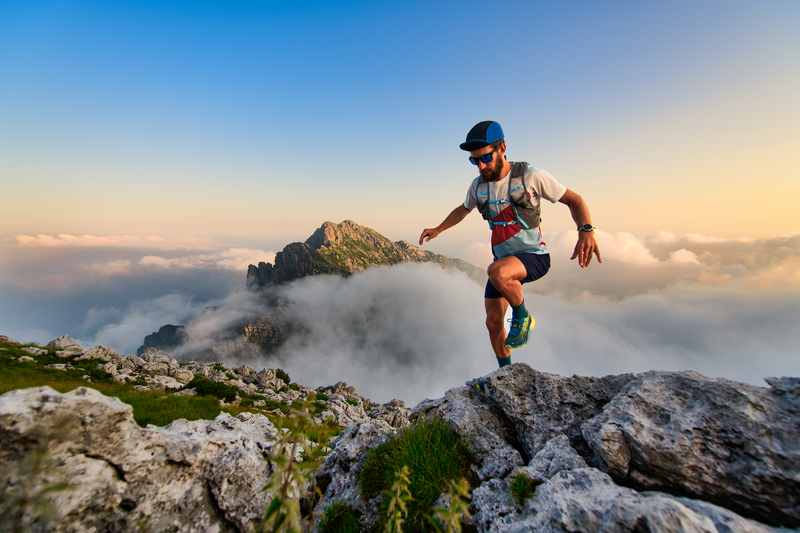
94% of researchers rate our articles as excellent or good
Learn more about the work of our research integrity team to safeguard the quality of each article we publish.
Find out more
ORIGINAL RESEARCH article
Front. Neurosci. , 23 December 2020
Sec. Neuropharmacology
Volume 14 - 2020 | https://doi.org/10.3389/fnins.2020.599646
This article is part of the Research Topic Drug Repurposing in Neurodegenerative and Neuropsychiatric Disorders View all 21 articles
Alcohol use disorder (AUD) is a chronic relapsing condition characterized by compulsive alcohol-seeking behaviors, with serious detrimental health consequences. Despite high prevalence and societal burden, available approved medications to treat AUD are limited in number and efficacy, highlighting a critical need for more and novel pharmacotherapies. Glucagon-like peptide-1 (GLP-1) is a gut hormone and neuropeptide involved in the regulation of food intake and glucose metabolism via GLP-1 receptors (GLP-1Rs). GLP-1 analogs are approved for clinical use for diabetes and obesity. Recently, the GLP-1 system has been shown to play a role in the neurobiology of addictive behaviors, including alcohol seeking and consumption. Here we investigated the effects of different pharmacological manipulations of the GLP-1 system on escalated alcohol intake and preference in male Wistar rats exposed to intermittent access 2-bottle choice of 10% ethanol or water. Administration of AR231453 and APD668, two different agonists of G-protein receptor 119, whose activation increases GLP-1 release from intestinal L-cells, did not affect voluntary ethanol intake. By contrast, injections of either liraglutide or semaglutide, two long-acting GLP-1 analogs, potently decreased ethanol intake. These effects, however, were transient, lasting no longer than 48 h. Semaglutide, but not liraglutide, also reduced ethanol preference on the day of injection. As expected, both analogs induced a reduction in body weight. Co-administration of exendin 9-39, a GLP-1R antagonist, did not prevent liraglutide- or semaglutide-induced effects in this study. Injection of exendin 9-39 alone, or blockade of dipeptidyl peptidase-4, an enzyme responsible for GLP-1 degradation, via injection of sitagliptin, did not affect ethanol intake or preference. Our findings suggest that among medications targeting the GLP-1 system, GLP-1 analogs may represent novel and promising pharmacological tools for AUD treatment.
Alcohol use disorder (AUD) is a chronic relapsing condition characterized by compulsive alcohol-seeking, loss of control in limiting alcohol intake, and the emergence of a negative emotional state that leads to dependence on alcohol and serious detrimental health consequences (Rehm et al., 2009; Koob, 2015). The Food and Drug Administration (FDA) approved medications to treat AUD have shown efficacy, but their effect sizes are sub-optimal. Therefore, there is a critical need for investigating new pharmacotherapies targeting alternative pathways involved in the neurobiology of AUD (Klein, 2016; Farokhnia et al., 2019a; Witkiewitz et al., 2019).
Glucagon-like peptide-1 (GLP-1) is a gut neuropeptide hormone involved in the regulation of food intake and glucose homeostasis via GLP-1 receptors (GLP-1Rs). GLP-1 is produced both in the periphery, mainly by intestinal L-cells (Novak et al., 1987), and in several brain regions including the nucleus tractus solitarius (NTS), mainly by preproglucagon (PPG) neurons (Larsen et al., 1997; Merchenthaler et al., 1999). In vivo, the half-life of GLP-1 is very short (1.5 min following intravenous dosing and 1.5 h following subcutaneous dosing in humans), mainly due to degradation by the dipeptidyl peptidase-4 (DPP-4) enzyme (Deacon et al., 1995a, b). GLP-1R is a member of the secretin-like class B family of G-protein coupled receptors (GPCRs) expressed in various peripheral tissues, including vagal afferents, hepatic portal system and pancreatic β-cells, where they regulate food intake and glycemia by delaying gastric emptying, decreasing glucagon release and glucose-dependent stimulation of insulin secretion (Orskov et al., 1988; Flint et al., 2001; Kanoski et al., 2011). The critical role of GLP-1 in glucose homeostasis, food intake, and energy metabolism has led to the development of multiple FDA-approved GLP-1 analogs, such as exenatide, liraglutide, and semaglutide, for type 2 diabetes treatment (Agersø et al., 2002; Estall and Drucker, 2006; Eng et al., 2014; Lau et al., 2015). Liraglutide is also approved for the treatment of obesity. To overcome the rapid enzymatic degradation of GLP-1 and to prolong GLP-1R activation, liraglutide and semaglutide were designed by adding fatty-acid chains to the GLP-1 peptide, enhancing their binding affinity to albumin and protecting them against DPP-4 enzymatic degradation and renal filtration, while preserving their GLP-1R potency (Knudsen and Lau, 2019). Due to their prolonged half-lives, liraglutide and semaglutide are referred to as long-acting GLP-1 analogs, and are prescribed once daily and once weekly, respectively (Knudsen and Lau, 2019).
In the central nervous system (CNS), GLP-1Rs are expressed in several brain regions involved in regulating metabolism and energy balance, with preferential localization in inhibitory GABAergic neurons over excitatory glutamatergic neurons (Hayes et al., 2010; Cork et al., 2015; Fortin et al., 2020; Graham et al., 2020). In the hypothalamus, activation of GLP-1Rs has been shown to regulate food intake, glycemia, and stress responses (Kinzig et al., 2003; Sandoval et al., 2008; Hayes et al., 2010; Ghosal et al., 2017). GLP-1Rs are also found in reward-related brain regions that regulate appetitive and consummatory behaviors, such as the nucleus accumbens (NAc), ventral tegmental area (VTA), and amygdala (Göke et al., 1995; Alvarez et al., 1996; Merchenthaler et al., 1999; Cork et al., 2015; Graham et al., 2020). Recently, rodent studies have shown that GLP-1 analogs reduce the rewarding effects of several drugs of abuse, including alcohol, primarily via activation of central GLP-1Rs (Egecioglu et al., 2013a, b; Shirazi et al., 2013; Suchankova et al., 2015; Sirohi et al., 2016; Vallöf et al., 2016, 2019b). In humans, genetic variation in GLP-1R was found to be associated with increased risk of AUD, increased alcohol self-administration in a laboratory setting, and brain activity when being notified about the receipt of a monetary reward (Suchankova et al., 2015). Hence, GLP-1 analogs, already deemed to have a favorable safety profile in humans, are increasingly gaining attention as a novel therapeutic approach to treat AUD.
Recent studies have also renewed interest in the G protein-coupled receptor 119 (GPR119), a member of the class A of rhodopsin-like GPCRs, for its role in glucose homeostasis (Fredriksson et al., 2003; Soga et al., 2005). GPR119 is expressed in limited tissues, including the pancreatic islets and gastrointestinal tract. Activation of GPR119 via both direct actions on pancreatic β-cells and indirect actions on intestinal L-cells results in increased glucose-dependent insulin release and increased gene expression of proglucagon, a biosynthetic precursor of GLP-1, with subsequent release of endogenous GLP-1, glucose-dependent insulinotropic peptide, neurotensin, and peptide YY, leading to improvement of glucose tolerance in rodents and humans (Overton et al., 2006; Chu et al., 2007, 2008; Hansen et al., 2011; Katz et al., 2011; Lan et al., 2012; Chepurny et al., 2013; Mandøe et al., 2015; Hassing et al., 2016; Han et al., 2018; Matsumoto et al., 2018). Its restricted tissue distribution, relative selectivity in enhancing GLP-1 release, and tolerability of its agonists have made GPR119 an attractive therapeutic target for developing orally bioavailable agonists for type 2 diabetes treatment (Terauchi et al., 2018; Yang et al., 2018), and potentially for other related disorders.
In the present study we investigated the effects of different pharmacological manipulations of the GLP-1 system on escalated voluntary ethanol intake and preference, water intake, and body weight in male Wistar rats, in order to determine the potential utility of these compounds in the treatment of AUD. Specifically, we tested two GPR119 agonists, AR231453 and APD668, two long-acting GLP-1 analogs, liraglutide and semaglutide, a blocker of DPP-4, sitagliptin, and another peptide putatively working as a GLP-1R antagonist, exendin 9-39 (Ex9-39).
All experiments were performed in accordance with the guidance of the National Institutes of Health on animal care and use and the University of California, Los Angeles, Animal Research Committee. All rats were housed individually in the vivarium under a 12h light/dark cycle (lights on at 6AM) and had ad libitum access to food and water during the entire experiment. Two distinct cohorts of rats were used. Rats were included in the analyses only if the average ethanol preference from the last 3 presentations prior to vehicle administration reached 45% or higher. In the first cohort, 12 out of 17 rats reached this criterion. Thus, a total of 12 male Wistar rats (Envigo) weighing 260–300 g at the start of the experiment were used in the first cohort to study the effects of AR231453, APD668, liraglutide and semaglutide (administered alone and in combination with exendin 9-39), and sitagliptin. In the second cohort, 13 out of 15 rats reached the criterion mentioned above. However, one rat was identified as an outlier (water intake), using the ROUT method combining robust regression and outlier removal with Q = 1% (Motulsky and Brown, 2006), and therefore was removed from the study. Thus, a total of 12 male Wistar rats weighing 270–290 g at the start of the experiment were used in the second cohort to study the effects of exendin 9-39 alone.
