- 1Walter and Eliza Hall Institute of Medical Research, Parkville, VIC, Australia
- 2Department of Medical Biology, University of Melbourne, Parkville, VIC, Australia
- 3Diamantina Institute, The University of Queensland, Woolloongabba, QLD, Australia
The Earth’s rotation around its axis, is one of the parameters that never changed since life emerged. Therefore, most of the organisms from the cyanobacteria to humans have conserved natural oscillations to regulate their physiology. These daily oscillations define the circadian rhythms that set the biological clock for almost all physiological processes of an organism. They allow the organisms to anticipate and respond behaviorally and physiologically to changes imposed by the day/night cycle. As other physiological systems, the immune system is also regulated by circadian rhythms and while diurnal variation in host immune responses to lethal infection have been observed for many decades, the underlying mechanisms that affect immune function and health have only just started to emerge. These oscillations are generated by the central clock in our brain, but neuroendocrine signals allow the synchronization of the clocks in peripheral tissues. In this review, we discuss how the neuroimmune interactions create a rhythmic activity of the innate lymphoid cells. We highlight how the disruption of these rhythmic regulations of immune cells can disturb homeostasis and lead to the development of chronic inflammation in murine models.
Introduction
The innate immune system is often seen as the first line of defense against invading pathogens, but it is now evident that they also carry out homeostatic functions by regulating essential pathways involved in tissue repair, mucosal barrier function, and metabolism. These functions have been particularly highlighted with the discovery of the innate lymphoid cells (ILCs) in early 2010’s (Vivier et al., 2018). In contrast to B and T lymphocytes, ILC activity is not modulated by antigen-specific receptors but by a dynamic integration of host-derived physiological signals (Seillet and Jacquelot, 2019). The ILC family comprises NK cells, ILC1, ILC2, and ILC3. Their constitutive activity at the body’s barrier surfaces ensures the maintenance of tissue homeostasis and immune protection (Vivier et al., 2018). ILC1 and NK cells are mainly involved in responses against intracellular pathogens and tumor surveillance (Seillet et al., 2020a). ILC2 initiate type-2 immune responses which are critical to allergy and anti-parasite responses (Fallon et al., 2006). They also mediate tissue repair through the secretion of amphiregulin (Monticelli et al., 2011). Enhanced ILC2 function in the lung is associated with asthma (Chang et al., 2011) while in adipose tissue, decreased ILC2 cytokine production is associated with obesity and insulin resistance (Molofsky et al., 2013). ILC3 are greatly enriched in the intestine where they mediate anti-bacterial responses (Sawa et al., 2011). They produce the interleukin (IL)-22 which promotes colonization of the gut by beneficial commensal bacteria that protect against intestinal inflammation (Pickard et al., 2014). Decreased ILC3 functions are associated with impaired capacity to maintain the mucosal barrier intact (Satoh-Takayama et al., 2014). Recent studies have shown that ILC responses are modulated by rhythmically expressed neuropeptides. These recent advances could contribute to the understanding of the mechanisms that leads to increased incidence of chronic inflammatory diseases when circadian rhythms are disrupted.
Circadian rhythms are endogenous oscillations with a period close to 24 h. They are found in almost all living organisms. The temporal alignment of the internal physiology with the external environment is critical for survival and the evolution of species. Circadian rhythms are found in virtually all cells of the body and function autonomously. However, these oscillations need to be synchronized with the environment, and external signals such as the light, temperature, and food intake (Reppert and Weaver, 2002). Therefore, our lifestyle, physical activity, and feeding times are important components for the robustness of these rhythms. Light sensed by the retina is connected to the suprachiasmatic nucleus (SCN) in the hypothalamus. The SCN is also known as the master clock and is responsible for entraining peripheral circadian clocks distributed across the organism. All peripheral clocks are synchronized daily and coordinated by the SCN via the hypothalamic pituitary adrenal (HPA) axis and the autonomic nervous system (ANS; Gnocchi and Bruscalupi, 2017; Figure 1). Food intake can be aligned to natural feeding rhythms induced by the SCN and contribute to synchronize the peripheral clocks. Food intake can also be desynchronized with the SCN due to environmental changes, such as food restriction or temporally altered behavior.
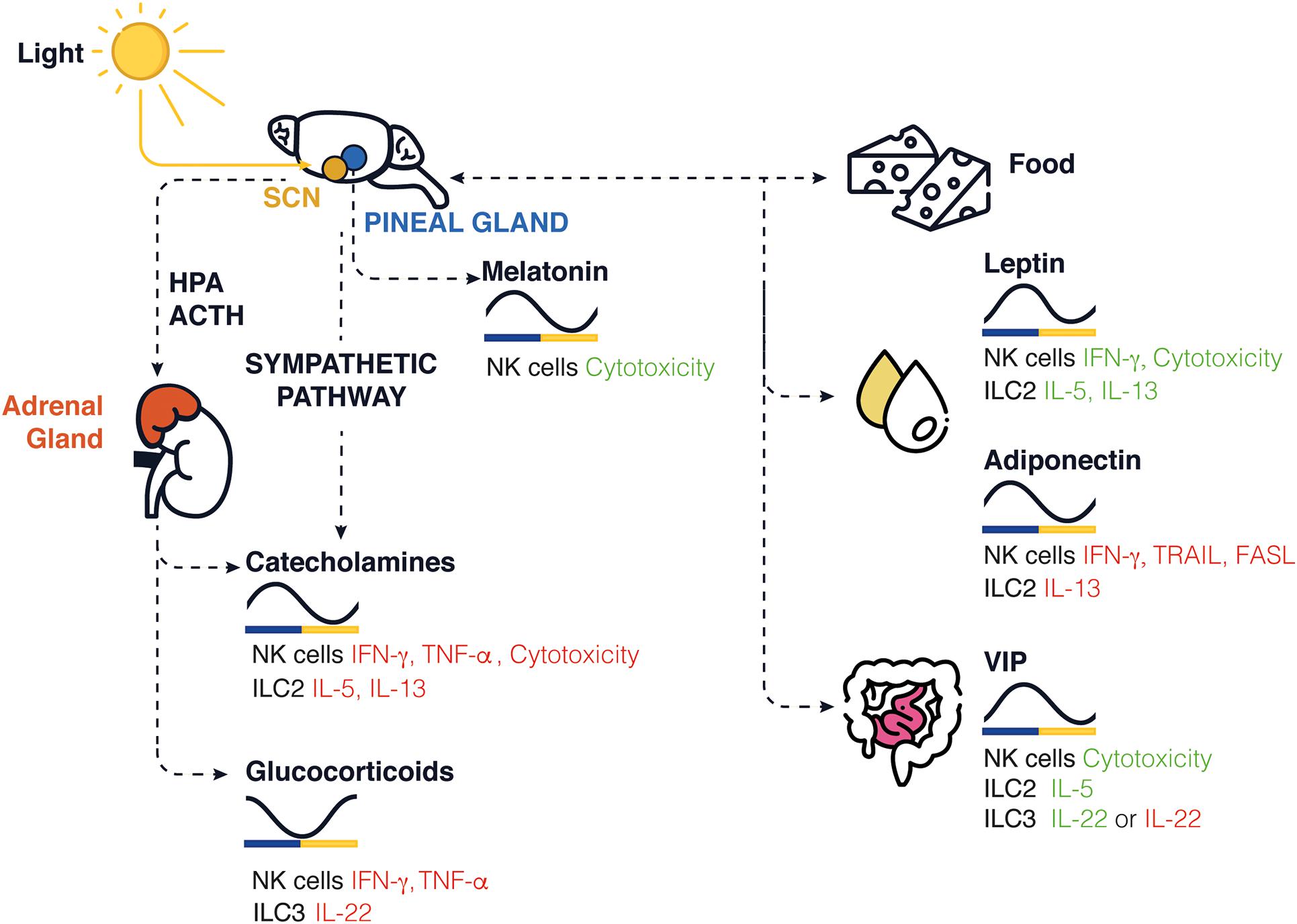
Figure 1. Schematic representation of circadian clock-mediated control of innate immune cells in rodents. In the brain, the SCN controls the rhythmic expression of GCs and catecholamines released in periphery, while the pineal gland controls the release of melatonin. The SCN controls the daily feeding/fasting (activity/rest) cycles, whereas food intake, stress hormone, sleep, and locomotor activity entrains and synchronizes peripheral clocks and the local release of neuropeptides such as leptin, adiponectin, and VIP. The levels found in nocturnal rodents for each molecule are shown across a 24-h period. Green/red represents an increase/decrease in cytokine production or cytotoxicity function.
