- 1Department of Trauma and Orthopedics, Peking University People’s Hospital, Peking University, Beijing, China
- 2Key Laboratory of Trauma and Neural Regeneration, Ministry of Education, Peking University, Beijing, China
- 3National Center for Trauma Medicine, Beijing, China
- 4Department of Orthopedics, PLA Strategic Support Force Characteristic Medical Center, Beijing, China
- 5Diabetic Foot Treatment Center, Peking University People’s Hospital, Peking University, Beijing, China
Peripheral nerve injury repair has been considered a difficult problem in the field of trauma for a long time. Conventional surgical methods are not applicable in some special types of nerve injury, prompting scholars to seek to develop more effective nerve translocation repair technologies. The purpose of this study was to explore the functional state of neurons in injured lower limbs after translocation repair, with a view to preliminarily clarify the molecular mechanisms underlying this process. Eighteen Sprague–Dawley rats were divided into the normal, tibial nerve in situ repair, and common peroneal nerve transposition repair tibial nerve groups. Nerve function assessment and immunohistochemical staining of neurofilament 200 (NF-200), protein kinase B (Akt), mammalian target of rapamycin (mTOR), and ribosomal protein S6 kinase (p70S6K) in the dorsal root ganglia were performed at 12 weeks after surgery. Tibial nerve function and neuroelectrophysiological analysis, osmic acid staining, muscle strength testing, and muscle fiber staining showed that the nerve translocation repair could restore the function of the recipient nerve to a certain extent; however, the repair was not as efficient as the in situ repair. Immunohistochemical staining showed that the translocation repair resulted in changes in the microstructure of neuronal cell bodies, and the expressions of Akt, mTOR, and p70S6K in the three dorsal root ganglia groups were significantly different (p < 0.05). This study demonstrates that the nerve translocation repair technology sets up a new reflex loop, with the corresponding neuroskeletal adjustments, in which, donor neurons dominate the recipient nerves. This indicates that nerve translocation repair technology can lead to neuronal remodeling and is important as a supplementary treatment for a peripheral nerve injury. Furthermore, the Akt/mTOR/p70S6K signaling pathway may be involved in the formation of the new neural reflex loop created as a result of the translocation repair.
Introduction
Peripheral nerve injury is a common clinical condition, mainly caused by trauma such as traction, compression, cutting, and ischemia (Huckhagel et al., 2018). Peripheral nerve damage can lead to a sensory and motor dysfunction, ranging from paresthesia to limb paralysis, in the corresponding innervated area. The repair of the damaged nerves in a manner that can restore as much of the effector function as possible has, thus, always been a challenging issue in the field of trauma surgery.
The best repair method for non-defective peripheral nerve injury is tension-free nerve in situ suture. In case of damaged nerves with defects, the gold standard for repair is an autologous nerve transplantation; however, this often leads to the donor zone innervation. As a result, scientists began to search for new nerve injury treatments that could replace the nerve transplantation. Nerve conduit is a recent research feature. The use of different biochemical materials and the selection of different ultrastructures make the nerve conduit more closely fit to the real nerve tissue and promote the regeneration of peripheral nerves. These new types of conduits have achieved good results in laboratory researches (Qian et al., 2018a, b, 2019). However, in the face of nerve injury similar to a nerve root avulsion injury, the nerve conduits still have certain limitations. Consequently, the importance of a nerve transposition repair has been recognized. Zheng et al. (2018) used a healthy C7 brachial plexus nerve to repair a limb hemiplegia caused by cerebral palsy. Kawabata et al. (2001) used the adjacent small nerves to repair the nerve root avulsion, as well as post-ganglion injury near the foramina, resulting in the partial restoration of the injured limb movement. During the post-transposition repair process, the donor nerve dominates the damaged target organ, which means that a new and effective nerve reflex arc is established. The donor neuron is given a new functional position to control a new effector. The re-use of the damaged target organs illustrates that the new reflex arc is regulated by a mechanism that has caused a functional remodeling of the relevant nerve conduction pathway constituent cells of the nerve center, which was the donor neuron.
Using a fluorescent retrograde tracing and an enlarged model of two nerves to repair two nerves, our group previously proved that relative neurons in the spinal cord were reshaped when the effector was changed (Yu et al., 2016). However, the activation state and mechanisms of the signal transduction pathways involved in the central remodeling remained unclear.
Protein kinase B (Akt) signaling involves many pathways implicated in several biological functions, including cell proliferation, migration, and survival, and it is also closely related to tissue regeneration (Chen et al., 2013). Similarly, the mammalian target of rapamycin/ribosomal protein S6 kinase (mTOR/p70S6K) as a downstream effector of Akt activation is also a control center for cell growth and aging, especially involving the activation of the phosphatidylinositol 3-kinase/Akt signaling pathway and mTOR phosphorylation induced by Akt (Fingar and Blenis, 2004; Loewith and Hall, 2011).
This study explored the regulation of Akt/mTOR/p70S6K/toll-like receptor-4 (TLR-4) during an effector-induced neuronal structural and functional remodeling after a nerve transposition and aimed to clarify the possible mechanisms underlying this central nervous system remodeling.
Materials and Methods
Animals
Eighteen female Sprague–Dawley rats, aged 6 weeks and weighing 225 ± 25 g (Beijing Vital River Laboratory Animal Technology Co., Ltd., China), were randomly selected. The experimental animals were caged in a specific-pathogen free area at the Experimental Animal Center of Peking University People’s Hospital at 24 ± 2°C, 50–55% relative humidity, a 12 h light/dark cycle, and with free access to standard pellet feed and clean drinking water. This study was approved by the Ethics Committee of the People’s Hospital of Peking University, China (approval No. 2015-50). All the experimental procedures followed were in accordance with the Laboratory Animal Management Regulations of Peking University People’s Hospital.
Surgery
All animals were randomly divided equally into three groups – the sham operation group (N group, n = 6), in situ repair group (TN-TN group, n = 6), and common peroneal nerve transposition repair tibial nerve group (PN-TN group, n = 6).
The skin of the rats was cut along the long axis of the right hind leg to expose the sciatic nerve and its branches after anesthesia using isoflurane gas (5%, 100 mL/min). For N group animals, the wound layers were closed thereafter. For TN-TN and PN-TN group animals, the tibial nerve and common peroneal nerve were cut off about 5 mm from the sciatic nerve bifurcation. The two stumps of the common peroneal nerve were ligated and sutured to the adjacent muscles in the TN-TN group. The proximal tibial nerve and the distal common peroneal nerve were ligated and sutured to the adjacent muscles in the PN-TN group. A deacetylated chitin tube (inner diameter, 0.8 mm) was used to suture the two stumps of the residual nerve in each group; the gap between the stumps was about 2 mm. Subsequently, the wound layers were sutured after irrigation with normal saline (An et al., 2015).
Tibial Nerve Function Index (TFI)
The rats were passed through an imprinted box which the footprints could be recorded by a camera in the SPF level barrier environment at 12 weeks after the operation, and their gait patterns were recorded using a digital video camera (Canon; Tokyo, Japan). Three variables were measured: print length (PL): the longest distance of a single footprint; toe spread (TS): the distance from the 1st to 5th toe line; and the width of the middle toe (intermediary toe spread, IT): the distance from the 2nd to 4th toe line. The right foot data were used as the experimental (E) data and left foot data as the normal (N) data. The following three factors were then calculated: footprint length factor (PL factor, PLF) = (EPL–NPL)/NPL; footprint width factor (TS factor, TSF) = (ETS–NTS)/NTS; and intermediate toe width factor (IT factor, ITF) = (EIT–NIT)/NIT. The TFI was calculated using the Bain-Mackinnon-Hunter formula as follows: TFI = −37.2 (PLF) + 104.4 (TSF) + 45.6 (ITF)–8.8.
Neuroelectrophysiological Examination
Rats were anesthetized using isoflurane gas (5.0%, 100 mL/min) after gait recording was completed. The skin was cut along the original surgical incision, the sciatic nerve was bluntly separated, and the tibialis anterior and gastrocnemius muscles were exposed. Stimulation electrodes were placed 1.0 cm apart at the distal and proximal ends of the conduit. Induction electrodes were placed on the tibialis anterior muscle. An electrophysiology instrument (Oxford Instruments Inc., Oxford, United Kingdom) was set to a rectangular pulse (duration: 0.1 ms, current: 0.09 mA, frequency: 1 Hz). The differences in t and latency between the adjacent far and near conduction times were recorded and calculated, respectively. Motor nerve conduction velocity was 0.01/t.