Ethanol (EtOH, 95%, Decon Labs Inc., King of Prussia, PA, United States), meeting the United States Pharmacopeia (USP) specifications, was used to make all EtOH-containing solutions. The intermittent access to 2-bottle choice (IA2BC) drinking paradigm has been previously described (Simms et al., 2008; Meyer et al., 2013). This model leads rats to gradually escalate EtOH intake to high levels (4–6 g/kg/24h) without resorting to sucrose fadeout procedures or forced EtOH administration (Wise, 1975; Cippitelli et al., 2012). The IA2BC paradigm provides a platform to address multiple aspects of alcohol abuse in a rat model, including transition from social-like drinking to excessive alcohol consumption, binge drinking, alcohol seeking, relapse, and neuroadaptations related to excessive alcohol intake (Carnicella et al., 2014). Although some labs, including ours, report minimal alcohol deprivation effects (Simms et al., 2008; Meyer et al., 2013), others report marked withdrawal behaviors, such as anxiety-like symptoms and hyperalgesia after withdrawal from > 8 weeks of IA2BC (Kang et al., 2019; Li et al., 2019; Fu et al., 2020). Peak plasma EtOH concentrations obtained following 30 min of IA2BC 20% EtOH range between 4 and 93 mg/dL in Wistar rats (Simms et al., 2008); with a blood EtOH concentration of > 80 mg/dL meeting the criteria of the National Institute on Alcohol Abuse and Alcoholism (NIAAA) for binge drinking in humans. The model also has some predictive validity, e.g., the FDA-approved medication acamprosate attenuates drinking in the IA2BC but not in continuous access models (Simms et al., 2008; Cippitelli et al., 2012). All fluids were presented in 250-ml graduated plastic bottles with stainless steel low-leak drinking spouts accessible to rats through the top of their home cage. Rats were given access to 1 bottle of drinking water and 1 bottle of EtOH (10%, w/v) solution for a 24-h period on Mondays, Wednesdays, and Fridays. Bottles were weighed at the beginning of each 24-h drinking period, at approximately 1 h before the dark cycle (pre-injection). Measurements were taken to the nearest 1/100 g. The weight of each rat was also measured at the start of the 24-h drinking period and used to calculate the grams of solution consumed per kilogram of body weight per 24-h drinking session (g/kg/day). Preference for EtOH was calculated as the ratio of EtOH-containing solution over the total fluid consumed in a 24-h drinking session. Experimental EtOH-containing solutions were prepared in drinking water provided by UCLA veterinary staff. Upon completion of each drinking session, the EtOH-containing solution was replaced with a second water bottle until the next presentation. The rats had unlimited access to 2 bottles of water over the weekend after the 24-h measurements were taken on Saturday. Bottle placement was alternated each drinking session to control for side preferences. EtOH presentations were made until after the rats had reached a steady high level of drinking before drug administration. After reaching steady high level of drinking, rats were habituated to be immobilized in a plastic cone (DC 200, Braintree Scientific, Inc., Braintree, MA, United States) for about 5 s, just prior to EtOH presentation, in order to habituate them to the condition experienced during drug or vehicle injection. For injections, rats were immobilized in a plastic cone for the entire duration of the injection (∼5 s). Effects of drugs on body weight were determined by comparing the body weight measured during the day of injection (pre-injection) to the body weight measured at subsequent post-injection time-points.
All drugs were injected intraperitoneally between 4 and 5 PM, just before the start of the IA2BC EtOH presentation. Doses were chosen based on each drug’s EC50/IC50 or their reported effectiveness at modulation of EtOH intake. Vehicle was composed of DMSO:Tween 80:saline at 1:1:10 ratio. Two different GPR119 agonists, AR231453 (Millipore Sigma, Burlington, MA, United States) and APD668 (AOBIOUS, Gloucester, MA, United States), were used to stimulate GLP-1 release (Semple et al., 2008, 2011). AR231453 was dissolved in vehicle to a final dilution of 4 mg/ml and was injected at a concentration of 3 mg/kg. To overcome potential solubility issues, APD668 was dissolved in two different vehicles (DMSO or polyethylene glycol 400 – PEG-8) to a final dilution of 8 mg/ml and was injected at a concentration of 6 mg/kg. Two long-acting GLP-1 analogs, liraglutide (Cayman Chemical, Ann Arbor, MI, United States) and semaglutide (MedChem Express, Monmouth Junction, NJ, United States) were used (Larsen et al., 2001; Lau et al., 2015). Liraglutide and semaglutide were dissolved in vehicle to a final dilution of 1 mg/ml and injected at a concentration of 0.1 mg/kg. Exendin 9-39 (Ex9-39; Bachem, Torrance, CA, United States), a GLP-1R antagonist (Schepp et al., 1994), was dissolved in saline to a final dilution of 0.13 mg/ml and was injected at a concentration of 0.1 mg/kg. When co-administered with liraglutide (0.1 mg/kg) or semaglutide (0.1 mg/kg), Ex9-39 was injected at a concentration of 0.15 mg/kg or 0.19 mg/kg, respectively. Sitagliptin phosphate (Millipore Sigma, Burlington, MA, United States), an inhibitor of DPP-4, was dissolved in saline to a final dilution of 33.3 mg/ml and was injected at a concentration of 25 mg/kg.
Statistical analyses were performed using Prism 7 (GraphPad Software Inc., La Jolla, CA, United States) and data were expressed as mean ± standard error of the mean (SEM). All data sets were tested for outliers using the ROUT method (Motulsky and Brown, 2006). Two-way repeated-measures analyses of variance (RM ANOVA) was used to assess significance of the effects of treatment (vehicle vs. drug), time-point (baseline, injection day, + 2 days) and treatment × time-point interaction on EtOH intake, EtOH preference, and water intake. Given that the treatment × time-point interaction effect was our primary outcome of interest, when the interaction term was found to be significant, Tukey’s post hoc test was used to further identify statistical significance between treatments and/or time-points. For the two-way RM ANOVAs, the baseline time-point represents the average of 3 presentations prior to vehicle/drug injection. The injection day time-point represents the value of EtOH intake, EtOH preference or water intake measured 24-h post-injection. The detailed two-way RM ANOVA tables, including treatment, time-point, and treatment × time-point interaction effects are presented in Supplementary Tables 1–3. Statistical power was calculated each time a two-way RM ANOVA revealed a significant treatment × time-point interaction effect, using G∗Power (version 3.1.9.6, Ute Clames), and was reported only when the value was below 0.8. We found two instances where the statistical power was lower than 0.8 (EtOH intake under APD668 (DMSO) and Ex9-39), and report these results as potentially false positives. Because body weight was consistently increasing as the cohort’s aged, we used one-way RM ANOVAs, followed by Dunnett’s post hoc tests, to assess the effect of each drug on body weight. For these one-way RM ANOVAs, the pre-injection time-point represents the body weight measured 1 h prior to vehicle/drug injection on the day of injection, which was compared to the body weight measured at subsequent post-injection time-points. For AR231453 and APD668 experiments, the pre-injection body weight was compared to two post-injection time-points (+ 2-day and + 5-day). For liraglutide and semaglutide experiments (with and without Ex9-39), the pre-injection body weight was compared to five post-injection time-points (+ 2-day, + 5-day, + 7-day, + 9-day, and + 12-day) to assess the time course of recovery from the drug-induced weight loss. For sitagliptin experiments, the pre-injection body weight was compared to two post-injection time-points (+ 2-day and + 7-day; body weights measured from the + 5-day time-point were accidently lost). For the Ex9-39 alone experiment, the pre-injection body weight was compared to two post-injection time-points (+ 2-day and + 5-day). A value of p < 0.05 was considered statistically significant (∗p < 0.05, ∗∗p < 0.01, ∗∗∗p < 0.001).
Activation of GPR119 can increase the release of plasma GLP-1 in rodents and humans (Chu et al., 2008; Hansen et al., 2011; Katz et al., 2011; Lan et al., 2012; Mandøe et al., 2015; Han et al., 2018; Matsumoto et al., 2018). Since GLP-1R activation has been shown to modulate EtOH consumption (Shirazi et al., 2013; Vallöf et al., 2016), we first investigated whether activation of GPR119 alters EtOH intake and preference, as well as water intake and body weight, using two different GPR119 agonists, AR231453 (3 mg/kg) or APD668 (6 mg/kg) (Figures 1A–D). For AR231453, the two-way RM ANOVAs did not find significant treatment × time-point interaction effects on EtOH intake (F(2,22) = 1.89, p = 0.17) (Figure 2A), EtOH preference (F(2,22) = 0.14, p = 0.87) (Figure 2D), or water intake (F(2,22) = 0.18, p = 0.83) (Figure 2G). For APD668 dissolved in DMSO, a significant treatment × time-point interaction effect was observed on EtOH intake (F(2,22) = 5.75, p = 0.0098) (Figure 2B), but due to low statistical power (power = 0.51), these results were concluded to be potentially false positive. Moreover, APD668 (DMSO) did not affect EtOH preference (treatment × time-point interaction: F(2,22) = 2.49, p = 0.11) (Figure 2E), or water intake (treatment × time-point interaction: F(2,22) = 2.85, p = 0.08) (Figure 2H). Consistent with these findings, APD668 dissolved in PEG did not affect EtOH intake (treatment × time-point interaction: F(2,22) = 2.79, p = 0.083) (Figure 2C), EtOH preference (treatment × time-point interaction: F(2,22) = 1.77, p = 0.19) (Figure 2F), or water intake (treatment × time-point interaction: F(2,22) = 2.44, p = 0.11 (Figure 2I).
Figure 1. Overall measurements of EtOH intake, EtOH preference, water intake, and body weight during the experiment. (A–D) Dashed lines represent the time of injection of each drug. Vehicle was injected at day 36. AR2314553 (light green circle) was injected at day 43. APD668 (DMSO) (green circle) was injected at day 68. APD668 (PEG) (dark green circle) was injected at day 92. Liraglutide (Lira, light blue circle) was injected at day 108. Semaglutide (Sema, lavender circle) was injected at day 122. Liraglutide + Ex9-39 (Lira + Ex9-39, blue circle) was injected at day 143. Semaglutide + Ex9-39 (Sema + Ex9-39, purple circle) was injected at day 171. Sitagliptin (Sitag, yellow circle) was injected at day 199. (A) Time course of the average EtOH intake (g/kg/day) (n = 12 rats). EtOH intake was measured on Mondays, Wednesdays, and Fridays from day 1 to 206. From day 1 to 29, rats progressively drank higher amounts of EtOH 10% (v/w) until reaching a plateau. (B) Time course of the average EtOH preference (%/day) (n = 12 rats). EtOH preference was calculated as the ratio of EtOH-containing solution over the total fluid consumed in a 24-h drinking session (see section “Materials and Methods”). EtOH preference progressively increased until reaching a plateau. (C) Time course of the average water intake (g/kg/day) (n = 12 rats). Water intake progressively decreased until reaching a plateau. (D) Time course of the average body weight (g) (n = 12 rats). The average body weight was 280 ± 2.96 g at day 1, and progressively increased throughout the experiment to reach an average of 623 ± 22.56 g at day 206.
Figure 2. Effects of GPR119 agonists [AR231453, APD668 (DMSO), and APD668 (PEG)], compared to vehicle, on EtOH intake, preference, water intake, and body weight. Bars and circles represent the mean and individual data points, respectively. (A–I) Baseline represents the average of 3 presentations prior to vehicle/drug injection. (A–C) No significant treatment × time-point interaction effects on EtOH intake were found (note: the interaction term for APD668 (DMSO) was statistically significant, but due to low power, these results were concluded to be potentially false positive). (D–F) No significant treatment × time-point interaction effects on EtOH preference were found. (G–I) No significant treatment × time-point interaction effects on water intake were found. (J,K) Significant increases in body weight were observed under vehicle and AR231453, as shown by significant increases in body weight measured at 2-day (+ 2d) and 5-day (+ 5d) post-injection compared to the body weight measured on the day of injection (pre-injection). (L,M) No significant changes in body weight were observed under APD668 (DMSO) or APD668 (PEG), as shown by the lack of significant increases in body weight measured at 2-day (+ 2d) and 5-day (+ 5d) post-injection compared to the body weight measured on the day of injection (pre-injection). ***p < 0.001.
