- 1Department of BioSciences, Rice University, Houston, TX, United States
- 2Department of Psychology, Djavad Mowafaghian Centre for Brain Health, The University of British Columbia, Vancouver, BC, Canada
- 3Departments of Neurology, Neuroscience and Psychiatry & Behavioral Sciences, Baylor College of Medicine, Houston, TX, United States
Parkinson’s disease (PD) is a neurodegenerative disorder that is characterized by symptoms that impact both motor and non-motor domains. Outside of motor impairments, PD patients are at risk for impulse control disorders (ICDs), which include excessively disabling impulsive and compulsive behaviors. ICD symptoms in PD (PD + ICD) can be broadly conceptualized as a synergistic interaction between dopamine agonist therapy and the many molecular and circuit-level changes intrinsic to PD. Aside from discontinuing dopamine agonist treatment, there remains a lack of consensus on how to best address ICD symptoms in PD. In this review, we explore recent advances in the molecular and neuroanatomical mechanisms underlying ICD symptoms in PD by summarizing a rapidly accumulating body of clinical and preclinical studies, with a special focus on the utility of rodent models in gaining new insights into the neurochemical basis of PD + ICD. We also discuss the relevance of these findings to the broader problem of impulsive and compulsive behaviors that impact a range of neuropsychiatric syndromes.
Introduction
Parkinson’s disease (PD) affects more than 10 million people worldwide (Dorsey et al., 2018; Rocca, 2018). Motor symptoms of PD are best appreciated as a cardinal “triad” of tremor, rigidity, and bradykinesia and can include akinesia and postural instability in late stages (Jankovic, 2008). Additionally, a non-motor prodromal phase, which includes changes in sleep, mood, olfactory, and autonomic function, may precede motor symptoms by up to a decade (Chaudhuri et al., 2006; Ishihara and Brayne, 2006; Masala et al., 2018). Unfortunately, non-motor symptoms often persist during the motor phase of PD, compounding overall disability and augmenting the need for institutionalization. This review focuses on one particular constellation of non-motor symptoms termed “impulse control disorders” (ICDs) and highlights recent studies that provide important updates on pathophysiology.
The pathological hallmark of PD is depigmentation, cell loss, and gliosis within the substantia nigra (SN) (Dauer and Przedborski, 2003), which is comprised of dopaminergic neurons that project to the striatum to form the nigrostriatal dopamine pathway, crucial for the stimulation of voluntary movement (Haber, 2014). Lewy bodies, neurotoxic structures composed mainly of abnormally phosphorylated and aggregated alpha-synuclein, are thought to propagate nigral degeneration (Kouli et al., 2018) and subsequently extend beyond nigral circuits. In fact, the rostro-caudal extent of alpha-synuclein accumulation has been extensively explored as a potential etiology for non-motor symptoms that affect multiple neural systems, including rapid eye movement sleep behavior disorder (pontine and medullary nuclei), constipation (autonomic enteric neurons), and cognitive impairment (frontal and other cortical regions) (Adler and Beach, 2016; Atik et al., 2016).
Impulse control and related behaviors (ICRBs) are characterized by the inability to assert self-control in emotions and behaviors, which can lead to compulsive and/or impulsive actions that harm oneself or others (American Psychiatric Association, 2013). Compulsivity includes repetitive and consciously unwanted (ego-dystonic) behaviors that subjects describe as being necessary to perform (Berlin and Hollander, 2014). Impulsivity is the reduction or absence of forethought or planning before making decisions and can be divided into motor and decision impulsivity (Winstanley, 2011). Common ICDs experienced by PD patients include pathological gambling, binge eating, hypersexuality, and compulsive buying. ICD development in PD patients (PD + ICD) is commonly observed as an iatrogenic side effect of dopamine agonists (DAAs), a type of dopamine replacement therapy (DRT), and symptoms usually improve with reduction of DAA treatment (You et al., 2018; Kelly et al., 2020). In the following sections, we critically examine preclinical and clinical data that explore the neuroanatomical and molecular bases for this pathophysiological synergy. Importantly, we conclude by highlighting the significance of the rodent model in pushing this research niche forward and discussing broader implications for the more general problem of ICRBs that are a feature of several neuropsychiatric syndromes. Articles reviewed in this manuscript were identified through a PubMed search utilizing keyword combinations including “Parkinson’s disease,” “impulse control disorders,” “rat or mouse,” and “animal model.”
Mechanisms Underlying PD + ICD: Human Studies
About 14–40% of PD patients experience symptoms of ICDs (Erga et al., 2017; Baig et al., 2019). Risk factors for PD + ICD include duration and dose of DAA treatment, male gender, comorbid apathy, anxiety, and depression (Weintraub et al., 2010a, 2013). DAA treatment, in particular, is strongly associated with a significantly increased risk of developing ICDs (Bódi et al., 2009; Garcia-Ruiz et al., 2014). Further, various psychological factors such as increased stress, greater illness identity, and negative coping strategies have also been found to predict PD + ICD (Garlovsky et al., 2016). ICD symptoms in newly diagnosed drug-naïve PD patients actually seem to be comparable to that of the general population, around 20% (Antonini et al., 2011; Weintraub et al., 2013). However, recent research has suggested a difference in the nature of ICRBs among these populations, whereby delay discounting (with preference for smaller and more immediate rewards over larger but more delayed rewards) and deficits in reflection impulsivity (ability to collect sufficient information prior to decision-making) better predict ICDs in the PD and general populations, respectively (Izzo et al., 2020).
Alterations in dopamine receptor signaling are a central property of PD + ICD. D1-like receptors (D1R), which include D1 and D5, are coupled to excitatory cyclic AMP (cAMP) signaling, and are broadly implicated in reward-based learning (Nakanishi et al., 2014). D2-like receptors (D2R), which include D2, D3, and D4, are coupled to cAMP inhibition and play a prominent role in avoidance learning (Draoui et al., 2020). DAAs such as pramipexole, ropinirole, rotigotine, and apomorphine are much more selective for D2R than D1R (Millan et al., 2002). Positron emission tomography (PET) approaches have indicated decreased D2R binding (Steeves et al., 2009; Stark et al., 2018) and relatively unchanged D1R binding in the ventral striatum in PD + ICD (Payer et al., 2015) compared with PD patients without ICDs (PD-ICD). These findings suggest that ICD symptoms may reflect DAA-mediated D2R overstimulation preventing punishment learning wherein adverse consequences of actions are ignored when making decisions, while the effects of D1R-mediated direct pathway and positive reinforcement learning are intact.
The density of dopamine transporters (DAT), responsible for the reuptake of dopamine from the synaptic cleft, may also play a key role. Single photon emission computed tomography (Cilia et al., 2010; Voon et al., 2014; Navalpotro-Gomez et al., 2019) and PET (Lee et al., 2014) investigations have demonstrated reduced striatal DAT binding in PD + ICD compared to PD-ICD. Furthermore, two studies have shown that decreased DAT availability precedes ICD development following DRTs and that the severity of ICDs experienced is inversely correlated with DAT density (Vriend et al., 2014; Smith et al., 2016), highlighting the potential use of baseline DAT density as a marker of future ICD risk in PD patients.
In studies of network-level dysfunction, resting-state functional magnetic resonance imaging (fMRI) approaches in medicated PD + ICD patients have indicated reduced cortico-striatal connectivity (Carriere et al., 2015; Ruitenberg et al., 2018) between the cingulate cortex and the nucleus accumbens (Hammes et al., 2019) and dorsal striatum (Cilia et al., 2011; Tessitore et al., 2017a). fMRI studies in which medicated subjects performed tasks to measure impulsivity have also observed lower activation in the prefrontal cortex (PFC) and striatum during these tasks in PD + ICD (Filip et al., 2018). Interestingly, several studies have identified more diffuse alterations in neural networks in newly diagnosed, drug-naïve PD patients who later developed ICDs or exhibited greater impulsivity compared to PD-ICD patients and healthy controls. This includes disruptions to numerous white matter tracts (Mojtahed Zadeh et al., 2018); decreased activation of various mesolimbic and mesocortical areas (van der Vegt et al., 2013; Vriend et al., 2015); and changes in connectivity in the default-mode, central executive, and salience networks (Tessitore et al., 2017b) in PD + ICD, indicating more widespread network-level correlates of predisposition to PD + ICD.