Tetanic Muscle Contraction Strength
The gastrocnemius was dissected and isolated after the end of the electrophysiological test. The hind limb was fixed on a specially made holding frame, with the distal end of the gastrocnemius connected to a tension sensor such that the holding frame kept the gastrocnemius and the tension sensor aligned. The initial tension was maintained at a fixed level (0 < F < 0.1 N). An electrophysiological system was then used to generate an initial electric stimulation (intensity: 0.9 mA, wavelength: 0.1 ms, frequency: 1 Hz). The stimulation electric current was subsequently strengthened until the waveform of the tetanic contraction induced stopped increasing. A biomedical signal acquisition and processing system (PCLAB-UE; Beijing Microsignal Star Inc., Beijing, China) was used to record the waveform of the tetanic contraction of the gastrocnemius on both sides. The amplitudes of the waves were measured, and the ratio of the wave amplitude of the experimental side to that of the untreated normal control side was used as the overall recovery rate of muscle strength.
Osmic Acid Staining
After the end of the tetanic muscle contraction strength test, 5 mm of nerve tissue was taken from the distal end of the suture point. The tissue samples were fixed in 4% paraformaldehyde overnight and rinsed for 8 h. Then, the samples were soaked in 1% osmic acid solution overnight in a fume hood. After 12 h of rinsing, the sample was dehydrated using an alcohol gradient. After embedding in transparent paraffin, sample slices (3 μm each) were prepared, dewaxed, subjected to alcohol gradient dehydration, and transparent xylene and neutral gum sealing. The sections were then observed under a microscope (Olympus Corporation, Tokyo, Japan); five 400–fold magnification fields of view were randomly selected for each tissue sample to count the average number of the myelinated nerve fibers using the Image Pro plus 6.0 (Media Cybernetics Inc., Rockville, MD, United States) (An et al., 2015).
Muscle Wet Weight Weighing
The Achilles tendon was cut off to detach the gastrocnemius muscles from the distal and proximal stumps after the nerves were dissected. The muscles were weighed on an electronic balance after the blood had been wiped.
Muscle Hematoxylin and Eosin (HE) Staining
Transverse sectioning of the muscle samples was performed for HE staining after fixing with paraformaldehyde, dehydrating with graded ethanol solutions, and embedding in paraffin wax. The cross-sections of the muscle fibers were photographed under a 400–fold magnification, and five fields were selected in the upper left, lower left, upper right, lower right, and center of the cross-section of the muscle fibers for quantification of the muscular fiber diameters in each field using Image Pro plus 6.0.
Muscle Gomori Staining
The muscle sections were immersed in Gomori staining solution (Beijing Solarbio Science & Technology Co., Ltd., Beijing, China) for 60 min at 37°C in a sealed environment. Then, the sections were washed thrice with running water and thrice with distilled water. After being air-dried, the sections were sealed using glycerin gelatin, and the muscle fibers were subsequently viewed under a microscope.
Dorsal Root Ganglion (DRG) HE Staining
The L5 dorsal root ganglion was excised, and the tissues were fixed, dehydrated, sliced, and dewaxed, as mentioned above. After HE staining, the sections were observed under a microscope.
DRG Immunohistochemical Staining
Primary antibodies against neurofilament 200 (NF-200), Akt, mTOR, p70S6K, and toll like receptor 4 (TLR-4) were purchased from Abcam, Cambridge, the UK (ab82259 for NF-200, ab179463 for Akt, ab32028 for mTOR, ab32529 for p70S6K, ab22048 for TLR-4). The ganglion tissue was fixed, dehydrated, sliced, and dewaxed as described above. Pepsins were used for antigen retrieval. After washing with phosphate-buffered saline, tissues were incubated in 3% hydrogen peroxide solution for 10 min. After washing, the samples were incubated with primary antibody and then with secondary antibody, stained using 3,3′-diaminobenzidine, and observed under the microscope. Samples from the three groups were probed for the same proteins at the same time. Hematoxylin counterstain time was 3 min. Samples were subjected to a gradient alcohol dehydration and transparent xylene and neutral gum sealing; thereafter, sections were observed under a microscope. Five fields of view were randomly selected from each section and the average integrated option density (IOD) of protein positive was measured using the Image Pro plus 6.0.
Statistical Analysis
Statistical analyses were performed using the SPSS 11.0 (SPSS Inc., Chicago, IL, United States). All data are expressed as mean ± standard deviation. Independent t-tests were used for two-group comparisons, such as TFI. When the data met the homogeneity of variance, the one way ANOVA was used for comparing the differences between the groups. The non-parametric test (Kruskal-Wallis k samples) was chosen when the data did not meet the homogeneity of variance which involves the pairwise comparison of every combination of group pairs. Statistical significance was defined as P < 0.05.
Results
General Observations
All rats survived to the end of the experiments with bright hair. No systemic or local infections or other post-operative complications were observed. Rats in the PN-TN and TN-TN groups showed flexion deformities in the right lower limbs and atrophy of the palmar muscles, but no obvious autophagy in the toes. After the exposure to the surgical field, there was no obvious inflammatory response around the conduits. The partially absorbed conduits were found to adhere slightly to the surrounding tissue. The nerves in the conduits were observed to have grown smoothly to the terminal nerves after stripping of the incomplete cannula and local soft tissue carefully. No obvious neurofibroma formation was found at any nerve ligation or suture site (Figures 1, 2).
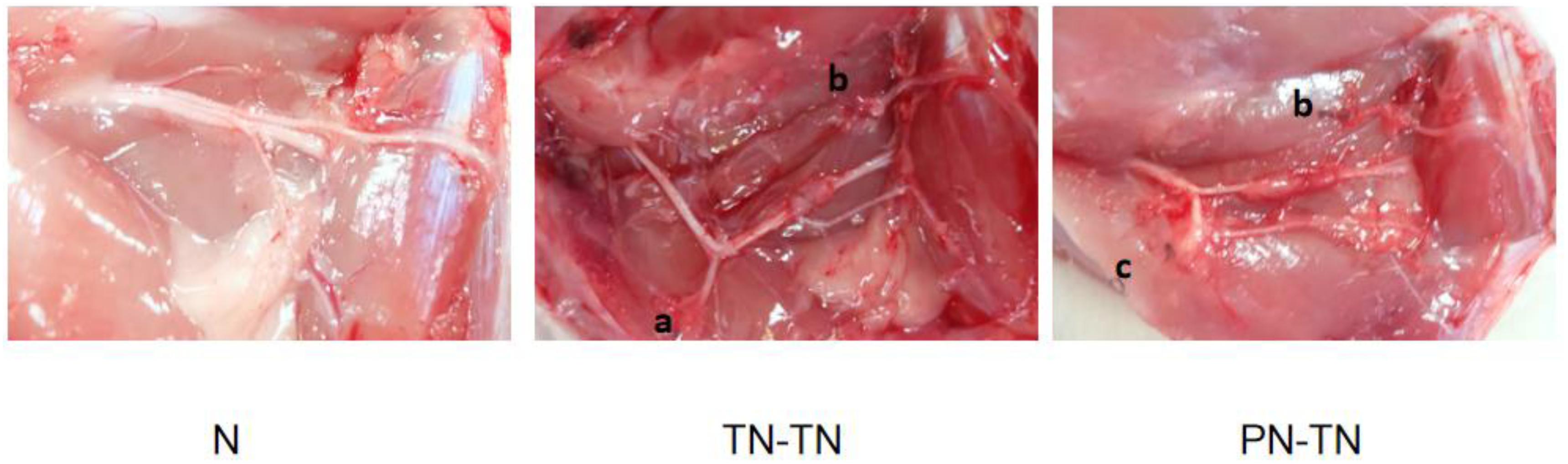
Figure 1. Nerve distribution. (a) Ligature suture point on the proximal stump of the common peroneal nerve; (b) ligature suture point on the distal stump of the common peroneal nerve and (c) ligature suture point on the proximal stump of the tibial nerve.