As expected, and consistent with the overall trend as the rats aged (Figure 1D), body weight gain was observed following vehicle injection at 2-day (+ 2d) and 5-day (+ 5d) post-injection (F(2,22) = 67.27, p < 0.001; post hoc test, pre-injection vs. + 2d, p < 0.001; pre-injection vs. + 5d, p < 0.001) (Figure 2J). Similarly, increases in body weight was found at + 2d and + 5d following AR231453 injection (F(2,22) = 29.17, p < 0.001; post hoc test, pre-injection vs. + 2d, p < 0.001; pre-injection vs. + 5d, p < 0.001) (Figure 2K). In contrast, rats injected with APD668 (dissolved in either DMSO or PEG) did not gain weight following injections (APD668 (DMSO): F(2,22) = 0.7, p = 0.45; APD668 (PEG): F(2,22) = 1.1, p = 0.33) suggesting a putative effect of APD668 in body weight regulation (i.e., prevention of weight gain in this case) (Figures 2L,M).
GLP-1R agonists have been shown to decrease voluntary EtOH intake in rodents suggesting a role for these ligands in the treatment of AUD (Shirazi et al., 2013; Vallöf et al., 2016). We found that both liraglutide (0.1 mg/kg) and semaglutide (0.1 mg/kg), two long-acting GLP-1 analogs, produced significant decreases in EtOH intake (liraglutide: treatment × time-point interaction: F(2,22) = 47.5, p < 0.001; post hoc test, baseline vs. liraglutide injection day: p < 0.001; semaglutide: treatment × time-point interaction: F(2,22) = 65.75, p < 0.001; post hoc test, baseline vs. semaglutide injection day: p < 0.001). Of note, the effects of liraglutide and semaglutide were transient, lasting no longer than the 2-day post-injection time-point (liraglutide: baseline vs. + 2d: p = 0.89; semaglutide: baseline vs. + 2d: p = 0.35) (Figures 3A,B).
Figure 3. Effects of GLP-1 analogs (liraglutide and semaglutide), compared to vehicle, on EtOH intake, preference, water intake, and body weight. Bars and circles represent the mean and individual data points, respectively. (A–F) Baseline represents the average of 3 presentations prior to vehicle/drug injection. (A) A significant treatment × time-point interaction effect on EtOH intake was found for liraglutide; under liraglutide, EtOH intake at the injection day was lower than baseline and 2-day post-injection (+ 2d). (B) A significant treatment × time-point interaction effect on EtOH intake was found for semaglutide; under semaglutide, EtOH intake at the injection day was lower than baseline and + 2d. (C) A significant treatment × time-point interaction effect on EtOH preference was found for liraglutide; under liraglutide, EtOH preference at + 2d was lower than baseline. (D) A significant treatment × time-point interaction effect on EtOH preference was found for semaglutide; under semaglutide, EtOH preference at the injection day was lower than baseline and + 2d. (E) A significant treatment × time-point interaction effect on water intake was found for liraglutide; under liraglutide, water intake at + 2d was higher than baseline and the injection day. (F) No significant treatment × time-point interaction effect on water intake was found for semaglutide. (G) Significant changes in body weight were observed under liraglutide; body weight at + 2d, + 5d, + 7d, and + 9d was lower than the body weight measured prior injection on injection day (pre-injection). (H) Significant changes in body weight were observed under semaglutide; body weight at + 2d was lower, and at + 12d was higher, than the body weight measured prior injection on injection day (pre-injection). *p < 0.05, **p < 0.01, ***p < 0.001.
Statistical analyses also showed a significant treatment × time-point interaction for the effects of liraglutide on EtOH preference (F(2,22) = 4.29, p = 0.03). However, post hoc testing only found a significance difference between baseline and 2-day post-injection time-points (baseline vs. liraglutide injection day: p = 0.18; baseline vs. + 2d: p = 0.011; liraglutide injection day vs. + 2d: p = 0.38) (Figure 3C). We also found that liraglutide significantly altered water intake with a significant increase at the 2-day post-injection time-point (treatment × time-point interaction: F(2,22) = 17.78, p < 0.001; post hoc test, baseline vs. + 2d: p < 0.001; liraglutide injection day vs. + 2d: p < 0.001) (Figure 3E). These data suggest that liraglutide-induced increase in water intake at + 2d, while not altering EtOH intake at this time-point (i.e., EtOH intake returned back to baseline), may be responsible for the decrease in EtOH preference observed at 2-day post-injection, rather than a direct effect on alcohol preference. By contrast, semaglutide significantly decreased EtOH preference at the injection day time-point (treatment × time-point interaction: F(2,22) = 14.01, p < 0.001; post hoc test, baseline vs. semaglutide injection day, p < 0.001; semaglutide injection day vs. + 2d, p < 0.001), without affecting water intake (treatment × time-point interaction: F(2,22) = 2.03, p = 0.16) (Figures 3D,F). These data suggest that while both GLP-1 analogs can reduce EtOH intake, semaglutide appears to be more selective for EtOH as it also reduced EtOH preference without affecting water intake.
Body weight was influenced by both GLP-1 analogs. Liraglutide induced long-lasting decreases in body weight up to 9 days post-injection (+ 9d) (F(5,55) = 101.2, p < 0.001; post hoc test, pre-injection vs. + 2d, p < 0.001; pre-injection vs. + 5d, p < 0.001; pre-injection vs. + 7d, p = 0.0012; pre-injection vs. + 9d, p = 0.014) (Figure 3G). By contrast, semaglutide induced only a transient decrease in body weight lasting no longer than 48 h, with a significant increase observed at 12 days post-injection (F(5,55) = 19.4, p < 0.001; post hoc test, pre-injection vs. + 2d, p = 0.005; pre-injection vs. + 12d, p = 0.02) (Figure 3H).
Ex9-39 has been shown to antagonize the effects of GLP-1R activation in rodents and humans (Thorens et al., 1993; Wang et al., 1995; Edwards et al., 1999; Vallöf et al., 2019a). Blockade of GLP-1R by co-administration of Ex9-39 did not prevent the decrease in EtOH intake induced by liraglutide (treatment × time-point interaction: F(2,22) = 49.22, p < 0.001; post hoc test, baseline vs. liraglutide + Ex9-39 injection day, p < 0.001; liraglutide + Ex9-39 injection day vs. + 2d, p < 0.001) (Figure 4A). Similarly, co-administration of Ex9-39 did not prevent the decrease in EtOH intake induced by semaglutide (treatment × time-point interaction: F(2,22) = 62.89, p < 0.001; post hoc test, baseline vs. semaglutide + Ex9-39 injection day, p < 0.001; baseline vs. + 2d, p = 0.005; semaglutide + Ex9-39 injection day vs. + 2d, p < 0.001) (Figure 4B).
Figure 4. Effects of GLP-1 analogs (liraglutide and semaglutide) co-administered with a GLP-1R antagonist (Ex9-39), compared to vehicle, on EtOH intake, preference, water intake, and body weight. Bars and circles represent the mean and individual data points, respectively. (A–F) Baseline represents the average of 3 presentations prior to vehicle/drug injection. (A) A significant treatment × time-point interaction effect on EtOH intake was found for liraglutide + Ex9-39; under liraglutide + Ex9-39, EtOH intake at the injection day was lower than baseline and 2-day post-injection (+ 2d). (B) A significant treatment × time-point interaction effect on EtOH intake was found for semaglutide + Ex9-39; under semaglutide + Ex9-39, EtOH intake at the injection day was lower than baseline and + 2d, and EtOH intake at + 2d was higher than baseline. (C) A significant treatment × time-point interaction effect on EtOH preference was found for liraglutide + Ex9-39; under liraglutide + Ex9-39, EtOH preference at + 2d was lower than baseline. (D) A significant treatment × time-point interaction effect on EtOH preference was found for semaglutide + Ex9-39; under semaglutide + Ex9-39, EtOH preference at the injection day was lower than baseline and + 2d. (E) A significant treatment × time-point interaction effect on water intake was found for liraglutide + Ex9-39; under liraglutide + Ex9-39, water intake at + 2d was higher than baseline and the injection day. (F) No significant treatment × time-point interaction effect on water intake was found for semaglutide + Ex9-39. (G) Significant changes in body weight were observed under liraglutide + Ex9-39; body weight at + 2d, and + 7d was lower than the body weight measured on injection day (pre-injection). (H) Significant changes in body weight were observed under semaglutide + Ex9-39; body weight at + 2d was lower than the body weight measured on injection day (pre-injection). *p < 0.05, **p < 0.01, ***p < 0.001.
Similar to liraglutide alone, liraglutide + Ex9-39 significantly decreased EtOH preference only at 2-day post-injection (treatment × time-point interaction: F(2,22) = 3.75, p = 0.04; post hoc test, baseline vs. + 2d, p = 0.03) (Figure 4C), which was concomitant with significant increase in water intake (treatment × time-point interaction: F(2,22) = 12.04, p < 0.001; post hoc test, baseline vs. + 2d, p < 0.001, liraglutide + Ex9-39 vs. + 2d, p < 0.001) (Figure 4E). Co-administration of Ex9-39 did not prevent the decrease in EtOH preference induced by semaglutide (treatment × time-point interaction: F(2,22) = 3.83, p = 0.037; post hoc test, baseline vs. semaglutide + Ex9-39, p = 0.009; semaglutide + Ex9-39 vs. + 2d, p = 0.015) (Figure 4D). Semglutide + Ex9-39 did not affect water intake (treatment × time-point interaction: F(2,22) = 1.1, p = 0.35) (Figure 4F).
Liraglutide + Ex9-39 induced a transient decrease in body weight (F(5,55) = 30.65, p < 0.001; post hoc test, pre-injection vs. + 2d, p < 0.001; pre-injection vs. + 7d, p = 0.02) (Figure 4G). Similarly, semaglutide + Ex9-39 induced a reduction in body weight at 2-day post-injection (F(5,55) = 18.5, p < 0.001; post hoc test, pre-injection vs. + 2d, p = 0.01) (Figure 4H).
Next, we investigated whether increasing endogenous GLP-1 levels by preventing its enzymatic degradation via DPP-4 could decrease EtOH intake and preference. Here, we found that sitagliptin did not affect EtOH intake (treatment × time-point interaction: F(2,22) = 0.95, p = 0.40) (Figure 5A), EtOH preference (treatment × time-point interaction: F(2,22) = 0.55, p = 0.58) (Figure 5B), or water intake (treatment × time-point interaction: F(2,22) = 1.32, p = 0.29) (Figure 5C). In addition, no changes in body weight were observed with sitagliptin (F(2,22) = 1.44, p = 0.26) (Figure 5D).
Figure 5. Effects of a DPP4 inhibitor (sitagliptin), compared to vehicle, on EtOH intake, preference, water intake, and body weight. Bars and circles represent the mean and individual data points, respectively. (A–C) Baseline represents the average of 3 presentations prior to vehicle/drug injection. No significant treatment × time-point interaction effects on EtOH intake, EtOH preference, or water intake were found. (D) No significant changes in body weight were observed.