Finally, genetic association studies have demonstrated that the heritability of PD + ICD is around 57% (Kraemmer et al., 2016). Polymorphisms in genes encoding dopamine receptors (Lee et al., 2009; Zainal Abidin et al., 2015; Krishnamoorthy et al., 2016; Erga et al., 2018; McDonell et al., 2018; Redenšek et al., 2020), dopamine transporters (Cilia et al., 2016; Cormier-Dequaire et al., 2018; Redenšek et al., 2019, 2020), and proteins involved in dopamine metabolism (Ziegler et al., 2014; Kraemmer et al., 2016; Jesús et al., 2020) have been linked to increased PD + ICD risk. Interestingly, genetic modifiers of ICD risk appear to extend beyond the dopamine axis, including genes involved in glutamate (Lee et al., 2009; Zainal Abidin et al., 2015), serotonin, and (Lee et al., 2012; Cilia et al., 2016; Kraemmer et al., 2016) opioid (Kraemmer et al., 2016; Cormier-Dequaire et al., 2018; Erga et al., 2018) signaling, and other signal transduction genes (Hoenicka et al., 2015; Prud’hon et al., 2020) in PD + ICD. These data, summarized in Figure 1, illustrate a complex genetic landscape of ICD risk and implicate a range of non-dopaminergic systems that may be harnessed for treatment.
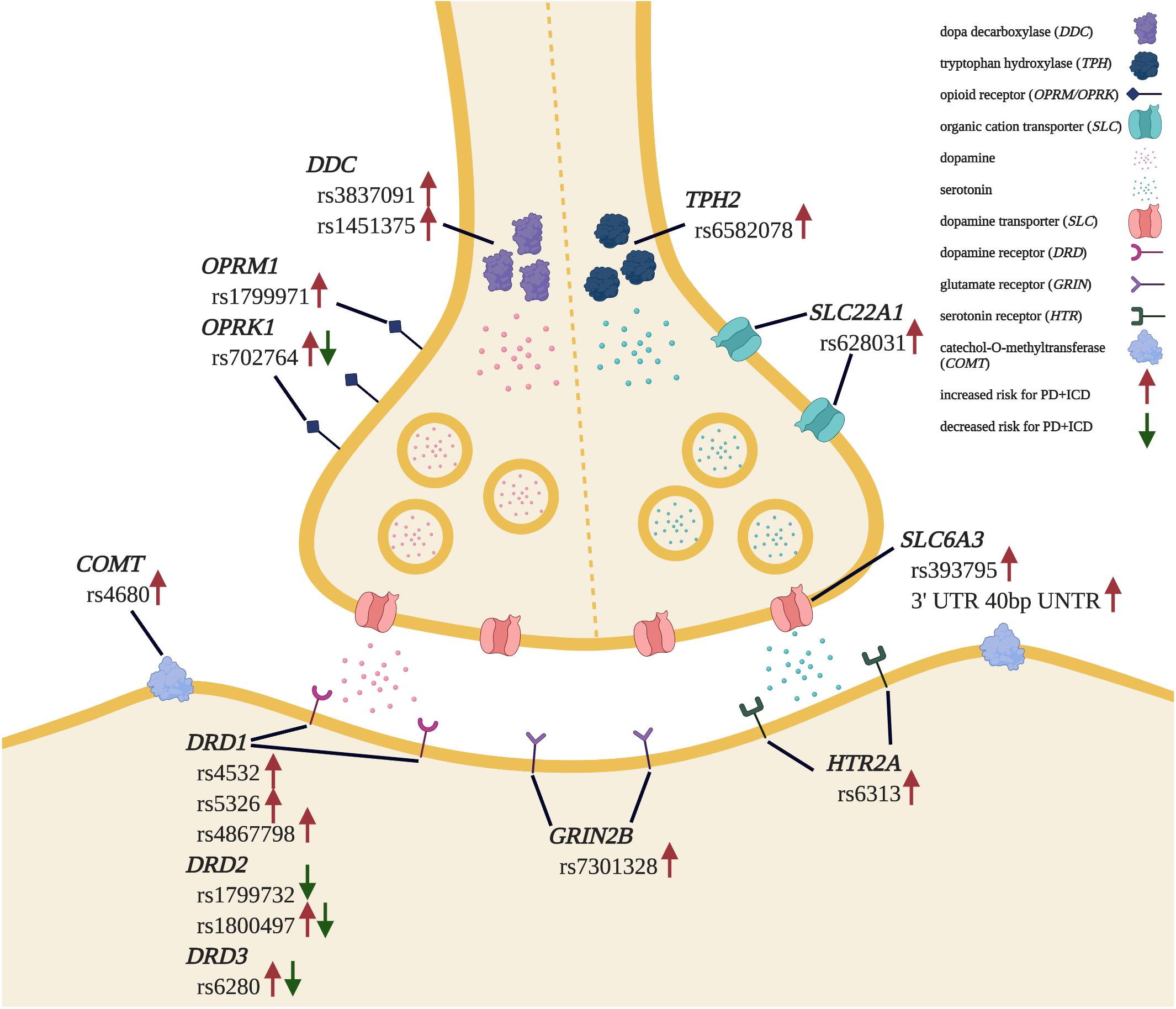
Figure 1. Genetic landscape of PD + ICD risk. Genetic polymorphisms found to be associated with increased and/or decreased risk for ICD development in PD patients are indicated. Adapted from “Synaptic Cleft” by BioRender.com (2020). Retrieved from https://app.biorender.com/biorender-templates.
Mechanisms Underlying PD + ICD: Rodent Studies
The psychopharmacological profile of D2R agonists in healthy rodents is complex: D2R agonists increase food consumption (Martin-Iverson and Dourish, 1988) and overall wakefulness (Qu et al., 2010) but tend to decrease locomotor activity (Li et al., 2010). The rewarding or aversive properties of DAAs in healthy rodents can be assessed through conditioned place preference/aversion assays (CPP/CPA) wherein injection with a DAA is repeatedly coupled with one chamber, while the control stimulus (saline injection) is paired with another chamber. On test day, the subject can freely explore all chambers. D1R agonists induce significant CPA, while D2R agonists enhance CPP, illustrating the predominant role of D2Rs in the reinforcing properties of DAAs (Zengin-Toktas et al., 2013). While some studies have reported that D2R agonist-mediated reinforcement is similar in both sham and PD-induced rats (Engeln et al., 2013; Zengin-Toktas et al., 2013), other studies have observed that lower doses of DAAs are sufficient to induce CPP in PD-induced rats compared with sham controls (Riddle et al., 2012; Loiodice et al., 2017). Since striatonigral degeneration itself results in a reduction in striatal dopamine levels, the subthreshold effects of DAAs may be related to baseline underactivation of D2Rs (Gallo, 2019). Further studies are clearly required to clarify the upstream mediators and downstream outcomes of D2R agonists in PD-like circuits.
A variety of tasks have been developed to measure impulsivity in rodents through quantitative assessments of the speed and accuracy of decision-making (Figure 2). For instance, in the 5-choice serial reaction time task (5-CSRTT) subjects are placed in an operant chamber with five apertures and a food reward tray and trained to receive a reward when they correctly nose-poke under the illuminated aperture in a timely fashion. Here motor impulsivity and compulsivity are reflected in the frequency of premature and perseverative responses, respectively (Higgins and Silenieks, 2017). In one study, PD-induced rats displayed a significant increase in premature response rate immediately following lesioning of the SN pars compacta, and DAA treatment further exacerbated this effect (Jiménez-Urbieta et al., 2019). Protocols that incorporate differential reinforcement of low rate of responding (DRL) and fixed consecutive number (FCN) schedules can also assess rodent motor impulsivity. In DRL, subjects must wait for a predetermined amount of time before responding to get a reward. In FCN, subjects must maintain their response at one station for a fixed number of times before responding at a second station to get a reward. One study incorporating DRL and FCN protocols found that nigrostriatal lesioning was sufficient to cause an increase in both types of motor impulsivity. DAA treatment exacerbated this phenotype, such that following treatment, rats that exhibited the highest rates of impulsivity were lesioned and also displayed high levels of pre-lesion impulsivity (Engeln et al., 2016).