Circadian rhythms are generated in SCN neurons using transcriptional feedback loops that take 24 h to complete. In mammals, the main loop is initiated by the transcription factors CLOCK and BMAL1 (Reppert and Weaver, 2002). These proteins induce the expression of period genes (Per1, Per2, and Per3) and cryptochrome genes (Cry1 and Cry2) that will then inhibit the expression of CLOCK and BMAL1 genes (Xu et al., 2015). This core feedback loop is modulated by additional transcription factors involving Rev-Erbα and the retinoic acid receptor (RAR)-related orphan receptor (ROR) family that ensures the rhythmic expression of BMAL1 (Sato et al., 2004; Yanofsky et al., 2013). Finally, the D-box binding protein (DBP) can activate BMAL1 while Nfil3 will inhibit the expression of clock genes (Mitsui et al., 2001). These transcription factors not only regulate the expression of their own inhibitors but also drive the rhythmic accumulation of target genes also known as clock-controlled genes (Zhang et al., 2014).
In addition to this molecular regulation, the central clock acts as a pacemaker that temporally aligns the peripheral clocks through neural outputs from the ANS, glucocorticoid (GC) hormones and a variety of neuropeptides released by nerves within tissues. While the regulation of ILC by the circadian molecular clock genes have been recently reviewed elsewhere (Wang and Colonna, 2020), we will highlight how the nervous and immune systems interact together. We will discuss the impact of neurohormones on immune cell activity and how they can potentially rhythmically modulate ILC responses.
Sympathetic Autonomous Nervous System and the Hypothalamic–Pituitary–Adrenal Axis
Adrenergic System
The adrenergic system is regulated by the sympathetic nervous system (SNS), through the production of catecholamines [epinephrine (EP), norepinephrine (NE)], and via HPA axis. Circulating catecholamine levels exhibit circadian rhythmicity, with EP levels typically rising during the day and falling at night in humans (Linsell et al., 1985) and in an opposite way in rats (De Boer and Van der Gugten, 1987; Schiffer et al., 2001) while data in mice are lacking. EP and NE engage β1- and β2-adrenergic receptors (β2AR), which are commonly found on bronchial smooth and cardiac muscles. Modulating β-adrenergic receptor signaling is, therefore, a common pharmacological strategy used for treatment of asthma (Sears et al., 1990) and cardiovascular conditions (Milano et al., 1994).
The activation of β2AR in human NK cell line inhibits TNF-α, IFN-γ, granzyme B, and perforin expressions (Sun et al., 2018). In humans, EP induces the mobilization of cytotoxic lymphocytes including the NK cells in the circulation (Dimitrov et al., 2010) which could explain the increased number of circulating NK cells during the active period when EP peaks (Dimitrov et al., 2007). In mice, adrenergic signaling inhibits the cytotoxic activity of the NK cells (De Lorenzo et al., 2015), controls NK cell expansion during viral infection (Diaz-Salazar et al., 2020), and inhibits IFN-γ production in hepatic NK cells (Wieduwild et al., 2020). This inhibition was not observed in ILC1 in the liver or splenic NK cells, suggesting a tissue and cell-specific control. In the absence of β2AR signaling, liver NK cells had higher IFN-γ production that resulted in increased resistance to infections associated with better control of viral replication and reduced tissue damage (Wieduwild et al., 2020). A rhythmic expression of TNF-α, IFN-γ, and granzyme B has been observed in NK cells (Logan et al., 2011). Logan et al. reported that levels of NE peaked in the morning in the spleen of rats, and found that the levels of TNF-α, IFN-γ, and granzyme B expression was inhibited during the light phase (resting phase in rodents), while during dark phase, when levels of norepinephrine are reduced, their transcripts were increased (Logan et al., 2011). It is interesting to note that in rats, peak of NE in the spleen is different from the EP level found in the blood, peaking during the active period at night (De Boer and Van der Gugten, 1987; Schiffer et al., 2001). This highlights how neurotransmitters can locally modulate the activity of immune cells. Following splenic sympathectomy, oscillation of granzyme-B, and TNF-α expression in NK cells are abolished, demonstrating the role of the SNS in the entrainment of their rhythmic expression in these cells. This circadian regulation of NK cells by adrenergic signaling could explain the reduced cytotoxicity of NK cells in animals under chronic shift-lag (Logan et al., 2012) and sleep deprivation (De Lorenzo et al., 2015).
Norepinephrine also inhibits type 2 immune responses by impairing ILC2 proliferation and function through its binding to β2AR (Moriyama et al., 2018). After infection with N. brasiliensis, β2AR-deficient mice have increased intestinal ILC2 infiltration and ILC2-derived IL-13 production. Thus, β2AR acts as a molecular rheostat to control innate immune responses to prevent excessive tissue damage and the development of chronic inflammation (Moriyama et al., 2018). However, a direct link between β2AR signaling and the circadian regulation of ILC2 remains to be established.
Glucocorticoids
Adrenal glands have a key role in synchronizing peripheral clocks downstream of the SCN through the rhythmic secretion of GC. The GC are steroid hormones and their concentrations in the blood oscillate in a circadian manner, peaking in the morning and nadir at night, in diurnal animals. GC can also be released in pulsatile rhythm. While the circadian expression of GC relies on the SCN and the HPA axis (Spiga et al., 2014), the ultradian rhythm occurs independently of SCN (Waite et al., 2012). GC can regulate the expression of clock genes (Balsalobre et al., 2000). GC-responsive elements are found in Per1/2 genes and Rev-erbα and Rorα are negatively regulated by GC in mice (Surjit et al., 2011).
