronal cilia in the central nervous system (CNS) (Kirschen and Xiong, 2017; Park S.M. et al., 2019). Indeed, it has long been observed that primary cilia are present not only in mature neurons but also in several kinds of cells, including neural crest cells, neural progenitor cells, and glial cells (Etchevers et al., 2019; Park S.M. et al., 2019; Yusifov et al., 2021). Moreover, primary cilia are organelles that undergo assembly and disassembly depending on the type of cell or developmental processes. Therefore, intensive studies on non-neuronal cilia have been emphasized to comprehensively understand the function of primary cilia in the nervous system.
Glial cells are the main components of the nervous system; they are roughly equal to the number of neurons and play important functional and structural roles in supporting neurons during development and regeneration. There are several types of glial cells both in the CNS and the peripheral nervous system (PNS): astrocytes, oligodendrocytes (OLGs), and microglial cells in the CNS and Schwann cells, satellite glial cells, enteric glial cells, and olfactory ensheathing cells in the PNS (Compston et al., 1997; Jessen and Mirsky, 2005; Domingues et al., 2016). Indeed, the presence of primary cilia has already been observed in astrocytes and OLGs of the CNS and in Schwann cells of the PNS, although studies on the role of glia cilia are in elementary stages (Bishop et al., 2007; Kasahara et al., 2014; Falcon-Urrutia et al., 2015).
Several neuropathies with defective axonal regeneration result from glial cell dysfunction (Lehmann and Hoke, 2010; Gritsch et al., 2014; Li et al., 2019), and glial impairment due to nerve injury leads to inflammatory neuropathy, which affects myelination (Willison and Yuki, 2002). Many studies have attempted to treat demyelination-associated diseases in a variety of ways, including immune suppression using steroids (Rahmlow and Kantarci, 2013; Klein et al., 2019; Gwathmey and Grogan, 2020); however, there have been no targeted therapies developed till date. Recent studies have reported that OLGs progenitor cells (OPCs) derived from neural stem cells are effective in regenerating damaged axons through myelination activation (Murphy and Franklin, 2017) and that astrocytes derived from embryonic stem cells are effective in protecting neurons from neurotoxicity and death (Barbeito, 2018). This suggests that therapies focusing on the function of glial cells could be an alternative treatment for neuropathy. Accordingly, in this review, we will address some recent findings regarding the importance of targeting glial cells, especially primary cilia of glia, for the treatment of developmental and degenerative diseases of the nervous system (Table 1).
Primary Cilium in Astrocytes: A Key Modulator of Neuronal Signaling in the Central Nervous System
Astrocytes, the most abundant glial cells in the CNS, are important for the formation and maintenance of the blood-brain barrier to secrete a variety of neurotrophic factors during synaptogenesis, neuronal differentiation, and neuronal survival (Carson et al., 2006; Fakhoury, 2018). In neurodegenerative diseases, astrocytes elevate the production of cytotoxic molecules, including reactive oxygen species (ROS), and inflammatory mediators, including COX2, IL-1β, TNF-α, and IL-6 (Akiyama et al., 2000; McGeer and McGeer, 2002). Moreover, in inflammatory conditions, astrocytes affect surrounding cells in response to pro-inflammatory cytokines/chemokines and nitric oxide secreted by immune cells present in the CNS (Rothhammer and Quintana, 2015; Sofroniew, 2015). For example, the pro-inflammatory factors, whose production is increased by astrocytes, induce apoptosis of neighboring neurons (Simi et al., 2007; McCoy and Tansey, 2008), and this leads to several CNS neurodegenerative diseases, such as Alzheimer’s disease (AD), Parkinson’s disease (PD), and Huntington’s disease (HD) (Habib et al., 2020).
Observations of primary cilia in astrocytes (Bishop et al., 2007; Danilov et al., 2009; Yoshimura et al., 2011; Kasahara et al., 2014) and the identification of Shh signaling molecules such as smoothened (SMO) and patched 1 receptor (PTCH1) in the cilia of astrocytes (Yoshimura et al., 2011) have led to an understanding of the role of astrocyte cilia in CNS development and diseases (Figure 1). Several previous studies have suggested that astrocyte cilia may play an important role in Shh signaling to control cellular biological processes in inflammatory conditions (Amankulor et al., 2009; Jin et al., 2015; Allahyari et al., 2019); however, only little direct evidence related to this finding has been reported. In order to explain recent insights related to the role of astrocyte cilia in inflammatory CNS diseases, we cover several experimental results that suggest a direct involvement of astrocyte cilia in some representative neurodegenerative diseases in this review.
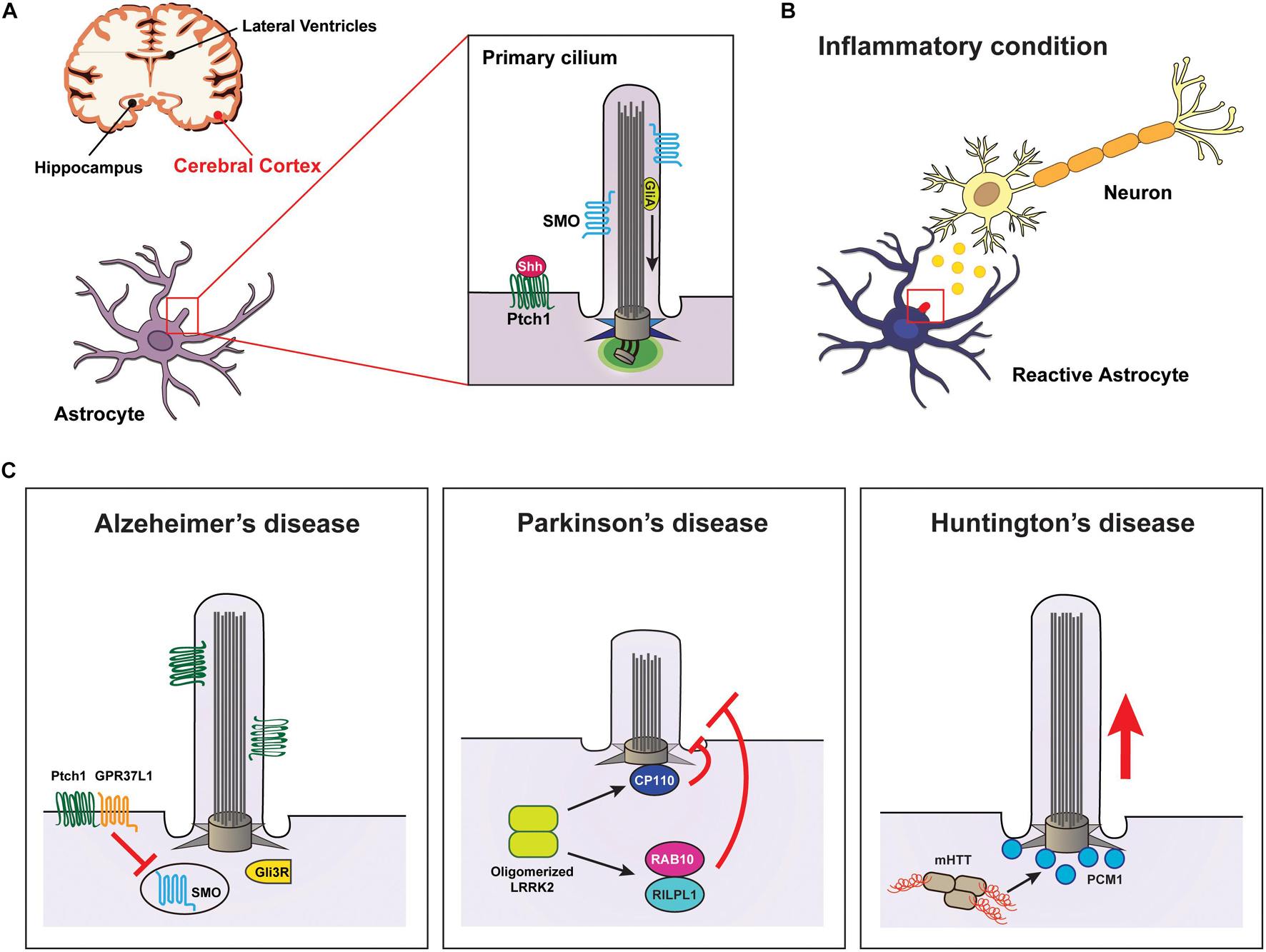
Figure 1. Signaling through the primary cilia in astrocytes is associated with neurodegenerative diseases. (A) Diagram showing the primary cilia of astrocytes associated with diseases occurring in the cerebral cortex. (B) Neuroinflammation affects Shh signal transduction through the primary cilia in astrocytes. (C) Examples of neurodegenerative diseases involved in impairments of primary cilia in astrocytes. Elevated Aβ attenuates Shh signaling in astrocyte cilia, leading to Alzheimer’s disease. Defects in LRRK2 cause Parkinson’s disease by inhibiting astrocyte cilia formation by preventing the removal of CP110 from the ciliary base or by inducing a complex of RILPL1 and RAB10. Huntington’s disease results from mutated HTT-induced PCM1 accumulation at the ciliary base of astrocytes.
Alzheimer’s Disease
Alzheimer’s disease is clinically characterized by impaired memory and cognitive function due to a gradual loss of neurons. The pathological hallmark of AD is the extracellular plaque deposition of insoluble amyloid-beta (Aβ) peptide, a flame-shaped neurofibrillary tangle (NFT) of the microtubule-binding protein TAU, which induces neuroinflammation in the brain (Fakhoury, 2018). Recent findings have suggested that astrocytes play a role in promoting neurodegenerative processes in AD by increasing neurotoxicity via secretion of inflammatory cytokines under activation of Aβ (De Strooper and Karran, 2016; Liao et al., 2016; Keren-Shaul et al., 2017). Notably, increased Aβ deposition affects the assembly and length control of primary cilia in astrocytes, thereby inhibiting the activation of the Shh signaling pathway and eventually disrupting neuronal survival (Vorobyeva and Saunders, 2018).
The G protein-coupled receptor 37-like 1 (GPR37L1) is expressed in cerebellar Bergmann glia (BG) astrocytes specifically, and it is involved in the proliferation and differentiation of cerebellar granule neurons and the maturation of Purkinje neurons (Valdenaire et al., 1998; Meyer et al., 2013). Previous studies have reported that murine Gpr37l1 physically interacts with Ptch1 in the periciliary membrane of BG cells (Marazziti et al., 2013). Moreover, a recent study found that GPR37L1 binds to PTCH1 to regulate Shh signal transduction by inducing the ciliary translocation of SMO in cerebellar astrocytes (La Sala et al., 2020; Figure 1C and Table 1).
In mice AD models, particularly, serotonin receptors of type 6 (5-HT6) regulates neuronal cilia length, morphology, and composition that are related to cognition (Brodsky et al., 2017; Hu et al., 2017). 5-HT6 is also expressed in astrocytes and localized in the primary cilia (Hirst et al., 1997; Jiang et al., 2019; Barbeito et al., 2021). The binding of serotonin to 5-HT6 activates the Shh pathway by stimulating GPCR-dependent cAMP signaling (Jiang et al., 2019).
Astrocytes from mice with nervous system injury exhibited a disturbance of Shh signaling in the vicinity of the lesion site in a study (Allahyari et al., 2019). Intriguingly, activation of Shh signaling also affects the proliferation of reactive astrocytes under chronic injury conditions (Sirko et al., 2013). Additionally, activated Shh signaling inhibits inflammation by limiting leukocyte invasion under injury conditions (Allahyari et al., 2019). It is notable that astrocytes responding to Shh signals affect not only themselves but also neurons as they reduce the expression of GFAP in neurons to promote neuroprotection (Sirko et al., 2013; Ugbode et al., 2017). Furthermore, Shh signaling activated in neurons acts as a physiological cue for astrocytes (Amankulor et al., 2009; Farmer et al., 2016). Thus, these findings suggest the importance of Shh signal transduction in communication between neurons and astrocytes in the CNS, particularly in injury-induced neurotoxic inflammatory states. Taken together, these studies suggest that primary cilia in astrocytes are pivotal in regulating Shh signaling to facilitate proliferation and maturation of neural stem and progenitor cells to repair the damaged CNS, including AD.
Parkinson’s Disease
Parkinson’s disease is a neurodegenerative disease characterized by the degeneration of ventral midbrain dopaminergic (DA) neurons and the accumulation of α-synuclein-positive cytoplasmic inclusions in neurons (Dauer and Przedborski, 2003; Surmeier, 2018). Although PD has been largely regarded as a DA neuronal disease, recent studies have indicated the involvement of astrocytes; in particular, some PD-related genes appear to play important roles in several astrocyte functions, such as the control of inflammation, autophagy, calcium signaling, and neuroprotection (Hernandez et al., 2016; Booth et al., 2017). For instance, mutations in leucine-rich repeat kinase 2 (LRRK2), the most frequent causative gene identified in PD (Zimprich et al., 2004; Trinh et al., 2014), result in dysfunction of astrocytes in the clearance of α-synuclein, thereby decreasing the number of DA neurons (Greggio et al., 2006). LRRK2 is a multifunctional protein comprising several domains at its C-terminus, including a leucine-rich domain (LRR), a GTPase domain, the carboxy-terminal region of the Ras domain, a kinase domain, and a WD40 domain (Zimprich et al., 2004; Greggio and Cookson, 2009). LRRK2 is constitutively expressed in most of cells in the nervous system, including neurons, astrocytes, microglia, and OLGs (Miklossy et al., 2006). However, most LRRK2-related studies on PD pathogenesis have been limited in neurons. Loss of LRRK2 in cortical neurons causes mitochondrial dysfunction due to decreased Ca2+ extrusion of Na+/Ca2+/Li+ exchanger (NCLX) efflux (Ludtmann et al., 2019) and reduction in glutamatergic transmission and synaptic protein expression (Beccano-Kelly et al., 2014). In addition, in PD, LRRK2 mutations induce cell death by activating kinase activity (Greggio et al., 2006) or microtubule-associated neurotoxicity and neurodegeneration by oligomerizing LRRK2 (Kett et al., 2012; Dhekne et al., 2018; Watanabe et al., 2020).
Based on the function of LRRK in controlling microtubule dynamics through direct interaction with microtubules (Gillardon, 2009; Kett et al., 2012), a potential role for LRRK2 in ciliary biogenesis has been suggested. Several studies have reported the key roles of LRRK2 in ciliogenesis: LRRK2 is involved in the removal of CP110 and the recruitment of TTBK2 at the mother centriole (the base of cilium) (Sobu et al., 2021). It is also involved in the phosphorylation of RAB10 on binding to RILPL1 (Steger et al., 2017; Dhekne et al., 2018; Figure 1C and Table 1). In addition, the data that LRRK2 interacts with microtubule components, such as TUBB, and affects microtubule acetylation (Law et al., 2014) suggest that LRRK2 may be involved in axonemal tubulin acetylation for cilia assembly. Thus, these previous findings, including that inhibition of Shh leads to neuronal damage by interfering with neuroprotection against neurotoxicity (Patel et al., 2017), collectively suggest that mechanisms regulating primary cilia generation in astrocytes may be a target for the treatment of neurodegenerative diseases such as PD.
Huntington’s Disease
Huntington’s disease, the most common autosomal dominant neurodegenerative disease, presents with pathological features such as neuronal dysfunction associated with excessive movement and cognitive impairment. It is well known that CAG triplet repeat expansion, encoding polyglutamine, within huntingtin (HTT) leads to the production of mutant HTT (mHTT) fragments, which is a major cause of HD (Finkbeiner, 2011). Previous study findings, including that mHTT is specifically expressed in astrocytes in a mouse model of HD (Bradford et al., 2009), have suggested that astrocytes are critically involved in the pathological mechanism of HD (Diaz-Castro et al., 2019; Gray, 2019). Remarkably, a recent study reported that a reduction of mHTT in astrocytes in an HD mouse model resulted in recovery of neuron functions, including neuroprotection (Wood et al., 2019). However, the molecular mechanisms underlying the pathology of HD in astrocytes remain poorly understood. The interaction of HTT with microtubules regulates microtubule-dependent transport for cilia formation by binding to huntingtin-associated protein 1 (HAP1) and pericentriolar material 1 (PCM1) proteins (Keryer et al., 2011; Table 1). Accordingly, mHTT induces the accumulation of PCM1 around the centrosomes, leading to the formation of abnormally long cilia that might be involved in inhibiting neuroprotection (Keryer et al., 2011; Figure 1C).