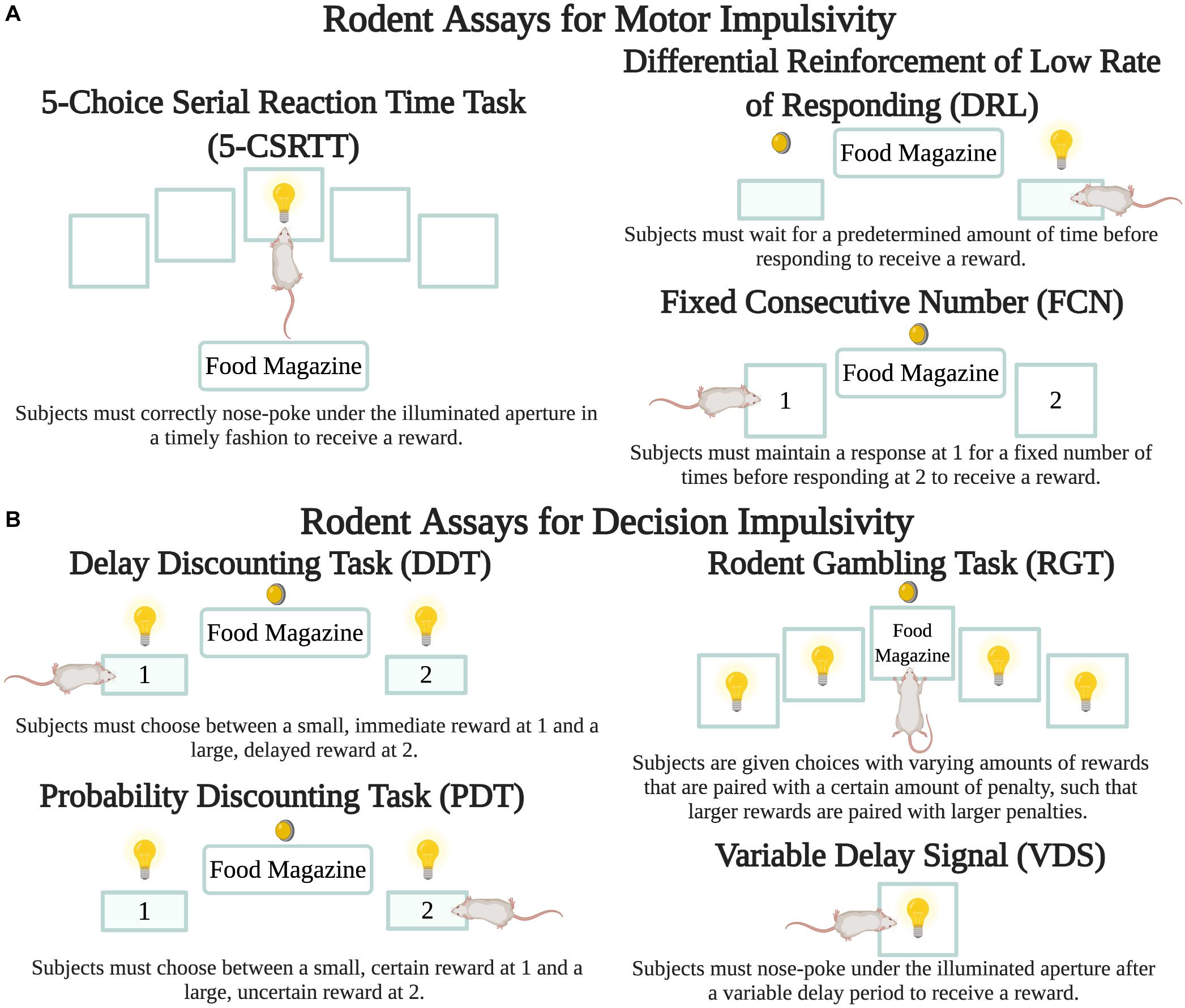
Figure 2. Rodent assays to measure impulsivity. While there are a number of variants for each protocol, the figure depicts the basic details of each. Lightbulbs are cue lights, circular lights are house lights, filled-in rectangles are pressable levers, and transparent squares are apertures for nose pokes. (A) Motor impulsivity can be measured through the 5-CSRTT (Higgins and Silenieks, 2017) as well as the DRL and FCN schedules (Engeln et al., 2016). (B) Decision impulsivity can be measured through the DDT (Tedford et al., 2015), PDT (Rokosik and Napier, 2012), and RGT (Tremblay et al., 2019). VDS can measure both motor and decision impulsivity (Jiménez-Urbieta et al., 2020). Created with BioRender.com.
Genetic models of PD have received some emphasis. Mice with a deletion in SNCA (encoding alpha-synuclein) displayed decreased motor impulsivity compared with wild-type mice (Peña-Oliver et al., 2012). In a follow-up study, it was determined that while SNCA deletion decreases motor impulsivity, it does not affect risky decision-making, measured using the rodent gambling task (RGT), described at the end of this section (Peña-Oliver et al., 2014). The involvement of other neurotransmitter systems in impulsivity has also been revealed through rodent studies. When mice with striatal and PFC lesions performed the 3-CSRTT, a variant of the 5-CSRTT with fewer choices, the amounts of striatal dopamine and norepinephrine were lower and prefrontal serotonin was the same for lesioned mice compared to wild-type mice. However, the ratio of 5-hydroxyindoleacetic acid (a serotonin metabolite) to serotonin was significantly decreased in the PFC of lesioned mice and strongly correlated with increased premature responses, suggesting a mediating role for these neurotransmitters in PD + ICD (Maiti et al., 2016).
In the delay discounting task (DDT) subjects initially prefer the large reinforcer, but as the delay to this reinforcer increases, preference for the smaller and rapid reward grows. This shift in preference is significantly enhanced in treatment-naïve rats with dopaminergic lesions of the dorsolateral striatum (Tedford et al., 2015). However, another DDT study found that lesioning of the dorsal striatum alone did not exacerbate impulsivity (Magnard et al., 2018). The probability discounting (PDT), variable delay-to-signal (VDS) and gambling tasks are also used to assess risky decision-making. In PDT, rodents are presented with choices with two possible outcomes- a small but certain reward or a larger but uncertain reward. As trials progress, the likelihood of receiving the larger reward decreases. Here impulsivity is choosing the larger reward, regardless of whether there is a high or low probability of attaining the reward. Using PDT, pramipexole increased preference for the large reinforcer by approximately 30–45% even at the greatest uncertainty levels in PD-induced rats (Rokosik and Napier, 2012). Even when the expected value of both options remains the same, such as when the uncertain outcome is twice the size as the guaranteed reward but only delivered on 50% of trials, chronic ropinirole administration increased choice for the large-but-uncertain outcome (Tremblay et al., 2017; Russell et al., 2020). The size of the effect was comparable in lesioned and non-lesioned rats.