The GC are well known immunosuppressors of the innate immune responses and are widely used in clinics to treat chronic inflammatory disorders. They have been shown to inhibit the synthesis of various cytokines including TNF-α, IL-1β, IL-2, IL-4, IL-5, IL-6, IL-12, GM-CSF, and IFN-γ in both human and mice (Brattsand and Linden, 1996; Bhattacharyya et al., 2007; Li et al., 2015; Quatrini et al., 2018). GC limits the inflammation and therefore prevents tissue damages. Oscillations in GC concentrations directly impact the rhythmic regulation of the expression of these pro-inflammatory cytokines. Consequently, GC are partially responsible for the observed circadian variations of inflammatory symptoms that suffer patients with asthma and rheumatoid arthritis and many of whom have worsening of symptoms in early morning (Petty, 1988; Cutolo et al., 2003).
In mice, IL-7R expression is regulated by GR in a diurnal rhythm manner and has been shown to promote T lymphocyte survival and recruitment in lymph nodes (Shimba et al., 2018). ILC are strongly dependent on IL-7R signaling for their development and survival but it is still unclear whether GC similarly regulate IL-7R signaling on ILC in a circadian manner. In NK cells, GR deficiency promotes IFN-γ production, reducing mouse survival in response to toxoplasma and mouse cytomegalovirus infections (Quatrini et al., 2018). Similarly, GCs inhibit murine and human ILC3 function as reduced IL-22 production was observed when ILC3 were stimulated with the steroid hormones (Seshadri et al., 2018).
Interestingly, in patients with adrenal insufficiency who require lifelong GC replacement, the circadian administration of GC can partially restore the number of NK cells in circulation (Venneri et al., 2018), while acute administration of cortisol has no effect (Olnes et al., 2016). This suggesting that the timing of GC expression is important to regulate NK cell trafficking.
Melatonin
At night, the SCN acts on the pineal gland to induce the synthesis of melatonin. Consequently, high plasmatic levels are found in the middle of the night and minimal during the day in mice and humans. Melatonin production is not restricted to the pineal gland, but can also be secreted by the retina, kidneys and the digestive tract in humans (Messner et al., 2001), however, most of the mouse strains do not produce significant amounts of melatonin (Kennaway, 2019). We therefore need to be careful when translating mouse studies to humans. This neurohormone mediates its effects through specific membrane receptors, named melatonin-1 receptors (MT1), MT2, and MT3. In the absence of the pineal gland, the murine NK cell cytotoxic function is decreased. Surprisingly, a single injection of melatonin is able to restore NK cell activity but not when melatonin is administered chronically for 9 days (del Gobbo et al., 1989). Studies have shown a time-dependent influence of melatonin as in vivo administration of melatonin induces a significant increase of murine NK cells in spleen and bone marrow (Currier et al., 2000). In pinealectomized rats, the frequency of NK cells in the blood and spleen is increased during the day compared to sham controls (McNulty et al., 1990). Human peripheral lymphocytes cultured in the presence of melatonin show an inhibition of NK cell activity (Lewinski et al., 1989), but chronic administration of melatonin augmented the cytolytic activity and the circulating number of NK cells (Angeli et al., 1988).
Neuropeptidergic Pathways
Vasoactive Intestinal Peptide
The vasoactive intestinal peptide (VIP) is a neurotransmitter expressed in neurons found in brain and peripheral tissues such as the lung and gut. In the SCN, VIP is essential for the normal circadian rhythmicity in clock neurons (Aton et al., 2005; Todd et al., 2020) and can induce the expression of PER1/2 (Hamnett et al., 2019). In tissues, VIP is a potent vasodilator but is also involved in other physiological processes, including coordination of gastrointestinal motility, mucus, and enzymatic secretions in response to feeding (Aton et al., 2005; Todd et al., 2020). In mice, food intake induces the release of VIP from enteric neurons creating a rhythmic expression of VIP in the gut (Talbot et al., 2020; Seillet et al., 2020b). The release of VIP in gut and lungs stimulates ILC2 through VIP receptor type 2 (VIPR2) to induce IL-5 production (Nussbaum et al., 2013). The cyclic release of VIP in response to feeding induces a rhythmic production of IL-5 by ILC2s. This circadian expression of IL-5 is detectable in the blood circulation and appears to regulate systemic eosinophil numbers (Nussbaum et al., 2013). In the intestine, VIP-VIPR2 signaling regulates ILC3-derived IL-22 expression (Talbot et al., 2020; Seillet et al., 2020b). While we observed a positive effect of VIP on IL-22 secretion (Seillet et al., 2020b), Talbot et al. (2020) made opposite observations. Despite these, yet unresolved, discrepancies, both studies demonstrated the importance of circadian regulations for ILC function to maintain intestinal homeostasis. While we showed that VIP signaling protects against exacerbated gut inflammation, Talbot and colleagues found that the inhibition of IL-22 by VIP in ILC3 allows the optimal absorption of nutrients (Talbot et al., 2020).
Additional studies have revealed that VIP increased human NK cell cytotoxic function after viral infection (Azzari et al., 1992) and polarized T cell responses by regulating dendritic cells functions. Collectively, these data suggest that VIP can differentially promote inflammation or its resolution in a circadian dependent manner by promoting anti-inflammatory type 2 immune responses, and preventing Th1 infiltration in inflammatory sites.
Adiponectin
The adiponectin is exclusively secreted by adipocytes, and regulates body energy homeostasis, lipid storage, and adipogenesis (Stern et al., 2016). Its expression is controlled by the clock machinery and peaks at the onset of the feeding phase in mice (Barnea et al., 2015) or early/late morning for human (Gomez-Abellan et al., 2010). Adiponectin induces hypothalamic and peripheral clock genes expression and enhances food intake (Hashinaga et al., 2013; Tsang et al., 2020) as adiponectin-deficient mice have reduced Bmal1 and Per2 expressions and reduce food intake during the dark phase (active phase) but experience increase food intake during light phase (resting phase; Tsang et al., 2020). In contrast, overexpression of the adipokine in the liver induces local expression of the clock genes Bmal1, Dbp, Cry2, and Per2 (Hashinaga et al., 2013), indicating a direct effect of this adipokine on the cell-intrinsic circadian rhythm.
The adiponectin differentially impacts immune cells and can trigger both pro- and anti-inflammatory responses (Luo and Liu, 2016). High levels of adiponectin in mice result in an alteration of the adipose tissue immune cell composition, and a shift operates from a pro- to an anti-inflammatory immune profile leading to an improvement of insulin resistance in models of type 2 diabetes (Kim et al., 2007). NK cells also play a critical role in murine adipose tissue homeostasis, fine-tuning macrophage functions, and dysregulated NK cells are found in obesity (O’Shea and Hogan, 2019; Ferno et al., 2020). Both murine and human NK cells express the adiponectin receptors (Wilk et al., 2013; Jasinski-Bergner et al., 2017). Human NK cells stimulated with various TLR ligands and treated with adiponectin showed reduced IFN-γ production and degranulation capacities (Wilk et al., 2013). Conversely, in adiponectin-deficient mice, while an accumulation of mature NK cells (CD27lowCD11bhi) is found in the spleen, impaired NK cell degranulation and cytotoxicity are observed and are associated with decreased expression of the activating ligand NKG2D (Wilk et al., 2013). The addition of adiponectin to IL-2 stimulated NK cells leads to impaired cytotoxicity associated with reduced surface expression of FasL and TRAIL, and IFN-γ production (Kim et al., 2006). Recently, adiponectin was shown to suppress ILC2 proliferation and cytokine production and to decrease IL-33-driven ILC2 activation, thus acting as a negative regulator of ILC2 function in adipose tissue (Wang et al., 2021). While no direct link has demonstrated an influence of adiponectin on ILC function in a circadian manner, indirect evidence would suggest a rhythmically regulation of NK cell function by this adipokine. Indeed, adiponectin controls the expression of clock genes which are known to directly influence NK cell activity in rodents (Arjona and Sarkar, 2005, 2006, 2008). The disruption of the Per2 or Bmal1 in NK cells reduced IFN-γ, TNF-α, granzyme B, and perforin expressions (Liu et al., 2006; Arjona and Sarkar, 2008). Furthermore, murine NK cell cytotoxic function peaks during the active phase (Arjona et al., 2004; Arjona and Sarkar, 2005, 2006) which coincides with feeding time and higher levels of adiponectin (Barnea et al., 2015; Tsang et al., 2020), however, this interplay remains to be confirmed.
