- 1Department of Translational Research and of New Surgical and Medical Technologies, University of Pisa, Pisa, Italy
- 2Department of Electrical, Electronic and Information Engineering “Guglielmo Marconi,” University of Bologna, Cesena, Italy
- 3Department of Radiology, Versilia Hospital, Azienda USL Toscana Nord Ovest, Camaiore, Italy
- 4Orthopedics Unit, St. Barth Hospital, Sarzana, Italy
- 5Department of Orthopedics, University of Genoa, Genoa, Italy
- 6Institut des Maladies Neurodégénératives, Université de Bordeaux, Bordeaux, France
- 7Department of Developmental Neuroscience, IRCCS Fondazione Stella Maris, Pisa, Italy
In order to assess possible influences of occlusion on motor performance, we studied by functional magnetic resonance imaging (fMRI) the changes in the blood oxygenation level dependent (BOLD) signal induced at brain level by a finger to thumb motor task in a population of subjects characterized by an asymmetric activation of jaw muscles during clenching (malocclusion). In these subjects, appropriate occlusal correction by an oral orthotic (bite) reduced the masticatory asymmetry. The finger to thumb task was performed while the subject’s dental arches were touching, in two conditions: (a) with the teeth in direct contact (Bite OFF) and (b) with the bite interposed between the arches (Bite ON). Both conditions required only a very slight activation of masticatory muscles. Maps of the BOLD signal recorded during the movement were contrasted with the resting condition (activation maps). Between conditions comparison of the activation maps (Bite OFF/Bite ON) showed that, in Bite OFF, the BOLD signal was significantly higher in the trigeminal sensorimotor region, the premotor cortex, the cerebellum, the inferior temporal and occipital cortex, the calcarine cortex, the precuneus on both sides, as well as in the right posterior cingulate cortex. These data are consistent with the hypothesis that malocclusion makes movement performance more difficult, leading to a stronger activation of (a) sensorimotor areas not dealing with the control of the involved body part, (b) regions planning the motor sequence, and (c) the cerebellum, which is essential in motor coordination. Moreover, the findings of a higher activation of temporo-occipital cortex and precuneus/cingulus, respectively, suggest that, during malocclusion, the movement occurs with an increased visual imagery activity, and requires a stronger attentive effort.
Introduction
There is a debate as to whether trigeminal sensorimotor signals may affect posture and movement. In particular, postural control seems to be influenced by the occlusal condition (Ohlendorf et al., 2014; Julià-Sánchez et al., 2015; see, however, Perinetti, 2006), temporomandibular joint (TMJ) disorders (Chaves et al., 2014) and orofacial motor activity (Kushiro and Goto, 2011; Ringhof et al., 2015). These data are consistent with the observation that sensorimotor signals elicited during isometric clenching are very effective in increasing the excitability of spinal motoneurons (Miyahara et al., 1996). On the other hand, correction of TMJ disorders by splints does not seem to affect motor performance (McArdle et al., 1984), while there is evidence that changes in the occlusal condition may affect locomotion (Okubo et al., 2010; Maurer et al., 2015) and the associated postural stabilization in normal subjects (Ohlendorf et al., 2014), as well as the overall motility in Parkinsonian patients (Nomoto et al., 2013). Finally, recent evidence indicates that in subjects showing an unbalance in sensorimotor orofacial activity (consisting in an asymmetric activation of left and right masseter muscles during clenching) the reestablishment of a symmetric condition by bite wearing enhances the speed of execution in a complex sensorimotor task (De Cicco et al., 2016).
If the occlusal condition does affect body movements, it is reasonable to assume that it will also modify also the movement-elicited local changes in cerebral blood. So far, the effects of occlusal condition on the movement-elicited changes in cerebral blood flow have been investigated only for orofacial movements. In particular, wearing a splint during jaw tapping movements decreased bilaterally the blood oxygenation level dependent (BOLD) signal in the primary and secondary sensorimotor areas, putamen, inferior parietal/prefontal cortex and anterior insula, thus indicating a decrease in the blood flow to these regions during the task. At variance, the BOLD signal increased in the prefrontal, temporal, parietal, and occipital lobes, as well as in the cerebellar network (Lotze et al., 2012).
The purpose of the present investigation was to document whether correction of an unbalanced occlusal condition may modify the pattern of brain activation induced by finger movements. For this purpose, we have analyzed, in a group of subjects showing an unbalance in sensorimotor orofacial activity during clenching, the changes in the BOLD signal elicited by a typical finger to thumb task (Shibasaki et al., 1993) and their modulation by occlusal correction.
Materials and Methods
Subjects
Eight subjects (4 females) of age between 49 and 69 were enrolled in the study. At maximal jaw opening, clicking sounds were present in all the subjects and two of them were also reporting pain. In two subjects, clicks appeared also at the beginning of jaw lowering. None of them was affected by neurological or psychiatric disorders. Subjects were asked to avoid caffeine containing drinks and smoking at least 2 h prior the experimental session. The study was carried out in accordance with the recommendations of the Ethical Committee of the University of Pisa. According to the Declaration of Helsinki, each subject signed an informed consent, approved by the local Ethical Committee.
MR Acquisition Protocol
All MR exams were performed using a 1.5T MR system (MAGNETOM Avanto, Siemens Healthcare, Erlangen, Germany) equipped with 45 mT/m gradients and a quadrature head coil.
The examination protocol included (a) axial high resolution contiguous 3D T1-weighted images that were obtained with a MPRAGE sequence [repetition time (TR) = 1.900 ms, echo time (TE) = 3.4 ms, inversion time (TI) = 1100 ms, flip angle = 15°, slice thickness = 0.9 mm, field of view (FOV) = 256 mm × 256 mm, matrix size = 256 × 256, number of excitations (NEX) = 2] and (b) axial T2-weighted images that were obtained with a fluid attenuated inversion recovery (FLAIR) sequence (TR = 9.000 ms, TE = 88 ms, TI = 2.500 ms, slice thickness = 3 mm, FOV = 230 mm × 172.5 mm, matrix size = 256 × 154, turbo factor = 16, NEX = 1). Functional images were collected using a T2∗- weighted echo-planar imaging (EPI) sequence (TR = 3000 ms, TE = 50 ms, FA = 90, slice thickness = 4 mm; FOV = 256 mm × 256 mm, matrix size 64 × 64; number of slices = 37). The slices were oriented along and parallel to the bi-commissural plane and centered to cover the entire brain.
The task consisted of a self-paced continuous finger to thumb task, performed with the right hand, without visual feedback, that the subjects, laying supine within the scanner, repeated before data acquisition until they were able to perform correctly. The subjects were visually monitored throughout the scanning time and no gross change in performance could be observed.
The functional magnetic resonance imaging (fMRI) sequences were acquired while the subjects performed the task while their dental arches were touching in two different conditions: (a) with the teeth in direct contact (Bite OFF) and (b) with an orthotic (bite) interposed between the arches (Bite ON). Both conditions required only a very slight activation of masticatory muscles. A block design was used, where four blocks of finger to thumb task (T) were alternated with four blocks of rest (R) according to the sequence RTRTRTRT, for each condition. Start and end of each block were instructed by delivering “go” and “stop” auditory signals. Each block, in which 10 scans were acquired, lasted 30 s, for a total of 80 scans/run in 4 min. Before each run, two dummy volumes were acquired and discarded from analysis to avoid T1-related relaxation effects. The first condition tested (Bite OFF or Bite ON) was changed from subject to subject in pseudorandom order. In order to minimize head movements, head pads and forehead straps were used. The subjects were visually monitored throughout the scanning time also for non-task related movements.
