- 1School of Pharmacy, China Medical University, Shenyang, China
- 2China Medical University-The Queen’s University of Belfast Joint College, China Medical University, Shenyang, China
Extrapancreatic nerves project to pancreatic islets directly or converge onto intrapancreatic ganglia. Intrapancreatic ganglia constitute a complex information-processing center that contains various neurotransmitters and forms an endogenous neural network. Both intrapancreatic ganglia and extrapancreatic nerves have an important influence on pancreatic endocrine function. This review introduces the histomorphology, innervation, neurochemistry, and electrophysiological properties of intrapancreatic ganglia/neurons, and summarizes the modulatory effects of intrapancreatic ganglia and extrapancreatic nerves on endocrine function.
Introduction
Intrapancreatic ganglia were first described by Langerhans in 1869 and have been studied over several decades (Sha et al., 1995, 1996, 1997, 2001b; Liu and Kirchgessner, 1997; Love and Szebeni, 1999b; Sha and Szurszewski, 1999; Yi et al., 2005; Shen et al., 2016). While intrapancreatic ganglia have been traditionally considered to be simple parasympathetic relays, growing evidence suggests that, similar to the enteric nervous system (ENS), intrapancreatic ganglia may constitute a complex information-processing center containing various neurotransmitters and form an endogenous neural network (Gylfe and Tengholm, 2014; Shen et al., 2016; Tang et al., 2018b). Although the influence of extrapancreatic nerves, especially autonomic nerves, on pancreatic endocrine function has been extensively studied, the role of intrapancreatic ganglia in endocrine and exocrine functions has garnered far less attention (Sha et al., 2001b; Love et al., 2007; Gylfe and Tengholm, 2014; Rodriguez-Diaz and Caicedo, 2014). In this review, we will focus on research studies from the past 30 years. We will introduce the histomorphology, innervation, neurochemistry, and electrophysiological properties of intrapancreatic ganglia/neurons, and summarize the modulatory effects of intrapancreatic ganglia and extrapancreatic nerves on endocrine function.
Anatomy and Morphology of Intrapancreatic Ganglia
Intrapancreatic ganglia are composed of pancreatic neurons, glial cells, and extrinsic and intrinsic nerve fibers. They are located over or alongside nerve trunks in the interlobular, acinar, or insular connective tissues, or within lobules and islets (Liu and Kirchgessner, 1997; Tang et al., 2018a). Intrapancreatic ganglia can be oval, round, or polygonal in shape, and vary in size (Shen et al., 2016). Most intrapancreatic ganglia are found alongside or inside nerve trunks that are branches of larger nerve trunks traveling along the pancreaticoduodenal artery in the head of the pancreas (Liu and Kirchgessner, 1997). Ganglia are also found along nerve bundles from the celiac and superior mesenteric plexuses, and the splenic nerves in the body and tail of the pancreas (Love et al., 2007). The ganglia in the head are larger and contain more neurons than those in the body and tail of the pancreas (Sha et al., 1996). In the rabbit pancreas, the larger ganglia (≥6 neurons) often appear to be encapsulated and connect to larger nerve trunks, while the smaller ganglia are similar to grape clusters; single pancreatic neurons are also found within islets (Love and Szebeni, 1999b). The peri-lobular ganglia are larger than intra-parenchymal ganglia. The ganglia of patients with type 2 diabetes are larger than those of individuals without diabetes (Tang et al., 2018a). The number of neurons in each pancreatic ganglion is different in different species. For example, rabbit ganglia contain 1 to 35 neurons per ganglion [mean ± standard error of the mean (SEM), 4 ± 1] (Love and Szebeni, 1999b), while rat ganglia contain 2–24 neurons per ganglion (mean ± SEM, 8.8 ± 0.5) (Shen et al., 2016). Although the neurons are typically oval or round, triangular neurons are also found. Most of the neurons are bipolar or multipolar, and unipolar neurons are rare. While the dendritic neuropil of neurons is variable in shape, most of the dendrites are typically short and stubby (Sha et al., 1996). Most nerve fibers of intrapancreatic ganglia are unmyelinated. The unmyelinated nerve bundles have a fine axonal structure, with a large number of microtubules present in the center. Some axons contain both small, clear vesicles and large dense-cored vesicles, while others appear to contain only small, clear vesicles (Love et al., 2007). The axons of pancreatic neurons project to all pancreatic effector cells, including islets, pancreatic ducts, acini, blood vessels, and other intrapancreatic ganglia, indicating that intrapancreatic ganglia may play an important role in pancreatic functions, including endocrine function (Niebergall-Roth and Singer, 2001; Love et al., 2007).