Further studies are warranted to ascertain the role and function of adiponectin on ILC subsets at steady state, over the course of metabolic syndromes, and during circadian misalignment.
Leptin
Leptin is mainly secreted by adipocytes and follows diurnal variations. In both humans and rodents, the leptin plasma levels peak at night before progressively decreasing, reaching a nadir during the day (Langendonk et al., 1998; Gavrila et al., 2003; Bodosi et al., 2004; Arble et al., 2011). In rodents, starvation induced a decrease in leptin levels and timed-restricted food availability inverted leptin plasma concentrations (Ahima et al., 1996; Bodosi et al., 2004). Furthermore, in humans, circadian misalignment decreases the leptin plasma levels compared to normal alignment (Scheer et al., 2009). Circadian disruptions through thermal lesions of the hypothalamic SCN or in Cry–/– and Per–/– deficient mice completely abolish the diurnal variation of leptin plasma levels (Kalsbeek et al., 2001; Kettner et al., 2015), indicating that circadian clocks control the rhythmic oscillation of the leptin plasma, independently of external food cues, potentially through direct regulation of gene expression. Indeed, the heterodimer BMAL1:CLOCK is capable of binding to the promoter of the leptin gene following a circadian rhythm and regulating C/EBP-α mediated leptin transcription (Kettner et al., 2015). Finally, chronic circadian disruption promotes leptin resistance in murine CNS (Kettner et al., 2015) which is known to be associated with obesity (Flier, 2004).
Mice lacking leptin (ob/ob) or its receptor (db/db) expression show immune deficiencies suggesting a direct role of the leptin signaling on the immune system (Bennett et al., 1996; Lord et al., 1998; Howard et al., 1999; Sanchez-Margalet et al., 2003; De Rosa et al., 2007). Both murine and human NK cells express variable levels of the short and long forms of the leptin receptor (Zhao et al., 2003; Lamas et al., 2013; Laue et al., 2015; Keustermans et al., 2017; Bahr et al., 2018). Particularly, obese patients who experience high leptin plasma levels have impaired NK cell phenotype and function, a reversible compromised state when there is a diminution of leptin plasma levels-associated with fat mass reduction (Jahn et al., 2015; Laue et al., 2015; Bahr et al., 2018). In leptin receptor deficient db/db mice, NK cell numbers in blood, spleen, liver and lungs are all reduced and cells have impaired cytolytic capacities compared to wild type control animals (Tian et al., 2002). In addition, leptin signaling-deficient mice injected with B16 melanoma or LLC cells have increased number of lung metastases compared to control mice (Mori et al., 2006). Stimulation of human NK cells with leptin promotes NK cell metabolism, proliferation, and cytotoxic functions (Zhao et al., 2003; Wrann et al., 2012; Lamas et al., 2013). Interestingly, while short term exposure of NK cells to physiological doses of leptin stimulates NK cells, long-term stimulation inhibits NK cell cytotoxicity and cytokine production (Wrann et al., 2012). Thus, dependent on the levels and duration of the stimulation, leptin may differently influence immune cell responses. The impact of leptin on other ILC subsets is only beginning to emerge. Leptin enhances type-2 responses in allergic rhinitis and stimulates ILC2 proliferation and IL-13 production in both humans and mice (Zheng et al., 2016; Wen et al., 2020; Zeng et al., 2020). Given the role of ILC2 on adipose tissue homeostasis and allergy reactions, leptin and other adiponectin would certainly modulate widely the function of these innate immune cells in health and diseases. Thus, further studies are warranted to delineate the role of these hormones on non-NK cell ILC.
Concluding Remarks
In our modern societies, the prevalence of circadian rhythm disruption is rising due to our lifestyle or might be imposed by extending working hours and night shift. Nonetheless, circadian rhythm disruption is yet to be recognized as a major public health issue; but accumulating evidence shows that circadian rhythms are an important part of our healing process and control inflammation. As medicine is evolving toward a more personalized approach, circadian regulation of immune responses will be an aspect to consider. Studying circadian rhythms at a global physiological lens will help us to understand how alteration of our natural rhythms by environmental cues or our behavior will impact the metabolism, hormonal signaling and immune function. It is crucial to increase our understanding of the circadian patterns of immune responses and how they are regulated by central and peripheral clocks to enable discovery of chronotherapeutic approaches for optimal timing of therapy administration toward effective measures for treating inflammatory diseases, allergies, and infections. Because animal studies are used to reduce the complexity of parameters influencing circadian rhythms (e.g., temperature, food availability, and external stressor), it will be important to optimize the experimental setups, including the control of external circadian rhythm perturbations (such as, manipulation of light-dark cycles, sleep restriction, or time-restricted feeding) to develop the best translational approaches.
Author Contributions
All authors listed have made a substantial, direct and intellectual contribution to the work, and approved it for publication.
Funding
This work was supported by grants and fellowships from the National Health and Medical Research Council (NHMRC) of Australia (CS, GNT1098832; CS and GTB, GNT1165443; GTB, GNT1135898 and GNT2002265), Australian Research Career development Fellowship (CS; APP1123000). This work was made possible through Victorian State Government Operational Infrastructure Support and Australian Government NHMRC IRIIS.
Conflict of Interest
The authors declare that the research was conducted in the absence of any commercial or financial relationships that could be construed as a potential conflict of interest.
Acknowledgments
The authors apologize to all investigators whose works were not cited in this article due to space limitations.
References
Ahima, R. S., Prabakaran, D., Mantzoros, C., Qu, D., Lowell, B., Maratos-Flier, E., et al. (1996). Role of leptin in the neuroendocrine response to fasting. Nature 382, 250–252. doi: 10.1038/382250a0
Angeli, A., Gatti, G., Satori, M. L., Del Ponte, D., and Carignola, R. (1988). “Effect of exogenous melatonin on human natural killer (NK) cell activity. An approach to the immunomodulatory role of the pineal gland,” in The Pineal Gland and Cancer, eds D. Gupta, A. Attanasio, and R. J. Reiter (Tübingen: Muller and Bass), 145–156.