In the VDS task, a visual stimulus signals the start of a time period during which a nose-poke response is rewarded after a variable delay period (Carvalho et al., 2017). Premature responses during the delay period reflect impulsivity, while perseverative responses after a correct response reflect compulsivity. Using VDS, low pramipexole doses (0.25 mg/kg) increased premature responses (motor impulsivity) and higher pramipexole doses (3 mg/kg) additionally precipitated delay intolerance (decision impulsivity). Moreover, “pre-existing” measures of impulsivity and those measured after lesioning of the dorsolateral striatum were both positively associated with impulsivity following DAA administration (Jiménez-Urbieta et al., 2020). Finally, in the RGT, subjects are generally given choices with varying amounts of rewards that are paired with a certain amount of penalty, such that larger rewards are paired with larger penalties and therefore result in fewer rewards during the course of a session (de Visser et al., 2011). An RGT study showed that chronic ropinirole significantly increased premature responses but did not alter preference for uncertain outcomes when the DAA was given following task acquisition (Tremblay et al., 2019). In the rodent slot machine task (rSMT) subjects respond to three flashing lights that are analogous to the three wheels of a slot machine. If all three lights are set to “on,” the subject needs to respond on the left-hand lever to collect its food reward. On any other trial type, the subject should start a new trial, equivalent to “rolling again,” or incur a time-out penalty. Using rSMT, subjects performed nearly twice the number of trials, and were less sensitive to loss events within the task following chronic ropinirole administration (Cocker et al., 2017, 2019).
Treatments for PD + ICD
Currently, the most common method to manage PD + ICD is withdrawing DAA treatment following ICRB manifestation. However, this management option has many caveats, including the possibility of dopamine agonist withdrawal syndrome (exacerbation of motor and certain psychiatric symptoms following DAA withdrawal), ICD onset due to other treatments, and behavioral relapse of ICRBs even following DAA elimination (Kelly et al., 2020). A number of pharmacologically distinct psychotropic agents have been examined as interventions, but consensus treatment recommendations are still absent as these studies have typically employed small sample sizes. Positive results have been observed with naltrexone, an opioid antagonist (Bosco et al., 2012; Verholleman et al., 2020); citalopram, a selective serotonin reuptake inhibitor (SSRI) (Ye et al., 2014); atomoxetine, a selective norepinephrine reuptake inhibitor (Kehagia et al., 2014; Rae et al., 2016); valproate, an anticonvulsant mood stabilizer (Hicks et al., 2011); clozapine, an atypical antipsychotic (Rotondo et al., 2010); and amantadine, a weak N-methyl-D-aspartate (NMDA) receptor antagonist (Thomas et al., 2010). However, naltrexone (Papay et al., 2014), various SSRIs (Bosco et al., 2012; Jeon and Bortolato, 2020), amantadine (Thomas et al., 2010; Weintraub et al., 2010b), and several atypical antipsychotics and glutamatergic modulators (Jeon and Bortolato, 2020) have also been reported to have no or negative impact on PD + ICD. In rodent PD models, positive effects have been observed with agonists of G protein-coupled receptor 52 (Russell et al., 2020); propranolol, a β-adrenoceptor antagonist (Cocker et al., 2019); and mirtazapine, an atypical antidepressant (Holtz et al., 2016). Whether non-pharmacological interventions such as deep brain stimulation (DBS) ameliorates or worsens ICRBs in PD patients is still debated. While most human studies indicated that DBS improves PD + ICD, some have reported the development of short-term de novo ICDs following DBS (Amami et al., 2015; Rossi et al., 2017; Kim et al., 2018; Lo Buono et al., 2020; Santin et al., 2020). In rodents, decreased risk-taking and loss-chasing was reported following lesioning to the subthalamic nucleus (STN), possibly pinpointing this region as a target for DBS and other treatments for PD + ICD (Breysse et al., 2020). While DBS to the STN reduced risky decision-making in rodents with high levels of pre-existing impulsivity (Adams et al., 2017), in PD-induced rodents, DBS to the STN (Anderson et al., 2020) and globus pallidus internus increased impulsivity (Summerson et al., 2014). As a field, these data point to the need for more comprehensive therapeutics that simultaneously alleviate psychiatric and motor symptoms in patients with PD + ICD.
Conclusion
Understanding PD + ICD pathophysiology remains a work in progress. While the role of DAAs is generally accepted, the specific molecular interactions that precipitate ICRBs are largely unknown. Human studies have looked into alterations in dopamine receptor subtype expression, dopamine transporter expression, neural networks, and genetic polymorphisms that may predispose PD patients to ICD development, building a complex working model of PD + ICD etiology. Rodent models of PD are essential to test these specific hypotheses, and we are blessed to have access to a rich complement of preclinical protocols (Figure 2) to quantitatively dissect and distinguish features of ICRBs in rodents. These models and assays also provide a substrate to investigate pharmacological and non-pharmacological treatments that ameliorate ICRBs and should be complemented with simultaneous assays of PD motor dysfunction. Not surprisingly, many ICD risk-altering genetic variants (Figure 1) have been linked to other neuropsychiatric syndromes marked by impulsivity and compulsivity, including substance dependence, pathological gambling, and attention-deficit/hyperactivity disorder (Hollander and Rosen, 2000). Thus, cellular and neuroanatomical insights gathered from the study of ICDs in PD patients may have broad relevance beyond PD alone.
Author Contributions
AA wrote the first draft of the manuscript. All authors contributed to manuscript revision and approved the submitted version.
Funding
AA received funding from Rice University’s Office of Undergraduate Research and Inquiry and Department of BioSciences. VK is supported by the Office of Research at the Baylor College of Medicine. CAW is supported by the Canadian Institutes of Health Research (CIHR) Project Grant PJT-162312.
Conflict of Interest
The authors declare that the research was conducted in the absence of any commercial or financial relationships that could be construed as a potential conflict of interest.
References
Adams, W. K., Haar, C. V., Tremblay, M., Cocker, P. J., Silveira, M. M., Kaur, S., et al. (2017). Deep-brain stimulation of the subthalamic nucleus selectively decreases risky choice in risk-preferring rats. eNeuro 4:ENEURO.0094-17.2017. doi: 10.1523/ENEURO.0094-17.2017
Adler, C. H., and Beach, T. G. (2016). Neuropathological basis of nonmotor manifestations of Parkinson’s disease. Mov. Disord. 31, 1114–1119. doi: 10.1002/mds.26605
Amami, P., Dekker, I., Piacentini, S., Ferré, F., Romito, L. M., Franzini, A., et al. (2015). Impulse control behaviours in patients with Parkinson’s disease after subthalamic deep brain stimulation: de novo cases and 3-year follow-up. J. Neurol. Neurosurg. Psychiatry 86, 562–564. doi: 10.1136/jnnp-2013-307214
American Psychiatric Association (ed.) (2013). Diagnostic and Statistical Manual of Mental Disorders: DSM-5, 5th Edn. Washington, DC: American Psychiatric Association.
Anderson, C., Sheppard, D., and Dorval, A. D. (2020). Parkinsonism and subthalamic deep brain stimulation dysregulate behavioral motivation in a rodent model. Brain Res. 1736:146776. doi: 10.1016/j.brainres.2020.146776
Antonini, A., Siri, C., Santangelo, G., Cilia, R., Poletti, M., Canesi, M., et al. (2011). Impulsivity and compulsivity in drug-naïve patients with Parkinson’s disease. Mov. Disord. 26, 464–468. doi: 10.1002/mds.23501
Atik, A., Stewart, T., and Zhang, J. (2016). Alpha-synuclein as a biomarker for Parkinson’s disease. Brain Pathol. 26, 410–418. doi: 10.1111/bpa.12370
Baig, F., Kelly, M. J., Lawton, M. A., Ruffmann, C., Rolinski, M., Klein, J. C., et al. (2019). Impulse control disorders in Parkinson disease and RBD: a longitudinal study of severity. Neurology 93, e675–e687. doi: 10.1212/WNL.0000000000007942
Berlin, G. S., and Hollander, E. (2014). Compulsivity, impulsivity, and the DSM-5 process. CNS Spectr. 19, 62–68. doi: 10.1017/S1092852913000722
Bódi, N., Kéri, S., Nagy, H., Moustafa, A., Myers, C. E., Daw, N., et al. (2009). Reward-learning and the novelty-seeking personality: a between- and within-subjects study of the effects of dopamine agonists on young Parkinson’s patients. Brain 132, 2385–2395. doi: 10.1093/brain/awp094
Bosco, D., Plastino, M., Colica, C., Bosco, F., Arianna, S., Vecchio, A., et al. (2012). Opioid antagonist naltrexone for the treatment of pathological gambling in Parkinson disease. Clin. Neuropharmacol. 35, 118–120. doi: 10.1097/WNF.0b013e31824d529b
Breysse, E., Meffre, J., Pelloux, Y., Winstanley, C. A., and Baunez, C. (2020). Decreased risk-taking and loss-chasing after subthalamic nucleus lesion in rats. Eur. J. Neurosci. e14895. doi: 10.1111/ejn.14895. [Epub ahead of print].
