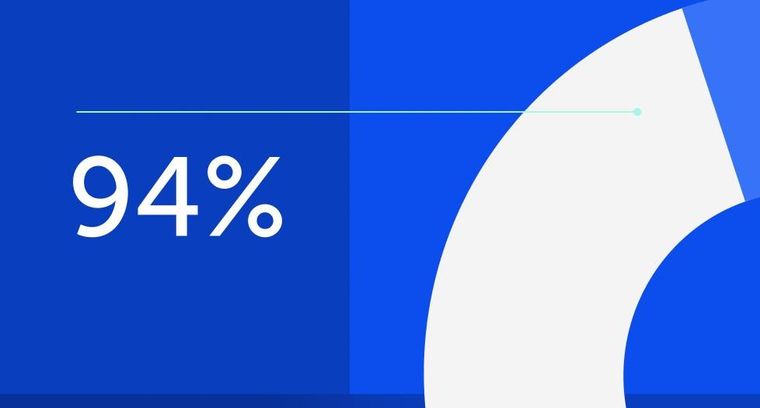
94% of researchers rate our articles as excellent or good
Learn more about the work of our research integrity team to safeguard the quality of each article we publish.
Find out more
MINI REVIEW article
Front. Neurosci., 04 February 2019
Sec. Neurodegeneration
Volume 13 - 2019 | https://doi.org/10.3389/fnins.2019.00049
Alzheimer’s disease (AD) is a progressive neurodegenerative disorder and the most common cause of dementia. Accumulating experimental evidence shows the important linkage between tumor necrosis factor-α (TNF) and AD, but the exact role of TNF in AD is still not completely understood. Although TNF-inhibitors are successfully used for treating several diseases, total inhibition of TNF can cause side effects, particularly in neurological diseases. This is attributed to the opposing roles of the two TNF receptors. TNF receptor 1 (TNFR1) predominantly mediates inflammatory and pro-apoptotic signaling pathways, whereas TNF receptor 2 (TNFR2) is neuroprotective and promotes tissue regeneration. Therefore, the specific activation of TNFR2 signaling, either by directly targeting TNFR2 via TNFR2 agonists or by blocking TNFR1 signaling with TNFR1-selective antagonists, seems a promising strategy for AD therapy. This mini-review discusses the involvement of TNFR2 and its signaling pathway in AD and outlines its potential application as therapeutic target. A better understanding of the function of TNFR2 may lead to the development of a treatment for AD.
The pleiotropic pro-inflammatory cytokine tumor necrosis factor-α (TNF), known as a member of the TNF superfamily of ligands, is a master regulator of the innate and adaptive immune system. TNF plays a vital role in the initiation and orchestration of inflammation and immunity (Aggarwal, 2003; Apostolaki et al., 2010; Fischer and Maier, 2015), and is potentially a key player in several neurodegenerative disorders, including Alzheimer’s disease (AD) (McAlpine and Tansey, 2008).
Alzheimer’s disease is an age-related neurodegenerative disorder associated with severe cognitive impairment such as memory deficits, deterioration of visuospatial skills and executive dysfunction. Its neuropathological hallmarks include both positive and negative features. Positive lesions consist of amyloid plaques, neurofibrillary tangles, neuropil threads, tau protein hyperphosphorylation, and glial activation. Negative lesions include losses of neurons, neuropil, and synaptic elements. Besides the well-known pathological characteristics mentioned above, neuroinflammation has been considered a major contributor to AD, in which TNF is an important mediator (McAlpine and Tansey, 2008).
Tumor necrosis factor is expressed as a 26-kDa monomeric type II transmembrane protein (tmTNF) that can be cleaved to release a 17-kDa soluble monomeric protein (solTNF) by the matrix metalloprotease TNF converting enzyme (TACE/ADAM17) (Aggarwal, 2000; Idriss and Naismith, 2000). TNF binds to two cognate receptors, the 55-kDa TNF receptor 1 (TNFR1, p55, TNFRSF1A) and the 75-kDa TNF receptor 2 (TNFR2, p75, TNFRSF1B), that mediate a variety of cellular responses. In general, TNFR1 predominantly exerts pro-inflammatory effects and TNFR2 is neuroprotective and promotes tissue homeostasis and regeneration. The identification of TNF expression around amyloidogenic plaques in human AD post-mortem brain tissue was the first indication of its possible involvement in AD (Dickson, 1997). At the protein level, elevated serum and plasma levels of TNF have been detected in patients with AD (Fillit et al., 1991; Bruunsgaard et al., 1999; Collins et al., 2000; Alvarez et al., 2007; Tan et al., 2007). Moreover, overproduction of inflammatory mediators in the AD brain occurs when microglia become chronically activated. It has been suggested that resident microglia fail to efficiently phagocytose amyloid beta (Aβ) because of elevated levels of proinflammatory cytokines, including TNF (Koenigsknecht-Talboo and Landreth, 2005). Besides, cell culture studies showed that TNF increased apoptosis of neurons treated with Aβ (Blasko et al., 1997) and affected Aβ production (Blasko et al., 1999, 2000). Furthermore, at the genetic level, three TNF polymorphisms were found to be associated with AD (Collins et al., 2000). For example, the TNF G308A promoter polymorphisms was found to increase the risk of AD in certain people (Kang et al., 2015; Wang, 2015). As TNFR2 activation has recently emerged as a potential therapeutic approach for neurodegeneration (Dong et al., 2016), we will here introduce TNFR2, focusing on the interrelation of TNFR2 and AD, and summarize a potential therapeutic strategy to specifically target TNFR2 in AD.