Previous studies have shown that HAP1 interacts with Abelson helper integration site 1, which plays a role in primary cilia-mediated Wnt signal transduction (Sheng et al., 2008; Lancaster et al., 2011), and that mHTT induces the accumulation of cytoplasmic β-catenin (Godin et al., 2010; Ghatak and Raha, 2018). In addition, in the process of ciliogenesis, mHTT affects membrane trafficking by interfering with the activity of small GTPases, such as RAB8 and RAB11 (Li et al., 2009, 2010; Knodler et al., 2010). Taken together, these data suggest that HTT may be essential for neuroprotection-related Wnt signaling by regulating primary cilia assembly through activation of RAB8 and RAB11 in astrocytes, and they also suggest that abnormalities of astrocyte cilia may be closely associated with HD pathogenesis.
Potential Role of Astrocyte Cilia in NF-κB Signaling
The role of primary cilia in astrocytes, which are glial cells involved in various processes such as neuronal support, homeostasis maintenance, and neuronal transmission, remains unclear in neurodegenerative diseases. However, the findings that astrocytes have primary cilia and multiple neurological disease mechanisms are associated with ciliary functions have generated immense interest in the role of astrocyte cilia. Several inflammation-related signaling pathways, including the Shh, Wnt, and NF-κB signaling pathways, are controlled by primary cilia (Wann and Knight, 2012; Baek et al., 2017). Thus, previous studies have reported an involvement of primary cilia in many inflammatory diseases such as obesity, polycystic kidney disease, and osteoarthritis (Song et al., 2017; Ritter et al., 2018; Sheffield et al., 2018). Although the role of primary cilia in inflammatory signaling-related neurological diseases has not been much investigated, it has been reported that the induction of inflammation by lipopolysaccharide treatment shortens the length of primary cilia in hippocampal neurons (Baek et al., 2017).
Persistent activation of NF-κB, which is considered a master regulator of inflammation (Hayden and Ghosh, 2012), is associated with several neurodegenerative diseases including AD, PD, and ALS (Ju Hwang et al., 2019; Singh et al., 2020; Kallstig et al., 2021). Based on the important role of astrocytes in the regulation of neuroinflammation (Colombo and Farina, 2016), studies on the mechanism of NF-κB signaling regulation in astrocytes have gained interest. Previous studies have revealed that inactivation of NF-κB in astrocytes reduces the expression of chemokines in mice with spinal cord injury (Brambilla et al., 2005) and decreases demyelination in mice with vascular dementia (Saggu et al., 2016). These findings suggest the importance of the inhibitory regulation of NF-κB signaling in astrocytes to prevent neurodegenerative diseases.
Notably, in a mouse model, activation of NF-κB signaling specific for astrocytes not only induced the secretion of several chemokines but also impaired ependymal ciliary formation (Lattke et al., 2012). Further studies have revealed that primary cilia of fibroblasts and chondrocytes in response to inflammation are involved in NF-κB signaling through IKK activity (Wann and Knight, 2012; Wann et al., 2014). However, direct evidence for the effect of NF-κB signaling regulation on ciliary formation in astrocytes is lacking. Nevertheless, previous findings suggest the importance of primary cilia of astrocytes in regulating NF-κB signaling to prevent or treat inflammation-associated neurodegenerative diseases.
Primary Cilium in Oligodendrocytes: A Key Mediator for Myelination in the Central Nervous System
OLGs differentiated from OPCs are involved in CNS myelination, a process that wraps around nerve axons by producing multiple layers of myelin that extend from the cell membrane (Nave, 2010; Stadelmann et al., 2019). These cells are susceptible to cytotoxicity/excitotoxicity, and defects in OLGs result in several neurological diseases, including AD, multiple sclerosis (MS), and schizophrenia (Kuhn et al., 2019). Impairment of the OLG differentiation process is associated with aging and the pathogenesis of MS (Tiane et al., 2019). The role of primary cilia in the pathological mechanisms of demyelinating diseases has not been investigated much, but recent studies have reported that major ciliary molecules, such as kinesin family member 3A (KIF3A) and intraflagellar transport 81, and Shh signaling components, such as SMO and PTCH1, are expressed in mouse cortical OPCs (Cahoy et al., 2008; Zhang et al., 2014; Figure 2A). It was further found that primary cilia of OPCs were disassembled during differentiation into OLGs (Falcon-Urrutia et al., 2015). Notably, in a mouse model, depletion of KIF3A in OPCs inhibited OLG proliferation and differentiation by affecting cilia-dependent Shh signaling and subsequently induced motor defects due to a decrease in myelinated axons (Cullen et al., 2021). Interestingly, the abnormal length of primary cilia in OPCs led to impairment of OLG differentiation (Dugas et al., 2010; Hudish et al., 2016). Therefore, control of ciliary biogenesis, including assembly and disassembly, during OLG differentiation could be a potential target for the treatment of demyelinating disorders.
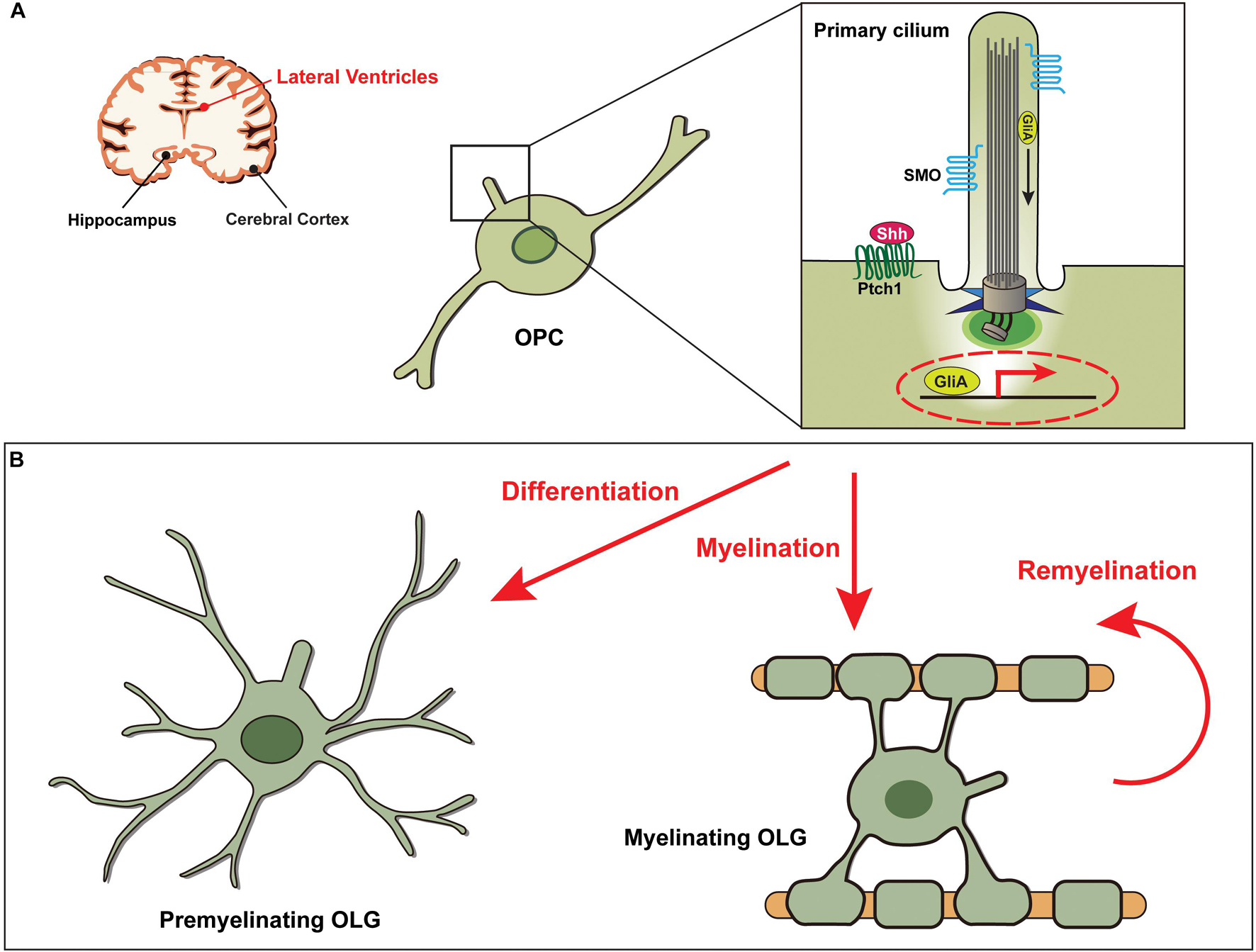
Figure 2. Shh signaling through the primary cilia in OPCs is essential for the development of oligodendrocytes. (A) Diagram showing the primary cilia of oligodendrocyte progenitor cells (OPCs) associated with functions in the lateral ventricles. (B) Shh signaling is activated by binding of the Shh ligand to the Ptch1 receptor, leading to the release of SMO from Ptch1 and its translocation into the primary ciliary axoneme. GliA, the activator form of Gli, then induces transcription of Shh target genes, which are involved in differentiation of OPCs to oligodendrocytes (OLGs) and in (re)myelination of OLGs.
Multiple Sclerosis
Multiple sclerosis is a chronic inflammation-induced demyelinating disease that results in progressive neurological impairment in the CNS. As the CNS is damaged, the OPCs in the injured regions differentiate into OLGs. If this mechanism is disturbed, less OLGs are produced, resulting in MS (Dulamea, 2017; Kuhn et al., 2019). Hence, previous studies have suggested that the control of remyelination by differentiated OLGs during neuronal protection/regeneration is pivotal in the treatment of MS (Stangel et al., 2017; Baldassari et al., 2019). Accordingly, several therapeutic candidate targets, which are mainly involved in the development or myelination of OLGs via Shh, Wnt, and LINGO1 signaling, have been proposed (Fancy et al., 2009; Cole et al., 2017; Ineichen et al., 2017).
In particular, attention has been paid to Shh signal transduction since it is a key trigger for regulating the differentiation and remyelination by OLGs (Thomas et al., 2014; Traiffort et al., 2016; Sanchez and Armstrong, 2018). In the process of remyelination, the expression of Shh signaling-associated molecules, including SMO and GLI1, is increased, and Shh treatment induces the generation of OPCs and OLGs (Ferent et al., 2013; Table 1). Furthermore, it has been demonstrated that treatment with Shh at injury sites increased axonal myelination by inducing OPC recruitment into the damaged area (Thomas et al., 2014). It is notable that in a chronically demyelinated corpus callosum, a subpopulation of neural stem cells responding to Shh is able to generate OPCs and, thus, induce remyelination (Sanchez et al., 2018). However, the molecular mechanisms involved in OPC development and OLG remyelination by Shh signaling remain elusive. Thus, further studies to elucidate the principal molecular axes may accelerate the development of potential therapies for MS. Based on the requirement of primary cilia for Shh signal transduction essential for tissue development and homeostasis (Briscoe and Therond, 2013; Anvarian et al., 2019), we assume that primary cilia of OPCs are likely to play a key role during differentiation into OLGs (Figure 2B).
Leukodystrophies
Leukodystrophies, which refer to disorders with wasting (dystrophy) and white matter (leuko) in the brain, are heterogeneous neurodegenerative diseases that primarily affect myelination in the CNS (Vanderver et al., 2015). Defects in the development and myelination of OLGs are closely related to the pathogenesis of leukodystrophies (Raymond, 2017). A recent study that performed high-content screening using small molecules with mouse samples suggested that the Shh signaling pathway may be a potential therapeutic target for leukodystrophies (Atzmon et al., 2018). The mechanism by which the compounds work is probably associated with the Shh-dependent myelination of OLGs. In addition, there is a possibility that primary cilia of OLGs are involved in Shh signaling for the regulation of myelination (Figure 2B).
The identification of mutations in tubulin beta class IVA (TUBB4A) in hypomyelination with atrophy of the basal ganglia and cerebellum, a type of leukodystrophy, prompted us to elucidate the role of TUBB4A in OLGs (van der Knaap et al., 2002). Recent studies using cell type-specific RNA-sequencing analyses have shown that TUBB4A is highly expressed in OLGs compared to that in neurons, astrocytes, and microglia in the CNS (Zhang et al., 2014). Corresponding to the involvement of TUBB4A, an isoform of β-tubulin, in microtubule assembly (Simons et al., 2013), mutations in TUBB4A lead to disruption of microtubule stability and polymerization in OLGs (Curiel et al., 2017; Table 1). In addition, mice with mutations in TUBB4A develop severe perturbation of myelin due to microtubule abnormalities in OLGs, resulting hypomyelination and demyelination (Duncan et al., 2017). Although the essential roles of microtubules in various cellular functions, including the development of OLGs, are well known (Lunn et al., 1997; Song et al., 2001; Zuchero et al., 2015), the TUBB4A-mediated mechanism of myelination remains unclear. Given the role of TUBB4A in microtubule assembly (Tischfield et al., 2011; Simons et al., 2013; Duncan et al., 2017), however, it is possible to speculate that TUBB4A may be involved in the assembly of primary cilia in OLGs. Indeed, other tubulin proteins, such as TUBB4 and TUBB4B act as key regulators by interacting with KIF11 in cilia assembly (van Dam et al., 2019; Janke and Magiera, 2020; Zalenski et al., 2020). Taken together, in the absence of treatments for demyelinating diseases in the CNS, studies investigating the role of primary cilia in microtubule-associated myelination processes will help better understand the disease mechanisms.
Primary Cilium in Schwann Cells: A Key Signaling Modulator in the Peripheral Nervous System
In the PNS, Schwann cells are counterparts of OLGs in the CNS. There are two types of Schwann cells, myelinating and non-myelinating, both of which interact with axons to regulate the function of surrounding cells, including neurons and muscle cells (Campana, 2007; Barton et al., 2017; Boerboom et al., 2017). While myelinating Schwann cells induce myelination progression in response to several signaling pathways, such as the PI3K/Akt/mTOR, Wnt, and Nrg1/ErbB signaling (Norrmen and Suter, 2013; Boerboom et al., 2017; Torii et al., 2019), non-myelinating Schwann cells primarily respond to ErbB signaling to regulate axonal, muscle, and neuromuscular junction development (Carroll et al., 1997; Garratt et al., 2000; Newbern and Birchmeier, 2010). Damage to Schwann cells thus leads to either demyelinating diseases or neurotrophic diseases along with axonal defects (Boerboom et al., 2017; Park H.T. et al., 2019). In response to nerve injury, Schwann cells participate in nerve regeneration by activation of their features for de-differentiation and reprogramming (Chen et al., 2007; Martini et al., 2008; Jessen and Mirsky, 2016). Moreover, during the repair process of injured nerves, an increase in cytokines induces an immune response through the activation of C-Jun in Schwann cells (Arthur-Farraj et al., 2012).
Since the first identification of primary cilia in the autonomic nerves and ganglia of adult rats in the PNS (Grillo and Palay, 1963), only a few studies on the role of glia cilia have been reported. Although Schwann cells have emerged as a pivotal therapeutic target for PNS disorders (Lehmann and Hoke, 2010; Barton et al., 2017), direct evidence for the role of cilia in Schwann cells in disease-related mechanisms is very limited. The Shh signal pathway is involved in proliferation and myelination during the development of Schwann cells, and more importantly, it is activated to promote nerve regeneration after injury (Hashimoto et al., 2008; Yoshimura and Takeda, 2012). Hence, the findings of studies focusing on the role of primary cilia of Schwann cells in myelination, particularly on their role in related signaling such as Shh, are noteworthy (Yoshimura and Takeda, 2012; Figure 3). In the following sections, we discuss the involvement of Schwann cell cilia in the regulation of nerve regeneration and mechanisms of neurodegenerative diseases and highlight the importance of investigating the functions of Schwann cell cilia in PNS disorders.