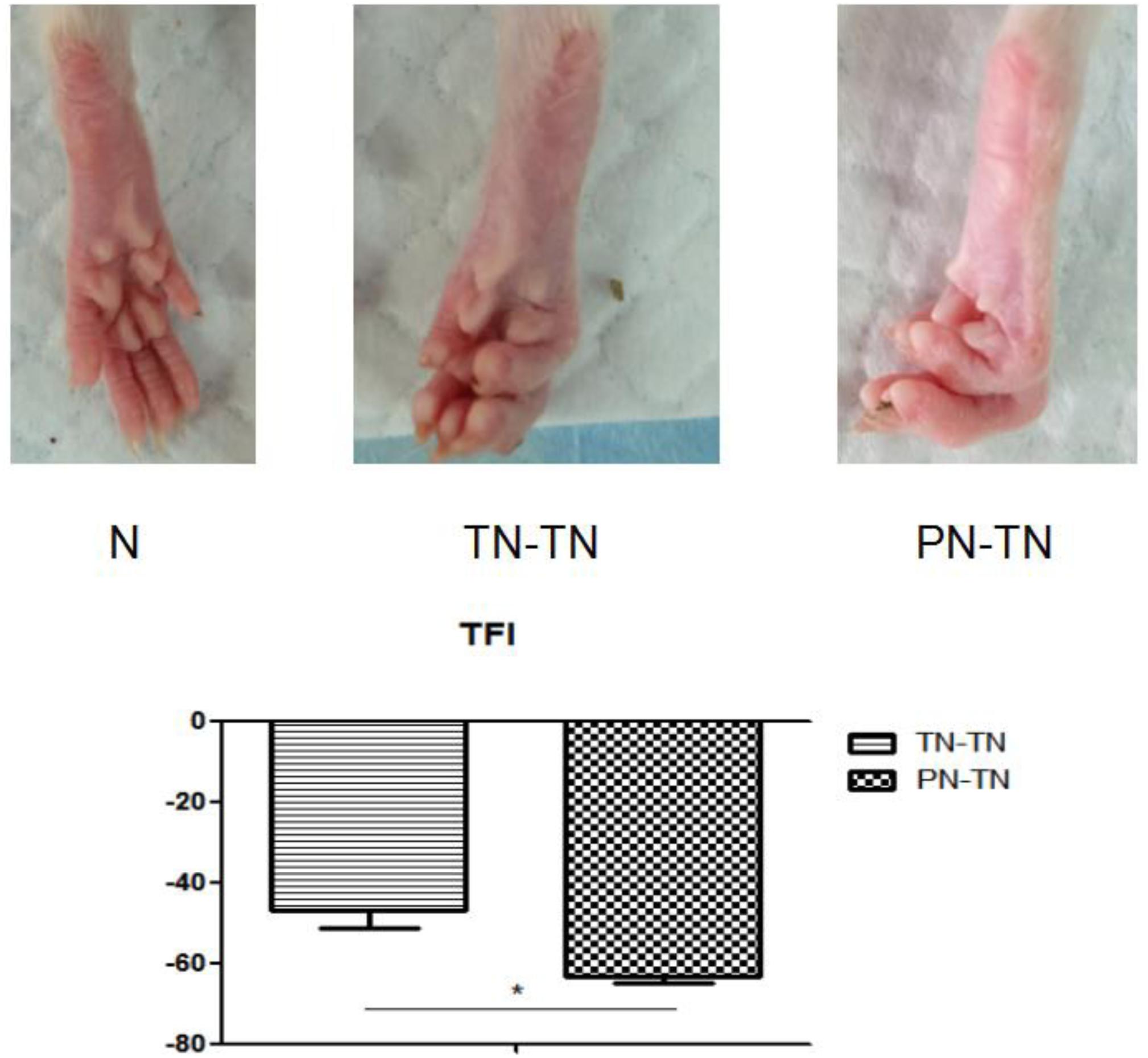
Figure 2. The first row shows the morphology of the right hind paw of a rat from each of the three groups. It can be seen that the toes of the rat in the N group were naturally separated with full plantar muscles. In the TN-TN group, the five-toed curling deformity was mild, and the plantar muscles were slightly atrophied. The five-toe curling deformity was obvious, and the plantar muscles were obviously atrophied in the PN-TN group. The second row shows the TFIs of PT-TN and TN-TN group. *P < 0.05.
TFI
The TFIs of the PN-TN and TN-TN groups were -63.37 ± 1.46 and -47.13 ± 4.07, respectively, and the difference between the two groups was statistically significant (P < 0.001, tested by independent t-tests) (Figure 2).
Quantification of Nerve Fibers and Electrophysiological Examination
The results of the neural acid staining are shown in Figure 3. The number of the myelinated nerve fibers was 3878.43 ± 135.26 in the N group, 3,618.88 ± 257.76 in the TN-TN group, and 3584.48 ± 184.67 in the PN-TN group (Table 1). There was no significant difference in the number of the myelinated nerve fibers between the groups (P > 0.05).
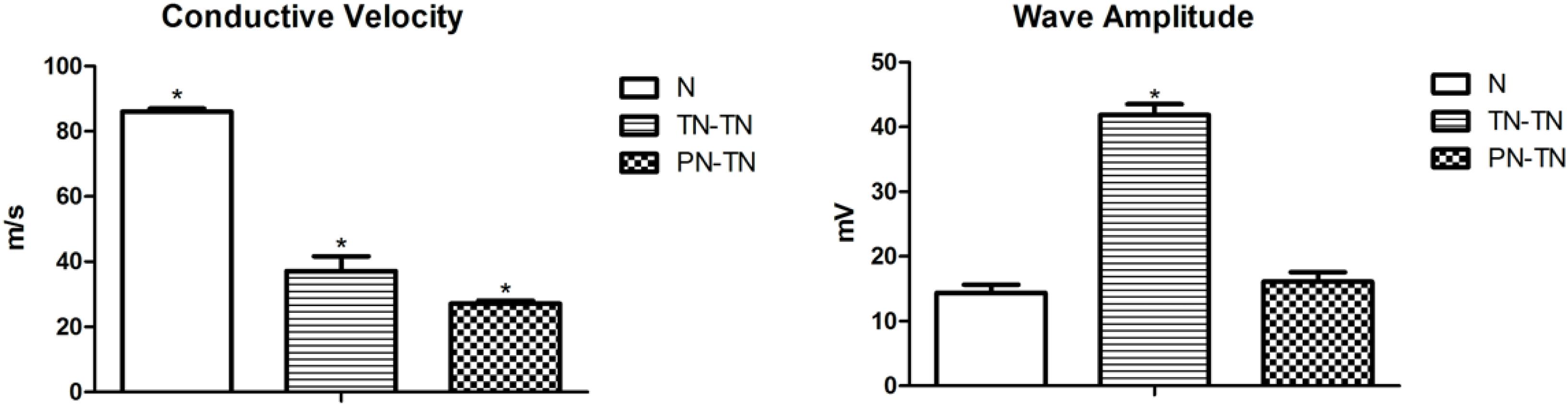
Figure 3. Nerve conduction velocity was significantly different between pairs, with the highest in the N group and the lowest in the PN-TN group. The TN-TN group had the largest amplitude, which was significantly higher than that of the other two groups. *P < 0.05 vs. other groups.
The nerve conduction velocity and compound action potential amplitude data are shown in Table 1. The nerve conduction velocity in the surgical groups was worse than that in the normal group [P = 0.001, tested by the non-parametric test (Kruskal-Wallis k samples)]. The nerve conduction velocity in the TN-TN group was significantly higher than that in the PN-TN group (p < 0.001, tested by one way ANOVA). The composite action potential amplitude of the TN-TN group was significantly higher than those of the other two groups (P < 0.001 vs. N group and P < 0.001 vs. PN-TN group, tested by one way ANOVA); however, there was no significant difference in this regard between the PN-TN group and the N group (P = 0.093, tested by one way ANOVA) (Figure 4).
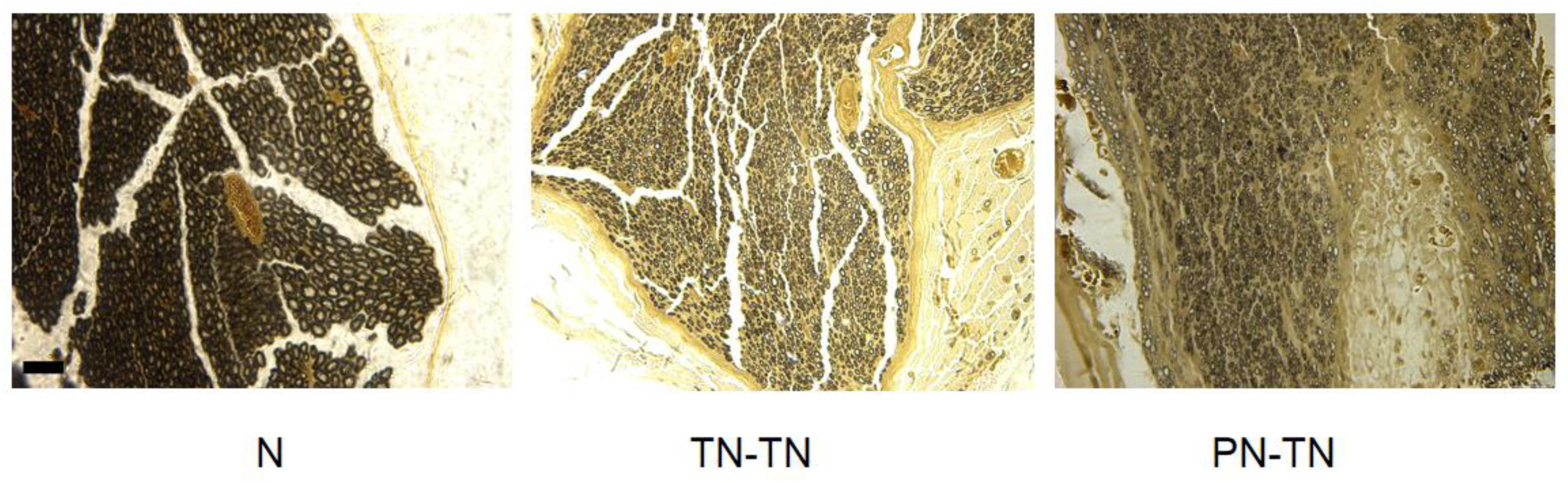
Figure 4. Osmic acid staining. The myelinated nerve fibers in the N group were evenly distributed and the axon area was significantly larger than those of the other two groups. Both the TN-TN group and the PN-TN group had different degrees of fiber invasion and unevenly distributed myelinated nerve fibers. Scale bar: 25 μm.