Tumor necrosis factor receptor 1 is ubiquitously expressed in virtually all cell types and tissues, and its activation can be induced by either solTNF or tmTNF, with a preference for solTNF. TNFR2 is predominately expressed in cells of the immune system, especially regulatory T (Treg) cells, and by endothelial cells, and preferentially binds tmTNF (Grell, 1995; Grell et al., 1998). TNFR1 and TNFR2 are single-pass transmembrane glycoproteins with 28% homology mainly in the extracellular domain, that is comprised of four cysteine-rich motif (Locksley et al., 2001). However, the intracellular domains of the TNF receptors are largely unrelated, lacking homologous sequences (Lewis et al., 1991), suggesting that different signaling functions originate from the two distinct receptors. TNFR1 contains an intracellular death domain (DD) that binds TNFR1-associated death domain protein (TRADD) of Fas-associated death domain (FADD), primarily involved in signaling for cell death. While TNFR2 does not contain a cytoplasmic DD, it interacts with TNF-associated factor 2 (TRAF2), and mainly yields cell survival (Wajant et al., 2003). TNFR2 consists of 439 amino acids, with the first 235 amino acids forming the extracellular domain, 30 amino acids forming the transmembrane domain, and 174 amino acids forming the cytoplasmic domain with a TRAF2 binding site. TRAF2 can bind TRAF1, TRAF3, inhibitor of apoptosis protein 1 (cIAP1) and inhibitor of apoptosis protein 2 (cIAP2) (Rothe et al., 1994, 1995b).
Tumor necrosis factor signaling can elicit various cellular responses through TNFR1 and TNFR2 depending on a variety of factors including the cellular metabolic state and the presence of the adaptor proteins. In fact, the differences in the intracellular signaling pathways such as nuclear factor kappa-B (NF-κB), p38, c-jun N-terminal kinase (JNK), and the ceramide/sphingomyelinase signaling pathway, result in multifarious processes including inflammation, proliferation, cell migration, apoptosis, and necrosis (Eissner et al., 2000, 2004; Harashima et al., 2001; Ware, 2005). TNFR2-mediated signaling activates inflammatory and pro-survival signaling pathways via interaction with adaptor proteins of TRAF1 and TRAF2, and in turn, also the cIAPs and the NF-κB pathway (Rothe et al., 1994, 1995a; Rao et al., 1995) (Figure 1). TNFR2 can also activate the phosphatidyl inositol (PI) 3-kinase/Akt pathway to promote cell survival and proliferation (Marchetti et al., 2004; Dolga et al., 2008; Fischer et al., 2011). In addition, there could be a cross talk between TNFR1 and TNFR2 because of the central role that TRAF2, TRAF1, and cIAP play in both TNFR1 and TNFR2 signaling pathways (Naudé et al., 2011). For instance, it was shown that the depletion of TRAF2 caused by TNFR2 activation accelerates the activation of TNFR1-dependent caspase 8 (Wang et al., 1998; Fotin-Mleczek et al., 2002; Li et al., 2002). In general, TNFRs cross talk is elusive and regulated by both the physiological environment and signaling kinetics between TNFR1 and TNFR2.
Figure 1. Molecular signaling pathway of TNFR2. The interaction of the membrane form of TNF with TNFR2 promotes cell survival through the activation of the complex signaling cascade. AIP1, ASK interacting protein 1; Akt, serine–threonine kinase; ASK1, apoptosis signal-regulating kinase 1; cFLIP, caspase-8 homolog FLICE-inhibitory protein; cIAP, cellular inhibitor of apoptosis protein; FADD, Fas-associated death domain protein; IKK, IκB kinase; IκBα, inhibitor of kappa B; JNK, c-Jun N-terminal kinase; NFκB, nuclear factor kappa B; MAPKs, phosphorylate mitogen-activated kinase; MKK, mitogen-activated protein kinase kinase; NIK, NFκB-inducing kinase; PI3K, phosphoinositide 3-kinases; RIP, receptor interacting protein; TRADD, TNF receptor-associated death domain; TRAF, TNF receptor-associated factor.
Mounting evidence convincingly shows that TNF expression is increased in AD by various methods and means, including genetic (Collins et al., 2000), protein expression (Álvarez et al., 2007) and histological (Zhao et al., 2003) approaches. Considering that TNFR1 exerts inflammatory and pro-apoptotic functions, whereas TNFR2 has a neuroprotective function, it is of utmost importance to define the expression of both receptors in AD. For instance, studies in human post-mortem AD brain tissues have demonstrated that TNFR1 levels are increased while TNFR2 levels are decreased (Zhao et al., 2003; Cheng et al., 2010). Furthermore, Cheng et al. (2010) showed that in the AD brain, TNF had a higher likelihood to bind to TNFR1 than to TNFR2, which might explain the prominent role of TNFR1 in the pathophysiology of AD. Strikingly, deletion of TNFR2 in an AD mouse model exacerbated AD pathology through TNFR1 (Jiang et al., 2014). In this same study, a later overexpression of TNFR2 counteracted the aggravation of the disease (Jiang et al., 2014). This finding reinforces a neuroprotective function of TNFR2 in AD pathology. Furthermore, in a mouse model of acute retinal ischemia, Fontaine et al. (2002) demonstrated that, after inducing retinal damage, mice lacking TNFR1 showed a significant reduction in neurodegeneration while mice lacking TNFR2 showed a significant increase in neurodegeneration. Similarly, deletion of TNFR1 in an AD mouse model resulted in a reduction in the generation of Aβ plaques and an enhancement in learning and memory (He et al., 2007; Olleros et al., 2010), indicating the contribution of TNFR1 to neurodegeneration. Lastly, a recent study demonstrated in AD mouse models that abrogation of TNFR1 results in decreased brain inflammation and in an improvement of amyloidosis and blood–brain barrier integrity (Steeland et al., 2018).
Altogether, these results support the hypothesis that increased expression of TNFR1 aggravates AD pathology, whereas TNFR2 is able to prevent it. Therefore, TNFR1 and TNFR2 represent promising therapeutic targets for the treatment or prevention of AD.
Existing therapies that target TNF for clinical use are based on monoclonal antibodies that inhibit its signaling via its receptors. These therapies have been approved for treatment of several inflammatory and autoimmune diseases, including rheumatoid arthritis, psoriatic arthritis or plaque psoriasis. The therapeutic potential of these anti-TNF antibodies has more recently been studied in neurodegenerative diseases. Infliximab is a chimeric IgG1 monoclonal antibody that has a high binding affinity for human TNF. Two similar studies that were performed in AD mouse models showed that intracerebroventricular injection of infliximab reduced tau phosphorylation, TNF levels and Aβ plaques (Shi et al., 2011a), and improved visual recognition memory (Kim et al., 2016). Furthermore, intrathecal treatment of infliximab in a female case with AD showed a significant improvement in cognition (Shi et al., 2011b).
