- 1Department of Pharmacy and Biomedical Sciences, La Trobe Institute for Molecular Science, La Trobe University, Bendigo, VIC, Australia
- 2Department of Physiology, School of Biomedical Sciences, The University of Melbourne, Melbourne, VIC, Australia
The impact of brown adipose tissue (BAT) metabolism on understanding energy balance in humans is a relatively new and exciting field of research. The pathogenesis of obesity can be largely explained by an imbalance between caloric intake and energy expenditure, but the underlying mechanisms are far more complex. Traditional non-selective sympathetic activators have been used to artificially elevate energy utilization, or suppress appetite, however undesirable side effects are apparent with the use of these pharmacological interventions. Understanding the role of BAT, in relation to human energy homeostasis has the potential to dramatically offset the energy imbalance associated with obesity. This review discusses paradoxical effects of caffeine on peripheral adenosine receptors and the possible role of adenosine in increasing metabolism is highlighted, with consideration to the potential of central rather than peripheral mechanisms for caffeine mediated BAT thermogenesis and energy expenditure. Research on the complex physiology of adipose tissue, the embryonic lineage and function of the different types of adipocytes is summarized. In addition, the effect of BAT on overall human metabolism and the extent of the associated increase in energy expenditure are discussed. The controversy surrounding the primary β-adrenoceptor involved in human BAT activation is examined, and suggestions as to the lack of translational findings from animal to human physiology and human in vitro to in vivo models are provided. This review compares and distinguishes human and rodent BAT effects, thus developing an understanding of human BAT thermogenesis to aid lifestyle interventions targeting obesity and metabolic syndrome. The focus of this review is on the effect of BAT thermogenesis on overall metabolism, and the potential therapeutic effects of caffeine in increasing metabolism via its effects on BAT.
Introduction
Increases in the prevalence of overweight and obesity are a major health problem in people of all ages (Engin, 2017), with considerable concern in the rise of metabolic syndrome in children and adolescents (Gepstein and Weiss, 2019). Obesity is the accumulation of excess adipose tissue that has adverse impacts on physical healthy lifestyle patterns (Block et al., 2004; Drewnowski and Specter, 2004; Boardman et al., 2005; Abell et al., 2007; Gibson, 2011; Corral et al., 2012; Quick et al., 2013; Wang et al., 2013), and is positively correlated with poor health outcomes in cardiovascular disease, diabetes, cancer, and an array of musculoskeletal disorders (Anandacoomarasamy et al., 2008; Dehal et al., 2011; Liu et al., 2011; Willey et al., 2011; Ma et al., 2012; Vranian et al., 2012; Zhang and Rodriguez-Monguio, 2012). In addition to these chronic diseases, obesity is a major risk factor for higher risk of mortality due to COVID-19 (Pettit et al., 2020).
The development of obesity can largely be explained by an imbalance between caloric intake and total metabolic activity (Schwartz et al., 2017). Lifestyle interventions consisting of diet and exercise are common treatments for obesity, premised on the mistaken assumption that correcting the energy imbalance will lead to weight loss. However, there is a growing body of evidence suggesting that simple caloric restriction and exercise are insufficient on their own to promote and maintain weight loss (Greenway, 2015; Vettor et al., 2020). A lack of effective options for long-term weight reduction and subsequent weight maintenance exacerbates the enormity of the obesity problem; individuals who successfully complete behavioral and dietary weight-loss programs eventually regain most of the lost weight. A meta-analysis of 29 long term weight loss studies show that within 2 years more than half of the lost weight is regained, and by 5 years more than 80% of the lost weight is regained (Anderson et al., 2001). Consequently, identifying pharmacological therapies that may promote weight loss, through increased energy expenditure via thermogenesis, may be a way to augment current interventions for long term weight reduction (Ursino et al., 2009; Nedergaard and Cannon, 2010; Dulloo, 2011; Whittle et al., 2013).
Although the pathogenesis of obesity is far from fully understood, a large amount of effort is being undertaken to ameliorate obesity itself. Understanding the role of brown adipose tissue (BAT), in relation to human energy homeostasis and the potential to pharmacologically increase metabolism is an exciting and active research interest. Early estimates have suggested that increasing BAT thermogenesis in 50 g of BAT can increase metabolism by as much as 25% (Brooks et al., 2005). White adipose tissue (WAT) is distinctly different from BAT both in terms of physiology and embryonic development (Lee et al., 2013b). In simple terms, WAT stores energy, and BAT dissipates energy and releases heat (Cannon and Nedergaard, 2004). Surprisingly, in terms of development, BAT is closer in lineage to skeletal muscle rather than adipose tissue (Timmons et al., 2007; Cannon and Nedergaard, 2008; Seale et al., 2008; Kajimura et al., 2010; Lepper and Fan, 2010; Petrovic et al., 2010), with a large amount of mitochondria located in both BAT and skeletal muscle compared with WAT (Porter et al., 2016). This allows for the oxidative demand and heat production of activated BAT (Porter et al., 2016).
Activation of BAT thermogenesis is regulated by the sympathetic nervous system through β-adrenoreceptors in rodents, and is presumed to also be the case in humans (Cannon and Nedergaard, 2004). The neural pathway that controls the regulation of BAT thermogenesis has been thoroughly investigated and described in rats (Morrison and Nakamura, 2011). While there is overlap (Nazari et al., 2020), the neural pathway controlling the cardiovascular system appears to be different to that which controls thermoregulatory reflexes (Rathner et al., 2008). Sympathetic premotor neurons in the rostral raphe pallidus neurons regulate sympathetic outflow to BAT (Morrison, 2016) and are likely only weakly baroreceptor sensitive (Rathner et al., 2001), whereas the premotor neurons in the rostral ventrolateral medulla mediates baroreflex related sympathetic discharge (Morrison, 1999). Understanding the role of the different neural nuclei within the BAT regulatory pathway, particularly within the hypothalamus, is paramount to identifying pharmacological substances that may selectively activate BAT thermogenesis and increase energy expenditure, without necessarily altering whole body homeostasis.
Caffeine is the psycho-stimulant component of coffee, other beverages and various supplements (e.g., certain pre-workout powders) (Smith, 2002). There is ample evidence that caffeine increases thermogenesis acutely in both rodents and humans (Trimble, 1963; Velickovic et al., 2019), however the direct mechanism is unclear. Certain studies investigating the thermogenic and metabolic effects of caffeine have focused on caffeine acting directly on the BAT tissue at high doses (Astrup et al., 1990). However, these previous studies fail to consider that caffeine acts centrally to increase arousal (Ferre, 2010). This raises the possibility that previously reported caffeine evoked thermogenesis may have a central component, through acting on the thermoregulatory pathway underlying BAT thermogenesis. It remains uncertain if low, non-anxiogenic doses of caffeine, which activate arousal pathways centrally, are sufficient to activate thermogenesis. The focus of this review is on the effect of BAT thermogenesis on overall metabolism, and the potential therapeutic effects of caffeine in increasing metabolism via its effects on BAT.
Therapeutic and Thermogenic Effects of Caffeine
Caffeine is a purine alkaloid (Ashihara et al., 2008) found in many food products and beverages and is likely the most widely consumed psycho-active drug worldwide being the psycho-stimulant component of coffee and other beverages and supplements (Smith, 2002). Caffeine has a number of physiological effects including increases in human endurance, physical performance, cognition, resting energy expenditure, and improvements in behavioral functions such as mood (Glade, 2010). Previous studies have demonstrated therapeutic effects of caffeine in metabolic parameters, hypertension, and hepatic fibrosis, which are components of metabolic syndrome. Caffeine (0.1% in drinking water) has been shown to decrease insulin concentrations and plasma glucose in rats fed high-fat and high-sucrose diets, respectively, with a decrease of mean arterial pressure in the two pathological models (Conde et al., 2012). Additionally, caffeine consumption attenuated weight gain adiposity in rats fed a high-fat diet (Conde et al., 2012). In human patients undergoing liver biopsy for clinical indications, a higher daily caffeine consumption is associated with a decrease in the severity of liver fibrosis (Modi et al., 2010).
Caffeine contained in coffee has been shown to induce lipolysis, thermogenesis, insulin secretion, and fat oxidation in both non-obese and obese humans (Astrup et al., 1990; Bracco et al., 1995; Acheson et al., 2004). In fact, consuming six cups of coffee (600 mg) within 12 h would be expected to induce a 100 kcal increase in daily energy expenditure (Dulloo et al., 1989). However, to date, there is little evidence that coffee consumption promotes significant weight loss in humans. This appears to be due to habituation to caffeine-induced catecholamine responses and lipolysis with prolonged use (Dekker et al., 2007).
Caffeine has been administered therapeutically in combination with ephedrine (mixed α and β-adrenoceptor agonist) to increase metabolism and activate BAT thermogenesis in rodents (Kim et al., 2011). Ephedrine itself has been shown to activate BAT thermogenesis in lean humans acutely but not in obese humans (Carey et al., 2013, 2015). However, both of these drugs have notable side effects, and caffeine at high doses is known to have significant adverse cardiovascular effects on heart rate and blood pressure (Hoehn-Saric and McLeod, 2000). Within the central nervous system, caffeine acts as an non-specific adenosine receptor antagonist (Ribeiro and Sebastiao, 2010). Previous research investigating caffeine’s physiological effects have generally focused on peripheral mechanisms (Astrup et al., 1990; Velickovic et al., 2019). However, in terms of arousal, caffeine’s effect is central (Ferre, 2010), suggesting that caffeine evoked thermogenesis may have a central component. In rodent studies, low (but stimulatory) doses of caffeine have previously activated orexinergic neurons in the dorsomedial hypothalamus and the perifornical areas of the lateral hypothalamus (Murphy et al., 2003; Sakurai, 2007). These regions of the hypothalamus have been shown to regulate sympathetic nerve activity to interscapular BAT, leading to thermogenesis in rodents (Tupone et al., 2011).
Caffeine works centrally to promote arousal through antagonism of the adenosine A1A receptor (Murphy et al., 2003), which releases orexinergic neurons from inhibition (Murphy et al., 2003). This suggests that central effects of caffeine on BAT thermogenesis are through its antagonistic action on the A1A receptor (Table 1). This observation is harmonious with a role of orexin/hypocretin as a key neuropeptide in the regulation of energy homeostasis and the sleep-wake cycle (Sakurai, 2007). It has been demonstrated that there is an increase in both brain adenosine and A1A receptor in the hypothalamus associated with the development of obesity in mice (Wu et al., 2017). Finally, antagonism of A1A receptor with systemic caffeine has been shown to reduce body weight, increase BAT thermogenesis and increase oxygen consumption in high fat diet-induced obese rats (Wu et al., 2017).
The signaling of adenosine is transmitted via adenosine A1 and A3 receptors through Gi/Go family or by A2A and A2B receptors via Gs family G proteins (Table 1; Fredholm et al., 2011). The Gi/Go family proteins inhibit adenylate cyclase (Francken et al., 1998) [which synthesizes cyclic adenosine monophosphate (cAMP) from ATP (Rivera-Oliver and Díaz-Ríos, 2014)], and Gs family stimulate adenylate cyclase (Graziano et al., 1987) thereby modulating the amount of cAMP available for downstream intracellular signaling. Activation of A1 or A3 receptors work through Gi/Go family (Table 1) and lower cAMP whereas A2A or A2B work through Gs and increase cAMP (Cully, 2014). Lipolysis in WAT is inhibited via activation of A1A receptors (Johansson et al., 2008) which are abundantly expressed on WAT cells and act via Gi/Go family to reduce cAMP (Trost and Schwabe, 1981; Mersmann et al., 1997; Tatsis-Kotsidis and Erlanger, 1999). Increases in intracellular cAMP promote lipolysis (Arner, 1976). Furthermore, adenosine A2A and A2B receptor agonists increase lipolysis and BAT thermogenesis (Gnad et al., 2014), whereas adenosine A1A receptor antagonism promotes increases in heart rate and oxygen consumption. Expression of A2A receptors is increased in cold-exposed mice as well as in brown adipocytes in response to norepinephrine or intracellular cAMP (Gnad et al., 2014). As caffeine is a non-specific adenosine receptor antagonist it may influence multiple peripheral mechanisms that act paradoxically upon adipose tissue and thermogenesis. As such there remains potential to investigate effects of combination therapy of caffeine and selective adenosine A2A or A2B agonists on thermogenesis. Such a combination therapy may increase the efficacy of caffeine.
In addition to antagonizing adenosine receptors, caffeine is a non-selective phosphodiesterase inhibitor (Moustafa and Feldman, 2014). Phosphodiesterase converts cAMP to adenosine monophosphate, thus caffeine’s action on phosphodiesterase increases intracellular cAMP (Boswell-Smith et al., 2006). Raising cellular cAMP could possibly be a peripheral mechanism by which caffeine increases UCP1 (uncoupling protein 1, which facilitates a futile cycle in the mitochondria of brown/beige adipocytes) activity (Fukano et al., 2016). β3-adrenoceptors are G protein coupled receptors linked to Gs proteins. Activation of β3-adrenoceptors leads to increased cAMP production, which in turn activates protein kinase A, which stimulates lipolysis, releasing free fatty acids and activating UCP1 (Himms-Hagen, 1989; Cannon and Nedergaard, 2004). Additional experiments investigating the effects of protein kinase A inhibition or cAMP concentration may provide further information on the role of cAMP levels in the caffeine response. As such there remains potential to combine stimulatory doses of caffeine with β3-adrenoceptor agonists.
