- 1Department of Physiology, UT Southwestern Medical Center at Dallas, Dallas, TX, United States
- 2Laboratory of Molecular Neurodegeneration, Peter the Great St. Petersburg State Polytechnic University, Saint Petersburg, Russia
Sigma-1 receptor (S1R) is a multi-functional, ligand-operated protein situated in endoplasmic reticulum (ER) membranes and changes in its function and/or expression have been associated with various neurological disorders including amyotrophic lateral sclerosis/frontotemporal dementia, Alzheimer’s (AD) and Huntington’s diseases (HD). S1R agonists are broadly neuroprotective and this is achieved through a diversity of S1R-mediated signaling functions that are generally pro-survival and anti-apoptotic; yet, relatively little is known regarding the exact mechanisms of receptor functioning at the molecular level. This review summarizes therapeutically relevant mechanisms by which S1R modulates neurophysiology and implements neuroprotective functions in neurodegenerative diseases. These mechanisms are diverse due to the fact that S1R can bind to and modulate a large range of client proteins, including many ion channels in both ER and plasma membranes. We summarize the effect of S1R on its interaction partners and consider some of the cell type- and disease-specific aspects of these actions. Besides direct protein interactions in the endoplasmic reticulum, S1R is likely to function at the cellular/interorganellar level by altering the activity of several plasmalemmal ion channels through control of trafficking, which may help to reduce excitotoxicity. Moreover, S1R is situated in lipid rafts where it binds cholesterol and regulates lipid and protein trafficking and calcium flux at the mitochondrial-associated membrane (MAM) domain. This may have important implications for MAM stability and function in neurodegenerative diseases as well as cellular bioenergetics. We also summarize the structural and biochemical features of S1R proposed to underlie its activity. In conclusion, S1R is incredibly versatile in its ability to foster neuronal homeostasis in the context of several neurodegenerative disorders.
Introduction
Sigma-1 receptor (S1R) is a ligand-operated protein that modulates activity of several client proteins from its position within the membrane of the endoplasmic reticulum (ER). It is widely expressed in multiple organs including the nervous system (Gundlach et al., 1986) and it has important roles in modulation of neuronal physiology (Maurice et al., 2006a) and synaptic plasticity (Takebayashi et al., 2004; Tsai et al., 2009; Kourrich et al., 2012). Autosomal recessive loss-of-function mutations in S1R are primarily associated with amyotrophic lateral sclerosis/frontotemporal dementia (ALS/FTD) (Luty et al., 2010; Al-Saif et al., 2011; Kim et al., 2014; Li et al., 2015; Ullah et al., 2015; Gregianin et al., 2016; Horga et al., 2016; Watanabe et al., 2016), but polymorphisms in S1R also affect risk of developing Alzheimer’s disease (AD) (Uchida et al., 2005; Maruszak et al., 2007; Huang et al., 2011; Fehér et al., 2012). Many S1R agonists are neuroprotective and loss of S1R accelerates neurodegenerative phenotypes (Maurice and Lockhart, 1997; Nakazawa et al., 1998; Smith et al., 2008; Mavlyutov et al., 2011, 2013; Mancuso et al., 2012; Ono et al., 2014; Ryskamp et al., 2017, 2019; Maurice et al., 2018). Neuroprotection from S1R activation is achieved by a diversity of signaling functions that promote cellular homeostasis and synaptic stability. In this review we summarize therapeutically relevant mechanisms by which the ligand-operated chaperone S1R modulates neurophysiology, counteracting its dysregulation from pathogenic stressors.
Modulation of Neurophysiology by S1R
Sigma-1 receptor is a 223 amino acid-long transmembrane protein residing in the ER membrane. S1R preferentially localizes to the specific microdomains of the ER called mitochondrial-associated membranes (MAM), where it can regulate InsP3R-dependent calcium flux from the ER to mitochondria (Hayashi and Su, 2007), lipid dynamics (Hayashi and Su, 2005), MAM stability (Watanabe et al., 2016), and the ER stress response (Mori et al., 2013). The MAM domain is also important for synthesis and transport of lipids and protein folding (Weng et al., 2017b). Under resting conditions, S1R forms an inert complex with GRP78/BiP protein (Hayashi and Su, 2007). When activated by agonists or ER calcium depletion, S1R dissociates from BiP and redistributes to the entire ER network (Hayashi and Su, 2007), freeing it to interact with and modulate several client proteins including InsP3Rs inside and outside of the MAM domain as well as plasmalemmal ion channels, GPCRs, and kinases (summarized in Table 1).
Sigma-1 receptor agonists do not noticeably alter ER calcium signaling under resting conditions (Hayashi et al., 2000), but they can influence ER calcium release triggered by Gq-coupled receptors (Hayashi and Su, 2007; Ryskamp et al., 2017). S1R chaperones InsP3R3 to the MAM domain and prevents its degradation, enhancing Ca2+ flux into mitochondria (Hayashi and Su, 2007). This augments ATP production (Griffiths, 2009), but, in excess, it could also trigger the mitochondrial permeability transition (Lemasters et al., 2009). By contrast, InsP3R1, which is the predominant InsP3R isoform in neurons and has important signaling functions outside of the MAM, is negatively regulated by agonist-stimulated S1R in certain cell types like striatal medium spiny neurons (MSNs) (Ryskamp et al., 2017). Regulation ER calcium homeostasis and signaling by S1R has important implications for neurodegenerative diseases and this will be discussed later.
Engagement of S1R with plasmalemmal channels and receptors is responsible for S1R-dependent fine-tuning of neuronal excitability (Kourrich et al., 2012). As many of S1R’s interaction partners function in the plasma membrane, it was proposed that activated S1R translocates from the ER to the plasma membrane where it binds to client proteins and acts as a chaperone or an auxiliary subunit (McCann and Su, 1990; Morin-Surun et al., 1999; Hayashi et al., 2000; Aydar et al., 2002). However, this assumption is often based on experiments in which S1R and/or its interaction partner are overexpressed, raising several caveats that call this interpretation into question (Su et al., 2016). Alternatively, S1R may interact with plasma membrane proteins from its position in the ER like STIM proteins (Mavlyutov et al., 2010, 2015a) and/or regulate the maturation and/or trafficking of certain proteins to the plasma membrane (Crottes et al., 2011; Delint-Ramirez et al., 2018).
Sigma-1 receptor activation alters neuronal excitability through its interactions with voltage-gated ion channels. Voltage-gated sodium (Nav) channels augment neuronal depolarization and mediate action potentials. S1R ligands dissociate S1R from Nav1.5, leading to suppressed Nav1.5 activity (Johannessen et al., 2009; Balasuriya et al., 2012). This action can be evoked by the endogenous S1R agonist N,N-dimethyltryptamine (DMT) and is partially opposed by the endogenous S1R antagonist progesterone (Johannessen et al., 2011). S1R agonists also limit excitability by inhibiting other Nav channels including Nav1.2 and Nav1.4 (Johannessen et al., 2009; Gao et al., 2012). Voltage-gated potassium (Kv) channels respond to membrane depolarization during action potentials by releasing positively charged potassium ions from the cytosol to restore a hyperpolarized resting membrane potential and limit hyperexcitability. When S1R is activated by cocaine, S1R binds to the voltage-gated potassium channel Kv1.2 and enhances trafficking of Kv1.2 to the plasma membrane, decreasing excitability of dopamine D1 receptor (D1R)-expressing MSNs in the shell of the nucleus accumbens (Delint-Ramirez et al., 2018). S1R expression and activity also regulates the cardiac Kv channel hERG through control of maturation and trafficking (Crottes et al., 2011) and this function appears to be dependent on cholesterol and not S1R ligands, possibly suggesting a role for lipid rafts in S1R client protein assembly and trafficking (Balasuriya et al., 2014). S1R appears to basally regulate Kv1.3 and Kv1.4 independent of agonist-stimulation (Aydar et al., 2002; Kinoshita et al., 2012). By means of such interactions, S1R regulates neuronal excitability.
Sigma-1 receptor also influences synaptic functions through modulation of the NMDA receptor (NMDAR) activity. Physiological NMDAR activation can induce hippocampal long-term potentiation (LTP) (Lu et al., 2001), spine maturation (Tada and Sheng, 2006) and learning (Morris et al., 1986), but pathophysiological levels of NMDAR activity triggers excitotoxicity (Rothman and Olney, 1987). S1R facilitates NMDA receptor signaling and neurotransmission in hippocampal neurons (Monnet et al., 1990, 1992, 1995), possibly through altering responses to calcium signals (e.g., inhibiting Ca2+-activated SK channels) and promoting expression of NMDA receptor subunits and their trafficking to the plasma membrane (Martina et al., 2007; Pabba et al., 2014). S1R can also obviate negative-regulation of NDMARs by cannabinoid 1 receptor (CB1R) (Sanchez-Blazquez et al., 2014). These interactions enhance neuronal firing and maturation of mushroom spines from NMDA receptor activation (Monnet et al., 1990; Martina et al., 2007; Pabba et al., 2014). Modulation of calcium signaling by S1R may regulate synaptic plasticity through stimulation of CaMKII, PKC, and ERK (Moriguchi et al., 2011).
Sigma-1 receptor agonists may promote synaptic plasticity and neuronal resilience in large part through their common ability to upregulate BDNF secretion and TrkB receptor signaling both in vitro and in vivo (Kikuchi-Utsumi and Nakaki, 2008; Peviani et al., 2014). For example, pridopidine, a potent S1R receptor agonist, promotes neurotrophic signaling via BDNF, ERK, and AKT pathways (Ono et al., 2014; Geva et al., 2016; Kusko et al., 2018; Ionescu et al., 2019). S1R agonists appear to activate TrkB both through BDNF-dependent (Kimura et al., 2013) and independent mechanisms (Ka et al., 2016). This may involve regulation of BDNF expression and processing as well as direct interactions of S1R with the TrkB receptor (Fujimoto et al., 2012; Kimura et al., 2013; Ka et al., 2016). S1R also stimulates signaling by other receptor tyrosine kinases including the epidermal growth factor receptor (EGFR) (Takebayashi et al., 2004) and the platelet-derived growth factor receptor (PDGFR) (Yao et al., 2011). Stimulation of neurotrophic receptors confers neuroprotection through control of gene expression.
Indirect regulation of transcriptional activity by S1R contributes to its neuroprotective properties. For example, S1R may prevent neuronal death by upregulating expression of the antiapoptotic mitochondrial protein Bcl-2 (Meunier and Hayashi, 2010; Zhang et al., 2012). S1R regulates transcription through interactions with inositol-requiring enzyme 1 (IRE1) and emerin. S1R facilitates dimerization of the ER stress sensor and endonuclease IRE1 at the MAM domain, leading to splicing-dependent activation of the transcription factor XBP1, which goes on to upregulate several ER chaperones (Mori et al., 2013). S1R also decreases IRE1-driven inflammation (Rosen et al., 2019), which may be important for microglial reactivity and migration to and from injury sites (Moritz et al., 2015). As the ER membrane is contiguous with the nuclear envelope, activated S1R can move to the nuclear envelope where it regulates transcription through its recruitment of emerin and then chromatin-remodeling factors (Tsai et al., 2015a).
A microarray study involving knockdown of S1R in cultured hippocampal neurons revealed altered transcription in pathways controlling protein ubiquitination, sterol biosynthesis, oxidative stress, and actin dynamics (Tsai et al., 2012). Knockdown of S1R reduces the size of dendritic spine size in hippocampal neurons, indicating that it actively supports stability of mature spines (Tsai et al., 2009; Fisher et al., 2016; Ryskamp et al., 2019). This was initially proposed to involve its role in regulating oxidative stress and Rac-GTP signaling (Tsai et al., 2009), but may also involve modulation of calcium homeostasis in conditions of disease (Ryskamp et al., 2019). Knockout of S1R is associated with increased formation of reactive oxygen species (ROS) and decreased expression and activity of NRF2, which promotes expression and activation of antioxidant molecules under conditions of stress (Wang et al., 2015). This may explain how S1R suppresses generation of ROS (Meunier and Hayashi, 2010). Interestingly, spine shrinkage from knocking down S1R was prevented by reducing oxidative stress (Tsai et al., 2009).
Finally, in addition to protein–protein modulation, S1R was shown to interact with lipids. S1R localizes to lipid rafts – detergent-resistant microdomains of the ER – where it binds cholesterol at sterol-binding sites and S1R agonists such as SKF-10047 displace S1R and its binding partners from lipid rafts possibly through out-competing cholesterol binding (Hayashi and Su, 2003; Palmer et al., 2007). S1R targets to galactosyl-rich microdomains of the ER and is potentially involved in regulation of the differentiation of oligodendocytes and myelination (Hayashi and Su, 2004) as well as lipid transport to the myelin membrane (Weng et al., 2017a). S1R also supports axonal growth through promoting myristoylation of p35, which increases its degradation and thereby decreases p25/CDK5-dependent hyperphosphorylation of Tau (Tsai et al., 2015b).
This review only scratches the surface with regard to S1R’s multiplicitous roles in neurophysiology/neuroprotection and provides a glimpse into the specificity of its actions in differing cell types. It is tempting to speculate that the nature of modulation by S1R depends on the levels of S1R and its interaction partners in a given cell type (e.g., preferential interactions of S1R with cell-type abundant effectors) as well as many circumstantial factors such as the current physiological/pathophysiological state of the cell and signaling events (e.g., presence/absence of S1R ligands). To better understand possible actions of S1R, we summarized S1R interaction partners and the effects of S1R ligands and knockdown/overexpression on these protein–protein interactions (Figure 1 and Table 1).
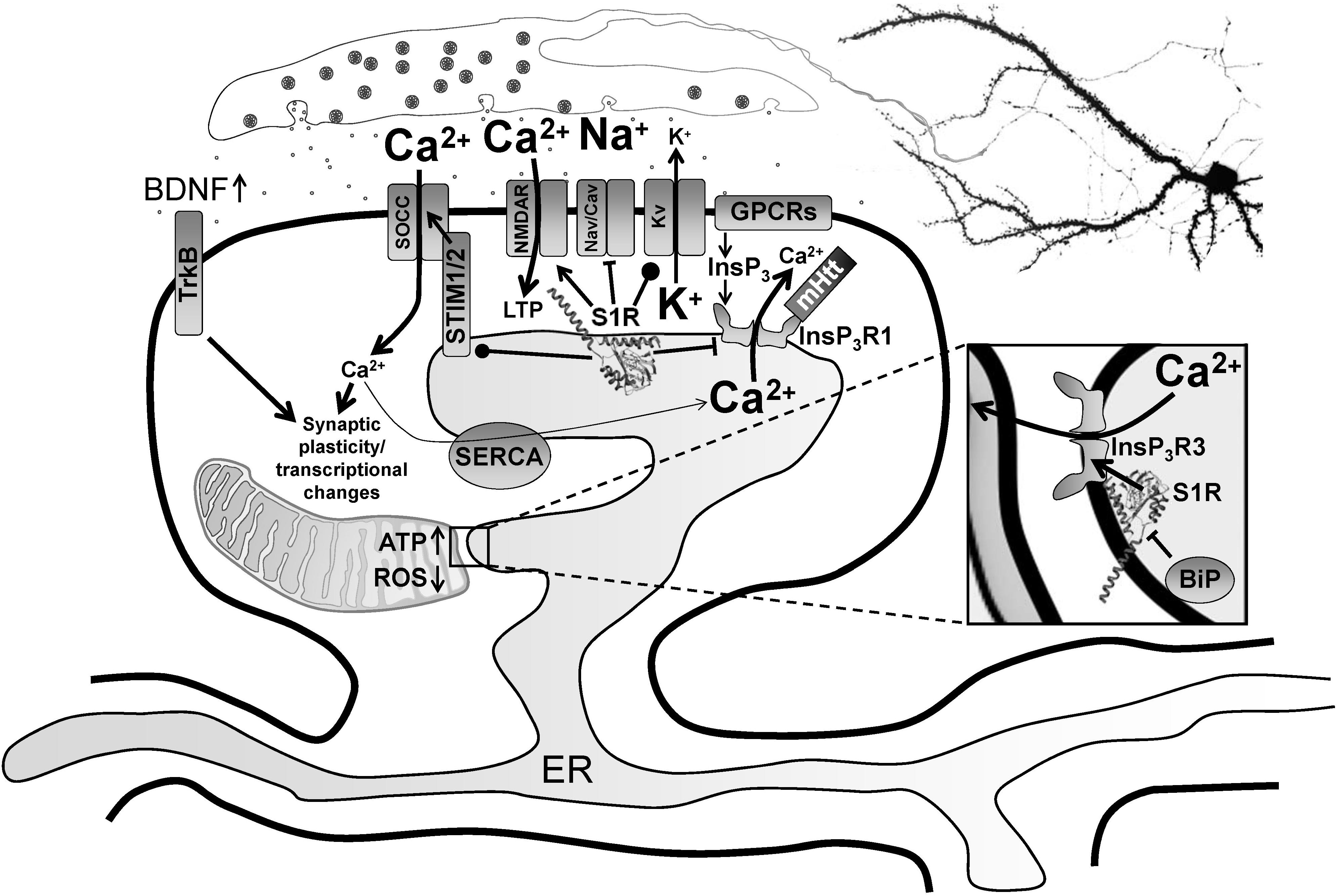
Figure 1. Modulation of neurophysiology by S1R. Normally residing at the MAM, S1R is released from BiP upon activation from ER calcium depletion, ER stress or agonist stimulation, freeing it to interact with its client proteins. Within the MAM, S1R regulates lipid dynamics and chaperones InsP3R3 to the MAM, facilitating calcium flux from the ER to mitochondria. This enhances ATP production. S1R’s actions on transcriptional pathways counteract oxidative stress through upregulation of antioxidants. Once activated, S1R redistributes to the entire ER network where it interacts with additional targets including InsP3R1, STIM1 and several plasma membrane ion channels and receptors. For example, S1R activation by pridopidine in striatal MSNs attenuates ER calcium release from InsP3R1 when it is hyperactive in HD from mutant Huntingtin protein, leading to suppression of synaptotoxic signals mediated by store-operated calcium entry channels (SOCCs). Conversely, nSOC pathway activity is important for mushroom spine stability in AD, but it is downregulated from reduced ER calcium leakage in AD models. In hippocampal neurons, S1R decreases ER calcium levels, possibly though positive regulation of presenilin leak channels (not shown). This restores nSOC pathway activity and promotes mushroom spine stability. S1R activation also limits excitotoxicity by decreasing activity of Nav and Cav channels, while promoting activity of some Kv channels. S1R enhances NMDAR activity, which is important for induction of LTP as well as activation of calcium-dependent transcription factors. S1R also modulates several GPCRs, which can influence several physiological processes including monoamine neurotransmission. Moreover, S1R activation promotes synaptic plasticity and neuronal survival through upregulation of BDNF expression and secretion as well as direct stimulation of TrkB receptors. S1R monomers are shown with the crystal structure adapted from Schmidt et al. (2016), but S1R ligand-dependent oligomerization/monomerization may confer specificity in its diverse actions.