Another biologic anti-TNF drug is etanercept, a combination of the extracellular domain of TNFR2 linked to the Fc portion of a human IgG1. A pilot study conducted in 15 AD patients that received perispinal administration of etanercept resulted in a reduction of cognitive deficits based on the AD Assessment Scale-Cognitive Subscale, the Severe Impairment Battery, and the Mini-Mental State Examination (Tobinick et al., 2006). A follow-up study by the same research group (Tobinick and Gross, 2008) reported a significant cognitive improvement in one AD patient within minutes after perispinal treatment with etanercept. Moreover, treatment of etanercept in an AD mouse model resulted in enhanced cognition as well as a reduction in the hippocampal levels of TNF (Detrait et al., 2014). Additionally, an experiment performed in rats showed that peripheral administration of labeled etanercept is able to penetrate in the CNS (Tobinick et al., 2009), whereas a later similar study in rats reported no penetration of labeled etanercept when injected into the perispinal area (Roerink et al., 2015). These contradicting studies question the mechanism of action of etanercept.
Another limitation of the abovementioned etanercept studies in AD patients is that they did not include a control group receiving placebo and the long-term effects of etanercept were not stated. In fact, Atigari and Healy (2014) described the appearance of psychiatric-like symptoms related to schizophrenia in a patient receiving etanercept with no previous psychiatric history. Furthermore, Butchart et al. (2015) did not find significant differences in cognition nor behavior between AD patients receiving placebo or etanercept in a randomized, placebo-controlled, double-blind study. Nonetheless, they reported a higher prevalence of infections in the etanercept group compared with the placebo group (Butchart et al., 2015). Moreover, Sicotte and Voskuhl (2001) reported that a patient receiving etanercept as treatment for rheumatoid arthritis developed multiple sclerosis and optic neuritis.
The use of a similar biological anti-TNF drug, lenercept, was unsuccessful as treatment for relapsing-remitting multiple sclerosis in a placebo-controlled, double-blind phase 2 study. Indeed, patients receiving lenercept experienced an earlier and significant aggravation of the disease compared with patients receiving placebo (Group, 1999). Other studies have also related TNF blockade with the appearance of autoimmune-like syndromes, like lupus, neuropathies and demyelinating disorders (Prinz, 2011). Finally, Deepak et al. (2013) also claimed a relation between anti-TNF therapies and the development of infections and neurological events.
These studies collectively show that anti-TNF therapies may be disadvantageous, especially in the treatment of neurological diseases. In this respect, total inhibition of TNF might nullify its positive effects, particularly via its TNFR2. Given the evidence described, we will focus on new experimental therapies that selectively interfere with the TNF receptors signaling for the treatment of neurodegenerative diseases:
As concluded in the previous section, TNFR1 plays a key role in neuroinflammation and apoptosis occurring AD. Since solTNF primarily activates TNFR1, a specific inhibition of solTNF would, in principle, block the functions of TNFR1 without preventing the neuroprotective functions of TNFR2.
XPro-1595 is a selective solTNF inhibitor (Figure 2). Results from animal studies show that XPro-1595 improves pathologies in models for neurodegenerative diseases. First, in experimental autoimmune encephalomyelitis (EAE), a multiple sclerosis model, XPro-1595 improved remyelination and reduced CNS lesions and neuroinflammation (Brambilla et al., 2011; Evangelidou et al., 2014). Besides, in a similar study, Taoufik et al. (2011) demonstrated that inhibition of solTNF by XPro-1595 significantly decreased the levels of pro-inflammatory cytokines in the brain and protected mice in the context of EAE. A later study supported the ability of XPro-1595 to ameliorate EAE symptoms in mice, although, in this case, the presence of TNFR2 in oligodendrocytes was necessary (Madsen et al., 2016). This study shows that the solely inhibition of solTNF and, thus, TNFR1 by XPro-1595 might not be sufficient to protect mice from EAE. Moreover, in mice with spinal cord injury, treatment with XPro-1595 significantly increased the protein levels of TNFR2 in the injured area, and enhanced cognition (Novrup et al., 2014). Likewise, a study on acute spinal cord injury in rats that were treated with XPro-1595 showed a significant improvement in immune dysfunction and autonomic dysreflexia symptoms (Mironets et al., 2018). In a mouse model of cerebral ischemia, intravenous administration of XPro-1595 improved motor functions and reduced inflammation (Clausen et al., 2014). Additionally, in a mouse model of Huntington’s disease, intracerebroventricular infusion of XPro-1595 resulted in an improvement of motor functions, increased neuronal viability and decreased neuroinflammation (Hsiao et al., 2014).
Figure 2. Schematic of TNF signaling via its two receptors TNFR1 and TNFR2 as well as the existing potential therapeutic approaches targeting TNFR2, including solTNF inhibitor [e.g., xPro1595 (Olleros et al., 2010; Shi et al., 2011a)], TNFR1-specific antagonist [e.g., ATROSAB (Kim et al., 2016)], and TNFR2-specific agonists [e.g., TNF-scTNFR2 (Fischer et al., 2011) and EHD2-scTNFR2 (Dong et al., 2016)]. solTNF, soluble tumor necrosis factor; tmTNF, transmembrane tumor necrosis factor.
Furthermore, in a recent study using a rat model of Parkinson’s disease, peripheral injection of XPro-1595 significantly reduced apoptosis, inflammation, and loss of dopaminergic neurons (Barnum et al., 2014). This study also demonstrated that XPro-1595 can effectively reach the brain by crossing the blood–brain barrier (Barnum et al., 2014). In an AD context, Cavanagh et al. (2016), showed that XPro-1595 was able to prevent synaptic loss when treating mice at an early stage. Finally, MacPherson et al. (2017) showed that systemic administration of XPro-1595 reduced Aβ plaques in the subiculum and restored long-term potentiation in a mouse model of AD. Overall, these findings indicate that XPro-1595 is an effective therapy in disease models where neuroinflammation events are present, suggesting that it could also be used as a potential therapy to treat AD.