Gastrocnemius Wet Weight and Strength
The gastrocnemius muscle weight of the surgery group was significantly lower than that of the normal group (P < 0.001, tested by one way ANOVA) (Table 2). The nutritional status of the gastrocnemius muscle in the TN-TN group was significantly better than that in the PN-TN group (Figure 5).
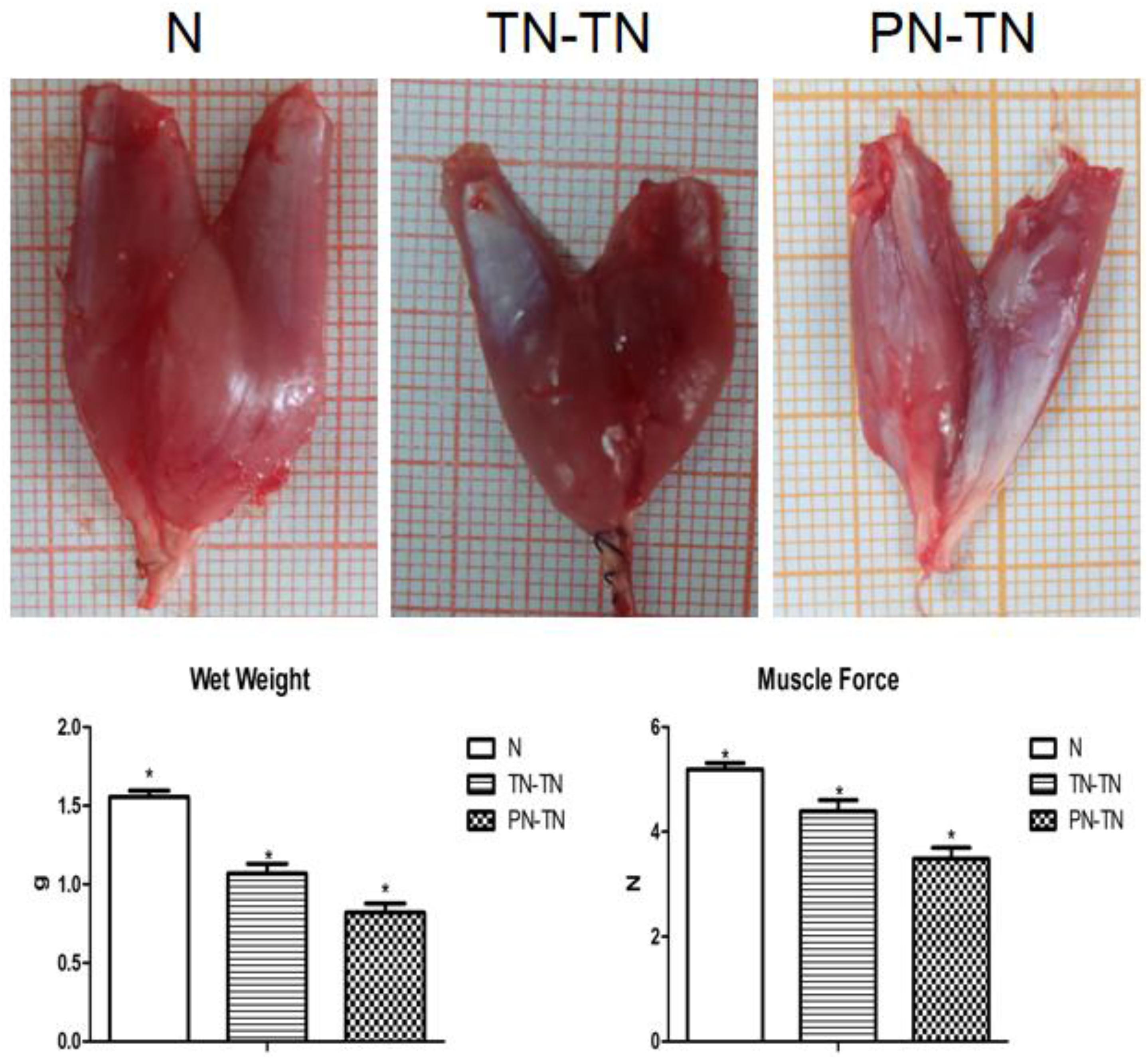
Figure 5. The first row shows the appearance of the right gastrocnemius muscle in each group. The gastrocnemius muscles in the N group were large while gastrocnemius atrophy was significant in the PT-TN group. The second row shows the muscle wet weights and strengths. Consistent with the appearance of the muscles, the muscle status of the N group was the best and that of the PN-TN group was the worst. *P < 0.05 vs. other groups.
The muscle forces of the three groups showed the same trend as that of the wet weight (Table 2). Muscle strength was the highest in the N group and the lowest in the PN-TN group. There was a significant difference in muscle strength between the three groups (P < 0.001, tested by one way ANOVA).
Muscle Fibers Morphology
The muscle fiber diameters were 34.54 ± 7.25, 23.33 ± 3.58, and 18.67 ± 2.94 μm for the N, TN-TN, and PN-TN groups, respectively; the differences between the three groups were statistically significant (P < 0.001, tested by one way ANOVA). Based on the results of the Gomori staining, no mitochondrial lesions were observed in the muscle fibers in any of the three groups (Figure 6).
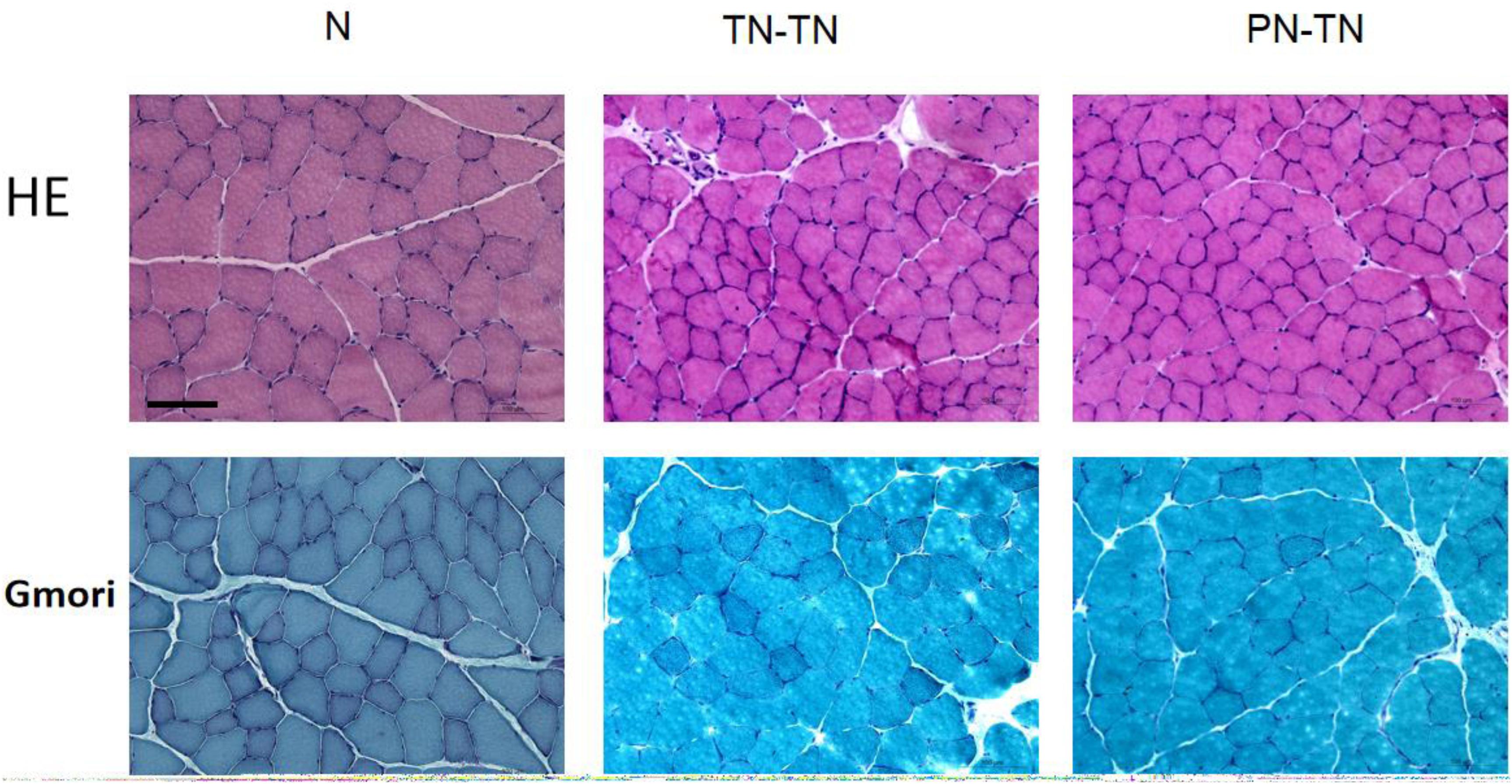
Figure 6. Morphology of muscle fibers. The muscle fibers in group N were distinctly angular and closely arranged. In the TN-TN and PN-TN groups, oval-shaped muscle fibers could be seen and the gap between the muscle fibers was widened. The muscle fiber diameter of the N group was significantly larger than those of the other two groups. The Gomori staining was normal and there were no signs of mitochondrial disease. Scale bar: 100 μm.