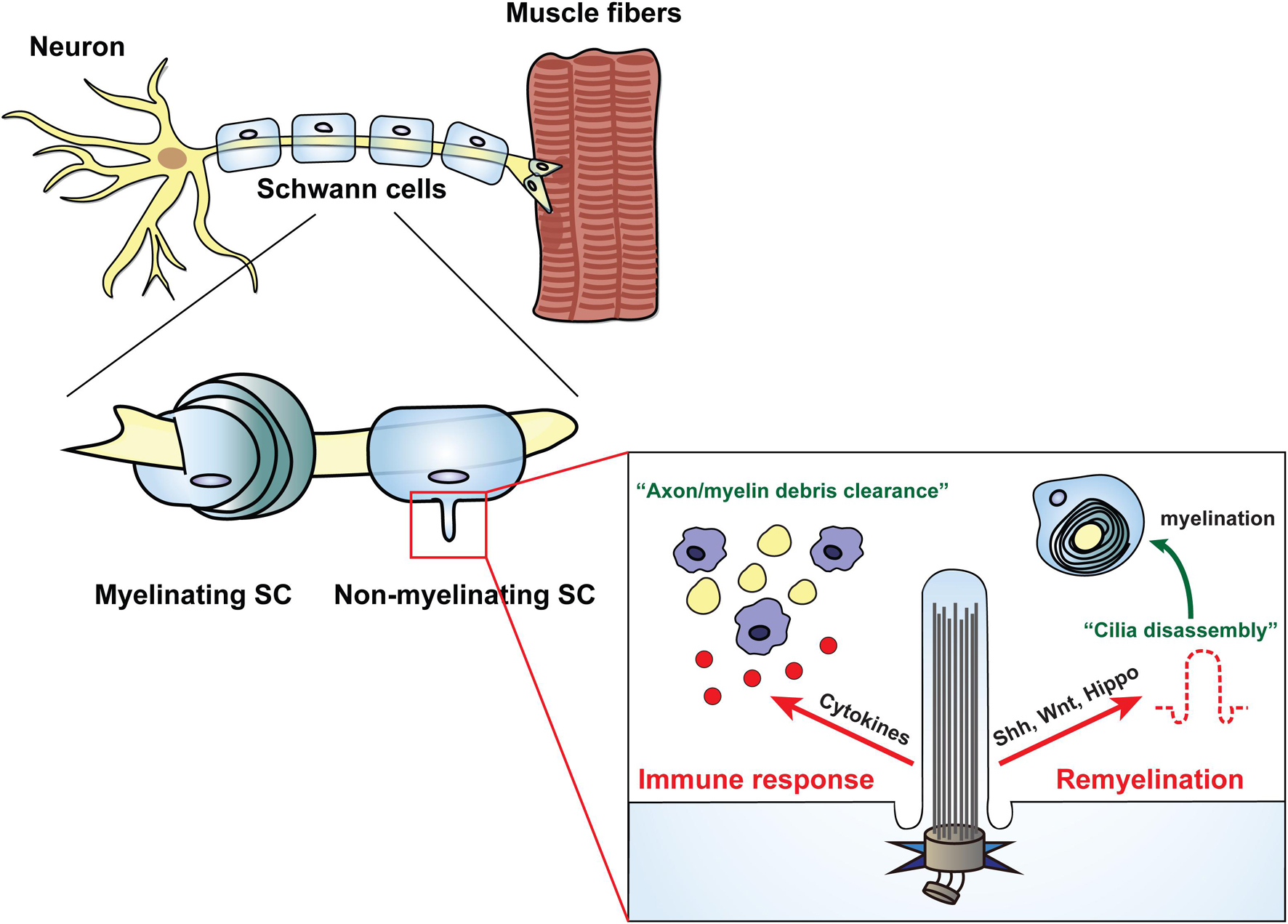
Figure 3. Schwann cells regulate various cellular processes through the primary cilia. Primary cilia generated in non-myelinating Schwann cells are involved in cellular events through dynamic biogenesis of assembly and disassembly. In the immune response state, the primary cilia of Schwann cells induce clearance of axon debris by macrophages through activation of inflammatory cytokines. In PNS injury, Schwann cells play a role in axonal regeneration by inducing remyelination through the disassembly of primary cilia, which is dependent on several signal transductions such as Shh, Wnt, and Hippo.
Amyotrophic Lateral Sclerosis
Amyotrophic lateral sclerosis (ALS), characterized by progressive muscle weakness and ultimately fatal loss of muscle function, is a well-known peripheral neurodegenerative disease. It is alternatively called motor neuron disease and is generally diagnosed using nerve conduction studies, electromyography, and muscle function evaluation (Daube, 2000). Several causative genes, such as ALS2, DCTN1, VAPB, ANG, TDP-43, FUS, and SOD1, have been identified from patients with ALS. Mutations in the representative gene SOD1 are involved in demyelination, motor neuronal degeneration, and decreased regeneration (McCord, 1994; Andrus et al., 1998; Beers et al., 2006; Yamanaka et al., 2008; Lobsiger et al., 2009). Although the role of SOD1 in motor neurons has been relatively actively studied, little research has been conducted on the role of SOD1 in glial cells. Indeed, overexpression of SOD1 induces oxidative stress and lipid peroxidation in both motor neurons and glial cells, including Schwann cells (Rosen, 1993; Pasinelli and Brown, 2006).
Previously, it was reported that mice with non-myelinating Schwann cells expressing a point mutation form of SOD1 (SOD1 G93A) showed severe motor neuronal degeneration, reduced neuronal regeneration, and accelerated ALS disease pathologies (Lobsiger et al., 2009). Although the relationship between primary cilia in Schwann cells and ALS pathological mechanisms has not yet been elucidated, studies using a mouse model harboring an SOD1 (G93A) mutation have suggested the involvement of primary cilia in motor neuronal functions (Ma et al., 2011; Osking et al., 2019). Notably, mutated SOD1 (G93A) increases the expression of ACTIN cytoskeleton genes (Perrin et al., 2005; de Oliveira et al., 2013). In addition, SOD1 (G93A) mutant mice showed increased expression of several Wnt signaling-related genes, including both canonical and non-canonical Wnts in the spinal cord (Yu et al., 2013; Table 1). ACTIN-mediated cellular dynamics is important not only for neuronal function but also for ciliary formation and function (Smith et al., 2020), and Wnt signaling is important for initiating myelination (Tawk et al., 2011) and controlling ciliary formation and function (Lee, 2020). Accordingly, studies determining whether primary cilia in Schwann cells are involved in the pathological mechanism of ALS through the regulation of Wnt signaling or ACTIN-mediated cellular function would be of interest.
Charcot Marie Tooth Disease
Charcot Marie Tooth disease (CMT) is a common hereditary neurological disorder of the PNS, in which the muscles of the hands and feet are gradually lost, making movement difficult (Pareyson and Marchesi, 2009). Among the subtypes of CMT, type 1 is mainly caused by impairment of Schwann cells that play a role in myelination, and the mutations identified in CMT type 1 are found in genes coding for myelination regulators such as PMP22, MPZ, and EGR2 (Hattori et al., 2003). These myelin proteins are expressed in myelinating Schwann cells and are involved in responding to signals that control myelination. For instance, in the process of myelination, MPZ in Schwann cells is essential for responding to cAMP signals derived from neuronal axons (LeBlanc et al., 1992). cAMP signaling plays a critical role in the process of Schwann cell development, including proliferation and myelination (Morgan et al., 1991). Of note, primary cilia are involved in cAMP signaling through adenylate cyclase type III, a representative cilia protein in the nervous system (Wang et al., 2011; Sterpka and Chen, 2018; Table 1). These data suggest that studies investigating the role of primary cilia in myelination-related signal transduction in Schwann cells will help to better understand the mechanisms of demyelinating diseases, such as CMT.
As studies related to the repair mechanism of Schwann cell myelination for the treatment of peripheral degenerative diseases have gained traction, the investigation of the role of Schwann cell cilia in nerve regeneration has also attracted attention (Jessen and Mirsky, 2016). Several recent studies have suggested that primary cilia in Schwann cells are involved in myelination-related signal transduction, such as Shh, Wnt, and Hippo signaling (Tawk et al., 2011; Yoshimura and Takeda, 2012; Morton et al., 2013; Grove et al., 2017; Jiang et al., 2019; Mc Fie et al., 2020; Figure 3 and Table 1). In mouse primary Schwann cells, major Shh components such as SMO and PTCH1 are localized at the base of primary cilia, and these activate Shh signal transduction (Wilson and Stainier, 2010; Yoshimura and Takeda, 2012). Treatment with Shh or SMO agonist results in an increase in myelin segments, suggesting the potential involvement of Schwann cell cilia in regulating myelination (Parmantier et al., 1999; Wilson and Stainier, 2010).
In the MSC80 mouse Schwann cell line, key molecules of canonical Wnt signaling, such as WNT1, LRP6, DSH, GSK3β, β-catenin, and TCF/LEF1, are expressed prior to activation of the myelination regulators PMP22 and MPZ (Tawk et al., 2011). Given that canonical Wnt signaling regulates cilia assembly through WNT3A or cilia disassembly through WNT5A (Lee et al., 2012; Kyun et al., 2020), it is possible to speculate that primary cilia in Schwann cells may be involved in Wnt-mediated myelination. Yes-associated protein (YAP) and transcriptional coactivator with PDZ-binding motif (TAZ), are Hippo signaling regulators, have a key role in the cytoplasm of ciliated cells, but these translocate into the nucleus and induce cell proliferation in the context of ciliogenesis suppression (Rausch and Hansen, 2020). Notably, both YAP and TAZ are expressed in myelinating Schwann cells, but not in non-myelinating Schwann cells (Grove et al., 2017; Jeanette et al., 2021). Thus, this suggests a potential role for primary cilia-mediated Hippo signaling in myelination. More intriguingly, YAP and TAZ play a role in remyelination during Schwann cells-mediated regeneration induced by nerve injury (Grove et al., 2020; Jeanette et al., 2021). Taken together, these data suggest that primary cilia in Schwann cells may be essential in myelination involving several signaling pathways; thus, control of Schwann cell ciliogenesis could be a potential target for the treatment of PNS demyelinating disorders.
Functional Potential of Schwann Cell Cilia in Nerve Regeneration–Related Signaling
Calcium Signaling
Given that the primary cilia in chondrocytes are essential for mechanotransduction via ATP-induced Ca2+ signaling, it can be speculated that primary cilia in Schwann cells may regulate mitochondrial mechanotransduction through Ca2+ signaling molecules, such as inositol 1.4.5-triphosphate (IP3) (Powell et al., 2003; Wann et al., 2012; Phua et al., 2015); IP3 and its receptor are also present in Schwann cells, and they play an important role in regulating mitochondrial Ca2+ signaling during myelination (Powell et al., 2003; Ino et al., 2015). It is noteworthy that in a Xenopus model, primary cilia are reduced when IP3 signaling is inhibited, suggesting that IP3 signaling is involved in ciliogenesis (Hatayama et al., 2011). Particularly, in Schwann cells, P2Y2 and P2RY2, which produce IP3 and increase the level of mitochondrial Ca2+, appear to be associated with ciliogenesis (Weisman et al., 2012; Table 1).
Cell Adhesion Molecule-Mediated Signaling
Another notable molecule associated with ciliogenesis of Schwann cells is Polysialic acid (PSA), a homopolymer of α2, 8-linked sialic acid expressed in the nervous systems. PSA is involved in axon guidance and activation of neuronal cell adhesion molecules (NCAMs) (Tang et al., 1994; Lavdas et al., 2006; Papastefanaki et al., 2007). Recent reports have revealed that the PSA-NCAM complex is decreased in the brains of diseased humans and rodents (Varea et al., 2005; Murray et al., 2016), and modified PSA-NCAM complexes can be seen in several neurodegenerative diseases such as AD and PD (Murray et al., 2016). In the PNS, NCAMs are expressed in the membranes of Schwann cells, and these regulate injury-induced remyelination by making a complex with PSA (Angata and Fukuda, 2003; Lavdas et al., 2006; Papastefanaki et al., 2007). Studies using zebrafish models have reported that PSA and NCAMs exist in primary cilia of the CNS; however, this has not yet been investigated in the PNS (Matsumoto et al., 2019). Furthermore, the number of cells expressing NCAMs and PSA was reduced when primary cilia formation was inhibited in the mouse brain (neural stem cells), suggesting an important role of cilia in NCAM-PSA complex-mediated cell proliferation in the CNS (Han et al., 2008; Table 1). Based on these studies conducted in the CNS, we assume that the NCAM-PSA complex present in Schwann cells may also be associated with primary cilia in the PNS.
Several cell adhesion molecules (CAMs), including NCAM, myelin-associated glycoprotein, neuregulin1, neural adhesion molecules (L1), integrin β1, and N-cadherin, are essential for Schwann cell development as well as for cell-cell connection in the PNS (Quarles, 2002; Spiegel and Peles, 2002; Bartsch, 2003; Poliak and Peles, 2003; Michailov et al., 2004; Feltri and Wrabetz, 2005; Taveggia et al., 2005). In particular, CAMs are secreted as autocrine factors from Schwann cells activated by Shh signals during myelination (Parmantier et al., 1999; Hashimoto et al., 2008). Given the important roles of primary cilia for Shh signaling in the control of myelination by glial cells (Yoshimura and Takeda, 2012; Falcon-Urrutia et al., 2015), it is likely that primary cilia in Schwann cells are involved in the secretion of CAMs, including NCAM, to prevent neurodegeneration or to induce nerve regeneration.
Neuromuscular Junction-Involved Signaling
Many neurodegenerative diseases of the PNS have features of muscle defects such as muscle weakness, cramps, numbness, and tingling in the hands and feet (Burakgazi and Hoke, 2010; No authors listed, 2010; van Sloten et al., 2011). Typically, these muscle-related symptoms result from impaired innervation at the neuromuscular junction (NMJ), which is regulated not only by muscle cells but also by neurons and Schwann cells (Gordon, 2020). Thus, the sophisticated regulation of cellular communication between these cells is closely related to disease mechanisms in the PNS. In cellular communication, Schwann cells are involved in NMJ formation through TGFβ signaling and signal transmission in the NMJ via Ca2+ (Robitaille, 1998; Castonguay and Robitaille, 2001; Sugiura and Lin, 2011; Fuentes-Medel et al., 2012; Kerr et al., 2014; Barik et al., 2016). Under a condition of nerve injury, Schwann cells communicate with neurons by releasing several molecules, such as cytokines, chemokines, and growth factors, to activate neuronal function (Ydens et al., 2013; Wei et al., 2019). These molecules are also involved in the immune responses of Schwann cells that protect neurons from nerve damages and control neurotrophic factors-mediated signaling for nerve regeneration (Agthong et al., 2009; Kidd et al., 2013; Ydens et al., 2013).
Neurotrophic Factor-Mediated Signaling
Neurotrophic factors, including brain-derived neurotrophic factor and glial cell line-derived neurotrophic factor, are essential throughout Schwann cell development process, including the migration, proliferation, and (re)myelination stages (Yamauchi et al., 2004; Iwase et al., 2005; Madduri and Gander, 2010). Thus, misregulation of neurotrophic factor-mediated signaling in Schwann cells results in neuronal cell death and defects in axonal regeneration, which in turn leads to the failure of neuronal repair and muscle innervation (Arthur-Farraj et al., 2012; Glat et al., 2016; Guy et al., 2019). Based on the findings that primary cilia are associated with several signal transductions, previous studies have suggested the involvement of primary cilia in neurotrophic factors-dependent signaling in Schwann cells (Yamauchi et al., 2004; Yoshimura and Takeda, 2012). It is noteworthy that Rho GTPases, regulators of ciliogenesis (Kakiuchi et al., 2019), play a key role in neurotrophic factor-mediated signaling in Schwann cells (Yamauchi et al., 2004), and Schwann cells involved in regeneration have primary cilia (Yoshimura and Takeda, 2012). In conclusion, these findings suggest that primary cilia in Schwann cells are essential for signaling to control nerve regeneration (Figure 3). Moreover, the regulatory mechanisms for Schwann cell ciliogenesis could be a potential target for PNS regeneration therapy.