In addition to inhibition of TNFR1 signaling by removing its most important ligand solTNF, TNFR1 antagonists may serve to inhibit TNFR1 signaling. To this end, Zettlitz et al. (2010) generated an antagonistic TNFR1-specific antibody (ATROSAB), which is able to interfere with the different signaling pathways of TNFR1 (Figure 2). The efficacy of ATROSAB has not yet been tested in AD, but it has been assessed in a nucleus basalis magnocellularis (NBM) chemical lesion model. This in vivo model is generated by an exposure to glutamate, which causes neuronal cell death and, mimics acute neurodegenerative diseases. The NBM lesion model provokes an activation of macrophages and microglia (inflammation) and a loss of cholinergic fibers similar to that in AD (Dong et al., 2016). Treatment with ATROSAB or with a TNFR2 agonist (the latter discussed in the section “Stimulation of TNFR2 by TNFR2 Agonist”) reverted these symptoms and protected from memory deficits and excitotoxicity. Besides, by blocking TNFR1, ATROSAB shifted the TNF signaling toward TNFR2, and showed to be neuroprotective in this lesion model (Dong et al., 2016). Importantly, a recent study that tested ATROSAB in the EAE multiple sclerosis model demonstrated that treatment with ATROSAB was able to significantly mitigate EAE symptoms and delay the disease onset, proving the potential efficacy of ATROSAB in this neurodegenerative disease model (Williams et al., 2018). Accordingly, ATROSAB may represent a potential therapy for treating AD.
Instead of inhibiting TNFR1 signaling in order to prevent cell death, one can promote the signaling through TNFR2 in order to stimulate cell survival. The neuroprotective role of TNFR2 signaling has been reported in several studies (Fontaine et al., 2002; Marchetti et al., 2004; Patel et al., 2012; Maier et al., 2013; Fischer et al., 2014). Hence, Fischer et al. (2011) developed a soluble human TNFR2 agonist (TNC-scTNFR2) that selectively mimics tmTNF, augmenting TNFR2 activation (Figure 2). This agonist proved to protect against neuronal cell death induced by oxidative stress (Fischer et al., 2011), which is a common hallmark of neurodegenerative diseases, including AD. Dong et al. (2016) evaluated the efficacy of another selective TNFR2 agonist (EHD2-scTNFR2) in combination with ATROSAB in the NMB lesion model (Dong et al., 2016). This combination of TNFR1 antagonist and TNFR2 agonist selectively inhibited TNFR1 and enhanced TNFR2 activation, acquiring a potent neuroprotective effect, as revealed by an improvement in memory and cell viability, and a reduction in the loss of cholinergic fibers and inflammation. Overall, this study (Dong et al., 2016) demonstrated that the combination of the antagonistic TNFR1-specific antibody ATROSAB and the selective TNFR2 agonist EHD2-scTNFR2 is effective to treat an acute neurodegenerative disorder caused by glutamate-induced excitotoxicity. Thus, it is plausible that applying this strategy will serve to treat other neurological disorders, like AD.
The discovery of the apparent dual role of TNF through its two receptors has initiated extensive research into new possibilities to treat neuroinflammation, a common hallmark of neurodegenerative diseases. The initial discovery of anti-TNF therapies led to inconclusive results due to the potential side effects that were reported. Therefore, the development of specific TNFR1 antagonists and solTNF inhibitors (ATROSAB and XPro-1595) that ameliorate inflammation and apoptosis, and TNFR2 agonists that enhance neuro-regeneration and tissue homeostasis, are promising strategies to treat neurodegeneration. As discussed in this mini-review, a considerable number of studies have shown the efficacy of targeting TNF receptors in several neurodegenerative diseases, suggesting that these drugs might have potential in the therapy of AD. In the future, a deeper understanding of the diverse molecular pathways of TNF signaling can contribute to the discovery of more specific and refined strategies to treat AD and other neurodegenerative diseases.
NO-C and YW wrote the manuscript. PN, PDD, IZ, and UE reviewed and edited it, and provided key guidance.
The authors declare that the research was conducted in the absence of any commercial or financial relationships that could be construed as a potential conflict of interest.
PN, PDD, and UE are supported by ZonMW Deltaplan Dementie Memorabel and Alzheimer Nederland (733050815). PN was funded by Alzheimer Nederland (WE. 13-2015-19) and NeuroSearch Antwerp. UE was supported by the Foundation MS Research Nederland 15 – 898 MS. YW receives funding from the China Scholarship Council (CSC) program (Grant No: 201607040062). NO-C was supported by ZonMW Deltaplan Dementie Memorabel. IZ was supported by the Dutch Technology Foundation TTW, which is part of the Netherlands Organization for Scientific Research (NWO), and which is partly funded by the Ministry of Economic Affairs.