fMRI Data Analysis
Functional data were analyzed using FSL 4.1.4 software [Oxford Center for Functional Magnetic Resonance Imaging of the Brain (FMRIB) software library1], one of the two major and most widely employed neuroimaging analysis tools (Jenkinson et al., 2012). The following pre-statistics processing were applied to the 80 fMRI scans: motion correction using MCFLIRT (Jenkinson et al., 2002), non-brain removal using BET (Smith, 2002), spatial smoothing using a 8 mm FWHM Gaussian kernel, grand-mean intensity normalization of the entire 4D data set by a single multiplicative factor and high-pass temporal filtering. For each subject, the mean (across voxels) voxel absolute displacement (each time point with respect to the reference image, i.e., to the middle-time point rsfMRI image) calculated by MCFLIRT was less than 1 mm (equal to 1/4 the voxel dimension) and, for this reason, none of the subjects was excluded for excessive head motion. The high resolution T1-weighted images were co-registered in a standard space [Montreal Neurological Institute (MNI) 152 Brain]. This allowed assessment of activation areas in terms of the MNI coordinate system. Registration of EPI functional images to the individual high resolution T1-weighted image and standard space was carried out using affine transformation with 12 degrees of freedom (Jenkinson and Smith, 2001). Registration from high resolution structural to standard space was then further refined using FNIRT non-linear registration (Andersson et al., 2007a,b). The FMRIB’s improved linear model (Woolrich et al., 2001) was adopted for statistical analysis in order to determine the activation maps of signal changes between active vs. rest periods. Single-subject cluster analysis was performed on voxels having Z (Gaussianised T) > 3.1 and a (cluster-based corrected) significance threshold of p = 0.05 (Worsley, 2001). In each Bite ON and the Bite OFF condition, a within-group analysis was carried out using one-sample t-test to assess the differences between the active and passive blocks and a fixed effects model using FLAME (FMRIB’s Local Analysis of Mixed Effects) (Beckmann et al., 2003; Woolrich et al., 2004) by forcing the random effects variance to zero (Woolrich, 2008). The Z-statistical maps derived from the within-group analyses underwent a cluster thresholding with a Z threshold of 3.1 and a cluster p threshold of 0.05.
To assess possible differences in the BOLD activation pattern between the Bite ON and Bite OFF condition, a between-conditions analysis was carried out using paired t-test and a fixed effects model. Z (Gaussianised T/F) statistic images were thresholded using clusters determined by Z > 3.1 and a (corrected) cluster significance threshold of p = 0.05. We inserted the subject’s age as covariate variable in both within-group and within-condition models.
The coordinates of the local activation maxima observed in the present study within the clusters with a significant condition effect were inserted in the Brain Map Sleuth2 and in the NeuroSynth Database3, so to retrieve similarly localized activations reported in previously studies during different sensorimotor and cognitive tasks. The Yale Brain Map4 and the automated anatomical labeling (AAL) atlas (Tzourio-Mazoyer et al., 2002) were utilized in order to define the position of the local activation maxima within the Brodmann areas (BA) and within the different cortical/subcortical/cerebellar regions, respectively.
Electromyographic Evaluation and Cusp Bite Manufacturing
The evaluation of the electromyographic (EMG) activity of jaw muscles during clenching was performed in a separate session, before fMRI acquisition. Only subjects with an asymmetry in EMG activity [200(EMGleft-EMGright)/(EMGleft+EMGright)] higher than 15% were enrolled in the study. They were submitted to a transcutaneous electrical nerve stimulation (TENS) of trigeminal motor branches (Noaham and Kumbang, 2008) for 15 min. Jaw muscles stimulation was made by means of four couples (cathode/anode) of electrodes (1600 mm2 of surface) positioned on both side at the level of incisura sigmoidea and of the submental region. Repeated contractions of masseters and mandible depressor muscles were obtained by means of biphasic (cathodal/anodal) current pulses (0.54 ms duration, 21–25 mA intensity), delivered by two I.A.C.E.R. stimulators (Martellago, Venice, Italy). The intensity of the left and right stimuli was adjusted in order to obtain a symmetric muscle activation (evaluated by EMG recording), while the frequency corresponded to 40 and to 0.618 Hz for mandible depressor and elevator muscles, respectively. These patterns led to an alternated contraction/relaxation of masseters and to a tonic contraction of depressor muscles, resulting in small amplitude mandibular movements (1 mm). Following TENS, the mandibular resting posture was lowered and a dental impression was obtained in the new relative position of dental arches by placing a self-hardening material between them. This dental impression was used to manufacture a cusp bite (Dao et al., 1994) modeled on the inferior dental arch. Cusp bite placement reduced the myoelectric asymmetry observed during clenching (Figure 1), which decreased to less than 15% in all the subjects.
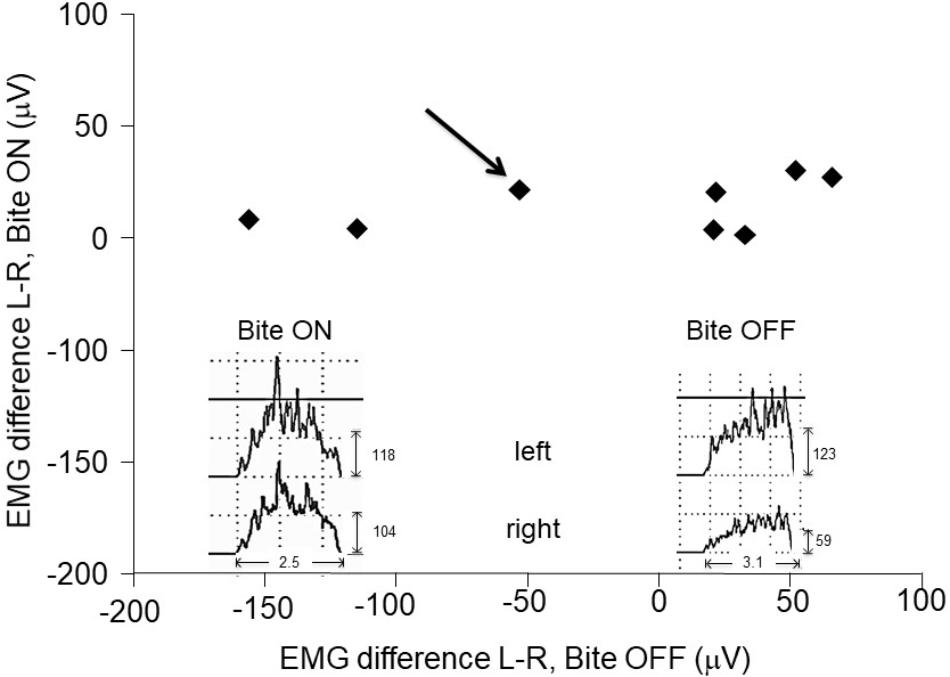
Figure 1. Asymmetries in EMG activity: effects of the bite. Scatter plot of left-right differences observed in masseter in EMG activity during clenching with (Bite ON, in ordinates) and without (Bite OFF, in abscissas) occlusal correction by bite wearing. The insets represent the EMG raw data recorded in a representative subject (indicated by the black arrow). Note the narrow range of the EMG asymmetry following occlusal correction.
Comparison of EMG activities during clenching in Bite ON and Bite OFF was performed by paired t-test.
Results
EMG Asymmetries
During biting with normal (uncorrected) occlusion (Bite OFF), all subjects showed an evident left-right asymmetry in the activity of the masseter (see Figure 1), whose absolute value corresponded to 64.3 ± 49.2 μV (mean ± SD of the difference between the two sides). As shown in Figure 1, wearing a cusp bite (Bite ON) reduced the asymmetry in EMG activity, which reached, on the average, the value of 12.3 ± 4.13 μV (paired t-test, p < 0.022). The reduction in the EMG asymmetry was due to a significant increase in the activity of the hypotonic masseter muscle, which raised from 35.8 ± 27.1 to 70.1 ± 23.6 μV (paired t-test, p < 0.009) joined to a non-significant activity decrease on the hypertonic side (from 100.0 ± 56 to 73.1 ± 30.1 μV: paired t-test, p < 0.101).
Comparison Between Bite ON and Bite OFF Conditions
In both Bite OFF and Bite ON conditions, the finger to thumb task modified significantly the BOLD signal with respect to rest in several cortical and subcortical structures. As shown in Figure 2, within the different regions recruited by the task the extent of activated voxels was larger in Bite OFF with respect to Bite ON.