Innervation of Intrapancreatic Ganglia
Sympathetic Efferent Fibers
Preganglionic efferent sympathetic fibers project from cell bodies in the lateral horn of the spinal cord (C8-L3) to paravertebral and prevertebral sympathetic ganglia (Llewellyn-Smith, 2009; Figure 1). The fibers projecting from the prevertebral ganglia (the celiac and superior mesenteric ganglia) enter the pancreas directly or with other autonomic nerves (Ahrén, 2000; Gilon and Henquin, 2001; Love et al., 2007). The celiac plexus mainly includes four branches: the anterior hepatic plexus, posterior hepatic plexus, splenic plexus, and plexus accompanying the transverse pancreatic artery (Dolensek et al., 2015). These branches are distributed in different locations of the human pancreas. The head of the pancreas receives the anterior and posterior hepatic plexuses, which are distributed along the hepatic artery and located in the dorsal aspect of the portal vein. The body and tail of the pancreas are innervated by the other two plexuses. Some nerves originate from the superior mesenteric ganglia and enter the uncinate process of the pancreas along the inferior pancreaticoduodenal artery. There also exist plexuses that surround the main pancreatic duct, but pass through the pancreas independently of the blood vessels (Yi et al., 2003). The postganglionic sympathetic fibers project to intrapancreatic ganglia, islets, ducts, and blood vessels, and release several neurotransmitters such as norepinephrine (NE), galanin, and neuropeptide Y (NPY) (Ahrén, 1999; Di Cairano et al., 2016). Preganglionic sympathetic fibers also enter the pancreas directly and terminate at the intrapancreatic ganglia (Havel et al., 1989).
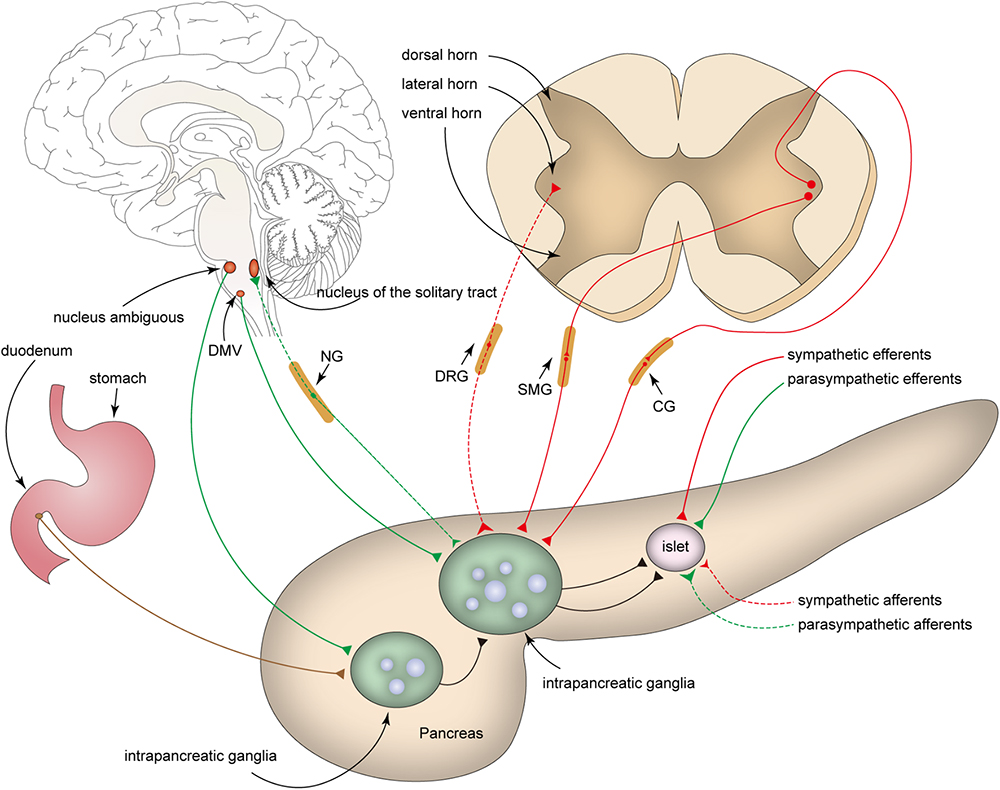
Figure 1. Innervation of intrapancreatic ganglia. Intrapancreatic ganglia receive inputs from sympathetic fibers (red), parasympathetic fibers (green), enteric plexus (brown), and nerves of other intrapancreatic ganglia. The parasympathetic efferent fibers (green solid line) originate from the dorsal motor nucleus of the vagus (DMV) and ambiguous nucleus, whereas the cell bodies of sympathetic efferent fibers (red solid line) project from the lateral horn of the spinal cord to the celiac ganglia (CG), superior mesenteric ganglia (SMG), or paravertebral ganglia (not show in the Figure). The cell bodies of parasympathetic (green dashed line) and sympathetic (red dashed line) afferents are positioned in the nodose ganglia (NG) and the dorsal root ganglia (DRG), respectively.
Parasympathetic Efferent Fibers
Most preganglionic parasympathetic fibers supplying the pancreas originate from the dorsal motor nucleus of the vagus (DMV) in the medulla, and their cell bodies are rarely observed in the nucleus ambiguous (Love et al., 2007; Chandra and Liddle, 2013; Rodriguez-Diaz and Caicedo, 2014; Ma and Vella, 2018; Figure 1). Vagus nerve fibers pass through the hiatus of the esophagus into the abdomen and are divided into five distinct branches that subsequently innervate different organs. The vagus nerve fibers that innervate the pancreas include the hepatic and bilateral gastric branches (primary), and bilateral celiac branches (secondary) (Niebergall-Roth and Singer, 2001; Love et al., 2007; Chandra and Liddle, 2014).