Intake of caffeine improves fructose-induced insulin resistance and hypertension by enhancing central insulin signaling in rats (Yeh et al., 2014). This is through enhancing insulin sensitivity within the nucleus of the solitary tract to prevent hypertension by increasing nitric oxide production. Adenosine A2A receptor signaling pathway in the nucleus of the solitary tract mediates nitric oxide production related with the control of blood pressure (Ho et al., 2008). This is important because the nucleus of the solitary tract has received considerable attention for its role in the regulation of metabolism and body temperature (Székely, 2000; Cao et al., 2010; Grill and Hayes, 2012). Activation of the adenosine A1A receptor within the neurons of the nucleus of the solitary tract leads to a hypometabolic state, inhibiting BAT thermogenesis, reducing energy expenditure and inhibiting shivering thermogenesis in rats (Tupone et al., 2013). Suggesting that chronic activation of adenosine A1A receptors (lowering cAMP levels) in the nucleus of the solitary tract can reduce energy expenditure and induce a metabolic imbalance potentially pre-disposing individuals to obesity and metabolic disease. As caffeine is an antagonist at the adenosine A1A receptor, perhaps these effects may be attenuated or reversed with caffeine treatment. Interestingly, chronic caffeine consumption diminishes diabetic symptoms by increasing insulin sensitivity via more efficient insulin signaling (Park et al., 2007). Moreover, caffeine alleviates fructose-induced metabolic disturbances in rats (Yeh et al., 2014). However, several researchers have concluded that caffeine consumption may increase blood pressure, thus leading to an elevated risk of hypertension (Savoca et al., 2004; Noordzij et al., 2005). Conversely, most epidemiological studies have shown that the habitual intake of caffeinated coffee does not increase the risk of hypertension, (Savoca et al., 2004; Geleijnse, 2008) and chronic caffeine intake has been found to prevent diet-induced hypertension in rats (Conde et al., 2012). These observations suggest a central mechanism for caffeine evoked BAT thermogenesis and increases in energy expenditure, that have possible applications in the clinical care of individuals with metabolic disease, hypertension, and other obesity related diseases.
Physiology of Adenosine Receptors – As a Mechanistic Understanding of the Therapeutic Effects of Caffeine
The purinergic nucleoside signaling molecule adenosine is found within the sympathetic nervous system (Gourine et al., 2009) and in the central nervous system, and is both a precursor and breakdown product of adenosine-triphosphate (Abbracchio et al., 2009). The A2A receptor is highly expressed on BAT and appears to have a major role in BAT activation and recruitment of brown adipocytes (Gnad et al., 2014) which involves the proliferation and differentiation of precursor cells, and also hypertrophy of mature brown adipocytes. A2A receptor agonists increase lipolysis in both murine and human brown adipocytes (Gnad et al., 2014) as downstream Gs family G proteins will be activated raising cellular cAMP. Additionally, these same A2A receptor agonists improved glucose tolerance, increased energy expenditure, increased [18F]fluorodeoxyglucose (FDG) uptake in BAT, induced recruitment of brown adipocytes and protected mice from diet-induced obesity (Gnad et al., 2014). These findings suggest promising thermogenic effects of adenosine via direct action on BAT. Previous studies of the effects of adenosine on brown adipocytes show lipolysis is inhibited in rodents after exposure to an A1A adenosine receptor agonist (Woodward and Saggerson, 1986; Table 1).
The Complex Physiology of Adipose Tissue
“Adipose tissues” found in mammals contain three types of adipocytes (white, brown, and beige/brite) and are categorized into two types of tissue (WAT and BAT) (Lee et al., 2013b; Wu and Spiegelman, 2013). White adipocytes predominantly make up WAT, with a smaller number of beige adipocytes mixed in Lee et al. (2013b), and BAT being predominantly brown adipocytes (Lidell et al., 2013; Rosenwald and Wolfrum, 2014). Some tissue plasticity occurs because in humans these brown adipocytes seem to be displaced by white adipocytes under conditions of aging (Contreras et al., 2014; Zoico et al., 2019). However, it is unclear whether these are truly white adipocytes or if there is some form of reverse browning. While historically WAT was considered simply as a store of energy (in the form of triglycerides), it is now known that it can also act as an endocrine organ and secrete adipokines (Coelho et al., 2013). Adipokines are pro-inflammatory chemical signaling molecules secreted by adipose tissue (Mancuso, 2016). In obese patients, these adipokines contribute to low-grade systemic inflammation (Tilg and Moschen, 2006). Progranulin, lipocalin−2, adiponectin, and leptin are adipokines which link obesity to the immune system, and are potential therapeutic targets in obesity-related diseases, such as diabetes mellitus (Reinehr and Roth, 2018), rheumatoid arthritis (Carrion et al., 2019), and osteoarthritis (Tu et al., 2019).
Each class of adipocyte is functionally, biochemically, and morphologically distinct. Beige adipocytes are typically dispersed in WAT (Zoico et al., 2019) and, inactivated, can look indistinguishable from their white adipocyte neighbors. However, sympathetic activity, can induce these cells to “brown” (Young et al., 1984; Sidossis et al., 2015), which is associated with increased whole-body metabolic rate (Sidossis et al., 2015), and this browning of WAT is attenuated in β3-adrenoceptor knockout mice (Jimenez et al., 2003). Browning is a term that describes the emergence of beige adipocytes in WAT and is a reversible process which represents adaptation to increased thermogenic demand. Morphological differences are easily observed between BAT and WAT, but are less obvious between white adipocytes and beige adipocytes (Ikeda et al., 2018). White adipocytes have a large single lipid droplet, containing energy stored as triglycerides, with the nucleus displaced to the periphery of the cell (Cinti, 2006). Similar to white adipocytes, beige adipose tissue are unilocular, however have smaller and multiple lipid droplets (Park et al., 2014). In contrast, brown adipocytes typically have a polygonal shape, and multilocular lipid droplets (de Jong et al., 2019).
The embryonic development of adipocytes, both white and brown, is common with skeletal muscle, other connective tissues, and bone that are all generally accepted to arise from progenitor cells of the mesoderm (Gesta et al., 2007; Billon et al., 2008). Several studies indicate that the formation of BAT shares a closer relationship with skeletal muscle, rather than WAT (Timmons et al., 2007; Cannon and Nedergaard, 2008; Seale et al., 2008; Kajimura et al., 2010; Lepper and Fan, 2010; Petrovic et al., 2010). White adipocytes derive from lateral mesoderm, and are myf-5 negative precursor cells (Sanchez-Gurmaches and Guertin, 2014). This contrasts with brown adipocytes which derive from myf-5 positive precursor cells of the paraxial mesoderm (Sanchez-Gurmaches and Guertin, 2014; Ikeda et al., 2018). Myf-5 is a necessary gene transcription regulator for the development of myoblast (Ustanina et al., 2007). Cold exposure is known to induce proliferation of rodent vascular endothelial cells and pre-adipocytes (Bukowiecki et al., 1986; Geloen et al., 1992; Klingenspor, 2003; Lee et al., 2015) in BAT. The pre-adipocytes differentiate into mature brown adipocytes, resulting in BAT hyperplasia and enhanced BAT function. It is interesting to speculate that in humans, in our centrally heated houses we may keep newborns too warm, perhaps providing an environmental setting reducing brown adipocyte proliferation contributing to the emergence of obesity and type II diabetes in adolescents. Consistent with this, rat pups reared for 8 weeks in cool environment (18°C) and then housed in a thermoneutral environment for 4 weeks had 15% greater interscapular BAT mass than rats reared in 30°C environment prior to housing at thermoneutrality (Morrison et al., 2000). Additionally, rodent mature brown adipocytes have the ability to proliferate upon the activation of β3-adrenoceptor, as increases in the number of brown adipocytes expressing UCP1 following cold exposure (Fukano et al., 2016). This is confirmed as inhibition of β3-adrenoceptor with an antagonist (SR59230A, 1 mg/kg) reduces the number of proliferating brown adipocytes during cold exposure of 10°C (Fukano et al., 2016).
Both rodent and human brown adipocytes contain large numbers of mitochondria (Porter et al., 2016), and therefore skeletal muscle and BAT have similar oxidative capacities (Porter et al., 2016). Furthermore, compared to WAT, BAT is also highly vascularized in humans, this allows for the oxidative demand and heat dissipation into the body (Cypess et al., 2009).
Effect of Bat Thermogenesis on Metabolism
The finding that BAT activity (Vijgen et al., 2011; Madden and Morrison, 2016; Loh et al., 2017) and volume (Leitner et al., 2017) is inversely related with adiposity in adult humans (Wang et al., 2015) has encouraged the assessment of the role of BAT in metabolic regulation. There is evidence that the metabolic activity of BAT can offset some of the energy imbalance associated with weight gain. Estimates of energy consumption are made on the basis of some experimentally derived assumptions (Carpentier et al., 2018), as indicated in Table 2, suggest that BAT thermogenesis can contribute up to 10% of resting energy expenditure. This is consistent with measured increases in energy expenditure in humans after β3-adrenoceptor activation (Cypess et al., 2015; O’Mara et al., 2020). Interestingly, comparisons between summer and winter seasons in adult humans following mild cold exposure (15°C) for 3 h, shows an increase in non-shivering thermogenesis and resting metabolic rate by 7% in summer and 11.5% in winter (van Ooijen et al., 2004). While increasing non-shivering thermogenesis does not exactly mean increasing BAT thermogenesis, it is certainly a component. The higher metabolic response in winter compared with summer indicates cold acclimatization, that is, adaption of non-shivering thermogenesis over time. This suggests that acute studies on BAT may not be sensitive enough to detect a maximal BAT response as environmental conditions may impact on the total measured energy consumption. Studies investigating a thermogenic response following chronic exposure to a treatment may yield compounded results over time.
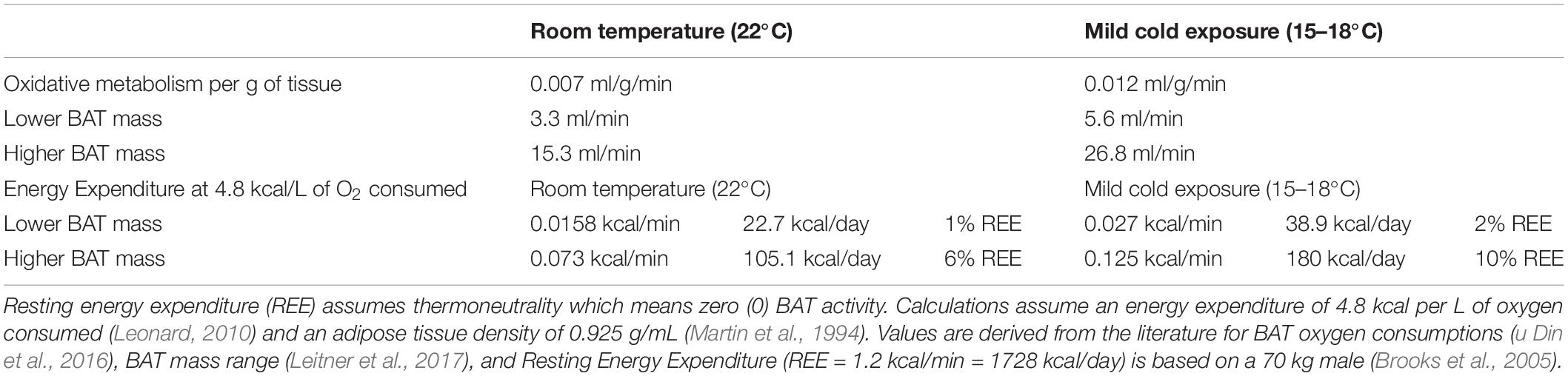
Table 2. Brown adipose tissue (BAT) oxidative metabolism and contribution to total body energy expenditure.
Clear evidence of the mechanism for BAT activation in humans remains to be determined. Increases in the thermogenic capacity of humans and rodents can occur in response to repeated or long term exposure to cold stimuli or pharmacological activation with β3-adrenergic receptor agonists in vivo (Cousin et al., 1992; Yoneshiro et al., 2013; Cypess et al., 2015; Hanssen et al., 2016; Finlin et al., 2018; O’Mara et al., 2020). Part of the increased thermogenic capacity is due to the browning of WAT (Contreras et al., 2016). Therefore, prolonging the activation of BAT can increase BAT mass (Cannon and Nedergaard, 2004), resulting from proliferation and hypertrophy of beige adipocytes which trigger browning of WAT (Wankhade et al., 2016).