Conclusion and Perspective
Primary cilia play a variety of essential roles in the development of the nervous system and are implicated in several neurological diseases. Many studies focusing on the function of neuronal primary cilia have provided insights into the importance of targeting cilia-mediated signaling for the treatment of neurological diseases (Kirschen and Xiong, 2017). However, as these studies were focused on neurons, our understanding of the mechanisms of neurological diseases associated with damage to other types of cells, such as glial cells, is limited. There is growing evidence that primary cilia-mediated signaling in glial cells may be closely linked to the pathogenesis of several neurological diseases (Sterpka and Chen, 2018). In this review, we have presented the latest findings related to glial primary cilia to facilitate understanding of the cilia-mediated function in each glial cell type. In particular, we sought to understand the relationship between the signaling pathways regulated by disease-causing genes and primary cilia in glial cells in each neurodegenerative disease.
Inhibition of the progression of chronic systemic inflammation has been proposed as an approach for the treatment of neurodegenerative diseases (Norden et al., 2015). From this perspective, NF-κB is considered a major target, and Aβ peptide, a major causative molecule in AD, is implicated in the regulation of inflammatory responses through NF-κB activation (Liu et al., 2014). In addition, several related studies have reported that inhibition of NF-κB signaling reduces Aβ-induced neurotoxicity in neuron and glial cells (Yan et al., 2020; Zamani et al., 2020). It is also notable that Aβ peptide induces aberrant cilia formation by interfering with Shh signaling (Vorobyeva and Saunders, 2018). Moreover, LRRK2, a causative molecule in PD, plays an important role in ciliogenesis by activating ciliary regulators, such as RAB8A and RAB10 (Steger et al., 2016, 2017; Alessi and Sammler, 2018), and in inflammatory responses by regulating NF-κB signaling (Kim et al., 2012; Lopez de Maturana et al., 2016). Therefore, the regulatory mechanism of cilia formation may be a novel target for treating neurodegenerative diseases by controlling NF-κB-mediated inflammation. In conclusion, the potential role of astrocyte cilia in the regulation of inflammation in the nervous system implies that the regulatory mechanisms for ciliogenesis in astrocytes could be a novel target for the treatment of neurodegenerative diseases such as AD, PD, and HD. Moreover, screening of anti-inflammatory drugs and small molecules targeting the primary cilia will help discover and develop therapeutic agents for these diseases in the future.
It has been previously suggested that regulation of microtubule dynamics is essential for the development of glial cells, such as OLGs and Schwann cells in the CNS and PNS, respectively (Richter-Landsberg, 2008; Bauer et al., 2009; Yokota et al., 2009; Eom et al., 2011). In particular, OLGs that undergo morphological changes from OPCs during differentiation/maturation for myelination (Miller, 2002) require adequate microtubule dynamics. The regulation of microtubule (de)polymerization and stabilization is critical for interactions of OLGs with neuronal axons (Fu et al., 2019; Lee and Hur, 2020), and the assembled microtubules are important for intracellular transportation of myelin-related proteins, including MBP (Smith, 2004). Histone deacetylases, including SIRT2 and HDAC6, are well known ciliary proteins that modulate cilia disassembly (Pugacheva et al., 2007; Zhou et al., 2014; Lim et al., 2020), and recently, they have been shown to be involved in the microtubules-mediated remodeling of OLGs (Hubbert et al., 2002; Southwood et al., 2007; Noack et al., 2014). In addition, a study has revealed that stathmin, a negative regulator of microtubule dynamics (Ozon et al., 2002), interferes with OLG differentiation, leading to demyelinating diseases such as MS (Liu et al., 2005). In Schwann cells, microtubule-associated protein 1 B binds to α1-syntrophin (Fuhrmann-Stroissnigg et al., 2012), a member of the adaptors acting on signal transduction, or microtubules (Togel et al., 1998) and regulates microtubule dynamics for Schwann cell migration (Bouquet et al., 2007). Although studies demonstrating a direct association of misregulation of microtubule dynamics in Schwann cells with neurodegenerative diseases are lacking, these previous findings suggest that adequate control of primary cilia-involved microtubule dynamics in glial cells can be a target for the treatment of demyelinating diseases.
Since impairment of autophagy mechanism has been frequently reported as a major cause in neurodegenerative disorders (Ravikumar et al., 2005; Lee et al., 2010; Nixon and Yang, 2011; Gomez-Suaga et al., 2012; MacLeod et al., 2013), autophagy regulation may be a target for the treatment of neurological diseases involving glial cells. Mutations in PRESENILIN 1, a major genetic factor in AD, disrupt autolysosomal proteolysis and accelerate the onset and severity of AD (Lee et al., 2010; Nixon and Yang, 2011). Mutations in LRRK2 leading to endosomal-lysosomal trafficking, lysosomal pH and calcium regulation are implicated in PD pathogenesis (Gomez-Suaga et al., 2012; MacLeod et al., 2013). Autophagosomal accumulation induced by the mutated dynein complex increases aggregation-prone proteins in ALS (Ravikumar et al., 2005). Similar to that in other types of cells, autophagy is critical for the developmental processes of OLG and Schwann cells, including for myelination (Jang et al., 2015; Bankston et al., 2019). Indeed, disruption of autophagy-related regulators or signaling affects myelin regulators, such as MBP, resulting in impaired myelination (Dello Russo et al., 2013). Additionally, proper autophagy is essential for removing myelin debris derived from injury to Schwann cells (Gomez-Sanchez et al., 2015; Jang et al., 2015). During ciliogenesis, the reciprocal interactions between ciliary proteins and autophagy molecules are tightly controlled (Pampliega et al., 2013; Tang et al., 2013; Orhon et al., 2015). Hence, these studies suggest that primary cilia of glial cells are involved in the regulation of autophagy during the process of myelination and that the cilia-mediated autophagy mechanism may be a novel target for the treatment of myelination-related diseases. Taken together, studies on the regulatory mechanisms and functions of primary cilia in glial cells will pave a new path for the development of novel paradigm therapies as well as provide a comprehensive understanding of the pathological mechanisms of neurodegenerative diseases in both the CNS and PNS.
Author Contributions
SK, HJ, and JL produced ideas for review contents and wrote the manuscript. All authors contributed to the article and approved the submitted version.
Funding
This work was supported by the National Research Foundation (2021R1A2C3004572 and 2021R1A4A2001389 to JL, 2020R1A6A3A13075788 to SK, and 2021R1A6A3A13041249 to HJ), which is funded by the Korean government’s the Ministry of Science, ICT and Future Planning (MSIP).
Conflict of Interest
The authors declare that the research was conducted in the absence of any commercial or financial relationships that could be construed as a potential conflict of interest.
Publisher’s Note
All claims expressed in this article are solely those of the authors and do not necessarily represent those of their affiliated organizations, or those of the publisher, the editors and the reviewers. Any product that may be evaluated in this article, or claim that may be made by its manufacturer, is not guaranteed or endorsed by the publisher.
References
Agthong, S., Koonam, J., Kaewsema, A., and Chentanez, V. (2009). Inhibition of MAPK ERK impairs axonal regeneration without an effect on neuronal loss after nerve injury. Neurol. Res. 31, 1068–1074. doi: 10.1179/174313209x380883
Akiyama, H., Barger, S., Barnum, S., Bradt, B., Bauer, J., and Cole, G. M. (2000). Inflammation and Alzheimer’s disease. Neurobiol. Aging 21, 383–421.
Allahyari, R. V., Clark, K. L., Shepard, K. A., and Garcia, A. D. R. (2019). Sonic hedgehog signaling is negatively regulated in reactive astrocytes after forebrain stab injury. Sci. Rep. 9:565.
Amankulor, N. M., Hambardzumyan, D., Pyonteck, S. M., Becher, O. J., Joyce, J. A., and Holland, E. C. (2009). Sonic hedgehog pathway activation is induced by acute brain injury and regulated by injury-related inflammation. J. Neurosci. 29, 10299–10308. doi: 10.1523/jneurosci.2500-09.2009
Andrus, P. K., Fleck, T. J., Gurney, M. E., and Hall, E. D. (1998). Protein oxidative damage in a transgenic mouse model of familial amyotrophic lateral sclerosis. J. Neurochem. 71, 2041–2048. doi: 10.1046/j.1471-4159.1998.71052041.x
Angata, K., and Fukuda, M. (2003). Polysialyltransferases: major players in polysialic acid synthesis on the neural cell adhesion molecule. Biochimie 85, 195–206. doi: 10.1016/s0300-9084(03)00051-8
Anvarian, Z., Mykytyn, K., Mukhopadhyay, S., Pedersen, L. B., and Christensen, S. T. (2019). Cellular signalling by primary cilia in development, organ function and disease. Nat. Rev. Nephrol. 15, 199–219. doi: 10.1038/s41581-019-0116-9
Arthur-Farraj, P. J., Latouche, M., Wilton, D. K., Quintes, S., Chabrol, E., Banerjee, A., et al. (2012). c-Jun reprograms Schwann cells of injured nerves to generate a repair cell essential for regeneration. Neuron 75, 633–647. doi: 10.1016/j.neuron.2012.06.021
Atzmon, A., Herrero, M., Sharet-Eshed, R., Gilad, Y., Senderowitz, H., and Elroy-Stein, O. (2018). Drug Screening Identifies Sigma-1-Receptor as a Target for the Therapy of VWM Leukodystrophy. Front. Mol. Neurosci. 11:336. doi: 10.3389/fnmol.2018.00336
Baek, H., Shin, H. J., Kim, J. J., Shin, N., Kim, S., Yi, M. H., et al. (2017). Primary cilia modulate TLR4-mediated inflammatory responses in hippocampal neurons. J. Neuroinflammation 14:189.
Baldassari, L. E., Feng, J., Clayton, B. L. L., Oh, S. H., Sakaie, K., Tesar, P. J., et al. (2019). Developing therapeutic strategies to promote myelin repair in multiple sclerosis. Expert Rev. Neurother. 19, 997–1013. doi: 10.1080/14737175.2019.1632192
Bankston, A. N., Forston, M. D., Howard, R. M., Andres, K. R., Smith, A. E., Ohri, S. S., et al. (2019). Autophagy is essential for oligodendrocyte differentiation, survival, and proper myelination. Glia 67, 1745–1759.
Barbeito, L. (2018). Astrocyte-based cell therapy: new hope for amyotrophic lateral sclerosis patients? Stem Cell Res. Ther. 9:241.
Barbeito, P., Tachibana, Y., Martin-Morales, R., Moreno, P., Mykytyn, K., Kobayashi, T., et al. (2021). HTR6 and SSTR3 ciliary targeting relies on both IC3 loops and C-terminal tails. Life Sci. Alliance 4:e202000746. doi: 10.26508/lsa.202000746
Barik, A., Li, L., Sathyamurthy, A., Xiong, W. C., and Mei, L. (2016). Schwann Cells in Neuromuscular Junction Formation and Maintenance. J. Neurosci. 36, 9770–9781. doi: 10.1523/jneurosci.0174-16.2016
Barton, M. J., John, J. S., Clarke, M., Wright, A., and Ekberg, J. (2017). The Glia Response after Peripheral Nerve Injury: a Comparison between Schwann Cells and Olfactory Ensheathing Cells and Their Uses for Neural Regenerative Therapies. Int. J. Mol. Sci. 18:287. doi: 10.3390/ijms18020287
Bartsch, U. (2003). Neural CAMS and their role in the development and organization of myelin sheaths. Front. Biosci. 8:d477–d490. doi: 10.2741/1028
Basson, M. A. (2012). Signaling in cell differentiation and morphogenesis. Cold Spring Harb. Perspect. Biol. 4:a008151.
Bauer, N. G., Richter-Landsberg, C., and Ffrench-Constant, C. (2009). Role of the oligodendroglial cytoskeleton in differentiation and myelination. Glia 57, 1691–1705. doi: 10.1002/glia.20885
Beccano-Kelly, D. A., Kuhlmann, N., Tatarnikov, I., Volta, M., Munsie, L. N., Chou, P., et al. (2014). Synaptic function is modulated by LRRK2 and glutamate release is increased in cortical neurons of G2019S LRRK2 knock-in mice. Front. Cell. Neurosci. 8:301. doi: 10.3389/fncel.2014.00301
Beers, D. R., Henkel, J. S., Xiao, Q., Zhao, W., Wang, J., Yen, A. A., et al. (2006). Wild-type microglia extend survival in PU.1 knockout mice with familial amyotrophic lateral sclerosis. Proc. Natl. Acad. Sci. U. S. A. 103, 16021–16026. doi: 10.1073/pnas.0607423103
Bishop, G. A., Berbari, N. F., Lewis, J., and Mykytyn, K. (2007). Type III adenylyl cyclase localizes to primary cilia throughout the adult mouse brain. J. Comp. Neurol. 505, 562–571. doi: 10.1002/cne.21510
Bloodgood, R. A. (2009). From central to rudimentary to primary: the history of an underappreciated organelle whose time has come. The primary cilium. Methods Cell Biol. 94, 3–52.
Boerboom, A., Dion, V., Chariot, A., and Franzen, R. (2017). Molecular Mechanisms Involved in Schwann Cell Plasticity. Front. Mol. Neurosci. 10:38. doi: 10.3389/fnmol.2017.00038
Booth, H. D. E., Hirst, W. D., and Wade-Martins, R. (2017). The Role of Astrocyte Dysfunction in Parkinson’s Disease Pathogenesis. Trends Neurosci. 40, 358–370. doi: 10.1016/j.tins.2017.04.001
Bouquet, C., Ravaille-Veron, M., Propst, F., and Nothias, F. (2007). MAP1B coordinates microtubule and actin filament remodeling in adult mouse Schwann cell tips and DRG neuron growth cones. Mol. Cell. Neurosci. 36, 235–247. doi: 10.1016/j.mcn.2007.07.002
Bradford, J., Shin, J. Y., Roberts, M., Wang, C. E., Li, X. J., and Li, S. (2009). Expression of mutant huntingtin in mouse brain astrocytes causes age-dependent neurological symptoms. Proc. Natl. Acad. Sci. U. S. A. 106, 22480–22485. doi: 10.1073/pnas.0911503106
Brambilla, R., Bracchi-Ricard, V., Hu, W. H., Frydel, B., Bramwell, A., Karmally, S., et al. (2005). Inhibition of astroglial nuclear factor kappaB reduces inflammation and improves functional recovery after spinal cord injury. J. Exp. Med. 202, 145–156. doi: 10.1084/jem.20041918
Breunig, J. J., Sarkisian, M. R., Arellano, J. I., Morozov, Y. M., Ayoub, A. E., Sojitra, S., et al. (2008). Primary cilia regulate hippocampal neurogenesis by mediating sonic hedgehog signaling. Proc. Natl. Acad. Sci. U. S. A. 105, 13127–13132. doi: 10.1073/pnas.0804558105
Briscoe, J., and Therond, P. P. (2013). The mechanisms of Hedgehog signalling and its roles in development and disease. Nat. Rev. Mol. Cell Biol. 14, 416–429. doi: 10.1038/nrm3598
Brodsky, M., Lesiak, A. J., Croicu, A., Cohenca, N., Sullivan, J. M., and Neumaier, J. F. (2017). 5-HT6 receptor blockade regulates primary cilia morphology in striatal neurons. Brain Res. 1660, 10–19. doi: 10.1016/j.brainres.2017.01.010
Burakgazi, A. Z., and Hoke, A. (2010). Respiratory muscle weakness in peripheral neuropathies. J. Peripher. Nerv. Syst. 15, 307–313. doi: 10.1111/j.1529-8027.2010.00293.x
Cahoy, J. D., Emery, B., Kaushal, A., Foo, L. C., Zamanian, J. L., Christopherson, K. S., et al. (2008). A transcriptome database for astrocytes, neurons, and oligodendrocytes: a new resource for understanding brain development and function. J. Neurosci. 28, 264–278. doi: 10.1523/jneurosci.4178-07.2008
Campana, W. M. (2007). Schwann cells: activated peripheral glia and their role in neuropathic pain. Brain Behav. Immun. 21, 522–527. doi: 10.1016/j.bbi.2006.12.008
Carroll, S. L., Miller, M. L., Frohnert, P. W., Kim, S. S., and Corbett, J. A. (1997). Expression of neuregulins and their putative receptors, ErbB2 and ErbB3, is induced during Wallerian degeneration. J. Neurosci. 17, 1642–1659. doi: 10.1523/jneurosci.17-05-01642.1997
Carson, M. J., Thrash, J. C., and Walter, B. (2006). The cellular response in neuroinflammation: the role of leukocytes, microglia and astrocytes in neuronal death and survival. Clin. Neurosci. Res. 6, 237–245. doi: 10.1016/j.cnr.2006.09.004
Castonguay, A., and Robitaille, R. (2001). Differential regulation of transmitter release by presynaptic and glial Ca2+ internal stores at the neuromuscular synapse. J. Neurosci. 21, 1911–1922. doi: 10.1523/jneurosci.21-06-01911.2001
Chen, Z. L., Yu, W. M., and Strickland, S. (2007). Peripheral regeneration. Annu. Rev. Neurosci. 30, 209–233.