Carriere, N., Lopes, R., Defebvre, L., Delmaire, C., and Dujardin, K. (2015). Impaired corticostriatal connectivity in impulse control disorders in Parkinson disease. Neurology 84, 2116–2123. doi: 10.1212/WNL.0000000000001619
Carvalho, M. M., Campos, F. L., Marques, M., Soares-Cunha, C., Kokras, N., Dalla, C., et al. (2017). Effect of levodopa on reward and impulsivity in a rat model of Parkinson’s disease. Front. Behav. Neurosci. 11:145. doi: 10.3389/fnbeh.2017.00145
Chaudhuri, K. R., Healy, D. G., and Schapira, A. H. (2006). Non-motor symptoms of Parkinson’s disease: diagnosis and management. Lancet Neurol. 5, 235–245. doi: 10.1016/S1474-4422(06)70373-8
Cilia, R., Benfante, R., Asselta, R., Marabini, L., Cereda, E., Siri, C., et al. (2016). Tryptophan hydroxylase type 2 variants modulate severity and outcome of addictive behaviors in Parkinson’s disease. Parkinsonism Relat. Disord. 29, 96–103. doi: 10.1016/j.parkreldis.2016.05.017
Cilia, R., Cho, S. S., van Eimeren, T., Marotta, G., Siri, C., Ko, J. H., et al. (2011). Pathological gambling in patients with Parkinson’s disease is associated with fronto-striatal disconnection: a path modeling analysis. Mov. Disord. 26, 225–233. doi: 10.1002/mds.23480
Cilia, R., Ko, J. H., Cho, S. S., van Eimeren, T., Marotta, G., Pellecchia, G., et al. (2010). Reduced dopamine transporter density in the ventral striatum of patients with Parkinson’s disease and pathological gambling. Neurobiol. Dis. 39, 98–104. doi: 10.1016/j.nbd.2010.03.013
Cocker, P. J., Lin, M. Y., Tremblay, M., Kaur, P. J., Lin, M. Y., Tremblay, M., Kaur, S., and Winstanley, C. A. (2019). The β-adrenoceptor blocker propranolol ameliorates compulsive-like gambling behaviour in a rodent slot machine task: implications for iatrogenic gambling disorder. Eur. J. Neurosci. 50, 2401–2414. doi: 10.1111/ejn.14070
Cocker, P. J., Tremblay, M., Kaur, S., and Winstanley, C. A. (2017). Chronic administration of the dopamine D2/3 agonist ropinirole invigorates performance of a rodent slot machine task, potentially indicative of less distractible or compulsive-like gambling behaviour. Psychopharmacology 234, 137–153. doi: 10.1007/s00213-016-4447-y
Cormier-Dequaire, F., Bekadar, S., Anheim, M., Lebbah, S., Pelissolo, A., Krack, P., et al. (2018). Suggestive association between OPRM1 and impulse control disorders in Parkinson’s disease. Mov. Disord. 33, 1878–1886. doi: 10.1002/mds.27519
Dauer, W., and Przedborski, S. (2003). Parkinson’s disease: mechanisms and models. Neuron 39, 889–909. doi: 10.1016/S0896-6273(03)00568-3
de Visser, L., Homberg, J. R., Mitsogiannis, M., Zeeb, F. D., Rivalan, M., Fitoussi, A., et al. (2011). Rodent versions of the iowa gambling task: opportunities and challenges for the understanding of decision-making. Front. Neurosci. 5:109. doi: 10.3389/fnins.2011.00109
Dorsey, E. R., Elbaz, A., Nichols, E., Abd-Allah, F., Abdelalim, A., Adsuar, J. C., et al. (2018). Global, regional, and national burden of Parkinson’s disease, 1990–2016: a systematic analysis for the Global Burden of Disease Study 2016. Lancet Neurol. 17, 939–953. doi: 10.1016/S1474-4422(18)30295-3
Draoui, A., El Hiba, O., Aimrane, A., El Khiat, A., and Gamrani, H. (2020). Parkinson’s disease: from bench to bedside. Rev. Neurol. 176, 543–559. doi: 10.1016/j.neurol.2019.11.002
Engeln, M., Ahmed, S. H., Vouillac, C., Tison, F., Bezard, E., and Fernagut, P.-O. (2013). Reinforcing properties of Pramipexole in normal and parkinsonian rats. Neurobiol. Dis. 49, 79–86. doi: 10.1016/j.nbd.2012.08.005
Engeln, M., Ansquer, S., Dugast, E., Bezard, E., Belin, D., and Fernagut, P.-O. (2016). Multi-facetted impulsivity following nigral degeneration and dopamine replacement therapy. Neuropharmacology 109, 69–77. doi: 10.1016/j.neuropharm.2016.05.013
Erga, A. H., Alves, G., Larsen, J. P., Tysnes, O. B. R., and Pedersen, K. F. (2017). Impulsive and compulsive behaviors in Parkinson’s disease: the Norwegian ParkWest study. J. Parkinsons. Dis. 7, 183–191. doi: 10.3233/JPD-160977
Erga, A. H., Dalen, I., Ushakova, A., Chung, J., Tzoulis, C., Tysnes, O. B., et al. (2018). Dopaminergic and opioid pathways associated with impulse control disorders in Parkinson’s disease. Front. Neurol. 9:109. doi: 10.3389/fneur.2018.00109
Filip, P., Linhartová, P., Hlavatá, P., Šumec, R., Baláž, M., Bareš, M., et al. (2018). Disruption of multiple distinctive neural networks associated with impulse control disorder in Parkinson’s disease. Front. Hum. Neurosci. 12:462. doi: 10.3389/fnhum.2018.00462
Gallo, E. F. (2019). Disentangling the diverse roles of dopamine D2 receptors in striatal function and behavior. Neurochem. Int. 125, 35–46. doi: 10.1016/j.neuint.2019.01.022
Garcia-Ruiz, P. J., Castrillo, J. C. M., Alonso-Canovas, A., Barcenas, A. H., Vela, L., Alonso, P. S., et al. (2014). Impulse control disorder in patients with Parkinson’s disease under dopamine agonist therapy: a multicentre study. J. Neurol. Neurosurg. Psychiatry 85, 840–844. doi: 10.1136/jnnp-2013-306787
Garlovsky, J. K., Simpson, J., Grünewald, R. A., and Overton, P. G. (2016). Impulse control disorders in Parkinson’s disease: predominant role of psychological determinants. Psychol. Health 31, 1391–1414. doi: 10.1080/08870446.2016.1218879
Haber, S. N. (2014). The place of dopamine in the cortico-basal ganglia circuit. Neuroscience 282, 248–257. doi: 10.1016/j.neuroscience.2014.10.008
Hammes, J., Theis, H., Giehl, K., Hoenig, M. C., Greuel, A., Tittgemeyer, M., et al. (2019). Dopamine metabolism of the nucleus accumbens and fronto-striatal connectivity modulate impulse control. Brain 142, 733–743. doi: 10.1093/brain/awz007
Hicks, C. W., Pandya, M. M., Itin, I., and Fernandez, H. H. (2011). Valproate for the treatment of medication-induced impulse-control disorders in three patients with Parkinson’s disease. Parkinsonism Relat. Disord. 17, 379–381. doi: 10.1016/j.parkreldis.2011.03.003
Higgins, G. A., and Silenieks, L. B. (2017). Rodent test of attention and impulsivity: the 5-choice serial reaction time task. Curr. Protoc. Pharmacol. 78, 5.49.1–5.49.34. doi: 10.1002/cpph.27