Molecular Analysis of S1R
Sigma-1 receptor is a promising therapeutic target in the treatment of neurodegenerative diseases as it stabilizes the function of several intracellular systems through its role as a chaperone when activated by a variety of ligands with neuroprotective properties. Despite S1R pluripotency, relatively little is known regarding the mechanisms of receptor functioning and regulation at the molecular level. There is evidence that the structural organization of S1R and its conformational state are important determinants of S1R activity. However, the structural basis for S1R functionality remains poorly understood.
Originally, S1R was characterized as a type 1 transmembrane protein with only one transmembrane domain (Hanner et al., 1996). Hydrophobicity analysis confirmed a single-pass transmembrane topology of S1R (Kekuda et al., 1996), however, subsequent studies predicted a two transmembrane domain model of S1R topology (Aydar et al., 2002; Ortega-Roldan et al., 2013). For example, Aydar and colleagues proposed two-transmembrane domains (TM1 a.a. 11–29 and TM2 a.a. 80–100) based on antibody staining experiments with expression of S1R fused to GFP at the N- or C-terminus in Xenopus oocytes (Aydar et al., 2002). Immunolabeling of the GFP-tags was absent without membrane permeabilization, but was detected after permeabilization with 0.5% acetone, leading them to conclude that both the N- and the C-termini are located near the plasma membrane but within the cytoplasm. By contrast, the topological model proposed by Hayashi and Su situates S1R in ER membranes with both N- and C-terminal regions oriented to the ER lumen (Hayashi and Su, 2007). This was based on immunocytochemical staining of endogenous S1R in CHO cells with antibodies targeting N- and C-termini. Similar to results of Aydar et al. (2002), no labeling was detected without permeabilization, suggesting that S1R is not in the plasma membrane. Permeabilization of plasma and ER membranes with CHAPS or Triton X-100 enabled staining for all antibodies with a distribution similar to the shape of the ER. When the plasma membrane was permeabilized with streptolysin-O, staining was present only for the antibody targeting the loop domain. The discrepancy between the topology models of Aydar et al. (2002) and Hayashi and Su (2007) may have arisen from altered membrane insertion of GFP-fused S1R and/or cell type specific differences in S1R localization. For example, Hayashi and Su note that fusion of YFP to the C-terminus of S1R, but not the N-terminus, mirrors the distribution of endogenous S1R (Hayashi and Su, 2007).
The two-pass transmembrane model was widely accepted for a long time and has been used as a structural basis for molecular modeling and ligand docking studies (Laurini et al., 2011). However, the crystal structure for human S1R was recently solved revealing a single transmembrane domain structure (Schmidt et al., 2016). According to this study, a short N-terminus faces the ER lumen while the C-terminal domain of protein is oriented to the cytosolic side (Schmidt et al., 2016).
Adding another possible model of S1R topology to the mix, Mavylutov et al. (2018) fused ascorbate peroxidase 2 (APEX2) to the N- or C-terminus of S1R and used electron microscopy to visualize deposition of 3,3′-diaminobenzidine outside or inside of the ER of ND7/23 cells and dorsal root ganglion (DRG) neurons. This experiment indicated that the N-terminus of S1R faces the cytosol, whereas the C-terminus is located in the ER lumen. This is consistent with the one transmembrane model suggested by the crystal structure, but suggests the orientation of S1R positions the bulk of its structure in the lumen of the ER with only a short N-terminus facing the cytosol. All of the experiments probing the topology and orientation of S1R have caveats that are important to keep in mind including specificity of antibodies and membrane permeabilization as well as alterations to S1R from protein fusions and crystallization conditions.
Schmidt et al. (2016) determined the first crystal structure of full-length human S1R using X-ray analysis. They expressed FLAG-tagged S1R in Sf9 insect cells, purified it using the detergent lauryl maltose neopentyl glycol (LMNG) and crystallized it using the lipidic cubic phase (LCP) method (Caffrey et al., 2012). According to the crystal structure, S1R homomers consist of three protein subunits, with each protomer having one transmembrane domain (Figure 2A). The transmembrane alpha-helices of trimers (encompassing amino acid residues 8 to 32) are separated from each other and located in the corners of the complex, while the C-terminal cytosolic domains of each protomer organize the trimeric structure and form highly conserved ligand-binding sites. The membrane proximal surface of each C-terminal domain is tightly associated with the cytosolic surface of the ER membrane. Each C-terminal domain contains a cupin-like β-barrel that can envelop a ligand (Figure 2A).
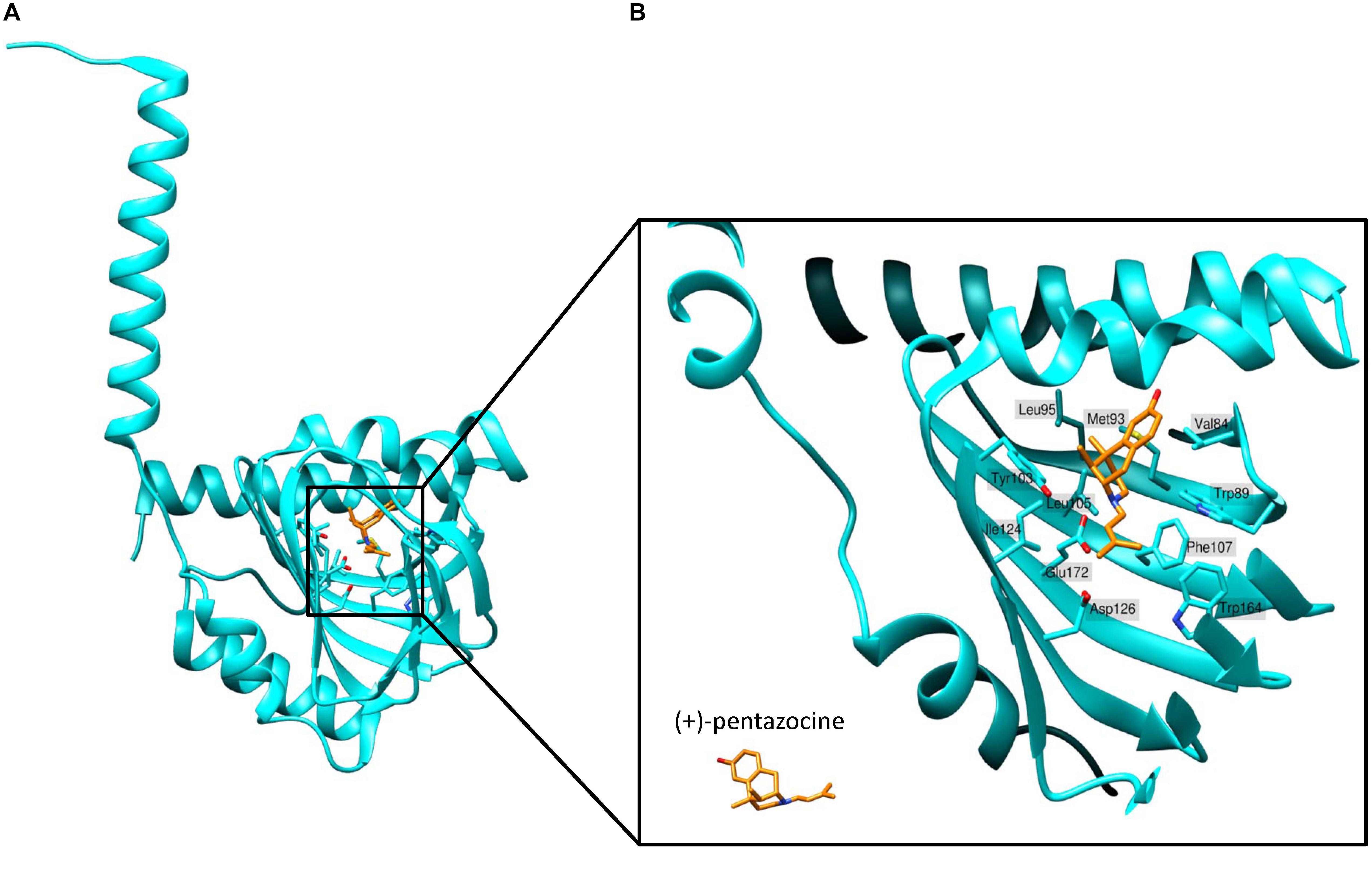
Figure 2. Structure of the ligand-binding site of S1R bound to agonist (+)-pentazocine. (A) The overall structure of a sigma-1 receptor subunit bound to (+)-pentazocine (PDB ID: 6DK1) based on (Schmidt et al., 2018). (B) A close up of the binding pocket showing the key amino acids involved in coordination of the ligand. (+)-pentazocine is shown in orange. Glu172 interacts with (+)-pentazocine’s nitrogen atom (blue) and both Tyr103 and Asp126 facilitate this through creating hydrogen bonds with Glu172. Other amino acids including Val84, Trp89, Met93, Leu95, Tyr103, Leu105, Phe107, Ile124, and Trp164 help to form the primarily hydrophobic binding pocket and stabilize the ligand through Van der Waals interactions.
An accurate model of the S1R ligand binding pocket is necessary for rational drug design aimed at the targeted treatment of neurodegenerative diseases. Mapping of the S1R ligand binding site was carried out in a large number of studies using mutational analysis and photoreactive probe labeling (Yamamoto et al., 1999; Chen et al., 2007; Pal et al., 2007, 2008). The first identified amino acids that are important for ligand binding include Ser99, Tyr103, Leu105, and Leu106 (Yamamoto et al., 1999). The results of these studies are highly consistent with the structural model of S1R obtained via X-ray crystal analysis.
Sigma-1 receptor crystal structures harboring chemically distinct ligands (the high-affinity, selective S1R ligands PD144418 and 4-IBP) show that both ligands bind in similar positions, forming electrostatic interactions with the highly conserved amino acid residue Glu172 (Figure 2B). The amino acid Asp126 which is also essential for ligand binding forms a hydrogen bond with Glu172 (Schmidt et al., 2016). With the exception of only two amino acid residues, the S1R active site is hydrophobic and is occluded from aqueous solution. Other amino residues involved in ligand coordination are as follows: Val84, Trp89, Met93, Leu95, Tyr103, Leu105, Phe107, Ile124, Trp164, and Leu182 (Figure 2B). Additionally, Tyr103 creates a hydrogen bond with Glu172, which is apparently necessary for the formation of the binding pocket (Figure 2B). Indeed, in earlier works a significant decrease in the binding activity of the mutant Tyr103Phe was shown (Yamamoto et al., 1999). The highly occluded structure of the binding pocket raises the questions about the pathway of ligand entry and explains the very slow ligand binding kinetics.
Schmidt et al. (2018) conducted additional structural studies and molecular dynamics (MD) simulation experiments to reconstruct the ligand binding mechanism in detail. They solved crystal structures of S1R bound to the classical antagonists haloperidol and NE-100 as well as the agonist (+)-pentazocine. The obtained structures were highly similar to each other and did not differ significantly from the previously determined trimeric structures of S1R. They share a similar organization of the ligand-binding pocket. The overall conformations of S1R in complex with the antagonists or agonist remain almost identical with the exception of a difference in the position of (+)-pentazocine in the ligand binding site. On the basis of structural data and MD simulations the authors suggest that agonist binding leads to conformational changes of S1R compared to the unliganded form of receptor or antagonist bound S1R. MD simulations were used to characterize conformational rearrangements occurring during ligand association. Ligand association was characterized as a two-step process: (1) the hydrogen bonds between Trp136 and Ala161 break, leading to receptor “lid” opening, and (2) the interior of the receptor separates, exposing the binding pocket and allowing ligand entry.
Schmidt et al. (2018) provided valuable insights on the structural basis for a ligand binding mechanism and describe the potential conformational differences between agonist and antagonist bound S1R. However, they do not explain the functional role of agonist/antagonist actions and the physiological relevance of agonist-induced structural rearrangements of S1R. The relationship between the ligand-receptor association and the subsequent biological response remains unclear.
Extensive evidence indicates that S1R exists in multiple oligomeric states (Chu et al., 2013; Gromek et al., 2014; Mishra et al., 2015) and recent studies suggest that ligand binding induces a shift in the oligomeric state of S1R (Gromek et al., 2014; Mishra et al., 2015; Hong W.C. et al., 2017), which could in turn lead to the various functional responses. For example, high-molecular weight forms of S1R have been detected in rat liver microsomal membranes using radioactive photosensitive labels (Pal et al., 2007). Oligomeric forms of S1R corresponding to hexamers, tetramers, octamers were also identified by size-exclusion chromatography (SEC) (Gromek et al., 2014). Analysis by SDS-PAGE after chemical crosslinking of individual oligomeric forms of MBP fused S1R has also confirmed the presence of monomers, tetramers and high molecular weight S1R oligomers (Mavlyutov et al., 2011). Cell-based fluorescence resonance energy transfer (FRET) studies have also confirmed the existence of several oligomeric states (Mishra et al., 2015) and revealed that agonists stabilize low-molecular-weight species, whereas antagonists favor oligomers. This model suggests that monomeric form of S1R is an “active” conformation involved in interactions with client proteins (Figure 3). On the other hand, Gromek et al. (2014) found similar stabilizing effects of agonists and antagonists on S1R oligomeric states. However, the detergents used in the purification procedure of their experiments do not reflect the native lipid environment of membrane proteins, potentially limiting the validity of this finding. While the crystal structure of S1R has a trimeric fold, size-exclusion, cross-linking and multi-angle light scattering (SEC-MALS) experiments have revealed a wide range of oligomeric states from 140 up to 400 kDa (Schmidt et al., 2016). Thus, it remains unclear which oligomeric form(s) of S1R exists in vivo and which state(s) is(are) functionally active.
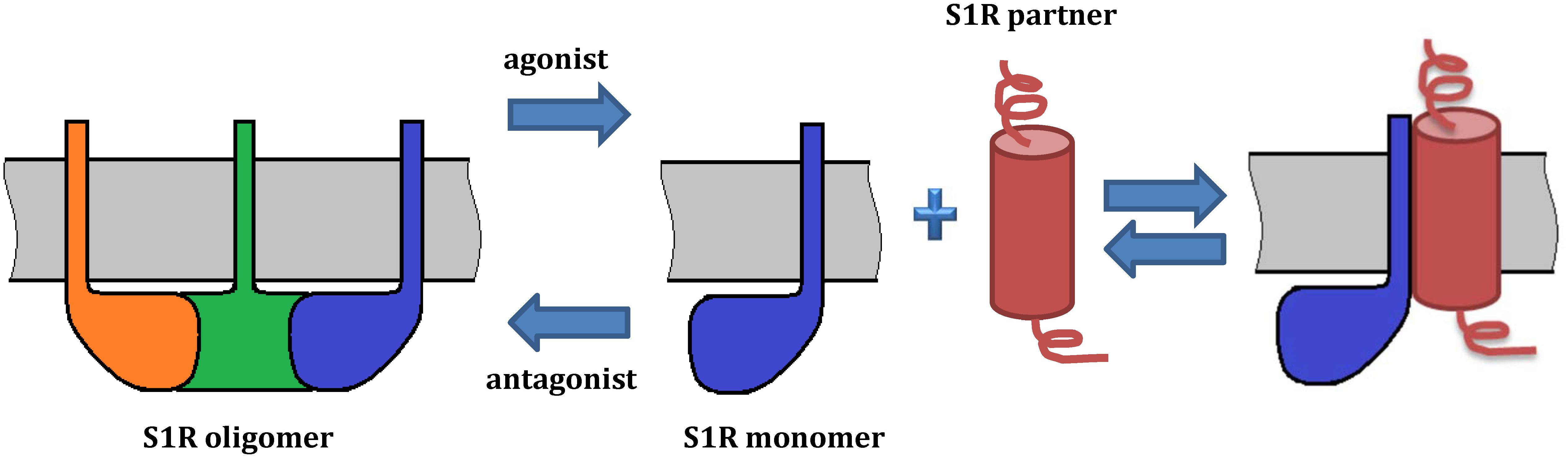
Figure 3. Model of S1R oligomerization and its functional role. The model is based on Mishra et al. (2015) and Hong W.C. et al. (2017). On the left, a S1R trimer is shown. Agonists promote dissociation of S1R into monomers, which may redistribute to other subcellular compartments and associate with client proteins. By contrast, antagonists prevent such interactions by stabilizing S1R oligomerization. Ligands regulate the interactions of S1R with its protein partners. While oligomeric forms of S1R have a demonstrated ability to bind ligands, S1R monomers may lose this property. As several oligomeric forms of S1R have been reported, they may also have functional roles and oligomer-specific interaction partners.