Arble, D. M., Vitaterna, M. H., and Turek, F. W. (2011). Rhythmic leptin is required for weight gain from circadian desynchronized feeding in the mouse. PLoS One 6:e25079. doi: 10.1371/journal.pone.0025079
Arjona, A., Boyadjieva, N., and Sarkar, D. K. (2004). Circadian rhythms of granzyme B, perforin, IFN-gamma, and NK cell cytolytic activity in the spleen: effects of chronic ethanol. J. Immunol. 172, 2811–2817. doi: 10.4049/jimmunol.172.5.2811
Arjona, A., and Sarkar, D. K. (2005). Circadian oscillations of clock genes, cytolytic factors, and cytokines in rat NK cells. J. Immunol. 174, 7618–7624. doi: 10.4049/jimmunol.174.12.7618
Arjona, A., and Sarkar, D. K. (2006). Evidence supporting a circadian control of natural killer cell function. Brain Behav. Immun. 20, 469–476. doi: 10.1016/j.bbi.2005.10.002
Arjona, A., and Sarkar, D. K. (2008). Are circadian rhythms the code of hypothalamic-immune communication? Insights from natural killer cells. Neurochem. Res. 33, 708–718. doi: 10.1007/s11064-007-9501-z
Aton, S. J., Colwell, C. S., Harmar, A. J., Waschek, J., and Herzog, E. D. (2005). Vasoactive intestinal polypeptide mediates circadian rhythmicity and synchrony in mammalian clock neurons. Nat. Neurosci. 8, 476–483. doi: 10.1038/nn1419
Azzari, C., Rossi, M. E., Resti, M., Caldini, A. L., Carbonella, R., Ciappi, S., et al. (1992). VIP restores natural killer cell activity depressed by hepatitis B surface antigen. Viral Immunol. 5, 195–200. doi: 10.1089/vim.1992.5.195
Bahr, I., Jahn, J., Zipprich, A., Pahlow, I., Spielmann, J., and Kielstein, H. (2018). Impaired natural killer cell subset phenotypes in human obesity. Immunol. Res. 66, 234–244. doi: 10.1007/s12026-018-8989-4
Balsalobre, A., Brown, S. A., Marcacci, L., Tronche, F., Kellendonk, C., Reichardt, H. M., et al. (2000). Resetting of circadian time in peripheral tissues by glucocorticoid signaling. Science 289, 2344–2347. doi: 10.1126/science.289.5488.2344
Barnea, M., Chapnik, N., Genzer, Y., and Froy, O. (2015). The circadian clock machinery controls adiponectin expression. Mol. Cell. Endocrinol. 399, 284–287. doi: 10.1016/j.mce.2014.10.018
Bennett, B. D., Solar, G. P., Yuan, J. Q., Mathias, J., Thomas, G. R., and Matthews, W. (1996). A role for leptin and its cognate receptor in hematopoiesis. Curr. Biol. 6, 1170–1180. doi: 10.1016/s0960-9822(02)70684-2
Bhattacharyya, S., Brown, D. E., Brewer, J. A., Vogt, S. K., and Muglia, L. J. (2007). Macrophage glucocorticoid receptors regulate Toll-like receptor 4-mediated inflammatory responses by selective inhibition of p38 MAP kinase. Blood 109, 4313–4319. doi: 10.1182/blood-2006-10-048215
Bodosi, B., Gardi, J., Hajdu, I., Szentirmai, E., Obal, F. Jr., and Krueger, J. M. (2004). Rhythms of ghrelin, leptin, and sleep in rats: effects of the normal diurnal cycle, restricted feeding, and sleep deprivation. Am. J. Physiol. Regul. Integr. Comp. Physiol. 287, R1071–R1079.
Brattsand, R., and Linden, M. (1996). Cytokine modulation by glucocorticoids: mechanisms and actions in cellular studies. Aliment. Pharmacol. Ther. 10(Suppl. 2), 81–90; discussion 91–82.
Chang, Y. J., Kim, H. Y., Albacker, L. A., Baumgarth, N., McKenzie, A. N., Smith, D. E., et al. (2011). Innate lymphoid cells mediate influenza-induced airway hyper-reactivity independently of adaptive immunity. Nat. Immunol. 12, 631–638. doi: 10.1038/ni.2045
Currier, N. L., Sun, L. Z., and Miller, S. C. (2000). Exogenous melatonin: quantitative enhancement in vivo of cells mediating non-specific immunity. J. Neuroimmunol. 104, 101–108. doi: 10.1016/s0165-5728(99)00271-4
Cutolo, M., Seriolo, B., Craviotto, C., Pizzorni, C., and Sulli, A. (2003). Circadian rhythms in RA. Ann. Rheum. Dis. 62, 593–596.
De Boer, S. F., and Van der Gugten, J. (1987). Daily variations in plasma noradrenaline, adrenaline and corticosterone concentrations in rats. Physiol. Behav. 40, 323–328. doi: 10.1016/0031-9384(87)90054-0
De Lorenzo, B. H., de Oliveira Marchioro, L., Greco, C. R., and Suchecki, D. (2015). Sleep-deprivation reduces NK cell number and function mediated by beta-adrenergic signalling. Psychoneuroendocrinology 57, 134–143. doi: 10.1016/j.psyneuen.2015.04.006
De Rosa, V., Procaccini, C., Cali, G., Pirozzi, G., Fontana, S., Zappacosta, S., et al. (2007). A key role of leptin in the control of regulatory T cell proliferation. Immunity 26, 241–255. doi: 10.1016/j.immuni.2007.01.011
del Gobbo, V., Libri, V., Villani, N., Calio, R., and Nistico, G. (1989). Pinealectomy inhibits interleukin-2 production and natural killer activity in mice. Int. J. Immunopharmacol. 11, 567–573. doi: 10.1016/0192-0561(89)90187-2
Diaz-Salazar, C., Bou-Puerto, R., Mujal, A. M., Lau, C. M., von Hoesslin, M., Zehn, D., et al. (2020). Cell-intrinsic adrenergic signaling controls the adaptive NK cell response to viral infection. J. Exp. Med. 217, e20190549.
Dimitrov, S., Lange, T., and Born, J. (2010). Selective mobilization of cytotoxic leukocytes by epinephrine. J. Immunol. 184, 503–511. doi: 10.4049/jimmunol.0902189
Dimitrov, S., Lange, T., Nohroudi, K., and Born, J. (2007). Number and function of circulating human antigen presenting cells regulated by sleep. Sleep 30, 401–411. doi: 10.1093/sleep/30.4.401
Fallon, P. G., Ballantyne, S. J., Mangan, N. E., Barlow, J. L., Dasvarma, A., Hewett, D. R., et al. (2006). Identification of an interleukin (IL)-25-dependent cell population that provides IL-4, IL-5, and IL-13 at the onset of helminth expulsion. J. Exp. Med. 203, 1105–1116. doi: 10.1084/jem.20051615
Ferno, J., Strand, K., Mellgren, G., Stiglund, N., and Bjorkstrom, N. K. (2020). Natural killer cells as sensors of adipose tissue stress. Trends Endocrinol. Metab. 31, 3–12. doi: 10.1016/j.tem.2019.08.011
Flier, J. S. (2004). Obesity wars: molecular progress confronts an expanding epidemic. Cell 116, 337–350.
Gavrila, A., Peng, C. K., Chan, J. L., Mietus, J. E., Goldberger, A. L., and Mantzoros, C. S. (2003). Diurnal and ultradian dynamics of serum adiponectin in healthy men: comparison with leptin, circulating soluble leptin receptor, and cortisol patterns. J. Clin. Endocrinol. Metab. 88, 2838–2843. doi: 10.1210/jc.2002-021721
Gnocchi, D., and Bruscalupi, G. (2017). Circadian rhythms and hormonal homeostasis: pathophysiological implications. Biology (Basel) 6:8.