A previous study showed that a single dose of the GLP-1R antagonist Ex9-39 increased voluntary EtOH intake in rats (Shirazi et al., 2013). Considering these data and our findings that co-administration of Ex9-39 did not block the effects of liraglutide or semaglutide on EtOH intake, we investigated, in a different cohort of rats, whether injection of Ex9-39 alone, compared to vehicle, would affect EtOH intake, preference, water intake, and/or body weight (Figures 6A–D). A significant treatment × time-point interaction effect of Ex9-39 on EtOH intake was observed (F(2,22) = 3.8, p = 0.038) (Figure 6E), but due to low statistical power (power = 0.57), there results were concluded to be potentially false positive. Moreover, Ex9-39 did not affect EtOH preference (treatment × time-point interaction: F(2,22) = 0.19, p = 0.82) (Figure 6F), or water intake (treatment × time-point interaction: F(2,22) = 0.01, p = 0.99) (Figure 6G). We also found that body weight significantly increased at 2- and 5-day post-injection of Ex9-39 (F(2,22) = 8.56, p = 0.01; post hoc test, pre-injection vs. + 2d, p = 0.04; pre-injection vs. + 5d, p = 0.02) (Figure 6H).
Figure 6. Overall measurements of EtOH intake, EtOH preference, water intake, and body weight for the second cohort of rats (n = 12). (A–D) Dashed lines represent the time of injection of vehicle (day 45) and Ex9-39 (day 52). (E–G) Effects of Ex9-39, compared to vehicle, on EtOH intake, preference, water intake; bars and circles represent the mean and individual data points, respectively. Baseline represents the average of 3 presentations prior to vehicle/drug injection. No significant treatment × time-point interaction effects on EtOH intake, EtOH preference, or water intake were found (note: the effect of the interaction term on EtOH intake was statistically significant, but due to low power, these results were concluded to be potentially false positive). (H) Effects of Ex9-39 on body weight. Significant changes in body weight were observed; body weight at + 2d and + 5d was higher than the body weight measured on injection day (pre-injection). *p < 0.05.
Increasing evidence points to an important role of gut-brain peptides, including GLP-1, in modulating the biobehavioral correlates of alcohol use (Thorsell and Mathé, 2017; Jerlhag, 2018; Farokhnia et al., 2019b; von Holstein-Rathlou and Gillum, 2019; Hopf, 2020). Here, we found that GLP-1 analogs, liraglutide and semaglutide, potently, albeit transiently, decreased voluntary EtOH intake. Notably, semaglutide also reduced alcohol preference. Administration of Ex9-39, an inhibitor of GLP-1R, failed to prevent liraglutide- and semaglutide-induced decreases in EtOH intake. Sitagliptin, a blocker of DPP-4, the enzyme responsible for GLP-1 degradation, did not affect EtOH intake or preference. Similarly, activation of GPR119, which has been shown to promote endogenous GLP-1 release, had no effect on EtOH intake. Altogether, our findings support the hypothesis that direct pharmacological agonism of the GLP-1 receptor may represent an effective approach for AUD treatment.
Several recent studies in rodents have highlighted the role of the GLP-1 system in regulating addictive behaviors related to different drugs of abuse, including nicotine, cocaine, opioids, and alcohol, suggesting a new and promising pharmacotherapeutic target for drug addiction (Egecioglu et al., 2013a, b; Graham et al., 2013; Shirazi et al., 2013; Harasta et al., 2015; Suchankova et al., 2015; Sørensen et al., 2015; Sirohi et al., 2016; Vallöf et al., 2016, 2019b, 2020; Fortin and Roitman, 2017; Tuesta et al., 2017; Bornebusch et al., 2019; Zhang et al., 2020). Here, we described, for the first time, that liraglutide and semaglutide had similar transient inhibitory effects on EtOH intake, but different effects on EtOH preference. In humans, liraglutide and semaglutide have an estimated half-life of 13 and 165 h, respectively (Agersø et al., 2002; Kapitza et al., 2015). Because of its longer half-life and long-acting properties, semaglutide is injected once weekly, as opposed to liraglutide which is injected once daily (Hall et al., 2018). In animals, liraglutide and semaglutide have an estimated half-life of 23 and 64 h, respectively, after subcutaneous administration (Lau et al., 2015). Although previous studies have investigated acute administration of liraglutide, they only reported its effects over a 24-h period, leaving room for speculation about possible time course and persistence of its effect on EtOH intake (Shirazi et al., 2013; Sirohi et al., 2016; Vallöf et al., 2016). The lack of long-lasting effects of GLP-1 analogs on EtOH intake after acute injection could be due to a faster metabolism of GLP-1 analogs in EtOH drinking animals and/or the need for repeated injections. In support of this hypothesis, long-lasting effects of liraglutide and dulaglutide on EtOH intake have been demonstrated after their daily administration for several consecutive weeks (Vallöf et al., 2016, 2020). However, relatively long-lasting effects of GLP-1 analogs on body weight were observed in the present study. Altogether, these findings suggest that the suppressing effects of GLP-1 analogs on EtOH intake and body weight may be mediated through different mechanisms – a question beyond the scope of the present study.
Malaise and nausea are common side effects of GLP-1 analogs (Buse et al., 2009; Hall et al., 2018), and could be, at least partially, responsible for their effects on EtOH intake, food intake, and body weight. Indeed, concentration higher than 50 μg/kg (i.p.) of liraglutide induces conditioned taste aversion (CTA) (Kanoski et al., 2012). However, lower dose of liraglutide (10 μg/kg, i.p.) has been found to reduce chow consumption without inducing CTA, suggesting that nausea does not solely account for the effects of higher concentration of liraglutide on food intake (Kanoski et al., 2012). In this study, we found that liraglutide significantly decreased EtOH intake without affecting EtOH preference on the day of injection. However, liraglutide significantly decreased EtOH preference at 2-day post-injection, while concomitantly increasing water intake at this time-point. These data tentatively suggest that liraglutide’s effects may not be selective for EtOH. Indeed, liraglutide has been shown to affect water intake after peritoneal injection (McKay et al., 2011). In contrast to liraglutide, semaglutide significantly decreased EtOH preference and did not affect water intake, suggesting that its effects are more selective for EtOH. Consistent with our findings, previous studies suggest that the effects of GLP-1 analogs in reducing EtOH intake are due to reduced EtOH rewarding properties rather than nausea, which is a common side effect of GLP-1 analogs (Buse et al., 2009; Egecioglu et al., 2013b; Shirazi et al., 2013; Sirohi et al., 2016; Vallöf et al., 2016, 2019a, 2020; Hall et al., 2018). Altogether, our results suggest that semaglutide is more specific for alcohol than liraglutide, and given its more favorable prescription dosing (i.e., once a week), may be better suited for use in patients with AUD. However, clinical trials will be needed to test and compare the safety, efficacy, and patients’ compliance to semaglutide and liraglutide as AUD treatment options.
Previous findings that the inhibitory effect of exendin-4 on EtOH intake was blunted in mice lacking GLP-1Rs in the CNS suggest that central GLP-1Rs play a key role in mediating the effects of GLP-1 analogs on EtOH intake (Sirohi et al., 2016). A recent study demonstrated that microinjection of Ex9-39 into the NTS was able to prevent the suppressing effect of exendin-4 on EtOH-induced increase in locomotor activity (Vallöf et al., 2019a). Here, we showed that co-administration of Ex9-39 with liraglutide or semaglutide did not prevent the reduction in EtOH intake and weight. The lack of inhibitory effects of Ex9-39 on GLP-1 analog actions could be due to differences in the half-lives of the drugs. While the exact metabolism profile of Ex9-39 is unclear, it is expected that its half-life would be similar to that of its parent peptide, exendin-4 (Göke et al., 1993), which is 33 min after intravenous and 2.5 h after subcutaneous administration in humans (Edwards et al., 1999; Kolterman et al., 2005). The putatively shorter half-life of Ex9-39, compared to both liraglutide and semaglutide (see above), could provide an explanation for the lack of effectiveness of Ex9-39 in blocking the effects of GLP-1 analogs in this study. The lack of inhibitory effect of Ex9-39 could also be due to insufficient blockade of central GLP-1Rs, which are critical in mediating reduced EtOH intake (Sirohi et al., 2016). Because Ex9-39 was administered intraperitoneally, it is possible that Ex9-39 had limited access to the brain leading to insufficient inhibition of central GLP-1Rs. A previous study showed that Ex9-39 administration increased EtOH intake (Shirazi et al., 2013). Here, we found that Ex9-39, administered alone, did not affect EtOH intake, which may also suggest a lack of relative effectiveness of the drug, at least in the context of our experiments. The discrepancy in the effects of Ex9-39 in Shirazi et al. (2013) and our study could also be due to differences in experimental designs. In our study, each rat was its own control (within-subject design); therefore, the EtOH/water intake over the 24-h period after injection was directly compared to each subject’s basal intake. In contrast, the between-subject comparisons performed by Shirazi et al. (2013) compared EtOH/water intake from two different groups of animals. The within-subject design of this study allowed us to control the potential confounding factors and account for individual differences in baseline EtOH consumption. Additional studies are needed to further investigate the effects of systemic GLP-1R antagonism and its effectiveness in blocking central GLP-1R-mediated effects on alcohol consummatory behaviors.
Several studies have started to unravel the putative neuronal networks and cellular pathways underlying the beneficial effects of GLP-1 analogs in reducing alcohol intake. Initially, GLP-1 analogs were thought to cross the blood brain barrier (BBB), but increasing evidence suggest that this may not be the case, and instead, they may reach the CNS via the circumventricular organs (CVOs), characterized by loose and permeable BBB (Kastin and Akerstrom, 2003; Hunter and Hölscher, 2012; Secher et al., 2014; Ast et al., 2020; Gabery et al., 2020). The effects of GLP-1 analogs on alcohol-related behaviors have been attributed to the activation of central, not peripheral, GLP-1Rs (Sirohi et al., 2016). GLP-1Rs are expressed in brain areas involved in the development and maintenance of AUD, such as NAc, VTA, amygdala, and paraventricular nucleus of the hypothalamus (PVN) (Cork et al., 2015; Graham et al., 2020). Activation of GLP-1Rs has been shown to modulate the increased activity of the mesolimbic dopamine system induced by alcohol (Egecioglu et al., 2013b; Vallöf et al., 2016, 2019a). Peripheral administration of liraglutide prevents alcohol-induced increase in dopamine release in the NAc in rats (Vallöf et al., 2016), while microinjection of exendin-4 into the NAc shell was shown to reduce EtOH intake and block EtOH-induced increase in locomotor activity (Vallöf et al., 2019b). These findings suggest that the effects of GLP-1 analogs on alcohol consumption may result from decreased alcohol-induced dopamine release in the NAc, which in turn, attenuates the rewarding properties of alcohol.