A number of parasympathetic preganglionic fibers terminate at the intrapancreatic ganglia to form synapses (Chandra and Liddle, 2009). Acetylcholine (ACh) is released from both the preganglionic and postganglionic parasympathetic nerve terminals. In addition, vasoactive intestinal polypeptide (VIP), gastrin-releasing peptide (GRP), pituitary adenylate cyclase-activating polypeptide (PACAP), and nitric oxide (NO) are released from the postganglionic parasympathetic nerve fibers (Wang et al., 1999; Gilon and Henquin, 2001; Love et al., 2007; Di Cairano et al., 2016). NPY and galanin exist in both sympathetic and parasympathetic nerve fibers of the pancreas in pigs and mice (Pettersson et al., 1987; Sheikh et al., 1988; Ahrén, 2000).
Enteropancreatic Plexus
The ENS within the wall of the gastrointestinal tract is often considered to be the “local brain” that regulates gastrointestinal functions. Enteric neurons in the myenteric plexus of the gastric antrum and proximal duodenum also project out of the gut to the intrapancreatic ganglia (Kirchgessner and Gershon, 1990; Chandrasekharan and Srinivasan, 2007). Cholinergic enteropancreatic neurons form excitatory nicotinic synapses on pancreatic neurons. In addition, some enteropancreatic nerves, such as the PACAP-containing nerves, are peptidergic. PACAP is often considered as a neuromodulator that strengthens pancreatic secretion (Kirchgessner and Liu, 2001). The fibers of serotonergic enteropancreatic neurons form inhibitory axo-axonic synapses to inhibit amylase secretion (Kirchgessner and Gershon, 1995). Enteropancreatic neurons form nicotinic synapses in intrapancreatic ganglia; however, other excitatory pathways remain unknown. The role of enteric nerves in controlling the endocrine functions of the pancreas has not been completely elucidated. The ENS may be a therapeutic target for the regulation of glucose metabolism (Abot et al., 2018a,b).
Afferent Nerve Fibers
Intrapancreatic ganglia receive both sympathetic and parasympathetic afferents, most of which are sensitive to capsaicin. These afferent fibers transmit sensory information from the pancreas to the central nervous system (Dolensek et al., 2015).
Sympathetic afferents are thought to exit the pancreas along the postganglionic sympathetic fibers within the splanchnic nerves and the celiac plexus to the dorsal root ganglia (DRG, T6-L2) (Love et al., 2007; Rodriguez-Diaz and Caicedo, 2014; Dolensek et al., 2015). Pancreatic parasympathetic afferents originate from the nodose ganglia (NG) (Lartigue, 2016). While pancreatic parasympathetic afferent fibers primarily supply the blood vessels, ducts, acini, and islets, few parasympathetic afferents are found in intrapancreatic ganglia (Love et al., 2007). Although the central targets of vagal afferents remain largely unclear, the nucleus of the solitary tract (NTS) is considered to be the main relay center for vagal afferent nerves (Renehan et al., 1995; Dolensek et al., 2015). Sympathetic and parasympathetic afferent fibers contain either substance P (SP) or calcitonin gene-related peptide (CGRP), or both (Rodriguez-Diaz and Caicedo, 2014).
Neurotransmitters of Intrapancreatic Ganglia
A variety of neurotransmitters can be identified in the intrapancreatic ganglia (Figure 2). Studies show that choline acetyltransferase (CHAT)-immunopositive neuronal cell bodies and nerve fibers are present in the pancreas of many species (Liu et al., 1998; Love and Szebeni, 1999a; Wang et al., 1999), suggesting that ACh is not only released from parasympathetic efferents and enteropancreatic fibers, but also from the neurons in the intrapancreatic ganglia. However, intrapancreatic ganglia are not innervated by cholinergic nerve fibers in the sheep pancreas (Arciszewski and Zacharko-Siembida, 2007), which suggests that the cholinergic innervation of islets from pancreatic neurons is species-dependent.