Obesity results in increased storage of triglycerides in both skeletal muscle cells (Goodpaster et al., 2000) and brown adipocytes (Shimizu et al., 2014). The increased storage of triglycerides has negative effects on lipolysis and inhibits glucose metabolism, leading to reduced muscle (and BAT) glucose utilization (Boden et al., 2001; Boden, 2003, 2006). Activation of BAT utilizes free fatty acids as a substrate (Penicaud et al., 2000), thus increasing fat catabolism. Prolonged BAT activation in humans improves insulin sensitivity (Finlin et al., 2018), glucose metabolism and insulin secretion (O’Mara et al., 2020). Increases in circulating plasma free fatty acids results in impaired insulin secretion (Kashyap et al., 2003), and insulin resistance (Boden et al., 2001; Boden, 2006), thereby increasing blood glucose levels. Therefore, activation of BAT and beige adipocytes have the potential to address the lipid and glucose imbalance associated with metabolic disorders in humans such as type 2 diabetes mellitus with significant implications for health care.
The Primary β-Adrenoreceptor Involved in Human Bat Activation (β1, β2, or β3)
Sympathetic nervous system activity, via β-adrenergic receptors is a significant regulator of BAT thermogenesis (Cannon and Nedergaard, 2004). The primary β-adrenoreceptor involved in human BAT activation remains contentious. In rodents, BAT is primarily induced through β3-adrenoreceptor stimulation (Bachman et al., 2002) although β1-adrenoceptors can provide a functionally compensatory mechanism in β3-adrenoceptor knockout mice (Chernogubova et al., 2005). β3-adrenoceptors were also thought to be the primary adrenoceptor of human brown adipocytes (Cypess et al., 2015). In vivo treatment with the β3-agonist mirabegron [approved for human use in the treatment of overactive bladder (Khullar et al., 2013)] at a dose of 200 mg, four times the recommend therapeutic dose, increases whole body energy expenditure and BAT thermogenesis as detected by [18F] fluorodeoxyglucose (18 FDG, glucose PET analog) uptake (Cypess et al., 2015; Baskin et al., 2018; O’Mara et al., 2020). However, these doses are accompanied by significant increases in heart rate and mean arterial pressure (Cypess et al., 2015; Baskin et al., 2018; Finlin et al., 2018; O’Mara et al., 2020), possibly implying that at such doses mirabegron is not selective to β3-adrenoceptors. Mirabegron has 446 times higher affinity for β3 than β1 or β2-adrenoceptor subtype (Takasu et al., 2007).
Since the β3-adrenoreceptor is highly expressed in few organs, such as the urinary bladder, gall bladder, and BAT and, significantly, is absent from blood vessels, specifically targeting the β3-adrenoreceptor may be an attractive option for therapeutically augmenting non-shivering thermogenesis, as it would be expected that there may be limited adverse effects (Schena and Caplan, 2019). Evidence for a physiological effect of β3-adrenergic receptor activity on the human heart is still being debated (Berkowitz et al., 1995; Gauthier et al., 1996, 1999; Schena and Caplan, 2019). β3 adrenergic receptors decrease the force of contraction of the ventricles (Tavernier et al., 2003) and promote release of nitric oxide (Calvert et al., 2011), which in turn may have positive impact on cardiac health.
Other β3-adrenoceptor agonists that show effects in rodents, have limited effects when tested on humans (Weyer et al., 2008). Various β3-adrenoreceptor agonists, such as L-796568 (Larsen et al., 2002), ZD7114 and ZD2079 (Buemann et al., 2000), Ro 40-2148 (Jequier et al., 1992), and BRL 26830 (Connacher et al., 1988, 1992) have been used in clinical trials to test for efficacy to induce weight loss, but have produced limited effects. Furthermore, unforeseen effects such as tremor, and tachycardia were observed (Connacher et al., 1990), suggestive of potential β1- and β2-adrenoceptor co-activation. The β3-adrenoceptor is expressed in both white and brown adipocytes in both rodents and humans (Chamberlain et al., 1999; Schena and Caplan, 2019), however in humans β3-adrenoceptor mRNA levels are much lower (Schena and Caplan, 2019; Blondin et al., 2020). While in vitro analysis of brown adipocytes reveals that β1-adrenoreceptors are the predominant adrenoreceptor in both an immortalized cell line and human BAT biopsies (Riis-Vestergaard et al., 2020). Furthermore, immunohistochemical analysis identifies β3-adrenoceptors in intact human adipocytes and ventricular myocardium, consistent with evidence that β3-adrenoceptors can mediate lipolysis in human white adipocytes (De Matteis et al., 2002), and a negative inotropic effect within the ventricular myocardium (Gauthier et al., 2000). Thus, in contrast to rodent BAT the adrenergic receptors involved in humans are still being debated. These differences between species may be due to multiple factors, such as differences in the ligand-binding pocket in the β3-adrenoceptor, expression patterns of β3-adrenoceptor or cross-reactivity with the β1-adrenoceptor causing cardiovascular adverse effects in humans, or bioavailability (Arch, 2011; Dehvari et al., 2018; Loh et al., 2019).
In humans, BAT activation can be inhibited with treatment of the β-adrenergic antagonist propranolol (80 mg), at doses typically used to inhibit β1-adrenoceptors (Soderlund et al., 2007). Propranolol is a significantly weaker antagonist of β3- than β1- or β2-adrenoceptors (Baker, 2005). The implication of this, is that human BAT thermogenesis may be mediated through β1- or β2-adrenoceptors, even though β3-adrenoceptors are present. However, whether the doses of propranolol used were high enough to block not only β1- but also β3-adrenoceptors or if human BAT is stimulated mainly via β1-adrenoceptors, is not clarified in this study (Soderlund et al., 2007). Propranolol has higher selectivity for β2- rather than β1-adrenoceptors (Baker, 2005), raising the possibility that it is the β2- not β1-adrenoceptor largely involved in this response. These questions can largely be addressed, as there are specific β1-adrenoceptor antagonists, such as CGP 20712A, which has ∼1,000–10,000-fold selectivity for the β1-adrenoceptor (pKB, ∼8.4–9.5) relative to the β2- (pKB ∼5.1) and β3-adrenoceptors (pKB ∼4.2–4.7) (Seifert, 2011).
In vitro analysis of brown adipocytes shows that UCP1 mRNA expression is raised 6 to 12 fold by dobutamine, a β1-adrenoceptor agonist, and eight fold by isoproterenol (a non-selective β-adrenoceptor agonist), whereas neither procaterol (β2-adrenoceptor agonist), CL314.432, or mirabegron (β3-adrenoceptor agonists) affected UCP1 (Riis-Vestergaard et al., 2020). Isoproterenol induced UCP1 mRNA expression is attenuated by 62.5%, whereas isoproterenol induced UCP1 mRNA levels were unaffected by siRNA silencing the β3-adrenoceptor (Riis-Vestergaard et al., 2020). Together these results suggest that adrenergic stimulation of UCP1 may mainly be mediated through β1-adrenoreceptors (Riis-Vestergaard et al., 2020), however, the role of β2-adrenoceptor was not assessed and requires clarification.
Formoterol is a highly subtype selective β2-adrenoceptor agonist with 646 times higher affinity for human β2 over β3, and 331 times higher affinity for β2 over β1-adrenoceptor (Baker, 2010). Brown adipocyte oxygen consumption was increased significantly by formoterol and knock down of the β2-adrenergic receptor impaired thermogenesis (Blondin et al., 2020). Formoterol promoted BAT thermogenesis in vitro, that could not be achieved using therapeutic doses of the β3 agonist mirabegron (50 mg), but was at 200 mg (Blondin et al., 2020). Increases in energy expenditure, fatty acid oxidation stimulation and thermogenesis in response to oral administration of formoterol (160 μg) (Lee et al., 2013a), are similar to what is observed with mirabegron (200 mg) (Blondin et al., 2020). However, the source of thermogenesis was not determined following administration of formoterol. Although, oral administration of formoterol increases energy expenditure by 13% and fat utilization by 23%, without inducing tachycardia, six out of eight participants reported palpitation, tremor, two lost appetite, and one experienced insomnia (Lee et al., 2013a).
While the findings from Blondin et al. (2020) provide evidence for β2-adrenergic stimulation of brown adipocytes in vitro, there is still a role for the other adrenoceptors. Chronic mirabegron [100 mg being the dose for maximal efficacy (Chapple et al., 2013)], treatment for 4 weeks increased BAT activity in healthy non-obese females (O’Mara et al., 2020). The initial dose of mirabegron on day 1 increased participants resting energy expenditure by 10.7%; interestingly the baseline resting energy expenditure on day 28 was 5.8% higher than the baseline resting energy expenditure prior to drug exposure (O’Mara et al., 2020). However, participants in this study served as their own control and showed acute increases in heart rate (6 beats per minute) and systolic blood pressure (8 mmHg) (O’Mara et al., 2020). Though, it should be clearly stated that these acute cardiovascular effects are not apparent following chronic (28 day) treatment of the drug (O’Mara et al., 2020). Similarly increases in systolic blood pressure of 10 mmHg and heart rate of 11 beats per minute, were observed following acute administration of mirabegron (200 mg) (Blondin et al., 2020). Although these increases in systolic blood pressure and heart rate are not seemingly large, a 10 mmHg increase in systolic blood pressure to a hypertensive patient may in fact be substantial and will likely reduce the use of mirabegron (100 mg) clinically in obese patients. As such, perhaps a future experiment administering mirabegron to patients on β1- and β2-adrenoceptor antagonists may ameliorate the previously reported cardiovascular effects. There is also potential for a more selective β3-agonist to improve these apparent adverse cardiovascular effects as mirabegron exerts a cardio-stimulant and cardio-depressant effect which is unrelated to β3-adrenoceptor activation (Mo et al., 2017). Additionally, a combination therapy of a β3-agonist with stimulatory, but non-anxiogenic doses of caffeine remains a possibility.
Nonetheless, it is possible human BAT express all three β-adrenoceptors subtypes or there is a bias in how a β-adrenoceptor behaves that influences its response. Surprisingly, there has been little work undertaken investigating the primary β-adrenoreceptor in human BAT. This is potentially due to a limited access of BAT biopsy material for both primary cell cultures and explant cultures (Lee et al., 2016). Further investigations using different β-adrenoceptor agonists in vivo or a combination of agonists and antagonists are needed to understand receptor composition of β-adrenoceptors in human BAT. There is much to be learned regarding pharmacological BAT activating agents that mimic the effects of cold exposure in humans, as whole body and BAT thermogenesis can both increase in response to cold stimuli, while heart rate decreases significantly (Cypess et al., 2012). In addition, increases in BAT glucose uptake and whole-body energy expenditure can occur independently of direct stimulation of BAT thermogenesis (Blondin et al., 2017).
Sympathetic Control of Bat
The regulation of sympathetic activity provides a complex homeostatic mechanism that precisely regulates the functional crosstalk of organs involved in balancing energy expenditure and caloric intake (Villarroya and Vidal-Puig, 2013). In hamsters, sympathetic nerve activity to WAT drives lipolysis, this provides free fatty acids for sympathetically mediated increases in BAT thermogenesis (Brito et al., 2008), suggesting that increasing BAT activity will have direct lipolytic consequences.
The neural mechanisms involved in the thermoregulatory control of BAT are well understood in rodents (Morrison, 2016). There is circumstantial evidence that human BAT is under sympathetic control, as human brown adipocytes express adrenoreceptors (Virtanen et al., 2009; Cypess et al., 2015; Blondin et al., 2020), certain adrenergic agonists have led to BAT thermogenesis (Kim et al., 2011; Cypess et al., 2015) and recent evidence of a nerve supply to BAT-like tissue in humans (Sievers et al., 2020). However, there is a lack of understanding of the physiological signals that drive sympathetic nerve activity to BAT in humans. Evidence suggests that mild environmental cooling (14–19°C) (Cypess et al., 2012; Chen et al., 2013) is adequate to activate BAT thermogenesis.
Based on the limited data available from neuroimaging studies the neural circuit underlying thermoregulation in humans, seems to involve the cerebral cortex and hypothalamus (Egan et al., 2005). Additionally, human rostral medullary raphé neurons are selectively activated in response to a thermoregulatory challenge and point to the location of thermoregulatory neurons similar to those of the raphé pallidus nucleus in rodents (McAllen et al., 2006). A human cadaveric study has also identified a nerve branch to supraclavicular tissue with a similar morphology to BAT, histological analysis of the tissue shows tyrosine hydroxylase immunoreactive structures, which likely represent sympathetic axons (Sievers et al., 2020). These points, taken together, suggest that certain structures that are involved in rodent neural circuitry underlying thermoregulation are also involved in the human circuitry. However, additional research is needed to draw comparisons between the two.
Orexins (hypocretins) are neuropeptides that are synthesized in specific neurons located in the lateral hypothalamus, and perifornical lateral hypothalamus. Activation of the lateral hypothalamic neurons significantly increases BAT sympathetic nerve activity in rodents (Cerri and Morrison, 2005). Orexinergic neurons in the perifornical lateral hypothalamus project to the rostral raphe’ pallidus. This increases the excitability of BAT sympathetic premotor neurons. Orexins have a recognized role in the management of body temperature and controlling heart rate, energy expenditure and BAT thermogenesis (Cao and Morrison, 2003; Sellayah et al., 2011; Girault et al., 2012; Messina et al., 2014). Additionally, direct injection of orexin into the rostral raphe’ pallidus increases BAT sympathetic nerve activity in rats (Madden et al., 2012). In rat studies, low (but stimulatory) doses of caffeine have previously activated orexinergic neurons in the dorsomedial hypothalamus and the perifornical areas of the lateral hypothalamus (Murphy et al., 2003; Sakurai, 2007), suggesting a possible central mechanism for caffeine evoked thermogenesis.