Cole, K. L. H., Early, J. J., and Lyons, D. A. (2017). Drug discovery for remyelination and treatment of MS. Glia 65, 1565–1589. doi: 10.1002/glia.23166
Colombo, E., and Farina, C. (2016). Astrocytes: key Regulators of Neuroinflammation. Trends Immunol. 37, 608–620. doi: 10.1016/j.it.2016.06.006
Compston, A., Zajicek, J., Sussman, J., Webb, A., Hall, G., Muir, D., et al. (1997). Glial lineages and myelination in the central nervous system. J. Anat. 190, 161–200. doi: 10.1046/j.1469-7580.1997.19020161.x
Cullen, C. L., O’Rourke, M., Beasley, S. J., Auderset, L., Zhen, Y., Pepper, R. E., et al. (2021). Kif3a deletion prevents primary cilia assembly on oligodendrocyte progenitor cells, reduces oligodendrogenesis and impairs fine motor function. Glia 69, 1184–1203. doi: 10.1002/glia.23957
Curiel, J., Rodriguez Bey, G., Takanohashi, A., Bugiani, M., Fu, X., Wolf, N. I., et al. (2017). TUBB4A mutations result in specific neuronal and oligodendrocytic defects that closely match clinically distinct phenotypes. Hum. Mol. Genet. 26, 4506–4518. doi: 10.1093/hmg/ddx338
Danilov, A. I., Gomes-Leal, W., Ahlenius, H., Kokaia, Z., Carlemalm, E., and Lindvall, O. (2009). Ultrastructural and antigenic properties of neural stem cells and their progeny in adult rat subventricular zone. Glia 57, 136–152. doi: 10.1002/glia.20741
Daube, J. R. (2000). Electrodiagnostic studies in amyotrophic lateral sclerosis and other motor neuron disorders. Muscle Nerve 23, 1488–1502.
Dauer, W., and Przedborski, S. (2003). Parkinson’s disease: mechanisms and models. Neuron 39, 889–909.
de Oliveira, G. P., Alves, C. J., and Chadi, G. (2013). Early gene expression changes in spinal cord from SOD1(G93A) Amyotrophic Lateral Sclerosis animal model. Front. Cell. Neurosci. 7:216. doi: 10.3389/fncel.2013.00216
De Strooper, B., and Karran, E. (2016). The Cellular Phase of Alzheimer’s Disease. Cell 164, 603–615.
Dello Russo, C., Lisi, L., Feinstein, D. L., and Navarra, P. (2013). mTOR kinase, a key player in the regulation of glial functions: relevance for the therapy of multiple sclerosis. Glia 61, 301–311. doi: 10.1002/glia.22433
Dhekne, H. S., Yanatori, I., Gomez, R. C., Tonelli, F., Diez, F., Schule, B., et al. (2018). A pathway for Parkinson’s Disease LRRK2 kinase to block primary cilia and Sonic hedgehog signaling in the brain. Elife 7:e40202.
Diaz-Castro, B., Gangwani, M. R., Yu, X., Coppola, G., and Khakh, B. S. (2019). Astrocyte molecular signatures in Huntington’s disease. Sci. Transl. Med. 11:eaaw8546. doi: 10.1126/scitranslmed.aaw8546
Domingues, H. S., Portugal, C. C., Socodato, R., and Relvas, J. B. (2016). Oligodendrocyte, Astrocyte, and Microglia Crosstalk in Myelin Development, Damage, and Repair. Front. Cell Dev. Biol. 4:71. doi: 10.3389/fcell.2016.00071
Dugas, J. C., Cuellar, T. L., Scholze, A., Ason, B., Ibrahim, A., Emery, B., et al. (2010). Dicer1 and miR-219 Are required for normal oligodendrocyte differentiation and myelination. Neuron 65, 597–611. doi: 10.1016/j.neuron.2010.01.027
Dulamea, A. O. (2017). Role of Oligodendrocyte Dysfunction in Demyelination, Remyelination and Neurodegeneration in Multiple Sclerosis. Adv. Exp. Med. Biol. 958, 91–127. doi: 10.1007/978-3-319-47861-6_7
Duncan, I. D., Bugiani, M., Radcliff, A. B., Moran, J. J., Lopez-Anido, C., Duong, P., et al. (2017). A mutation in the Tubb4a gene leads to microtubule accumulation with hypomyelination and demyelination. Ann. Neurol. 81, 690–702. doi: 10.1002/ana.24930
Duronio, R. J., and Xiong, Y. (2013). Signaling pathways that control cell proliferation. Cold Spring Harb. Perspect. Biol. 5:a008904.
Eom, T. Y., Stanco, A., Weimer, J., Stabingas, K., Sibrack, E., Gukassyan, V., et al. (2011). Direct visualization of microtubules using a genetic tool to analyse radial progenitor-astrocyte continuum in brain. Nat. Commun. 2:446.
Etchevers, H. C., Dupin, E., and Le Douarin, N. M. (2019). The diverse neural crest: from embryology to human pathology. Development 146:dev169821.
Fakhoury, M. (2018). Microglia and Astrocytes in Alzheimer’s Disease: implications for Therapy. Curr. Neuropharmacol. 16, 508–518. doi: 10.2174/1570159x15666170720095240
Falcon-Urrutia, P., Carrasco, C. M., Lois, P., Palma, V., and Roth, A. D. (2015). Shh Signaling through the Primary Cilium Modulates Rat Oligodendrocyte Differentiation. PLoS One 10:e0133567. doi: 10.1371/journal.pone.0133567
Fancy, S. P., Baranzini, S. E., Zhao, C., Yuk, D. I., Irvine, K. A., Kaing, S., et al. (2009). Dysregulation of the Wnt pathway inhibits timely myelination and remyelination in the mammalian CNS. Genes Dev. 23, 1571–1585. doi: 10.1101/gad.1806309
Farmer, W. T., Abrahamsson, T., Chierzi, S., Lui, C., Zaelzer, C., Jones, E. V., et al. (2016). Neurons diversify astrocytes in the adult brain through sonic hedgehog signaling. Science 351, 849–854. doi: 10.1126/science.aab3103
Feltri, M. L., and Wrabetz, L. (2005). Laminins and their receptors in Schwann cells and hereditary neuropathies. J. Peripher. Nerv. Syst. 10, 128–143. doi: 10.1111/j.1085-9489.2005.0010204.x
Ferent, J., Zimmer, C., Durbec, P., Ruat, M., and Traiffort, E. (2013). Sonic Hedgehog signaling is a positive oligodendrocyte regulator during demyelination. J. Neurosci. 33, 1759–1772. doi: 10.1523/jneurosci.3334-12.2013
Fu, M. M., McAlear, T. S., Nguyen, H., Oses-Prieto, J. A., Valenzuela, A., Shi, R. D., et al. (2019). The Golgi Outpost Protein TPPP Nucleates Microtubules and Is Critical for Myelination. Cell 179, 132–146.e14.
Fuentes-Medel, Y., Ashley, J., Barria, R., Maloney, R., Freeman, M., and Budnik, V. (2012). Integration of a retrograde signal during synapse formation by glia-secreted TGF-beta ligand. Curr. Biol. 22, 1831–1838. doi: 10.1016/j.cub.2012.07.063
Fuhrmann-Stroissnigg, H., Noiges, R., Descovich, L., Fischer, I., Albrecht, D. E., Nothias, F., et al. (2012). The light chains of microtubule-associated proteins MAP1A and MAP1B interact with alpha1-syntrophin in the central and peripheral nervous system. PLoS One 7:e49722. doi: 10.1371/journal.pone.0049722
G, A., S, P. K., B, N., and Bs, N. (2014). HIV Associated Sensory Neuropathy. J. Clin. Diagn. Res. 8, MC04–MC07. doi: 10.7860/JCDR/2014/8143.4531
Garratt, A. N., Voiculescu, O., Topilko, P., Charnay, P., and Birchmeier, C. (2000). A dual role of erbB2 in myelination and in expansion of the schwann cell precursor pool. J. Cell Biol. 148, 1035–1046. doi: 10.1083/jcb.148.5.1035
Ghatak, S., and Raha, S. (2018). Beta catenin is regulated by its subcellular distribution and mutant huntingtin status in Huntington’s disease cell STHdhQ111/HdhQ111. Biochem. Biophys. Res. Commun. 503, 359–364. doi: 10.1016/j.bbrc.2018.06.034
Gillardon, F. (2009). Leucine-rich repeat kinase 2 phosphorylates brain tubulin-beta isoforms and modulates microtubule stability–a point of convergence in parkinsonian neurodegeneration? J. Neurochem. 110, 1514–1522. doi: 10.1111/j.1471-4159.2009.06235.x
Giordano, L., Vignoli, A., Pinelli, L., Brancati, F., Accorsi, P., Faravelli, F., et al. (2009). Joubert syndrome with bilateral polymicrogyria: clinical and neuropathological findings in two brothers. Am. J. Med. Genet. A 149A, 1511–1515. doi: 10.1002/ajmg.a.32936
Glat, M. J., Benninger, F., Barhum, Y., Ben-Zur, T., Kogan, E., Steiner, I., et al. (2016). Ectopic Muscle Expression of Neurotrophic Factors Improves Recovery After Nerve Injury. J. Mol. Neurosci. 58, 39–45. doi: 10.1007/s12031-015-0648-9
Godin, J. D., Poizat, G., Hickey, M. A., Maschat, F., and Humbert, S. (2010). Mutant huntingtin-impaired degradation of beta-catenin causes neurotoxicity in Huntington’s disease. EMBO J. 29, 2433–2445. doi: 10.1038/emboj.2010.117
Gomez-Sanchez, J. A., Carty, L., Iruarrizaga-Lejarreta, M., Palomo-Irigoyen, M., Varela-Rey, M., Griffith, M., et al. (2015). Schwann cell autophagy, myelinophagy, initiates myelin clearance from injured nerves. J. Cell Biol. 210, 153–168. doi: 10.1083/jcb.201503019
Gomez-Suaga, P., Luzon-Toro, B., Churamani, D., Zhang, L., Bloor-Young, D., Patel, S., et al. (2012). Leucine-rich repeat kinase 2 regulates autophagy through a calcium-dependent pathway involving NAADP. Hum. Mol. Genet. 21, 511–525. doi: 10.1093/hmg/ddr481
Goncalves, N. P., Vaegter, C. B., and Pallesen, L. T. (2018). Peripheral Glial Cells in the Development of Diabetic Neuropathy. Front. Neurol. 9:268. doi: 10.3389/fneur.2018.00268
Gordon, T. (2020). Peripheral Nerve Regeneration and Muscle Reinnervation. Int. J. Mol. Sci. 21:8652. doi: 10.3390/ijms21228652
Greggio, E., and Cookson, M. R. (2009). Leucine-rich repeat kinase 2 mutations and Parkinson’s disease: three questions. ASN Neuro 1:e00002.
Greggio, E., Jain, S., Kingsbury, A., Bandopadhyay, R., Lewis, P., Kaganovich, A., et al. (2006). Kinase activity is required for the toxic effects of mutant LRRK2/dardarin. Neurobiol. Dis. 23, 329–341. doi: 10.1016/j.nbd.2006.04.001
Grillo, M. A., and Palay, S. L. (1963). Ciliated Schwann cells in the autonomic nervous system of the adult rat. J. Cell Biol. 16, 430–436. doi: 10.1083/jcb.16.2.430
Gritsch, S., Lu, J., Thilemann, S., Wortge, S., Mobius, W., Bruttger, J., et al. (2014). Oligodendrocyte ablation triggers central pain independently of innate or adaptive immune responses in mice. Nat. Commun. 5:5472.
Grove, M., Kim, H., Santerre, M., Krupka, A. J., Han, S. B., Zhai, J., et al. (2017). YAP/TAZ initiate and maintain Schwann cell myelination. Elife 6:e20982.
Grove, M., Lee, H., Zhao, H., and Son, Y. J. (2020). Axon-dependent expression of YAP/TAZ mediates Schwann cell remyelination but not proliferation after nerve injury. Elife 9:e50138.
Guy, R., Grynspan, F., Ben-Zur, T., Panski, A., Lamdan, R., Danon, U., et al. (2019). Human Muscle Progenitor Cells Overexpressing Neurotrophic Factors Improve Neuronal Regeneration in a Sciatic Nerve Injury Mouse Model. Front. Neurosci. 13:151. doi: 10.3389/fnins.2019.00151
Habib, N., McCabe, C., Medina, S., Varshavsky, M., Kitsberg, D., vir-Szternfeld, R. D., et al. (2020). Disease-associated astrocytes in Alzheimer’s disease and aging. Nat. Neurosci. 23, 701–706.
Han, Y. G., Spassky, N., Romaguera-Ros, M., Garcia-Verdugo, J. M., Aguilar, A., Schneider-Maunoury, S., et al. (2008). Hedgehog signaling and primary cilia are required for the formation of adult neural stem cells. Nat. Neurosci. 11, 277–284. doi: 10.1038/nn2059
Hashimoto, M., Ishii, K., Nakamura, Y., Watabe, K., Kohsaka, S., and Akazawa, C. (2008). Neuroprotective effect of sonic hedgehog up-regulated in Schwann cells following sciatic nerve injury. J. Neurochem. 107, 918–927.
Hatayama, M., Mikoshiba, K., and Aruga, J. (2011). IP3 signaling is required for cilia formation and left-right body axis determination in Xenopus embryos. Biochem. Biophys. Res. Commun. 410, 520–524. doi: 10.1016/j.bbrc.2011.06.014
Hattori, N., Yamamoto, M., Yoshihara, T., Koike, H., Nakagawa, M., and Yoshikawa, H. (2003). Demyelinating and axonal features of Charcot-Marie-Tooth disease with mutations of myelin-related proteins (PMP22, MPZ and Cx32): a clinicopathological study of 205 Japanese patients. Brain 126, 134–151. doi: 10.1093/brain/awg012
Hayden, M. S., and Ghosh, S. (2012). NF-kappaB, the first quarter-century: remarkable progress and outstanding questions. Genes Dev. 26, 203–234. doi: 10.1101/gad.183434.111
Hernandez, D. G., Reed, X., and Singleton, A. B. (2016). Genetics in Parkinson disease: mendelian versus non-Mendelian inheritance. J. Neurochem. 139, 59–74. doi: 10.1111/jnc.13593
Hildebrandt, F., Attanasio, M., and Otto, E. (2009). Nephronophthisis: disease mechanisms of a ciliopathy. J. Am. Soc. Nephrol. 20, 23–35. doi: 10.1681/asn.2008050456
Hirst, W. D., Price, G. W., Rattray, M., and Wilkin, G. P. (1997). Identification of 5-hydroxytryptamine receptors positively coupled to adenylyl cyclase in rat cultured astrocytes. Br. J. Pharmacol. 120, 509–515. doi: 10.1038/sj.bjp.0700921
Hu, L., Wang, B., and Zhang, Y. (2017). Serotonin 5-HT6 receptors affect cognition in a mouse model of Alzheimer’s disease by regulating cilia function. Alzheimers Res. Ther. 9:76.
Hubbert, C., Guardiola, A., Shao, R., Kawaguchi, Y., Ito, A., Nixon, A., et al. (2002). HDAC6 is a microtubule-associated deacetylase. Nature 417, 455–458. doi: 10.1038/417455a
Hudish, L. I., Galati, D. F., Ravanelli, A. M., Pearson, C. G., Huang, P., and Appel, B. (2016). miR-219 regulates neural progenitors by dampening apical Par protein-dependent Hedgehog signaling. Development 143, 2292–2304.