Hoenicka, J., García-Ruiz, P. J., Ponce, G., Herranz, A., Martínez-Rubio, D., Pérez-Santamarina, E., et al. (2015). The addiction-related gene ANKK1 in Parkinsonian patients with impulse control disorder. Neurotox. Res. 27, 205–208. doi: 10.1007/s12640-014-9504-x
Hollander, E., and Rosen, J. (2000). Impulsivity. J. Psychopharmacol. 14, S39–S44. doi: 10.1177/02698811000142S106
Holtz, N. A., Tedford, S. E., Persons, A. L., Grasso, S. A., and Napier, T. C. (2016). Pharmacologically distinct pramipexole-mediated akinesia vs. risk-taking in a rat model of Parkinson’s disease. Prog. Neuropsychopharmacol. Biol. Psychiatry 70, 77–84. doi: 10.1016/j.pnpbp.2016.05.004
Ishihara, L., and Brayne, C. (2006). A systematic review of depression and mental illness preceding Parkinson’s disease. Acta Neurol. Scand. 113, 211–220. doi: 10.1111/j.1600-0404.2006.00579.x
Izzo, V. A., Donati, M. A., Torre, E., Ramat, S., and Primi, C. (2020). Impulse control disorders in Parkinson’s disease versus in healthy controls: a different predictive model. J. Neuropsychol. 14, 318–332. doi: 10.1111/jnp.12193
Jankovic, J. (2008). Parkinson’s disease: clinical features and diagnosis. J. Neurol. Neurosurg. Psychiatry 79, 368–376. doi: 10.1136/jnnp.2007.131045
Jeon, N., and Bortolato, M. (2020). What drugs modify the risk of iatrogenic impulse-control disorders in Parkinson’s disease? A preliminary pharmacoepidemiologic study. PLoS One 15:e0227128. doi: 10.1371/journal.pone.0227128
Jesús, S., Periñán, M. T., Cortés, C., Buiza-Rueda, D., Macías-García, D., Adarmes, A., et al. (2020). Integrating genetic and clinical data to predict impulse control disorders in Parkinson’s disease. Eur. J. Neurol. 28, 459–468. doi: 10.1111/ene.14590
Jiménez-Urbieta, H., Gago, B., Quiroga-Varela, A., Rodríguez-Chinchilla, T., Merino-Galán, L., Delgado-Alvarado, M., et al. (2020). Motor impulsivity and delay intolerance are elicited in a dose-dependent manner with a dopaminergic agonist in parkinsonian rats. Psychopharmacology 237, 2419–2431. doi: 10.1007/s00213-020-05544-6
Jiménez-Urbieta, H., Gago, B., Quiroga-Varela, A., Rodríguez-Chinchilla, T., Merino-Galán, L., Oregi, A., et al. (2019). Pramipexole-induced impulsivity in mildparkinsonian rats: a model of impulse control disorders in Parkinson’s disease. Neurobiol. Aging 75, 126–135. doi: 10.1016/j.neurobiolaging.2018.11.021
Kehagia, A. A., Housden, C. R., Regenthal, R., Barker, R. A., Müller, U., Rowe, J., et al. (2014). Targeting impulsivity in Parkinson’s disease using atomoxetine. Brain 137, 1986–1997. doi: 10.1093/brain/awu117
Kelly, M. J., Baig, F., Hu, M. T.-M., and Okai, D. (2020). Spectrum of impulse control behaviours in Parkinson’s disease: pathophysiology and management. J. Neurol. Neurosurg. Psychiatry 91, 703–711. doi: 10.1136/jnnp-2019-322453
Kim, A., Kim, Y. E., Kim, H.-J., Yun, J. Y., Yang, H.-J., Lee, W.-W., et al. (2018). A 7-year observation of the effect of subthalamic deep brain stimulation on impulse control disorder in patients with Parkinson’s disease. Parkinsonism Relat. Disord. 56, 3–8. doi: 10.1016/j.parkreldis.2018.07.010
Kouli, A., Torsney, K. M., and Kuan, W.-L. (2018). “Parkinson’s disease: etiology, neuropathology, and pathogenesis,” in Parkinson’s Disease: Pathogenesis and Clinical Aspects, eds T. B. Stoker and J. C. Greenland (Brisbane: Codon Publications).
Kraemmer, J., Smith, K., Weintraub, D., Guillemot, V., Nalls, M. A., Cormier-Dequaire, F., et al. (2016). Clinical-genetic model predicts incident impulse control disorders in Parkinson’s disease. J. Neurol. Neurosurg. Psychiatry 87, 1106–1111. doi: 10.1136/jnnp-2015-312848
Krishnamoorthy, S., Rajan, R., Banerjee, M., Kumar, H., Sarma, G., Krishnan, S., et al. (2016). Dopamine D3 receptor Ser9Gly variant is associated with impulse control disorders in Parkinson’s disease patients. Parkinsonism Relat. Disord. 30, 13–17. doi: 10.1016/j.parkreldis.2016.06.005
Lee, J.-Y., Jeon, B. S., Kim, H.-J., and Park, S.-S. (2012). Genetic variant of HTR2A associates with risk of impulse control and repetitive behaviors in Parkinson’s disease. Parkinsonism Relat. Disord. 18, 76–78. doi: 10.1016/j.parkreldis.2011.08.009
Lee, J.-Y., Lee, E. K., Park, S. S., Lim, J.-Y., Kim, H. J., Kim, J. S., et al. (2009). Association of DRD3 and GRIN2B with impulse control and related behaviors in Parkinson’s disease. Mov. Disord. 24, 1803–1810. doi: 10.1002/mds.22678
Lee, J.-Y., Seo, S. H., Kim, Y. K., Yoo, H. B., Kim, Y. E., Song, I. C., et al. (2014). Extrastriatal dopaminergic changes in Parkinson’s disease patients with impulse control disorders. J. Neurol. Neurosurg. Psychiatry 85, 23–30. doi: 10.1136/jnnp-2013-305549
Li, S.-M., Collins, G. T., Paul, N. M., Grundt, P., Newman, A. H., Xu, M., et al. (2010). Yawning and locomotor behavior induced by dopamine receptor agonists in mice and rats. Behav. Pharmacol. 21, 171–181. doi: 10.1097/FBP.0b013e32833a5c68
Lo Buono, V., Palmeri, R., Stroscio, G., Corallo, F., Di Lorenzo, G., Sorbera, C., et al. (2020). The effect on deep brain stimulation of subthalamic nucleus and dopaminergic treatment in Parkinson disease. Medicine (Baltimore) 99:e21578. doi: 10.1097/MD.0000000000021578
Loiodice, S., Winlow, P., Dremier, S., Hanon, E., Dardou, D., Ouachikh, O., et al. (2017). Pramipexole induced place preference after L-dopa therapy and nigral dopaminergic loss: linking behavior to transcriptional modifications. Psychopharmacology (Berl) 234, 15–27. doi: 10.1007/s00213-016-4430-7
Magnard, R., Vachez, Y., Carcenac, C., Boulet, S., Houeto, J.-L., Savasta, M., et al. (2018). Nigrostriatal dopaminergic denervation does not promote impulsive choice in the rat: implication for impulse control disorders in Parkinson’s disease. Front. Behav. Neurosci. 12:312. doi: 10.3389/fnbeh.2018.00312
Maiti, P., Gregg, L. C., and McDonald, M. P. (2016). MPTP-induced executive dysfunction is associated with altered prefrontal serotonergic function. Behav. Brain Res. 298, 192–201. doi: 10.1016/j.bbr.2015.09.014
Martin-Iverson, M. T., and Dourish, C. T. (1988). Role of dopamine D-1 and D-2 receptor subtypes in mediating dopamine agonist effects on food consumption in rats. Psychopharmacology 96, 370–374. doi: 10.1007/BF00216064