Recently the model for sympathetic control of BAT in rodents proposed by Morrison (2016) has been challenged (Saper and Machado, 2020). A series of studies in mice have demonstrated that pre-optic neurons that trigger cooling when activated, express a number of genetic markers, involving several that encode a protein fragment named pyroglutamylated RF-amide peptide (Wang et al., 2019; Hrvatin et al., 2020; Takahashi et al., 2020). Pyroglutamylated RF-amide peptide is a hypothalamic neuropeptide also expressed in median pre-optic neurons (Takahashi et al., 2020). The suggestion is that key neurons involved in causing hypothermia are not located in the medial pre-optic area, but are potentially found in the median pre-optic area and project directly to the dorsomedial hypothalamus. However, the data from the Morrison model is primarily from rat studies (Morrison et al., 2014). It is entirely possible that the neural organization in mice is different to that in rats. For example, in rats cutaneous vasoconstriction is not dependent on the dorsomedial hypothalamus in response to cold stimuli or activation of the febrile response (Rathner et al., 2008). A finding that is ignored by the proposed new model and has not been replicated in mice. A recent study demonstrates that inhibition of the median pre-optic area prevents cold evoked BAT thermogenesis in rats suggesting that a population of neurons within the median pre-optic area are required to be activated to drive BAT thermogenesis (da Conceição et al., 2020). This identifies the median pre-optic area as a primary source of glutamatergic excitation of BAT sympatho-excitatory neurons within the dorsomedial hypothalamus (da Conceição et al., 2020). It is yet to be determined whether these hypothalamic projecting neurons within the median pre-optic area excite thermogenesis promoting dorsomedial hypothalamic neurons during prostaglandin E2 or caffeine evoked BAT thermogenesis. Caffeine may act on neurons within the median pre-optic area, as this nuclei involves both sleep-wake neurons (Vanini et al., 2020) and energy metabolism neurons (da Conceição et al., 2020).
The protein fragment pyroglutamylated RF-amide is known to activate the G protein-coupled receptor, GPR103, which mediates orexigenic effects in rodents (Chartrel et al., 2003), and has previously been implicated in food intake, sympathetic regulation and anxiety (Takayasu et al., 2006; Okamoto et al., 2016). Central administration of pyroglutamylated RF-amide stimulates high fat food intake in rodents (Primeaux et al., 2008). In addition to stimulating food intake, pyroglutamylated RF-amide reduces thermogenesis, and increases body weight, fat mass and adipogenesis (Moriya et al., 2006; Mulumba et al., 2010). Given the role of pyroglutamylated RF- amide in orexin signaling and anxiety, there remains a possibility that caffeine may influence the effect of this protein. The proposed new model of thermoregulation, involving a population of excitatory neurons in the median pre-optic area expressing pyroglutamylated RF-amide, that connect directly to the dorsomedial hypothalamus (Hrvatin et al., 2020; Takahashi et al., 2020), is largely based on genetic molecular observations in knockout mouse models. Although knockout models can create odd effects as systems balance to cope with a lack of gene product (Teng et al., 2013), these observations are further validated using pharmacological and electrophysiological procedures on rats (da Conceição et al., 2020). Together, these findings provide new insights into the complexity of sympathetic thermoregulatory circuit. Gaining a better understanding of this central circuit is particularly important in developing new therapeutic approaches for augmenting BAT thermogenic energy expenditure, to improve energy homeostasis.
Interestingly, control of BAT in rats appears to follow a neural pathway which is exclusive and distinct from that which controls the cardiovascular system (Morrison, 1999). Additionally, there is evidence that indicates the premotor neurons in the central nervous system that regulate BAT are in fact specific and are separate from those regulating cutaneous vasomotor activity (Rathner et al., 2008). Studies that have observed increased interscapular BAT activity in rodents through increased nerve activity have demonstrated that this increase is accompanied by increases in heart rate and mean arterial pressure (Cerri and Morrison, 2005; Tupone et al., 2011). However, central, and systemic administration of stimulatory, but non-anxiogenic doses of caffeine increases interscapular BAT temperature in rats, without increasing core temperature, or increasing heart rate and mean arterial pressure (Van Schaik et al., 2021). These findings are further strengthened by increased neuronal activity, as measured by c-Fos-immunoreactivity within subregions of the hypothalamic area, previously implicated in regulating BAT thermogenesis. These include the perifornical area of the lateral hypothalamus, the lateral hypothalamus, and the dorsomedial hypothalamus following doses of caffeine administered either systemically or centrally (Van Schaik et al., 2021). These are areas known to that contain orexinergic neurons (Murphy et al., 2003; Berthoud et al., 2005; Cerri and Morrison, 2005; Tupone et al., 2011). The direct effects of caffeine in each of these brain regions remain unclear, and whether doses of caffeine comparable to the doses used in this study can activate BAT thermogenesis, without a cardio-dynamic effect in humans is unclear. However, it is clear that caffeine, at stimulatory doses act via the central nervous system to increase BAT thermogenesis in rodents.
This suggests that non-selective sympathetic activators may be beneficial as weight control agents. However, there remains a certain amount of risk with global sympathetic activation. Historically, therapeutic drugs that have been successful in creating negative energy balance weight loss in humans via non-selective activation of the sympathetic nervous system have been associated with cardiovascular side effects (Table 3). These adverse effects have prevented their use clinically (Yen and Ewald, 2012). For future sympathetic based strategies to increase energy expenditure through activation of BAT to be clinically successful, they will need to firstly enhance the sensitivity of BAT to the sympathetic nervous system or selectively stimulate BAT sympathetic nerve activity. These strategies may ameliorate the negative cardiovascular effects of non-selective sympathetic activation, while offering new ways for specifically promoting energy expenditure and decreasing the metabolic compensatory responses to chronic caloric restriction.
Conclusion
This review identifies significant differences in the development and function of WAT, BAT, and beige adipocytes. While BAT activation increases energy expenditure and improved energy homeostasis may assist in controlling obesity, the negative implications of non-selective activation of the sympathetic nervous system on the cardiovascular system are also clear. The neuro-circuitry of thermoregulation and knowledge of the neurochemical and functional properties of BAT activation are quite well understood in rodents. However, more research is needed into what primary β-adrenoreceptor is involved in human BAT activation, as this remains a contentious topic. This will assist in better understanding the function of BAT in humans and aid in translating findings from animal studies into human models. Whether caffeine evoked BAT thermogenesis in humans is a result of systemic or central activation remains unclear, however it is clear that caffeine evoked BAT thermogenesis in rodents is a result of central activation. Caffeine potentially exerts its effects on human BAT via acting on the neural pathway underlying metabolism. In particular, through activating orexinergic neurons in the perifornical lateral hypothalamus and lateral hypothalamus, and the antagonism of adenosine receptors more broadly.
Author Contributions
LVS wrote the manuscript with the rest of contributing revisions and input from JAR, RG, CK, and HRI. All authors contributed to scientific review and discussion of the manuscript.
Conflict of Interest
The authors declare that the research was conducted in the absence of any commercial or financial relationships that could be construed as a potential conflict of interest.
Acknowledgments
Support of the research contributing to this review: Defence Science Institute (LSV).
References
Abbracchio, M. P., Burnstock, G., Verkhratsky, A., and Zimmermann, H. (2009). Purinergic signalling in the nervous system: an overview. Trends Neurosci. 32, 19–29. doi: 10.1016/j.tins.2008.10.001
Abell, J. E., Egan, B. M., Wilson, P. W., Lipsitz, S., Woolson, R. F., and Lackland, D. T. (2007). Age and race impact the association between BMI and CVD mortality in women. Public Health Reports 122, 507–512.
Abenhaim, L., Moride, Y., Brenot, F., Rich, S., Benichou, J., Kurz, X., et al. (1996). Appetite-suppressant drugs and the risk of primary pulmonary hypertension. International primary pulmonary hypertension study group. N. Engl. J. Med. 335, 609–616. doi: 10.1056/NEJM199608293350901
Acheson, K. J., Gremaud, G., Meirim, I., Montigon, F., Krebs, Y., Fay, L. B., et al. (2004). Metabolic effects of caffeine in humans: lipid oxidation or futile cycling? Am. J. Clin. Nutr. 79, 40–46. doi: 10.1093/ajcn/79.1.40
Anandacoomarasamy, A., Caterson, I., Sambrook, P., Fransen, M., and March, L. (2008). The impact of obesity on the musculoskeletal system. Int. J. Obes. (Lond) 32, 211–222. doi: 10.1038/sj.ijo.0803715
Anderson, J. W., Konz, E. C., Frederich, R. C., and Wood, C. L. (2001). Long-term weight-loss maintenance: a meta-analysis of US studies. Am. J. Clin. Nutr. 74, 579–584. doi: 10.1093/ajcn/74.5.579
Arch, J. R. (2011). Challenges in β3-Adrenoceptor Agonist Drug Development. London: SAGE Publications Sage UK.
Arner, P. (1976). Relationship between intracellular cyclic AMP and lipolysis in human adipose tissue. Acta Medica Scand. 200, 179–186.
Astrup, A., Toubro, S., Cannon, S., Hein, P., Breum, L., and Madsen, J. (1990). Caffeine: a double-blind, placebo-controlled study of its thermogenic, metabolic, and cardiovascular effects in healthy volunteers. Am. J. Clin. Nutr. 51, 759–767. doi: 10.1093/ajcn/51.5.759
Ashihara, H., Sano, H., and Crozier, A. (2008). Caffeine and related purine alkaloids: biosynthesis, catabolism, function and genetic engineering. Phytochemistry 69, 841–856. doi: 10.1016/j.phytochem.2007.10.029
Bachman, E. S., Dhillon, H., Zhang, C. Y., Cinti, S., Bianco, A. C., Kobilka, B. K., et al. (2002). betaAR signaling required for diet-induced thermogenesis and obesity resistance. Science 297, 843–845. doi: 10.1126/science.1073160
Baker, J. G. (2005). The selectivity of β−adrenoceptor antagonists at the human β1, β2 and β3 adrenoceptors. Br. J. Pharmacol. 144, 317–322.
Baker, J. G. (2010). The selectivity of β−adrenoceptor agonists at human β1−, β2−and β3−adrenoceptors. Br. J. Pharmacol. 160, 1048–1061.
Baskin, A. S., Linderman, J. D., Brychta, R. J., McGehee, S., Anflick-Chames, E., Cero, C., et al. (2018). Regulation of human adipose tissue activation, gallbladder size, and bile acid metabolism by a beta3-adrenergic receptor agonist. Diabetes 67, 2113–2125. doi: 10.2337/db18-0462
Berkowitz, D. E., Nardone, N. A., Smiley, R. M., Price, D. T., Kreutter, D. K., Fremeau, R. T., et al. (1995). Distribution of β3-adrenoceptor mRNA in human tissues. Eur. J. Pharmacol. Mol. Pharmacol. 289, 223–228.
Berthoud, H. R., Patterson, L. M., Sutton, G. M., Morrison, C., and Zheng, H. (2005). Orexin inputs to caudal raphe neurons involved in thermal, cardiovascular, and gastrointestinal regulation. Histochem. Cell Biol. 123, 147–156.
Billon, N., Monteiro, M. C., and Dani, C. (2008). Developmental origin of adipocytes: new insights into a pending question. Biol. Cell 100, 563–575.
Block, J. P., Scribner, R. A., and DeSalvo, K. B. (2004). Fast food, race/ethnicity, and income: a geographic analysis. Am. J. Prev. Med. 27, 211–217. doi: 10.1016/j.amepre.2004.06.007
Blondin, D. P., Frisch, F., Phoenix, S., Guerin, B., Turcotte, E. E., Haman, F., et al. (2017). Inhibition of intracellular triglyceride lipolysis suppresses cold-induced brown adipose tissue metabolism and increases shivering in humans. Cell Metab. 25, 438–447. doi: 10.1016/j.cmet.2016.12.005
Blondin, D. P., Nielsen, S., Kuipers, E. N., Severinsen, M. C., Jensen, V. H., Miard, S., et al. (2020). Human brown adipocyte thermogenesis is driven by β2-AR stimulation. Cell Metab. 32, 287–300.e287.
Boardman, J. D., Saint Onge, J. M., Rogers, R. G., and Denney, J. T. (2005). Race differentials in obesity: the impact of place. J. Health Soc. Behav. 46, 229–243. doi: 10.1177/002214650504600302
Boden, G. (2003). Effects of free fatty acids (FFA) on glucose metabolism: significance for insulin resistance and type 2 diabetes. Exp. Clin. Endocrinol. Diab. 111, 121–124. doi: 10.1055/s-2003-39781
Boden, G. (2006). Fatty acid-induced inflammation and insulin resistance in skeletal muscle and liver. Curr. Diab. Rep. 6, 177–181. doi: 10.1007/s11892-006-0031-x
Boden, G., Lebed, B., Schatz, M., Homko, C., and Lemieux, S. (2001). Effects of acute changes of plasma free fatty acids on intramyocellular fat content and insulin resistance in healthy subjects. Diabetes 50, 1612–1617. doi: 10.2337/diabetes.50.7.1612
Boswell-Smith, V., Spina, D., and Page, C. P. (2006). Phosphodiesterase inhibitors. Br. J. Pharmacol. 147(Suppl 1), S252–S257. doi: 10.1038/sj.bjp.0706495
Bracco, D., Ferrarra, J.-M., Arnaud, M. J., Jequier, E., and Schutz, Y. (1995). Effects of caffeine on energy metabolism, heart rate, and methylxanthine metabolism in lean and obese women. Am. J. Physiol. Endocrinol. Metab. 269, E671–E678.