Recent studies have highlighted the importance of NTS GLP-1Rs in mediating the effects of GLP-1 analogs. Selective knockdown of NTS GLP-1Rs attenuates liraglutide-induced decreases in food intake and body weight (Fortin et al., 2020). Moreover, local activation of NTS GLP-1Rs decreases EtOH intake and prevents EtOH-induced accumbal dopamine release (Vallöf et al., 2019a). Located in the brainstem, the NTS is a key regulator of the gut-brain axis, the immune system, and the integration of autonomic functions (Andresen and Kunze, 1994; Marty et al., 2008; Zoccal et al., 2014). The NTS is the primary source of GLP-1-producing neurons, also known as preproglucagon (PPG) neurons, in the CNS, which are believed to play a critical role in addictive behaviors, as they project to and receive inputs from several regions involved in reward processing and consummatory behaviors (Larsen et al., 1997; Merchenthaler et al., 1999; Llewellyn-Smith et al., 2011; Holt et al., 2019). Selective activation of NTS PPG neurons, using chemogenetic techniques, has been shown to mimic the suppressing effects of GLP-1 on food consumption (Gaykema et al., 2017; Holt et al., 2019). Additional optogenetic studies found that the NTS-to-PVN GLP-1 neural pathway is critical for anorectic properties of PPG neurons (Liu et al., 2017). Altogether, these findings suggest that the effects of GLP-1 analogs on alcohol intake may be mediated by NTS GLP-1R-expressing neurons, while their effects on food intake may be mediated by NTS PPG neurons, which could also explain the transient vs. long-lasting effects of liraglutide and semaglutide on EtOH intake vs. body weight, respectively.
Due to the difficulties in developing orally bioavailable non-peptide agonists at GLP-1Rs, and class B GPCRs of the secretin family in general, numerous studies have focused their interest on identifying novel class A rhodopsin-like GPCRs with similar pancreatic β-cells expression and G-protein coupling to GLP-1R. From this effort, GPR119 emerged as a promising therapeutic target for the development of orally active, non-peptide, small-molecule agonists to treat type 2 diabetes and obesity (Koole et al., 2010; Yoshida et al., 2010; Semple et al., 2011, 2012; Melancon et al., 2012; Hothersall et al., 2015; Spasov et al., 2017; Fang et al., 2020a). Administration of GPR119 agonists has been shown to increase plasma GLP-1 and insulin, resulting in improved glucose homeostasis in rodents and humans (Chu et al., 2008; Hansen et al., 2011; Katz et al., 2011; Mandøe et al., 2015; Han et al., 2018; Matsumoto et al., 2018). Here, we tested two different synthetic GPR119 agonists. In contrast to GLP-1 analogs, GPR119 agonists failed to reduce EtOH intake and body weight. Several explanations could account for the lack of effectiveness of GPR199 agonists in the present study. Since we did not measure blood GLP-1 levels, it is possible that the injections of GPR119 agonists did not lead to physiologically relevant increases in blood GLP-1. Because of the relatively short half-lives of AR231453 and APD668, estimated at around 1 h after oral and intravenous administration, respectively, it is also possible that GPR119 agonists may not be able to produce sustained increases in endogenous GLP-1 levels necessary to mimic the effects of GLP-1 analogs (Chu et al., 2008; Semple et al., 2008, 2011). In support of this hypothesis, studies have demonstrated that co-administration of GPR119 agonists with a DPP-4 inhibitor, to prevent rapid degradation of GLP-1, allows for a greater and sustained increase in blood GLP-1 and enhanced effects on glucose homeostasis (Chu et al., 2008; Bahirat et al., 2017; Park et al., 2017). Although we showed that inhibition of DPP-4 with sitagliptin did not affect EtOH intake, co-injection of DPP-4 with GPR119 agonists could be more effective in increasing endogenous GLP-1 levels (Chu et al., 2008) and potentially influencing EtOH drinking. Future studies will be needed to investigate the effects of newly developed compounds with both potent DPP-4 inhibition and GPR119 agonistic activity (Fang et al., 2020b; Li et al., 2020). It is also possible that exposure to alcohol via the IA2BC paradigm may damage GLP-1 producing intestinal L-cells. Alcohol administration was shown to induce intestinal hyperpermeability and endotoxemia (Keshavarzian et al., 2009), which could result in deficient release of endogenous GLP-1 and subsequently blunt the potential effects of endogenous GLP-1 stimulators on alcohol-related outcomes.
In summary, findings of the present study suggest that, among different classes of drugs with modulatory effects on the GLP-1 system, GLP-1 analogs hold the strongest promise to be effective in reducing alcohol consumption, and semaglutide may overall be a better drug candidate than liraglutide in this regard. Preclinical studies are still needed to further characterize the cellular mechanisms and neuronal circuitry which mediate the suppressant effects of GLP-1 analogs on alcohol intake. It is also important to note that chronic exposure to alcohol and alcohol dependence lead to dysregulation of several neural circuits, such as the mesolimbic dopamine and the corticotropin releasing factor systems (Diana et al., 1993; Weiss et al., 1996; Volkow et al., 2007; Roberto et al., 2010; Marty and Spigelman, 2012; Liang et al., 2014a, b; Herman et al., 2016; Marty et al., 2020), which could alter the beneficial effects of GLP-1 analogs observed in non-dependent subjects – an important question that should be explored in future studies. Overall, the present study adds to the growing literature suggesting that the GLP-1 receptor could be a novel and promising pharmacotherapeutic target for AUD. If the results are confirmed in proof-of-concept human studies, repurposing of the readily available FDA-approved medications acting on the GLP-1 system would allow for a quick and efficient medication development process to add new options to the armamentarium of pharmacotherapies for AUD.
The original contributions presented in the study are included in the article/Supplementary Material, further inquiries can be directed to the corresponding author/s.
The animal study was reviewed and approved by the University of California, Los Angeles, Animal Research Committee.
VM, MF, LL, and IS designed the experiments. VM, JM, and YM conducted the experiments and analyzed the data. VM prepared and wrote the manuscript. MF, LL, and IS prepared and edited the manuscript. LL and IS supervised the project. All authors contributed to the article and approved the submitted version.
This work was supported by NIH grants AA024527 (IS), T32DA024635 (JM), NIH intramural funding ZIA-DA000635 and ZIA-AA000218 (Clinical Psychoneuroendocrinology and Neuropsychopharmacology Section, LL) jointly supported by NIDA and NIAAA, a fellowship from the Center on Compulsive Behaviors funded by the NIH Deputy Director for Intramural Research Innovation Award (MF), and a Pathways Research Award® funded by the Alkermes Pathways Research Awards Program® (MF). The funding organizations did not have any role in the study design, execution, or interpretation of the results. The content of this article is solely the responsibility of the authors and does not necessarily represent the official views of the National Institutes of Health.
The authors declare that the research was conducted in the absence of any commercial or financial relationships that could be construed as a potential conflict of interest.
We would like to thank Andrew Liao, Riley Vo, Inga Yenokian, Sonia Lele, Nicole Gamboa, Tara Foroohar, Diba Nayeri, Rhea Plawat, Karla Rodriguez, and Michel Horvat for their help in collecting and analyzing the data.
The Supplementary Material for this article can be found online at: https://www.frontiersin.org/articles/10.3389/fnins.2020.599646/full#supplementary-material
Agersø, H., Jensen, L. B., Elbrønd, B., Rolan, P., and Zdravkovic, M. (2002). The pharmacokinetics, pharmacodynamics, safety and tolerability of NN2211, a new long-acting GLP-1 derivative, in healthy men. Diabetologia 45, 195–202. doi: 10.1007/s00125-001-0719-z
Alvarez, E., Roncero, I., Chowen, J. A., Thorens, B., and Blázquez, E. (1996). Expression of the glucagon-like peptide-1 receptor gene in rat brain. J. Neurochem. 66, 920–927. doi: 10.1046/j.1471-4159.1996.66030920.x
Andresen, M. C., and Kunze, D. L. (1994). Nucleus tractus solitarius–gateway to neural circulatory control. Annu. Rev. Physiol. 56, 93–116. doi: 10.1146/annurev.ph.56.030194.000521
Ast, J., Arvaniti, A., Fine, N. H. F., Nasteska, D., Ashford, F. B., Stamataki, Z., et al. (2020). Super-resolution microscopy compatible fluorescent probes reveal endogenous glucagon-like peptide-1 receptor distribution and dynamics. Nat. Commun. 11:467. doi: 10.1038/s41467-020-14309-w
Bahirat, U. A., Shenoy, R. R., Goel, R. N., and Nemmani, K. V. (2017). APD668, a G protein-coupled receptor 119 agonist improves fat tolerance and attenuates fatty liver in high-trans fat diet induced steatohepatitis model in C57BL/6 mice. Eur. J. Pharmacol. 801, 35–45. doi: 10.1016/j.ejphar.2017.02.043
Bornebusch, A. B., Fink-Jensen, A., Wörtwein, G., Seeley, R. J., and Thomsen, M. (2019). Glucagon-like peptide-1 receptor agonist treatment does not reduce abuse-related effects of opioid drugs. eNeuro 6:ENEURO.0443-18.2019.