Sigma-1 receptor oligomerization is disrupted by mutations in the GXXXG motif corresponding to amino acid residues 87–91 (Gromek et al., 2014; Ortega-Roldan et al., 2015). The GXXXG motif was previously through to reside in the second transmembrane domain and mediate subunit association via transmembrane alpha helices. However, the crystal structure suggests that it forms a beta-hairpin structure inside the oligomerization interface (Schmidt et al., 2018). The distance between Cα atoms of Gly88 in each protomer is about 6 Å (Schmidt et al., 2018). Thus, mutations of this residue can sterically interfere with subunit association. Gromek et al. (2014) demonstrated that mutations within the GXXXG motif cause a shift toward the monomeric state of S1R. Interestingly, this is associated with a significant decrease in ligand binding, suggesting that ligand binding affinity may depend on S1R oligomerization processes. While oligomeric forms of S1R have a demonstrated ability to bind ligands, S1R monomers may lose this property (Gromek et al., 2014). Mutations within the GXXXG motif also decrease S1R expression, which may indicate reduced stability of GXXXG mutants (Gromek et al., 2014).
The oligomerization interface was further characterized with crystallographic data, leading to identification of key amino acids involved in subunit interactions. The sequence of the oligomerization interface is highly conserved among species, confirming its physiological importance. The trimerization surface is formed largely by hydrophobic residues within the C-terminal cupin domain. For example, a Phe191 residue from each protomer forms inter-subunit contacts. There are the polar interactions between sidechains of Thr141, His54, and Glu55. The amino acids Trp81, Phe83, Met90, Ala92 and Leu111, His116, Arg119, Trp136, Ala161, Trp169, Asp188, Phe191, Ser192, Gln194, Asp195, and Thr198 are also engaged in formation of the trimerization interface (Schmidt et al., 2016).
Despite the detailed characterization of oligomerization interface and comprehensive studies of ligand-induced structural rearrangements, the significance of S1R oligomer-monomer transitions in the regulation of S1R functions remains unknown. To correlate structural rearrangements observed in vitro with physiological responses, it has been proposed that the physiological significance of S1R oligomerization may be linked to the protein–protein interactions of S1R with its partners (Gromek et al., 2014; Yano et al., 2018). For example, the monomeric form of S1R interacts with Nav1.5, acid-sensing channels and D1R (Carnally et al., 2010; Navarro et al., 2010; Balasuriya et al., 2012). Two groups identified a direct and agonist-dependent interaction between S1R and the dopamine transporter (DAT), resulting in attenuated DA efflux and calcium signals evoked by methamphetamine (Hong W.C. et al., 2017; Sambo et al., 2017). Hong J. et al. (2017) suggested that agonists induce dissociation of S1R multimers into monomers which in turn interact with DAT. Mutational analyses have shown that the interaction site is located in the transmembrane domain of S1R.
As mentioned above, initiation of S1R activity was also proposed to involve ligand-, calcium-, or ER stress-dependent dissociation of S1R from binding immunoglobulin protein (BiP), which is a chaperone located in the lumen of the ER (Hayashi and Su, 2007). Recently, Yano et al. (2018) used a novel bioluminescence resonance energy transfer (BRET) assay to study the ligand-mediated oligomerization of S1R. They revealed the distinct effects of agonists and antagonists on S1R homomerization, consistent with previous results (Hong W.C. et al., 2017). Interestingly, while the agonist pentazocine facilitated interaction of BiP and S1R, haloperidol induced the dissociation of S1R from BiP. Thus, S1R ligands may regulate the association between S1R and BiP through controlling S1R oligomerization and monomerization. This is likely also relevant to S1R interactions with its client proteins (Figure 3).
It would be interesting to know more clearly how S1R associates with various proteins located in the ER lumen, ER membrane, cytoplasm and plasma membrane and to resolve the conflicting models of S1R topology and orientation. Given the topology model proposed by Mavylutov et al. (2018), the bulk of S1R may face the ER lumen. This topology is consistent with the well-described interaction of S1R with BiP, but raises it questions about how S1R interacts with proteins in the cytosol with only a small cytosolic N-terminal tail. Perhaps S1R has two or more structural elements or configurations responsible for the binding of S1R to different proteins. The structural and biological mechanisms of such interactions remain to be fully elucidated.
S1R as a Target for Treating Neurodegenerative Diseases
Many S1R agonists are anti-amnestic, synaptogenetic, and neuroprotective in conditions of neuronal stress (Antonini et al., 2009; Hindmarch and Hashimoto, 2010; Ruscher et al., 2011; Bolshakova et al., 2016). They also mitigate disease and symptoms in experimental models of ALS (Mancuso et al., 2012; Ono et al., 2014; Peviani et al., 2014; Ionescu et al., 2019), Alzheimer’s disease (AD) (Meunier and Hayashi, 2010; Fisher et al., 2016; Maurice and Goguadze, 2017; Hall et al., 2018; Goguadze et al., 2019; Ryskamp et al., 2019), Parkinson’s disease (PD) (Francardo et al., 2014; Francardo et al., 2019) Huntington’s disease (HD) (Squitieri et al., 2015; Geva et al., 2016; Bol’shakova et al., 2017; Garcia-Miralles et al., 2017; Ryskamp et al., 2017; Kusko et al., 2018) stroke (Allahtavakoli and Jarrott, 2011; Ruscher et al., 2011, 2012; Sato et al., 2014; Urfer et al., 2014) and traumatic brain injury (Dong et al., 2016). By contrast, S1R deficiency exacerbates progression of neurological disorders (Mavlyutov et al., 2011, 2013; Ha et al., 2012; Francardo et al., 2014; Miki et al., 2015; Maurice et al., 2018) as well as symptoms commonly associated with neurodegenerative diseases. For example, pharmacological inhibition of S1R leads to mushroom spine loss in hippocampal cultures (Ryskamp et al., 2019) and this could be related to memory impairments from the anti-psychotic drug and S1R antagonist haloperidol (KD for S1R ∼3 nM) (Abdel-Salam et al., 2012). S1R knockout (KO) mice have several phenotypes resulting from neuronal dysfunction and late-onset neurodegeneration (Sabino et al., 2009; Ha et al., 2011; Sha et al., 2015). These data collectively highlight the innate, neuroprotective properties of S1R activity. The following sections summarize genetic associations of S1R mutations/polymorphisms with neurodegenerative diseases, examples of neuroprotection in respective disorders by S1R agonists, and possible mechanisms of action.
S1R in Amyotrophic Lateral Sclerosis/Frontotemporal Dementia (ALS/FTD)
Amyotrophic lateral sclerosis is a fatal neurodegenerative disease featuring progressive weakness of skeletal muscles due to upper and/or lower motor neuron dysfunction and loss. Several recessive, loss-of-function mutations in S1R have been associated with ALS, distal hereditary motor neuropathy and/or FTD (Luty et al., 2010; Al-Saif et al., 2011; Kim et al., 2014; Li et al., 2015; Ullah et al., 2015; Gregianin et al., 2016; Horga et al., 2016; Watanabe et al., 2016). S1R is highly expressed in motor neurons (Gundlach et al., 1986; Mavlyutov et al., 2010), suggesting a possible cell autonomous mechanism of motor neuron degeneration in these patients. Although S1R KO mice do not develop an overt ALS phenotype (Langa et al., 2003), they have deficits in locomotion and motor performance (Mavlyutov et al., 2010) related to muscle weakness, axonal degeneration, and motor neuron loss (Bernard-Marissal et al., 2015). KO of S1R accelerates the onset and progression of ALS in the SOD1G93A mouse model of ALS (Mavlyutov et al., 2013), whereas the S1R agonists PRE-084 and SA4503 are neuroprotective and extend survival of SOD1G93A mice (Mancuso et al., 2012; Ono et al., 2014). PRE-084 is also protective in wobbler mice, which develop spontaneous motor neuron degeneration (Peviani et al., 2014). PRE-084 is a derivative of phencyclidine (PCP) with nanomolar affinity for S1R and negligible affinity for PCP receptors and GPCRs (Su et al., 1991). SA4503 has low nanomolar affinity for S1R, low micromolar affinity for S2R, and little affinity for 36 other receptors, ion channels and second messenger systems (Matsuno et al., 1996). Treatment of SOD1G93A mice with the S1R agonist pridopidine improves axonal transport (e.g., of BDNF, GDNF, and mitochondria) and BDNF secretion while attenuating atrophy of neuromuscular junctions, muscle fibers and motor neurons (Ionescu et al., 2019) (pharmacological properties summarized in HD section). Pridopidine treatment reduces the prevalence of SOD1 aggregates in spinal cords of SOD1G93A mice (Ionescu et al., 2019). S1R activity may additionally protect motor neurons by reducing their excitability through facilitation of potassium channel activity (Mavlyutov et al., 2015b). Motor neuron degeneration from the absence of S1R is associated with reduced contacts between mitochondria and ER, ER stress, calcium dysregulation (Bernard-Marissal et al., 2015) and this may help to explain pathology in ALS patients with mutations in S1R.
Role of S1R in Parkinson’s Disease (PD)
Dopamine receptors play important roles in learning and memory, motivation and movement and S1R agonists modulate dopaminergic signaling through multiple mechanisms. This has primarily been studied in the context of psychostimulant research, but these results may be important for understanding regulation of dopamine neurotransmission and its dysregulation in HD and PD. S1R appears to differentially regulate dopamine D1 and D2 receptors, as S1R activation by cocaine inhibits D2R (Navarro et al., 2013) and prevents histamine H3 receptor-dependent inhibition of the dopamine D1 receptor, stimulating Gs, recruitment of β-arrestin and phosphorylation of ERK1/2 (Moreno et al., 2014). Although S1R activation does not affect basal dopamine neurotransmission, it attenuates methamphetamine-induced and DAT-dependent increases in firing of dopamine neurons (Sambo et al., 2017). It also interacts directly with the DAT and attenuates calcium signals evoked by methamphetamine (Sambo et al., 2017). As a result, S1R limits hyperactivity, motivated behavior and reinforcement from methamphetamine (Sambo et al., 2017).
Abnormalities in movement and cognition in PD result from degeneration of dopaminergic neurons projecting from the substantia nigra to the striatum. S1R is expressed in these neurons (Hong W.C. et al., 2017) and it can bidirectionally modulate NMDAR-dependent release of dopamine in striatal brain slice experiments (Gonzalez-Alvear and Werling, 1994). S1R may be decreased in striatal regions that are preferentially affected in PD (Mishina et al., 2005), which could contribute to neuropathology as indicated by studies with S1R KO mice. Similar to PD patients, S1R KO mice have age-related deficits in motor behavior and degeneration of dopaminergic neurons (Hong W.C. et al., 2017). This appears to be related to aggregation and phosphorylation of α-synuclein which may be driven by phosphorylation of eIF2α from ER stress and proteasomal dysfunction (Hong W.C. et al., 2017). Pharmacological inhibition of ER stress prevented oligomerization of α-synuclein, dopaminergic neuron loss and motor impairments in S1R KO mice (Hong W.C. et al., 2017).
Recent studies found that S1R agonists are protective in PD models. For example, chronic treatment with PRE-084 gradually improves PD-like motor deficits from unilateral intrastriatal 6-hydroxydopamine (6-OHDA) lesions (hemiparkinsonian model) when treatment onset was prompt (Francardo et al., 2014). This treatment suppressed neuroinflammation while increasing levels of neurotrophic factors, monoamines (e.g., dopamine and serotonin), dopaminergic innervation of the striatum, and nigral neuron survival (Francardo et al., 2014). Low dose pridopidine treatment (0.3 mg/kg) of unilaterally 6-OHDA-lesioned mice partially protected nigral dopaminergic cell bodies and increased dopaminergic fiber density in the motor striatum (Francardo et al., 2019). This was associated with a gradual restoration of forelimb use (cylinder test, stepping test) and prevention of rotational bias toward the ipsilateral side (Francardo et al., 2019). The delayed recovery of motor function corresponds roughly with the expected timeline of pridopidine-dependent dopaminergic axon sprouting (Francardo et al., 2019). Treatment efficacy was absent in S1R KO mice, which had reduced loss of dopaminergic neurons in the substantia nigra pars compacta, but a greater loss of dopaminergic fibers in the striatum compared to wild-type mice (Francardo et al., 2019). The increased vulnerability of S1R knockout mice to axonal degeneration in the nigrostriatal pathway could relate S1R’s ability to promote growth and repair of neurites (Francardo et al., 2019). The neuroresorative effects of pridopidine were associated with upregulation of neurotrophic factors (BDNF, GDNF, pERK1/2) and associated signaling in the striatum and substantia nigra as well as reduced microglial activation (Francardo et al., 2019).
Role of S1R in Huntington’s Disease (HD)
Huntington’s disease (HD) patients suffer from psychiatric, motor and cognitive disturbances that gradually worsen, leading to dementia, cachexia and eventually death. HD is a dominantly inherited neurodegenerative disease resulting from a CAG trinucleotide repeat expansion in exon 1 of the Huntingtin gene (>35 CAG repeats), leading to expression of mutant Huntingtin (mHtt) protein with an elongated polyglutamine tract. mHtt is broadly expressed throughout the body, but striatal MSNs are preferentially vulnerable in HD. The most significant contributions of mHtt to HD pathology remains a matter of debate and intense investigation. The CAG expansion compromises normal functions of Htt and disrupts cellular functioning through gain of mHtt function mechanisms (Imarisio et al., 2008; Kim et al., 2009), with possible toxic contributions from repeat-associated non-AUG translation (Banez-Coronel et al., 2015). This results in oxidative damage, glial reactivity, altered intracellular signaling, metabolism and energy levels, impaired axonal transport, transcriptional dysregulation, aberrant calcium regulation associated with ER stress, synapse loss and excitotoxicity (Zhai et al., 2005; Mochel and Haller, 2011; Leitman et al., 2013; Ryskamp et al., 2017).
Initial studies on the potential role of S1R in HD pathology were carried out with cellular models of HD. Hyrskyluoto et al. (2013), found that expression of mHtt (N-terminal huntingtin fragment proteins with 120 polyQ repeats or full-length Htt protein with 75 repeats) downregulates S1R expression in neuronal PC6.3 cells. There were no differences in S1R expression in control cells expressing the N-terminal fragment of Htt with 18 polyQ repeats or wild-type Htt. Administration of the selective S1R agonist PRE-084 prevented mHtt-dependent downregulation of S1R, SOD1, SOD2, thioredoxin 2, and Bcl-XL in neuronal PC6.3 cells (Hyrskyluoto et al., 2013). However, S1R expression appears to be upregulated in the striatum of YAC128 HD mice at 12 months of age and in the striatum of patients with advanced HD, possibly as an effort to compensate for ER calcium dysregulation and stress (Ryskamp et al., 2017). PRE-084 also decreased caspase-3 cleavage and oxidative stress and upregulated calpastatin, NF-κB-p65 levels and NF-κB signaling in mHtt expressing PC6.3 cells, enhancing their viability (Hyrskyluoto et al., 2013). Hyrskyluoto et al. (2013) proposed that the neuroprotective properties of S1R activity involved modulation of the calpastatin/calpain system, increasing NF-κB signaling and thereby upregulating antioxidants and decreasing ROS levels.
Another study demonstrated that large neuronal nuclear inclusions were strongly positive for S1R in human brains affected by polyglutamine diseases and intranuclear inclusion body disease (Miki et al., 2014). Also, S1R immunostaining colocalized with most intranuclear mHtt aggregates in HeLa cells expressing the N-terminal fragment of mHtt. Downregulation of S1R with antisense RNA increased the amount of mHtt aggregates in both the cytoplasm and nucleus. This was reproduced by treatment with the proteasome inhibitor epoxomicin. Moreover, proteasome activity was significantly lower following knockdown of S1R.
Pridopidine’s Mechanism of Action in the Treatment of HD
Clinical trials with pridopidine indicate that it has efficacy in treating motor symptoms of HD (Lundin et al., 2010; De Yebenes et al., 2011; Esmaeilzadeh et al., 2011; Huntington, 2013; Reilmann et al., 2019) and recent evidence suggests that the therapeutic effect of pridopidine involves S1R. Pridopidine was originally dubbed a “dopamine stabilizer” based on behavioral experiments showing that it can both decrease locomotion in hyperactive rodents (e.g., from D-amphetamine or MK-801) and increase locomotion in hypoactive rodents (e.g., animals that have habituated to their environment or co-treated with the VMAT inhibitor tetrabenazine) (Ponten et al., 2010; Waters et al., 2014; Sahlholm et al., 2015). Increased locomotion in hypoactive rodents may relate to pridopidine’s ability to increase dopamine and norepinephrine in the cortex and subcortical areas (Ponten et al., 2010), which may also explain increased firing in prefrontal pyramidal neurons (Gronier et al., 2013). Pridopidine’s ability to bidirectionally normalize activity levels may have particular utility in the treatment of HD in which patients develop hyperkinetic motor disturbances followed by hypoactivity later in disease. The mechanism of action was initially proposed to involve low-affinity/fast-off negative modulation of dopamine D2 receptors with a slight binding preference for the agonist binding site when the receptor is in the active, catalytic, high-affinity state (Nilsson et al., 2004; Rung et al., 2008; Waters et al., 2014), but the affinity of pridopidine for D2 receptors of dopamine is relatively low being in the micromolar range (Dyhring et al., 2010). Unlike classical D2 receptor antagonists, pridopidine does not induce hypoactivity or catalepsy (Waters et al., 2018). Pridopidine also has micomolar affinity for several additional GPCRs including adrenergic alpha 2A/C receptors, serotonergic 5HT1A and 5HT2A receptors, and histamine H3 receptors (Gronier et al., 2013) and these interactions may influence levels of extracellular monoamines and glutamatergic neurotransmission (Waters et al., 2014). The effects of pridopidine in behavioral assays are not fully blocked in D2R receptor knockout mice (Svensson et al., 2009), prompting further investigation into potential molecular targets of pridopidine. More recently, pridopidine was found to have a high affinity (kD = ∼80 nM) for S1R (Sahlholm et al., 2013) and it primarily binds S1R rather than D2 receptors in vivo at behaviorally relevant doses (Sahlholm et al., 2015), suggesting that S1R might mediate the therapeutic effects of pridopidine.