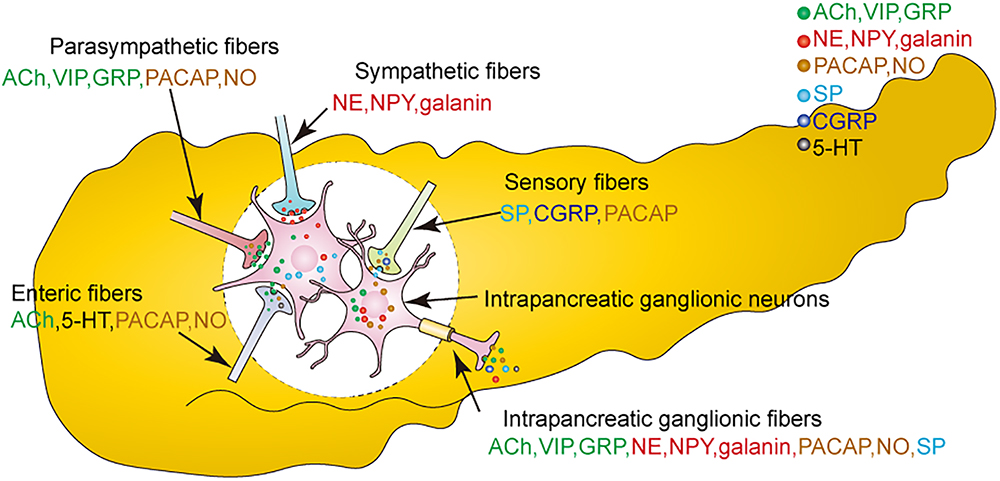
Figure 2. Neurotransmitters are released from both extrinsic nerves and intrinsic intrapancreatic ganglia. Neurotransmitters are, respectively released from parasympathetic fibers, sympathetic fibers, sensory fibers, enteric fibers, the intrapancreatic ganglionic fibers. ACh, acetylcholine; GRP, gastrin-releasing peptide; VIP, vasoactive intestinal peptide; NO, nitric oxide; NE, noradrenaline; NPY, neuropeptide Y; PACAP, pituitary adenylate cyclase activating polypeptide; 5-HT, serotonin; CGRP, calcitonin gene-related peptide; SP, substance P.
It has been shown that intrinsic noradrenaline-containing neurons are present in the pancreases of rats and newborn guinea pigs. Dense catecholamine-containing nerves with few cell bodies are observed in the intrapancreatic ganglia of rabbits. Moreover, high performance liquid chromatography (HPLC) reveals higher levels of NE in the ganglia of the head and neck regions than in those of the body of the pancreas (Yi and Love, 2005a). It has also been reported that CHAT is colocalized with dopamine-β-hydroxylase (DβH), indicating that ACh and NE can be released from the same neurons. However, the origin of these fibers that release both ACh and NE remains unknown (Liu et al., 1998). Recent studies have also shown an increased density of noradrenergic nerve fibers in the islets of obese mice compared to control mice (Giannulis et al., 2014).
Apart from ACh and NE, many neuropeptides are also released from the intrapancreatic ganglia (De Giorgio et al., 1992a). VIP and GRP are located in both neuronal cell bodies and fibers of the intrapancreatic ganglia (De Giorgio et al., 1992b, 1993; Myojin et al., 2000). VIP is present in most intrapancreatic ganglionic cell bodies, while the number of neuronal cell bodies containing GRP is much lower (De Giorgio et al., 1992b). External PACAP originates from vagal nerves, sensory nerves, or enteropancreatic nerves, while intrinsic PACAP has been observed in the neurons of intrapancreatic ganglia (Hannibal and Fahrenkrug, 2000; Kirchgessner and Liu, 2001). PACAP is co-localized with VIP in the neurons of the rat pancreas (Hannibal and Fahrenkrug, 2000). PACAP is also co-localized with galanin, SP, and corticotrophin-releasing factor (CRF) in the intrapancreatic ganglia of the sheep pancreas (Arciszewski et al., 2015). Moreover, both galanin and NPY can be observed in intrapancreatic ganglia of the bovine pancreas (Furuzawa et al., 1996; Myojin et al., 2000; Adeghate et al., 2001), with galanin being more frequently observed than NPY (Myojin et al., 2000).
Besides sensory nerves, approximately 50% of the SP in the pancreas originates from intrapancreatic ganglia (Shen et al., 2016). CGRP-positive fibers are observed in intrapancreatic ganglia, but not in cell bodies (Silvestre et al., 1996; Myojin et al., 2000). Methionine-enkephalin-positive neurons are occasionally found in bovine intrapancreatic ganglia (Myojin et al., 2000).
Nicotinamide adenine dinucleotide phosphate (NADPH)-diaphorase (d) and nitric oxide synthase (NOS) levels have been used as markers of NO production in the intrapancreatic ganglia (Sha et al., 1995; Liu and Kirchgessner, 1997). This demonstrates that apart from enteric and vagal nerve fibers, nitrinergic nerves are also present in the intrapancreatic ganglia (Wang et al., 1999; Chaudhury, 2014). Most NADPH-d-positive neurons show immunoreactivity for VIP or galanin in the chicken pancreas (Hiramatsu et al., 1994). Moreover, ACh is co-localized with VIP or NOS or both in most cholinergic neurons (Myojin et al., 2000).
Electrophysiological Properties and Synaptic Potentials of Pancreatic Neurons
The electrophysiological properties of intrapancreatic ganglionic neurons have been characterized through intracellular recording in guinea pigs, rabbits, cats, and dogs (King et al., 1989; Sha et al., 1996; Liu and Kirchgessner, 1997; Sha and Szurszewski, 1999; Love, 2000). The resting membrane potentials (RMP), input resistances (IR), action potential (AP) thresholds, AP amplitudes, time constant, and after-spike hyperpolarization (ASH) durations are similar in different species, as are the electrophysiological properties of pancreatic neurons in different regions of the pancreas (Sha et al., 1996). Electrophysiological properties of pancreatic neurons are summarized in Table 1. Pancreatic neurons have higher IR than other autonomic neurons. Most of the pancreatic neurons are classified as neurons with phasic firing patterns. A small number (9%) of rabbit pancreatic neurons show spontaneous low amplitude oscillations that resemble pacemaker potentials (Love, 2000).