Brito, N. A., Brito, M. N., and Bartness, T. J. (2008). Differential sympathetic drive to adipose tissues after food deprivation, cold exposure or glucoprivation. Am. J. Physiol. Regul. Integr. Comp. Physiol. 294, R1445–R1452. doi: 10.1152/ajpregu.00068.2008
Brooks, G. A., Fahey, T. D., and Baldwin, K. M. (2005). Human Bioenergetics and its Applications. Exercise Physiology, 4th Edn. New York, NY: Mc Graw Hill, 122–125.
Bruno, A., Nolte, K. B., and Chapin, J. (1993). Stroke associated with ephedrine use. Neurology 43, 1313–1313.
Buemann, B., Toubro, S., and Astrup, A. (2000). Effects of the two β 3-agonists, ZD7114 and ZD2079 on 24 hour energy expenditure and respiratory quotient in obese subjects. Int. J. Obes. 24, 1553–1560.
Bukowiecki, L. J., Geloen, A., and Collet, A. J. (1986). Proliferation and differentiation of brown adipocytes from interstitial cells during cold acclimation. Am. J. Physiol. 250(6 Pt 1), C880–C887. doi: 10.1152/ajpcell.1986.250.6.C880
Calvert, J. W., Condit, M. E., Aragón, J. P., Nicholson, C. K., Moody, B. F., Hood, R. L., et al. (2011). Exercise protects against myocardial ischemia–reperfusion injury via stimulation of β3-adrenergic receptors and increased nitric oxide signaling: role of nitrite and nitrosothiols. Circ. Res. 108, 1448–1458.
Cannon, B., and Nedergaard, J. (2004). Brown adipose tissue: function and physiological significance. Physiol. Rev. 84, 277–359. doi: 10.1152/physrev.00015.2003
Cao, W.-H., Madden, C. J., and Morrison, S. F. (2010). Inhibition of brown adipose tissue thermogenesis by neurons in the ventrolateral medulla and in the nucleus tractus solitarius. Am. J. Physiol. Regulatory Integrative Comp. Physiol. 299, R277–R290.
Cao, W. H., and Morrison, S. F. (2003). Disinhibition of rostral raphe pallidus neurons increases cardiac sympathetic nerve activity and heart rate. Brain Res. 980, 1–10. doi: 10.1016/s0006-8993(03)02981-0
Carey, A. L., Formosa, M. F., Van Every, B., Bertovic, D., Eikelis, N., Lambert, G. W., et al. (2013). Ephedrine activates brown adipose tissue in lean but not obese humans. Diabetologia 56, 147–155.
Carey, A. L., Pajtak, R., Formosa, M. F., Van Every, B., Bertovic, D. A., Anderson, M. J., et al. (2015). Chronic ephedrine administration decreases brown adipose tissue activity in a randomised controlled human trial: implications for obesity. Diabetologia 58, 1045–1054. doi: 10.1007/s00125-015-3543-6
Carpentier, A. C., Blondin, D. P., Virtanen, K. A., Richard, D., Haman, F., and Turcotte, E. E. (2018). Brown adipose tissue energy metabolism in humans. Front. Endocrinol. (Lausanne) 9:447. doi: 10.3389/fendo.2018.00447
Carrion, M., Frommer, K. W., Perez-Garcia, S., Muller-Ladner, U., Gomariz, R. P., and Neumann, E. (2019). The adipokine network in rheumatic joint diseases. Int. J. Mol. Sci. 20:4091. doi: 10.3390/ijms20174091
Cerri, M., and Morrison, S. F. (2005). Activation of lateral hypothalamic neurons stimulates brown adipose tissue thermogenesis. Neuroscience 135, 627–638. doi: 10.1016/j.neuroscience.2005.06.039
Chamberlain, P., Jennings, K., Paul, F., Cordell, J., Berry, A., Holmes, S., et al. (1999). The tissue distribution of the human β 3-adrenoceptor studied using a monoclonal antibody: direct evidence of the β 3-adrenoceptor in human adipose tissue, atrium and skeletal muscle. Int. J. Obesity Related Metab. Disord. 23, 1057–1065.
Chapple, C. R., Kaplan, S. A., Mitcheson, D., Klecka, J., Cummings, J., Drogendijk, T., et al. (2013). Randomized double-blind, active-controlled phase 3 study to assess 12-month safety and efficacy of mirabegron, a β3-adrenoceptor agonist, in overactive bladder. Eur. Urol. 63, 296–305.
Chartrel, N., Dujardin, C., Anouar, Y., Leprince, J., Decker, A., Clerens, S., et al. (2003). Identification of 26RFa, a hypothalamic neuropeptide of the RFamide peptide family with orexigenic activity. Proc. Natl. Acad. Sci. U.S.A. 100, 15247–15252. doi: 10.1073/pnas.2434676100
Chen, K. Y., Brychta, R. J., Linderman, J. D., Smith, S., Courville, A., Dieckmann, W., et al. (2013). Brown fat activation mediates cold-induced thermogenesis in adult humans in response to a mild decrease in ambient temperature. J. Clin. Endocrinol. Metab. 98, E1218–E1223. doi: 10.1210/jc.2012-4213
Chernogubova, E., Hutchinson, D. S., Nedergaard, J., and Bengtsson, T. (2005). Alpha1- and beta1-adrenoceptor signaling fully compensates for beta3-adrenoceptor deficiency in brown adipocyte norepinephrine-stimulated glucose uptake. Endocrinology 146, 2271–2284. doi: 10.1210/en.2004-1104
Cinti, S. (2006). The role of brown adipose tissue in human obesity. Nutr. Metab. Cardiovasc. Dis. 16, 569–574. doi: 10.1016/j.numecd.2006.07.009
Coelho, M., Oliveira, T., and Fernandes, R. (2013). Biochemistry of adipose tissue: an endocrine organ. Arch. Med. Sci. 9, 191–200. doi: 10.5114/aoms.2013.33181
Conde, S. V., Nunes da Silva, T., Gonzalez, C., Mota Carmo, M., Monteiro, E. C., and Guarino, M. P. (2012). Chronic caffeine intake decreases circulating catecholamines and prevents diet-induced insulin resistance and hypertension in rats. Br. J. Nutr. 107, 86–95. doi: 10.1017/S0007114511002406
Connacher, A. A., Bennet, W. M., and Jung, R. T. (1992). Clinical studies with the β-adrenoceptor agonist BRL 26830A. Am. J. Clin. Nutr. 55(1 Suppl), 258S–261S.
Connacher, A. A., Jung, R. T., and Mitchell, P. E. (1988). Weight loss in obese subjects on a restricted diet given BRL 26830A, a new atypical beta adrenoceptor agonist. Br. Med. J. (Clin Res Ed) 296, 1217–1220. doi: 10.1136/bmj.296.6631.1217
Connacher, A. A., Lakie, M., Powers, N., Elton, R. A., Walsh, E. G., and Jung, R. T. (1990). Tremor and the anti-obesity drug BRL 26830A. Br. J. Clin. Pharmacol. 30, 613–615. doi: 10.1111/j.1365-2125.1990.tb03821.x
Connolly, H. M., Crary, J. L., McGoon, M. D., Hensrud, D. D., Edwards, B. S., Edwards, W. D., et al. (1997). Valvular heart disease associated with fenfluramine-phentermine. N. Engl. J. Med. 337, 581–588. doi: 10.1056/NEJM199708283370901
Contreras, C., Nogueiras, R., Dieguez, C., Medina-Gomez, G., and Lopez, M. (2016). Hypothalamus and thermogenesis: heating the BAT, browning the WAT. Mol. Cell Endocrinol. 438, 107–115. doi: 10.1016/j.mce.2016.08.002
Contreras, G. A., Lee, Y. H., Mottillo, E. P., and Granneman, J. G. (2014). Inducible brown adipocytes in subcutaneous inguinal white fat: the role of continuous sympathetic stimulation. Am. J. Physiol. Endocrinol. Metab. 307, E793–E799. doi: 10.1152/ajpendo.00033.2014
Corral, I., Landrine, H., Hao, Y., Zhao, L., Mellerson, J. L., and Cooper, D. L. (2012). Residential segregation, health behavior and overweight/obesity among a national sample of African American adults. J. Health Psychol. 17, 371–378.
Cousin, B., Cinti, S., Morroni, M., Raimbault, S., Ricquier, D., Penicaud, L., et al. (1992). Occurrence of brown adipocytes in rat white adipose tissue: molecular and morphological characterization. J. Cell Sci. 103 (Pt 4), 931–942.
Cully, M. (2014). Obesity: adenosine protects from diet-induced obesity. Nat. Rev. Drug Discov. 13, 886–887. doi: 10.1038/nrd4490
Cypess, A. M., Chen, Y. C., Sze, C., Wang, K., English, J., Chan, O., et al. (2012). Cold but not sympathomimetics activates human brown adipose tissue in vivo. Proc. Natl. Acad. Sci. U.S.A. 109, 10001–10005.
Cypess, A. M., Lehman, S., Williams, G., Tal, I., Rodman, D., Goldfine, A. B., et al. (2009). Identification and importance of brown adipose tissue in adult humans. N. Engl. J. Med. 360, 1509–1517. doi: 10.1056/NEJMoa0810780
Cypess, A. M., Weiner, L. S., Roberts-Toler, C., Franquet Elia, E., Kessler, S. H., Kahn, P. A., et al. (2015). Activation of human brown adipose tissue by a beta3-adrenergic receptor agonist. Cell Metab. 21, 33–38. doi: 10.1016/j.cmet.2014.12.009
da Conceição, E. P. S., Morrison, S. F., Cano, G., Chiavetta, P., and Tupone, D. (2020). Median preoptic area neurons are required for the cooling and febrile activations of brown adipose tissue thermogenesis in rat. Sci. Rep. 10, 1–16.
de Jong, J. M., Sun, W., Pires, N. D., Frontini, A., Balaz, M., Jespersen, N. Z., et al. (2019). Human brown adipose tissue is phenocopied by classical brown adipose tissue in physiologically humanized mice. Nat. Metab. 1, 830–843.
De Matteis, R., Arch, J. R., Petroni, M. L., Ferrari, D., Cinti, S., and Stock, M. J. (2002). Immunohistochemical identification of the beta(3)-adrenoceptor in intact human adipocytes and ventricular myocardium: effect of obesity and treatment with ephedrine and caffeine. Int. J. Obes. Relat. Metab. Disord. 26, 1442–1450. doi: 10.1038/sj.ijo.0802148
Dehal, A., Garrett, T., Tedders, S. H., Arroyo, C., Afriyie-Gyawu, E., and Zhang, J. (2011). Body mass index and death rate of colorectal cancer among a national cohort of US adults. Nutr. Cancer 63, 1218–1225.
Dehvari, N., da Silva Junior, E. D., Bengtsson, T., and Hutchinson, D. S. (2018). Mirabegron: potential off target effects and uses beyond the bladder. Br. J. Pharmacol. 175, 4072–4082. doi: 10.1111/bph.14121
Dekker, M. J., Gusba, J. E., Robinson, L. E., and Graham, T. E. (2007). Glucose homeostasis remains altered by acute caffeine ingestion following 2 weeks of daily caffeine consumption in previously non-caffeine-consuming males. Br. J. Nutr. 98, 556–562.
Drewnowski, A., and Specter, S. E. (2004). Poverty and obesity: the role of energy density and energy costs. Am. J. Clin. Nutr. 79, 6–16. doi: 10.1093/ajcn/79.1.6
Dulloo, A., Geissler, C., Horton, T., Collins, A., and Miller, D. (1989). Normal caffeine consumption: influence on thermogenesis and daily energy expenditure in lean and postobese human volunteers. Am. J. Clin. Nutr. 49, 44–50.
Dulloo, A. G. (2011). The search for compounds that stimulate thermogenesis in obesity management: from pharmaceuticals to functional food ingredients. Obesity Rev. 12, 866–883.
Egan, G. F., Johnson, J., Farrell, M., McAllen, R., Zamarripa, F., McKinley, M. J., et al. (2005). Cortical, thalamic, and hypothalamic responses to cooling and warming the skin in awake humans: a positron-emission tomography study. Proc. Natl. Acad. Sci. U.S.A. 102, 5262–5267. doi: 10.1073/pnas.0409753102
Engin, A. (2017). “The definition and prevalence of obesity and metabolic syndrome,” in Obesity and Lipotoxicity. Advances in Experimental Medicine and Biology, eds A. Engin and A. Engin (Cham: Springer), 1–17.