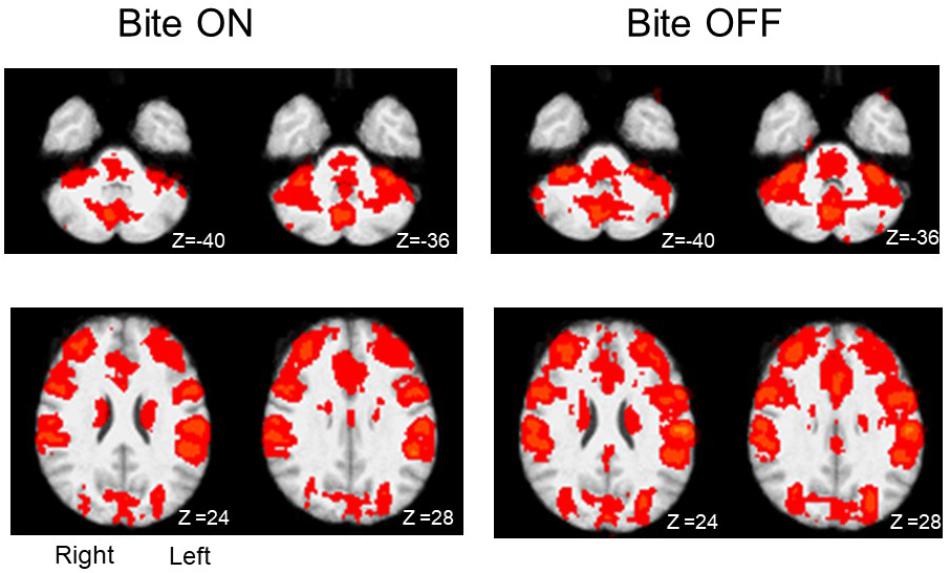
Figure 2. Activation maps (task-rest) obtained during finger to thumb task in Bite ON and Bite OFF conditions. The red areas indicate regions characterized by significant activation during finger to thumb task in Bite ON and Bite OFF conditions. Right and left side are indicated in the section at Z 24, in the Bite ON column (the images are depicted from a bottom view). Upper row: cerebellum. Lower row: regions around the central sulcus. Z coordinates in mm in the MNI standard space are indicated under each section.
Comparison of activation maps (task minus resting condition) obtained in Bite OFF and Bite ON indicated the presence of clusters of voxels showing a significantly higher task-related activation in Bite OFF, while the opposite behavior has never been observed. All these clusters are shown in Figure 3, while the MNI coordinates of the corresponding centers of gravity (GOGs) are indicated in Table 1, together with the number of significant voxels. Data about the localization of the local activation maxima are given in Table 2 for each of the significant clusters. Three clusters (two on the right and one on the left side) were located in the lower part of the precentral gyrus, in an area corresponding to the trigeminal sensorimotor region (Penfield and Boldrey, 1937). An additional cluster lied within the post central gyrus, at the level of the body somatotopic area above the hand region. In the precentral gyrus, the clusters included BA 4 and 6, while postcentral activation interested the primary somatosensory region and BA 40 on the right side.
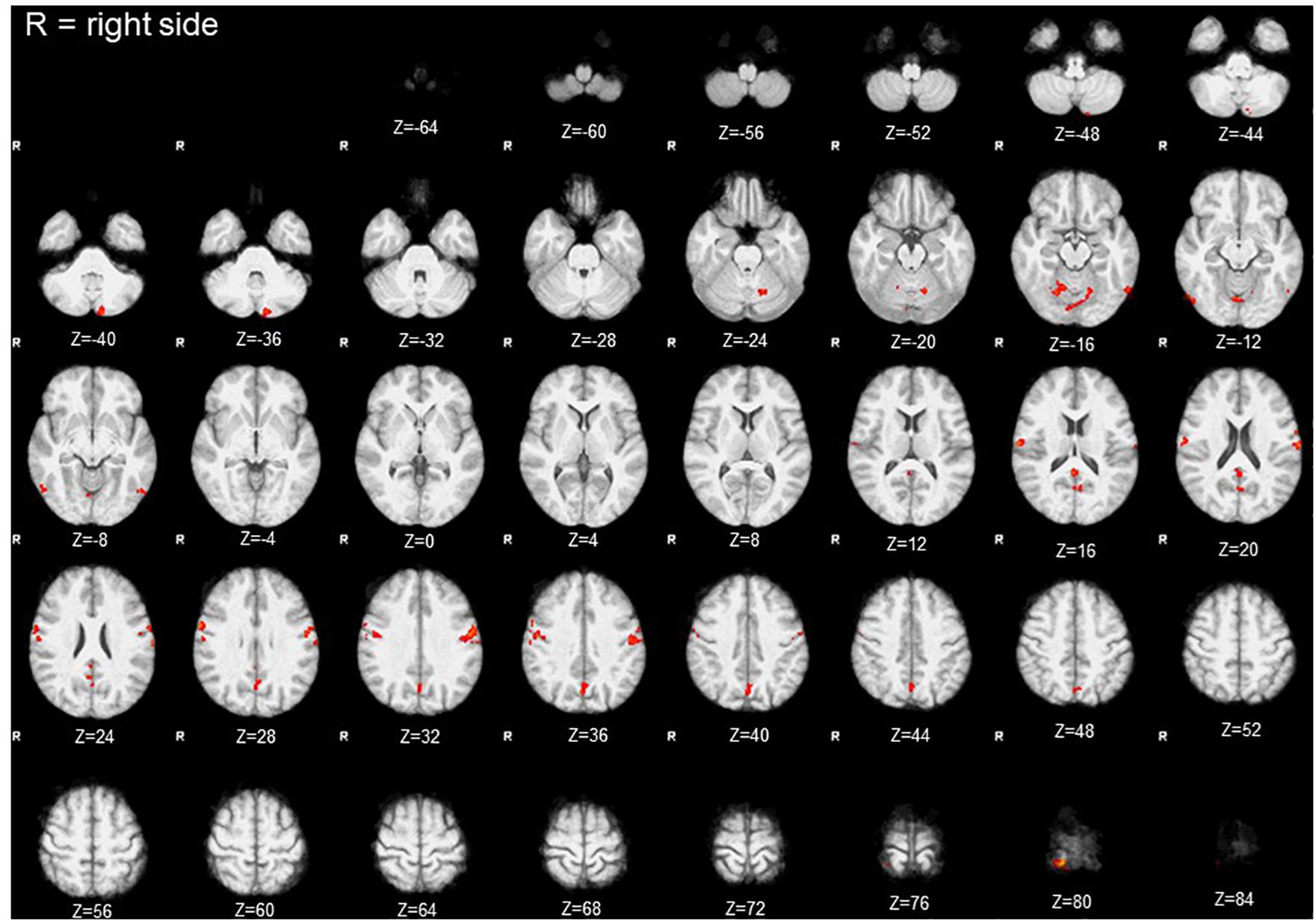
Figure 3. Clusters with significant condition effect (Bite OFF>Bite ON). Red areas indicate clusters with significant differences between the activations maps (task-rest) obtained in Bite OFF and Bite ON conditions during finger to thumb task. The letter R indicates the right side (the images are depicted from a bottom view). Z coordinates in mm in the MNI standard space are indicated under each section.