Pancreatic neurons exhibit both fast and slow excitatory postsynaptic potentials (fEPSPs and sEPSPs). Stimulation of nerve bundles attached to intrapancreatic ganglia evokes multiple fEPSPs (Sha et al., 1996, 1997; Liu and Kirchgessner, 1997; Love, 2000) that are blocked by hexamethonium in 75% of the neurons, while non-cholinergic fEPSPs are observed in 25% of the neurons (Love, 2000). Repetitive stimulation evokes muscarinic and non-cholinergic sEPSPs, whose duration and amplitude are positively related to the stimulus trains; hexamethonium has no impact on the sEPSPs (Sha et al., 1996; Liu and Kirchgessner, 1997). sEPSPs are evoked by repetitive stimulation using atropine or BRL24924, a 5-HT1p receptor antagonist (Sha et al., 1996, 1997). Spontaneous fEPSPs are usually observed at low frequency, and action potentials (APs) are also observed due to summation of subthreshold fEPSPs (King et al., 1989). Approximately 41% of the intrapancreatic neurons in the head and body of the pancreas show spontaneous fEPSPs and APs, while only 31% of the intrapancreatic neurons in the pancreatic tail show spontaneous fEPSPs and APs (Sha et al., 1996). Pancreatic neurons are capable of coordinating intrinsic activity owing to the rhythmic bursts of fEPSPs and APs in interconnected ganglia (Love, 2000). Inhibitory postsynaptic potentials (IPSPs) have not been reported yet. Intrapancreatic ganglia of rabbits in all three regions of pancreas exhibit paired-pulse facilitation (PPF) or depression (PPD) of fEPSPs. PPF peaking and disappearing have shorter inter-stimulus intervals than PPD. PPF is observed mainly in the pancreatic head and neck (60%), while PPD is observed in the body of the pancreas. In the head/neck region, facilitation during the initial 1–2 s of train stimulation is reduced by mid-train depression (2–5 s) of a greater magnitude. The frequency of postsynaptic APs is inversely related to the magnitude of mid-train depression, suggesting that the electrophysiological activities of pancreatic neurons can regulate synaptic strength. Train stimulation in the head or neck of the pancreas is seen to be followed by brief post-train augmentation of fEPSPs (Sha et al., 1996; Love, 2000; Yi and Love, 2005b). Presynaptic post-train depression results in a decrease in ACh release. Regional differences in electrophysiological activities may reflect regional differences in pancreatic function (Yi and Love, 2005b; Love et al., 2007).
Neurotransmitters of external or internal origin are observed to affect ganglionic transmission. In cats, ACh evokes fast depolarization with a decrease in IR, an effect mediated by nicotinic receptors. Most fEPSPs are activated by nicotinic receptor subunits in ganglionic neurons and few fEPSPs are not sensitive to hexamethonium, indicating that there exist non-cholinergic receptors in the ganglia. The slow transmission is mediated by M1 receptors through an increase in the IR (Sha et al., 1997). However, muscarine can convert synaptic APs of rabbit pancreatic neurons into fEPSPs without any changes in the RMP or IR (Love, 2000). The electrical activity of intrapancreatic ganglia has a vital influence on amplitude regulation of pulsatile insulin secretion by isolated ganglia together with the adjacent pancreatic parenchyma (Sha et al., 2001b).
A higher percentage of neurons respond to adrenergic agonists in the pancreatic head than in the body or the tail of the pancreas, a finding consistent with the fact that the density of noradrenergic innervation to intrapancreatic ganglia in the pancreas head is higher than in the body or tail (Yi et al., 2004). A predominance of inhibitory α2 receptors in the body of the pancreas contributes to the inhibition of ganglionic transmission, and excitatory α1 receptors in the ganglia from the head/neck regions participate in the facilitation of ganglionic transmission. Presynaptic post-train depression (PTD) is mediated by NE and other unknown neurotransmitters (Yi and Love, 2005a).
PACAP depolarizes pancreatic neurons via the activation of ganglionic PAC1 receptors and may enhance endocrine secretion (Kirchgessner and Liu, 2001).
While NO evokes membrane hyperpolarization in neurons of the cat pancreas, the membrane IR does not markedly change. Inhibition of NO production may enhance release of ACh to evoke fEPSPs and increase the amplitude of sEPSPs (Sha et al., 1995).