DRG Morphology
The morphology and distribution of the dorsal root ganglion neurons were not significantly different among the three groups; the neurons in all three groups were clustered and near the spinal cord. The expression of NF-200 in the PN-TN group was significantly higher than that in the other two groups. The expression of NF-200 in the TN-TN group was slightly higher than that in the sham operation group, but the difference was not statistically significant (Figure 7).
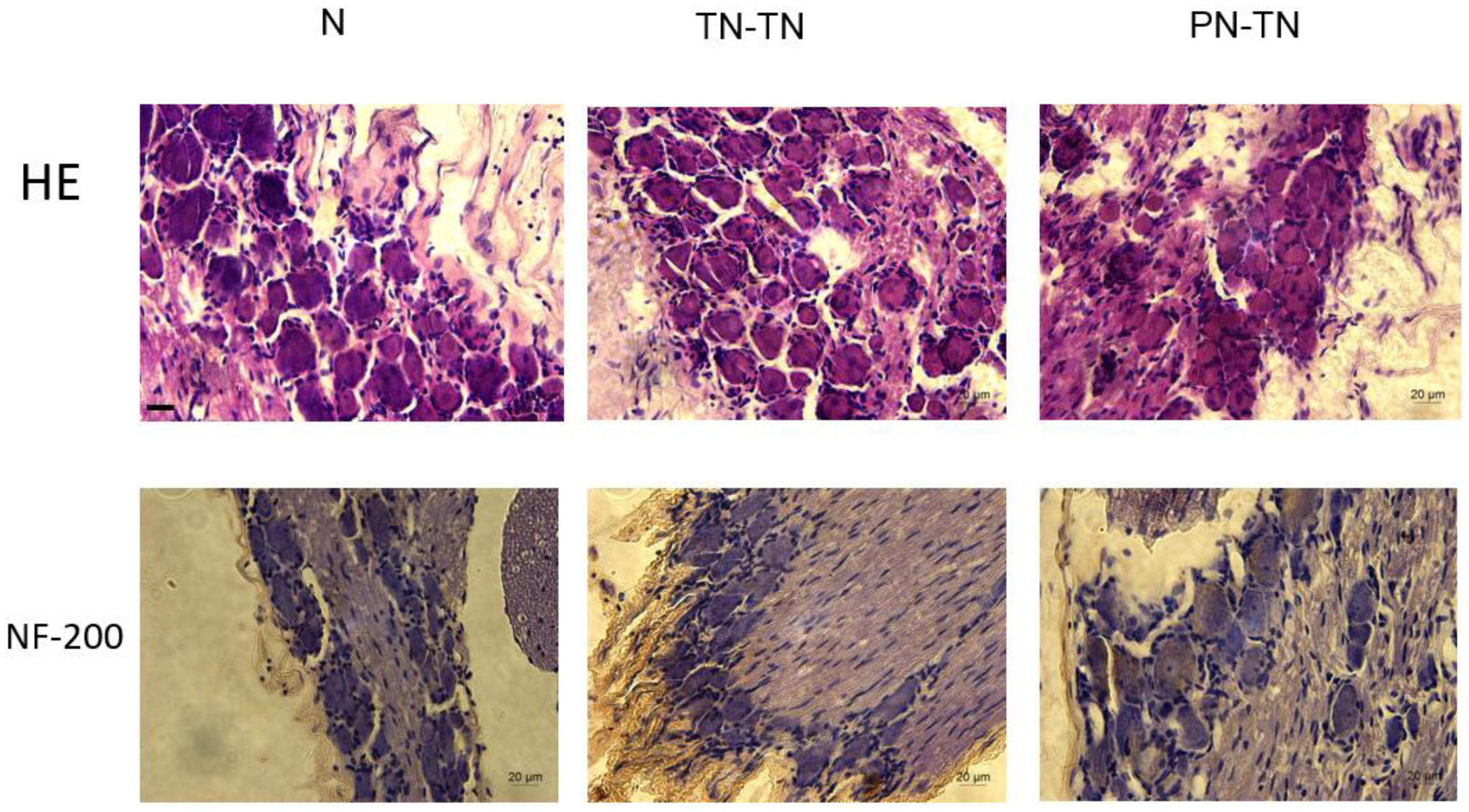
Figure 7. DRG morphology and immunohistochemical staining. Yellow-brown staining represents NF-200 positive cells. Scale bar: 20 μm.
Expression of Akt/mTOR/p70S6K
The expression levels of AKT and p70S6K were highest in the normal group, followed by those in the TN-TN group; expression was the lowest in the PN-TN group. The expression of mTOR was the lowest in the normal group and slightly higher in the TN-TN group, while the PN-TN group showed the highest expression. The differences in the expression levels of these three proteins between the three groups were statistically significant (Figure 8 and Table 3).
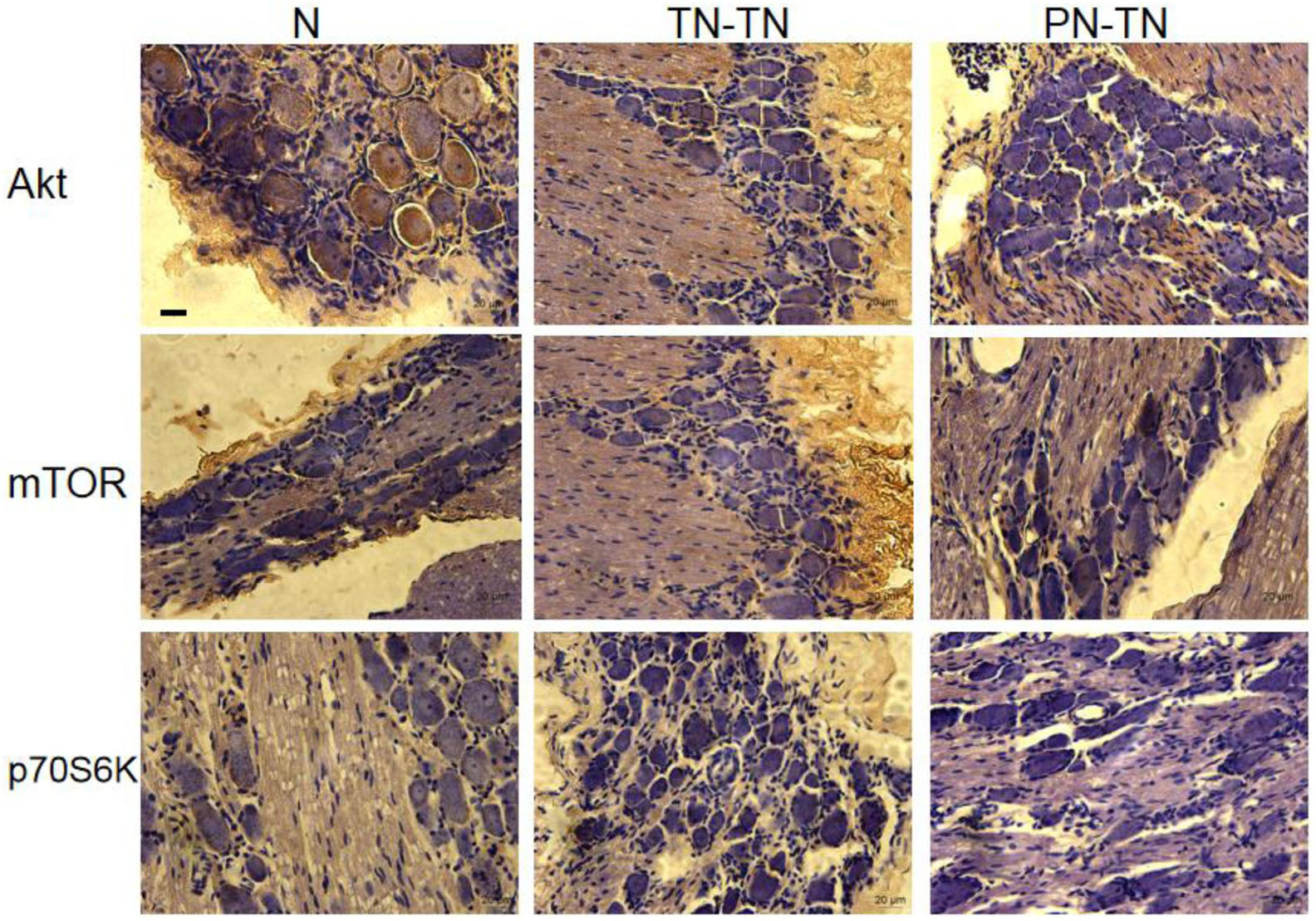
Figure 8. Expression of Akt/mTOR/p70S6K in the DRG. Yellow-brown staining represents protein expression, with a more intense color representing higher expression levels. Scale bar: 20 μm.