Gomez-Abellan, P., Gomez-Santos, C., Madrid, J. A., Milagro, F. I., Campion, J., Martinez, J. A., et al. (2010). Circadian expression of adiponectin and its receptors in human adipose tissue. Endocrinology 151, 115–122. doi: 10.1210/en.2009-0647
Hamnett, R., Crosby, P., Chesham, J. E., and Hastings, M. H. (2019). Vasoactive intestinal peptide controls the suprachiasmatic circadian clock network via ERK1/2 and DUSP4 signalling. Nat. Commun. 10:542.
Hashinaga, T., Wada, N., Otabe, S., Yuan, X., Kurita, Y., Kakino, S., et al. (2013). Modulation by adiponectin of circadian clock rhythmicity in model mice for metabolic syndrome. Endocr. J. 60, 483–492.
Howard, J. K., Lord, G. M., Matarese, G., Vendetti, S., Ghatei, M. A., Ritter, M. A., et al. (1999). Leptin protects mice from starvation-induced lymphoid atrophy and increases thymic cellularity in ob/ob mice. J. Clin. Invest. 104, 1051–1059. doi: 10.1172/jci6762
Jahn, J., Spielau, M., Brandsch, C., Stangl, G. I., Delank, K. S., Bahr, I., et al. (2015). Decreased NK cell functions in obesity can be reactivated by fat mass reduction. Obesity (Silver Spring) 23, 2233–2241. doi: 10.1002/oby.21229
Jasinski-Bergner, S., Buttner, M., Quandt, D., Seliger, B., and Kielstein, H. (2017). Adiponectin and its receptors are differentially expressed in human tissues and cell lines of distinct origin. Obes. Facts 10, 569–583. doi: 10.1159/000481732
Kalsbeek, A., Fliers, E., Romijn, J. A., La Fleur, S. E., Wortel, J., Bakker, O., et al. (2001). The suprachiasmatic nucleus generates the diurnal changes in plasma leptin levels. Endocrinology 142, 2677–2685. doi: 10.1210/endo.142.6.8197
Kennaway, D. J. (2019). Melatonin research in mice: a review. Chronobiol. Int. 36, 1167–1183. doi: 10.1080/07420528.2019.1624373
Kettner, N. M., Mayo, S. A., Hua, J., Lee, C., Moore, D. D., and Fu, L. (2015). Circadian dysfunction induces leptin resistance in mice. Cell Metab. 22, 448–459. doi: 10.1016/j.cmet.2015.06.005
Keustermans, G., van der Heijden, L. B., Boer, B., Scholman, R., Nuboer, R., Pasterkamp, G., et al. (2017). Differential adipokine receptor expression on circulating leukocyte subsets in lean and obese children. PLoS One 12:e0187068. doi: 10.1371/journal.pone.0187068
Kim, J. Y., van de Wall, E., Laplante, M., Azzara, A., Trujillo, M. E., Hofmann, S. M., et al. (2007). Obesity-associated improvements in metabolic profile through expansion of adipose tissue. J. Clin. Invest. 117, 2621–2637. doi: 10.1172/jci31021
Kim, K. Y., Kim, J. K., Han, S. H., Lim, J. S., Kim, K. I., Cho, D. H., et al. (2006). Adiponectin is a negative regulator of NK cell cytotoxicity. J. Immunol. 176, 5958–5964. doi: 10.4049/jimmunol.176.10.5958
Lamas, B., Goncalves-Mendes, N., Nachat-Kappes, R., Rossary, A., Caldefie-Chezet, F., Vasson, M. P., et al. (2013). Leptin modulates dose-dependently the metabolic and cytolytic activities of NK-92 cells. J. Cell. Physiol. 228, 1202–1209. doi: 10.1002/jcp.24273
Langendonk, J. G., Pijl, H., Toornvliet, A. C., Burggraaf, J., Frolich, M., Schoemaker, R. C., et al. (1998). Circadian rhythm of plasma leptin levels in upper and lower body obese women: influence of body fat distribution and weight loss. J. Clin. Endocrinol. Metab. 83, 1706–1712. doi: 10.1210/jcem.83.5.4717
Laue, T., Wrann, C. D., Hoffmann-Castendiek, B., Pietsch, D., Hubner, L., and Kielstein, H. (2015). Altered NK cell function in obese healthy humans. BMC Obes 2:1. doi: 10.1186/s40608-014-0033-1
Lewinski, A., Zelazowski, P., Sewerynek, E., Zerek-Melen, G., Szkudlinski, M., and Zelazowska, E. (1989). Melatonin-induced suppression of human lymphocyte natural killer activity in vitro. J. Pineal Res. 7, 153–164. doi: 10.1111/j.1600-079x.1989.tb00663.x
Li, C. C., Munitic, I., Mittelstadt, P. R., Castro, E., and Ashwell, J. D. (2015). Suppression of dendritic cell-derived IL-12 by endogenous glucocorticoids is protective in LPS-induced sepsis. PLoS Biol. 13:e1002269. doi: 10.1371/journal.pbio.1002269
Linsell, C. R., Lightman, S. L., Mullen, P. E., Brown, M. J., and Causon, R. C. (1985). Circadian rhythms of epinephrine and norepinephrine in man. J. Clin. Endocrinol. Metab. 60, 1210–1215. doi: 10.1210/jcem-60-6-1210
Liu, J., Malkani, G., Shi, X., Meyer, M., Cunningham-Runddles, S., Ma, X., et al. (2006). The circadian clock Period 2 gene regulates gamma interferon production of NK cells in host response to lipopolysaccharide-induced endotoxic shock. Infect. Immun. 74, 4750–4756. doi: 10.1128/iai.00287-06
Logan, R. W., Arjona, A., and Sarkar, D. K. (2011). Role of sympathetic nervous system in the entrainment of circadian natural-killer cell function. Brain Behav. Immun. 25, 101–109. doi: 10.1016/j.bbi.2010.08.007
Logan, R. W., Zhang, C., Murugan, S., O’Connell, S., Levitt, D., Rosenwasser, A. M., et al. (2012). Chronic shift-lag alters the circadian clock of NK cells and promotes lung cancer growth in rats. J. Immunol. 188, 2583–2591. doi: 10.4049/jimmunol.1102715
Lord, G. M., Matarese, G., Howard, J. K., Baker, R. J., Bloom, S. R., and Lechler, R. I. (1998). Leptin modulates the T-cell immune response and reverses starvation-induced immunosuppression. Nature 394, 897–901. doi: 10.1038/29795
Luo, Y., and Liu, M. (2016). Adiponectin: a versatile player of innate immunity. J. Mol. Cell. Biol. 8, 120–128. doi: 10.1093/jmcb/mjw012
McNulty, J. A., Relfson, M., Fox, L. M., Fox, L. M., Kus, L., Handa, R. J., et al. (1990). Circadian analysis of mononuclear cells in the rat following pinealectomy and superior cervical ganglionectomy. Brain Behav. Immun. 4, 292–307. doi: 10.1016/0889-1591(90)90033-m
Messner, M., Huether, G., Lorf, T., Ramadori, G., and Schworer, H. (2001). Presence of melatonin in the human hepatobiliary-gastrointestinal tract. Life Sci. 69, 543–551. doi: 10.1016/s0024-3205(01)01143-2