Aggarwal, B. B. (2000). Tumour necrosis factors receptor associated signalling molecules and their role in activation of apoptosis, JNK and NF-κB. Ann. Rheum. Dis. 59(Suppl. 1), i6–i16. doi: 10.1136/ard.59.suppl_1.i6
Aggarwal, B. B. (2003). Signalling pathways of the TNF superfamily: a double-edged sword. Nat. Rev. Immunol. 3, 745–756. doi: 10.1038/nri1184
Álvarez, A., Cacabelos, R., Sanpedro, C., García-Fantini, M., and Aleixandre, M. (2007). Serum TNF-alpha levels are increased and correlate negatively with free IGF-I in Alzheimer disease. Neurobiol. Aging 28, 533–536. doi: 10.1016/j.neurobiolaging.2006.02.012
Alvarez, A., Cacabelos, R., Sanpedro, C., García-Fantini, M., and Aleixandre, M. (2007). Serum TNF-alpha levels are increased and correlate negatively with free IGF-I in Alzheimer disease. Neurobiol. Aging 28, 533–536. doi: 10.1016/j.neurobiolaging.2006.02.012
Apostolaki, M., Armaka, M., Victoratos, P., and Kollias, G. (2010). Cellular mechanisms of TNF function in models of inflammation and autoimmunity. Curr. Dir. Autoimmun. 11, 1–26. doi: 10.1159/000289195
Atigari, O. V., and Healy, D. (2014). Schizophrenia-like disorder associated with etanercept treatment. BMJ Case Rep. 2014:bcr2013200464. doi: 10.1136/bcr-2013-200464
Barnum, C. J., Chen, X., Chung, J., Chang, J., Williams, M., Grigoryan, N., et al. (2014). Peripheral administration of the selective inhibitor of soluble tumor necrosis factor (TNF) XPro 1595 attenuates nigral cell loss and glial activation in 6-OHDA hemiparkinsonian rats. J. Parkinsons Dis. 4, 349–360. doi: 10.3233/JPD-140410
Blasko, I., Marx, F., Steiner, E., Hartmann, T., and Grubeck-Loebenstein, B. (1999). TNFα plus IFNγ induce the production of Alzheimer β-amyloid peptides and decrease the secretion of APPs. Res. Commun. 13, 63–68.
Blasko, I., Schmitt, T. L., Steiner, E., Trieb, K., and Grubeck-Loebenstein, B. (1997). Tumor necrosis factor alpha augments amyloid beta protein (25-35) induced apoptosis in human cells. Neurosci. Lett. 238, 17–20. doi: 10.1016/S0304-3940(97)00845-8
Blasko, I., Veerhuis, R., Stampfer-Kountchev, M., Saurwein-Teissl, M., Eikelenboom, P., and Grubeck-Loebenstein, B. (2000). Costimulatory effects of interferon gamma and interleukin-1beta or tumor necrosis factor alpha on the synthesis of Abeta1-40 and Abeta1-42 by human astrocytes. Neurobiol. Dis. 7, 682–689. doi: 10.1006/nbdi.2000.0321
Brambilla, R., Ashbaugh, J. J., Magliozzi, R., Dellarole, A., Karmally, S., Szymkowski, D. E., et al. (2011). Inhibition of soluble tumour necrosis factor is therapeutic in experimental autoimmune encephalomyelitis and promotes axon preservation and remyelination. Brain 134, 2736–2754. doi: 10.1093/brain/awr199
Bruunsgaard, H., Andersen-Ranberg, K., Jeune, B., Pedersen, A. N., Skinhøj, P., and Pedersen, B. K. (1999). A high plasma concentration of TNF-alpha is associated with dementia in centenarians. J. Gerontol. A Biol. Sci. Med. Sci. 54, 357–364. doi: 10.1093/gerona/54.7.M357
Butchart, J., Brook, L., Hopkins, V., Teeling, J., Puntener, U., Culliford, D., et al. (2015). Etanercept in Alzheimer disease: a randomized, placebo controlled, double-blind, phase 2 trial. Neurology 84, 2161–2168. doi: 10.1212/WNL.0000000000001617
Cavanagh, C., Tse, Y. C., Nguyen, H. B., Krantic, S., Breitner, J. C., Quirion, R., et al. (2016). Inhibiting tumor necrosis factor-α before amyloidosis prevents synaptic deficits in an Alzheimer’s disease model. Neurobiol. Aging 47, 41–49. doi: 10.1016/j.neurobiolaging.2016.07.009
Cheng, X., Yang, L., He, P., Li, R., and Shen, Y. (2010). Differential activation of tumor necrosis factor receptors distinguishes between brains from Alzheimer’s disease and non-demented patients. J. Alzheimers Dis. 19, 621–630. doi: 10.3233/JAD-2010-1253
Clausen, B. H., Degn, M., Martin, N. A., Couch, Y., Karimi, L., Ormhøj, M., et al. (2014). Systemically administered anti-TNF therapy ameliorates functional outcomes after focal cerebral ischemia. J. Neuroinflammation 11:203. doi: 10.1186/s12974-014-0203-6
Collins, J. S., Perry, R. T., Watson, B. Jr., Harrell, L. E., Acton, R. T., Blacker, D., et al. (2000). Association of a haplotype for tumor necrosis factor in siblings with late-onset Alzheimer disease: the NIMH Alzheimer disease genetics initiative. Am. J. Med. Genet. Part A 96, 823–830. doi: 10.1002/1096-8628(20001204)96:6<823::AID-AJMG26>3.0.CO;2-I
Deepak, P., Stobaugh, D. J., Sherid, M., Sifuentes, H., and Ehrenpreis, E. D. (2013). Neurological events with tumour necrosis factor alpha inhibitors reported to the Food and Drug Administration Adverse Event Reporting System. Aliment. Pharmacol. Ther. 38, 388–396. doi: 10.1111/apt.12385
Detrait, E. R., Danis, B., Lamberty, Y., and Foerch, P. (2014). Peripheral administration of an anti-TNF-alpha receptor fusion protein counteracts the amyloid induced elevation of hippocampal TNF-alpha levels and memory deficits in mice. Neurochem. Intern. 72, 10–13. doi: 10.1016/j.neuint.2014.04.001