Discussion
The repair of a nerve damage has always been a key issue for neuroscientists around the world; however, the development of more effective treatments for limb paralysis and dysfunction due to a nerve damage has been stagnant (Palmisano et al., 2019). At present, the number of patients with dysfunction after a peripheral nerve injury in China is close to 20 million, and it is increasing at a rate of nearly 2 million per year (Fowler et al., 2015). In case of some special types of nerve injury, such as a nerve root laceration and avulsion, traditional surgical techniques such as tension-free nerve adventitia implantation, autologous nerve transplantation, and nerve cannula have limited efficacy (Kubota et al., 2011; Rebowe et al., 2018; Sun et al., 2019). Zhang et al. creatively proposed C7 nerve transposition from the healthy side to repair the paralyzed upper limb and gained nice effects (Jiang et al., 2018; Zheng et al., 2018). The use of an adjacent nerve transposition to repair paralysis and the use of the brachial plexus nerve of the affected limb of children for similar purposes has also had encouraging results. The recent popularization and application of the Shore reflex arc has also been beneficial for some patients with urinary incontinence (Lam Van Ba et al., 2018). In our laboratory, we found that when the effector changes were dominated by the neuron clusters in the spinal cord, the function and anatomical distribution of its neurons changed, which meant that the transposition repair method built a new, functional reflection loop (Yu et al., 2016).
In our earlier studies, we also found that, during the process of nerve injury and repair, the functional state of effectors induces the remodeling of the central nervous system. The survival status of the neuronal cell body determines the regeneration potential of the nerve after a peripheral nerve injury and subsequent surgical repair of the injured nerve. Furthermore, the specific selection of the distal and proximal ends of the nerve fiber and the multi-factor regulation of the regeneration state determines the nerve regeneration process. The central nervous system will recognize the changes of the peripheral effectors and regulate their function when the regenerated nerve fiber establishes a new connection with a well-preserved peripheral effector. At the same time, the peripheral effector also induces the corresponding structural and functional remodeling of neurons at all levels. Thus, the nerve function after repair reflected the original function of the repaired peripheral effector.
By enabling the transmission of the internal and external signals, the protein kinase signal cascade plays a very important role in the regulation of many cellular processes, including cell survival, proliferation, differentiation, growth cessation, and apoptosis. With the deepening of our understanding of the protein signaling mechanisms and the exploration of the role of protein signaling cascades under normal physiological conditions, the role of protein signaling cascades under pathological conditions has attracted attention. Extracellular signals, such as mechanical forces transmitted by trauma, stimulate the protein kinase cascade, which can lead to the induction and activation of the transcription factors that can regulate gene expression related to cell growth and apoptosis, thereby affecting the damaged cell and tissue repair and plasticity. The post-injury persistent activation properties of some protein kinases suggest the existence of critical time windows for the treatment of various nervous system injuries (Neary, 2005).
Akt is at the center of a cellular signaling network that plays an important role in key biological functions and cellular processes (Manning and Toker, 2017). mTOR is an important downstream signaling molecule in the Akt pathway and can be activated through phosphorylation by AKT. It is generally believed that mTOR exerts its role through phosphorylation to activate two downstream signaling molecules: the eukaryotic promoter 4E binding protein 1 and P70S6K (Burnett et al., 1998).
Akt is also specifically involved in the neural processes such as neuronal development (Jaworski et al., 2005; Kumar et al., 2005), differentiation (Otaegi et al., 2006; Lim et al., 2007), survival, axonal growth (Sango et al., 2008; Toth et al., 2008), and mediation of the synaptic plasticity (Wang et al., 2003; Horwood et al., 2006). In addition, some studies (Dudek et al., 1997; Owada et al., 1997; Brunet et al., 2001) have also shown that the post-optic nerve and hypoglossal nerve injury increase in Akt expression is related to both the anti-apoptotic effects and axon regeneration. After a brain injury (Namikawa et al., 2000; Noshita et al., 2002; Kretz et al., 2005) and spinal cord injury (Hung et al., 2007; Shioda et al., 2007, 2008; Chen et al., 2008), its enhanced expression has neuroprotective effects and is beneficial for the recovery of neural function. As a central regulator of cell growth, cell cycle progression, survival, and differentiation, mTOR also plays an important role in the physiological and pathological processes of the nervous system (Sakurai et al., 2003; Yu et al., 2005), and its possible role in central nervous system trauma has also attracted considerable attention. Studies have shown that mTOR is involved in the regulation and control of the size of neurons in the dentate gyrus and hippocampus (Hay and Sonenberg, 2004). As an important meeting point for multiple signaling pathways, mTOR has been shown to control the neuronal development (Kwon et al., 2006; Swiech et al., 2008), differentiation (Jaworski and Sheng, 2006; Muir et al., 2019), and regeneration by regulating global or local protein synthesis. Several studies (Dash et al., 2006; Bekinschtein et al., 2007; Parsons et al., 2010) have also reported that mTOR activation is related to memory formation and spatial memory. By regulating the downstream component p70S6K, mTOR has also been shown to upregulate the expression of long-term potentiating effector proteins required for synaptic connections (Tang et al., 2002).
In this study, we transposed the common peroneal nerve to repair the tibial nerve and compared the results with those of in situ repair of the tibial nerve as a control to explore the possible mechanisms underlying the successful establishment of an effective reflex arc by the transposition repair. In PN-TN group, the continuity of the nerve had been maintained and well repaired with regard to both the microstructure and the macrostructure. Furthermore, its electrical signal transmission ability had been restored, resulting in the innervation of the tibialis anterior muscle; certain systolic functions of this muscle had been restored, and rats in the PN-TN group could exercise the dorsiflexion function. This suggested that the effector changes induced neurons to undergo a functional remodeling. However, in the process of transposition repair, there was still a certain gap in the recovery of neural function compared with that in in situ nerved repair. Between the three groups, there were significant differences in the nerve conduction velocity, compound action potential amplitude, myelinated nerve fiber number, myelin sheath thickness, and muscle wet weight. The repair group was worse than the sham operation group, while the PN-TN group was worse than the TN-TN group.
Interestingly, after the immunohistochemical staining for NF-200 in the dorsal root ganglia, it was found that the expression levels of N group, TN-TN group, and PN-TN group increased gradually with obvious differences. It was suggested that the effector could induce the neuron to undergo a structural remodeling to adapt to the new reflex loop from a neuroskeletal structure perspective. This change might be related to the regeneration of axonal buds during the nerve repair. Peripheral nerves possess the ability of axillary bud regeneration during the regeneration process, which means that a proximal axon can generate several axillary buds and connect to the distal nerve stump. This theory provides the anatomical foundation for small nerve translocation to repair large nerves. Even with in situ nerve adventitia suture, more distal nerve fibers are more enlarged than the proximal nerve fibers in the early stage of nerve regeneration. However, during the long-term recovery, axillary bud trimming will occur, and the enlargement ratio will gradually approach one (Jianping et al., 2012; Barbe et al., 2017). Therefore, the proximal nerves in both the PN-TN and TN-TN groups developed lateral axis buds when the distal access channel was fixed but were translocated relative to the corresponding neurons of the original tibial nerve. Compared to the neurons in the spinal cord corresponding to the original tibial nerve, the number of axillary buds corresponding to the transfigured and repaired peroneal neurons would be higher, which meant a more complicated axoplasm transport process and a complicated neural skeleton. Therefore, the expression of NF-200, a neuroskeletal proteins, in the DRG of the PN-TN group was higher than that of the TN-TN group.
Akt/mTOR/p70S6K is a protease cascade signaling pathway related to cell proliferation, with phosphorylation as the activation mode. Significant differences in Akt, mTOR, and p70S6K expression was observed among the three groups in this study. Compared with that in the other two groups, the expression of Akt and p70S6K was lower in the PN-TN group, while the expression of mTOR was higher. It is suggested that in the process of an effector–induced neuronal structural and functional remodeling, different repair methods ultimately affect the neuron’s survival state; thus translocation repair results in a special, new, and effective reflection arc different from that in in situ repair.
The limitation of this study was that only the spontaneous changes of the Akt/mTOR/p70S6K signaling pathway during an effector – induced neuronal remodeling were investigated, and the remodeling process involving these three small molecule inhibitors were not clarified clearly. More complex regulatory mechanisms involving the three proteins were not explored. However, it was the first time to explore the molecular mechanism of a spinal cord remodeling caused by the lower limbs nerve transposition repair. We will do more in-depth research on this basis in the future.
In summary, this study proved that the translocation repair is also an effective nerve repair method. This kind of repair could promote the structural and functional remodeling of the central neurons and result in the formation of a new reflex arc. Furthermore, the Akt/mTOR/p70S6K signaling pathway might play an important role in the central nervous system remodeling.