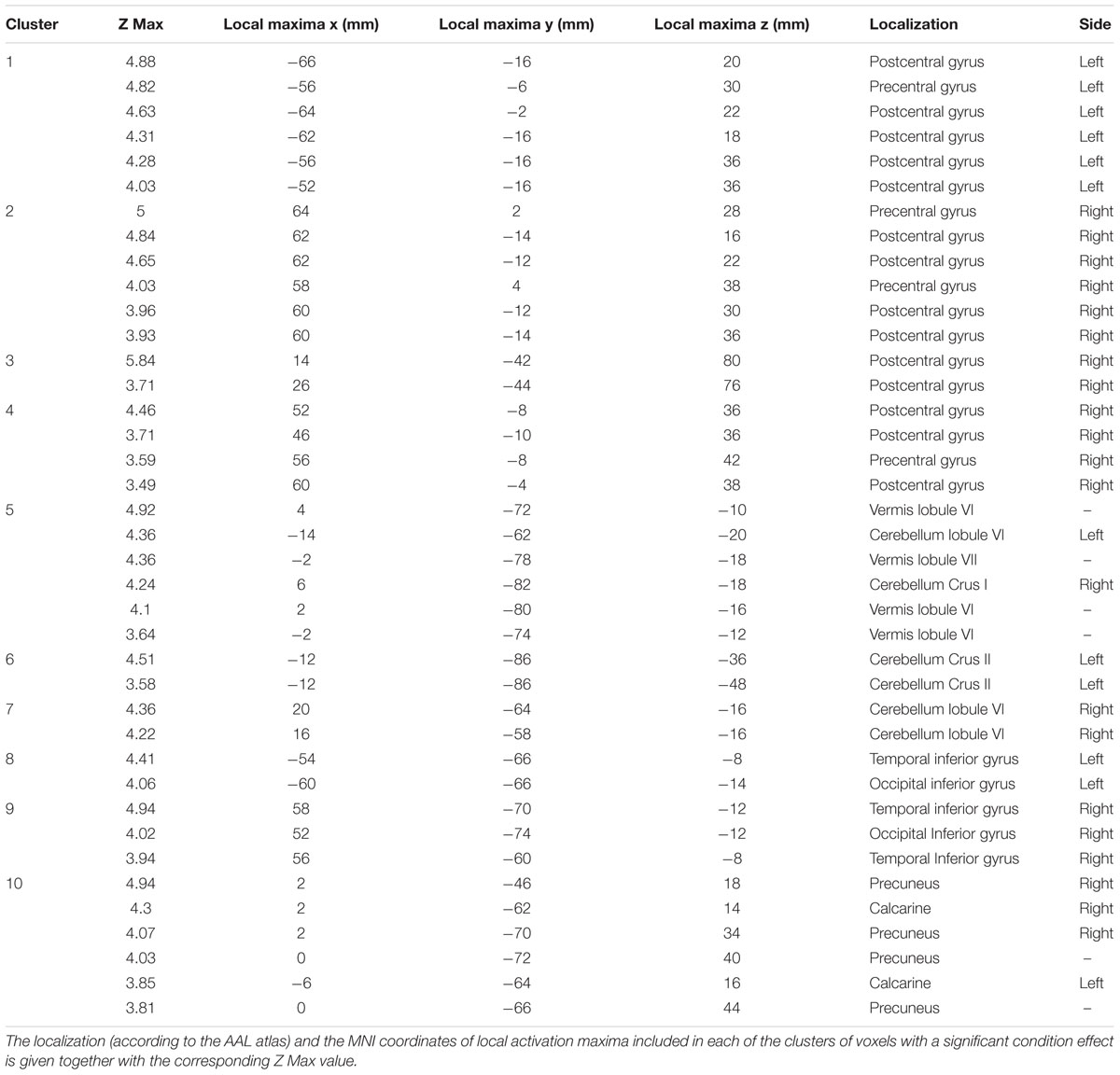
Table 2. Coordinates and localization of local activation maxima observed within each of the ten clusters showing a significant condition effect.
Within the cerebellum, three clusters were observed. One of them was centered in paravermal region of the lobule VI on the right side. The second one, was limited to Crus II in the left hemisphere. Finally, the third cluster encompassed the vermal cortex of lobule VI and, to some extent of lobule VII, as well as the paravermal cortex of Crus I on the right side and that of lobule VI on the left.
Finally, two roughly symmetrical clusters could be found at the level of right and left inferior temporal/occipital lobes, while, caudally on the inner brain surface, a cluster of significant voxel extended bilaterally from the calcarine cortex down to the precuneus, expanding in the posterior cingulate region of the right side.
Discussion
Difference in BOLD Signal Between Bite OFF and Bite ON: General Considerations
The present data indicate that the increase in cerebral blood flow elicited by a motor task involving the fingers can be influenced by the occlusal condition. In fact, when subjects wore a bite that balanced the occlusion, the increase in BOLD signal elicited by finger movements was significantly reduced at the level of frontoparietal sensorimotor regions, cerebellum, temporo-occipital, and midline posterior regions.
So far the effect of malocclusion was tested only on jaw-related brain activation, which was enhanced (Lotze et al., 2012), similarly to what observed for hand movements-related activation in the present study. It is known that cortical activation decreases with increasing skill and automaticity (see Saling and Phillips, 2007, for ref) of the performed movement, probably due to a higher coupling between sensorimotor regions (Wu et al., 2008). So, a higher Bite OFF activation indicates that malocclusion may be detrimental to motor performance, imposing a higher attentive effort (see Wu et al., 2008) and, possibly, leading to a decoupling of the areas involved in the task.
Fronto-Parietal Sensorimotor Regions
As shown in Table 2, three clusters (1,2,4) were located in the fronto-parietal sensorimotor regions of both sides. They largely overlapped with the trigeminal regions of the primary motor and somatosensory cortex, which have been defined by stimulation experiments in humans (Penfield and Boldrey, 1937) and show activation during different types of orofacial sensorimotor and speaking tasks (Watanabe et al., 2004; Grabski et al., 2012; Iida et al., 2012; Lotze et al., 2012; Quintero et al., 2013). Since the cortical activation elicited by movement of a given body part spreads outside of the corresponding sensorimotor representation (Stippich et al., 2007), the larger activation of the sensorimotor trigeminal region in Bite OFF could be to a less selective recruitment of somatotopic map and/or to an increased recruitment of inhibitory interneurons which suppress the output of the regions inappropriate for the motor task in execution. In the former case, the higher activation in Bite OFF observed within the primary somatosensory cortex could be due to (a) a stronger orofacial input elicited by activation of masticatory muscles during finger movements and/or (b) to a stronger reactivation by efference copies from the trigeminal motor region (Cui et al., 2014).
Sensorimotor clusters extend from the primary motor region (BA 4) to BA 6 that, in monkey, controls not only orofacial, but also finger movements (Rizzolatti et al., 1988). If this region has the same function in monkeys and humans, its higher activation in Bite OFF could reflect a more difficult planning of the finger to thumb sequence. Indeed, fMRI experiments in humans have shown that regions overlapping with the significant clusters in BA 6 are activated during planning and execution of finger and hands movements (Jankowski et al., 2009; Plata Bello et al., 2015).
Postcentral clusters extend into the right parietal associative cortex (area 40), whose lesion impairs language function in humans (Sakurai et al., 2010). However, networks located in the corresponding areas of the brain monkey are involved in the control of finger movements (Vingerhoets, 2014). If this is the case also in humans, the higher activation of these regions in Bite OFF condition could reflect a higher attentive cost and a reduced skill of the performed finger movements (see Saling and Phillips, 2007). This hypothesis is in agreement with the fact that, in humans, these areas are activated not only during orofacial (Watanabe et al., 2004; Quintero et al., 2013) but also during hand sensorimotor activities, such as imagery and execution of hand movements (de Vries et al., 2009) and active tactile discrimination (Stoeckel et al., 2003).
A fourth cluster (cluster 2, see Table 1) was confined to the trunk-arm region (Penfield and Boldrey, 1937) of the primary somatosensory cortex and to the neighboring BA 5 and 7. Its higher activation in Bite OFF could reflect a higher feedback from the trunk and arm during finger movements, possibly due a less focalized postural adjustment (Caronni and Cavallari, 2009).
Cerebellar Structures
Within the cerebellum a significant cluster included the vermal cortex of lobules VI and VII, together with the paravermal cortex of right lobule VI and left Crus I. A second cluster was formed by left Crus II and a third one by the paravermal cortex of the right lobule VI. It is known that in humans, lobule VI is connected with the motor and premotor cortical regions, lobule VII with parietal and prefrontal regions while Crus I and Crus II with the lateral prefrontal cortex (Stoodley and Schmahmann, 2018). In all these regions, during finger to thumb task, the Bite OFF condition requires a higher cerebellar involvement for correctly driving fronto-parietal circuits. Since the cerebellum is particularly involved in motor coordination (Manto and Bastian, 2007), this increased activation is in agreement with the hypothesis that movement performance deteriorates in Bite OFF imposing an heavier computational burden on cerebellar circuits (Wu et al., 2008).
fMRI studies indicate that these cerebellar areas are activated during orofacial sensorimotor activity (Eldeghaidy et al., 2011; Thürling et al., 2011; Wong et al., 2011; Grabski et al., 2012; Quintero et al., 2013; Shoi et al., 2014), hand movements planning and execution (Jankowski et al., 2009; Callan et al., 2012), as well as during sensory and cognitive performances that may take place during the finger to thumb task, with particular reference to its spatial and temporal aspects (Diedrichsen et al., 2006; Majerus et al., 2007; O’Reilly et al., 2008; Grahn and McAuley, 2009; Mullally and Maguire, 2011; Brown and Stern, 2014; Onuki et al., 2015).