Dickson, D. W. (1997). The pathogenesis of senile plaques. J. Neuropathol. Exp. Neurol. 56, 321–339. doi: 10.1097/00005072-199704000-00001
Dolga, A. M., Nijholt, I. M., Ostroveanu, A., Ten, Bosch Q, Luiten, P. G., and Eisel, U. L. (2008). Lovastatin induces neuroprotection through tumor necrosis factor receptor 2 signaling pathways. J. Alzheimers Dis. 13, 111–122. doi: 10.3233/JAD-2008-13201
Dong, Y., Fischer, R., Naudé, P. J., Maier, O., Nyakas, C., Duffey, M., et al. (2016). Essential protective role of tumor necrosis factor receptor 2 in neurodegeneration. Proc. Natl. Acad. Sci. U.S.A. 113, 12304–12309. doi: 10.1073/pnas.1605195113
Eissner, G., Kirchner, S., Lindner, H., Kolch, W., Janosch, P., Grell, M., et al. (2000). Reverse signaling through transmembrane TNF confers resistance to lipopolysaccharide in human monocytes and macrophages. J. Immunol. 164, 6193–6198. doi: 10.4049/jimmunol.164.12.6193
Eissner, G., Kolch, W., and Scheurich, P. (2004). Ligands working as receptors: reverse signaling by members of the TNF superfamily enhance the plasticity of the immune system. Cytokine Growth Factor Rev. 15, 353–366. doi: 10.1016/j.cytogfr.2004.03.011
Evangelidou, M., Karamita, M., Vamvakas, S. S., Szymkowski, D. E., and Probert, L. (2014). Altered expression of oligodendrocyte and neuronal marker genes predicts the clinical onset of autoimmune encephalomyelitis and indicates the effectiveness of multiple sclerosis–directed therapeutics. J. Immunol. 192, 4122–4133. doi: 10.4049/jimmunol.1300633
Fillit, H., Ding, W. H., Buee, L., Kalman, J., Altstiel, L., Lawlor, B., et al. (1991). Elevated circulating tumor necrosis factor levels in Alzheimer’s disease. Neurosci. Lett. 129, 318–320. doi: 10.1016/0304-3940(91)90490-K
Fischer, R., and Maier, O. (2015). Interrelation of oxidative stress and inflammation in neurodegenerative disease: role of TNF. Oxid. Med. Cell. Longev. 2015:610813. doi: 10.1155/2015/610813
Fischer, R., Maier, O., Siegemund, M., Wajant, H., Scheurich, P., and Pfizenmaier, K. (2011). A TNF receptor 2 selective agonist rescues human neurons from oxidative stress-induced cell death. PLoS One 6:e27621. doi: 10.1371/journal.pone.0027621
Fischer, R., Wajant, H., Kontermann, R., Pfizenmaier, K., and Maier, O. (2014). Astrocyte-specific activation of TNFR2 promotes oligodendrocyte maturation by secretion of leukemia inhibitory factor. Glia 62, 272–283. doi: 10.1002/glia.22605
Fontaine, V., Mohand-Said, S., Hanoteau, N., Fuchs, C., Pfizenmaier, K., and Eisel, U. (2002). Neurodegenerative and neuroprotective effects of tumor necrosis factor (TNF) in retinal ischemia: opposite roles of TNF receptor 1 and TNF receptor. J. Neurosci. 22:RC216.
Fotin-Mleczek, M., Henkler, F., Samel, D., Reichwein, M., Hausser, A., Parmryd, I., et al. (2002). Apoptotic crosstalk of TNF receptors: TNF-R2-induces depletion of TRAF2 and IAP proteins and accelerates TNF-R1-dependent activation of caspase. J. Cell Sci. 115, 2757–2770.
Grell, M. (1995). Tumor necrosis factor (TNF) receptors in cellular signaling of soluble and membrane-expressed TNF. J. Inflammation 47, 8–17.
Grell, M., Wajant, H., Zimmermann, G., and Scheurich, P. (1998). The type 1 receptor (CD120a) is the high-affinity receptor for soluble tumor necrosis factor. Proc. Natl. Acad. Sci. U.S.A. 95, 570–575. doi: 10.1073/pnas.95.2.570
Group, T. L. S. (1999). The Lenercept Multiple Sclerosis Study Group and The University of British Columbia MS/MRI Analysis Group TNF neutralization in MS: results of a randomized, placebo-controlled multicenter study. Neurology 53, 457–465. doi: 10.1212/WNL.53.3.457
Harashima, S., Horiuchi, T., Hatta, N., Morita, C., Higuchi, M., Sawabe, T., et al. (2001). Outside-to-inside signal through the membrane TNF-α induces E-selectin (CD62E) expression on activated human CD4+ T cells. J. Immunol. 166, 130–136. doi: 10.4049/jimmunol.166.1.130
He, P., Zhong, Z., Lindholm, K., Berning, L., Lee, W., Lemere, C., et al. (2007). Deletion of tumor necrosis factor death receptor inhibits amyloid β generation and prevents learning and memory deficits in Alzheimer’s mice. J. Cell Biol. 178, 829–841. doi: 10.1083/jcb.200705042
Hsiao, H. Y., Chiu, F. L., Chen, C. M., Wu, Y. R., Chen, H. M., Chen, Y. C., et al. (2014). Inhibition of soluble tumor necrosis factor is therapeutic in Huntington’s disease. Mol. Genet. 15, 4328–4344. doi: 10.1093/hmg/ddu151
Idriss, H. T., and Naismith, J. H. (2000). TNFα and the TNF receptor superfamily: structure-function relationship (s). Microsc. Res. Tech. 50, 184–195. doi: 10.1002/1097-0029(20000801)50:3<184::AID-JEMT2>3.0.CO;2-H
Jiang, H., He, P., Xie, J., Staufenbiel, M., Li, R., and Shen, Y. (2014). Genetic deletion of TNFRII gene enhances the Alzheimer-like pathology in an APP transgenic mouse model via reduction of phosphorylated IκBα. Hum. Mol. Genet. 23, 4906–4918. doi: 10.1093/hmg/ddu206
Kang, H. J., Kim, J. M., Kim, S. W., Shin, I. S., Park, S. W., Kim, Y. H., et al. (2015). Associations of cytokine genes with Alzheimer’s disease and depression in an elderly Korean population. J. Neurol. Neurosurg. Psychiatry 86, 1002–1007. doi: 10.1136/jnnp-2014-308469