Recent studies show that activation of S1R by pridopidine might be disease-modifying in HD. Squitieri et al. (2015) found that pridopidine reduces motor symptoms of R6/2 mice, improving performance on the horizontal ladder task and open-field locomotor measurements when treatment was started presymptomatically (5–6 mg/kg via daily intraperitoneal injections) (Squitieri et al., 2015). Pridopidine also extends their lifespan (Squitieri et al., 2015). In vivo treatment also normalized striatal BDNF and DARPP32 levels (Squitieri et al., 2015; Geva et al., 2016; Garcia-Miralles et al., 2017; Kusko et al., 2018), while decreasing the size and amount of mHtt aggregates (Squitieri et al., 2015). 150 μM pridopidine reduced apoptosis and restored pERK1/2 levels in a mouse striatal knock-in cellular HD model (STHdh111/111) and these effects were blocked by the S1R antagonist NE-100 (Squitieri et al., 2015). Ryskamp et al. (2017) found that low nanomolar concentrations of pridopidine and the structurally similar S1R agonist (+)3-PPP are neuroprotective in another cellular model of HD (Ryskamp et al., 2017). Both compounds stabilized synaptic connections between cortical and striatal MSNs in primary corticostriatal co-cultures prepared from from YAC128 HD mouse pups. Deletion of S1R with Cas9 prevented pridopidine and 3-PPP from rescuing dendritic spine loss in HD MSNs. Interestingly, S1R deletion also resulted in significant spine loss in WT MSNs. This observation indicated an important role for S1R in maintaining MSN spine stability. A synaptoprotective action of pridopidine was further supported by a series of Ca2+ imaging experiments. Previous studies demonstrated that abnormal Ca2+ signaling in post-synaptic spines is responsible for their destabilization in HD MSNs (Wu et al., 2016, 2018). Decreased ER Ca2+ levels due to mHtt-induced InsP3R1 hyperactivity (Tang et al., 2003, 2009) increases neuronal store-operated calcium entry (nSOC) in HD MSNs to synaptotoxic levels (Wu et al., 2011, 2016, 2018). Pridopidine treatment of corticostriatal co-cultures from YAC128 mice prevented InsP3R1 hyperactivity, restored ER Ca2+ levels, and decreased nSOC in HD MSNs (Ryskamp et al., 2017). Deletion of S1R WT MSNs resulted in depletion of ER Ca2+ content, suggesting that it might stabilize MSN spines through homeostatic control of ER Ca2+ levels (Ryskamp et al., 2017). Deletion of S1R in HD MSNs prevented the normalization of ER Ca2+ by pridopidine (Ryskamp et al., 2017). The selective S1R agonist PRE-084 also prevents dendritic spine loss in HD MSNs and this rescue is blunted by the S1R antagonist NE-100 (Bol’shakova et al., 2017). These findings suggest that in addition to the ability of pridopidine to mitigate motor symptoms of HD, it may also foster synaptic and neuronal viability via activation of S1R.
Consistent with this, Eddings et al. (2019) found that pridopidine and 3-PPP protects mouse primary striatal and cortical neurons from expression of mHtt (22 vs. 58 CAG repeats with the first 586 amino acids of Htt), as measured by imaging nuclear condensation in apoptotic cells and neuronal morphology. Pridopidine also rescued HD patient-derived induced pluripotent stem cells (Eddings et al., 2019). The S1R antagonist NE-100 or genetic ablation of S1R blocked the neuroprotective effects (Eddings et al., 2019). Although BDNF was also protective and is upregulated by S1R stimulation, blockade of BDNF signaling with the TrkB receptor antagonist ANA-12 did not impede the neuroprotective effects of pridopidine (Eddings et al., 2019). However, ANA-12, like NE-100, suppressed pridopidine’s ability to prevent mitochondrial depolarization from mHtt, as measured using tetramethyl rhodamine methyl ester (TMRM) (Eddings et al., 2019). These data indicate that S1R activation by pridopidine or 3-PPP is neuroprotective, but neuroprotection is not entirely mediated by BDNF signaling.
Pridopidine activates neuroprotective pathways that are compromised in HD (e.g., BDNF and AKT pathways), improving behavioral and transcriptional deficits in mouse models of HD (Geva et al., 2016; Garcia-Miralles et al., 2017; Kusko et al., 2018). Consistent with its ability to promote neuronal plasticity and survival, pridopidine upregulates expression BDNF, dopamine D1 receptor, AKT/PI3K and glucocorticoid pathway components and stimulates BDNF secretion in an S1R-dependent fashion (Geva et al., 2016; Kusko et al., 2018). Microarray and qPCR studies showed that pridopidine upregulates several genes downstream of BDNF including EGR1, EGR2, KLF5, CDKN1A, Homer1a, and Arc (Geva et al., 2016; Kusko et al., 2018). BDNF overexpression is sufficient to rescue many phenotypic characteristics of YAC128 HD mice (e.g., motor performance, cognitive deficits, synaptic density) (Xie et al., 2010), further suggesting that BDNF signaling could be an important contributor to neuroprotection following S1R activation. The idea that some of the beneficial effects of pridopidine in HD models can be mediated through BDNF signaling was supported by recent experimental evidence from Smith-Dijak et al. (2019). Synaptic scaling was suppressed in YAC128 cultures, as determined by recording the amplitude and frequency of mEPSCs after blockade of activity-dependent neurotransmission with TTX. Synaptic scaling was restored in YAC128 neurons by pharmacological activation of S1R with pridopidine or 3-PPP through BDNF-TrkB signaling (Smith-Dijak et al., 2019). Given that AKT is a potent pro-survival kinase, its upregulation may help promote neuronal resilience by phosphorylating apoptotic proteins (e.g., BAD and GSK3) and forkhead family transcription factors (e.g., FOXOs) (Geva et al., 2016; Kusko et al., 2018). Also, the calcium regulating genes calbindin and Homer1a are downregulated in the striatum of Q175 and YAC128 HD mice and they are both upregulated by pridopidine treatment (Ryskamp et al., 2017). These results indicate that when activated by pridopidine S1R acts on several transcriptional networks to foster neuronal function and survival in HD models.
A recent study demonstrated that pridopidine improves motor performance in YAC128 HD mice as well as anxiety- and depressive-like phenotypes, but it was unable to prevent striatal and corpus callosum atrophy (Garcia-Miralles et al., 2017), indicating that S1R agonism is insufficient to completely mitigate HD neuropathology and complementary treatment strategies should be considered. While pridopidine might be insufficient to completely prevent HD progression, when taken together the data on its effects in HD models and in HD patients shows that it can mitigate symptoms and is likely has the capacity to modify disease.
Role of S1R in Alzheimer’s Disease (AD)
Alzheimer’s disease (AD) is the most pervasive cause of dementia in elderly people and it involves progressive impairment of memory and other cognitive faculties from damage to the hippocampus and other parts of the brain. Age is the main risk factor for the sporadic form of the disease. Early onset of AD is characterized by the development of the disease before the age of 65 and most of these cases result from autosomal dominant inherited mutations in amyloid precursor protein (APP), presenilin-1 (PSEN1) or presenilin-2 protein (PSEN2). Autosomal dominant inheritance accounts for about 1% of all cases of AD. When APP is cleaved by β- and γ-secretases, Aβ is formed with a length of 39 to 42 amino acid residues (Hardy, 2009). Presenilins are part of the γ-secretase protease complex and are key catalytic subunits. In AD mutations in the APP and PSEN1, PSEN2 genes promote the formation of an extracellular fragment of Aβ with a length of 42 amino acid residues (Aβ42), the accumulation of which contributes to the formation of amyloid oligomers. Recently, it has been demonstrated that Aβ is generated intracellularly at the MAM domain and may influence functioning of the ER, mitochondria, and MAM (Schreiner et al., 2015). Given this finding and the importance of S1R at MAM domains (Hayashi and Su, 2007; Watanabe et al., 2016), it is perhaps not surprising that common S1R polymorphisms influence risk of developing AD (Uchida et al., 2005; Maruszak et al., 2007; Fehér et al., 2012). In fact, certain genetic combinations of S1R and apolipoprotein E (APOE) genotypes synergistically increase the risk of AD (Huang et al., 2011).
Several S1R agonists have anti-amnestic properties, overcoming learning and memory impairments from amyloid-β toxicity or scopolamine (Maurice and Goguadze, 2017). S1R agonists promote neurogenesis in the hippocampus (Moriguchi et al., 2013) and they may mitigate memory impairment because they can stabilize mature, mushroom spines (Ryskamp et al., 2019), which serve as sites of robust synaptic connections encoding lasting information (Bourne and Harris, 2007; Hayashi-Takagi et al., 2015). Mushroom spine loss may underlie memory defects in models of AD, as hippocampal neuron mushroom spines are lost in vitro and in vivo in both presenilin-1-M146V knock-in (PS1-KI) and APP knockin (APP-KI) models of AD (Sun et al., 2014; Zhang et al., 2015). As postmortem and in vivo brain imaging studies have demonstrated a reduced density of S1R in the brains of patients with AD (Jansen et al., 1993; Mishina et al., 2008) and S1R knockdown destabilizes mushroom spines (Tsai et al., 2009; Fisher et al., 2016; Ryskamp et al., 2019), downregulation of S1R may contribute to AD pathology. Consistent with this, knockout of S1R in APPSwe AD mice increases oxidative stress within the hippocampus and exacerbates memory impairments (Maurice and Goguadze, 2017; Maurice et al., 2018). The novel positive S1R modulator (±)-2-(3-chlorophenyl)-3,3,5,5-tetramethyl-2-oxo-oxazaphosphinane (OZP002) (Gundlach et al., 1986; Maurice et al., 2006a; Tsai et al., 2009) was also neuroprotective in pharmacological and genetic models of AD. It potentiated the antidepressant effect of the S1R agonist igmesine and prevented scopolamine-induced learning deficits in the Y maze test and passive avoidance test. Its effect was blocked by NE-100 or in S1R knockout mice (Maurice et al., 2019).
Treatment of hippocampal cultures with Aβ42 oligomers induces loss of mushroom spines (Popugaeva et al., 2015; Zhang et al., 2015) and Aβ42 accumulation in hippocampal cultures prepared from APP knock-in mice also causes mushroom spine loss (Zhang et al., 2015). This is also observed in vivo (Zhang et al., 2015). Pridopidine and 3-PPP prevent mushroom spine loss from both of these sources of Aβ toxicity in hippocampal neuronal cultures (Ryskamp et al., 2019). Pridopidine treatment normalized synaptic functioning, preventing LTP deficits caused by Aβ42 oligomers (Ryskamp et al., 2019). Pridopidine and 3-PPP also prevented mushroom spine loss in hippocampal cultures prepared from PS1-KI mice (Ryskamp et al., 2019) that model familial AD (Guo et al., 1999). Importantly, oral treatment with pridopidine rescued mushroom spines in vivo in PS1-KI mice (Ryskamp et al., 2019), suggesting this as a viable treatment strategy for memory deficits in familial AD.
AF710B also stabilized mushroom spines in vitro in hippocampal cultures prepared from AD mice (PS1-KI and APP-KI models) (Fisher et al., 2016). AF710B was found to potently and selectively stimulate the M1 muscarinic acetylcholine receptor (M1R) and S1R (Fisher et al., 2016). AF710B binds to M1R outside of its orthosteric binding site, suggesting an allosteric mechanism of action (Fisher et al., 2016). This is supported by data showing that 0.1 nM AF710B enhances the affinity and potency of the M1R agonist carbachol (Fisher et al., 2016). The mechanism by which AF710B activates S1R is less clear, but the anti-amnestic properties of AF710B appear to require S1R, because the S1R antagonist NE-100 can suppress them (Fisher et al., 2016). No significant binding was observed with other targets in a screen involving 83 GPCRs, ion channels and transporters (Fisher et al., 2016). Treatment of 3xTg-AD mice with AF710B (10 μg/kg delivered by intraperitoneal injections daily for 2 months) reduced levels of BACE1, Aβ1–42, plaques, p25/CDK5, GSK-3β activity, Tau phosphorylation and memory deficits in the Morris water-maze (Fisher et al., 2016). It was previously known that M1R activation improves cognition and reduces AD-like pathology in animal models (Caccamo et al., 2006; Medeiros et al., 2011), but the combined activity of AF710B at both M1R and S1R might make it particularly therapeutic. Indeed, in vivo treatment of McGill-R-Thy1-APP transgenic rats also reduced amyloid burden and inflammation while enhancing synaptogenesis and cognition (Fisher et al., 2016). Another mixed muscarinic/σ1R agonist, ANAVEX2-73, was able to mitigate Aβ25–35-induced tau phosphorylation and Aβ1–42 seeding in mice (Lahmy et al., 2013) and may help to preserve cognition in preliminary clinical trials clinical studies with AD patients (Macfarlane et al., 2016).
In addition to S1R agonists, positive allosteric modulators that do not compete with the (+)-pentazocine binding site might have therapeutic value. For example, SKF-83959 shows promise in the 6-OHDA-induced rat model of Parkinson’s disease (Zhang et al., 2005, 2007; Guo et al., 2013). Also, OZP002 attenuated learning deficits from scopolamine, ICV injection of amyloid Aβ25–35, or the APPSwe transgene and protected against neurotoxicity associated with ICV injection of amyloid Aβ25–35 (Vavers et al., 2019). Several selective allosteric modulators for S1R have been discovered (methylphenylpiracetam and SOMCL-668) (see Vavers et al., 2019 for a review) and it will be interesting to see whether they are efficacious in models of neurodegenerative diseases. Hinting at potential utility, SOMCL-668 enhanced (+)-SKF-10047-stimulated neurite growth and BDNF production in an S1R-dependent manner (Wang et al., 2016). Although both direct agonists and positive allosteric modulators may have therapeutic promise, it is unclear which are better candidates for clinical trials.
Recent data suggests that S1R functionally interacts with presenilin 1 (PS1) and presenilin 2 (PS2), which are implicated in AD. Although cleaved PS1 is the catalytic subunit in the γ-secretase complex, the holoprotein version of PS1 functions as a passive leak channel in the ER membrane (Tu et al., 2006; Nelson et al., 2007; Zhang et al., 2010). Similarly, PS2 forms a calcium leak channel in the ER (Tu et al., 2006; Nelson et al., 2007; Zhang et al., 2010). Many familial AD-causing mutations in either PS1 or PS2 disrupt tonic Ca2+ release from the ER via PS1 and PS2 leak channels, increasing the concentration of calcium in the ER (Tu et al., 2006; Zhang et al., 2010). Pridopidine promotes ER calcium homeostasis by decreasing luminal calcium levels in cultured hippocampal neurons from WT, PS1-KI and conditional presenilin double-knockout mice (PS1flox/flox/PS2–/–) infected with lenti-NLS-GFP as well as in neurons expression Cas9 and gRNA targeting the PS1 gene (Ryskamp et al., 2019). However, this effect is lost in PS1flox/flox/PS2–/– hippocampal neurons infected with lenti-NLS-GFP-Cre (Ryskamp et al., 2019). Knockout of PS1, PS2, or PS1/2 causes mushroom spine loss in hippocampal neurons (Ryskamp et al., 2019). Consistent with functional data, pridopidine can compensate for this and restore mushroom spine integrity in presenilin 1 or presenilin 2 knockout neurons, but not in PS1/2 double knockout neurons (Ryskamp et al., 2019). Consistent with the spine loss phenotype in PS1 and/or PS2 KO neurons, inactivation of PS1 in the mouse forebrain causes mild cognitive deficits, whereas inactivation of both PS1 and PS2 severely impacts cognition and synaptic plasticity, leading to neurodegeneration (Sun et al., 2005). PS1 and PS2 both contribute to resting ER calcium homeostasis and form a redundant ER calcium leak pathway (Tu et al., 2006; Nelson et al., 2007) that is required for pridopidine to decrease the concentration of ER calcium and stabilize mushroom spines (Ryskamp et al., 2019). The reason for this is unclear, but S1R agonists might decrease ER calcium in hippocampal neurons by enhancing ER calcium leakage either by modulating leak activity of PS channels or increasing the prevalence of PS holoprotein in the ER membrane. The later possibility is intriguing because S1R might impede catalytic cleavage of PS1, reducing its incorporation into the γ-secretase complex. This could underlie the ability of S1R agonists to reduce Aβ42 accumulation and aggregation (Fisher et al., 2016). However, more work is needed to characterize how S1R activity normalizes ER calcium homeostasis and mushroom spine prevalence while mitigating other hallmarks of AD neuropathology.