Ineichen, B. V., Plattner, P. S., Good, N., Martin, R., Linnebank, M., and Schwab, M. E. (2017). Nogo-A Antibodies for Progressive Multiple Sclerosis. CNS Drugs 31, 187–198. doi: 10.1007/s40263-017-0407-2
Ino, D., Sagara, H., Suzuki, J., Kanemaru, K., Okubo, Y., and Iino, M. (2015). Neuronal Regulation of Schwann Cell Mitochondrial Ca(2+) Signaling during Myelination. Cell Rep. 12, 1951–1959. doi: 10.1016/j.celrep.2015.08.039
Iqbal, A., Baldrighi, M., Murdoch, J. N., Fleming, A., and Wilkinson, C. J. (2020). Alpha-synuclein aggresomes inhibit ciliogenesis and multiple functions of the centrosome. Biol. Open 9:bio054338.
Iwase, T., Jung, C. G., Bae, H., Zhang, M., and Soliven, B. (2005). Glial cell line-derived neurotrophic factor-induced signaling in Schwann cells. J. Neurochem. 94, 1488–1499. doi: 10.1111/j.1471-4159.2005.03290.x
Jang, S. Y., Shin, Y. K., Park, S. Y., Park, J. Y., Rha, S. H., Kim, J. K., et al. (2015). Autophagy is involved in the reduction of myelinating Schwann cell cytoplasm during myelin maturation of the peripheral nerve. PLoS One 10:e0116624. doi: 10.1371/journal.pone.0116624
Janke, C., and Magiera, M. M. (2020). The tubulin code and its role in controlling microtubule properties and functions. Nat. Rev. Mol. Cell Biol. 21, 307–326. doi: 10.1038/s41580-020-0214-3
Jeanette, H., Marziali, L. N., Bhatia, U., Hellman, A., Herron, J., Kopec, A. M., et al. (2021). YAP and TAZ regulate Schwann cell proliferation and differentiation during peripheral nerve regeneration. Glia 69, 1061–1074. doi: 10.1002/glia.23949
Jessen, K. R., and Mirsky, R. (2005). The origin and development of glial cells in peripheral nerves. Nat. Rev. Neurosci. 6, 671–682. doi: 10.1038/nrn1746
Jessen, K. R., and Mirsky, R. (2016). The repair Schwann cell and its function in regenerating nerves. J. Physiol. 594, 3521–3531. doi: 10.1113/jp270874
Jiang, J. Y., Falcone, J. L., Curci, S., and Hofer, A. M. (2019). Direct visualization of cAMP signaling in primary cilia reveals up-regulation of ciliary GPCR activity following Hedgehog activation. Proc. Natl. Acad. Sci. U. S. A. 116, 12066–12071.
Jin, Y., Raviv, N., Barnett, A., Bambakidis, N. C., Filichia, E., and Luo, Y. (2015). The shh signaling pathway is upregulated in multiple cell types in cortical ischemia and influences the outcome of stroke in an animal model. PLoS One 10:e0124657. doi: 10.1371/journal.pone.0124657
Ju Hwang, C., Choi, D. Y., Park, M. H., and Hong, J. T. (2019). NF-kappaB as a Key Mediator of Brain Inflammation in Alzheimer’s Disease. CNS Neurol. Disord. Drug Targets 18, 3–10. doi: 10.2174/1871527316666170807130011
Kakiuchi, A., Kohno, T., Kakuki, T., Kaneko, Y., Konno, T., Hosaka, Y., et al. (2019). Rho-kinase and PKCalpha Inhibition Induces Primary Cilia Elongation and Alters the Behavior of Undifferentiated and Differentiated Temperature-sensitive Mouse Cochlear Cells. J. Histochem. Cytochem. 67, 523–535. doi: 10.1369/0022155419841013
Kallstig, E., McCabe, B. D., and Schneider, B. L. (2021). The Links between ALS and NF-kappaB. Int. J. Mol. Sci. 22:3875.
Kasahara, K., Miyoshi, K., Murakami, S., Miyazaki, I., and Asanuma, M. (2014). Visualization of astrocytic primary cilia in the mouse brain by immunofluorescent analysis using the cilia marker Arl13b. Acta Med. Okayama 68, 317–322.
Keren-Shaul, H., Spinrad, A., Weiner, A., Matcovitch-Natan, O., vir-Szternfeld, R. D., Ulland, T. K., et al. (2017). Type Associated with Restricting Development of Alzheimer’s Disease. Cell 169, 1276–1290. e17.
Kerr, K. S., Fuentes-Medel, Y., Brewer, C., Barria, R., Ashley, J., Abruzzi, K. C., et al. (2014). Glial wingless/Wnt regulates glutamate receptor clustering and synaptic physiology at the Drosophila neuromuscular junction. J. Neurosci. 34, 2910–2920. doi: 10.1523/jneurosci.3714-13.2014
Keryer, G., Pineda, J. R., Liot, G., Kim, J., Dietrich, P., Benstaali, C., et al. (2011). Ciliogenesis is regulated by a huntingtin-HAP1-PCM1 pathway and is altered in Huntington disease. J. Clin. Invest. 121, 4372–4382. doi: 10.1172/jci57552
Kett, L. R., Boassa, D., Ho, C. C., Rideout, H. J., Hu, J., Terada, M., et al. (2012). LRRK2 Parkinson disease mutations enhance its microtubule association. Hum. Mol. Genet. 21, 890–899. doi: 10.1093/hmg/ddr526
Kidd, G. J., Ohno, N., and Trapp, B. D. (2013). Biology of Schwann cells. Handb. Clin. Neurol. 115, 55–79.
Kim, B., Yang, M. S., Choi, D., Kim, J. H., Kim, H. S., Seol, W., et al. (2012). Impaired inflammatory responses in murine Lrrk2-knockdown brain microglia. PLoS One 7:e34693. doi: 10.1371/journal.pone.0034693
Kirschen, G. W., and Xiong, Q. (2017). Primary cilia as a novel horizon between neuron and environment. Neural. Regen. Res. 12, 1225–1230. doi: 10.4103/1673-5374.213535
Klein, O. A., Drummond, A., Mhizha-Murira, J. R., Mansford, L., and dasNair, R. (2019). Effectiveness of cognitive rehabilitation for people with multiple sclerosis: a meta-synthesis of patient perspectives. Neuropsychol. Rehabil. 29, 491–512. doi: 10.1080/09602011.2017.1309323
Knodler, A., Feng, S., Zhang, J., Zhang, X., Das, A., Peranen, J., et al. (2010). Coordination of Rab8 and Rab11 in primary ciliogenesis. Proc. Natl. Acad. Sci. U. S. A. 107, 6346–6351. doi: 10.1073/pnas.1002401107
Kuhn, S., Gritti, L., Crooks, D., and Dombrowski, Y. (2019). Oligodendrocytes in Development, Myelin Generation and Beyond. Cells 8:1424. doi: 10.3390/cells8111424
Kyun, M. L., Kim, S. O., Lee, H. G., Hwang, J. A., Hwang, J., Soung, N. K., et al. (2020). Wnt3a Stimulation Promotes Primary Ciliogenesis through beta-Catenin Phosphorylation-Induced Reorganization of Centriolar Satellites. Cell Rep. 30, 1447–1462.e5.
La Sala, G., Di Pietro, C., Matteoni, R., Bolasco, G., Marazziti, D., and Tocchini-Valentini, G. P. (2020). Gpr37l1/prosaposin receptor regulates Ptch1 trafficking, Shh production, and cell proliferation in cerebellar primary astrocytes. J. Neurosci. Res. [Epub Online ahead of print]. doi: 10.1002/jnr.24775
Lancaster, M. A., Schroth, J., and Gleeson, J. G. (2011). Subcellular spatial regulation of canonical Wnt signalling at the primary cilium. Nat. Cell Biol. 13, 700–707. doi: 10.1038/ncb2259
Lattke, M., Magnutzki, A., Walther, P., Wirth, T., and Baumann, B. (2012). Nuclear factor kappaB activation impairs ependymal ciliogenesis and links neuroinflammation to hydrocephalus formation. J. Neurosci. 32, 11511–11523. doi: 10.1523/jneurosci.0182-12.2012
Lavdas, A. A., Franceschini, I., Dubois-Dalcq, M., and Matsas, R. (2006). Schwann cells genetically engineered to express PSA show enhanced migratory potential without impairment of their myelinating ability in vitro. Glia 53, 868–878. doi: 10.1002/glia.20340
Law, B. M., Spain, V. A., Leinster, V. H., Chia, R., Beilina, A., Cho, H. J., et al. (2014). interaction between leucine-rich repeat kinase 2 and specific beta-tubulin isoforms regulates tubulin acetylation. J. Biol. Chem. 289, 895–908. doi: 10.1074/jbc.m113.507913
LeBlanc, A. C., Windebank, A. J., and Poduslo, J. F. (1992). P0 gene expression in Schwann cells is modulated by an increase of cAMP which is dependent on the presence of axons. Brain Res. Mol. Brain Res. 12, 31–38. doi: 10.1016/0169-328x(92)90065-j
Lee, B. Y., and Hur, E. M. (2020). A Role of Microtubules in Oligodendrocyte Differentiation. Int. J. Mol. Sci. 21:1062. doi: 10.3390/ijms21031062
Lee, J. H., Yu, W. H., Kumar, A., Lee, S., Mohan, P. S., Peterhoff, C. M., et al. (2010). Lysosomal proteolysis and autophagy require presenilin 1 and are disrupted by Alzheimer-related PS1 mutations. Cell 141, 1146–1158. doi: 10.1016/j.cell.2010.05.008
Lee, K. H. (2020). Involvement of Wnt signaling in primary cilia assembly and disassembly. FEBS J. 287, 5027–5038. doi: 10.1111/febs.15579
Lee, K. H., Johmura, Y., Yu, L. R., Park, J. E., Gao, Y., Bang, J. K., et al. (2012). Identification of a novel Wnt5a-CK1varepsilon-Dvl2-Plk1-mediated primary cilia disassembly pathway. EMBO J. 31, 3104–3117. doi: 10.1038/emboj.2012.144
Lehmann, H. C., and Hoke, A. (2010). Schwann cells as a therapeutic target for peripheral neuropathies. CNS Neurol. Disord. Drug Targets 9, 801–806. doi: 10.2174/187152710793237412
Lempp, C., Spitzbarth, I., Puff, C., Cana, A., Kegler, K., Techangamsuwan, S., et al. (2014). New aspects of the pathogenesis of canine distemper leukoencephalitis. Viruses 6, 2571–2601. doi: 10.3390/v6072571
Li, T., Chen, X., Zhang, C., Zhang, Y., and Yao, W. (2019). An update on reactive astrocytes in chronic pain. J. Neuroinflammation 16:140.
Li, X., Standley, C., Sapp, E., Valencia, A., Qin, Z. H., Kegel, K. B., et al. (2009). Mutant huntingtin impairs vesicle formation from recycling endosomes by interfering with Rab11 activity. Mol. Cell. Biol. 29, 6106–6116. doi: 10.1128/mcb.00420-09
Li, X., Valencia, A., Sapp, E., Masso, N., Alexander, J., Reeves, P., et al. (2010). Aberrant Rab11-dependent trafficking of the neuronal glutamate transporter EAAC1 causes oxidative stress and cell death in Huntington’s disease. J. Neurosci. 30, 4552–4561. doi: 10.1523/jneurosci.5865-09.2010
Liao, M. C., Muratore, C. R., Gierahn, T. M., Sullivan, S. E., Srikanth, P., De Jager, P. L., et al. (2016). Single-Cell Detection of Secreted Abeta and sAPPalpha from Human IPSC-Derived Neurons and Astrocytes. J. Neurosci. 36, 1730–1746. doi: 10.1523/jneurosci.2735-15.2016
Lim, J., Son, J., Ryu, J., and Kim, J. E. (2020). SIRT2 Affects Primary Cilia Formation by Regulating mTOR Signaling in Retinal Pigmented Epithelial Cells. Int. J. Mol. Sci. 21:2240. doi: 10.3390/ijms21062240
Liu, A., Stadelmann, C., Moscarello, M., Bruck, W., Sobel, A., Mastronardi, F. G., et al. (2005). Expression of stathmin, a developmentally controlled cytoskeleton-regulating molecule, in demyelinating disorders. J. Neurosci. 25, 737–747. doi: 10.1523/jneurosci.4174-04.2005
Liu, R. T., Wang, A., To, E., Gao, J., Cao, S., Cui, J. Z., et al. (2014). Vinpocetine inhibits amyloid-beta induced activation of NF-kappaB, NLRP3 inflammasome and cytokine production in retinal pigment epithelial cells. Exp. Eye Res. 127, 49–58. doi: 10.1016/j.exer.2014.07.003
Lobsiger, C. S., Boillee, S., McAlonis-Downes, M., Khan, A. M., Feltri, M. L., Yamanaka, K., et al. (2009). Schwann cells expressing dismutase active mutant SOD1 unexpectedly slow disease progression in ALS mice. Proc. Natl. Acad. Sci. U. S. A. 106, 4465–4470. doi: 10.1073/pnas.0813339106
Lopez de Maturana, R., Lang, V., Zubiarrain, A., Sousa, A., Vazquez, N., Gorostidi, A., et al. (2016). Mutations in LRRK2 impair NF-kappaB pathway in iPSC-derived neurons. J. Neuroinflammation 13:295.
Ludtmann, M. H. R., Kostic, M., Horne, A., Gandhi, S., Sekler, I., and Abramov, A. Y. (2019). LRRK2 deficiency induced mitochondrial Ca(2+) efflux inhibition can be rescued by Na(+)/Ca(2+)/Li(+) exchanger upregulation. Cell Death Dis. 10:265.
Lunn, K. F., Baas, P. W., and Duncan, I. D. (1997). Microtubule organization and stability in the oligodendrocyte. J. Neurosci. 17, 4921–4932. doi: 10.1523/jneurosci.17-13-04921.1997
Ma, X., Peterson, R., and Turnbull, J. (2011). Adenylyl cyclase type 3, a marker of primary cilia, is reduced in primary cell culture and in lumbar spinal cord in situ in G93A SOD1 mice. BMC Neurosci. 12:71. doi: 10.1186/1471-2202-12-71
MacLeod, D. A., Rhinn, H., Kuwahara, T., Zolin, A., Di Paolo, G., McCabe, B. D., et al. (2013). RAB7L1 interacts with LRRK2 to modify intraneuronal protein sorting and Parkinson’s disease risk. Neuron 77, 425–439. doi: 10.1016/j.neuron.2012.11.033
Madduri, S., and Gander, B. (2010). Schwann cell delivery of neurotrophic factors for peripheral nerve regeneration. J. Peripher. Nerv. Syst. 15, 93–103. doi: 10.1111/j.1529-8027.2010.00257.x
Marazziti, D., Di Pietro, C., Golini, E., Mandillo, S., La Sala, G., Matteoni, R., et al. (2013). Precocious cerebellum development and improved motor functions in mice lacking the astrocyte cilium-, patched 1-associated Gpr37l1 receptor. Proc. Natl. Acad. Sci. U. S. A. 110, 16486–16491. doi: 10.1073/pnas.1314819110
Martini, R., Fischer, S., Lopez-Vales, R., and David, S. (2008). Interactions between Schwann cells and macrophages in injury and inherited demyelinating disease. Glia 56, 1566–1577. doi: 10.1002/glia.20766
Matsumoto, M., Sawada, M., Garcia-Gonzalez, D., Herranz-Perez, V., Ogino, T., Bang Nguyen, H., et al. (2019). Dynamic Changes in Ultrastructure of the Primary Cilium in Migrating Neuroblasts in the Postnatal Brain. J. Neurosci. 39, 9967–9988. doi: 10.1523/jneurosci.1503-19.2019
Mc Fie, M., Koneva, L., Collins, I., Coveney, C. R., Clube, A. M., Chanalaris, A., et al. (2020). Ciliary proteins specify the cell inflammatory response by tuning NFkappaB signalling, independently of primary cilia. J. Cell Sci. 133:jcs239871.