Serotonergic nerve fibers are found in the intrapancreatic ganglia of guinea pigs and cats, but are rare or absent in rabbits (Ma and Szurszewski, 1996b; Liu and Kirchgessner, 1997; Yi et al., 2004). No 5-HT immunoreactive neurons are observed in cat intrapancreatic ganglia (Sha et al., 1996). Neurons in the pancreatic head have a higher excitability in response to 5-HT than those in the tail. The responses of neurons of the intrapancreatic ganglia to 5-HT with fast and slow depolarization are mediated by 5-HT3 and 5-HT1p receptors, respectively (Ma and Szurszewski, 1996b; Sha et al., 1996).
Cholecystokinin (CCK) has been shown to be present in nerve terminals which surround but are not in the intrapancreatic ganglia. The vagal and sympathetic nerve fibers may be the source of CCK in the pancreas. In cats, cholecystokinin octapeptide (CCK-8) can evoke slow depolarization by acting on CCKB postsynaptic receptors, and amplify nicotinic transmission by facilitating the release of ACh from preganglionic nerve terminals or by increasing the sensitivity of postsynaptic membranes to ACh (Cantor and Rehfeld, 1984; Ma and Szurszewski, 1996a).
Investigation of electrophysiological effects of gamma-aminobutyric acid (GABA) on cat pancreatic neurons indicates that GABA acts on the ganglia through GABAA receptors, which can cause depolarization and inhibit fEPSPs (Sha et al., 2001a). While endogenous GABA has been shown to be stored in and released from the ganglionic glial cells, the origin of the GABA remains unclear (Sha et al., 2001a). Leptin promotes fast synaptic transmission by acting on presynaptic nerve terminals of intrapancreatic ganglia in dogs (Sha and Szurszewski, 1999).
Neural Regulation of Islets
Intrapancreatic ganglia are the integration center for extrinsic nerve inputs and intrinsic neuronal inputs. This integration center plays an important role in regulating pancreatic endocrine function. The extrinsic nerves modulate pancreatic endocrine function either directly at the islet level or by going through the integration center (Gylfe and Tengholm, 2014; Di Cairano et al., 2016; Figure 3).
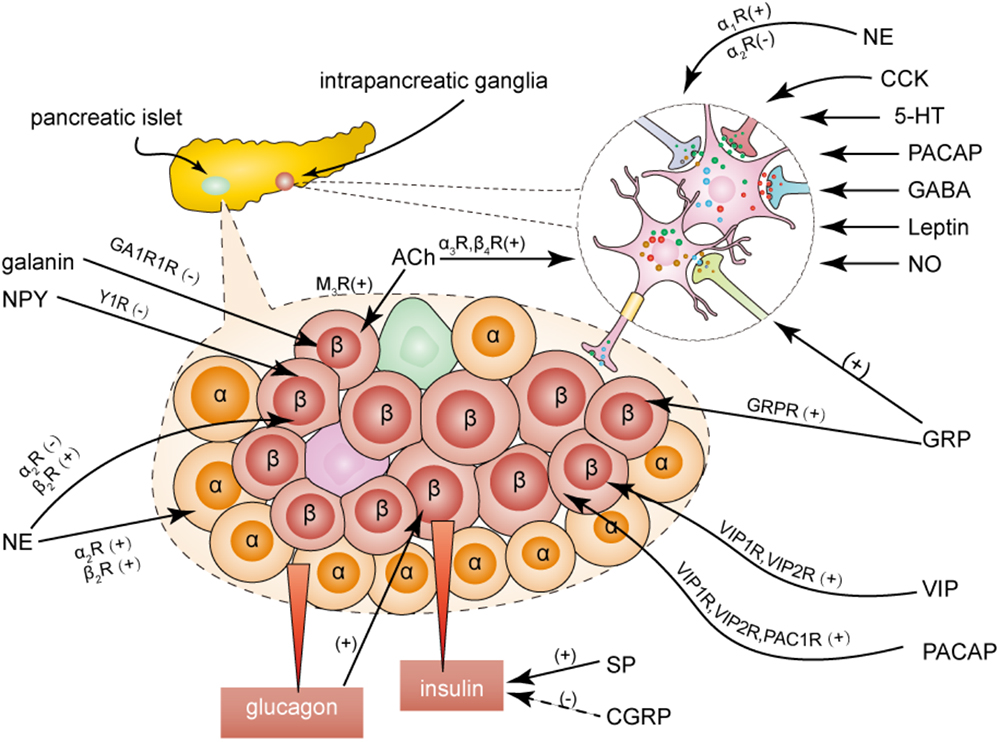
Figure 3. Effects of neurotransmitters on insulin secretion. Neurotransmitters modulate the insulin secretion by activating different receptors both directly at the islets and indirectly at the ganglionic level. ACh, acetylcholine; GRP, gastrin-releasing peptide; VIP, vasoactive intestinal peptide; NO, nitric oxide; NE, noradrenaline; NPY, neuropeptide Y; PACAP, pituitary adenylate cyclase activating polypeptide; 5-HT, serotonin; CCK, cholecystokinin; CGRP, calcitonin gene-related peptide; SP, substance P.