When ER calcium levels are chronically elevated from mutations in presenilin 1, suppression of neuronal store-operated calcium entry (nSOC) leads to destabilization of hippocampal mushroom spines (Sun et al., 2014; Zhang et al., 2016). Upregulating nSOC either pharmacologically or via overexpression of STIM2 or EB3 prevents PS1-KI mushroom spine loss (Sun et al., 2014; Zhang et al., 2016; Pchitskaya et al., 2017). Consistent with pridopidine’s ability to decrease ER calcium levels in hippocampal neurons, it increased nSOC in the spines of cultured PS1-KI neurons and activity of the nSOC pathway was required for the rescue of PS1-KI mushroom spines (Ryskamp et al., 2019). This suggests that pridopidine rescues PS1-KI mushroom spines through decreasing ER calcium levels and thereby stimulating nSOC pathway activity.
Highlighting the importance of the client protein milieu in determining the effects of S1R activity, S1R suppresses store-operated calcium entry (SOC) in other cell types outside of the hippocampus. In non-neuronal cells, treatment with S1R agonists or overexpression of S1R or suppresses SOC (Brailoiu et al., 2016; Srivats et al., 2016). This may involve S1R binding to STIM1 and disrupting the interaction of STIM1 and Orai1 (Srivats et al., 2016). Additionally, in MSNs from YAC128 mice that model Huntington’s disease pridopidine decreases supranormal nSOC, which is synaptotoxic to MSNs (Ryskamp et al., 2017). This contrasts with data involving hippocampal neurons in which pridopidine decreased ER calcium levels and enhanced nSOC (Ryskamp et al., 2019). This divergence indicates that the effect of S1R in a given cell type depends on the availability of S1R interaction partners. For instance, InsP3R1 constitutes the primary ER calcium release pathway in MSNs (Wu et al., 2016), whereas presenilins preferentially mediate leakage of ER calcium in hippocampal neurons (Tu et al., 2006; Nelson et al., 2007; Zhang et al., 2010). Additionally, although S1R suppresses STIM1-dependent SOC in HEK293 and CHO cells (Srivats et al., 2016), STIM2 is the predominant regulator of nSOC in hippocampal neurons (Sun et al., 2014). S1R might not bind and sequester STIM2 the way it does with STIM1 or if S1R does bind STIM2, it might enhance or minimally effect STIM2-gated nSOC. Thus, regulation of synaptic plasticity by S1R is likely to be multifaceted and highly dependent on the cellular context.
Conclusion
Sigma-1 receptor is incredibly versatile in its ability to foster neuronal homeostasis in the context of several neurodegenerative disorders. Several S1R agonists are FDA-approved (Ishikawa and Hashimoto, 2009), such as fluvoxamine (Nishimura et al., 2008) and donepezil (Maurice et al., 2006b) and they may be repurposed for the treatment of several neurodegenerative diseases. Additional S1R agonists such as pridopidine have shown promising results in preclinical studies and in clinical trials.
Author Contributions
All authors listed have made a substantial, direct and intellectual contribution to the work, and approved it for publication.
Funding
This research was supported by the National Institutes of Health (R01NS056224 and R01AG055577 to IB and F32NS093786 to DR), by the State grant 17.991.2017/4.6 to IB, by a grant from the RFBF according to the research project 18-34-00994 to NK, and by the Russian Scientific Fund grant 19-15-00184 to IB. IB holds the Carl J. and Hortense M. Thomsen Chair in Alzheimer’s Disease Research.
Conflict of Interest Statement
The authors declare that the research was conducted in the absence of any commercial or financial relationships that could be construed as a potential conflict of interest.
References
Abdel-Salam, O. M., El-Sayed El-Shamarka, M., Salem, N. A., El-Mosallamy, A. E., and Sleem, A. A. (2012). Amelioration of the haloperidol-induced memory impairment and brain oxidative stress by cinnarizine. EXCLI J. 11, 517–530.
Alam, S., Abdullah, C. S., Aishwarya, R., Orr, A. W., Traylor, J., Miriyala, S., et al. (2017). Sigmar1 regulates endoplasmic reticulum stress-induced C/EBP-homologous protein expression in cardiomyocytes. Biosci. Rep. 37:BSR20170898. doi: 10.1042/BSR20170898
Allahtavakoli, M., and Jarrott, B. (2011). Sigma-1 receptor ligand PRE-084 reduced infarct volume, neurological deficits, pro-inflammatory cytokines and enhanced anti-inflammatory cytokines after embolic stroke in rats. Brain Res. Bull. 85, 219–224. doi: 10.1016/j.brainresbull.2011.03.019
Al-Saif, A., Al-Mohanna, F., and Bohlega, S. (2011). A mutation in sigma-1 receptor causes juvenile amyotrophic lateral sclerosis. Ann. Neurol. 70, 913–919. doi: 10.1002/ana.22534
Antonini, V., Prezzavento, O., Coradazzi, M., Marrazzo, A., Ronsisvalle, S., Arena, E., et al. (2009). Anti-amnesic properties of (±)-PPCC, a novel sigma receptor ligand, on cognitive dysfunction induced by selective cholinergic lesion in rats. J. Neurochem. 109, 744–754. doi: 10.1111/j.1471-4159.2009.06000.x
Aydar, E., Palmer, C. P., Klyachko, V. A., and Jackson, M. B. (2002). The sigma receptor as a ligand-regulated auxiliary potassium channel subunit. Neuron 34, 399–410. doi: 10.1016/s0896-6273(02)00677-3
Balasuriya, D., D’Sa, L., Talker, R., Dupuis, E., Maurin, F., Martin, P., et al. (2014). A direct interaction between the sigma-1 receptor and the hERG voltage-gated K+ channel revealed by atomic force microscopy and homogeneous time-resolved fluorescence (HTRF(R)). J. Biol. Chem. 289, 32353–32363. doi: 10.1074/jbc.M114.603506
Balasuriya, D., Stewart, A. P., Crottès, D., Borgese, F., Soriani, O., and Edwardson, J. M. (2012). The sigma-1 receptor binds to the Nav1.5 voltage-gated Na+ channel with 4-fold symmetry. J. Biol. Chem. 287, 37021–37029. doi: 10.1074/jbc.M112.382077
Banez-Coronel, M., Ayhan, F., Tarabochia, A. D., Zu, T., Perez, B. A., Tusi, S. K., et al. (2015). RAN translation in huntington disease. Neuron 88, 667–677. doi: 10.1016/j.neuron.2015.10.038
Bernard-Marissal, N., Medard, J. J., Azzedine, H., and Chrast, R. (2015). Dysfunction in endoplasmic reticulum-mitochondria crosstalk underlies SIGMAR1 loss of function mediated motor neuron degeneration. Brain 138, 875–890. doi: 10.1093/brain/awv008
Bolshakova, A., Kukanova, E. O., Gainullina, A. N., Zhemkov, V. A., Korban, S. A., and Bezprozvanny, I. (2016). Sigma-1 receptor as a potential pharmacological target for the treatment of neuropathology. St. Petersburg Polytech. Univ. J.: Phys. Math. 2, 31–40. doi: 10.1016/j.spjpm.2016.03.003
Bol’shakova, A. V., Kraskovskaya, N. A., Gainullina, A. N., Kukanova, E. O., Vlasova, O. L., and Bezprozvanny, I. B. (2017). Neuroprotective effect of sigma1-receptors on the cell model of huntington’s disease. Bull. Exp. Biol. Med. 164, 252–258. doi: 10.1007/s10517-017-3968-7
Bourne, J., and Harris, K. M. (2007). Do thin spines learn to be mushroom spines that remember? Curr Opin Neurobiol 17, 381–386. doi: 10.1016/j.conb.2007.04.009
Brailoiu, G. C., Deliu, E. L., Console-Bram, M., Soboloff, J., Abood, M. E., Unterwald, E. M., et al. (2016). Cocaine inhibits store-operated Ca2+ entry in brain microvascular endothelial cells: critical role for sigma-1 receptors. Biochem. J. 473, 1–5. doi: 10.1042/BJ20150934
Caccamo, A., Oddo, S., Billings, L. M., Green, K. N., Martinez-Coria, H., Fisher, A., et al. (2006). M1 receptors play a central role in modulating AD-like pathology in transgenic mice. Neuron 49, 671–682. doi: 10.1016/j.neuron.2006.01.020
Caffrey, M., Li, D., and Dukkipati, A. (2012). Membrane protein structure determination using crystallography and lipidic mesophases: recent advances and successes. Biochemistry 51, 6266–6288. doi: 10.1021/bi300010w
Carnally, S. M., Johannessen, M., Henderson, R. M., Jackson, M. B., and Edwardson, J. M. (2010). Demonstration of a direct interaction between sigma-1 receptors and acid-sensing ion channels. Biophys. J. 98, 1182–1191. doi: 10.1016/j.bpj.2009.12.4293
Chen, Y., Hajipour, A. R., Sievert, M. K., Arbabian, M., and Ruoho, A. E. (2007). Characterization of the cocaine binding site on the sigma-1 receptor. Biochemistry 46, 3532–3542. doi: 10.1021/bi061727o
Chu, U. B., Ramachandran, S., Hajipour, A. R., and Ruoho, A. E. (2013). Photoaffinity labeling of the sigma-1 receptor with N-[3-(4-nitrophenyl) propyl]-N-dodecylamine: evidence of receptor dimers. Biochemistry 52, 859–868. doi: 10.1021/bi301517u
Church, J., and Fletcher, E. J. (1995). Blockade by sigma site ligands of high voltage-activated Ca2+ channels in rat and mouse cultured hippocampal pyramidal neurones. Br. J. Pharmacol. 116, 2801–2810. doi: 10.1111/j.1476-5381.1995.tb15929.x
Crottes, D., Martial, S., Rapetti-Mauss, R., Pisani, D. F., Loriol, C., Pellissier, B., et al. (2011). Sig1R protein regulates hERG channel expression through a post-translational mechanism in leukemic cells. J. Biol. Chem. 286, 27947–27958. doi: 10.1074/jbc.M111.226738
De Yebenes, J. G., Landwehrmeyer, B., Squitieri, F., Reilmann, R., Rosser, A., Barker, R. A., et al. (2011). Mermai, pridopidine for the treatment of motor function in patients with huntington’s disease (MermaiHD): a phase 3, randomised, double-blind, placebo-controlled trial. Lancet Neurol. 10, 1049–1057. doi: 10.1016/S1474-4422(11)70233-2
Delint-Ramirez, I., Garcia-Oscos, F., Segev, A., and Kourrich, S. (2018). Cocaine engages a non-canonical, dopamine-independent, mechanism that controls neuronal excitability in the nucleus accumbens. Mol. Psychiatry 2018, 1–2. doi: 10.1038/s41380-018-0092-7
Dong, H., Ma, Y., Ren, Z., Xu, B., Zhang, Y., Chen, J., et al. (2016). Sigma-1 receptor modulates neuroinflammation after traumatic brain injury. Cell. Mol. Neurobiol. 36, 639–645. doi: 10.1007/s10571-015-0244-0
Dyhring, T., Nielsen, E. O., Sonesson, C., Pettersson, F., Karlsson, J., Svensson, P., et al. (2010). The dopaminergic stabilizers pridopidine (ACR16) and (-)-OSU6162 display dopamine D(2) receptor antagonism and fast receptor dissociation properties. Eur. J. Pharmacol. 628, 19–26. doi: 10.1016/j.ejphar.2009.11.025
Eddings, C. R., Arbez, N., Akimov, S., Geva, M., Hayden, M. R., and Ross, C. A. (2019). Pridopidine protects neurons from mutant-huntingtin toxicity via the sigma-1 receptor. Neurobiol. Dis. 129, 118–129. doi: 10.1016/j.nbd.2019.05.009
Esmaeilzadeh, M., Kullingsjo, J., Ullman, H., Varrone, A., and Tedroff, J. (2011). Regional cerebral glucose metabolism after pridopidine (ACR16) treatment in patients with huntington disease. Clin. Neuropharmacol. 34, 95–100. doi: 10.1097/WNF.0b013e31821c31d8
Fehér, Á, Juhász, A., László, A., Kálmán, J Jr, Pákáski, M., Kálmán, J., et al. (2012). Association between a variant of the sigma-1 receptor gene and Alzheimer’s disease. Neurosci. Lett. 517, 136–139. doi: 10.1016/j.neulet.2012.04.046
Fisher, A., Bezprozvanny, I., Wu, L., Ryskamp, D. A., Bar-Ner, N., Natan, N., et al. (2016). AF710B, a novel M1/sigma1 agonist with therapeutic efficacy in animal models of alzheimer’s disease. Neurodegener. Dis. 16, 95–110. doi: 10.1159/000440864
Francardo, V., Bez, F., Wieloch, T., Nissbrandt, H., Ruscher, K., and Cenci, M. A. (2014). Pharmacological stimulation of sigma-1 receptors has neurorestorative effects in experimental parkinsonism. Brain 137, 1998–2014. doi: 10.1093/brain/awu107
Francardo, V., Geva, M., Bez, F., Denis, Q., Steiner, L., Hayden, M. R., et al. (2019). Pridopidine induces functional neurorestoration via the sigma-1 receptor in a mouse model of parkinson’s disease. Neurotherapeutics 16, 465–479. doi: 10.1007/s13311-018-00699-9
Fujimoto, M., Hayashi, T., Urfer, R., Mita, S., and Su, T. P. (2012). Sigma-1 receptor chaperones regulate the secretion of brain-derived neurotrophic factor. Synapse 66, 630–639. doi: 10.1002/syn.21549
Gao, X. F., Yao, J. J., He, Y. L., Hu, C., and Mei, Y. A. (2012). Sigma-1 receptor agonists directly inhibit Nav1.2/1.4 channels. PLoS One 7:e49384. doi: 10.1371/journal.pone.0049384
Garcia-Miralles, M., Geva, M., Tan, J. Y., Yusof, N., Cha, Y., Kusko, R., et al. (2017). Early pridopidine treatment improves behavioral and transcriptional deficits in YAC128 huntington disease mice. JCI Insight. 2:95665. doi: 10.1172/jci.insight.95665
Geva, M., Kusko, R., Soares, H., Fowler, K. D., Birnberg, T., Barash, S., et al. (2016). Pridopidine activates neuroprotective pathways impaired in huntington disease. Hum. Mol. Genet. 25, 3975–3987. doi: 10.1093/hmg/ddw238
Goguadze, N., Zhuravliova, E., Morin, D., Mikeladze, D., and Maurice, T. (2019). Sigma-1 receptor agonists induce oxidative stress in mitochondria and enhance complex i activity in physiological condition but protect against pathological oxidative stress. Neurotox. Res. 35, 1–18. doi: 10.1007/s12640-017-9838-2
Gonzalez-Alvear, G. M., and Werling, L. L. (1994). Regulation of [3H]dopamine release from rat striatal slices by sigma receptor ligands. J. Pharmacol. Exp. Ther. 271, 212–219.
Gregianin, E., Pallafacchina, G., Zanin, S., Crippa, V., Rusmini, P., Poletti, A., et al. (2016). Loss-of-function mutations in the SIGMAR1 gene cause distal hereditary motor neuropathy by impairing ER-mitochondria tethering and Ca2+ signalling. Hum. Mol. Genet. 25, 3741–3753. doi: 10.1093/hmg/ddw220
Griffiths, E. J. (2009). Mitochondrial calcium transport in the heart: physiological and pathological roles. J. Mol. Cell Cardiol. 46, 789–803. doi: 10.1016/j.yjmcc.2009.03.001
Gromek, K. A., Suchy, F. P., Meddaugh, H. R., Wrobel, R. L., LaPointe, L. M., Chu, U. B., et al. (2014). The oligomeric states of the purified sigma-1 receptor are stabilized by ligands. J. Biol. Chem. 289, 20333–20344. doi: 10.1074/jbc.M113.537993
Gronier, B., Waters, S., and Ponten, H. (2013). The dopaminergic stabilizer pridopidine increases neuronal activity of pyramidal neurons in the prefrontal cortex. J. Neural. Transm. 120, 1281–1294. doi: 10.1007/s00702-013-1002-4
Gundlach, A. L., Largent, B. L., and Snyder, S. H. (1986). Autoradiographic localization of sigma receptor binding sites in guinea pig and rat central nervous system with (+)3H-3-(3-hydroxyphenyl)-N-(1-propyl)piperidine. J. Neurosci. 6, 1757–1770. doi: 10.1523/jneurosci.06-06-01757.1986
Guo, L., Zhao, J., Jin, G., Zhao, B., Wang, G., and Zhang, et al. (2013). SKF83959 is a potent allosteric modulator of sigma-1 receptor. Mol. Pharmacol. 83, 577–586. doi: 10.1124/mol.112.083840
Guo, Q., Fu, W., Sopher, B. L., Miller, M. W., Ware, C. B., Martin, G. M., et al. (1999). Increased vulnerability of hippocampal neurons to excitotoxic necrosis in presenilin-1 mutant knock-in mice. Nat. Med. 5, 101–106. doi: 10.1038/4789
Ha, Y., Saul, A., Tawfik, A., Williams, C., Bollinger, K., Smith, R., et al. (2011). Late-onset inner retinal dysfunction in mice lacking sigma receptor 1 (sigmaR1). Invest. Ophthalmol. Vis. Sci. 52, 7749–7760. doi: 10.1167/iovs.11-8169
Ha, Y., Saul, A., Tawfik, A., Zorrilla, E. P., Ganapathy, V., and Smith, S. B. (2012). Diabetes accelerates retinal ganglion cell dysfunction in mice lacking sigma receptor 1. Mol. Vis. 18, 2860–2870.