Ferre, S. (2010). Role of the central ascending neurotransmitter systems in the psychostimulant effects of caffeine. J. Alzheimers Dis. 20(Suppl 1), S35–S49. doi: 10.3233/JAD-2010-1400
Finlin, B. S., Memetimin, H., Confides, A. L., Kasza, I., Zhu, B., Vekaria, H. J., et al. (2018). Human adipose beiging in response to cold and mirabegron. JCI Insight 3:e121510. doi: 10.1172/jci.insight.121510
Francken, B. J., Jurzak, M., Vanhauwe, J. F., Luyten, W. H., and Leysen, J. E. (1998). The human 5-ht5A receptor couples to Gi/Go proteins and inhibits adenylate cyclase in HEK 293 cells. Eur. J. Pharmacol. 361, 299–309. doi: 10.1016/s0014-2999(98)00744-4
Fredholm, B. B., Ap, I. J., Jacobson, K. A., Linden, J., and Muller, C. E. (2011). International union of basic and clinical pharmacology. LXXXI. Nomenclature and classification of adenosine receptors–an update. Pharmacol. Rev. 63, 1–34. doi: 10.1124/pr.110.003285
Fukano, K., Okamatsu-Ogura, Y., Tsubota, A., Nio-Kobayashi, J., and Kimura, K. (2016). Cold exposure induces proliferation of mature brown adipocyte in a ss3-adrenergic receptor-mediated pathway. PLoS One 11:e0166579. doi: 10.1371/journal.pone.0166579
Gauthier, C., Langin, D., and Balligand, J. L. (2000). Beta3-adrenoceptors in the cardiovascular system. Trends Pharmacol. Sci. 21, 426–431. doi: 10.1016/s0165-6147(00)01562-5
Gauthier, C., Tavernier, G., Charpentier, F., Langin, D., and Le Marec, H. (1996). Functional beta3-adrenoceptor in the human heart. J. Clin. Invest. 98, 556–562. doi: 10.1172/JCI118823
Gauthier, C., Tavernier, G., Trochu, J. N., Leblais, V., Laurent, K., Langin, D., et al. (1999). Interspecies differences in the cardiac negative inotropic effects of beta(3)-adrenoceptor agonists. J. Pharmacol. Exp. Ther. 290, 687–693.
Geleijnse, J. M. (2008). Habitual coffee consumption and blood pressure: an epidemiological perspective. Vascular Health Risk Manage. 4:963.
Geloen, A., Collet, A. J., and Bukowiecki, L. J. (1992). Role of sympathetic innervation in brown adipocyte proliferation. Am. J. Physiol. 263(6 Pt 2), R1176–R1181. doi: 10.1152/ajpregu.1992.263.6.R1176
Gepstein, V., and Weiss, R. (2019). Obesity as the main risk factor for metabolic syndrome in children. Front. Endocrinol. (Lausanne) 10:568. doi: 10.3389/fendo.2019.00568
Gesta, S., Tseng, Y. H., and Kahn, C. R. (2007). Developmental origin of fat: tracking obesity to its source. Cell 131, 242–256. doi: 10.1016/j.cell.2007.10.004
Gibson, D. M. (2011). The neighborhood food environment and adult weight status: estimates from longitudinal data. Am. J. Public Health 101, 71–78. doi: 10.2105/AJPH.2009.187567
Girault, E. M., Yi, C.-X., Fliers, E., and Kalsbeek, A. (2012). Orexins, feeding, and energy balance. Prog. Brain Res. 198, 47–64.
Gnad, T., Scheibler, S., von Kügelgen, I., Scheele, C., Kilić, A., Glöde, A., et al. (2014). Adenosine activates brown adipose tissue and recruits beige adipocytes via A 2A receptors. Nature 516, 395–399.
Goodpaster, B. H., Theriault, R., Watkins, S. C., and Kelley, D. E. (2000). Intramuscular lipid content is increased in obesity and decreased by weight loss. Metabolism 49, 467–472.
Gourine, A. V., Wood, J. D., and Burnstock, G. (2009). Purinergic signalling in autonomic control. Trends Neurosci. 32, 241–248. doi: 10.1016/j.tins.2009.03.002
Graziano, M. P., Casey, P. J., and Gilman, A. G. (1987). Expression of cDNAs for G proteins in Escherichia coli. Two forms of Gs alpha stimulate adenylate cyclase. J. Biol. Chem. 262, 11375–11381.
Greenway, F. L. (2015). Physiological adaptations to weight loss and factors favouring weight regain. Int. J. Obes. (Lond) 39, 1188–1196. doi: 10.1038/ijo.2015.59
Grill, H. J., and Hayes, M. R. (2012). Hindbrain neurons as an essential hub in the neuroanatomically distributed control of energy balance. Cell Metab. 16, 296–309. doi: 10.1016/j.cmet.2012.06.015
Haller, C. A., and Benowitz, N. L. (2000). Adverse cardiovascular and central nervous system events associated with dietary supplements containing ephedra alkaloids. N. Engl. J. Med. 343, 1833–1838. doi: 10.1056/NEJM200012213432502
Hanssen, M. J., van der Lans, A. A., Brans, B., Hoeks, J., Jardon, K. M., Schaart, G., et al. (2016). Short-term cold acclimation recruits brown adipose tissue in obese humans. Diabetes 65, 1179–1189. doi: 10.2337/db15-1372
Himms-Hagen, J. (1989). Brown adipose tissue thermogenesis and obesity. Prog. Lipid Res. 28, 67–115.
Ho, W., Lu, P., Hsiao, M., Hwang, H., and Tseng, Y. (2008). Adenosine modulates cardiovascular functions through activation of extracellular signal-regulated kinases 1 and 2 and endothelial nitric oxide synthase in the nucleus tractus solitarii of rats. Circulation 117, 773–780.
Hoehn-Saric, R., and McLeod, D. R. (2000). Anxiety and arousal: physiological changes and their perception. J. Affect. Disord. 61, 217–224.
Hrvatin, S., Sun, S., Wilcox, O. F., Yao, H., Lavin-Peter, A. J., Cicconet, M., et al. (2020). Neurons that regulate mouse torpor. Nature 583, 115–121.
Ikeda, K., Maretich, P., and Kajimura, S. (2018). The common and distinct features of brown and beige adipocytes. Trends Endocrinol. Metab. 29, 191–200. doi: 10.1016/j.tem.2018.01.001
James, W. P., Caterson, I. D., Coutinho, W., Finer, N., Van Gaal, L. F., Maggioni, A. P., et al. (2010). Effect of sibutramine on cardiovascular outcomes in overweight and obese subjects. N. Engl. J. Med. 363, 905–917. doi: 10.1056/NEJMoa1003114
Jequier, E., Munger, R., and Felber, J. (1992). Thermogenic effects of various β-adrenoceptor agonists in humans: their potential usefulness in the treatment of obesity. Am. J. Clin. Nutr. 55(1 Suppl), 249S–251S.
Jimenez, M., Barbatelli, G., Allevi, R., Cinti, S., Seydoux, J., Giacobino, J. P., et al. (2003). Beta 3-adrenoceptor knockout in C57BL/6J mice depresses the occurrence of brown adipocytes in white fat. Eur. J. Biochem. 270, 699–705. doi: 10.1046/j.1432-1033.2003.03422.x
Johansson, S. M., Lindgren, E., Yang, J. N., Herling, A. W., and Fredholm, B. B. (2008). Adenosine A1 receptors regulate lipolysis and lipogenesis in mouse adipose tissue-interactions with insulin. Eur. J. Pharmacol. 597, 92–101. doi: 10.1016/j.ejphar.2008.08.022
Kajimura, S., Seale, P., and Spiegelman, B. M. (2010). Transcriptional control of brown fat development. Cell Metab. 11, 257–262. doi: 10.1016/j.cmet.2010.03.005
Kashyap, S., Belfort, R., Gastaldelli, A., Pratipanawatr, T., Berria, R., Pratipanawatr, W., et al. (2003). A sustained increase in plasma free fatty acids impairs insulin secretion in nondiabetic subjects genetically predisposed to develop type 2 diabetes. Diabetes 52, 2461–2474. doi: 10.2337/diabetes.52.10.2461
Kernan, W. N., Viscoli, C. M., Brass, L. M., Broderick, J. P., Brott, T., Feldmann, E., et al. (2000). Phenylpropanolamine and the risk of hemorrhagic stroke. N. Engl. J. Med. 343, 1826–1832. doi: 10.1056/NEJM200012213432501
Khullar, V., Amarenco, G., Angulo, J. C., Cambronero, J., Høye, K., Milsom, I., et al. (2013). Efficacy and tolerability of mirabegron, a β3-adrenoceptor agonist, in patients with overactive bladder: results from a randomised European–Australian phase 3 trial. Eur. Urol. 63, 283–295.
Kim, J. K., Won, Y. W., Lim, K. S., Park, E. J., and Kim, Y. H. (2011). Anti-cancer effects of docetaxel loaded thermo-reversible hydrogels in a tumor xenograft mice model. J. Control Release 152(Suppl. 1) e44–e45. doi: 10.1016/j.jconrel.2011.08.112
Klingenspor, M. (2003). Cold-induced recruitment of brown adipose tissue thermogenesis. Exp. Physiol. 88, 141–148. doi: 10.1113/eph8802508
Lake, C. R., Gallant, S., Masson, E., and Miller, P. (1990). Adverse drug effects attributed to phenylpropanolamine: a review of 142 case reports. Am. J. Med. 89, 195–208. doi: 10.1016/0002-9343(90)90299-s
Larsen, T. M., Toubro, S., van Baak, M. A., Gottesdiener, K. M., Larson, P., Saris, W. H., et al. (2002). Effect of a 28-d treatment with L-796568, a novel β3-adrenergic receptor agonist, on energy expenditure and body composition in obese men. Am. J. Clin. Nutr. 76, 780–788.
Lee, P., Bova, R., Schofield, L., Bryant, W., Dieckmann, W., Slattery, A., et al. (2016). Brown adipose tissue exhibits a glucose-responsive thermogenic biorhythm in humans. Cell Metab. 23, 602–609. doi: 10.1016/j.cmet.2016.02.007
Lee, P., Day, R., Greenfield, J., and Ho, K. (2013a). Formoterol, a highly β 2-selective agonist, increases energy expenditure and fat utilisation in men. Int. J. Obes. 37, 593–597.
Lee, P., Swarbrick, M. M., and Ho, K. K. (2013b). Brown adipose tissue in adult humans: a metabolic renaissance. Endocr. Rev. 34, 413–438. doi: 10.1210/er.2012-1081
Lee, Y. H., Petkova, A. P., Konkar, A. A., and Granneman, J. G. (2015). Cellular origins of cold-induced brown adipocytes in adult mice. FASEB J. 29, 286–299. doi: 10.1096/fj.14-263038
Leitner, B. P., Huang, S., Brychta, R. J., Duckworth, C. J., Baskin, A. S., McGehee, S., et al. (2017). Mapping of human brown adipose tissue in lean and obese young men. Proc. Natl. Acad. Sci. U.S.A. 114, 8649–8654. doi: 10.1073/pnas.1705287114
Leo, P. J., Hollander, J. E., Shih, R. D., and Marcus, S. M. (1996). Phenylpropanolamine and associated myocardial injury. Ann. Emerg. Med. 28, 359–362. doi: 10.1016/s0196-0644(96)70038-0
Leonard, W. R. (2010). Measuring human energy expenditure and metabolic function: basic principles and methods. J. Anthropol. Sci. 88, 221–230.
Lepper, C., and Fan, C. M. (2010). Inducible lineage tracing of Pax7-descendant cells reveals embryonic origin of adult satellite cells. Genesis 48, 424–436. doi: 10.1002/dvg.20630
Lidell, M. E., Betz, M. J., Dahlqvist Leinhard, O., Heglind, M., Elander, L., Slawik, M., et al. (2013). Evidence for two types of brown adipose tissue in humans. Nat. Med. 19, 631–634. doi: 10.1038/nm.3017
Liu, X., Liu, M., Tsilimingras, D., and Schiffrin, E. L. (2011). Racial disparities in cardiovascular risk factors among diagnosed hypertensive subjects. J. Am. Soc. Hypertens. 5, 239–248.
Loh, R., Kingwell, B., and Carey, A. (2017). Human brown adipose tissue as a target for obesity management; beyond cold−induced thermogenesis. Obes. Rev. 18, 1227–1242.
Loh, R. K. C., Formosa, M. F., La Gerche, A., Reutens, A. T., Kingwell, B. A., and Carey, A. L. (2019). Acute metabolic and cardiovascular effects of mirabegron in healthy individuals. Diab. Obes. Metab. 21, 276–284. doi: 10.1111/dom.13516
Ma, Y., Hébert, J. R., Manson, J. E., Balasubramanian, R., Liu, S., Lamonte, M. J., et al. (2012). Determinants of racial/ethnic disparities in incidence of diabetes in postmenopausal women in the US: the Women’s Health Initiative 1993–2009. Diabetes care 35, 2226–2234.