In summary, the higher cerebellar activation observed during Bite OFF can be the expression of the need of a higher coordinative and planning effort; moreover, some of the trigeminal related regions such as left and right lobule VI may be sensitive to the higher activity of the trigeminal sensorimotor cortical regions to which they are coupled.
Temporooccipital Regions
As shown in Table 2, two significant clusters were located at the inferior temporal/occipital cortex of both sides. These areas belong to the “ventral streaming” regions, where neuronal activation leads to recognition of the seen objects and persons (Goodale, 1998). fMRI studies are consistent with these findings (Ardila et al., 2016). On these basis, it could be proposed that, during a finger to thumb task performed in Bite OFF, subjects have to produce a larger imagery effort in order to perform the movement, leading to a higher activation of structures involved in visual image recognition. According to this hypothesis, significant clusters described in the present study are activated during visual imagery (Nakao et al., 2011), visual memory load (Rahm et al., 2014), but also during visuospatial processing (Grabner et al., 2009; King and Miller, 2014), hand movement (Macaluso et al., 2007), trajectory prediction (Olsson and Lundström, 2013) and discomfort perceptions (Ogino et al., 2007). Finally, on the left side, activity has been observed also during clenching (Wong et al., 2011). All these activations might be justified by recruitment of circuits related to the visual recognition of actual/imaged objects and body parts during specific behaviors, such as the finger to thumb task.
Midline and Limbic Structures
Significant clusters were found bilaterally on the inner faces of the hemispheres, from the calcarine cortex to the precuneus, with an extension into the right posterior cingulate gyrus. The calcarine cortex represents visual processing area (Ffytche and Catani, 2005) and its larger activation during Bite OFF suggests that, in this condition, a higher imaginative effort occurs during finger movements. The precuneus (Cavanna and Trimble, 2006) is one of the brain regions more active at rest and contribute to the so-called “default mode of brain function” (DMF) (Raichle et al., 2001): these structures are hypoactive in conditions of reduced or abolished consciousness, such as sleep, pharmacological sedation and vegetative state and are likely contributing to overall alertness and attention (see Cavanna and Trimble, 2006, for ref.). Accordingly, the precuneus regions enlightened in the present study changes are activated during many sensorimotor and cognitive operations, such as eye movements (Simon et al., 2002), pointing (Astafiev et al., 2003), planning of finger movements (Jankowski et al., 2009), orofacial movement execution and learning (Arima et al., 2011), covert shift of attention to relevant spatial locations (Nagahama et al., 1999; Beauchamp et al., 2001), action observation and execution of observed actions (Chaminade et al., 2005), motor imagery (Ogiso et al., 2000; Malouin et al., 2003), visual memory tasks (Suchan et al., 2002), rhythm generation (Stevens et al., 2007). We may propose that, during malocclusion the performance of a sequence of finger displacements requires a higher attentive effort, possibly related to the spatial shift of the moving fingers and to the development of their visual image.
Significant clusters were also found in the posterior cingulate cortex on the right side. This region belong to DMF and is strongly coupled to the precuneus: its level of activation seems to increase with the level of arousal (Leech and Sharp, 2014). It has been proposed that it is involved in internally directed mental activity, in controlling the balance between internal and external attention and in the detection of environmental changes. The more simple explanation of the higher posterior cingulate activation during movement in Bite OFF is that this condition requires a higher mental and attentive effort. In effect, the region corresponding to the significant cluster in the posterior cingulate region has been also implicated in rhythm generation (Stevens et al., 2007), motor imagery (Malouin et al., 2003), planning of finger movements (Jankowski et al., 2009) and eye movements (Alvarez et al., 2010).
Final Considerations
Why should a trigeminal unbalance lead to a cortical activation pattern indicating a larger effort in task performance and in the associated processes? A possibility is that the asymmetric discharge of muscle spindles, periodontal and, possibly, TMJ receptors induced by malocclusion leads to an unbalance in hemispheric excitability. There is indeed evidence that a hemispheric unbalance may deteriorate neural functions (Lomber and Payne, 1996). Moreover, unilateral stimulation of sensory afferents may relapse the symptoms induced by asymmetric brain lesions (Vallar et al., 1993). What could be the pathways involved in the postulated tonic control of trigeminal afferents on brain excitability? Neurons in the mesencephalic trigeminal nucleus are chemically (Luo et al., 1991) and, possibly, electrically (Fujita et al., 2012) coupled with the noradrenergic locus coeruleus (LC) neurons, which project to the whole brain (Samuels and Szabadi, 2008), controlling sensorimotor and cognitive processes (Berridge and Waterhouse, 2003). So, an unbalance in trigeminal afferents may leads to an unbalance in LC activity and, as a consequence, in brain excitability, since LC projections show an ipsilateral dominance. According to this hypothesis, it has been documented that trigeminal unbalance associated to malocclusion leads to an asymmetry in pupil size (De Cicco et al., 2014, 2016), which is a reliable indicator of LC discharge (Kihara et al., 2015), while occlusal correction reduces both trigeminal and pupil size asymmetry (De Cicco et al., 2014, 2016) and boost performance in complex sensorimotor tasks (De Cicco et al., 2016).
We must acknowledge that these results were obtained on a limited number of subjects and this can lower the statistical power of the study (Costafreda, 2009) and prevent a further generalization to a random population. A low statistical power increases the risk of a type II error and lowers the ability of discriminating whether non-significant results are due to a true absence of the hypothesized effect or to a limited number of subjects with respect to their standard deviation (Mumford, 2012). However, the occurrence of significant differences within several brain regions between Bite ON and OFF conditions in spite of the small number of subjects analyzed, suggests that the occlusal condition modulates brain activation elicited by skilled fingers movements and prompt the use of the Bite ON/Bite OFF paradigm for further investigate the issue in a larger population.
Ethics Statement
This study was carried out in accordance with the recommendations of the Ethical Committee of the University of Pisa with written informed consent from all subjects. All subjects gave written informed consent in accordance with the Declaration of Helsinki. The protocol was approved by the Ethical Committee of the University of Pisa.
Author Contributions
MPTF screened available databanks of brain activation and wrote parts of results and discussion. SD performed analysis of fMRI data, providing the task-rest BOLD signal maps, writing the Methods section and contributing to results discussion. VDC ideated the project, performed the odonthoiatric evaluations and corrections and contributed to results discussion. DM supervised the experiments and wrote parts of results and discussion. CT performed fMRI signals acquisition and participated in writing the Methods and discussing results. BC, DC, and GR contribute to odonthoiatric evaluations and corrections. MB contributed to results discussion and writing the paper. CV contributed to results discussion. UF revised the paper, made major changes to data analysis, interpretation and discussion.
Funding
This work was supported by the “Ministero della Salute” grant no. GR-2011-02348998 and by grants of the University of Pisa. MPTF was supported by a fellowship of the I.A.C.E.R. S.r.l. Company (Martellago, Venice, Italy). The funders had no role in study design, data collection and analysis, decision to publish, or preparation of the manuscript.
Conflict of Interest Statement
The authors declare that the research was conducted in the absence of any commercial or financial relationships that could be construed as a potential conflict of interest.
Acknowledgments
We thank Mr. P. Orsini, Mr. F. Montanari, and Mrs. Cristina Pucci for valuable technical assistance.
Footnotes
- ^ http://www.fmrib.ox.ac.uk/fsl
- ^ http://brainmap.org/sleuth
- ^ http://neurosynth.org
- ^ http://sprout022.sprout.yale.edu/mni2tal/mni2tal.html
References
Alvarez, T. L., Alkan, Y., Gohel, S., Douglas Ward, B., and Biswal, B. B. (2010). Functional anatomy of predictive vergence and saccade eye movements in humans: a functional MRI investigation. Vis. Res. 50, 2163–2175. doi: 10.1016/j.visres.2010.08.018
Andersson, L. R., Jenkinson, M., and Smith, S. M. (2007a). Non-Linear Optimisation. FMRIB Technical Report No. TR07JA1.