Data Availability Statement
All datasets presented in this study are included in the article/supplementary material.
Ethics Statement
The animal study was reviewed and approved by the Ethics Committee of the People’s Hospital of Peking University, China.
Author Contributions
HX and PZ designed and supported the study, and reviewed the manuscript. YY and DL performed the experiments and analyzed the data. FY and XK assisted with the experiments. All authors contributed to the article and approved the submitted version.
Funding
This study was supported by the National Natural Science Foundation (Grant Nos. 31771322, 31571235, and 81671215), the Beijing Municipal Science and Technology Commission Science and Technology Nova Cross Project (Grant No. 2018019), the Educational Ministry New Century Excellent Talents Support Project (Grant No. BMU20110270), the Key Laboratory of Trauma and Neural Regeneration (Peking University), the Ministry of Education (Grant No. BMU2019XY007-01), the Ministry of Education Innovation Program of China (Grant No. IRT_16R01), and the National Key R&D Program of China (Grant No. 2016YFC1101604).
Conflict of Interest
The authors declare that the research was conducted in the absence of any commercial or financial relationships that could be construed as a potential conflict of interest.
References
An, S., Zhang, P., Peng, J., Deng, L., Wang, Z., Wang, Z., et al. (2015). Motor function recovery during peripheral nerve multiple regeneration. J. Tissue Engl. Regen. Med. 9, 415–423. doi: 10.1002/term.1833
Barbe, M. F., Gomez-Amaya, S., Braverman, A. S., Brown, J. M., Lamarre, N. S., Massicotte, V. S., et al. (2017). Evidence of vagus nerve sprouting to innervate the urinary bladder and clitoris in a canine model of lower motoneuron lesioned bladder. Neurourol. Urodyn. 36, 91–97. doi: 10.1002/nau.22904
Bekinschtein, P., Katche, C., Slipczuk, L. N., Igaz, L. M., Cammarota, M., Izquierdo, I., et al. (2007). mTOR signaling in the hippocampus is necessary for memory formation. Neurobiol. Learn. Mem. 87, 303–307. doi: 10.1016/j.nlm.2006.08.007
Brunet, A., Datta, S. R., and Greenberg, M. E. (2001). Transcription-dependent and -independent control of neuronal survival by the PI3K-Akt signaling pathway. Curr. Opin. Neurobiol. 11, 297–305. doi: 10.1016/s0959-4388(00)00211-7
Burnett, P. E., Barrow, R. K., Cohen, N. A., Snyder, S. H., and Sabatini, D. M. (1998). RAFT1 phosphorylation of the translational regulators p70 S6 kinase and 4E-BP1. Proc. Natl. Acad. Sci. U.S.A. 95, 1432–1437. doi: 10.1073/pnas.95.4.1432
Chen, A., Xiong, L. J., Tong, Y., and Mao, M. (2013). Neuroprotective effect of brain-derived neurotrophic factor mediated by autophagy through the PI3K/Akt/mTOR pathway. Mol. Med. Rep. 8, 1011–1016. doi: 10.3892/mmr.2013.1628
Chen, W. F., Jean, Y. H., Sung, C. S., Wu, G. J., Huang, S. Y., Ho, J. T., et al. (2008). Intrathecally injected granulocyte colony-stimulating factor produced neuroprotective effects in spinal cord ischemia via the mitogen-activated protein kinase and Akt pathways. Neuroscience 153, 31–43. doi: 10.1016/j.neuroscience.2008.01.062
Dash, P. K., Orsi, S. A., and Moore, A. N. (2006). Spatial memory formation and memory-enhancing effect of glucose involves activation of the tuberous sclerosis complex-Mammalian target of rapamycin pathway. J. Neurosci. 26, 8048–8056. doi: 10.1523/JNEUROSCI.0671-06.2006
Dudek, H., Datta, S. R., Franke, T. F., Birnbaum, M. J., Yao, R., Cooper, G. M., et al. (1997). Regulation of neuronal survival by the serine-threonine protein kinase Akt. Science 275, 661–665. doi: 10.1126/science.275.5300.661
Fingar, D. C., and Blenis, J. (2004). Target of rapamycin (TOR): an integrator of nutrient and growth factor signals and coordinator of cell growth and cell cycle progression. Oncogene 23, 3151–3171. doi: 10.1038/sj.onc.1207542
Fowler, J. R., Lavasani, M., Huard, J., and Goitz, R. J. (2015). Biologic strategies to improve nerve regeneration after peripheral nerve repair. J. Reconstr. Microsurg. 31, 243–248. doi: 10.1055/s-0034-1394091
Hay, N., and Sonenberg, N. (2004). Upstream and downstream of mTOR. Genes Dev 18, 1926–1945. doi: 10.1101/gad.1212704
Horwood, J. M., Dufour, F., Laroche, S., and Davis, S. (2006). Signalling mechanisms mediated by the phosphoinositide 3-kinase/Akt cascade in synaptic plasticity and memory in the rat. Eur. J. Neurosci. 23, 3375–3384. doi: 10.1111/j.1460-9568.2006.04859.x
Huckhagel, T., Nüchtern, J., Regelsberger, J., Lefering, R., and TraumaRegister, D. G. U. (2018). Nerve injury in severe trauma with upper extremity involvement: evaluation of 49,382 patients from the TraumaRegister DGU§between 2002 and 2015. Scand. J. Trauma Resusc. Emerg. Med. 26:76. doi: 10.1186/s13049-018-0546-6
Hung, K. S., Tsai, S. H., Lee, T. C., Lin, J. W., Chang, C. K., and Chiu, W. T. (2007). Gene transfer of insulin-like growth factor-I providing neuroprotection after spinal cord injury in rats. J. Neurosurg. Spine 6, 35–46. doi: 10.3171/spi.2007.6.1.35
Jaworski, J., and Sheng, M. (2006). The growing role of mTOR in neuronal development and plasticity. Mol. Neurobiol. 34, 205–219. doi: 10.1385/MN
Jaworski, J., Spangler, S., Seeburg, D. P., Hoogenraad, C. C., and Sheng, M. (2005). Control of dendritic arborization by the phosphoinositide-3’-kinase-Akt-mammalian target of rapamycin pathway. J. Neurosci. 25, 11300–11312. doi: 10.1523/JNEUROSCI.2270-05.2005
Jiang, Y., Wang, L., Lao, J., and Zhao, X. (2018). Total brachial plexus injury: contralateral C7 root transfer to the lower trunk versus the median nerve. Neural Regen. Res. 13, 1968–1973. doi: 10.4103/1673-5374.239444
Jianping, P., Xiaofeng, Y., Yanhua, W., Zhenwei, W., Yuhui, K., Chungui, X., et al. (2012). Different multiple regeneration capacities of motor and sensory axons in peripheral nerve. Artif. Cell. Blood Substit. Immobil. Biotechnol. 40, 309–316. doi: 10.3109/10731199.2012.657205
Kawabata, H., Shibata, T., Matsui, Y., and Yasui, N. (2001). Use of intercostal nerves for neurotization of the musculocutaneous nerve in infants with birth-related brachial plexus palsy. J. Neurosurg. 94, 386–391. doi: 10.3171/jns.2001.94.3.0386
Kretz, A., Happold, C. J., Marticke, J. K., and Isenmann, S. (2005). Erythropoietin promotes regeneration of adult CNS neurons via Jak2/Stat3 and PI3K/AKT pathway activation. Mol. Cell Neurosci. 29, 569–579. doi: 10.1016/j.mcn.2005.04.009
Kubota, S., Nishiura, Y., Hara, Y., Saijilafu, Abe, I., and Ochiai, N. (2011). Functional and morphological effects of indirect gradual elongation of peripheral nerve: electrophysiological and morphological changes at different elongation rates. Hand Surg. 16, 105–111. doi: 10.1142/S0218810411005199
Kumar, V., Zhang, M. X., Swank, M. W., Kunz, J., and Wu, G. Y. (2005). Regulation of dendritic morphogenesis by Ras-PI3K-Akt-mTOR and Ras-MAPK signaling pathways. J. Neurosci. 25, 11288–11299. doi: 10.1523/JNEUROSCI.2284-05.2005
Kwon, C. H., Luikart, B. W., Powell, C. M., Zhou, J., Matheny, S. A., Zhang, W., et al. (2006). Pten regulates neuronal arborization and social interaction in mice. Neuron 50, 377–388. doi: 10.1016/j.neuron.2006.03.023
Lam Van Ba, O., Barbe, M. F., Caremel, R., Aharony, S., Loutochin, O., Jacques, L., et al. (2018). Lumbar to sacral root rerouting to restore bladder function in a feline spinal cord injury model: urodynamic and retrograde nerve tracing results from a pilot study. Neurourol. Urodyn. 37, 153–162. doi: 10.1002/nau.23394