Madden, C. J., and Morrison, S. F. (2016). A high-fat diet impairs cooling-evoked brown adipose tissue activation via a vagal afferent mechanism. Am. J. Physiol. Endocrinol. Metab. 311, E287–E292. doi: 10.1152/ajpendo.00081.2016
Madden, C. J., Tupone, D., and Morrison, S. F. (2012). Orexin modulates brown adipose tissue thermogenesis. Biomol. Concepts 3, 381–386. doi: 10.1515/bmc-2011-0066
Mancuso, P. (2016). The role of adipokines in chronic inflammation. Immunotargets Ther. 5, 47–56. doi: 10.2147/ITT.S73223
Martin, A. D., Daniel, M. Z., Drinkwater, D. T., and Clarys, J. P. (1994). Adipose tissue density, estimated adipose lipid fraction and whole body adiposity in male cadavers. Int. J. Obes. Relat. Metab. Disord. 18, 79–83.
McAllen, R. M., Farrell, M., Johnson, J. M., Trevaks, D., Cole, L., McKinley, M. J., et al. (2006). Human medullary responses to cooling and rewarming the skin: a functional MRI study. Proc. Natl. Acad. Sci. U.S.A. 103, 809–813. doi: 10.1073/pnas.0509862103
Mersmann, H. J., Carey, G. B., and Smith, E. O. (1997). Adipose tissue beta-adrenergic and A1 adenosine receptors in suckling pigs. J. Anim. Sci. 75, 3161–3168. doi: 10.2527/1997.75123161x
Messina, G., Dalia, C., Tafuri, D., Monda, V., Palmieri, F., Dato, A., et al. (2014). Orexin-A controls sympathetic activity and eating behavior. Front. Psychol. 5:997. doi: 10.3389/fpsyg.2014.00997
Mo, W., Michel, M. C., Lee, X. W., Kaumann, A. J., and Molenaar, P. (2017). The beta3 -adrenoceptor agonist mirabegron increases human atrial force through beta1 -adrenoceptors: an indirect mechanism? Br. J. Pharmacol. 174, 2706–2715. doi: 10.1111/bph.13897
Modi, A. A., Feld, J. J., Park, Y., Kleiner, D. E., Everhart, J. E., Liang, T. J., et al. (2010). Increased caffeine consumption is associated with reduced hepatic fibrosis. Hepatology 51, 201–209.
Moriya, R., Sano, H., Umeda, T., Ito, M., Takahashi, Y., Matsuda, M., et al. (2006). RFamide peptide QRFP43 causes obesity with hyperphagia and reduced thermogenesis in mice. Endocrinology 147, 2916–2922. doi: 10.1210/en.2005-1580
Morrison, S. F. (1999). RVLM and raphe differentially regulate sympathetic outflows to splanchnic and brown adipose tissue. Am. J. Physiol. 276, R962–R973. doi: 10.1152/ajpregu.1999.276.4.R962
Morrison, S. F. (2016). Central neural control of thermoregulation and brown adipose tissue. Auton. Neurosci. 196, 14–24. doi: 10.1016/j.autneu.2016.02.010
Morrison, S. F., Madden, C. J., and Tupone, D. (2014). Central neural regulation of brown adipose tissue thermogenesis and energy expenditure. Cell Metab. 19, 741–756. doi: 10.1016/j.cmet.2014.02.007
Morrison, S. F., and Nakamura, K. (2011). Central neural pathways for thermoregulation. Front. Biosci. (Landmark Ed) 16:74–104. doi: 10.2741/3677
Morrison, S. F., Ramamurthy, S., and Young, J. B. (2000). Reduced rearing temperature augments responses in sympathetic outflow to brown adipose tissue. J. Neurosci. 20, 9264–9271. doi: 10.1523/JNEUROSCI.20-24-09264.2000
Moustafa, F., and Feldman, S. R. (2014). A review of phosphodiesterase-inhibition and the potential role for phosphodiesterase 4-inhibitors in clinical dermatology. Dermatol. Online J. 20:22608.
Mulumba, M., Jossart, C., Granata, R., Gallo, D., Escher, E., Ghigo, E., et al. (2010). GPR103b functions in the peripheral regulation of adipogenesis. Mol. Endocrinol. 24, 1615–1625. doi: 10.1210/me.2010-0010
Murphy, J. A., Deurveilher, S., and Semba, K. (2003). Stimulant doses of caffeine induce c-FOS activation in orexin/hypocretin-containing neurons in rat. Neuroscience 121, 269–275. doi: 10.1016/s0306-4522(03)00461-5
Nazari, S., Kourosh-Arami, M., Komaki, A., and Hajizadeh, S. (2020). Relative contribution of central and peripheral factors in superficial blood flow regulation following cold exposure. Physiol. Pharmacol. (Iran) 24, 89–100.
Nedergaard, J., and Cannon, B. (2010). The changed metabolic world with human brown adipose tissue: therapeutic visions. Cell Metab. 11, 268–272. doi: 10.1016/j.cmet.2010.03.007
Noordzij, M., Uiterwaal, C. S., Arends, L. R., Kok, F. J., Grobbee, D. E., and Geleijnse, J. M. (2005). Blood pressure response to chronic intake of coffee and caffeine: a meta-analysis of randomized controlled trials. J. Hypertens. 23, 921–928.
Okamoto, K., Yamasaki, M., Takao, K., Soya, S., Iwasaki, M., Sasaki, K., et al. (2016). QRFP-deficient mice are hypophagic, lean, hypoactive and exhibit increased anxiety-like behavior. PLoS One 11:e0164716. doi: 10.1371/journal.pone.0164716
O’Mara, A. E., Johnson, J. W., Linderman, J. D., Brychta, R. J., McGehee, S., Fletcher, L. A., et al. (2020). Chronic mirabegron treatment increases human brown fat, HDL cholesterol, and insulin sensitivity. J. Clin. Invest. 130, 2209–2219. doi: 10.1172/JCI131126
Park, A., Kim, W. K., and Bae, K. H. (2014). Distinction of white, beige and brown adipocytes derived from mesenchymal stem cells. World J. Stem Cells 6, 33–42. doi: 10.4252/wjsc.v6.i1.33
Park, S., Jang, J. S., and Hong, S. M. (2007). Long-term consumption of caffeine improves glucose homeostasis by enhancing insulinotropic action through islet insulin/insulin-like growth factor 1 signaling in diabetic rats. Metabolism 56, 599–607.
Penicaud, L., Cousin, B., Leloup, C., Lorsignol, A., and Casteilla, L. (2000). The autonomic nervous system, adipose tissue plasticity, and energy balance. Nutrition 16, 903–908.
Perrio, M. J., Wilton, L. V., and Shakir, S. A. (2007). The safety profiles of orlistat and sibutramine: results of prescription-event monitoring studies in England. Obesity (Silver Spring) 15, 2712–2722. doi: 10.1038/oby.2007.323
Petrovic, N., Walden, T. B., Shabalina, I. G., Timmons, J. A., Cannon, B., and Nedergaard, J. (2010). Chronic peroxisome proliferator-activated receptor gamma (PPARgamma) activation of epididymally derived white adipocyte cultures reveals a population of thermogenically competent, UCP1-containing adipocytes molecularly distinct from classic brown adipocytes. J. Biol. Chem. 285, 7153–7164. doi: 10.1074/jbc.M109.053942
Pettit, N. N., MacKenzie, E. L., Ridgway, J. P., Pursell, K., Ash, D., Patel, B., et al. (2020). Obesity is associated with increased risk for mortality among hospitalized patients with COVID−19. Obesity 28, 1806–1810.
Porter, C., Herndon, D. N., Chondronikola, M., Chao, T., Annamalai, P., Bhattarai, N., et al. (2016). Human and mouse brown adipose tissue mitochondria have comparable UCP1 function. Cell Metab. 24, 246–255.
Primeaux, S. D., Blackmon, C., Barnes, M. J., Braymer, H. D., and Bray, G. A. (2008). Central administration of the RFamide peptides, QRFP-26 and QRFP-43, increases high fat food intake in rats. Peptides 29, 1994–2000. doi: 10.1016/j.peptides.2008.07.024
Quick, V. M., McWilliams, R., and Byrd-Bredbenner, C. (2013). Fatty, fatty, two-by-four: weight-teasing history and disturbed eating in young adult women. Am. J. Public Health 103, 508–515. doi: 10.2105/AJPH.2012.300898
Rathner, J. A., Madden, C. J., and Morrison, S. F. (2008). Central pathway for spontaneous and prostaglandin E2-evoked cutaneous vasoconstriction. Am. J. Physiol. Regul. Integr. Comp. Physiol. 295, R343–R354. doi: 10.1152/ajpregu.00115.2008
Rathner, J. A., Owens, N. C., and McAllen, R. M. (2001). Cold−activated raphé−spinal neurons in rats. J. Physiol. 535, 841–854.
Reinehr, T., and Roth, C. L. (2018). Inflammation markers in type 2 diabetes and the metabolic syndrome in the pediatric population. Curr. Diab. Rep. 18:131. doi: 10.1007/s11892-018-1110-5
Ribeiro, J. A., and Sebastiao, A. M. (2010). Caffeine and adenosine. J. Alzheimers Dis. 20(Suppl. 1) S3–S15. doi: 10.3233/JAD-2010-1379
Riis-Vestergaard, M. J., Richelsen, B., Bruun, J. M., Li, W., Hansen, J. B., and Pedersen, S. B. (2020). Beta-1 and not Beta-3 adrenergic receptors may be the primary regulator of human brown adipocyte metabolism. J. Clin. Endocrinol. Metab. 105, e994–e1005.
Rivera-Oliver, M., and Díaz-Ríos, M. (2014). Using caffeine and other adenosine receptor antagonists and agonists as therapeutic tools against neurodegenerative diseases: a review. Life Sci. 101, 1–9. doi: 10.1016/j.lfs.2014.01.083
Rosenwald, M., and Wolfrum, C. (2014). The origin and definition of brite versus white and classical brown adipocytes. Adipocyte 3, 4–9. doi: 10.4161/adip.26232
Sakurai, T. (2007). The neural circuit of orexin (hypocretin): maintaining sleep and wakefulness. Nat. Rev. Neurosci. 8, 171–181. doi: 10.1038/nrn2092
Samenuk, D., Link, M. S., Homoud, M. K., Contreras, R., Theohardes, T. C., Wang, P. J., et al. (2002). Adverse cardiovascular events temporally associated with ma huang, an herbal source of ephedrine. Mayo Clin. Proc. 77, 12–16.
Sanchez-Gurmaches, J., and Guertin, D. A. (2014). Adipocyte lineages: tracing back the origins of fat. Biochim. Biophys. Acta 1842, 340–351. doi: 10.1016/j.bbadis.2013.05.027
Saper, C. B., and Machado, N. L. (2020). Flipping the switch on the body’s thermoregulatory system. Nature 583, 34–35.
Savoca, M. R., Evans, C. D., Wilson, M. E., Harshfield, G. A., and Ludwig, D. A. (2004). The association of caffeinated beverages with blood pressure in adolescents. Arch. Pediatr. Adolesc. Med. 158, 473–477.
Schena, G., and Caplan, M. J. (2019). Everything you always wanted to know about beta3-AR ∗ (∗ but were afraid to ask). Cells 8:357. doi: 10.3390/cells8040357
Schwartz, M. W., Seeley, R. J., Zeltser, L. M., Drewnowski, A., Ravussin, E., Redman, L. M., et al. (2017). Obesity pathogenesis: an endocrine society scientific statement. Endocr. Rev. 38, 267–296. doi: 10.1210/er.2017-00111
Seale, P., Bjork, B., Yang, W., Kajimura, S., Chin, S., Kuang, S., et al. (2008). PRDM16 controls a brown fat/skeletal muscle switch. Nature 454, 961–967. doi: 10.1038/nature07182
Seifert, R. (2011). CGP20712A. xPharm: The Comprehensive Pharmacology Reference. Amsterdam: Elsevier, 1–4. doi: 10.1016/B978-008055232-3.61426-6
Sellayah, D., Bharaj, P., and Sikder, D. (2011). Orexin is required for brown adipose tissue development, differentiation, and function. Cell Metab. 14, 478–490. doi: 10.1016/j.cmet.2011.08.010
Shimizu, I., Aprahamian, T., Kikuchi, R., Shimizu, A., Papanicolaou, K. N., MacLauchlan, S., et al. (2014). Vascular rarefaction mediates whitening of brown fat in obesity. J. Clin. Invest. 124, 2099–2112. doi: 10.1172/JCI71643
Sidossis, L. S., Porter, C., Saraf, M. K., Borsheim, E., Radhakrishnan, R. S., Chao, T., et al. (2015). Browning of subcutaneous white adipose tissue in humans after severe adrenergic stress. Cell Metab. 22, 219–227. doi: 10.1016/j.cmet.2015.06.022
Sievers, W., Rathner, J. A., Green, R. A., Kettle, C., Irving, H. R., Whelan, D. R., et al. (2020). Innervation of supraclavicular adipose tissue: a human cadaveric study. PLoS One 15:e0236286. doi: 10.1371/journal.pone.0236286
Smith, A. (2002). Effects of caffeine on human behavior. Food Chem. Toxicol. 40, 1243–1255. doi: 10.1016/s0278-6915(02)00096-0
Soderlund, V., Larsson, S. A., and Jacobsson, H. (2007). Reduction of FDG uptake in brown adipose tissue in clinical patients by a single dose of propranolol. Eur. J. Nucl. Med. Mol. Imaging 34, 1018–1022. doi: 10.1007/s00259-006-0318-9
Székely, M. (2000). The vagus nerve in thermoregulation and energy metabolism. Auton. Neurosci. 85, 26–38. doi: 10.1016/s1566-0702(00)00217-4
Takahashi, T. M., Sunagawa, G. A., Soya, S., Abe, M., Sakurai, K., Ishikawa, K., et al. (2020). A discrete neuronal circuit induces a hibernation-like state in rodents. Nature 583, 109–114. doi: 10.1038/s41586-020-2163-6
Takasu, T., Ukai, M., Sato, S., Matsui, T., Nagase, I., Maruyama, T., et al. (2007). Effect of (R)-2-(2-aminothiazol-4-yl)-4’-{2-[(2-hydroxy-2-phenylethyl)amino]ethyl} acetanilide (YM178), a novel selective beta3-adrenoceptor agonist, on bladder function. J. Pharmacol. Exp. Ther. 321, 642–647. doi: 10.1124/jpet.106.115840
Takayasu, S., Sakurai, T., Iwasaki, S., Teranishi, H., Yamanaka, A., Williams, S. C., et al. (2006). A neuropeptide ligand of the G protein-coupled receptor GPR103 regulates feeding, behavioral arousal, and blood pressure in mice. Proc. Natl. Acad. Sci. U.S.A. 103, 7438–7443. doi: 10.1073/pnas.0602371103
Tatsis-Kotsidis, I., and Erlanger, B. F. (1999). A1 adenosine receptor of human and mouse adipose tissues: cloning, expression, and characterization. Biochem. Pharmacol. 58, 1269–1277. doi: 10.1016/s0006-2952(99)00214-2
Tavernier, G., Toumaniantz, G., Erfanian, M., Heymann, M.-F., Laurent, K., Langin, D., et al. (2003). β3-Adrenergic stimulation produces a decrease of cardiac contractility ex vivo in mice overexpressing the human β3-adrenergic receptor. Cardiovasc. Res. 59, 288–296.