Hall, H., Iulita, M. F., Gubert, P., Aguilar, L. F., Ducatenzeiler, A., Fisher, A., et al. (2018). AF710B, an M1/sigma-1 receptor agonist with long-lasting disease-modifying properties in a transgenic rat model of Alzheimer’s disease. Alzheimers Dement 14, 811–823. doi: 10.1016/j.jalz.2017.11.009
Hanner, M., Moebius, F. F., Flandorfer, A., Knaus, H. G., Striessnig, J., Kempner, E., et al. (1996). Purification, molecular cloning, and expression of the mammalian sigma1-binding site. Proc. Natl. Acad. Sci. U. S. A. 93, 8072–8077. doi: 10.1073/pnas.93.15.8072
Hardy, J. (2009). The amyloid hypothesis for Alzheimer’s disease: a critical reappraisal. J. Neurochem. 110, 1129–1134. doi: 10.1111/j.1471-4159.2009.06181.x
Hayashi, T., Hayashi, E., Fujimoto, M., Sprong, H., and Su, T. P. (2012). The lifetime of UDP-galactose:ceramide galactosyltransferase is controlled by a distinct endoplasmic reticulum-associated degradation (ERAD) regulated by sigma-1 receptor chaperones. J. Biol. Chem. 287, 43156–43169. doi: 10.1074/jbc.M112.380444
Hayashi, T., Maurice, T., and Su, T. P. (2000). Ca(2+) signaling via sigma(1)-receptors: novel regulatory mechanism affecting intracellular Ca(2+) concentration. J. Pharmacol. Exp. Ther. 293, 788–798.
Hayashi, T., and Su, T. P. (2001). Regulating ankyrin dynamics: roles of sigma-1 receptors. Proc. Natl. Acad. Sci. U. S. A. 98, 491–496. doi: 10.1073/pnas.021413698
Hayashi, T., and Su, T. P. (2003). Sigma-1 receptors (sigma(1) binding sites) form raft-like microdomains and target lipid droplets on the endoplasmic reticulum: roles in endoplasmic reticulum lipid compartmentalization and export. J. Pharmacol. Exp. Ther. 306, 718–725. doi: 10.1124/jpet.103.051284
Hayashi, T., and Su, T. P. (2004). Sigma-1 receptors at galactosylceramide-enriched lipid microdomains regulate oligodendrocyte differentiation. Proc. Natl. Acad. Sci. U. S. A. 101, 14949–14954. doi: 10.1073/pnas.0402890101
Hayashi, T., and Su, T. P. (2005). The potential role of sigma-1 receptors in lipid transport and lipid raft reconstitution in the brain: implication for drug abuse. Life Sci. 77, 1612–1624. doi: 10.1016/j.lfs.2005.05.009
Hayashi, T., and Su, T. P. (2007). Sigma-1 receptor chaperones at the ER-mitochondrion interface regulate Ca(2+) signaling and cell survival. Cell 131, 596–610. doi: 10.1016/j.cell.2007.08.036
Hayashi-Takagi, A., Yagishita, S., Nakamura, M., Shirai, F., Wu, Y. I, Loshbaugh, A. L., et al. (2015). Labelling, and optical erasure of synaptic memory traces in the motor cortex. Nature 525, 333–338. doi: 10.1038/nature15257
Herrera, Y., Katnik, C., Rodriguez, J. D., Hall, A. A., Willing, A., Pennypacker, K. R., et al. (2008). sigma-1 receptor modulation of acid-sensing ion channel a (ASIC1a) and ASIC1a-induced Ca2+ influx in rat cortical neurons. J. Pharmacol. Exp. Ther. 327, 491–502. doi: 10.1124/jpet.108.143974
Hindmarch, I., and Hashimoto, K. (2010). Cognition and depression: the effects of fluvoxamine, a sigma-1 receptor agonist, reconsidered. Hum. Psychopharmacol. 25, 193–200. doi: 10.1002/hup.1106
Hong, J., Wang, L., Zhang, T., Zhang, B., and Chen, L. (2017). Sigma-1 receptor knockout increases alpha-synuclein aggregation and phosphorylation with loss of dopaminergic neurons in substantia nigra. Neurobiol. Aging 59, 171–183. doi: 10.1016/j.neurobiolaging.2017.08.007
Hong, W. C., Yano, H., Hiranita, T., Chin, F. T., McCurdy, C. R., Su, T. P., et al. (2017). The sigma-1 receptor modulates dopamine transporter conformation and cocaine binding and may thereby potentiate cocaine self-administration in rats. J. Biol. Chem. 292, 11250–11261. doi: 10.1074/jbc.M116.774075
Horga, A., Tomaselli, P. J., Gonzalez, M. A., Laura, M., Muntoni, F., Manzur, A. Y., et al. (2016). SIGMAR1 mutation associated with autosomal recessive Silver-like syndrome. Neurology 87, 1607–1612. doi: 10.1212/wnl.0000000000003212
Huang, Y., Zheng, L., Halliday, G., Dobson-Stone, C., Wang, Y., Tang, H. D., et al. (2011). Genetic polymorphisms in sigma-1 receptor and apolipoprotein E interact to influence the severity of Alzheimer’s disease. Curr. Alzheimer Res. 8, 765–770. doi: 10.2174/156720511797633232
Huntington, H. I. (2013). Study Group, a randomized, double-blind, placebo-controlled trial of pridopidine in huntington’s disease. Mov. Disord. 28, 1407–1415. doi: 10.1002/mds.25362
Hyrskyluoto, A., Pulli, I., Törnqvist, K., Ho, T. H., Korhonen, L., and Lindholm, D. (2013). Sigma-1 receptor agonist PRE084 is protective against mutant huntingtin-induced cell degeneration: involvement of calpastatin and the NF-kappaB pathway. Cell Death Dis. 4:e646. doi: 10.1038/cddis.2013.170
Imarisio, S., Carmichael, J., Korolchuk, V., Chen, C. W., Saiki, S., Rose, C., et al. (2008). Huntington’s disease: from pathology and genetics to potential therapies. Biochem. J. 412, 191–209. doi: 10.1042/BJ20071619
Ionescu, A., Gradus, T., Altman, T., Maimon, R., Saraf Avraham, N., Geva, M., et al. (2019). Targeting the sigma-1 receptor via pridopidine ameliorates central features of ALS pathology in a SOD1(G93A) model. Cell Death Dis. 10, 210. doi: 10.1038/s41419-019-1451-2
Ishikawa, M., and Hashimoto, K. (2009). The role of sigma-1 receptors in the pathophysiology of neuropsychiatric diseases. J. Receptor. Ligand Channel Res. 3, 25–36.
Ivanova, A. A., East, M. P., Yi, S. L., and Kahn, R. A. (2014). Characterization of recombinant ELMOD (cell engulfment and motility domain) proteins as GTPase-activating proteins (GAPs) for ARF family GTPases. J. Biol. Chem. 289, 11111–11121. doi: 10.1074/jbc.M114.548529
Jansen, K. L., Faull, R. L., Storey, P., and Leslie, R. A. (1993). Loss of sigma binding sites in the CA1 area of the anterior hippocampus in alzheimer’s disease correlates with CA1 pyramidal cell loss. Brain Res. 623, 299–302. doi: 10.1016/0006-8993(93)91441-t
Johannessen, M., Fontanilla, D., Mavlyutov, T., Ruoho, A. E., and Jackson, M. B. (2011). Antagonist action of progesterone at sigma-receptors in the modulation of voltage-gated sodium channels. Am. J. Physiol. Cell Physiol. 300, C328–C337. doi: 10.1152/ajpcell.00383.2010
Johannessen, M., Ramachandran, S., Riemer, L., Ramos-Serrano, A., Ruoho, A. E., and Jackson, M. B. (2009). Voltage-gated sodium channel modulation by sigma-receptors in cardiac myocytes and heterologous systems. Am. J. Physiol. Cell Physiol. 296, C1049–C1057. doi: 10.1152/ajpcell.00431.2008
Ka, M., Kook, Y. H., Liao, K., Buch, S., and Kim, W. Y. (2016). Transactivation of TrkB by Sigma-1 receptor mediates cocaine-induced changes in dendritic spine density and morphology in hippocampal and cortical neurons. Cell Death Dis. 7:e2414. doi: 10.1038/cddis.2016.319
Kekuda, R., Prasad, P. D., Fei, Y. J., Leibach, F. H., and Ganapathy, V. (1996). Cloning and functional expression of the human type 1 sigma receptor (hSigmaR1). Biochem. Biophys. Res. Commun. 229, 553–558. doi: 10.1006/bbrc.1996.1842
Kikuchi-Utsumi, K., and Nakaki, T. (2008). Chronic treatment with a selective ligand for the sigma-1 receptor chaperone, SA4503, up-regulates BDNF protein levels in the rat hippocampus. Neurosci. Lett. 440, 19–22. doi: 10.1016/j.neulet.2008.05.055
Kim, F. J., Kovalyshyn, I., Burgman, M., Neilan, C., Chien, C., Cand Pasternak, G. W., et al. (2010). Sigma 1 receptor modulation of G-protein-coupled receptor signaling: potentiation of opioid transduction independent from receptor binding. Mol. Pharmacol. 77, 695–703. doi: 10.1124/mol.109.057083
Kim, H. J., Kwon, M. J., Choi, W. J., Oh, K. W., Oh, S. I., Ki, C. S., et al. (2014). Mutations in UBQLN2 and SIGMAR1 genes are rare in korean patients with amyotrophic lateral sclerosis. Neurobiol. Aging 35, e7–e8. doi: 10.1016/j.neurobiolaging.2014.03.001
Kim, M. W., Chelliah, Y., Kim, S. W., Otwinowski, Z., and Bezprozvanny, I. (2009). Secondary structure of huntingtin amino-terminal region. Structure 17, 1205–1212. doi: 10.1016/j.str.2009.08.002
Kimura, Y., Fujita, Y., Shibata, K., Mori, M., and Yamashita, T. (2013). Sigma-1 receptor enhances neurite elongation of cerebellar granule neurons via TrkB signaling. PLoS One 8:e75760. doi: 10.1371/journal.pone.0075760
Kinoshita, M., Matsuoka, Y., Suzuki, T., Mirrielees, J., and Yang, J. (2012). Sigma-1 receptor alters the kinetics of Kv1.3 voltage gated potassium channels but not the sensitivity to receptor ligands. Brain Res. 1452, 1–9. doi: 10.1016/j.brainres.2012.02.070
Kourrich, S., Hayashi, T., Chuang, J. Y., Tsai, S. Y., Su, T. P., Bonci, A., et al. (2013). Dynamic interaction between sigma-1 receptor and Kv1.2 shapes neuronal and behavioral responses to cocaine. Cell 152, 236–247. doi: 10.1016/j.cell.2012.12.004
Kourrich, S., Su, T. P., Fujimoto, M., and Bonci, A. (2012). The sigma-1 receptor: roles in neuronal plasticity and disease. Trends Neurosci. 35, 762–771. doi: 10.1016/j.tins.2012.09.007
Kusko, R., Dreymann, J., Ross, J., Cha, Y., Escalante-Chong, R., Garcia-Miralles, M., et al. (2018). Large-scale transcriptomic analysis reveals that pridopidine reverses aberrant gene expression and activates neuroprotective pathways in the YAC128 HD mouse. Mol. Neurodegener. 13:25. doi: 10.1186/s13024-018-0259-3
Lahmy, V., Meunier, J., Malmstr, S., öm, Naert, G., Givalois, L., et al. (2013). Blockade of Tau hyperphosphorylation and Aβ 1–42 generation by the aminotetrahydrofuran derivative ANAVEX2-73, a mixed muscarinic and σ 1 receptor agonist, in a nontransgenic mouse model of Alzheimer’s disease. Neuropsychopharmacology 38:1706. doi: 10.1038/npp.2013.70
Langa, F., Codony, X., Tovar, V., Lavado, A., Gimenez, E., Cozar, P., et al. (2003). Generation and phenotypic analysis of sigma receptor type I (sigma 1) knockout mice. Eur. J. Neurosci. 18, 2188–2196. doi: 10.1046/j.1460-9568.2003.02950.x
Laurini, E., Col, V. D., Mamolo, M. G., Zampieri, D., Posocco, P., Fermeglia, M., et al. (2011). Homology model and docking-based virtual screening for ligands of the sigma1 receptor. ACS Med. Chem. Lett. 2, 834–839. doi: 10.1021/ml2001505
Leitman, J., Ulrich Hartl, F., and Lederkremer, G. Z. (2013). Soluble forms of polyQ-expanded huntingtin rather than large aggregates cause endoplasmic reticulum stress. Nat. Commun. 4:2753. doi: 10.1038/ncomms3753
Lemasters, J. J., Theruvath, T. P., Zhong, Z., and Nieminen, A. L. (2009). Mitochondrial calcium and the permeability transition in cell death. Biochim. Biophys. Acta 1787, 1395–1401. doi: 10.1016/j.bbabio.2009.06.009
Li, X., Hu, Z., Liu, L., Xie, Y., Zhan, Y., Zi, X., et al. (2015). A SIGMAR1 splice-site mutation causes distal hereditary motor neuropathy. Neurology 84, 2430–2437. doi: 10.1212/WNL.0000000000001680
Lu, W., Man, H., Ju, W., Trimble, W. S., MacDonald, J. F., and Wang, Y. T. (2001). Activation of synaptic NMDA receptors induces membrane insertion of new AMPA receptors and LTP in cultured hippocampal neurons. Neuron 29, 243–254. doi: 10.1016/s0896-6273(01)00194-5
Lundin, A., Dietrichs, E., Haghighi, S., Goller, M. L., Heiberg, A., Loutfi, G., et al. (2010). Efficacy and safety of the dopaminergic stabilizer pridopidine (ACR16) in patients with huntington’s disease. Clin. Neuropharmacol. 33, 260–264. doi: 10.1097/WNF.0b013e3181ebb285
Luty, A. A., Kwok, J. B., Dobson-Stone, C., Loy, C. T., Coupland, K. G., Karlstrom, H., et al. (2010). Sigma nonopioid intracellular receptor 1 mutations cause frontotemporal lobar degeneration-motor neuron disease. Ann. Neurol. 68, 639–649. doi: 10.1002/ana.22274
Macfarlane, S., Cecchi, M., Moore, D., Maruff, P., Zografidis, T., et al. (2016). safety and efficacy 31 week data of anavex 2-73 in a phase 2a study in mild-moderate alzheimer’s disease patients. Alzheimer’s Dementia J. Alzheimer’s Assoc. 12:1174.
Mancuso, R., Oliván, S., Rando, A., Casas, C., Osta, R., and Navarro, X. (2012). Sigma-1R agonist improves motor function and motoneuron survival in ALS mice. Neurotherapeutics 9, 814–826. doi: 10.1007/s13311-012-0140-y
Marriott, K. S., Prasad, M., Thapliyal, V., and Bose, H. S. (2012). sigma-1 receptor at the mitochondrial-associated endoplasmic reticulum membrane is responsible for mitochondrial metabolic regulation. J. Pharmacol. Exp. Ther. 343, 578–586. doi: 10.1124/jpet.112.198168
Martina, M., Turcotte, M. E., Halman, S., and Bergeron, R. (2007). The sigma-1 receptor modulates NMDA receptor synaptic transmission and plasticity via SK channels in rat hippocampus. J. Physiol. 578, 143–157. doi: 10.1113/jphysiol.2006.116178
Maruszak, A., Safranow, K., Gacia, M., Gabryelewicz, T., Slowik, A., Styczynska, M., et al. (2007). Sigma receptor type 1 gene variation in a group of Polish patients with alzheimer’s disease and mild cognitive impairment. Dement. Geriatr. Cogn. Disord. 23, 432–438. doi: 10.1159/000101990
Matsuno, K., Nakazawa, M., Okamoto, K., Kawashima, Y., and Mita, S. (1996). Binding properties of SA4503, a novel and selective σ1 receptor agonist. Eur. J. Pharmacol 306, 271–279. doi: 10.1016/0014-2999(96)00201-4
Maurice, T., and Goguadze, N. (2017). Sigma-1 (sigma1) receptor in memory and neurodegenerative diseases. Handb. Exp. Pharmacol. 244, 81–108. doi: 10.1007/164_2017_15
Maurice, T., Gregoire, C., and Espallergues, J. (2006a). Neuro(active)steroids actions at the neuromodulatory sigma1 (sigma1) receptor: biochemical and physiological evidences, consequences in neuroprotection. Pharmacol. Biochem. Behav. 84, 581–597. doi: 10.1016/j.pbb.2006.07.009
Maurice, T., Meunier, J., Feng, B., Ieni, J., and Monaghan, D. T. (2006b). Interaction with sigma(1) protein, but not N-methyl-D-aspartate receptor, is involved in the pharmacological activity of donepezil. J. Pharmacol. Exp. Ther. 317, 606–614. doi: 10.1124/jpet.105.097394
Maurice, T., and Lockhart, B. P. (1997). Neuroprotective and anti-amnesic potentials of sigma (sigma) receptor ligands. Prog. Neuropsychopharmacol. Biol. Psychiatry 21, 69–102. doi: 10.1016/s0278-5846(96)00160-1
Maurice, T., Strehaiano, M., Duhr, F., and Chevallier, N. (2018). Amyloid toxicity is enhanced after pharmacological or genetic invalidation of the sigma1 receptor. Behav. Brain Res. 339, 1–10. doi: 10.1016/j.bbr.2017.11.010
Maurice, T., Volle, J. N., Strehaiano, M., Crouzier, L., Pereira, C., Kaloyanov, N., et al. (2019). Neuroprotection in non-transgenic and transgenic mouse models of Alzheimer’s disease by positive modulation of sigma1 receptors. Pharmacol. Res. 144, 315–330. doi: 10.1016/j.phrs.2019.04.026
Mavlyutov, T. A., Epstein, M., and Guo, L. W. (2015a). Subcellular localization of the sigma-1 receptor in retinal neurons - an electron microscopy study. Sci. Rep. 5, 10689. doi: 10.1038/srep10689
Mavlyutov, T. A., Guo, L. W., Epstein, M. L., and Ruoho, A. E. (2015b). Role of the Sigma-1 receptor in Amyotrophic Lateral Sclerosis (ALS). J. Pharmacol. Sci. 127, 10–16. doi: 10.1016/j.jphs.2014.12.013
Mavlyutov, T. A., Epstein, M. L., Andersen, K. A., Ziskind-Conhaim, L., and Ruoho, A. E. (2010). The sigma-1 receptor is enriched in postsynaptic sites of C-terminals in mouse motoneurons. an anatomical and behavioral study. Neuroscience 167, 247–255. doi: 10.1016/j.neuroscience.2010.02.022
Mavlyutov, T. A., Epstein, M. L., Verbny, Y. I., Huerta, M. S., Zaitoun, I., Ziskind-Conhaim, L., et al. (2013). Lack of sigma-1 receptor exacerbates ALS progression in mice. Neuroscience 240, 129–134. doi: 10.1016/j.neuroscience.2013.02.035
Mavlyutov, T. A., Nickells, R. W., and Guo, L. W. (2011). Accelerated retinal ganglion cell death in mice deficient in the Sigma-1 receptor. Mol. Vis. 17, 1034–1043.