Buse, J. B., Rosenstock, J., Sesti, G., Schmidt, W. E., Montanya, E., Brett, J. H., et al. (2009). Liraglutide once a day versus exenatide twice a day for type 2 diabetes: a 26-week randomised, parallel-group, multinational, open-label trial (LEAD-6). Lancet 374, 39–47. doi: 10.1016/S0140-6736(09)60659-0
Carnicella, S., Ron, D., and Barak, S. (2014). Intermittent ethanol access schedule in rats as a preclinical model of alcohol abuse. Alcohol 48, 243–252. doi: 10.1016/j.alcohol.2014.01.006
Chepurny, O. G., Bertinetti, D., Diskar, M., Leech, C. A., Afshari, P., Tsalkova, T., et al. (2013). Stimulation of proglucagon gene expression by human GPR119 in enteroendocrine L-cell line GLUTag. Mol. Endocrinol. 27, 1267–1282. doi: 10.1210/me.2013-1029
Chu, Z. L., Carroll, C., Alfonso, J., Gutierrez, V., He, H., Lucman, A., et al. (2008). A role for intestinal endocrine cell-expressed g protein-coupled receptor 119 in glycemic control by enhancing glucagon-like Peptide-1 and glucose-dependent insulinotropic Peptide release. Endocrinology 149, 2038–2047. doi: 10.1210/en.2007-0966
Chu, Z. L., Jones, R. M., He, H., Carroll, C., Gutierrez, V., Lucman, A., et al. (2007). A role for beta-cell-expressed G protein-coupled receptor 119 in glycemic control by enhancing glucose-dependent insulin release. Endocrinology 148, 2601–2609. doi: 10.1210/en.2006-1608
Cippitelli, A., Damadzic, R., Singley, E., Thorsell, A., Ciccocioppo, R., Eskay, R. L., et al. (2012). Pharmacological blockade of corticotropin-releasing hormone receptor 1 (CRH1R) reduces voluntary consumption of high alcohol concentrations in non-dependent Wistar rats. Pharmacol. Biochem. Behav. 100, 522–529. doi: 10.1016/j.pbb.2011.10.016
Cork, S. C., Richards, J. E., Holt, M. K., Gribble, F. M., Reimann, F., and Trapp, S. (2015). Distribution and characterisation of Glucagon-like peptide-1 receptor expressing cells in the mouse brain. Mol. Metab. 4, 718–731. doi: 10.1016/j.molmet.2015.07.008
Deacon, C. F., Johnsen, A. H., and Holst, J. J. (1995a). Degradation of glucagon-like peptide-1 by human plasma in vitro yields an N-terminally truncated peptide that is a major endogenous metabolite in vivo. J. Clin. Endocrinol. Metab. 80, 952–957. doi: 10.1210/jcem.80.3.7883856
Deacon, C. F., Nauck, M. A., Toft-Nielsen, M., Pridal, L., Willms, B., and Holst, J. J. (1995b). Both subcutaneously and intravenously administered glucagon-like peptide I are rapidly degraded from the NH2-terminus in type II diabetic patients and in healthy subjects. Diabetes 44, 1126–1131. doi: 10.2337/diab.44.9.1126
Diana, M., Pistis, M., Carboni, S., Gessa, G. L., and Rossetti, Z. L. (1993). Profound decrement of mesolimbic dopaminergic neuronal activity during ethanol withdrawal syndrome in rats: electrophysiological and biochemical evidence. Proc. Natl. Acad. Sci. U.S.A. 90, 7966–7969. doi: 10.1073/pnas.90.17.7966
Edwards, C. M., Todd, J. F., Mahmoudi, M., Wang, Z., Wang, R. M., Ghatei, M. A., et al. (1999). Glucagon-like peptide 1 has a physiological role in the control of postprandial glucose in humans: studies with the antagonist exendin 9-39. Diabetes 48, 86–93. doi: 10.2337/diabetes.48.1.86
Egecioglu, E., Engel, J. A., and Jerlhag, E. (2013a). The glucagon-like peptide 1 analogue, exendin-4, attenuates the rewarding properties of psychostimulant drugs in mice. PLoS One 8:e69010. doi: 10.1371/journal.pone.0069010
Egecioglu, E., Steensland, P., Fredriksson, I., Feltmann, K., Engel, J. A., and Jerlhag, E. (2013b). The glucagon-like peptide 1 analogue Exendin-4 attenuates alcohol mediated behaviors in rodents. Psychoneuroendocrinology 38, 1259–1270. doi: 10.1016/j.psyneuen.2012.11.009
Eng, C., Kramer, C. K., Zinman, B., and Retnakaran, R. (2014). Glucagon-like peptide-1 receptor agonist and basal insulin combination treatment for the management of type 2 diabetes: a systematic review and meta-analysis. Lancet 384, 2228–2234. doi: 10.1016/S0140-6736(14)61335-0
Estall, J. L., and Drucker, D. J. (2006). Glucagon and glucagon-like peptide receptors as drug targets. Curr. Pharm. Des. 12, 1731–1750. doi: 10.2174/138161206776873671
Fang, Y., Zhang, S., Li, M., Xiong, L., Tu, L., Xie, S., et al. (2020a). Optimisation of novel 4, 8-disubstituted dihydropyrimido[5,4-b][1,4]oxazine derivatives as potent GPR119 agonists. J. Enzyme Inhib. Med. Chem. 35, 50–58. doi: 10.1080/14756366.2019.1681988
Fang, Y., Zhang, S., Wu, W., Liu, Y., Yang, J., Li, Y., et al. (2020b). Design and synthesis of tetrahydropyridopyrimidine derivatives as dual GPR119 and DPP-4 modulators. Bioorg. Chem. 94, 103390. doi: 10.1016/j.bioorg.2019.103390
Farokhnia, M., Browning, B. D., and Leggio, L. (2019a). Prospects for pharmacotherapies to treat alcohol use disorder: an update on recent human studies. Curr. Opin. Psychiatry 32, 255–265. doi: 10.1097/YCO.0000000000000519
Farokhnia, M., Faulkner, M. L., Piacentino, D., Lee, M. R., and Leggio, L. (2019b). Ghrelin: from a gut hormone to a potential therapeutic target for alcohol use disorder. Physiol. Behav. 204, 49–57. doi: 10.1016/j.physbeh.2019.02.008
Flint, A., Raben, A., Ersbøll, A. K., Holst, J. J., and Astrup, A. (2001). The effect of physiological levels of glucagon-like peptide-1 on appetite, gastric emptying, energy and substrate metabolism in obesity. Int. J. Obes. Relat. Metab. Disord. 25, 781–792. doi: 10.1038/sj.ijo.0801627
Fortin, S. M., Lipsky, R. K., Lhamo, R., Chen, J., Kim, E., Borner, T., et al. (2020). GABA neurons in the nucleus tractus solitarius express GLP-1 receptors and mediate anorectic effects of liraglutide in rats. Sci. Transl. Med. 12:eaay8071. doi: 10.1126/scitranslmed.aay8071
Fortin, S. M., and Roitman, M. F. (2017). Central GLP-1 receptor activation modulates cocaine-evoked phasic dopamine signaling in the nucleus accumbens core. Physiol. Behav. 176, 17–25. doi: 10.1016/j.physbeh.2017.03.019
Fredriksson, R., Höglund, P. J., Gloriam, D. E., Lagerström, M. C., and Schiöth, H. B. (2003). Seven evolutionarily conserved human rhodopsin G protein-coupled receptors lacking close relatives. FEBS Lett. 554, 381–388. doi: 10.1016/s0014-5793(03)01196-7
Fu, R., Mei, Q., Shiwalkar, N., Zuo, W., Zhang, H., Gregor, D., et al. (2020). Anxiety during alcohol withdrawal involves 5-HT2C receptors and M-channels in the lateral habenula. Neuropharmacology 163, 107863. doi: 10.1016/j.neuropharm.2019.107863
Gabery, S., Salinas, C. G., Paulsen, S. J., Ahnfelt-Rønne, J., Alanentalo, T., Baquero, A. F., et al. (2020). Semaglutide lowers body weight in rodents via distributed neural pathways. JCI Insight 5:e133429. doi: 10.1172/jci.insight.133429
Gaykema, R. P., Newmyer, B. A., Ottolini, M., Raje, V., Warthen, D. M., Lambeth, P. S., et al. (2017). Activation of murine pre-proglucagon-producing neurons reduces food intake and body weight. J. Clin. Invest. 127, 1031–1045. doi: 10.1172/JCI81335
Ghosal, S., Packard, A. E. B., Mahbod, P., Mcklveen, J. M., Seeley, R. J., Myers, B., et al. (2017). Disruption of glucagon-like peptide 1 signaling in sim1 neurons reduces physiological and behavioral reactivity to acute and chronic stress. J. Neurosci. 37, 184–193. doi: 10.1523/JNEUROSCI.1104-16.2016
Göke, R., Fehmann, H. C., Linn, T., Schmidt, H., Krause, M., Eng, J., et al. (1993). Exendin-4 is a high potency agonist and truncated exendin-(9-39)-amide an antagonist at the glucagon-like peptide 1-(7-36)-amide receptor of insulin-secreting beta-cells. J. Biol. Chem. 268, 19650–19655.
Göke, R., Larsen, P. J., Mikkelsen, J. D., and Sheikh, S. P. (1995). Distribution of GLP-1 binding sites in the rat brain: evidence that exendin-4 is a ligand of brain GLP-1 binding sites. Eur. J. Neurosci. 7, 2294–2300. doi: 10.1111/j.1460-9568.1995.tb00650.x
Graham, D. L., Durai, H. H., Trammell, T. S., Noble, B. L., Mortlock, D. P., Galli, A., et al. (2020). A novel mouse model of glucagon-like peptide-1 receptor expression: a look at the brain. J. Comp. Neurol. 528, 2445–2470. doi: 10.1002/cne.24905
Graham, D. L., Erreger, K., Galli, A., and Stanwood, G. D. (2013). GLP-1 analog attenuates cocaine reward. Mol. Psychiatry 18, 961–962. doi: 10.1038/mp.2012.141
Hall, S., Isaacs, D., and Clements, J. N. (2018). Pharmacokinetics and clinical implications of semaglutide: a new glucagon-like peptide (GLP)-1 receptor agonist. Clin. Pharmacokinet. 57, 1529–1538. doi: 10.1007/s40262-018-0668-z
Han, T., Lee, B. M., Park, Y. H., Lee, D. H., Choi, H. H., Lee, T., et al. (2018). YH18968, a Novel 1,2,4-triazolone G-protein coupled receptor 119 agonist for the treatment of type 2 diabetes mellitus. Biomol. Ther. 26, 201–209. doi: 10.4062/biomolther.2018.011
Hansen, K. B., Rosenkilde, M. M., Knop, F. K., Wellner, N., Diep, T. A., Rehfeld, J. F., et al. (2011). 2-Oleoyl glycerol is a GPR119 agonist and signals GLP-1 release in humans. J. Clin. Endocrinol. Metab. 96, E1409–E1417. doi: 10.1210/jc.2011-0647
Harasta, A. E., Power, J. M., Von Jonquieres, G., Karl, T., Drucker, D. J., Housley, G. D., et al. (2015). Septal glucagon-like peptide 1 receptor expression determines suppression of cocaine-induced behavior. Neuropsychopharmacology 40, 1969–1978. doi: 10.1038/npp.2015.47
Hassing, H. A., Engelstoft, M. S., Sichlau, R. M., Madsen, A. N., Rehfeld, J. F., Pedersen, J., et al. (2016). Oral 2-oleyl glyceryl ether improves glucose tolerance in mice through the GPR119 receptor. Biofactors 42, 665–673. doi: 10.1002/biof.1303
Hayes, M. R., De Jonghe, B. C., and Kanoski, S. E. (2010). Role of the glucagon-like-peptide-1 receptor in the control of energy balance. Physiol. Behav. 100, 503–510. doi: 10.1016/j.physbeh.2010.02.029
Herman, M. A., Contet, C., and Roberto, M. (2016). A functional switch in tonic GABA currents alters the output of central amygdala corticotropin releasing factor receptor-1 neurons following chronic ethanol exposure. J. Neurosci. 36, 10729–10741. doi: 10.1523/JNEUROSCI.1267-16.2016
Holt, M. K., Richards, J. E., Cook, D. R., Brierley, D. I., Williams, D. L., Reimann, F., et al. (2019). Preproglucagon neurons in the nucleus of the solitary tract are the main source of brain GLP-1, mediate stress-induced hypophagia, and limit unusually large intakes of food. Diabetes 68, 21–33. doi: 10.2337/db18-0729
Hopf, F. W. (2020). Recent perspectives on orexin/hypocretin promotion of addiction-related behaviors. Neuropharmacology 168:108013. doi: 10.1016/j.neuropharm.2020.108013