Milano, C. A., Allen, L. F., Rockman, H. A., Dolber, P. C., McMinn, T. R., Chien, K. R., et al. (1994). Enhanced myocardial function in transgenic mice overexpressing the beta 2-adrenergic receptor. Science 264, 582–586. doi: 10.1126/science.8160017
Mitsui, S., Yamaguchi, S., Matsuo, T., Ishida, Y., and Okamura, H. (2001). Antagonistic role of E4BP4 and PAR proteins in the circadian oscillatory mechanism. Genes Dev. 15, 995–1006. doi: 10.1101/gad.873501
Molofsky, A. B., Nussbaum, J. C., Liang, H. E., Van Dyken, S. J., Cheng, L. E., Mohapatra, A., et al. (2013). Innate lymphoid type 2 cells sustain visceral adipose tissue eosinophils and alternatively activated macrophages. J. Exp. Med. 210, 535–549. doi: 10.1084/jem.20121964
Monticelli, L. A., Sonnenberg, G. F., Abt, M. C., Alenghat, T., Ziegler, C. G., Doering, T. A., et al. (2011). Innate lymphoid cells promote lung-tissue homeostasis after infection with influenza virus. Nat. Immunol. 12, 1045–1054. doi: 10.1038/ni.2131
Mori, A., Sakurai, H., Choo, M. K., Obi, R., Koizumi, K., Yoshida, C., et al. (2006). Severe pulmonary metastasis in obese and diabetic mice. Int. J. Cancer 119, 2760–2767. doi: 10.1002/ijc.22248
Moriyama, S., Brestoff, J. R., Flamar, A. L., Moeller, J. B., Klose, C. S. N., Rankin, L. C., et al. (2018). beta2-adrenergic receptor-mediated negative regulation of group 2 innate lymphoid cell responses. Science 359, 1056–1061. doi: 10.1126/science.aan4829
Nussbaum, J. C., Van Dyken, S. J., von Moltke, J., Cheng, L. E., Mohapatra, A., Molofsky, A. B., et al. (2013). Type 2 innate lymphoid cells control eosinophil homeostasis. Nature 502, 245–248. doi: 10.1038/nature12526
Olnes, M. J., Kotliarov, Y., Biancotto, A., Cheung, F., Chen, J., Shi, R., et al. (2016). Effects of systemically administered hydrocortisone on the human immunome. Sci. Rep. 6:23002.
O’Shea, D., and Hogan, A. E. (2019). Dysregulation of natural killer cells in obesity. Cancers (Basel) 11:573. doi: 10.3390/cancers11040573
Petty, T. L. (1988). Circadian variations in chronic asthma and chronic obstructive pulmonary disease. Am. J. Med. 85, 21–23. doi: 10.1016/0002-9343(88)90237-9
Pickard, J. M., Maurice, C. F., Kinnebrew, M. A., Abt, M. C., Schenten, D., Golovkina, T. V., et al. (2014). Rapid fucosylation of intestinal epithelium sustains host-commensal symbiosis in sickness. Nature 514, 638–641. doi: 10.1038/nature13823
Quatrini, L., Wieduwild, E., Escaliere, B., Filtjens, J., Chasson, L., Laprie, C., et al. (2018). Endogenous glucocorticoids control host resistance to viral infection through the tissue-specific regulation of PD-1 expression on NK cells. Nat. Immunol. 19, 954–962. doi: 10.1038/s41590-018-0185-0
Reppert, S. M., and Weaver, D. R. (2002). Coordination of circadian timing in mammals. Nature 418, 935–941. doi: 10.1038/nature00965
Sanchez-Margalet, V., Martin-Romero, C., Santos-Alvarez, J., Goberna, R., Najib, S., and Gonzalez-Yanes, C. (2003). Role of leptin as an immunomodulator of blood mononuclear cells: mechanisms of action. Clin. Exp. Immunol. 133, 11–19. doi: 10.1046/j.1365-2249.2003.02190.x
Sato, T. K., Panda, S., Miraglia, L. J., Reyes, T. M., Rudic, R. D., McNamara, P., et al. (2004). A functional genomics strategy reveals Rora as a component of the mammalian circadian clock. Neuron 43, 527–537. doi: 10.1016/j.neuron.2004.07.018
Satoh-Takayama, N., Serafini, N., Verrier, T., Rekiki, A., Renauld, J. C., Frankel, G., et al. (2014). The chemokine receptor CXCR6 controls the functional topography of interleukin-22 producing intestinal innate lymphoid cells. Immunity 41, 776–788. doi: 10.1016/j.immuni.2014.10.007
Sawa, S., Lochner, M., Satoh-Takayama, N., Dulauroy, S., Berard, M., Kleinschek, M., et al. (2011). RORgammat+ innate lymphoid cells regulate intestinal homeostasis by integrating negative signals from the symbiotic microbiota. Nat. Immunol. 12, 320–326. doi: 10.1038/ni.2002
Scheer, F. A., Hilton, M. F., Mantzoros, C. S., and Shea, S. A. (2009). Adverse metabolic and cardiovascular consequences of circadian misalignment. Proc. Natl. Acad. Sci. U.S.A. 106, 4453–4458. doi: 10.1073/pnas.0808180106
Schiffer, S., Pummer, S., Witte, K., and Lemmer, B. (2001). Cardiovascular regulation in TGR(mREN2)27 rats: 24h variation in plasma catecholamines, angiotensin peptides, and telemetric heart rate variability. Chronobiol. Int. 18, 461–474. doi: 10.1081/cbi-100103969
Sears, M. R., Taylor, D. R., Print, C. G., Lake, D. C., Li, Q. Q., Flannery, E. M., et al. (1990). Regular inhaled beta-agonist treatment in bronchial asthma. Lancet 336, 1391–1396. doi: 10.1016/0140-6736(90)93098-a
Seillet, C., Brossay, L., and Vivier, E. (2020a). Natural killers or ILC1s? That is the question. Curr. Opin. Immunol. 68, 48–53. doi: 10.1016/j.coi.2020.08.009
Seillet, C., and Jacquelot, N. (2019). Sensing of physiological regulators by innate lymphoid cells. Cell. Mol. Immunol. 16, 442–451. doi: 10.1038/s41423-019-0217-1
Seillet, C., Luong, K., Tellier, J., Jacquelot, N., Shen, R. D., Hickey, P., et al. (2020b). The neuropeptide VIP confers anticipatory mucosal immunity by regulating ILC3 activity. Nat. Immunol. 21, 168–177. doi: 10.1038/s41590-019-0567-y
Seshadri, S., Pope, R. L., and Zenewicz, L. A. (2018). Glucocorticoids inhibit group 3 innate lymphocyte IL-22 production. J. Immunol. 201, 1267–1274. doi: 10.4049/jimmunol.1800484
Shimba, A., Cui, G., Tani-Ichi, S., Ogawa, M., Abe, S., Okazaki, F., et al. (2018). Glucocorticoids drive diurnal oscillations in T cell distribution and responses by inducing interleukin-7 receptor and CXCR4. Immunity 48, 286–298.e6.