Kim, D. H., Choi, S. M., Jho, J., Park, M. S., Kang, J., Park, S. J., et al. (2016). Infliximab ameliorates AD-associated object recognition memory impairment. Behav. Brain Res. 311, 384–391. doi: 10.1016/j.bbr.2016.06.001
Koenigsknecht-Talboo, J., and Landreth, G. E. (2005). Microglial phagocytosis induced by fibrillar beta-amyloid and IgGs are differentially regulated by proinflammatory cytokines. J. Neurosci. 25, 8240–8249. doi: 10.1523/JNEUROSCI.1808-05.2005
Lewis, M., Tartaglia, L. A., Lee, A., Bennett, G. L., Rice, G. C., Wong, G. H., et al. (1991). Cloning and expression of cDNAs for two distinct murine tumor necrosis factor receptors demonstrate one receptor is species specific. Proc. Natl. Acad. Sci. U.S.A. 88, 2830–2834. doi: 10.1073/pnas.88.7.2830
Li, X., Yang, Y., and Ashwell, J. D. (2002). TNF-RII and c-IAP1 mediate ubiquitination and degradation of TRAF. Nature 416, 345. doi: 10.1038/416345a
Locksley, R. M., Killeen, N., and Lenardo, M. J. (2001). The TNF and TNF receptor superfamilies. Cell 104, 487–501. doi: 10.1016/S0092-8674(01)00237-9
MacPherson, K. P., Sompol, P., Kannarkat, G. T., Chang, J., Sniffen, L., Wildner, M. E., et al. (2017). Peripheral administration of the soluble TNF inhibitor XPro1595 modifies brain immune cell profiles, decreases beta- amyloid plaque load, and rescues impaired long-term potentiation in 5xFAD mice. Neurobiol. Dis. 102, 81–95. doi: 10.1016/j.nbd.2017.02.010
Madsen, P. M., Motti, D., Karmally, S., Szymkowski, D. E., Lambertsen, K. L., Bethea, J. R., et al. (2016). Oligodendroglial TNFR2 mediates membrane TNF- dependent repair in experimental autoimmune encephalomyelitis by pro- moting oligodendrocyte differentiation and remyelination. J. Neurosci. 36, 5128–5143. doi: 10.1523/JNEUROSCI.0211-16.2016
Maier, O., Fischer, R., Agresti, C., and Pfizenmaier, K. (2013). TNF receptor 2 protects oligodendrocyte progenitor cells against oxidative stress. Biochem. Biophys. Res. Commun. 440, 336–341. doi: 10.1016/j.bbrc.2013.09.083
Marchetti, L., Klein, M., Schlett, K., Pfizenmaier, K., and Eisel, U. L. (2004). Tumor Necrosis Factor (TNF)-mediated Neuroprotection against Glutamate-induced Excitotoxicity Is Enhanced by N-Methyl-D-aspartate Receptor Activation Essential role of a tnf receptor 2-mediated phosphatidylinositol 3-kinase-dependent nf-κb pathway. J. Biol. Chem. 279, 32869–32881. doi: 10.1074/jbc.M311766200
McAlpine, F. E., and Tansey, M. G. (2008). Neuroinflammation and tumor necrosis factor signaling in the pathophysiology of Alzheimer’s disease. J. Inflamm. Res. 1, 29–39.
Mironets, E., Osei-Owusu, P., Bracchi-Ricard, V., Fischer, R., Owens, E. A., Ricard, J., et al. (2018). Soluble TNFα signaling within the spinal cord contributes to the development of autonomic dysreflexia and ensuing vascular and immune dysfunction after spinal cord injury. J. Neurosci. 25, 4146–4162. doi: 10.1523/JNEUROSCI.2376-17.2018
Naudé, P. J., den, Boer JA, Luiten, P. G., and Eisel, U. L. (2011). Tumor necrosis factor receptor cross-talk. FEBS J. 278, 888–898. doi: 10.1111/j.1742-4658.2011.08017.x
Novrup, H. G., Bracchi-Ricard, V., Ellman, D. G., Ricard, J., Jain, A., Runko, E., et al. (2014). Central but not systemic administration of XPro1595 is therapeutic following moderate spinal cord injury in mice. J. Neuroinflammation 11:159. doi: 10.1186/s12974-014-0159-6
Olleros, M. L., Vesin, D., Fotio, A. L., Santiago-Raber, M. L., Tauzin, S., Szymkowski, D. E., et al. (2010). Soluble TNF, but not membrane TNF, is critical in LPS-induced hepatitis. J. Hepatol. 53, 1059–1068. doi: 10.1016/j.jhep.2010.05.029
Patel, J. R., Williams, J. L., Muccigrosso, M. M., Liu, L., Sun, T., Rubin, J. B., et al. (2012). Astrocyte TNFR2 is required for CXCL12-mediated regulation of oligodendrocyte progenitor proliferation and differentiation within the adult CNS. Acta Neuropathol. 124, 847–860. doi: 10.1007/s00401-012-1034-0
Prinz, J. C. (2011). Autoimmune-like syndromes during TNF blockade: does infection have a role? Nat. Rev. Rheumatol. 7, 429–434. doi: 10.1038/nrrheum.2011.35
Rao, P., Hsu, K. C., and Chao, M. V. (1995). Upregulation of NF-κB-dependent gene expression mediated by the p75 tumor necrosis factor receptor. J. Interferon Cytokine Res. 15, 171–177. doi: 10.1089/jir.1995.15.171
Roerink, M. E., Groen, R. J., Franssen, G., Lemmers-van de Weem, B., Boerman, O. C., and van der Meer, J. W. (2015). Central delivery of iodine-125-labeled cetuximab, etanercept and anakinra after perispinal injection in rats: possible implications for treating Alzheimer’s disease. Alzheimers Res. Ther. 7:70. doi: 10.1186/s13195-015-0149-7
Rothe, M., Pan, M. G., Henzel, W. J., Ayres, T. M., and Goeddel, D. V. (1995a). The TNFR2-TRAF signaling complex contains two novel proteins related to baculoviral inhibitor of apoptosis proteins. Cell 83, 1243–1252.
Rothe, M., Sarma, V., Dixit, V. M., and Goeddel, D. V. (1995b). TRAF2-mediated activation of NF-kappa B by TNF receptor 2 and CD4. Science 269, 1424–1427.