McCord, J. M. (1994). Mutant mice, Cu,Zn superoxide dismutase, and motor neuron degeneration. Science 266, 1586–1587. doi: 10.1126/science.266.5190.1586-a
McCoy, M. K., and Tansey, M. G. (2008). TNF signaling inhibition in the CNS: implications for normal brain function and neurodegenerative disease. J. Neuroinflammation 5:45. doi: 10.1186/1742-2094-5-45
McGeer, P. L., and McGeer, E. G. (2002). Inflammatory processes in amyotrophic lateral sclerosis. Muscle Nerve 26, 459–470. doi: 10.1002/mus.10191
Meyer, R. C., Giddens, M. M., Schaefer, S. A., and Hall, R. A. (2013). GPR37 and GPR37L1 are receptors for the neuroprotective and glioprotective factors prosaptide and prosaposin. Proc. Natl. Acad. Sci. U. S. A. 110, 9529–9534. doi: 10.1073/pnas.1219004110
Michailov, G. V., Sereda, M. W., Brinkmann, B. G., Fischer, T. M., Haug, B., Birchmeier, C., et al. (2004). Axonal neuregulin-1 regulates myelin sheath thickness. Science 304, 700–703.
Miklossy, J., Arai, T., Guo, J. P., Klegeris, A., Yu, S., McGeer, E. G., et al. (2006). LRRK2 expression in normal and pathologic human brain and in human cell lines. J. Neuropathol. Exp. Neurol. 65, 953–963. doi: 10.1097/01.jnen.0000235121.98052.54
Miller, R. H. (2002). Regulation of oligodendrocyte development in the vertebrate CNS. Prog. Neurobiol. 67, 451–467. doi: 10.1016/s0301-0082(02)00058-8
Morgan, L., Jessen, K. R., and Mirsky, R. (1991). The effects of cAMP on differentiation of cultured Schwann cells: progression from an early phenotype (04+) to a myelin phenotype (P0+, GFAP-, N- CAM-, NGF-receptor-) depends on growth inhibition. J. Cell Biol. 112, 457–467. doi: 10.1083/jcb.112.3.457
Morgello, S., Estanislao, L., Simpson, D., Geraci, A., DiRocco, A., Gerits, P., et al. (2004). HIV-associated distal sensory polyneuropathy in the era of highly active antiretroviral therapy: the Manhattan HIV Brain Bank. Arch. Neurol. 61, 546–551. doi: 10.1001/archneur.61.4.546
Morton, P. D., Dellarole, A., Theus, M. H., Walters, W. M., Berge, S. S., and Bethea, J. R. (2013). Activation of NF-kappaB in Schwann cells is dispensable for myelination in vivo. J. Neurosci. 33, 9932–9936. doi: 10.1523/jneurosci.2483-12.2013
Murphy, N. A., and Franklin, R. J. M. (2017). Recruitment of endogenous CNS stem cells for regeneration in demyelinating disease. Prog. Brain Res. 231, 135–163. doi: 10.1016/bs.pbr.2016.12.013
Murray, H. C., Low, V. F., Swanson, M. E., Dieriks, B. V., Turner, C., Faull, R. L., et al. (2016). Distribution of PSA-NCAM in normal, Alzheimer’s and Parkinson’s disease human brain. Neuroscience 330, 359–375. doi: 10.1016/j.neuroscience.2016.06.003
Nave, K. A. (2010). Myelination and support of axonal integrity by glia. Nature 468, 244–252. doi: 10.1038/nature09614
Newbern, J., and Birchmeier, C. (2010). Nrg1/ErbB signaling networks in Schwann cell development and myelination. Semin Cell Dev. Biol. 21, 922–928. doi: 10.1016/j.semcdb.2010.08.008
Nixon, R. A., and Yang, D. S. (2011). Autophagy failure in Alzheimer’s disease–locating the primary defect. Neurobiol. Dis. 43, 38–45. doi: 10.1016/j.nbd.2011.01.021
No authors listed. (2010). Peripheral neuropathy. Muscle weakness, tingling toes can be warning signs. Mayo Clin Womens Healthsource 14, 4–5.
Noack, M., Leyk, J., and Richter-Landsberg, C. (2014). HDAC6 inhibition results in tau acetylation and modulates tau phosphorylation and degradation in oligodendrocytes. Glia 62, 535–547. doi: 10.1002/glia.22624
Norden, D. M., Muccigrosso, M. M., and Godbout, J. P. (2015). Microglial priming and enhanced reactivity to secondary insult in aging, and traumatic CNS injury, and neurodegenerative disease. Neuropharmacology 96, 29–41. doi: 10.1016/j.neuropharm.2014.10.028
Norrmen, C., and Suter, U. (2013). Akt/mTOR signalling in myelination. Biochem. Soc. Trans. 41, 944–950. doi: 10.1042/bst20130046
Orhon, I., Dupont, N., Pampliega, O., Cuervo, A. M., and Codogno, P. (2015). Autophagy and regulation of cilia function and assembly. Cell Death Differ. 22, 389–397. doi: 10.1038/cdd.2014.171
Ortega, J. A., Radonjic, N. V., and Zecevic, N. (2013). Sonic hedgehog promotes generation and maintenance of human forebrain Olig2 progenitors. Front. Cell. Neurosci. 7:254. doi: 10.3389/fncel.2013.00254
Osking, Z., Ayers, J. I., Hildebrandt, R., Skruber, K., Brown, H., Ryu, D., et al. (2019). SOD1 Mutants Enhance Neurite Outgrowth and Branching in Adult Motor Neurons. iScience 19, 448–449. doi: 10.1016/j.isci.2019.08.004
Ozon, S., Guichet, A., Gavet, O., Roth, S., and Sobel, A. (2002). Drosophila stathmin: a microtubule-destabilizing factor involved in nervous system formation. Mol. Biol. Cell 13, 698–710. doi: 10.1091/mbc.01-07-0362
Pampliega, O., Orhon, I., Patel, B., Sridhar, S., Diaz-Carretero, A., Beau, I., et al. (2013). Functional interaction between autophagy and ciliogenesis. Nature 502, 194–200. doi: 10.1038/nature12639
Papastefanaki, F., Chen, J., Lavdas, A. A., Thomaidou, D., Schachner, M., and Matsas, R. (2007). Grafts of Schwann cells engineered to express PSA-NCAM promote functional recovery after spinal cord injury. Brain 130, 2159–2174. doi: 10.1093/brain/awm155
Pareyson, D., and Marchesi, C. (2009). Diagnosis, natural history, and management of Charcot-Marie-Tooth disease. Lancet Neurol. 8, 654–667. doi: 10.1016/s1474-4422(09)70110-3
Park, H. T., Kim, J. K., and Tricaud, N. (2019). The conceptual introduction of the “demyelinating Schwann cell” in peripheral demyelinating neuropathies. Glia 67, 571–581. doi: 10.1002/glia.23509
Park, S. M., Jang, H. J., and Lee, J. H. (2019). Roles of Primary Cilia in the Developing Brain. Front. Cell. Neurosci. 13:218. doi: 10.3389/fncel.2019.00218
Parmantier, E., Lynn, B., Lawson, D., Turmaine, M., Namini, S. S., Chakrabarti, L., et al. (1999). Schwann cell-derived Desert hedgehog controls the development of peripheral nerve sheaths. Neuron 23, 713–724. doi: 10.1016/s0896-6273(01)80030-1
Pasinelli, P., and Brown, R. H. (2006). Molecular biology of amyotrophic lateral sclerosis: insights from genetics. Nat. Rev. Neurosci. 7, 710–723. doi: 10.1038/nrn1971
Patel, S. S., Tomar, S., Sharma, D., Mahindroo, N., and Udayabanu, M. (2017). Targeting sonic hedgehog signaling in neurological disorders. Neurosci. Biobehav. Rev. 74, 76–97. doi: 10.1016/j.neubiorev.2017.01.008
Perrin, F. E., Boisset, G., Docquier, M., Schaad, O., Descombes, P., and Kato, A. C. (2005). No widespread induction of cell death genes occurs in pure motoneurons in an amyotrophic lateral sclerosis mouse model. Hum. Mol. Genet. 14, 3309–3320. doi: 10.1093/hmg/ddi357
Phua, S. C., Lin, Y. C., and Inoue, T. (2015). An intelligent nano-antenna: primary cilium harnesses TRP channels to decode polymodal stimuli. Cell Calcium 58, 415–422. doi: 10.1016/j.ceca.2015.03.005
Poliak, S., and Peles, E. (2003). The local differentiation of myelinated axons at nodes of Ranvier. Nat. Rev. Neurosci. 4, 968–980. doi: 10.1038/nrn1253
Powell, J. A., Molgo, J., Adams, D. S., Colasante, C., Williams, A., Bohlen, M., et al. (2003). IP3 receptors and associated Ca2+ signals localize to satellite cells and to components of the neuromuscular junction in skeletal muscle. J. Neurosci. 23, 8185–8192. doi: 10.1523/jneurosci.23-23-08185.2003
Pugacheva, E. N., Jablonski, S. A., Hartman, T. R., Henske, E. P., and Golemis, E. A. (2007). HEF1-dependent Aurora A activation induces disassembly of the primary cilium. Cell 129, 1351–1363. doi: 10.1016/j.cell.2007.04.035
Qiu, L., LeBel, R. P., Storm, D. R., and Chen, X. (2016). Type 3 adenylyl cyclase: a key enzyme mediating the cAMP signaling in neuronal cilia. Int. J. Physiol. Pathophysiol. Pharmacol. 8, 95–108.
Quarles, R. H. (2002). Myelin sheaths: glycoproteins involved in their formation, maintenance and degeneration. Cell. Mol. Life Sci. 59, 1851–1871. doi: 10.1007/pl00012510
Rahmlow, M. R., and Kantarci, O. (2013). Fulminant demyelinating diseases. Neurohospitalist 3, 81–91. doi: 10.1177/1941874412466873
Rausch, V., and Hansen, C. G. (2020). The Hippo Pathway, YAP/TAZ, and the Plasma Membrane. Trends Cell Biol. 30, 32–48. doi: 10.1016/j.tcb.2019.10.005
Ravikumar, B., Acevedo-Arozena, A., Imarisio, S., Berger, Z., Vacher, C., O’Kane, C. J., et al. (2005). Dynein mutations impair autophagic clearance of aggregate-prone proteins. Nat. Genet. 37, 771–776. doi: 10.1038/ng1591
Raymond, G. V. (2017). Leukodystrophy: basic and Clinical. Adv. Neurobiol. 15, 365–382. doi: 10.1007/978-3-319-57193-5_14
Richter-Landsberg, C. (2008). The cytoskeleton in oligodendrocytes. Microtubule dynamics in health and disease. J. Mol. Neurosci. 35, 55–63. doi: 10.1007/s12031-007-9017-7
Ritter, A., Louwen, F., and Yuan, J. (2018). Deficient primary cilia in obese adipose-derived mesenchymal stem cells: obesity, a secondary ciliopathy? Obes. Rev. 19, 1317–1328. doi: 10.1111/obr.12716
Robitaille, R. (1998). Modulation of synaptic efficacy and synaptic depression by glial cells at the frog neuromuscular junction. Neuron 21, 847–855. doi: 10.1016/s0896-6273(00)80600-5
Rooryck, C., Pelras, S., Chateil, J. F., Cances, C., Arveiler, B., Verloes, A., et al. (2007). Bardet-biedl syndrome and brain abnormalities. Neuropediatrics 38, 5–9. doi: 10.1055/s-2007-981466
Rosen, D. R. (1993). Mutations in Cu/Zn superoxide dismutase gene are associated with familial amyotrophic lateral sclerosis. Nature 364, 59–62.
Rothhammer, V., and Quintana, F. J. (2015). Control of autoimmune CNS inflammation by astrocytes. Semin. Immunopathol. 37, 625–638. doi: 10.1007/s00281-015-0515-3
Saggu, R., Schumacher, T., Gerich, F., Rakers, C., Tai, K., Delekate, A., et al. (2016). Astroglial NF-kB contributes to white matter damage and cognitive impairment in a mouse model of vascular dementia. Acta Neuropathol. Commun. 4:76.
Sanchez, M. A., and Armstrong, R. C. (2018). Postnatal Sonic hedgehog (Shh) responsive cells give rise to oligodendrocyte lineage cells during myelination and in adulthood contribute to remyelination. Exp. Neurol. 299, 122–136. doi: 10.1016/j.expneurol.2017.10.010
Sanchez, M. A., Sullivan, G. M., and Armstrong, R. C. (2018). Genetic detection of Sonic hedgehog (Shh) expression and cellular response in the progression of acute through chronic demyelination and remyelination. Neurobiol. Dis. 115, 145–156. doi: 10.1016/j.nbd.2018.04.003
Sheffield, I. D., McGee, M. A., Glenn, S. J., Baek, D. Y., Coleman, J. M., Dorius, B. K., et al. (2018). Osteoarthritis-Like Changes in Bardet-Biedl Syndrome Mutant Ciliopathy Mice (Bbs1(M390R/M390R)): evidence for a Role of Primary Cilia in Cartilage Homeostasis and Regulation of Inflammation. Front. Physiol. 9:708. doi: 10.3389/fphys.2018.00708
Sheng, G., Xu, X., Lin, Y. F., Wang, C. E., Rong, J., Cheng, D., et al. (2008). Huntingtin-associated protein 1 interacts with Ahi1 to regulate cerebellar and brainstem development in mice. J. Clin. Invest. 118, 2785–2795. doi: 10.1172/jci35339
Simi, A., Tsakiri, N., Wang, P., and Rothwell, N. J. (2007). Interleukin-1 and inflammatory neurodegeneration. Biochem. Soc. Trans. 35, 1122–1126.
Simons, C., Wolf, N. I., McNeil, N., Caldovic, L., Devaney, J. M., Takanohashi, A., et al. (2013). A de novo mutation in the beta-tubulin gene TUBB4A results in the leukoencephalopathy hypomyelination with atrophy of the basal ganglia and cerebellum. Am. J. Hum. Genet. 92, 767–773. doi: 10.1016/j.ajhg.2013.03.018
Singh, S. S., Rai, S. N., Birla, H., Zahra, W., Rathore, A. S., and Singh, S. P. (2020). NF-kappaB-Mediated Neuroinflammation in Parkinson’s Disease and Potential Therapeutic Effect of Polyphenols. Neurotox. Res. 37, 491–507. doi: 10.1007/s12640-019-00147-2
Sirko, S., Behrendt, G., Johansson, P. A., Tripathi, P., Costa, M., Bek, S., et al. (2013). Reactive glia in the injured brain acquire stem cell properties in response to sonic hedgehog. [corrected]. Cell Stem Cell 12, 426–439. doi: 10.1016/j.stem.2013.01.019
Smith, C. E. L., Lake, A. V. R., and Johnson, C. A. (2020). Primary Cilia, Ciliogenesis and the Actin Cytoskeleton: a Little Less Resorption, A Little More Actin Please. Front. Cell Dev. Biol. 8:622822. doi: 10.3389/fcell.2020.622822
Smith, R. (2004). Moving molecules: mRNA trafficking in Mammalian oligodendrocytes and neurons. Neuroscientist 10, 495–500. doi: 10.1177/1073858404266759
Sobu, Y., Wawro, P. S., Dhekne, H. S., Yeshaw, W. M., and Pfeffer, S. R. (2021). Pathogenic LRRK2 regulates ciliation probability upstream of tau tubulin kinase 2 via Rab10 and RILPL1 proteins. Proc. Natl. Acad. Sci. U. S. A. 118:e2005894118. doi: 10.1073/pnas.2005894118
Sofroniew, M. V. (2015). Astrocyte barriers to neurotoxic inflammation. Nat. Rev. Neurosci. 16, 249–263.
Song, C. J., Zimmerman, K. A., Henke, S. J., and Yoder, B. K. (2017). Inflammation and Fibrosis in Polycystic Kidney Disease. Results Probl. Cell Differ. 60, 323–344. doi: 10.1007/978-3-319-51436-9_12
Song, J., Goetz, B. D., Baas, P. W., and Duncan, I. D. (2001). Cytoskeletal reorganization during the formation of oligodendrocyte processes and branches. Mol. Cell. Neurosci. 17, 624–636. doi: 10.1006/mcne.2001.0974
Sorrentino, Z. A., Goodwin, M. S., Riffe, C. J., Dhillon, J. S., Xia, Y., Gorion, K. M., et al. (2019). Unique alpha-synuclein pathology within the amygdala in Lewy body dementia: implications for disease initiation and progression. Acta Neuropathol. Commun. 7:142.