Teng, X., Dayhoff-Brannigan, M., Cheng, W. C., Gilbert, C. E., Sing, C. N., Diny, N. L., et al. (2013). Genome-wide consequences of deleting any single gene. Mol. Cell 52, 485–494. doi: 10.1016/j.molcel.2013.09.026
Teramae, C. Y., Connolly, H. M., Grogan, M., and Miller, F. A. Jr. (2000). Diet drug-related cardiac valve disease: the Mayo Clinic echocardiographic laboratory experience. Mayo Clin. Proc. 75, 456–461.
Tilg, H., and Moschen, A. R. (2006). Adipocytokines: mediators linking adipose tissue, inflammation and immunity. Nat. Rev. Immunol. 6, 772–783. doi: 10.1038/nri1937
Timmons, J. A., Wennmalm, K., Larsson, O., Walden, T. B., Lassmann, T., Petrovic, N., et al. (2007). Myogenic gene expression signature establishes that brown and white adipocytes originate from distinct cell lineages. Proc. Natl. Acad. Sci. U.S.A. 104, 4401–4406. doi: 10.1073/pnas.0610615104
Trost, T., and Schwabe, U. (1981). Adenosine receptors in fat cells. Identification by (-)-N6-[3H]phenylisopropyladenosine binding. Mol. Pharmacol. 19, 228–235.
Tu, C., He, J., Wu, B., Wang, W., and Li, Z. (2019). An extensive review regarding the adipokines in the pathogenesis and progression of osteoarthritis. Cytokine 113, 1–12.
Tupone, D., Madden, C. J., Cano, G., and Morrison, S. F. (2011). An orexinergic projection from perifornical hypothalamus to raphe pallidus increases rat brown adipose tissue thermogenesis. J. Neurosci. 31, 15944–15955. doi: 10.1523/JNEUROSCI.3909-11.2011
Tupone, D., Madden, C. J., and Morrison, S. F. (2013). Central activation of the A1 adenosine receptor (A1AR) induces a hypothermic, torpor-like state in the rat. J. Neurosci. 33, 14512–14525. doi: 10.1523/JNEUROSCI.1980-13.2013
u Din, M., Raiko, J., Saari, T., Kudomi, N., Tolvanen, T., Oikonen, V., et al. (2016). Human brown adipose tissue [15 O] O 2 PET imaging in the presence and absence of cold stimulus. Eur. J. Nuclear Med. Mol. Imaging 43, 1878–1886.
Ursino, M. G., Vasina, V., Raschi, E., Crema, F., and De Ponti, F. (2009). The β3-adrenoceptor as a therapeutic target: current perspectives. Pharmacol. Res. 59, 221–234.
Ustanina, S., Carvajal, J., Rigby, P., and Braun, T. (2007). The myogenic factor Myf5 supports efficient skeletal muscle regeneration by enabling transient myoblast amplification. Stem Cells 25, 2006–2016. doi: 10.1634/stemcells.2006-0736
van Ooijen, A. M., van Marken Lichtenbelt, W. D., van Steenhoven, A. A., and Westerterp, K. R. (2004). Seasonal changes in metabolic and temperature responses to cold air in humans. Physiol. Behav. 82, 545–553. doi: 10.1016/j.physbeh.2004.05.001
Van Schaik, L., Kettle, C., Green, R., Sievers, W., Hale, M., Irving, H., et al. (2021). Stimulatory, but not anxiogenic, doses of caffeine act centrally to activate interscapular brown adipose tissue thermogenesis in anesthetized male rats. Sci. Rep. 11:113. doi: 10.1038/s41598-020-80505-9
Vanini, G., Bassana, M., Mast, M., Mondino, A., Cerda, I., Phyle, M., et al. (2020). Activation of preoptic GABAergic or glutamatergic neurons modulates sleep-wake architecture, but not anesthetic state transitions. Curr. Biol. 30, 779.–787.
Velickovic, K., Wayne, D., Leija, H. A. L., Bloor, I., Morris, D. E., Law, J., et al. (2019). Caffeine exposure induces browning features in adipose tissue in vitro and in vivo. Sci. Rep. 9:9104. doi: 10.1038/s41598-019-45540-1
Vettor, R., Di Vincenzo, A., Maffei, P., and Rossato, M. (2020). Regulation of energy intake and mechanisms of metabolic adaptation or maladaptation after caloric restriction. Rev. Endocr. Metab. Disord. 21, 399–409. doi: 10.1007/s11154-020-09565-6
Vijgen, G. H., Bouvy, N. D., Teule, G. J., Brans, B., Schrauwen, P., and van Marken Lichtenbelt, W. D. (2011). Brown adipose tissue in morbidly obese subjects. PLoS One 6:e17247. doi: 10.1371/journal.pone.0017247
Villarroya, F., and Vidal-Puig, A. (2013). Beyond the sympathetic tone: the new brown fat activators. Cell Metab. 17, 638–643. doi: 10.1016/j.cmet.2013.02.020
Virtanen, K. A., Lidell, M. E., Orava, J., Heglind, M., Westergren, R., Niemi, T., et al. (2009). Functional brown adipose tissue in healthy adults. N. Engl. J. Med. 360, 1518–1525. doi: 10.1056/NEJMoa0808949
Vranian, M., Blaha, M., Silverman, M., Michos, E., Minder, C., Blumenthal, R., et al. (2012). The interaction of fitness, fatness, and cardiometabolic risk. J. Am. Coll. Cardiol. 59(13 Suppl.):E1754.
Wang, H., Steffen, L. M., Zhou, X., Harnack, L., and Luepker, R. V. (2013). Consistency between increasing trends in added-sugar intake and body mass index among adults: the Minnesota Heart Survey, 1980–1982 to 2007–2009. Am. J. Public Health 103, 501–507.
Wang, Q., Zhang, M., Xu, M., Gu, W., Xi, Y., Qi, L., et al. (2015). Brown adipose tissue activation is inversely related to central obesity and metabolic parameters in adult human. PLoS One 10:e0123795. doi: 10.1371/journal.pone.0123795
Wang, T. A., Teo, C. F., Åkerblom, M., Chen, C., Tynan-La Fontaine, M., Greiner, V. J., et al. (2019). Thermoregulation via temperature-dependent PGD2 production in mouse preoptic area. Neuron 103, 309.–322.
Wankhade, U. D., Shen, M., Yadav, H., and Thakali, K. M. (2016). Novel browning agents, mechanisms, and therapeutic potentials of brown adipose tissue. Biomed. Res. Int. 2016:2365609. doi: 10.1155/2016/2365609
Weyer, C., Gautier, J., and Danforth, E. Jr. (2008). Development of beta 3-adrenoceptor agonists for the treatment of obesity and diabetes an update. Diabetes Metab. 25, 11–21.
Whittle, A., Relat-Pardo, J., and Vidal-Puig, A. (2013). Pharmacological strategies for targeting BAT thermogenesis. Trends Pharmacol. Sci. 34, 347–355. doi: 10.1016/j.tips.2013.04.004
Wiener, I., Tilkian, A. G., and Palazzolo, M. (1990). Coronary artery spasm and myocardial infarction in a patient with normal coronary arteries: temporal relationship to pseudoephedrine ingestion. Cathet. Cardiovasc. Diagn. 20, 51–53. doi: 10.1002/ccd.1810200113
Willey, J. Z., Rodriguez, C. J., Carlino, R. F., Moon, Y. P., Paik, M. C., Boden-Albala, B., et al. (2011). Race-ethnic differences in the association between lipid profile components and risk of myocardial infarction: the Northern Manhattan Study. Am. Heart J. 161, 886–892.
Woodward, J. A., and Saggerson, E. D. (1986). Effect of adenosine deaminase, N6-phenylisopropyladenosine and hypothyroidism on the responsiveness of rat brown adipocytes to noradrenaline. Biochem. J. 238, 395–403. doi: 10.1042/bj2380395
Wu, J. C., and Spiegelman, B. M. (2013). Adaptive thermogenesis in adipocytes: is beige the new brown? Genes Dev. 27, 234–250.
Wu, L., Meng, J., Shen, Q., Zhang, Y., Pan, S., Chen, Z., et al. (2017). Caffeine inhibits hypothalamic A1R to excite oxytocin neuron and ameliorate dietary obesity in mice. Nat. Commun. 8:15904. doi: 10.1038/ncomms15904
Yang, T., Zollbrecht, C., Winerdal, M. E., Zhuge, Z., Zhang, X. M., Terrando, N., et al. (2016). Genetic abrogation of adenosine A3 receptor prevents uninephrectomy and high salt-induced hypertension. J. Am. Heart Assoc. 5:e003868. doi: 10.1161/JAHA.116.003868
Yeh, T.-C., Liu, C.-P., Cheng, W.-H., Chen, B.-R., Lu, P.-J., Cheng, P.-W., et al. (2014). Caffeine intake improves fructose-induced hypertension and insulin resistance by enhancing central insulin signaling. Hypertension 63, 535–541.
Yen, M., and Ewald, M. B. (2012). Toxicity of weight loss agents. J. Med. Toxicol. 8, 145–152. doi: 10.1007/s13181-012-0213-7
Yoneshiro, T., Aita, S., Matsushita, M., Kayahara, T., Kameya, T., Kawai, Y., et al. (2013). Recruited brown adipose tissue as an antiobesity agent in humans. J. Clin. Invest. 123, 3404–3408.
Young, P., Arch, J. R., and Ashwell, M. (1984). Brown adipose tissue in the parametrial fat pad of the mouse. FEBS Lett. 167, 10–14. doi: 10.1016/0014-5793(84)80822-4
Zhang, H., and Rodriguez-Monguio, R. (2012). Racial disparities in the risk of developing obesity-related diseases: a cross-sectional study. Ethn. Dis. 22, 308–316.
Keywords: caffeine, brown adipose tissue, thermogenesis, metabolic homeostasis, metabolsim
Citation: Van Schaik L, Kettle C, Green R, Irving HR and Rathner JA (2021) Effects of Caffeine on Brown Adipose Tissue Thermogenesis and Metabolic Homeostasis: A Review. Front. Neurosci. 15:621356. doi: 10.3389/fnins.2021.621356
Received: 26 October 2020; Accepted: 11 January 2021;
Published: 04 February 2021.
Edited by:
Youichirou Ootsuka, Flinders University, AustraliaReviewed by:
Miguel López, University of Santiago de Compostela, SpainChristopher J. Madden, Oregon Health and Science University, United States
Copyright © 2021 Van Schaik, Kettle, Green, Irving and Rathner. This is an open-access article distributed under the terms of the Creative Commons Attribution License (CC BY). The use, distribution or reproduction in other forums is permitted, provided the original author(s) and the copyright owner(s) are credited and that the original publication in this journal is cited, in accordance with accepted academic practice. No use, distribution or reproduction is permitted which does not comply with these terms.
*Correspondence: Lachlan Van Schaik, ai52YW5zY2hhaWtAbGF0cm9iZS5lZHUuYXU=
†ORCID: Lachlan Van Schaik, orcid.org/0000-0001-9589-661X; Christine Kettle, orcid.org/0000-0003-3223-4090; Rodney Green, orcid.org/0000-0001-8210-285X; Helen R. Irving, orcid.org/0000-0002-1514-0909; Joseph A. Rathner, orcid.org/0000-0002-8734-9402