Innervation of Islets
The modes of innervation of pancreatic islets vary across species. In mice, the postganglionic parasympathetic fibers contact all types of endocrine cells (α, β, δ, and PP cells) in the islets, while the postganglionic sympathetic fibers innervate α cells and smooth muscle cells of the blood vessels, but not the β cells (Dolensek et al., 2015). The islets are sparsely innervated in adult humans. Parasympathetic postganglionic fibers are observed to contact exocrine tissue preferentially. Most sympathetic nerves innervate smooth muscle cells of the blood vessels in the adult human pancreas. However, the human fetal pancreas is richly innervated (Taborsky, 2011; Krivova et al., 2016). Islet innervation is correlated with age and environmental factors in humans and rats (Proshchina et al., 2014; Mundinger et al., 2016; Tang et al., 2018b). The integration between epithelial cells and components of the nervous system may form the neuro-insular complexes seen during cellular differentiation (Krivova et al., 2016). In addition, the composition and cytoarchitecture of endocrine cells are also different in humans and rats (Rodriguez-Diaz and Caicedo, 2014; Thorens, 2014; Dolensek et al., 2015; Da Silva Xavier, 2018). Current findings indicate that different species may have different mechanisms for neural regulation of pancreatic endocrine function.
Intrapancreatic Ganglionic Regulation of Islets
As the integrated innervation enters the pancreas, intrapancreatic ganglia directly innervate islets, thereby modulating islet secretion.
Tract-tracing of intrapancreatic ganglionic neurons shows that intrapancreatic ganglia send nerve fibers directly to the islets (Kirchgessner and Pintar, 1991). This ganglion-islet association has been recently demonstrated by a three-dimensional panoramic histology study (Tang et al., 2018b). In vivo ganglionic nicotinic activation stimulates insulin and glucagon secretion in the mouse pancreas (Karlsson and Ahrén, 1998). Cholinergic nerves of the intrapancreatic ganglia regulate the oscillatory pattern of basal endocrine secretion (Karlsson and Ahrén, 1998; Fendler et al., 2009). Activation of postganglionic cholinergic nerves by GRP regulates insulin secretion in vivo (Karlsson et al., 1998). An electrophysiological study in a ganglion-islet attached preparation setting provides direct evidence for the modulation of insulin secretion by the intrapancreatic ganglia. The activity of intrapancreatic ganglionic neurons directly stimulates insulin secretion, while blockage of this activity removes the modulation (Sha et al., 2001b).
Sympathetic Regulation of Islets
Sympathetic nerves regulate pancreatic endocrine secretion with multiple direct and indirect mechanisms through their actions on islets, blood vessels, and intrapancreatic ganglia (Yi et al., 2005). Electrical stimulation of sympathetic nerves induces NE release and has an inhibitory effect on basal and glucose-stimulated insulin release by acting on the α2-adrenoceptor of β cells in dogs and calves. Sympathetic nerves do not participate in the inhibition of basal insulin secretion in rats, pigs, and humans (Ahrén, 2000). NE can also stimulate insulin secretion by acting on the β2-adrenoceptor of β cells or by acting on the α2- and β2-adrenoceptors of α cells, where glucagon secretion leads to an increase in insulin secretion (Ahrén, 2000). In general, the overall effect of NE is lowering the plasma insulin concentration (Rodriguez-Diaz and Caicedo, 2013). In humans, the primary targets of the sympathetic fibers are vascular smooth muscle cells, which can reduce blood flow to regulate secretion (Rodriguez-Diaz and Caicedo, 2013; Dolensek et al., 2015). Patients with type 1 diabetes show a severe loss of islet sympathetic nerves. Moreover, rats with short-term hyperglycemia show a dysfunction in the islet sympathetic innervation from the celiac ganglia, while hyperphagic weaning mice show markedly increased sympathetic innervation compared to control mice (Mundinger et al., 2015, 2016; Tang et al., 2018b).
Activation of the sympathetic pathway potentiates glucagon secretion and inhibits somatostatin secretion. However, the effects of sympathetic nerve activity on pancreatic polypeptide (PP) secretion are different across species, in that it enhances PP secretion in pig and human pancreases, but inhibits PP secretion in the dog pancreas (Ahrén, 1999; Gilon and Henquin, 2001; Rodriguez-Diaz and Caicedo, 2014).
Both NPY and galanin are known to mediate insulin secretion (Chandra and Liddle, 2013). NPY inhibits glucose-induced insulin secretion in rats and mice, but has no effect in dogs (Dunning et al., 1987; Pettersson et al., 1987; Morgan et al., 1998). Galanin inhibits insulin secretion in dogs but not in humans, as the innervation of galanin-containing nerves is abundant in dog islets, but rare in human pancreatic islets (Ahrén and Lindskog, 1992; Ahrén, 2000). Interestingly, both NPY and galanin have an insulinotropic effect on the pig pancreas (Sheikh et al., 1988). NPY binds to the Y1 receptor subtype, while galanin interacts with GA1R1 receptors in β cells to mediate insulin secretion (Parker et al., 1995; Morgan et al., 1998; Chandra and Liddle, 2009). Oral administration of galanin has been shown to improve insulin sensitivity by decreasing duodenal contraction in diabetic mice (Abot et al., 2018b).