Hothersall, J. D., Bussey, C. E., Brown, A. J., Scott, J. S., Dale, I., and Rawlins, P. (2015). Sustained wash-resistant receptor activation responses of GPR119 agonists. Eur. J. Pharmacol. 762, 430–442. doi: 10.1016/j.ejphar.2015.06.031
Hunter, K., and Hölscher, C. (2012). Drugs developed to treat diabetes, liraglutide and lixisenatide, cross the blood brain barrier and enhance neurogenesis. BMC Neurosci. 13:33. doi: 10.1186/1471-2202-13-33
Jerlhag, E. (2018). GLP-1 signaling and alcohol-mediated behaviors; preclinical and clinical evidence. Neuropharmacology 136, 343–349. doi: 10.1016/j.neuropharm.2018.01.013
Kang, S., Li, J., Zuo, W., Chen, P., Gregor, D., Fu, R., et al. (2019). Downregulation of M-channels in the lateral habenula mediates hyperalgesia during alcohol withdrawal in rats. Sci. Rep. 9:2714. doi: 10.1038/s41598-018-38393-7
Kanoski, S. E., Fortin, S. M., Arnold, M., Grill, H. J., and Hayes, M. R. (2011). Peripheral and central GLP-1 receptor populations mediate the anorectic effects of peripherally administered GLP-1 receptor agonists, liraglutide and exendin-4. Endocrinology 152, 3103–3112. doi: 10.1210/en.2011-0174
Kanoski, S. E., Rupprecht, L. E., Fortin, S. M., De Jonghe, B. C., and Hayes, M. R. (2012). The role of nausea in food intake and body weight suppression by peripheral GLP-1 receptor agonists, exendin-4 and liraglutide. Neuropharmacology 62, 1916–1927. doi: 10.1016/j.neuropharm.2011.12.022
Kapitza, C., Nosek, L., Jensen, L., Hartvig, H., Jensen, C. B., and Flint, A. (2015). Semaglutide, a once-weekly human GLP-1 analog, does not reduce the bioavailability of the combined oral contraceptive, ethinylestradiol/levonorgestrel. J. Clin. Pharmacol. 55, 497–504. doi: 10.1002/jcph.443
Kastin, A. J., and Akerstrom, V. (2003). Entry of exendin-4 into brain is rapid but may be limited at high doses. Int. J. Obes. Relat. Metab. Disord. 27, 313–318. doi: 10.1038/sj.ijo.0802206
Katz, L. B., Gambale, J. J., Rothenberg, P. L., Vanapalli, S. R., Vaccaro, N., Xi, L., et al. (2011). Pharmacokinetics, pharmacodynamics, safety, and tolerability of JNJ-38431055, a novel GPR119 receptor agonist and potential antidiabetes agent, in healthy male subjects. Clin. Pharmacol. Ther. 90, 685–692. doi: 10.1038/clpt.2011.169
Keshavarzian, A., Farhadi, A., Forsyth, C. B., Rangan, J., Jakate, S., Shaikh, M., et al. (2009). Evidence that chronic alcohol exposure promotes intestinal oxidative stress, intestinal hyperpermeability and endotoxemia prior to development of alcoholic steatohepatitis in rats. J. Hepatol. 50, 538–547. doi: 10.1016/j.jhep.2008.10.028
Kinzig, K. P., D’alessio, D. A., Herman, J. P., Sakai, R. R., Vahl, T. P., Figueiredo, H. F., et al. (2003). CNS glucagon-like peptide-1 receptors mediate endocrine and anxiety responses to interoceptive and psychogenic stressors. J. Neurosci. 23, 6163–6170. doi: 10.1523/JNEUROSCI.23-15-06163.2003
Klein, J. W. (2016). Pharmacotherapy for substance use disorders. Med. Clin. North Am. 100, 891–910. doi: 10.1016/j.mcna.2016.03.011
Knudsen, L. B., and Lau, J. (2019). The discovery and development of liraglutide and semaglutide. Front. Endocrinol. 10:155. doi: 10.3389/fendo.2019.00155
Kolterman, O. G., Kim, D. D., Shen, L., Ruggles, J. A., Nielsen, L. L., Fineman, M. S., et al. (2005). Pharmacokinetics, pharmacodynamics, and safety of exenatide in patients with type 2 diabetes mellitus. Am. J. Health Syst. Pharm. 62, 173–181. doi: 10.1093/ajhp/62.2.173
Koob, G. F. (2015). The dark side of emotion: the addiction perspective. Eur. J. Pharmacol. 753, 73–87. doi: 10.1016/j.ejphar.2014.11.044
Koole, C., Wootten, D., Simms, J., Valant, C., Sridhar, R., Woodman, O. L., et al. (2010). Allosteric ligands of the glucagon-like peptide 1 receptor (GLP-1R) differentially modulate endogenous and exogenous peptide responses in a pathway-selective manner: implications for drug screening. Mol. Pharmacol. 78, 456–465. doi: 10.1124/mol.110.065664
Lan, H., Lin, H. V., Wang, C. F., Wright, M. J., Xu, S., Kang, L., et al. (2012). Agonists at GPR119 mediate secretion of GLP-1 from mouse enteroendocrine cells through glucose-independent pathways. Br. J. Pharmacol. 165, 2799–2807. doi: 10.1111/j.1476-5381.2011.01754.x
Larsen, P. J., Fledelius, C., Knudsen, L. B., and Tang-Christensen, M. (2001). Systemic administration of the long-acting GLP-1 derivative NN2211 induces lasting and reversible weight loss in both normal and obese rats. Diabetes 50, 2530–2539. doi: 10.2337/diabetes.50.11.2530
Larsen, P. J., Tang-Christensen, M., Holst, J. J., and Orskov, C. (1997). Distribution of glucagon-like peptide-1 and other preproglucagon-derived peptides in the rat hypothalamus and brainstem. Neuroscience 77, 257–270. doi: 10.1016/s0306-4522(96)00434-4
Lau, J., Bloch, P., Schäffer, L., Pettersson, I., Spetzler, J., Kofoed, J., et al. (2015). Discovery of the once-weekly glucagon-like peptide-1 (GLP-1) analogue semaglutide. J. Med. Chem. 58, 7370–7380. doi: 10.1021/acs.jmedchem.5b00726
Li, G., Meng, B., Yuan, B., Huan, Y., Zhou, T., Jiang, Q., et al. (2020). The optimization of xanthine derivatives leading to HBK001 hydrochloride as a potent dual ligand targeting DPP-IV and GPR119. Eur. J. Med. Chem. 188, 112017. doi: 10.1016/j.ejmech.2019.112017
Li, J., Chen, P., Han, X., Zuo, W., Mei, Q., Bian, E. Y., et al. (2019). Differences between male and female rats in alcohol drinking, negative affects and neuronal activity after acute and prolonged abstinence. Int. J. Physiol. Pathophysiol. Pharmacol. 11, 163–176.
Liang, J., Lindemeyer, A. K., Suryanarayanan, A., Meyer, E. M., Marty, V. N., Ahmad, S. O., et al. (2014a). Plasticity of GABA(A) receptor-mediated neurotransmission in the nucleus accumbens of alcohol-dependent rats. J. Neurophysiol. 112, 39–50. doi: 10.1152/jn.00565.2013
Liang, J., Marty, V. N., Mulpuri, Y., Olsen, R. W., and Spigelman, I. (2014b). Selective modulation of GABAergic tonic current by dopamine in the nucleus accumbens of alcohol-dependent rats. J. Neurophysiol. 112, 51–60. doi: 10.1152/jn.00564.2013
Liu, J., Conde, K., Zhang, P., Lilascharoen, V., Xu, Z., Lim, B. K., et al. (2017). Enhanced AMPA receptor trafficking mediates the anorexigenic effect of endogenous glucagon-like peptide-1 in the paraventricular hypothalamus. Neuron 96, 897–909.e5. doi: 10.1016/j.neuron.2017.09.042
Llewellyn-Smith, I. J., Reimann, F., Gribble, F. M., and Trapp, S. (2011). Preproglucagon neurons project widely to autonomic control areas in the mouse brain. Neuroscience 180, 111–121. doi: 10.1016/j.neuroscience.2011.02.023
Mandøe, M. J., Hansen, K. B., Hartmann, B., Rehfeld, J. F., Holst, J. J., and Hansen, H. S. (2015). The 2-monoacylglycerol moiety of dietary fat appears to be responsible for the fat-induced release of GLP-1 in humans. Am. J. Clin. Nutr. 102, 548–555. doi: 10.3945/ajcn.115.106799
Marty, V., El Hachmane, M., and Amedee, T. (2008). Dual modulation of synaptic transmission in the nucleus tractus solitarius by prostaglandin E2 synthesized downstream of IL-1beta. Eur J. Neurosci. 27, 3132–3150. doi: 10.1111/j.1460-9568.2008.06296.x
Marty, V. N., Mulpuri, Y., Munier, J. J., and Spigelman, I. (2020). Chronic alcohol disrupts hypothalamic responses to stress by modifying CRF and NMDA receptor function. Neuropharmacology 167:107991. doi: 10.1016/j.neuropharm.2020.107991
Marty, V. N., and Spigelman, I. (2012). Long-lasting alterations in membrane properties, k(+) currents, and glutamatergic synaptic currents of nucleus accumbens medium spiny neurons in a rat model of alcohol dependence. Front. Neurosci. 6:86. doi: 10.3389/fnins.2012.00086
Matsumoto, K., Yoshitomi, T., Ishimoto, Y., Tanaka, N., Takahashi, K., Watanabe, A., et al. (2018). DS-8500a, an orally available g protein-coupled receptor 119 agonist, upregulates glucagon-like peptide-1 and enhances glucose-dependent insulin secretion and improves glucose homeostasis in type 2 diabetic rats. J. Pharmacol. Exp. Ther. 367, 509–517. doi: 10.1124/jpet.118.250019
McKay, N. J., Kanoski, S. E., Hayes, M. R., and Daniels, D. (2011). Glucagon-like peptide-1 receptor agonists suppress water intake independent of effects on food intake. Am. J. Physiol. Regul. Integr. Comp. Physiol. 301, R1755–R1764. doi: 10.1152/ajpregu.00472.2011
Melancon, B. J., Hopkins, C. R., Wood, M. R., Emmitte, K. A., Niswender, C. M., Christopoulos, A., et al. (2012). Allosteric modulation of seven transmembrane spanning receptors: theory, practice, and opportunities for central nervous system drug discovery. J. Med. Chem. 55, 1445–1464. doi: 10.1021/jm201139r
Merchenthaler, I., Lane, M., and Shughrue, P. (1999). Distribution of pre-pro-glucagon and glucagon-like peptide-1 receptor messenger RNAs in the rat central nervous system. J. Comp. Neurol. 403, 261–280.
Meyer, E. M., Long, V., Fanselow, M. S., and Spigelman, I. (2013). Stress increases voluntary alcohol intake, but does not alter established drinking habits in a rat model of posttraumatic stress disorder. Alcohol. Clin. Exp. Res. 37, 566–574. doi: 10.1111/acer.12012
Motulsky, H. J., and Brown, R. E. (2006). Detecting outliers when fitting data with nonlinear regression - a new method based on robust nonlinear regression and the false discovery rate. BMC Bioinformatics 7:123. doi: 10.1186/1471-2105-7-123
Novak, U., Wilks, A., Buell, G., and Mcewen, S. (1987). Identical mRNA for preproglucagon in pancreas and gut. Eur. J. Biochem. 164, 553–558. doi: 10.1111/j.1432-1033.1987.tb11162.x
Orskov, C., Holst, J. J., and Nielsen, O. V. (1988). Effect of truncated glucagon-like peptide-1 [proglucagon-(78-107) amide] on endocrine secretion from pig pancreas, antrum, and nonantral stomach. Endocrinology 123, 2009–2013. doi: 10.1210/endo-123-4-2009
Overton, H. A., Babbs, A. J., Doel, S. M., Fyfe, M. C., Gardner, L. S., Griffin, G., et al. (2006). Deorphanization of a G protein-coupled receptor for oleoylethanolamide and its use in the discovery of small-molecule hypophagic agents. Cell Metab. 3, 167–175. doi: 10.1016/j.cmet.2006.02.004
Park, Y. H., Choi, H. H., Lee, D. H., Chung, S. Y., Yang, N. Y., Kim, D. H., et al. (2017). YH18421, a novel GPR119 agonist exerts sustained glucose lowering and weight loss in diabetic mouse model. Arch. Pharm. Res. 40, 772–782. doi: 10.1007/s12272-017-0925-y
Rehm, J., Mathers, C., Popova, S., Thavorncharoensap, M., Teerawattananon, Y., and Patra, J. (2009). Global burden of disease and injury and economic cost attributable to alcohol use and alcohol-use disorders. Lancet 373, 2223–2233.