Lim, M. S., Nam, S. H., Kim, S. J., Kang, S. Y., Lee, Y. S., and Kang, K. S. (2007). Signaling pathways of the early differentiation of neural stem cells by neurotrophin-3. Biochem. Biophys. Res. Commun. 357, 903–909. doi: 10.1016/j.bbrc.2007.04.045
Loewith, R., and Hall, M. N. (2011). Target of rapamycin (TOR) in nutrient signaling and growth control. Genetics 189, 1177–1201. doi: 10.1534/genetics.111.133363
Manning, B. D., and Toker, A. (2017). AKT/PKB signaling: navigating the network. Cell 169, 381–405. doi: 10.1016/j.cell.2017.04.001
Muir, J., Lopez, J., and Bagot, R. C. (2019). Wiring the depressed brain: optogenetic and chemogenetic circuit interrogation in animal models of depression. Neuropsychopharmacology 44, 1013–1026. doi: 10.1038/s41386-018-0291-6
Namikawa, K., Honma, M., Abe, K., Takeda, M., Mansur, K., Obata, T., et al. (2000). Akt/protein kinase B prevents injury-induced motoneuron death and accelerates axonal regeneration. J. Neurosci. 20, 2875–2886. doi: 10.1523/JNEUROSCI.20-08-02875.2000
Neary, J. T. (2005). Protein kinase signaling cascades in CNS trauma. IUBMB Life 57, 711–718. doi: 10.1080/15216540500319143
Noshita, N., Lewen, A., Sugawara, T., and Chan, P. H. (2002). Akt phosphorylation and neuronal survival after traumatic brain injury in mice. Neurobiol. Dis. 9, 294–304. doi: 10.1006/nbdi.2002.0482
Otaegi, G., Yusta-Boyo, M. J., Vergano-Vera, E., Mendez-Gomez, H. R., Carrera, A. C., Abad, J. L., et al. (2006). Modulation of the PI 3-kinase-Akt signalling pathway by IGF-I and PTEN regulates the differentiation of neural stem/precursor cells. J. Cell Sci. 119, 2739–2748. doi: 10.1242/jcs.03012
Owada, Y., Utsunomiya, A., Yoshimoto, T., and Kondo, H. (1997). Expression of mRNA for Akt, serine-threonine protein kinase, in the brain during development and its transient enhancement following axotomy of hypoglossal nerve. J. Mol. Neurosci. 9, 27–33. doi: 10.1007/BF02789392
Palmisano, I., Danzi, M. C., Hutson, T. H., Zhou, L., McLachlan, E., and Serger, E. (2019). Epigenomic signatures underpin the axonal regenerative ability of dorsal root ganglia sensory neurons. Nat. Neurosci. 22, 1913–1924. doi: 10.1038/s41593-019-0490-4
Parsons, R. G., Gafford, G. M., and Helmstetter, F. J. (2010). Regulation of extinction-related plasticity by opioid receptors in the ventrolateral periaqueductal gray matter. Front. Behav. Neurosci. 4:44. doi: 10.3389/fnbeh.2010.00044
Qian, Y., Song, J., Zhao, X., Chen, W., Ouyang, Y., Yuan, W., et al. (2018a). 3D fabrication with integration molding of a graphene oxide/polycaprolactone nanoscaffold for neurite regeneration and angiogenesis. Adv. Sci. 5:1700499. doi: 10.1002/advs.201700499
Qian, Y., Yuan, W.-E., Cheng, Y., Yang, Y., Qu, X., and Fan, C. (2019). Concentrically integrative bioassembly of a three-dimensional black phosphorus nanoscaffold for restoring neurogenesis, angiogenesis, and immune homeostasis. Nano Lett. 19, 8990–9001. doi: 10.1021/acs.nanolett.9b03980
Qian, Y., Zhao, X., Han, Q., Chen, W., Li, H., and Yuan, W. (2018b). An integrated multi-layer 3D-fabrication of PDA/RGD coated graphene loaded PCL nanoscaffold for peripheral nerve restoration. Nat. Commun. 9:323. doi: 10.1038/s41467-017-02598-7
Rebowe, R., Rogers, A., Yang, X., Kundu, S. C., Smith, T. L., and Li, Z. (2018). Nerve repair with nerve conduits: problems, solutions, and future directions. J. Hand. Microsurg. 10, 61–65. doi: 10.1055/s-0038-1626687
Sakurai, M., Nagata, T., Abe, K., Horinouchi, T., Itoyama, Y., and Tabayashi, K. (2003). Oxidative damage and reduction of redox factor-1 expression after transient spinal cord ischemia in rabbits. J. Vasc. Surg. 37, 446–452. doi: 10.1067/mva.2003.100
Sango, K., Yanagisawa, H., Komuta, Y., Si, Y., and Kawano, H. (2008). Neuroprotective properties of ciliary neurotrophic factor for cultured adult rat dorsal root ganglion neurons. Histochem. Cell Biol. 130, 669–679. doi: 10.1007/s00418-008-0484-x
Shioda, N., Han, F., Morioka, M., and Fukunaga, K. (2008). Bis(1-oxy-2-pyridinethiolato)oxovanadium(IV) enhances neurogenesis via phosphatidylinositol 3-kinase/Akt and extracellular signal regulated kinase activation in the hippocampal subgranular zone after mouse focal cerebral ischemia. Neuroscience 155, 876–887. doi: 10.1016/j.neuroscience.2008.05.056
Shioda, N., Ishigami, T., Han, F., Moriguchi, S., Shibuya, M., Iwabuchi, Y., et al. (2007). Activation of phosphatidylinositol 3-kinase/protein kinase B pathway by a vanadyl compound mediates its neuroprotective effect in mouse brain ischemia. Neuroscience 148, 221–229. doi: 10.1016/j.neuroscience.2007.05.040
Sun, B. J., Tijerina, J. D., Agbim, U. N., Lee, G. K., and Nazerali, R. S. (2019). The quality of systematic reviews addressing peripheral nerve repair and reconstruction. J. Plast Reconstr. Aesthet. Surg. 72, 447–456. doi: 10.1016/j.bjps.2018.10.047
Swiech, L., Perycz, M., Malik, A., and Jaworski, J. (2008). Role of mTOR in physiology and pathology of the nervous system. Biochim. Biophys. Acta 1784, 116–132. doi: 10.1016/j.bbapap.2007.08.015
Tang, S. J., Reis, G., Kang, H., Gingras, A. C., Sonenberg, N., and Schuman, E. M. (2002). A rapamycin-sensitive signaling pathway contributes to long-term synaptic plasticity in the hippocampus. Proc. Natl. Acad. Sci. U.S.A. 99, 467–472. doi: 10.1073/pnas.012605299
Toth, C., Martinez, J. A., Liu, W. Q., Diggle, J., Guo, G. F., Ramji, N., et al. (2008). Local erythropoietin signaling enhances regeneration in peripheral axons. Neuroscience 154, 767–783. doi: 10.1016/j.neuroscience.2008.03.052
Wang, Q., Liu, L., Pei, L., Ju, W., Ahmadian, G., Lu, J., et al. (2003). Control of synaptic strength, a novel function of Akt. Neuron 38, 915–928. doi: 10.1016/s0896-6273(03)00356-8
Yu, F., Sugawara, T., Maier, C. M., Hsieh, L. B., and Chan, P. H. (2005). Akt/Bad signaling and motor neuron survival after spinal cord injury. Neurobiol. Dis. 20, 491–499. doi: 10.1016/j.nbd.2005.04.004
Yu, Y., Zhang, P., Han, N., Kou, Y., Yin, X., and Jiang, B. (2016). Collateral development and spinal motor reorganization after nerve injury and repair. Am. J. Transl. Res. 8, 2897–2911.
Keywords: peripheral nerve injury, translocation repair, remodeling, dorsal root ganglion, signaling pathway
Citation: Yuan Y, Li D, Yu F, Kang X, Xu H and Zhang P (2020) Effects of Akt/mTOR/p70S6K Signaling Pathway Regulation on Neuron Remodeling Caused by Translocation Repair. Front. Neurosci. 14:565870. doi: 10.3389/fnins.2020.565870
Received: 26 May 2020; Accepted: 25 August 2020;
Published: 29 September 2020.
Edited by:
Hari S. Sharma, Uppsala University, SwedenReviewed by:
Cunyi Fan, Shanghai Jiao Tong University, ChinaAlcides Chaux, Universidad del Norte, Paraguay
Copyright © 2020 Yuan, Li, Yu, Kang, Xu and Zhang. This is an open-access article distributed under the terms of the Creative Commons Attribution License (CC BY). The use, distribution or reproduction in other forums is permitted, provided the original author(s) and the copyright owner(s) are credited and that the original publication in this journal is cited, in accordance with accepted academic practice. No use, distribution or reproduction is permitted which does not comply with these terms.
*Correspondence: Hailin Xu, eHVoYWlsaW5mYUAxNjMuY29t; Peixun Zhang, emhhbmdwZWl4dW5AYmptdS5lZHUuY24=
†These authors have contributed equally to this work and share first authorship