Mavylutov, T., Chen, X., Guo, L., and Yang, J. (2018). APEX2- tagging of Sigma 1-receptor indicates subcellular protein topology with cytosolic N-terminus and ER luminal C-terminus. Protein Cell 9, 733–737. doi: 10.1007/s13238-017-0468-5
McCann, D. J., and Su, T. P. (1990). Haloperidol-sensitive (+)[3H]SKF-10,047 binding sites (sigma sites) exhibit unique distribution in rat brain subcellular fractions. Eur. J. Pharmacol. 188, 211–218. doi: 10.1016/0922-4106(90)90004-h
Medeiros, R., Kitazawa, M., Caccamo, A., Baglietto-Vargas, D., Estrada-Hernandez, T., Cribbs, D. H., et al. (2011). Loss of muscarinic M1 receptor exacerbates alzheimer’s disease–like pathology and cognitive decline. Am. J. Pathol. 179, 980–991. doi: 10.1016/j.ajpath.2011.04.041
Meunier, J., and Hayashi, T. (2010). Sigma-1 receptors regulate Bcl-2 expression by reactive oxygen species-dependent transcriptional regulation of nuclear factor KB. J. Pharmacol. Exp. Ther. 332, 388–397. doi: 10.1124/jpet.109.160960
Miki, Y., Mori, F., Kon, T., Tanji, K., Toyoshima, Y., Yoshida, M., et al. (2014). Accumulation of the sigma-1 receptor is common to neuronal nuclear inclusions in various neurodegenerative diseases. Neuropathology 34, 148–158. doi: 10.1111/neup.12080
Miki, Y., Tanji, K., Mori, F., and Wakabayashi, K. (2015). Sigma-1 receptor is involved in degradation of intranuclear inclusions in a cellular model of Huntington’s disease. Neurobiol. Dis. 74, 25–31. doi: 10.1016/j.nbd.2014.11.005
Mishina, M., Ishiwata, K., Ishii, K., Kitamura, S., Kimura, Y., Kawamura, K., et al. (2005). Function of sigma1 receptors in parkinson’s disease. Acta Neurol. Scand. 112, 103–107. doi: 10.1111/j.1600-0404.2005.00432.x
Mishina, M., Ohyama, M., Ishii, K., Kitamura, S., Kimura, Y., Oda, K., et al. (2008). Low density of sigma1 receptors in early Alzheimer’s disease. Ann. Nucl. Med. 22, 151–156. doi: 10.1007/s12149-007-0094-z
Mishra, A. K., Mavlyutov, T., Singh, D. R., Biener, G., Yang, J., Oliver, J. A., et al. (2015). The sigma-1 receptors are present in monomeric and oligomeric forms in living cells in the presence and absence of ligands. Biochem. J. 466, 263–271. doi: 10.1042/BJ20141321
Mochel, F., and Haller, R. G. (2011). Energy deficit in huntington disease: why it matters. J. Clin. Invest. 121, 493–499. doi: 10.1172/JCI45691
Monnet, F. P., Debonnel, G., and de, C. (1992). Montigny, In vivo electrophysiological evidence for a selective modulation of N-methyl-D-aspartate-induced neuronal activation in rat CA3 dorsal hippocampus by sigma ligands. J. Pharmacol. Exp. Ther. 261, 123–130.
Monnet, F. P., Debonnel, G., Junien, J. L., and De, C. (1990). Montigny, N-methyl-D-aspartate-induced neuronal activation is selectively modulated by sigma receptors. Eur. J. Pharmacol. 179, 441–445. doi: 10.1016/0014-2999(90)90186-a
Monnet, F. P., Mahe, V., Robel, P., and Baulieu, E. E. (1995). Neurosteroids, via sigma receptors, modulate the [3H]norepinephrine release evoked by N-methyl-D-aspartate in the rat hippocampus. Proc. Natl. Acad. Sci. U. S. A. 92, 3774–3778. doi: 10.1073/pnas.92.9.3774
Moreno, E., Moreno-Delgado, D., Navarro, G., Hoffmann, H. M., Fuentes, S., Rosell-Vilar, S., et al. (2014). Cocaine disrupts histamine H3 receptor modulation of dopamine D1 receptor signaling: sigma1-D1-H3 receptor complexes as key targets for reducing cocaine’s effects. J. Neurosci. 34, 3545–3558. doi: 10.1523/JNEUROSCI.4147-13.2014
Mori, T., Hayashi, T., Hayashi, E., and Su, T. P. (2013). Sigma-1 receptor chaperone at the ER-mitochondrion interface mediates the mitochondrion-ER-nucleus signaling for cellular survival. PLoS One 8:e76941. doi: 10.1371/journal.pone.0076941
Moriguchi, S., Shinoda, Y., Yamamoto, Y., Sasaki, Y., Miyajima, K., Tagashira, H., et al. (2013). Stimulation of the sigma-1 receptor by DHEA enhances synaptic efficacy and neurogenesis in the hippocampal dentate gyrus of olfactory bulbectomized mice. PLoS One 8:e60863. doi: 10.1371/journal.pone.0060863
Moriguchi, S., Yamamoto, Y., Ikuno, T., and Fukunaga, K. (2011). Sigma-1 receptor stimulation by dehydroepiandrosterone ameliorates cognitive impairment through activation of CaM kinase II, protein kinase C and extracellular signal-regulated kinase in olfactory bulbectomized mice. J. Neurochem. 117, 879–891. doi: 10.1111/j.1471-4159.2011.07256.x
Morin-Surun, M. P., Collin, T., Denavit-Saubie, M., Baulieu, E. E., and Monnet, F. P. (1999). Intracellular sigma1 receptor modulates phospholipase C and protein kinase C activities in the brainstem. Proc. Natl. Acad. Sci. U. S. A. 96, 8196–8199. doi: 10.1073/pnas.96.14.8196
Moritz, C., Berardi, F., Abate, C., and Peri, F. (2015). Live imaging reveals a new role for the sigma-1 (sigma1) receptor in allowing microglia to leave brain injuries. Neurosci. Lett. 591, 13–18. doi: 10.1016/j.neulet.2015.02.004
Morris, R. G., Anderson, E., Lynch, G. S., and Baudry, M. (1986). Selective impairment of learning and blockade of long-term potentiation by an N-methyl-D-aspartate receptor antagonist. AP5. Nature 319, 774–776. doi: 10.1038/319774a0
Mueller, B. H., Park, Y., Daudt, D. R., Ma, H. Y., Akopova, I., Stankowska, D. L., et al. (2013). Sigma-1 receptor stimulation attenuates calcium influx through activated L-type voltage gated calcium channels in purified retinal ganglion cells. Exp. Eye Res. 107, 21–31. doi: 10.1016/j.exer.2012.11.002
Nakazawa, M., Matsuno, K., and Mita, S. (1998). Activation of sigma1 receptor subtype leads to neuroprotection in the rat primary neuronal cultures. Neurochem. Int. 32, 337–343. doi: 10.1016/s0197-0186(97)00105-8
Natsvlishvili, N., Goguadze, N., Zhuravliova, E., and Mikeladze, D. (2015). Sigma-1 receptor directly interacts with Rac1-GTPase in the brain mitochondria. BMC Biochem. 16:11. doi: 10.1186/s12858-015-0040-y
Navarro, G., Moreno, E., Aymerich, M., Marcellino, D., McCormick, P. J., Mallol, J., et al. (2010). Direct involvement of sigma-1 receptors in the dopamine D1 receptor-mediated effects of cocaine. Proc. Natl. Acad. Sci. U. S. A. 107, 18676–18681. doi: 10.1073/pnas.1008911107
Navarro, G., Moreno, E., Bonaventura, J., Brugarolas, M., Farre, D., Aguinaga, D., et al. (2013). Cocaine inhibits dopamine D2 receptor signaling via sigma-1-D2 receptor heteromers. PLoS One 8:e61245. doi: 10.1371/journal.pone.0061245
Nelson, O., Tu, H., Lei, T., Bentahir, M., de, Strooper B, and Bezprozvanny, I. (2007). Familial Alzheimer disease-linked mutations specifically disrupt Ca2+ leak function of presenilin 1. J. Clin. Invest. 117, 1230–1239. doi: 10.1172/jci30447
Nilsson, M., Carlsson, A., Markinhuhta, K. R., Sonesson, C., Pettersson, F., Gullme, M., et al. (2004). The dopaminergic stabiliser ACR16 counteracts the behavioural primitivization induced by the NMDA receptor antagonist MK-801 in mice: implications for cognition. Prog. Neuropsychopharmacol. Biol. Psychiatry 28, 677–685. doi: 10.1016/j.pnpbp.2004.05.004
Nishimura, T., Ishima, T., Iyo, M., and Hashimoto, K. (2008). Potentiation of nerve growth factor-induced neurite outgrowth by fluvoxamine: role of sigma-1 receptors, IP3 receptors and cellular signaling pathways. PLoS One 3:e2558. doi: 10.1371/journal.pone.0002558
Ono, Y., Tanaka, H., Takata, M., Nagahara, Y., Noda, Y., Tsuruma, K., et al. (2014). SA4503, a sigma-1 receptor agonist, suppresses motor neuron damage in in vitro and in vivo amyotrophic lateral sclerosis models. Neurosci. Lett. 559, 174–178. doi: 10.1016/j.neulet.2013.12.005
Ortega-Roldan, J. L., Ossa, F., Amin, N. T., and Schnell, J. R. (2015). Solution NMR studies reveal the location of the second transmembrane domain of the human sigma-1 receptor. FEBS Lett. 589, 659–665. doi: 10.1016/j.febslet.2015.01.033
Ortega-Roldan, J. L., Ossa, F., and Schnell, J. R. (2013). Characterization of the human sigma-1 receptor chaperone domain structure and binding immunoglobulin protein (BiP) interactions. J. Biol. Chem. 288, 21448–21457. doi: 10.1074/jbc.M113.450379
Pabba, M., Wong, A. Y., Ahlskog, N., Hristova, E., Biscaro, D., Nassrallah, W., et al. (2014). NMDA receptors are upregulated and trafficked to the plasma membrane after sigma-1 receptor activation in the rat hippocampus. J. Neurosci. 34, 11325–11338. doi: 10.1523/JNEUROSCI.0458-14.2014
Pal, A., Chu, U. B., Ramachandran, S., Grawoig, D., Guo, L. W., Hajipour, A. R., et al. (2008). Juxtaposition of the steroid binding domain-like I and II regions constitutes a ligand binding site in the sigma-1 receptor. J. Biol. Chem. 283, 19646–19656. doi: 10.1074/jbc.M802192200
Pal, A., Hajipour, A. R., Fontanilla, D., Ramachandran, S., Chu, U. B., Mavlyutov, T., et al. (2007). Identification of regions of the sigma-1 receptor ligand binding site using a novel photoprobe. Mol. Pharmacol. 72, 921–933. doi: 10.1124/mol.107.038307
Palmer, C. P., Mahen, R., Schnell, E., Djamgoz, M. B., and Aydar, E. (2007). Sigma-1 receptors bind cholesterol and remodel lipid rafts in breast cancer cell lines. Cancer Res. 67, 11166–11175. doi: 10.1158/0008-5472.can-07-1771
Pchitskaya, E., Kraskovskaya, N., Chernyuk, D., Popugaeva, E., Zhang, H., Vlasova, O., et al. (2017). Stim2-Eb3 association and morphology of dendritic spines in hippocampal neurons. Sci. Rep. 7:17625. doi: 10.1038/s41598-017-17762-8
Peviani, M., Salvaneschi, E., Bontempi, L., Petese, A., Manzo, A., Rossi, D., et al. (2014). Neuroprotective effects of the Sigma-1 receptor (S1R) agonist PRE-084, in a mouse model of motor neuron disease not linked to SOD1 mutation. Neurobiol. Dis. 62, 218–232. doi: 10.1016/j.nbd.2013.10.010
Ponten, H., Kullingsj, J. Ö, Lagerkvist, S., Martin, P., Pettersson, F., Sonesson, C., et al. (2010). In vivo pharmacology of the dopaminergic stabilizer pridopidine. Eur. J. Pharmacol. 644, 88–95. doi: 10.1016/j.ejphar.2010.07.023
Popugaeva, E., Pchitskaya, E., Speshilova, A., Alexandrov, S., Zhang, H., Vlasova, O., et al. (2015). STIM2 protects hippocampal mushroom spines from amyloid synaptotoxicity. Mol. Neurodegener. 10:37. doi: 10.1186/s13024-015-0034-7
Reilmann, R., McGarry, A., Grachev, I. D., Savola, J. M., Borowsky, B., Eyal, E., et al. (2019). Huntington’s disease, and i. huntington study group, safety and efficacy of pridopidine in patients with Huntington’s disease (PRIDE-HD): a phase 2, randomised, placebo-controlled, multicentre, dose-ranging study. Lancet Neurol. 18, 165–176. doi: 10.1016/S1474-4422(18)30391-0
Rosen, D. A., Seki, S. M., Fernandez-Castaneda, A., Beiter, R. M., Eccles, J. D., Woodfolk, J. A., et al. (2019). Modulation of the sigma-1 receptor-IRE1 pathway is beneficial in preclinical models of inflammation and sepsis. Sci. Transl. Med. 11:eaax3130. doi: 10.1126/scitranslmed.aax3130
Rothman, S. M., and Olney, J. W. (1987). Excitotoxity and the NMDA receptor. Trends Neurosci. 10, 299–302. doi: 10.1016/0166-2236(87)90177-9
Rung, J. P., Rung, E., Helgeson, L., Johansson, A. M., Svensson, K., Carlsson, A., et al. (2008). Effects of (-)-OSU6162 and ACR16 on motor activity in rats, indicating a unique mechanism of dopaminergic stabilization. J. Neural. Transm. 115, 899–908. doi: 10.1007/s00702-008-0038-3
Ruscher, K., Inacio, A. R., Valind, K., Rowshan, Ravan, A., Kuric, E., et al. (2012). Effects of the sigma-1 receptor agonist 1-(3,4-dimethoxyphenethyl)-4-(3-phenylpropyl)-piperazine dihydro-chloride on inflammation after stroke. PLoS One 7:e45118. doi: 10.1371/journal.pone.0045118
Ruscher, K., Shamloo, M., Rickhag, M., Ladunga, I., Soriano, L., Gisselsson, L., et al. (2011). The sigma-1 receptor enhances brain plasticity and functional recovery after experimental stroke. Brain 134, 732–746. doi: 10.1093/brain/awq367
Ryskamp, D., Wu, J., Geva, M., Kusko, R., Grossman, I., Hayden, M., et al. (2017). The sigma-1 receptor mediates the beneficial effects of pridopidine in a mouse model of Huntington disease. Neurobiol. Dis. 97, 46–59. doi: 10.1016/j.nbd.2016.10.006
Ryskamp, D., Wu, L., Wu, J., Kim, D., Rammes, G., Geva, M., et al. (2019). Pridopidine stabilizes mushroom spines in mouse models of Alzheimer’s disease by acting on the sigma-1 receptor. Neurobiol. Dis. 124, 489–504. doi: 10.1016/j.nbd.2018.12.022
Sabino, V., Cottone, P., Parylak, S. L., Steardo, L., and Zorrilla, E. P. (2009). Sigma-1 receptor knockout mice display a depressive-like phenotype. Behav. Brain Res. 198, 472–476. doi: 10.1016/j.bbr.2008.11.036
Sahlholm, K., Arhem, P., Fuxe, K., and Marcellino, D. (2013). The dopamine stabilizers ACR16 and (-)-OSU6162 display nanomolar affinities at the sigma-1 receptor. Mol. Psychiatry 18, 12–14. doi: 10.1038/mp.2012.3
Sahlholm, K., Sijbesma, J. W., Maas, B., Kwizera, C., Marcellino, D., Ramakrishnan, N. K., et al. (2015). Waarde, Pridopidine selectively occupies sigma-1 rather than dopamine D2 receptors at behaviorally active doses. Psychopharmacology 232, 3443–3453. doi: 10.1007/s00213-015-3997-8
Sambo, D. O., Lin, M., Owens, A., Lebowitz, J. J., Richardson, B., Jagnarine, D. A., et al. (2017). The sigma-1 receptor modulates methamphetamine dysregulation of dopamine neurotransmission. Nat. Commun. 8:2228. doi: 10.1038/s41467-017-02087-x
Sanchez-Blazquez, P., Rodriguez-Munoz, M., Herrero-Labrador, R., Burgueno, J., Zamanillo, D., and Garzon, J. (2014). The calcium-sensitive Sigma-1 receptor prevents cannabinoids from provoking glutamate NMDA receptor hypofunction: implications in antinociception and psychotic diseases. Int. J. Neuropsychopharmacol. 17, 1943–1955. doi: 10.1017/S1461145714000029
Sato, S., Kawamata, T., Kobayashi, T., and Okada, Y. (2014). Antidepressant fluvoxamine reduces cerebral infarct volume and ameliorates sensorimotor dysfunction in experimental stroke. Neuroreport 25, 731–736. doi: 10.1097/WNR.0000000000000162
Schmidt, H. R., Betz, R. M., Dror, R. O., and Kruse, A. C. (2018). Structural basis for sigma1 receptor ligand recognition. Nat. Struct. Mol. Biol. 25, 981–987. doi: 10.1038/s41594-018-0137-2
Schmidt, H. R., Zheng, S., Gurpinar, E., Koehl, A., Manglik, A., and Kruse, A. C. (2016). Crystal structure of the human sigma1 receptor. Nature 532, 527–530. doi: 10.1038/nature17391
Schreiner, B., Hedskog, L., Wiehager, B., and Ankarcrona, M. (2015). Amyloid-beta peptides are generated in mitochondria-associated endoplasmic reticulum membranes. J. Alzheimers. Dis. 43, 369–374. doi: 10.3233/JAD-132543
Sha, S., Hong, J., Qu, W. J., Lu, Z. H., Li, L., Yu, W. F., et al. (2015). Sex-related neurogenesis decrease in hippocampal dentate gyrus with depressive-like behaviors in sigma-1 receptor knockout mice. Eur. Neuropsychopharmacol. 25, 1275–1286. doi: 10.1016/j.euroneuro.2015.04.021
Smith, S. B., Duplantier, J., Dun, Y., Mysona, B., Roon, P., Martin, P. M., et al. (2008). In vivo protection against retinal neurodegeneration by sigma receptor 1 ligand (+)-pentazocine. Invest. Ophthalmol. Vis. Sci. 49, 4154–4161. doi: 10.1167/iovs.08-1824
Smith-Dijak, A. I., Nassrallah, W. B., Zhang, L. Y. J., Geva, M., Hayden, M. R., and Raymond, L. A. (2019). Impairment and restoration of homeostatic plasticity in cultured cortical neurons from a mouse model of huntington disease. Front. Cell. Neurosci. 13:209. doi: 10.3389/fncel.2019.00209
Squitieri, F., Di, Pardo, A., Favellato, M., Amico, E., Maglione, V., et al. (2015). Pridopidine, a dopamine stabilizer, improves motor performance and shows neuroprotective effects in huntington disease R6/2 mouse model. J. Cell Mol. Med. 19, 2540–2548. doi: 10.1111/jcmm.12604
Srivats, S., Balasuriya, D., Pasche, M., Vistal, G., Edwardson, J. M., Taylor, C. W., et al. (2016). Sigma1 receptors inhibit store-operated Ca2+ entry by attenuating coupling of STIM1 to Orai1. J. Cell Biol. 213, 65–79. doi: 10.1083/jcb.201506022
Su, T. P., Su, T. C., Nakamura, Y., and Tsai, S. Y. (2016). The sigma-1 receptor as a pluripotent modulator in living systems. Trends Pharmacol. Sci. 37, 262–278. doi: 10.1016/j.tips.2016.01.003
Su, T. P., Wu, X., Cone, E. J., Shukla, K., Gund, T. M., Dodge, A. L., et al. (1991). Sigma compounds derived from phencyclidine: identification of PRE-084, a new, selective sigma ligand. J. Pharmacol. Exp. Ther. 259, 543–550.