Andersson, L. R., Jenkinson, M., and Smith, S. M. (2007b). Non-Linear Registration, Aka Spatial Normalization. FMRIB Technical Report No. TR07JA2.
Ardila, A., Bernal, B., and Rosselli, M. (2016). How localized are language brain areas? A review of brodmann areas involvement in oral language. Arch. Clin. Neuropsychol. 31, 112–122. doi: 10.1093/arclin/acv081
Arima, T., Yanagi, Y., Niddam, D. M., Ohata, N., Arendt-Nielsen, L., Minagi, S., et al. (2011). Corticomotor plasticity induced by tongue-task training in humans: a longitudinal fMRI study. Exp. Brain Res. 212, 199–212. doi: 10.1007/s00221-011-2719-7
Astafiev, S. V., Shulman, G. L., Stanley, C. M., Snyder, A. Z., Van Essen, D. C., and Corbetta, M. (2003). Functional organization of human intraparietal and frontal cortex for attending, looking, and pointing. J. Neurosci. 23, 4689–4699. doi: 10.1523/jneurosci.23-11-04689.2003
Beauchamp, M. S., Petit, L., Ellmore, T. M., Ingeholm, J., and Haxby, J. V. (2001). A parametric fMRI study of overt and covert shifts of visuospatial attention. Neuroimage 14, 310–321. doi: 10.1006/nimg.2001.0788
Beckmann, C. F., Jenkinson, M., and Smith, S. M. (2003). General multilevel linear modeling for group analysis in FMRI. Neuroimage 20, 1052–1063. doi: 10.1016/S1053-8119(03)00435-X
Berridge, C. W., and Waterhouse, B. D. (2003). The locus coeruleus-noradrenergic system: modulation of behavioral state and state-dependent cognitive processes. Brain Res. Brain Res. Rev. 42, 33–84. doi: 10.1016/s0165-0173(03)00143-7
Brown, T. I., and Stern, C. E. (2014). Contributions of medial temporal lobe and striatal memory systems to learning and retrieving overlapping spatial memories. Cereb. Cortex 24, 1906–1922. doi: 10.1093/cercor/bht041
Callan, D. E., Gamez, M., Cassel, D. B., Terzibas, C., Callan, A., Kawato, M., et al. (2012). Dynamic visuomotor transformation involved with remote flying of a plane utilizes the ‘Mirror Neuron’ system. PLoS One 7:e33873. doi: 10.1371/journal.pone.0033873
Caronni, A., and Cavallari, P. (2009). Supra-spinal circuits shape inhibitory postural adjustments anticipating voluntary index-finger flexion. Exp. Brain Res. 198, 19–28. doi: 10.1007/s00221-009-1931-1
Cavanna, A. E., and Trimble, M. R. (2006). The precuneus: a review of its functional anatomy and behavioural correlates. Brain 129, 564–583. doi: 10.1093/brain/awl004
Chaminade, T., Meltzoff, A. N., and Decety, J. (2005). An fMRI study of imitation: action representation and body schema. Neuropsychologia 43, 115–127. doi: 10.1016/j.neuropsychologia.2004.04.026
Chaves, T. C., Turci, A. M., Pinheiro, C. F., Sousa, L. M., and Grossi, D. B. (2014). Static body postural misalignment in individuals with temporomandibular disorders: a systematic review. Braz. J. Phys. Ther. 18, 481–501. doi: 10.1590/bjpt-rbf.2014.0061
Costafreda, S. G. (2009). Pooling FMRI data: meta-analysis, mega-analysis and multi-center studies. Front. Neuroinform. 3:33. doi: 10.3389/neuro.11.033.2009
Cui, F., Arnstein, D., Thomas, R. M., Maurits, N. M., Keysers, C., and Gazzola, V. (2014). Functional magnetic resonance imaging connectivity analyses reveal efference-copy to primary somatosensory area, BA2. PLoS One 9:e84367. doi: 10.1371/journal.pone.0084367
Dao, T. T., Lavigne, G. J., Charbonneau, A., Feine, J. S., and Lund, J. P. (1994). The efficacy of oral splints in the treatment of myofascial pain of the jaw muscles: a controlled clinical trial. Pain 56, 85–94. doi: 10.1016/0304-3959(94)90153-8
De Cicco, V., Barresi, M., Tramonti Fantozzi, M. P., Cataldo, E., Parisi, V., and Manzoni, D. (2016). Oral implant-prostheses: new teeth for a brighter brain. PLoS One 11:e0148715. doi: 10.1371/journal.pone.0148715
De Cicco, V., Cataldo, E., Barresi, M., Parisi, V., and Manzoni, D. (2014). Sensorimotor trigeminal unbalance modulates pupil size. Arch. Ital. Biol. 152, 1–12.
de Vries, P. M., de Jong, B. M., Bohning, D. E., Walker, J. A., George, M. S., and Leenders, K. L. (2009). Changes in cerebral activations during movement execution and imagery after parietal cortex TMS interleaved with 3T MRI. Brain Res. 1285, 58–68. doi: 10.1016/j.brainres.2009.06.006
Diedrichsen, J., Grafton, S., Albert, N., Hazeltine, E., and Ivry, R. B. (2006). Goal-selection and movement-related conflict during bimanual reaching movements. Cereb. Cortex 16, 1729–1738. doi: 10.1093/cercor/bhj108
Eldeghaidy, S., Marciani, L., McGlone, F., Hollowood, T., Hort, J., Head, K., et al. (2011). The cortical response to the oral perception of fat emulsions and the effect of taster status. J. Neurophysiol. 105, 2572–2581. doi: 10.1152/jn.00927.2010
Ffytche, D. H., and Catani, M. (2005). Beyond localization: from hodology to function. Philos. Trans. R. Soc. Lond. B Biol. Sci. 360, 767–779. doi: 10.1098/rstb.2005.1621
Fujita, K., Matsuo, K., Yuzuriha, S., Kawagishi, K., and Moriizumi, T. (2012). Cell bodies of the trigeminal proprioceptive neurons that transmit reflex contraction of the levator muscle are located in the mesencephalic trigeminal nucleus in rats. J. Plast. Surg. Hand Surg. 46, 383–388. doi: 10.3109/2000656X.2012.722094
Goodale, M. A. (1998). Vision for perception and vision for action in the primate brain. Novartis Found. Symp. 218, 21–34.