Masala, C., Solla, P., Liscia, A., Defazio, G., Saba, L., Cannas, A., et al. (2018). Correlation among olfactory function, motors’ symptoms, cognitive impairment, apathy, and fatigue in patients with Parkinson’s disease. J. Neurol. 265, 1764–1771. doi: 10.1007/s00415-018-8913-9
McDonell, K. E., van Wouwe, N. C., Harrison, M. B., Wylie, S. A., and Claassen, D. O. (2018). Taq1A polymorphism and medication effects on inhibitory action control in Parkinson disease. Brain Behav. 8:e01008. doi: 10.1002/brb3.1008
Millan, M. J., Maiofiss, L., Cussac, D., Audinot, V., Boutin, J.-A., and Newman-Tancredi, A. (2002). Differential actions of antiparkinson agents at multiple classes of monoaminergic receptor. I. A multivariate analysis of the binding profiles of 14 drugs at 21 native and cloned human receptor subtypes. J. Pharmacol. Exp. Ther. 303, 791–804. doi: 10.1124/jpet.102.039867
Mojtahed Zadeh, M., Ashraf-Ganjouei, A., Ghazi Sherbaf, F., Haghshomar, M., and Aarabi, M. H. (2018). White matter tract alterations in drug-naïve Parkinson’s disease patients with impulse control disorders. Front. Neurol. 9:163. doi: 10.3389/fneur.2018.00163
Nakanishi, S., Hikida, T., and Yawata, S. (2014). Distinct dopaminergic control of the direct and indirect pathways in reward-based and avoidance learning behaviors. Neuroscience 282, 49–59. doi: 10.1016/j.neuroscience.2014.04.026
Navalpotro-Gomez, I., Dacosta-Aguayo, R., Molinet-Dronda, F., Martin-Bastida, A., Botas-Peñin, A., Jimenez-Urbieta, H., et al. (2019). Nigrostriatal dopamine transporter availability, and its metabolic and clinical correlates in Parkinson’s disease patients with impulse control disorders. Eur. J. Nucl. Med. Mol. Imaging 46, 2065–2076. doi: 10.1007/s00259-019-04396-3
Papay, K., Xie, S. X., Stern, M., Hurtig, H., Siderowf, A., Duda, J. E., et al. (2014). Naltrexone for impulse control disorders in Parkinson disease: a placebo-controlled study. Neurology 83, 826–833. doi: 10.1212/WNL.0000000000000729
Payer, D. E., Guttman, M., Kish, S. J., Tong, J., Strafella, A., Zack, M., et al. (2015). [11C]-(+)-PHNO PET imaging of dopamine D(2/3) receptors in Parkinson’s disease with impulse control disorders. Mov. Disord. 30, 160–166. doi: 10.1002/mds.26135
Peña-Oliver, Y., Buchman, V. L., Dalley, J. W., Robbins, T. W., Schumann, G., Ripley, T. L., et al. (2012). Deletion of alpha-synuclein decreases impulsivity in mice. Genes Brain Behav. 11, 137–146. doi: 10.1111/j.1601-183X.2011.00758.x
Peña-Oliver, Y., Sanchez-Roige, S., Stephens, D. N., and Ripley, T. L. (2014). Alpha-synuclein deletion decreases motor impulsivity but does not affect risky decision making in a mouse Gambling Task. Psychopharmacology 231, 2493–2506. doi: 10.1007/s00213-013-3416-y
Prud’hon, S., Bekadar, S., Rastetter, A., Guégan, J., Cormier-Dequaire, F., Lacomblez, L., et al. (2020). Exome sequencing reveals signal transduction genes involved in impulse control disorders in Parkinson’s disease. Front. Neurol. 11:641. doi: 10.3389/fneur.2020.00641
Qu, W.-M., Xu, X.-H., Yan, M.-M., Wang, Y.-Q., Urade, Y., and Huang, Z.-L. (2010). Essential role of dopamine D2 receptor in the maintenance of wakefulness, but not in homeostatic regulation of sleep, in mice. J. Neurosci. 30, 4382–4389. doi: 10.1523/JNEUROSCI.4936-09.2010
Rae, C. L., Nombela, C., Rodríguez, P. V., Ye, Z., Hughes, L. E., Jones, P. S., et al. (2016). Atomoxetine restores the response inhibition network in Parkinson’s disease. Brain 139, 2235–2248. doi: 10.1093/brain/aww138
Redenšek, S., Flisar, D., Kojoviæ, M., Gregoriè Kramberger, M., Georgiev, D., Pirtošek, Z., et al. (2019). Dopaminergic pathway genes influence adverse events related to dopaminergic treatment in Parkinson’s disease. Front. Pharmacol. 10:8. doi: 10.3389/fphar.2019.00008
Redenšek, S., Jenko Bizjan, B., Trošt, M., and Dolžan, V. (2020). Clinical and clinical-pharmacogenetic models for prediction of the most common psychiatric complications due to dopaminergic treatment in Parkinson’s disease. Int. J. Neuropsychopharmacol. 23, 496–504. doi: 10.1093/ijnp/pyaa028
Riddle, J. L., Rokosik, S. L., and Napier, T. C. (2012). Pramipexole- and methamphetamine-induced reward-mediated behavior in a rodent model of Parkinson’s disease and controls. Behav. Brain Res. 233, 15–23. doi: 10.1016/j.bbr.2012.04.027
Rocca, W. A. (2018). The burden of Parkinson’s disease: a worldwide perspective. Lancet Neurol. 17, 928–929. doi: 10.1016/S1474-4422(18)30355-7
Rokosik, S. L., and Napier, T. C. (2012). Pramipexole-induced increased probabilistic discounting: comparison between a rodent model of Parkinson’s disease and controls. Neuropsychopharmacology 37, 1397–1408. doi: 10.1038/npp.2011.325
Rossi, P. J., De Jesus, S., Hess, C. W., Martinez-Ramirez, D., Foote, K. D., Gunduz, A., et al. (2017). Measures of impulsivity in Parkinson’s disease decrease after DBS in the setting of stable dopamine therapy. Parkinsonism Relat. Disord. 44, 13–17. doi: 10.1016/j.parkreldis.2017.08.006
Rotondo, A., Bosco, D., Plastino, M., Consoli, A., and Bosco, F. (2010). Clozapine for medication-related pathological gambling in Parkinson disease. Mov. Disord. 25, 1994–1995. doi: 10.1002/mds.23177
Ruitenberg, M. F. L., Wu, T., Averbeck, B. B., Chou, K. L., Koppelmans, V., and Seidler, R. D. (2018). Impulsivity in Parkinson’s disease is associated with alterations in affective and sensorimotor striatal networks. Front. Neurol. 9:279. doi: 10.3389/fneur.2018.00279
Russell, B., Barrus, M. M., Tremblay, M., Ma, L., Hrelja, K., Wong, C., et al. (2020). GPR52 agonists attenuate ropinirole-induced preference for uncertain outcomes. Behav. Neurosci. doi: 10.1037/bne0000391
Santin, M. D. N., Voulleminot, P., Vrillon, A., Hainque, E., Béreau, M., et al. (2020). Impact of subthalamic deep brain stimulation on impulse control disorders in Parkinson’s disease: a prospective study. Mov. Disord. doi: 10.1002/mds.28320
Smith, K. M., Xie, S. X., and Weintraub, D. (2016). Incident impulse control disorder symptoms and dopamine transporter imaging in Parkinson disease. J. Neurol. Neurosurg. Psychiatry 87, 864–870. doi: 10.1136/jnnp-2015-311827. [Epub ahead of print].