Roberto, M., Cruz, M. T., Gilpin, N. W., Sabino, V., Schweitzer, P., Bajo, M., et al. (2010). Corticotropin releasing factor-induced amygdala gamma-aminobutyric Acid release plays a key role in alcohol dependence. Biol. Psychiatry 67, 831–839. doi: 10.1016/j.biopsych.2009.11.007
Sandoval, D. A., Bagnol, D., Woods, S. C., D’alessio, D. A., and Seeley, R. J. (2008). Arcuate glucagon-like peptide 1 receptors regulate glucose homeostasis but not food intake. Diabetes 57, 2046–2054. doi: 10.2337/db07-1824
Schepp, W., Schmidtler, J., Riedel, T., Dehne, K., Schusdziarra, V., Holst, J. J., et al. (1994). Exendin-4 and exendin-(9-39)NH2: agonist and antagonist, respectively, at the rat parietal cell receptor for glucagon-like peptide-1-(7-36)NH2. Eur. J. Pharmacol. 269, 183–191. doi: 10.1016/0922-4106(94)90085-x
Secher, A., Jelsing, J., Baquero, A. F., Hecksher-Sørensen, J., Cowley, M. A., Dalbøge, L. S., et al. (2014). The arcuate nucleus mediates GLP-1 receptor agonist liraglutide-dependent weight loss. J. Clin. Invest. 124, 4473–4488. doi: 10.1172/JCI75276
Semple, G., Fioravanti, B., Pereira, G., Calderon, I., Uy, J., Choi, K., et al. (2008). Discovery of the first potent and orally efficacious agonist of the orphan G-protein coupled receptor 119. J. Med. Chem. 51, 5172–5175. doi: 10.1021/jm8006867
Semple, G., Lehmann, J., Wong, A., Ren, A., Bruce, M., Shin, Y. J., et al. (2012). Discovery of a second generation agonist of the orphan G-protein coupled receptor GPR119 with an improved profile. Bioorg. Med. Chem. Lett. 22, 1750–1755. doi: 10.1016/j.bmcl.2011.12.092
Semple, G., Ren, A., Fioravanti, B., Pereira, G., Calderon, I., Choi, K., et al. (2011). Discovery of fused bicyclic agonists of the orphan G-protein coupled receptor GPR119 with in vivo activity in rodent models of glucose control. Bioorg. Med. Chem. Lett. 21, 3134–3141. doi: 10.1016/j.bmcl.2011.03.007
Shirazi, R. H., Dickson, S. L., and Skibicka, K. P. (2013). Gut peptide GLP-1 and its analogue, Exendin-4, decrease alcohol intake and reward. PLoS One 8:e61965. doi: 10.1371/journal.pone.0061965
Simms, J. A., Steensland, P., Medina, B., Abernathy, K. E., Chandler, L. J., Wise, R., et al. (2008). Intermittent access to 20% ethanol induces high ethanol consumption in Long-Evans and Wistar rats. Alcohol. Clin. Exp. Res. 32, 1816–1823. doi: 10.1111/j.1530-0277.2008.00753.x
Sirohi, S., Schurdak, J. D., Seeley, R. J., Benoit, S. C., and Davis, J. F. (2016). Central & peripheral glucagon-like peptide-1 receptor signaling differentially regulate addictive behaviors. Physiol. Behav. 161, 140–144. doi: 10.1016/j.physbeh.2016.04.013
Soga, T., Ohishi, T., Matsui, T., Saito, T., Matsumoto, M., Takasaki, J., et al. (2005). Lysophosphatidylcholine enhances glucose-dependent insulin secretion via an orphan G-protein-coupled receptor. Biochem. Biophys. Res. Commun. 326, 744–751. doi: 10.1016/j.bbrc.2004.11.120
Sørensen, G., Reddy, I. A., Weikop, P., Graham, D. L., Stanwood, G. D., Wortwein, G., et al. (2015). The glucagon-like peptide 1 (GLP-1) receptor agonist exendin-4 reduces cocaine self-administration in mice. Physiol. Behav. 149, 262–268. doi: 10.1016/j.physbeh.2015.06.013
Spasov, A. A., Kosolapov, V. A., Babkov, D. A., and Maika, O. Y. (2017). Effect of GRP119 receptor agonist, compound MBX-2982, on activity of human glucokinase. Bull. Exp. Biol. Med. 163, 695–698. doi: 10.1007/s10517-017-3881-0
Suchankova, P., Yan, J., Schwandt, M. L., Stangl, B. L., Caparelli, E. C., Momenan, R., et al. (2015). The glucagon-like peptide-1 receptor as a potential treatment target in alcohol use disorder: evidence from human genetic association studies and a mouse model of alcohol dependence. Transl. Psychiatry 5:e583. doi: 10.1038/tp.2015.68
Terauchi, Y., Yamada, Y., Watada, H., Nakatsuka, Y., Shiosakai, K., Washio, T., et al. (2018). Efficacy and safety of the G protein-coupled receptor 119 agonist DS-8500a in Japanese type 2 diabetes mellitus patients with inadequate glycemic control on sitagliptin: a phase 2 randomized placebo-controlled study. J. Diabetes Investig. 9, 1333–1341. doi: 10.1111/jdi.12846
Thorens, B., Porret, A., Bühler, L., Deng, S. P., Morel, P., and Widmann, C. (1993). Cloning and functional expression of the human islet GLP-1 receptor. Demonstration that exendin-4 is an agonist and exendin-(9-39) an antagonist of the receptor. Diabetes 42, 1678–1682. doi: 10.2337/diab.42.11.1678
Thorsell, A., and Mathé, A. A. (2017). Neuropeptide Y in alcohol addiction and affective disorders. Front. Endocrinol. 8:178. doi: 10.3389/fendo.2017.00178
Tuesta, L. M., Chen, Z., Duncan, A., Fowler, C. D., Ishikawa, M., Lee, B. R., et al. (2017). GLP-1 acts on habenular avoidance circuits to control nicotine intake. Nat. Neurosci. 20, 708–716. doi: 10.1038/nn.4540
Vallöf, D., Kalafateli, A. L., and Jerlhag, E. (2019a). Brain region specific glucagon-like peptide-1 receptors regulate alcohol-induced behaviors in rodents. Psychoneuroendocrinology 103, 284–295. doi: 10.1016/j.psyneuen.2019.02.006
Vallöf, D., Kalafateli, A. L., and Jerlhag, E. (2020). Long-term treatment with a glucagon-like peptide-1 receptor agonist reduces ethanol intake in male and female rats. Transl. Psychiatry 10, 238. doi: 10.1038/s41398-020-00923-1
Vallöf, D., Maccioni, P., Colombo, G., Mandrapa, M., Jörnulf, J. W., Egecioglu, E., et al. (2016). The glucagon-like peptide 1 receptor agonist liraglutide attenuates the reinforcing properties of alcohol in rodents. Addict. Biol. 21, 422–437. doi: 10.1111/adb.12295
Vallöf, D., Vestlund, J., and Jerlhag, E. (2019b). Glucagon-like peptide-1 receptors within the nucleus of the solitary tract regulate alcohol-mediated behaviors in rodents. Neuropharmacology 149, 124–132. doi: 10.1016/j.neuropharm.2019.02.020
Volkow, N. D., Wang, G. J., Telang, F., Fowler, J. S., Logan, J., Jayne, M., et al. (2007). Profound decreases in dopamine release in striatum in detoxified alcoholics: possible orbitofrontal involvement. J. Neurosci. 27, 12700–12706. doi: 10.1523/JNEUROSCI.3371-07.2007
von Holstein-Rathlou, S., and Gillum, M. P. (2019). Fibroblast growth factor 21: an endocrine inhibitor of sugar and alcohol appetite. J. Physiol. 597, 3539–3548. doi: 10.1113/JP277117
Wang, Z., Wang, R. M., Owji, A. A., Smith, D. M., Ghatei, M. A., and Bloom, S. R. (1995). Glucagon-like peptide-1 is a physiological incretin in rat. J. Clin. Invest. 95, 417–421. doi: 10.1172/JCI117671
Weiss, F., Parsons, L. H., Schulteis, G., Hyytia, P., Lorang, M. T., Bloom, F. E., et al. (1996). Ethanol self-administration restores withdrawal-associated deficiencies in accumbal dopamine and 5-hydroxytryptamine release in dependent rats. J. Neurosci. 16, 3474–3485. doi: 10.1523/JNEUROSCI.16-10-03474.1996
Wise, R. A. (1975). Maximization of ethanol intake in the rat. Adv. Exp. Med. Biol. 59, 279–294. doi: 10.1007/978-1-4757-0632-1_19
Witkiewitz, K., Litten, R. Z., and Leggio, L. (2019). Advances in the science and treatment of alcohol use disorder. Sci. Adv. 5:eaax4043. doi: 10.1126/sciadv.aax4043
Yang, J. W., Kim, H. S., Choi, Y. W., Kim, Y. M., and Kang, K. W. (2018). Therapeutic application of GPR119 ligands in metabolic disorders. Diabetes Obes. Metab. 20, 257–269. doi: 10.1111/dom.13062
Yoshida, S., Ohishi, T., Matsui, T., Tanaka, H., Oshima, H., Yonetoku, Y., et al. (2010). Novel GPR119 agonist AS1535907 contributes to first-phase insulin secretion in rat perfused pancreas and diabetic db/db mice. Biochem. Biophys. Res. Commun. 402, 280–285. doi: 10.1016/j.bbrc.2010.10.015
Zhang, Y., Kahng, M. W., Elkind, J. A., Weir, V. R., Hernandez, N. S., Stein, L. M., et al. (2020). Activation of GLP-1 receptors attenuates oxycodone taking and seeking without compromising the antinociceptive effects of oxycodone in rats. Neuropsychopharmacology 45, 451–461. doi: 10.1038/s41386-019-0531-4
Keywords: glucagon-like peptide-1, liraglutide, semaglutide, alcohol intake, GPR119, dipeptidyl peptidase-4, rat
Citation: Marty VN, Farokhnia M, Munier JJ, Mulpuri Y, Leggio L and Spigelman I (2020) Long-Acting Glucagon-Like Peptide-1 Receptor Agonists Suppress Voluntary Alcohol Intake in Male Wistar Rats. Front. Neurosci. 14:599646. doi: 10.3389/fnins.2020.599646
Received: 27 August 2020; Accepted: 03 December 2020;
Published: 23 December 2020.
Edited by:
Nigel H. Greig, National Institute on Aging, National Institutes of Health (NIH), United StatesReviewed by:
Subhash C. Pandey, University of Illinois at Chicago, United StatesCopyright © 2020 Marty, Farokhnia, Munier, Mulpuri, Leggio and Spigelman. This is an open-access article distributed under the terms of the Creative Commons Attribution License (CC BY). The use, distribution or reproduction in other forums is permitted, provided the original author(s) and the copyright owner(s) are credited and that the original publication in this journal is cited, in accordance with accepted academic practice. No use, distribution or reproduction is permitted which does not comply with these terms.
*Correspondence: Igor Spigelman, aXNwaWdlbG1hbkBkZW50aXN0cnkudWNsYS5lZHU=; aWdvckB1Y2xhLmVkdQ==
†These authors share first authorship
Disclaimer: All claims expressed in this article are solely those of the authors and do not necessarily represent those of their affiliated organizations, or those of the publisher, the editors and the reviewers. Any product that may be evaluated in this article or claim that may be made by its manufacturer is not guaranteed or endorsed by the publisher.
Research integrity at Frontiers
Learn more about the work of our research integrity team to safeguard the quality of each article we publish.