Sun, S., Zhang, H., Liu, J., Popugaeva, E., Xu, N. J., Feske, S., et al. (2014). Reduced synaptic STIM2 expression and impaired store-operated calcium entry cause destabilization of mature spines in mutant presenilin mice. Neuron 82, 79–93. doi: 10.1016/j.neuron.2014.02.019
Sun, X., Beglopoulos, V., Mattson, M. P., and Shen, J. (2005). Hippocampal spatial memory impairments caused by the familial Alzheimer’s disease-linked presenilin 1 M146V mutation. Neurodegener. Dis. 2, 6–15. doi: 10.1159/000086426
Svensson, K., Falcone, J., Johansson, A., Perry, K., and Fell, M. (2009). “The actions of the dopamine stabilizer ACR16, but not (-)-OSU6162, in behavioral and neurochemical assays are not dependent on the presence of functional dopamine D2 receptors,” in Proceedings of the 39th Annual Meeting, Society for Neuroscience, Chicago.
Tada, T., and Sheng, M. (2006). Molecular mechanisms of dendritic spine morphogenesis. Curr. Opin. Neurobiol. 16, 95–101. doi: 10.1016/j.conb.2005.12.001
Takebayashi, M., Hayashi, T., and Su, T. P. (2004). A perspective on the new mechanism of antidepressants: neuritogenesis through sigma-1 receptors. Pharmacopsychiatry 37(Suppl. 3), S208–S213.
Tang, T. S., Guo, C., Wang, H., Chen, X., and Bezprozvanny, I. (2009). Neuroprotective effects of inositol 1,4,5-trisphosphate receptor C-terminal fragment in a huntington’s disease mouse model. J. Neurosci. 29, 1257–1266. doi: 10.1523/JNEUROSCI.4411-08.2009
Tang, T. S., Tu, H., Chan, E. Y., Maximov, A., Wang, Z., Wellington, C. L., et al. (2003). Huntingtin and huntingtin-associated protein 1 influence neuronal calcium signaling mediated by inositol-(1,4,5) triphosphate receptor type 1. Neuron 39, 227–239. doi: 10.1016/s0896-6273(03)00366-0
Tchedre, K. T., Huang, R. Q., Dibas, A., Krishnamoorthy, R. R., Dillon, G. H., Yorio, T., et al. (2008). Sigma-1 receptor regulation of voltage-gated calcium channels involves a direct interaction. Invest. Ophthalmol. Vis. Sci. 49, 4993–5002. doi: 10.1167/iovs.08-1867
Thomas, J. D., Longen, C. G., Oyer, H. M., Chen, N., Maher, C. M., Salvino, J. M., et al. (2017). Sigma1 targeting to suppress aberrant androgen receptor signaling in prostate cancer. Cancer Res. 77, 2439–2452. doi: 10.1158/0008-5472.CAN-16-1055
Tsai, S. Y., Chuang, J. Y., Tsai, M. S., Wang, X. F., Xi, Z. X., Hung, J. J., et al. (2015a). Sigma-1 receptor mediates cocaine-induced transcriptional regulation by recruiting chromatin-remodeling factors at the nuclear envelope. Proc. Natl. Acad. Sci. U. S. A. 112, E6562–E6570. doi: 10.1073/pnas.1518894112
Tsai, S. Y., Pokrass, M. J., Klauer, N. R., Nohara, H., and Su, T. P. (2015b). Sigma-1 receptor regulates Tau phosphorylation and axon extension by shaping p35 turnover via myristic acid. Proc. Natl. Acad. Sci. U. S. A. 112, 6742–6747. doi: 10.1073/pnas.1422001112
Tsai, S. Y., Hayashi, T., Harvey, B. K., Wang, Y., Wu, W. W., Shen, R. F., et al. (2009). Sigma-1 receptors regulate hippocampal dendritic spine formation via a free radical-sensitive mechanism involving Rac1xGTP pathway. Proc. Natl. Acad. Sci. U. S. A. 106, 22468–22473. doi: 10.1073/pnas.0909089106
Tsai, S. Y., Rothman, R. K., and Su, T. P. (2012). Insights into the Sigma-1 receptor chaperone’s cellular functions: a microarray report. Synapse 66, 42–51. doi: 10.1002/syn.20984
Tu, H., Nelson, O., Bezprozvanny, A., Wang, Z., Lee, S. F., Hao, Y. H., et al. (2006). Presenilins form ER Ca2+ leak channels, a function disrupted by familial Alzheimer’s disease-linked mutations. Cell 126, 981–993. doi: 10.1016/j.cell.2006.06.059
Uchida, N., Ujike, H., Tanaka, Y., Sakai, A., Yamamoto, M., Fujisawa, Y., et al. (2005). A variant of the sigma receptor type-1 gene is a protective factor for Alzheimer disease. Am. J. Geriatr. Psychiatry 13, 1062–1066. doi: 10.1176/appi.ajgp.13.12.1062
Ullah, M. I., Ahmad, A., Raza, S. I., Amar, A., Ali, A., Bhatti, A., et al. (2015). In silico analysis of SIGMAR1 variant (rs4879809) segregating in a consanguineous Pakistani family showing amyotrophic lateral sclerosis without frontotemporal lobar dementia. Neurogenetics 16, 299–306. doi: 10.1007/s10048-015-0453-1
Urfer, R., Moebius, H. J., Skoloudik, D., Santamarina, E., Sato, W., Mita, S., et al. (2014). Stroke Recovery Study, Phase II trial of the Sigma-1 receptor agonist cutamesine (SA4503) for recovery enhancement after acute ischemic stroke. Stroke 45, 3304–3310. doi: 10.1161/strokeaha.114.005835
Vavers, E., Zvejniece, L., Maurice, T., and Dambrova, M. (2019). Allosteric modulators of sigma-1 receptor: a review. Front. Pharmacol. 10:223. doi: 10.3389/fphar.2019.00223
Wang, J., Shanmugam, A., Markand, S., Zorrilla, E., Ganapathy, V., and Smith, S. B. (2015). Sigma 1 receptor regulates the oxidative stress response in primary retinal Muller glial cells via NRF2 signaling and system xc(−), the Na(+)-independent glutamate-cystine exchanger. Free Radic. Biol. Med. 86, 25–36. doi: 10.1016/j.freeradbiomed.2015.04.009
Wang, Y., Guo, L., Jiang, H. F., Zheng, L. T., Zhang, A., and Zhen, X. C. (2016). Allosteric modulation of sigma-1 receptors elicits rapid antidepressant activity. CNS Neurosci. ther. 22, 368–377. doi: 10.1111/cns.12502
Watanabe, S., Ilieva, H., Tamada, H., Nomura, H., Komine, O., Endo, F., et al. (2016). Mitochondria-associated membrane collapse is a common pathomechanism in SIGMAR1- and SOD1-linked ALS. EMBO Mol. Med. 8, 1421–1437. doi: 10.15252/emmm.201606403
Waters, S., Ponten, H., Klamer, D., and Waters, N. (2014). Co-administration of the dopaminergic stabilizer pridopidine and tetrabenazine in rats. J. Huntingtons Dis. 3, 285–298. doi: 10.3233/JHD-140108
Waters, S., Tedroff, J., Ponten, H., Klamer, D., Sonesson, C., and Waters, N. (2018). Pridopidine: overview of pharmacology and rationale for its use in Huntington’s disease. J. Huntingtons Dis. 7, 1–16. doi: 10.3233/JHD-170267
Weng, T. Y., Hung, D. T., Su, T. P., and Tsai, S. A. (2017a). Loss of sigma-1 receptor chaperone promotes astrocytosis and enhances the Nrf2 antioxidant defense. Oxid. Med. Cell Longev. 2017:4582135. doi: 10.1155/2017/4582135
Weng, T. Y., Tsai, S. A., and Su, T. P. (2017b). Roles of sigma-1 receptors on mitochondrial functions relevant to neurodegenerative diseases. J. Biomed. Sci. 24:74. doi: 10.1186/s12929-017-0380-6
Wu, J., Ryskamp, D., Birnbaumer, L., and Bezprozvanny, I. (2018). Inhibition of TRPC1-dependent store-operated calcium entry improves synaptic stability and motor performance in a mouse model of huntington’s disease. J. Huntingtons Dis. 7, 35–50. doi: 10.3233/JHD-170266
Wu, J., Ryskamp, D. A., Liang, X., Egorova, P., Zakharova, O., Hung, G., et al. (2016). Enhanced store-operated calcium entry leads to striatal synaptic loss in a huntington’s disease mouse model. J. Neurosci. 36, 125–141. doi: 10.1523/JNEUROSCI.1038-15.2016
Wu, J., Shih, H. P., Vigont, V., Hrdlicka, L., Diggins, L., Singh, C., et al. (2011). Neuronal store-operated calcium entry pathway as a novel therapeutic target for Huntington’s disease treatment. Chem. Biol. 18, 777–793. doi: 10.1016/j.chembiol.2011.04.012
Wu, Z., and Bowen, W. D. (2008). Role of sigma-1 receptor C-terminal segment in inositol 1,4,5-trisphosphate receptor activation: constitutive enhancement of calcium signaling in MCF-7 tumor cells. J. Biol. Chem. 283, 28198–28215. doi: 10.1074/jbc.M802099200
Xie, Y., Hayden, M. R., and Xu, B. (2010). BDNF overexpression in the forebrain rescues Huntington’s disease phenotypes in YAC128 mice. J. Neurosci. 30, 14708–14718. doi: 10.1523/JNEUROSCI.1637-10.2010
Yamamoto, H., Miura, R., Yamamoto, T., Shinohara, K., Watanabe, M., Okuyama, S., et al. (1999). Amino acid residues in the transmembrane domain of the type 1 sigma receptor critical for ligand binding. FEBS Lett. 445, 19–22. doi: 10.1016/s0014-5793(99)00084-8
Yano, H., Bonifazi, A., Xu, M., Guthrie, D. A., Schneck, S. N., Abramyan, A. M., et al. (2018). Pharmacological profiling of sigma 1 receptor ligands by novel receptor homomer assays. Neuropharmacology 133, 264–275. doi: 10.1016/j.neuropharm.2018.01.042
Yao, H., Duan, M., and Buch, S. (2011). Cocaine-mediated induction of platelet-derived growth factor: implication for increased vascular permeability. Blood 117, 2538–2547. doi: 10.1182/blood-2010-10-313593
Zhai, W., Jeong, H., Cui, L., Krainc, D., and Tjian, R. (2005). In vitro analysis of huntingtin-mediated transcriptional repression reveals multiple transcription factor targets. Cell 123, 1241–1253. doi: 10.1016/j.cell.2005.10.030
Zhang, C. L., Feng, Z. J., Liu, Y., Ji, X. H., Peng, J. Y., Zhang, X. H., et al. (2012). Methylphenidate enhances NMDA-receptor response in medial prefrontal cortex via sigma-1 receptor: a novel mechanism for methylphenidate action. PLoS One 7:e51910. doi: 10.1371/journal.pone.0051910
Zhang, H., and Cuevas, J. (2002). Sigma receptors inhibit high-voltage-activated calcium channels in rat sympathetic and parasympathetic neurons. J. Neurophysiol. 87, 2867–2879. doi: 10.1152/jn.2002.87.6.2867
Zhang, H., Ma, L., Wang, F., Chen, J., and Zhen, X. (2007). Chronic SKF83959 induced less severe dyskinesia and attenuated L-DOPA-induced dyskinesia in 6-OHDA-lesioned rat model of Parkinson’s disease. Neuropharmacology 53, 125–133. doi: 10.1016/j.neuropharm.2007.04.004
Zhang, H., Sun, S., Herreman, A., De Strooper, B., and Bezprozvanny, I. (2010). Role of presenilins in neuronal calcium homeostasis. J. Neurosci. 30, 8566–8580. doi: 10.1523/JNEUROSCI.1554-10.2010
Zhang, H., Sun, S., Wu, L., Pchitskaya, E., Zakharova, O., Fon Tacer, K., et al. (2016). Store-operated calcium channel complex in postsynaptic spines: a new therapeutic target for alzheimer’s disease treatment. J. Neurosci. 36, 11837–11850. doi: 10.1523/jneurosci.1188-16.2016
Zhang, H., Wu, L., Pchitskaya, E., Zakharova, O., Saito, T., Saido, T., et al. (2015). Neuronal store-operated calcium entry and mushroom spine loss in amyloid precursor protein knock-in mouse model of alzheimer’s disease. J Neurosci. 35, 13275–13286. doi: 10.1523/JNEUROSCI.1034-15.2015
Zhang, K., Zhao, Z., Lan, L., Wei, X., Wang, L., Liu, X., et al. (2017). Sigma-1 receptor plays a negative modulation on n-type calcium channel. Front. Pharmacol. 8:302. doi: 10.3389/fphar.2017.00302
Keywords: synapse, calcium, neuroprotection, Alzheimer’s, Huntington and Parkinson diseases, ALS (amyotrophic lateral sclerosis)
Citation: Ryskamp DA, Korban S, Zhemkov V, Kraskovskaya N and Bezprozvanny I (2019) Neuronal Sigma-1 Receptors: Signaling Functions and Protective Roles in Neurodegenerative Diseases. Front. Neurosci. 13:862. doi: 10.3389/fnins.2019.00862
Received: 23 May 2019; Accepted: 31 July 2019;
Published: 28 August 2019.
Edited by:
Tangui Maurice, INSERM U1198 Mécanismes Moléculaires dans les Démences Neurodégénératives, FranceReviewed by:
Wladyslaw-Lason, Institute of Pharmacology, Polish Academy of Sciences, PolandTomohisa Mori, Hoshi University, Japan
Copyright © 2019 Ryskamp, Korban, Zhemkov, Kraskovskaya and Bezprozvanny. This is an open-access article distributed under the terms of the Creative Commons Attribution License (CC BY). The use, distribution or reproduction in other forums is permitted, provided the original author(s) and the copyright owner(s) are credited and that the original publication in this journal is cited, in accordance with accepted academic practice. No use, distribution or reproduction is permitted which does not comply with these terms.
*Correspondence: Ilya Bezprozvanny, SWx5YS5CZXpwcm96dmFubnlAdXRzb3V0aHdlc3Rlcm4uZWR1