Grabner, R. H., Ischebeck, A., Reishofer, G., Koschutnig, K., Delazer, M., Ebner, F., et al. (2009). Fact learning in complex arithmetic and figural-spatial tasks: the role of the angular gyrus and its relation to mathematical competence. Hum. Brain Mapp. 30, 2936–2952. doi: 10.1002/hbm.20720
Grabski, K., Lamalle, L., and Sato, M. (2012). Somatosensory-motor adaptation of orofacial actions in posterior parietal and ventral premotor cortices. PLoS One 7:e49117. doi: 10.1371/journal.pone.0049117
Grahn, J. A., and McAuley, J. D. (2009). Neural bases of individual differences in beat perception. Neuroimage 47, 1894–1903. doi: 10.1016/j.neuroimage.2009.04.039
Iida, T., Sakayanagi, M., Svensson, P., Komiyama, O., Hirayama, T., Kaneda, T., et al. (2012). Influence of periodontal afferent inputs for human cerebral blood oxygenation during jaw movements. Exp. Brain Res. 216, 375–384. doi: 10.1007/s00221-011-2941-3
Jankowski, J., Scheef, L., Hüppe, C., and Boecker, H. (2009). Distinct striatal regions for planning and executing novel and automated movement sequences. Neuroimage 44, 1369–1379. doi: 10.1016/j.neuroimage.2008.10.059
Jenkinson, M., Bannister, P., Brady, M., and Smith, S. (2002). Improved optimization for the robust and accurate linear registration and motion correction of brain images. Neuroimage 17, 825–841. doi: 10.1016/s1053-8119(02)91132-8
Jenkinson, M., Beckmann, C. F., Behrens, T. E., Woolrich, M. W., and Smith, S. M. (2012). Fsl. Neuroimage 62, 782–790. doi: 10.1016/j.neuroimage.2011.09.015
Jenkinson, M., and Smith, S. (2001). A global optimisation method for robust affine registration of brain images. Med. Image Anal. 5, 143–156. doi: 10.1016/s1361-8415(01)00036-6
Julià-Sánchez, S., Álvarez-Herms, J., Gatterer, H., Burtscher, M., Pagès, T., and Viscor, G. (2015). Dental occlusion influences the standing balance on an unstable platform. Motor Control 19, 341–354. doi: 10.1123/mc.2014-0018
Kihara, K., Takeuchi, T., Yoshimoto, S., Kondo, H. M., and Kawahara, J. I. (2015). Pupillometric evidence for the locus coeruleus-noradrenaline system facilitating attentional processing of action-triggered visual stimuli. Front. Psychol. 6:827. doi: 10.3389/fpsyg.2015.00827
King, D. R., and Miller, M. B. (2014). Lateral posterior parietal activity during source memory judgments of perceived and imagined events. Neuropsychologia 53, 122–136. doi: 10.3758/s13415-015-0357-4
Kushiro, K., and Goto, F. (2011). Effect of masticating chewing gum on postural stability during upright standing. Neurosci. Lett. 487, 196–198. doi: 10.1016/j.neulet.2010.10.021
Leech, R., and Sharp, D. J. (2014). The role of the posterior cingulate cortex in cognition and disease. Brain 137, 12–32. doi: 10.1093/brain/awt162
Lomber, S. G., and Payne, B. R. (1996). Removal of two halves restores the whole: reversal of visual hemineglect during bilateral cortical or collicular inactivation in the cat. Vis. Neurosci. 13, 1143–1156. doi: 10.1017/s0952523800007781
Lotze, M., Lucas, C., Domin, M., and Kordass, B. (2012). The cerebral representation of temporomandibular joint occlusion and its alternation by occlusal splints. Hum. Brain Mapp. 33, 2984–2993. doi: 10.1002/hbm.21466
Luo, P. F., Wang, B. R., Peng, Z. Z., and Li, J. S. (1991). Morphological characteristics and terminating patterns of masseteric neurons of the mesencephalic trigeminal nucleus in the rat: an intracellular horseradish peroxidase labeling study. J. Comp. Neurol. 303, 286–299. doi: 10.1002/cne.903030210
Macaluso, E., Frith, C. D., and Driver, J. (2007). Delay activity and sensory-motor translation during planned eye or hand movements to visual or tactile targets. J. Neurophysiol. 98, 3081–3094. doi: 10.1152/jn.00192.2007
Majerus, S., Bastin, C., Poncelet, M., Van der Linden, M., Salmon, E., Collette, F., et al. (2007). Short-term memory and the left intraparietal sulcus: focus of attention? Further evidence from a face short-term memory paradigm. Neuroimage 35, 353–367. doi: 10.1016/j.neuroimage.2006.12.008
Malouin, F., Richards, C. L., Jackson, P. L., Dumas, F., and Doyon, J. (2003). Brain activations during motor imagery of locomotor-related tasks: a PET study. Hum. Brain Mapp. 19, 47–62. doi: 10.1002/hbm.10103
Manto, M., and Bastian, A. J. (2007). Cerebellum and the deciphering of motor coding. Cerebellum 6, 3–6. doi: 10.1080/14734220701234690
Maurer, C., Stief, F., Jonas, A., Kovac, A., Groneberg, D. A., Meurer, A., et al. (2015). Influence of the lower jaw position on the running pattern. PLoS One 10:e0135712. doi: 10.1371/journal.pone.0135712
McArdle, W. D., Goldstein, L. B., Last, F. C., Spina, R., Lichtman, S., Meyer, J. E., et al. (1984). Temporomandibular joint repositioning and exercise performance: a double-blind study. Med. Sci. Sports Exerc. 16, 228–233.
Miyahara, T., Hagiya, N., Ohyama, T., and Nakamura, Y. (1996). Modulation of human soleus H reflex in association with voluntary clenching of the teeth. J. Neurophysiol. 76, 2033–2041. doi: 10.1152/jn.1996.76.3.2033
Mullally, S. L., and Maguire, E. A. (2011). A new role for the parahippocampal cortex in representing space. J. Neurosci. 31, 7441–7449. doi: 10.1523/JNEUROSCI.0267-11.2011
Mumford, J. A. (2012). A power calculation guide for fMRI studies. Soc. Cogn. Affect. Neurosci. 7, 738–742. doi: 10.1093/scan/nss059
Nagahama, Y., Okada, T., Katsumi, Y., Hayashi, T., Yamauchi, H., Sawamoto, N., et al. (1999). Transient neural activity in the medial superior frontal gyrus and precuneus time locked with attention shift between object features. Neuroimage 10, 193–199. doi: 10.1006/nimg.1999.0451
Nakao, T., Sanematsu, H., Yoshiura, T., Togao, O., Murayama, K., Tomita, M., et al. (2011). fMRI of patients with social anxiety disorder during a social situation task. Neurosci. Res. 69, 67–72. doi: 10.1016/j.neures.2010.09.008
Noaham, K. E., and Kumbang, J. (2008). Transcutaneous electrical nerve stimulation (TENS) for chronic pain. Cochrane Database Syst. Rev. 3:CD003222. doi: 10.1002/14651858.CD003222.pub2
Nomoto, S., Nakamura, M., Sato, T., and Hisanaga, R. (2013). Occlusal treatment with bite splint improves dyskinesia in Parkinson’s disease patient: a case report. Bull. Tokyo Dent. Coll. 54, 157–161. doi: 10.2209/tdcpublication.54.157
Ogino, Y., Nemoto, H., Inui, K., Saito, S., Kakigi, R., and Goto, F. (2007). Inner experience of pain: imagination of pain while viewing images showing painful events forms subjective pain representation in human brain. Cereb. Cortex 17, 1139–1146. doi: 10.1093/cercor/bhl023
Ogiso, T., Kobayashi, K., and Sugishita, M. (2000). The precuneus in motor imagery: a magnetoencephalographic study. Neuroreport 11, 1345–1349. doi: 10.1097/00001756-200004270-00039
Ohlendorf, D., Seebach, K., Hoerzer, S., Nigg, S., and Kopp, S. (2014). The effects of a temporarily manipulated dental occlusion on the position of the spine: a comparison during standing and walking. Spine J. 14, 2384–2391. doi: 10.1016/j.spinee.2014.01.045
Okubo, M., Fujinami, Y., and Minakuchi, S. (2010). Effect of complete dentures on body balance during standing and walking in elderly people. J. Prosthodont. Res. 54, 42–47. doi: 10.1016/j.jpor.2009.09.002
Olsson, C. J., and Lundström, P. (2013). Using action observation to study superior motor performance: a pilot fMRI study. Front. Hum. Neurosci. 7:819. doi: 10.3389/fnhum.2013.00819
Onuki, Y., Van Someren, E. J., De Zeeuw, C. I., and Van der Werf, Y. D. (2015). Hippocampal-cerebellar interaction during spatio-temporal prediction. Cereb. Cortex 25, 313–321. doi: 10.1093/cercor/bht221
O’Reilly, J. X., Mesulam, M. M., and Nobre, A. C. (2008). The cerebellum predicts the timing of perceptual events. J. Neurosci. 28, 2252–2260. doi: 10.1523/JNEUROSCI.2742-07.2008