Spiga, F., Walker, J. J., Terry, J. R., and Lightman, S. L. (2014). HPA axis-rhythms. Compr Physiol 4, 1273–1298. doi: 10.1002/cphy.c140003
Stern, J. H., Rutkowski, J. M., and Scherer, P. E. (2016). Adiponectin, leptin, and fatty acids in the maintenance of metabolic homeostasis through adipose tissue crosstalk. Cell Metab. 23, 770–784. doi: 10.1016/j.cmet.2016.04.011
Sun, Z., Hou, D., Liu, S., Fu, W., Wang, J., and Liang, Z. (2018). Norepinephrine inhibits the cytotoxicity of NK92MI cells via the beta2adrenoceptor/cAMP/PKA/pCREB signaling pathway. Mol. Med. Rep. 17, 8530–8535.
Surjit, M., Ganti, K. P., Mukherji, A., Ye, T., Hua, G., Metzger, D., et al. (2011). Widespread negative response elements mediate direct repression by agonist-liganded glucocorticoid receptor. Cell 145, 224–241. doi: 10.1016/j.cell.2011.03.027
Talbot, J., Hahn, P., Kroehling, L., Nguyen, H., Li, D., and Littman, D. R. (2020). Feeding-dependent VIP neuron-ILC3 circuit regulates the intestinal barrier. Nature 579, 575–580. doi: 10.1038/s41586-020-2039-9
Tian, Z., Sun, R., Wei, H., and Gao, B. (2002). Impaired natural killer (NK) cell activity in leptin receptor deficient mice: leptin as a critical regulator in NK cell development and activation. Biochem. Biophys. Res. Commun. 298, 297–302. doi: 10.1016/s0006-291x(02)02462-2
Todd, W. D., Venner, A., Anaclet, C., Broadhurst, R. Y., De Luca, R., Bandaru, S. S., et al. (2020). Suprachiasmatic VIP neurons are required for normal circadian rhythmicity and comprised of molecularly distinct subpopulations. Nat. Commun. 11:4410.
Tsang, A. H., Koch, C. E., Kiehn, J. T., Schmidt, C. X., and Oster, H. (2020). An adipokine feedback regulating diurnal food intake rhythms in mice. Elife 9:e55388.
Venneri, M. A., Hasenmajer, V., Fiore, D., Sbardella, E., Pofi, R., Graziadio, C., et al. (2018). Circadian rhythm of glucocorticoid administration entrains clock genes in immune cells: a DREAM Trial Ancillary study. J. Clin. Endocrinol. Metab. 103, 2998–3009. doi: 10.1210/jc.2018-00346
Vivier, E., Artis, D., Colonna, M., Diefenbach, A., Di Santo, J. P., Eberl, G., et al. (2018). Innate lymphoid cells: 10 years on. Cell 174, 1054–1066.
Waite, E. J., McKenna, M., Kershaw, Y., Walker, J. J., Cho, K., Piggins, H. D., et al. (2012). Ultradian corticosterone secretion is maintained in the absence of circadian cues. Eur. J. Neurosci. 36, 3142–3150. doi: 10.1111/j.1460-9568.2012.08213.x
Wang, L., Luo, Y., Luo, L., Wu, D., Ding, X., Zheng, H., et al. (2021). Adiponectin restrains ILC2 activation by AMPK-mediated feedback inhibition of IL-33 signaling. J. Exp. Med. 218:e20191054.
Wang, Q., and Colonna, M. (2020). Keeping time in group 3 innate lymphoid cells. Nat. Rev. Immunol. 20, 720–726. doi: 10.1038/s41577-020-0397-z
Wen, Y., Zeng, Q., Luo, X., Ma, R., Tang, Y., and Liu, W. (2020). Leptin promoted IL-17 production from ILC2s in allergic rhinitis. Mediators Inflamm. 2020:9248479.
Wieduwild, E., Girard-Madoux, M. J., Quatrini, L., Laprie, C., Chasson, L., Rossignol, R., et al. (2020). Beta2-adrenergic signals downregulate the innate immune response and reduce host resistance to viral infection. J. Exp. Med. 217:e20190554.
Wilk, S., Jenke, A., Stehr, J., Yang, C. A., Bauer, S., Goldner, K., et al. (2013). Adiponectin modulates NK-cell function. Eur. J. Immunol. 43, 1024–1033. doi: 10.1002/eji.201242382
Wrann, C. D., Laue, T., Hubner, L., Kuhlmann, S., Jacobs, R., Goudeva, L., et al. (2012). Short-term and long-term leptin exposure differentially affect human natural killer cell immune functions. Am. J. Physiol. Endocrinol. Metab. 302, E108–E116.
Xu, H., Gustafson, C. L., Sammons, P. J., Khan, S. K., Parsley, N. C., Ramanathan, C., et al. (2015). Cryptochrome 1 regulates the circadian clock through dynamic interactions with the BMAL1 C terminus. Nat. Struct. Mol. Biol. 22, 476–484. doi: 10.1038/nsmb.3018
Yanofsky, V. R., Mitsui, H., Felsen, D., and Carucci, J. A. (2013). Understanding dendritic cells and their role in cutaneous carcinoma and cancer immunotherapy. Clin. Dev. Immunol. 2013:624123.
Zeng, Q., Luo, X., Tang, Y., Liu, W., and Luo, R. (2020). Leptin regulated ILC2 Cell through the PI3K/AKT pathway in allergic rhinitis. Mediators Inflamm. 2020:4176082.
Zhang, R., Lahens, N. F., Ballance, H. I., Hughes, M. E., and Hogenesch, J. B. (2014). A circadian gene expression atlas in mammals: implications for biology and medicine. Proc. Natl. Acad. Sci. U.S.A. 111, 16219–16224. doi: 10.1073/pnas.1408886111
Zhao, Y., Sun, R., You, L., Gao, C., and Tian, Z. (2003). Expression of leptin receptors and response to leptin stimulation of human natural killer cell lines. Biochem. Biophys. Res. Commun. 300, 247–252. doi: 10.1016/s0006-291x(02)02838-3
Keywords: circadian rhythm, neuroimmune interactions, homeostasis, inflammation, neuropeptide
Citation: Jacquelot N, Belz GT and Seillet C (2021) Neuroimmune Interactions and Rhythmic Regulation of Innate Lymphoid Cells. Front. Neurosci. 15:657081. doi: 10.3389/fnins.2021.657081
Received: 22 January 2021; Accepted: 29 March 2021;
Published: 29 April 2021.
Edited by:
Raghuveer Kavarthapu, National Institutes of Health (NIH), United StatesReviewed by:
Stephen Beesley, Florida State University, United StatesTanja Lange, University of Lübeck, Germany
Brian James Altman, University of Rochester, United States
Copyright © 2021 Jacquelot, Belz and Seillet. This is an open-access article distributed under the terms of the Creative Commons Attribution License (CC BY). The use, distribution or reproduction in other forums is permitted, provided the original author(s) and the copyright owner(s) are credited and that the original publication in this journal is cited, in accordance with accepted academic practice. No use, distribution or reproduction is permitted which does not comply with these terms.
*Correspondence: Cyril Seillet, c2VpbGxldEB3ZWhpLmVkdS5hdQ==