Stark, A. J., Smith, C. T., Lin, Y.-C., Petersen, K. J., Trujillo, P., van Wouwe, N. C., et al. (2018). Nigrostriatal and mesolimbic D2/3 receptor expression in Parkinson’s disease patients with compulsive reward-driven behaviors. J. Neurosci. 38, 3230–3239. doi: 10.1523/JNEUROSCI.3082-17.2018
Steeves, T. D. L., Miyasaki, J., Zurowski, M., Lang, A. E., Pellecchia, G., Van Eimeren, T., et al. (2009). Increased striatal dopamine release in Parkinsonian patients with pathological gambling: a [11C] raclopride PET study. Brain 132, 1376–1385. doi: 10.1093/brain/awp054
Summerson, S. R., Aazhang, B., and Kemere, C. T. (2014). Characterizing motor and cognitive effects associated with deep brain stimulation in the GPi of hemi-Parkinsonian rats. IEEE Trans. Neural Syst. Rehabil. Eng. 22, 1218–1227. doi: 10.1109/TNSRE.2014.2330515
Tedford, S. E., Persons, A. L., and Napier, T. C. (2015). Dopaminergic lesions of the dorsolateral striatum in rats increase delay discounting in an impulsive choice task. PLoS One 10:e0122063. doi: 10.1371/journal.pone.0122063
Tessitore, A., De Micco, R., Giordano, A., di Nardo, F., Caiazzo, G., Siciliano, M., et al. (2017a). Intrinsic brain connectivity predicts impulse control disorders in patients with Parkinson’s disease. Mov. Disord. 32, 1710–1719. doi: 10.1002/mds.27139
Tessitore, A., Santangelo, G., De Micco, R., Giordano, A., Raimo, S., Amboni, M., et al. (2017b). Resting-state brain networks in patients with Parkinson’s disease and impulse control disorders. Cortex 94, 63–72. doi: 10.1016/j.cortex.2017.06.008
Thomas, A., Bonanni, L., Gambi, F., Di Iorio, A., and Onofrj, M. (2010). Pathological gambling in Parkinson disease is reduced by amantadine. Ann. Neurol. 68, 400–404. doi: 10.1002/ana.22029
Tremblay, M., Barrus, M. M., Cocker, P. J., Baunez, C., and Winstanley, C. A. (2019). Increased motor impulsivity in a rat gambling task during chronic ropinirole treatment: potentiation by win-paired audiovisual cues. Psychopharmacology 236, 1901–1915. doi: 10.1007/s00213-019-5173-z
Tremblay, M., Silveira, M. M., Kaur, S., Hosking, J. G., Adams, W. K., Baunez, C., et al. (2017). Chronic D2/3 agonist ropinirole treatment increases preference for uncertainty in rats regardless of baseline choice patterns. Eur. J. Neurosci. 45, 159–166. doi: 10.1111/ejn.13332
van der Vegt, J. P. M., Hulme, O. J., Zittel, S., Madsen, K. H., Weiss, M. M., Buhmann, C., et al. (2013). Attenuated neural response to gamble outcomes in drug-naive patients with Parkinson’s disease. Brain 136, 1192–1203. doi: 10.1093/brain/awt027
Verholleman, A., Victorri-Vigneau, C., Laforgue, E., Derkinderen, P., Verstuyft, C., and Grall-Bronnec, M. (2020). Naltrexone use in treating hypersexuality induced by dopamine replacement therapy: impact of OPRM1 A/G polymorphism on its effectiveness. Int. J. Mol. Sci. 21:3002. doi: 10.3390/ijms21083002
Voon, V., Rizos, A., Chakravartty, R., Mulholland, N., Robinson, S., Howell, N. A., et al. (2014). Impulse control disorders in Parkinson’s disease: decreased striatal dopamine transporter levels. J. Neurol Neurosurg. Psychiatry 85, 148–152. doi: 10.1136/jnnp-2013-305395
Vriend, C., Gerrits, N. J. H. M., Berendse, H. W., Veltman, D. J., van den Heuvel, O. A., and van der Werf, Y. D. (2015). Failure of stop and go in de novo Parkinson’s disease–a functional magnetic resonance imaging study. Neurobiol. Aging 36, 470–475. doi: 10.1016/j.neurobiolaging.2014.07.031
Vriend, C., Nordbeck, A. H., Booij, J., van der Werf, Y. D., Pattij, T., Voorn, P., et al. (2014). Reduced dopamine transporter binding predates impulse control disorders in Parkinson’s disease. Mov. Disord. 29, 904–911. doi: 10.1002/mds.25886
Weintraub, D., Koester, J., Potenza, M. N., Siderowf, A. D., Stacy, M., Voon, V., et al. (2010a). Impulse control disorders in Parkinson disease: a cross-sectional study of 3090 patients. Arch. Neurol. 67, 589–595. doi: 10.1001/archneurol.2010.65
Weintraub, D., Papay, K., Siderowf, A. and Parkinson’s Progression Markers Initiative (2013). Screening for impulse control symptoms in patients with de novo Parkinson disease: a case-control study. Neurology 80, 176–180. doi: 10.1212/WNL.0b013e31827b915c
Weintraub, D., Sohr, M., Potenza, M. N., Siderowf, A. D., Stacy, M., Voon, V., et al. (2010b). Amantadine use associated with impulse control disorders in Parkinson disease in cross-sectional study. Ann. Neurol. 68, 963–968. doi: 10.1002/ana.22164
Winstanley, C. A. (2011). The utility of rat models of impulsivity in developing pharmacotherapies for impulse control disorders. Br. J. Pharmacol. 164, 1301–1321. doi: 10.1111/j.1476-5381.2011.01323.x
Ye, Z., Altena, E., Nombela, C., Housden, C. R., Maxwell, H., Rittman, T., et al. (2014). Selective serotonin reuptake inhibition modulates response inhibition in Parkinson’s disease. Brain 137, 1145–1155. doi: 10.1093/brain/awu032
You, H., Mariani, L.-L., Mangone, G., Le Febvre de Nailly, D., Charbonnier-Beaupel, F., and Corvol, J.-C. (2018). Molecular basis of dopamine replacement therapy and its side effects in Parkinson’s disease. Cell Tissue Res. 373, 111–135. doi: 10.1007/s00441-018-2813-2
Zainal Abidin, S., Tan, E. L., Chan, S.-C., Jaafar, A., Lee, A. X., Abd Hamid, M. H. N., et al. (2015). DRD and GRIN2B polymorphisms and their association with the development of impulse control behaviour among Malaysian Parkinson’s disease patients. BMC Neurol. 15:59. doi: 10.1186/s12883-015-0316-2
Zengin-Toktas, Y., Authier, N., Denizot, H., Chassain, C., Hafidi, A., Llorca, P. M., et al. (2013). Motivational properties of D2 and D3 dopamine receptors agonists and cocaine, but not with D1 dopamine receptors agonist and l-dopa, in bilateral 6-OHDA-lesioned rat. Neuropharmacology 70, 74–82. doi: 10.1016/j.neuropharm.2012.12.011
Keywords: impulse control disorders, rodent models, dopamine agonist, non-motor symptoms, Parkinson’s disease
Citation: Augustine A, Winstanley CA and Krishnan V (2021) Impulse Control Disorders in Parkinson’s Disease: From Bench to Bedside. Front. Neurosci. 15:654238. doi: 10.3389/fnins.2021.654238
Received: 15 January 2021; Accepted: 22 February 2021;
Published: 12 March 2021.
Edited by:
Gianfranco Spalletta, Santa Lucia Foundation (IRCCS), ItalyReviewed by:
Francesca Assogna, Santa Lucia Foundation (IRCCS), ItalyCarla Masala, University of Cagliari, Italy
Copyright © 2021 Augustine, Winstanley and Krishnan. This is an open-access article distributed under the terms of the Creative Commons Attribution License (CC BY). The use, distribution or reproduction in other forums is permitted, provided the original author(s) and the copyright owner(s) are credited and that the original publication in this journal is cited, in accordance with accepted academic practice. No use, distribution or reproduction is permitted which does not comply with these terms.
*Correspondence: Andrea Augustine, YWExMDBAcmljZS5lZHU=; YW5kcmVhYXVnQGdtYWlsLmNvbQ==