Rothe, M., Wong, S. C., Henzel, W. J., and Goeddel, D. V. (1994). A novel family of putative signal transducers associated with the cytoplasmic domain of the 75 kDa tumor necrosis factor receptor. Cell 78, 681–692. doi: 10.1016/0092-8674(94)90532-0
Shi, J. Q., Shen, W., Chen, J., Wang, B. R., Zhong, L. L., Zhu, Y. W., et al. (2011a). Anti-TNF-alpha reduces amyloid plaques and tau phosphorylation and induces CD11c-positive dendritic-like cell in the APP/PS1 transgenic mouse brains. Brain Res. 1368, 239–247. doi: 10.1016/j.brainres.2010.10.053
Shi, J. Q., Wang, B. R., Jiang, W. W., Chen, J., Zhu, Y. W., Zhong, L. L., et al. (2011b). Cognitive improvement with intrathecal administration of infliximab in a woman with Alzheimer’s disease. J. Am. Geriatr. Soc. 59, 1142–1144. doi: 10.1111/j.1532-5415.2011.03445.x
Sicotte, N. L., and Voskuhl, R. R. (2001). Onset of multiple sclerosis associated with anti-TNF therapy. Neurology 57, 1885–1888. doi: 10.1212/WNL.57.10.1885
Steeland, S., Gorlé, N., Vandendriessche, C., Balusu, S., Brkic, M., Van Cauwenberghe, C., et al. (2018). Counteracting the effects of TNF receptor-1 has therapeutic potential in Alzheimer’s disease. EMBO Mol. Med. 10:e8300. doi: 10.15252/emmm.201708300
Tan, Z. S., Beiser, A. S., Vasan, R. S., Roubenoff, R., Dinarello, C. A., Harris, T. B., et al. (2007). Inflammatory markers and the risk of Alzheimer disease: the Framingham Study. Neurology 68, 1902–1908. doi: 10.1212/01.wnl.0000263217.36439.da
Taoufik, E., Tseveleki, V., Chu, S. Y., Tselios, T., Karin, M., Lassmann, H., et al. (2011). Transmembrane tumour necrosis factor is neuroprotective and regulates experimental autoimmune encephalomyelitis via neuronal nuclear factor- kappaB. Brain 134(Pt 9), 2722–2735. doi: 10.1093/brain/awr203
Tobinick, E., Gross, H., Weinberger, A., and Cohen, H. (2006). TNF-alpha modulation for treatment of Alzheimer’s disease: a 6-month pilot study. MedGenMed 8:25.
Tobinick, E. L., Chen, K., and Chen, X. (2009). Rapid intracerebroventricular delivery of Cu-DOTA-etanercept after peripheral administration demonstrated by PET imaging. BMC Res. Notes 2:28. doi: 10.1186/1756-0500-2-28
Tobinick, E. L., and Gross, H. (2008). Rapid cognitive improvement in Alzheimer’s disease following perispinal etanercept administration. J. Neuroinflammation 5:2. doi: 10.1186/1742-2094-5-2
Wajant, H., Pfizenmaier, K., and Scheurich, P. (2003). Tumor necrosis factor signaling. Cell Death Differ. 10, 45–65. doi: 10.1038/sj.cdd.4401189
Wang, C. Y., Mayo, M. W., Korneluk, R. G., Goeddel, D. V., and Baldwin, A. S. Jr. (1998). NF-κB antiapoptosis: induction of TRAF1 and TRAF2 and c-IAP1 and c-IAP2 to suppress caspase-8 activation. Science 281, 1680–1683. doi: 10.1126/science.281.5383.1680
Wang, T. (2015). TNF-alpha G308A polymorphism and the susceptibility to Alzheimer’s disease: an updated meta-analysis. Arch. Med. Res. 46, 24–30. doi: 10.1016/j.arcmed.2014.12.006
Ware, C. F. (2005). Network communications: lymphotoxins. LIGHT, and TNF. Annu. Rev. Immunol. 23, 787–819. doi: 10.1146/annurev.immunol.23.021704.115719
Williams, S. K., Fairless, R., Maier, O., Liermann, P. C., Pichi, K., Fischer, R., et al. (2018). Anti-TNFR1 targeting in humanized mice ameliorates disease in a model of multiple sclerosis. Sci. Rep. 8:13628. doi: 10.1038/s41598-018-31957-7
Zettlitz, K. A., Lorenz, V., Landauer, K., Münkel, S., Herrmann, A., Scheurich, P., et al. (2010). ATROSAB, a humanized antagonistic anti-tumor necrosis factor receptor one-specific antibody. MAbs 2, 639–647. doi: 10.4161/mabs.2.6.13583
Keywords: tumor necrosis factor, Alzheimer’s disease, neurodegeneration, neuroprotection, agonists, antagonists
Citation: Ortí-Casañ N, Wu Y, Naudé PJW, De Deyn PP, Zuhorn IS and Eisel ULM (2019) Targeting TNFR2 as a Novel Therapeutic Strategy for Alzheimer’s Disease. Front. Neurosci. 13:49. doi: 10.3389/fnins.2019.00049
Received: 29 October 2018; Accepted: 18 January 2019;
Published: 04 February 2019.
Edited by:
Sadayuki Hashioka, Shimane University, JapanReviewed by:
Antonio Lucio Teixeira, University of Texas Health Science Center at Houston, United StatesCopyright © 2019 Ortí-Casañ, Wu, Naudé, De Deyn, Zuhorn and Eisel. This is an open-access article distributed under the terms of the Creative Commons Attribution License (CC BY). The use, distribution or reproduction in other forums is permitted, provided the original author(s) and the copyright owner(s) are credited and that the original publication in this journal is cited, in accordance with accepted academic practice. No use, distribution or reproduction is permitted which does not comply with these terms.
*Correspondence: Ulrich L. M. Eisel, dS5sLm0uZWlzZWxAcnVnLm5s
†These authors have contributed equally to this work
Disclaimer: All claims expressed in this article are solely those of the authors and do not necessarily represent those of their affiliated organizations, or those of the publisher, the editors and the reviewers. Any product that may be evaluated in this article or claim that may be made by its manufacturer is not guaranteed or endorsed by the publisher.
Research integrity at Frontiers
Learn more about the work of our research integrity team to safeguard the quality of each article we publish.