Southwood, C. M., Peppi, M., Dryden, S., Tainsky, M. A., and Gow, A. (2007). Microtubule deacetylases, SirT2 and HDAC6, in the nervous system. Neurochem. Res. 32, 187–195. doi: 10.1007/s11064-006-9127-6
Spassky, N., Han, Y. G., Aguilar, A., Strehl, L., Besse, L., Laclef, C., et al. (2008). Primary cilia are required for cerebellar development and Shh-dependent expansion of progenitor pool. Dev. Biol. 317, 246–259. doi: 10.1016/j.ydbio.2008.02.026
Spiegel, I., and Peles, E. (2002). Cellular junctions of myelinated nerves (Review). Mol. Membr. Biol. 19, 95–101. doi: 10.1080/09687680210130009
Stadelmann, C., Timmler, S., Barrantes-Freer, A., and Simons, M. (2019). Myelin in the Central Nervous System: structure, Function, and Pathology. Physiol. Rev. 99, 1381–1431. doi: 10.1152/physrev.00031.2018
Stangel, M., Kuhlmann, T., Matthews, P. M., and Kilpatrick, T. J. (2017). Achievements and obstacles of remyelinating therapies in multiple sclerosis. Nat. Rev. Neurol. 13, 742–754. doi: 10.1038/nrneurol.2017.139
Steger, M., Diez, F., Dhekne, H. S., Lis, P., Nirujogi, R. S., Karayel, O., et al. (2017). Systematic proteomic analysis of LRRK2-mediated Rab GTPase phosphorylation establishes a connection to ciliogenesis. Elife 6:e31012.
Steger, M., Tonelli, F., Ito, G., Davies, P., Trost, M., Vetter, M., et al. (2016). Phosphoproteomics reveals that Parkinson’s disease kinase LRRK2 regulates a subset of Rab GTPases. Elife 5:e12813.
Sterpka, A., and Chen, X. (2018). Neuronal and astrocytic primary cilia in the mature brain. Pharmacol. Res. 137, 114–121. doi: 10.1016/j.phrs.2018.10.002
Sugiura, Y., and Lin, W. (2011). Neuron-glia interactions: the roles of Schwann cells in neuromuscular synapse formation and function. Biosci. Rep. 31, 295–302. doi: 10.1042/bsr20100107
Surmeier, D. J. (2018). Determinants of dopaminergic neuron loss in Parkinson’s disease. FEBS J. 285, 3657–3668. doi: 10.1111/febs.14607
Tang, J., Rutishauser, U., and Landmesser, L. (1994). Polysialic acid regulates growth cone behavior during sorting of motor axons in the plexus region. Neuron 13, 405–414.
Tang, Z., Lin, M. G., Stowe, T. R., Chen, S., Zhu, M., Stearns, T., et al. (2013). Autophagy promotes primary ciliogenesis by removing OFD1 from centriolar satellites. Nature 502, 254–257. doi: 10.1038/nature12606
Taveggia, C., Zanazzi, G., Petrylak, A., Yano, H., Rosenbluth, J., Einheber, S., et al. (2005). Neuregulin-1 type III determines the ensheathment fate of axons. Neuron 47, 681–694. doi: 10.1016/j.neuron.2005.08.017
Tawk, M., Makoukji, J., Belle, M., Fonte, C., Trousson, A., Hawkins, T., et al. (2011). Wnt/beta-catenin signaling is an essential and direct driver of myelin gene expression and myelinogenesis. J. Neurosci. 31, 3729–3742. doi: 10.1523/jneurosci.4270-10.2011
Thomas, A. M., Seidlits, S. K., Goodman, A. G., Kukushliev, T. V., Hassani, D. M., Cummings, B. J., et al. (2014). Sonic hedgehog and neurotrophin-3 increase oligodendrocyte numbers and myelination after spinal cord injury. Integr. Biol. 6, 694–705. doi: 10.1039/c4ib00009a
Tiane, A., Schepers, M., Rombaut, B., Hupperts, R., Prickaerts, J., Hellings, N., et al. (2019). From OPC to Oligodendrocyte: an Epigenetic Journey. Cells 8:1236. doi: 10.3390/cells8101236
Tischfield, M. A., Cederquist, G. Y., Gupta, M. L. Jr., and Engle, E. C. (2011). Phenotypic spectrum of the tubulin-related disorders and functional implications of disease-causing mutations. Curr. Opin. Genet. Dev. 21, 286–294. doi: 10.1016/j.gde.2011.01.003
Togel, M., Wiche, G., and Propst, F. (1998). Novel features of the light chain of microtubule-associated protein MAP1B: microtubule stabilization, self interaction, actin filament binding, and regulation by the heavy chain. J. Cell Biol. 143, 695–707. doi: 10.1083/jcb.143.3.695
Torii, T., Miyamoto, Y., and Yamauchi, J. (2019). Cellular Signal-Regulated Schwann Cell Myelination and Remyelination. Adv. Exp. Med. Biol. 1190, 3–22. doi: 10.1007/978-981-32-9636-7_1
Traiffort, E., Zakaria, M., Laouarem, Y., and Ferent, J. (2016). Hedgehog: a Key Signaling in the Development of the Oligodendrocyte Lineage. J. Dev. Biol. 4:28. doi: 10.3390/jdb4030028
Trinh, J., Amouri, R., Duda, J. E., Morley, J. F., Read, M., Donald, A., et al. (2014). Comparative study of Parkinson’s disease and leucine-rich repeat kinase 2 p.G2019S parkinsonism. Neurobiol. Aging 35, 1125–1131.
Ugbode, C. I., Smith, I., Whalley, B. J., Hirst, W. D., and Rattray, M. (2017). Sonic hedgehog signalling mediates astrocyte crosstalk with neurons to confer neuroprotection. J. Neurochem. 142, 429–443. doi: 10.1111/jnc.14064
Valdenaire, O., Giller, T., Breu, V., Ardati, A., Schweizer, A., and Richards, J. G. (1998). A new family of orphan G protein-coupled receptors predominantly expressed in the brain. FEBS Lett. 424, 193–196. doi: 10.1016/s0014-5793(98)00170-7
van Dam, T. J. P., Kennedy, J., van der Lee, R., de Vrieze, E., Wunderlich, K. A., and Rix, S. (2019). CiliaCarta: an integrated and validated compendium of ciliary genes. PLoS One 14:e0216705. doi: 10.1371/journal.pone.0216705
van der Knaap, M. S., Naidu, S., Pouwels, P. J., Bonavita, S., van Coster, R., Lagae, L., et al. (2002). New syndrome characterized by hypomyelination with atrophy of the basal ganglia and cerebellum. AJNR Am. J. Neuroradiol. 23, 1466–1474.
van Sloten, T. T., Savelberg, H. H., Duimel-Peeters, I. G., Meijer, K., Henry, R. M., Stehouwer, C. D., et al. (2011). Peripheral neuropathy, decreased muscle strength and obesity are strongly associated with walking in persons with type 2 diabetes without manifest mobility limitations. Diabetes Res. Clin. Pract. 91, 32–39. doi: 10.1016/j.diabres.2010.09.030
Vanderver, A., Prust, M., Tonduti, D., Mochel, F., Hussey, H. M., Helman, G., et al. (2015). Case definition and classification of leukodystrophies and leukoencephalopathies. Mol. Genet. Metab. 114, 494–500. doi: 10.1016/j.ymgme.2015.01.006
Vandevelde, M., and Zurbriggen, A. (2005). Demyelination in canine distemper virus infection: a review. Acta Neuropathol. 109, 56–68. doi: 10.1007/s00401-004-0958-4
Varea, E., Nacher, J., Blasco-Ibanez, J. M., Gomez-Climent, M. A., Castillo-Gomez, E., Crespo, C., et al. (2005). PSA-NCAM expression in the rat medial prefrontal cortex. Neuroscience 136, 435–443. doi: 10.1016/j.neuroscience.2005.08.009
Vorobyeva, A. G., and Saunders, A. J. (2018). Amyloid-beta interrupts canonical Sonic hedgehog signaling by distorting primary cilia structure. Cilia 7:5.
Wang, Z., Phan, T., and Storm, D. R. (2011). The type 3 adenylyl cyclase is required for novel object learning and extinction of contextual memory: role of cAMP signaling in primary cilia. J. Neurosci. 31, 5557–5561. doi: 10.1523/jneurosci.6561-10.2011
Wann, A. K., and Knight, M. M. (2012). Primary cilia elongation in response to interleukin-1 mediates the inflammatory response. Cell. Mol. Life Sci. 69, 2967–2977. doi: 10.1007/s00018-012-0980-y
Wann, A. K., Chapple, J. P., and Knight, M. M. (2014). The primary cilium influences interleukin-1beta-induced NFkappaB signalling by regulating IKK activity. Cell. Signal. 26, 1735–1742. doi: 10.1016/j.cellsig.2014.04.004
Wann, A. K., Zuo, N., Haycraft, C. J., Jensen, C. G., Poole, C. A., McGlashan, S. R., et al. (2012). Primary cilia mediate mechanotransduction through control of ATP-induced Ca2+ signaling in compressed chondrocytes. FASEB J. 26, 1663–1671. doi: 10.1096/fj.11-193649
Watanabe, R., Buschauer, R., Bohning, J., Audagnotto, M., Lasker, K., Lu, T. W., et al. (2020). The In Situ Structure of Parkinson’s Disease-Linked LRRK2. Cell 182, 1508–1518.e16.
Wei, Z., Fei, Y., Su, W., and Chen, G. (2019). Emerging Role of Schwann Cells in Neuropathic Pain: receptors, Glial Mediators and Myelination. Front. Cell. Neurosci. 13:116. doi: 10.3389/fncel.2019.00116
Weisman, G. A., Ajit, D., Garrad, R., Peterson, T. S., Woods, L. T., Thebeau, C., et al. (2012). Neuroprotective roles of the P2Y(2) receptor. Purinergic Signal. 8, 559–578.
Wheway, G., Nazlamova, L., and Hancock, J. T. (2018). Signaling through the Primary Cilium. Front. Cell Dev. Biol. 6:8. doi: 10.3389/fcell.2018.00008
Willison, H. J., and Yuki, N. (2002). Peripheral neuropathies and anti-glycolipid antibodies. Brain 125, 2591–2625.
Wilson, C. W., and Stainier, D. Y. (2010). Vertebrate Hedgehog signaling: cilia rule. BMC Biol. 8:102. doi: 10.1186/1741-7007-8-102
Wong, K., Ren, X. R., Huang, Y. Z., Xie, Y., Liu, G., Saito, H., et al. (2001). Signal transduction in neuronal migration: roles of GTPase activating proteins and the small GTPase Cdc42 in the Slit-Robo pathway. Cell 107, 209–221.
Wood, T. E., Barry, J., Yang, Z., Cepeda, C., Levine, M. S., and Gray, M. (2019). Mutant huntingtin reduction in astrocytes slows disease progression in the BACHD conditional Huntington’s disease mouse model. Hum. Mol. Genet. 28, 487–500.
Yamanaka, K., Chun, S. J., Boillee, S., Fujimori-Tonou, N., Yamashita, H., Gutmann, D. H., et al. (2008). Astrocytes as determinants of disease progression in inherited amyotrophic lateral sclerosis. Nat. Neurosci. 11, 251–253.
Yamauchi, J., Chan, J. R., and Shooter, E. M. (2004). Neurotrophins regulate Schwann cell migration by activating divergent signaling pathways dependent on Rho GTPases. Proc. Natl. Acad. Sci. U. S. A. 101, 8774–8779. doi: 10.1073/pnas.0402795101
Yan, S., Xuan, Z., Yang, M., Wang, C., Tao, T., Wang, Q., et al. (2020). CSB6B prevents beta-amyloid-associated neuroinflammation and cognitive impairments via inhibiting NF-kappaB and NLRP3 in microglia cells. Int. Immunopharmacol. 81:106263. doi: 10.1016/j.intimp.2020.106263
Ydens, E., Lornet, G., Smits, V., Goethals, S., Timmerman, V., and Janssens, S. (2013). The neuroinflammatory role of Schwann cells in disease. Neurobiol. Dis. 55, 95–103. doi: 10.1016/j.nbd.2013.03.005
Yokota, Y., Kim, W. Y., Chen, Y., Wang, X., Stanco, A., Komuro, Y., et al. (2009). The adenomatous polyposis coli protein is an essential regulator of radial glial polarity and construction of the cerebral cortex. Neuron 61, 42–56. doi: 10.1016/j.neuron.2008.10.053
Yoshimura, K., and Takeda, S. (2012). Hedgehog signaling regulates myelination in the peripheral nervous system through primary cilia. Differentiation 83, S78–S85.
Yoshimura, K., Kawate, T., and Takeda, S. (2011). Signaling through the primary cilium affects glial cell survival under a stressed environment. Glia 59, 333–344. doi: 10.1002/glia.21105
Yu, L., Guan, Y., Wu, X., Chen, Y., Liu, Z., Du, H., et al. (2013). Wnt Signaling is altered by spinal cord neuronal dysfunction in amyotrophic lateral sclerosis transgenic mice. Neurochem. Res. 38, 1904–1913. doi: 10.1007/s11064-013-1096-y
Yusifov, E., Dumoulin, A., and Stoeckli, E. T. (2021). Investigating Primary Cilia during Peripheral Nervous System Formation. Int. J. Mol. Sci. 22:3176. doi: 10.3390/ijms22063176
Zalenski, A. A., Majumder, S., De, K., and Venere, M. (2020). An interphase pool of KIF11 localizes at the basal bodies of primary cilia and a reduction in KIF11 expression alters cilia dynamics. Sci. Rep. 10:13946.
Zamani, E., Parviz, M., Roghani, M., Hosseini, M., Mohseni-Moghaddam, P., and Nikbakhtzadeh, M. (2020). Netrin-1 protects the SH-SY5Y cells against amyloid beta neurotoxicity through NF-kappaB/Nrf2 dependent mechanism. Mol. Biol. Rep. 47, 9271–9277.
Zhang, Y., Chen, K., Sloan, S. A., Bennett, M. L., Scholze, A. R., O’Keeffe, S., et al. (2014). An RNA-sequencing transcriptome and splicing database of glia, neurons, and vascular cells of the cerebral cortex. J. Neurosci. 34, 11929–11947. doi: 10.1523/jneurosci.1860-14.2014
Zhou, X., Fan, L. X., Li, K., Ramchandran, R., Calvet, J. P., and Li, X. (2014). SIRT2 regulates ciliogenesis and contributes to abnormal centrosome amplification caused by loss of polycystin-1. Hum. Mol. Genet. 23, 1644–1655. doi: 10.1093/hmg/ddt556
Zimprich, A., Biskup, S., Leitner, P., Lichtner, P., Farrer, M., Lincoln, S., et al. (2004). Mutations in LRRK2 cause autosomal-dominant parkinsonism with pleomorphic pathology. Neuron 44, 601–607. doi: 10.1016/j.neuron.2004.11.005
Keywords: glia, primary cilia, myelination, nerve regeneration, neurological disease
Citation: Ki SM, Jeong HS and Lee JE (2021) Primary Cilia in Glial Cells: An Oasis in the Journey to Overcoming Neurodegenerative Diseases. Front. Neurosci. 15:736888. doi: 10.3389/fnins.2021.736888
Received: 06 July 2021; Accepted: 31 August 2021;
Published: 30 September 2021.
Edited by:
Naiara Akizu, Children’s Hospital of Philadelphia, United StatesReviewed by:
Sofia Lizarraga, University of South Carolina, United StatesMarco Onorati, University of Pisa, Italy
Copyright © 2021 Ki, Jeong and Lee. This is an open-access article distributed under the terms of the Creative Commons Attribution License (CC BY). The use, distribution or reproduction in other forums is permitted, provided the original author(s) and the copyright owner(s) are credited and that the original publication in this journal is cited, in accordance with accepted academic practice. No use, distribution or reproduction is permitted which does not comply with these terms.
*Correspondence: Ji Eun Lee, amlldW4ubGVlQHNra3UuZWR1
†These authors have contributed equally to this work