Penfield, W., and Boldrey, E. (1937). Somatic motor and sensory representation in the cerebral cortex of man as studied by electrical stimulation. Brain 60, 389–443. doi: 10.1093/brain/60.4.389
Perinetti, G. (2006). Dental occlusion and body posture: no detectable correlation. Gait Posture 24, 165–168. doi: 10.1016/j.gaitpost.2005.07.012
Plata Bello, J., Modroño, C., Marcano, F., and González-Mora, J. L. (2015). The effect of motor familiarity during simple finger opposition tasks. Brain Imaging Behav. 9, 828–838. doi: 10.1007/s11682-014-9340-x
Quintero, A., Ichesco, E., Schutt, R., Myers, C., Peltier, S., and Gerstner, G. E. (2013). Functional connectivity of human chewing: an fcMRI study. J. Dent. Res. 92, 272–278. doi: 10.1177/0022034512472681
Rahm, B., Kaiser, J., Unterrainer, J. M., Simon, J., and Bledowski, C. (2014). fMRI characterization of visual working memory recognition. Neuroimage 90, 413–422. doi: 10.1016/j.neuroimage.2013.12.017
Raichle, M. E., MacLeod, A. M., Snyder, A. Z., Powers, W. J., Gusnard, D. A., and Shulman, G. L. (2001). A default mode of brain function. Proc. Natl. Acad. Sci. U.S.A. 98, 676–682. doi: 10.1073/pnas.98.2.676
Ringhof, S., Stein, T., Potthast, W., Schindler, H. J., and Hellmann, D. (2015). Force-controlled biting alters postural control in bipedal and unipedal stance. J. Oral Rehabil. 42, 173–184. doi: 10.1111/joor.12247
Rizzolatti, G., Camarda, R., Fogassi, L., Gentilucci, M., Luppino, G., and Matelli, M. (1988). Functional organization of inferior area 6 in the macaque monkey. II. Area F5 and the control of distal movements. Exp. Brain Res. 71, 491–507. doi: 10.1007/bf00248742
Sakurai, Y., Asami, M., and Mannen, T. (2010). Alexia and agraphia with lesions of the angular and supramarginal gyri: evidence for the disruption of sequential processing. J. Neurol. Sci. 288, 25–33. doi: 10.1016/j.jns.2009.10.015
Saling, L. L., and Phillips, J. G. (2007). Automatic behaviour: efficient not mindless. Brain Res. Bull. 73, 1–20. doi: 10.1016/j.brainresbull.2007.02.009
Samuels, E. R., and Szabadi, E. (2008). Functional neuroanatomy of the noradrenergic locus coeruleus: its roles in the regulation of arousal and autonomic function part I: principles of functional organisation. Curr. Neuropharmacol. 6, 235–253. doi: 10.2174/157015908785777229
Shibasaki, H., Sadato, N., Lyshkow, H., Yonekura, Y., Honda, M., Nagamine, T., et al. (1993). Both primary motor cortex and supplementary motor area play an important role in complex finger movement. Brain 116, 1387–1398. doi: 10.1093/brain/116.6.1387
Shoi, K., Fueki, K., Usui, N., Taira, M., and Wakabayashi, N. (2014). Influence of posterior dental arch length on brain activity during chewing in patients with mandibular distal extension removable partial dentures. J. Oral Rehabil. 41, 486–495. doi: 10.1111/joor.12169
Simon, O., Mangin, J. F., Cohen, L., Le Bihan, D., and Dehaene, S. (2002). Topographical layout of hand, eye, calculation, and language-related areas in the human parietal lobe. Neuron 33, 475–487. doi: 10.1016/s0896-6273(02)00575-5
Smith, S. M. (2002). Fast robust automated brain extraction. Hum. Brain Mapp. 17, 143–155. doi: 10.1002/hbm.10062
Stevens, M. C., Kiehl, K. A., Pearlson, G., and Calhoun, V. D. (2007). Functional neural circuits for mental timekeeping. Hum. Brain Mapp. 28, 394–408. doi: 10.1002/hbm.20285
Stippich, C., Blatow, M., Durst, A., Dreyhaupt, J., and Sartor, K. (2007). Global activation of primary motor cortex during voluntary movements in man. Neuroimage 34, 1227–1237. doi: 10.1016/j.neuroimage.2006.08.046
Stoeckel, M. C., Weder, B., Binkofski, F., Buccino, G., Shah, N. J., and Seitz, R. J. (2003). A fronto-parietal circuit for tactile object discrimination: an event-related fMRI study. Neuroimage 19, 1103–1114. doi: 10.1016/s1053-8119(03)00182-4
Stoodley, C. J., and Schmahmann, J. D. (2018). Functional topography of the human cerebellum. Handb. Clin. Neurol. 54, 59–70. doi: 10.1016/B978-0-444-63956-1.00004-7
Suchan, B., Yágüez, L., Wunderlich, G., Canavan, A. G., Herzog, H., Tellmann, L., et al. (2002). Hemispheric dissociation of visual-pattern processing and visual rotation. Behav. Brain Res. 136, 533–544. doi: 10.1016/s0166-4328(02)00204-8
Thürling, M., Küper, M., Stefanescu, R., Maderwald, S., Gizewski, E. R., Ladd, M. E., et al. (2011). Activation of the dentate nucleus in a verb generation task: a 7T MRI study. Neuroimage 57, 1184–1191. doi: 10.1016/j.neuroimage.2011.05.045
Tzourio-Mazoyer, N., Landeau, B., Papathanassiou, D., Crivello, F., Etard, O., Delcroix, N., et al. (2002). Automated anatomical labeling of activations in SPM using a macroscopic anatomical parcellation of the MNI MRI single-subject brain. Neuroimage 15, 273–289. doi: 10.1006/nimg.2001.097
Vallar, G., Bottini, G., Rusconi, M. L., and Sterzi, R. (1993). Exploring somatosensory hemineglect by vestibular stimulation. Brain 116, 71–86. doi: 10.1093/brain/116.1.71
Vingerhoets, G. (2014). Contribution of the posterior parietal cortex in reaching, grasping, and using objects and tools. Front. Psychol. 5:151. doi: 10.3389/fpsyg.2014.00151
Watanabe, J., Sugiura, M., Miura, N., Watanabe, Y., Maeda, Y., Matsue, Y., et al. (2004). The human parietal cortex is involved in spatial processing of tongue movement-an fMRI study. Neuroimage 21, 1289–1299. doi: 10.1016/j.neuroimage.2003.10.024
Wong, D., Dzemidzic, M., Talavage, T. M., Romito, L. M., and Byrd, K. E. (2011). Motor control of jaw movements: an fMRI study of parafunctional clench and grind behavior. Brain Res. 1383, 206–217. doi: 10.1016/j.brainres.2011.01.096
Woolrich, M. (2008). Robust group analysis using outlier inference. Neuroimage 41, 286–301. doi: 10.1016/j.neuroimage.2008.02.042
Woolrich, M. W., Behrens, T. E., Beckmann, C. F., Jenkinson, M., and Smith, S. M. (2004). Multilevel linear modelling for FMRI group analysis using Bayesian inference. Neuroimage 21, 1732–1747. doi: 10.1016/j.neuroimage.2003.12.023
Woolrich, M. W., Ripley, B. D., Brady, M., and Smith, S. M. (2001). Temporal autocorrelation in univariate linear modeling of FMRI data. Neuroimage 14, 1370–1386. doi: 10.1016/j.neuroimage.2003.12.023
Worsley, K. J. (2001). “Statistical analysis of activation images,” in Functional MRI: an Introduction to Methods, eds P. Jezzard, P. M. Matthews, and S. M. Smith (Oxford: OUP).
Keywords: malocclusion, trigeminal input, bite, fingers movement, brain activation, fMRI, BOLD signal
Citation: Tramonti Fantozzi MP, Diciotti S, Tessa C, Castagna B, Chiesa D, Barresi M, Ravenna G, Faraguna U, Vignali C, De Cicco V and Manzoni D (2019) Unbalanced Occlusion Modifies the Pattern of Brain Activity During Execution of a Finger to Thumb Motor Task. Front. Neurosci. 13:499. doi: 10.3389/fnins.2019.00499
Received: 12 June 2018; Accepted: 30 April 2019;
Published: 17 May 2019.
Edited by:
Ching-Po Lin, National Yang-Ming University, TaiwanReviewed by:
Xin Di, New Jersey Institute of Technology, United StatesQing Cai, East China Normal University, China
Copyright © 2019 Tramonti Fantozzi, Diciotti, Tessa, Castagna, Chiesa, Barresi, Ravenna, Faraguna, Vignali, De Cicco and Manzoni. This is an open-access article distributed under the terms of the Creative Commons Attribution License (CC BY). The use, distribution or reproduction in other forums is permitted, provided the original author(s) and the copyright owner(s) are credited and that the original publication in this journal is cited, in accordance with accepted academic practice. No use, distribution or reproduction is permitted which does not comply with these terms.
*Correspondence: Diego Manzoni, ZGllZ28ubWFuem9uaUB1bmlwaS5pdA==
†These authors have contributed equally to this work