Parasympathetic Regulation of Islets
Insulin secretion is stimulated by the electrical activation of parasympathetic fibers and inhibited by vagotomy (Ahrén, 2000; Teff, 2008; Balbo et al., 2016). The density of parasympathetic axons is lower in the exocrine tissue of the pancreas in individuals with new-onset type 1 diabetes (Lundberg et al., 2017) than in control individuals. ACh released by postganglionic nerve fibers acts on muscarinic receptors of β-cells and stimulates insulin secretion directly (Van der Zee et al., 1992). The M3 receptor subtype plays an important role in regulating cholinergic nerves to induce secretion of islet hormones (Karlsson and Ahrén, 1993; Gautam et al., 2006; Miranda et al., 2016). ACh stimulates glucagon and PP secretion that can be blocked by atropine, and the effect of ACh on somatostatin secretion varies across species (Gilon and Henquin, 2001).
VIP, PACAP, and GRP can stimulate the secretion of glucagon and insulin. Given their similar structures, VIP and PACAP have common receptors called VIP1 and VIP2 receptors, whereas the PAC1 receptor is specific for PACAP (Kirchgessner and Liu, 2001). GRP directly acts on the islets via the GRP receptors (Karlsson and Ahrén, 1998). As the nerves containing VIP are found very close to α cells in dogs, α cells are more sensitive to VIP than β cells (Havel et al., 1997).
Sensory Nerve-Mediated Regulation of Islets
Sensory nerves regulate endocrine secretion through two mechanisms: (a) direct action on the islet receptors, and (b) formation of neural circuits with adrenergic nerves (Ahrén, 2000). CGRP inhibits glucose-induced insulin release and stimulates glucagon secretion (Ahrén et al., 1987; Silvestre et al., 1996), but the specific CGRP receptor that mediates these processes has not yet been identified. There is considerable variation in reports of the effects of SP on insulin release (Hermansen, 1980; Chiba et al., 1985; Shen et al., 2016). SP induces a marked increase in insulin secretion from the pancreas of healthy rats, but inhibits insulin secretion from the pancreas of diabetic rats, indicating that it may play a role in the onset of diabetes (Adeghate et al., 2001; Shen et al., 2016). It is generally considered that SP interacts with neurokinin G-protein-coupled receptors (NK-R) on islets. In rats, it is suggested that the role of vagal afferents is to suppress insulin secretion (Meyers et al., 2016). The role of sensory nerves in mediating glucose homeostasis remains unexplored.
Conclusion and Perspectives
Intrapancreatic ganglia constitute endogenous neural networks and release various neurotransmitters. They integrate both intrinsic and external nerve inputs to play an important role in pancreatic endocrine secretion. The mechanisms underlying the role of intrapancreatic ganglia in islet function remain relatively unclear. Further studies are needed to elucidate the complete mechanisms underlying the functions of intrapancreatic ganglia in normal physiological and disease states of the pancreas. This may provide us with novel ways to treat diseases of the pancreas.
Author Contributions
WL researched the articles and wrote the manuscript. GY, YL, and LS revised the draft before submission. All authors have made direct and intellectual contribution to the work, and approved it for publication.
Conflict of Interest Statement
The authors declare that the research was conducted in the absence of any commercial or financial relationships that could be construed as a potential conflict of interest.
Acknowledgments
This work was supported by the National Natural Science Foundation of China (Grant Nos. 81270900 and 81670492).
References
Abot, A., Cani, P. D., and Knauf, C. (2018a). Impact of intestinal peptides on the enteric nervous system: novel approaches to control glucose metabolism and food intake. Front. Endocrinol. 9:328. doi: 10.3389/fendo.2018.00328
Abot, A., Lucas, A., Bautzova, T., Bessac, A., Fournel, A., Le-Gonidec, S., et al. (2018b). Galanin enhances systemic glucose metabolism through enteric nitric oxide synthase-expressed neurons. Mol. Metab. 10, 100–108. doi: 10.1016/j.molmet.2018.01.020
Adeghate, E., Ponery, A. S., and Pallota, D. J. (2001). Distribution of vasoactive intestinal polypeptide, neuropeptide-Y and substance P and their effects on insulin secretion from the in vitro pancreas of normal and diabetic rats. Peptides 22, 99–107. doi: 10.1016/S0196-9781(00)00361-2
Ahrén, B. (1999). Regularion of insulin secretion by nerves and neuropeptides. Ann. Acad. Med. Singapore 28, 99–104.
Ahrén, B. (2000). Autonomic regulation of islet hormone secretion- implications for health and disease. Diabetologia 43, 393–410. doi: 10.1007/s001250051322
Ahrén, B., and Lindskog, S. (1992). Galanin and the regulation of islet hormone secretion. Int. J. Pancreatol. 11, 147–160.
Ahrén, B., Mårtensson, H., and Nobin, A. (1987). Effects of calcitonin gene-related peptide (CGRP) on islet hormone secretion in the pig. Diabetologia 30, 354–359. doi: 10.1007/BF00299030
Arciszewski, M., and Zacharko-Siembida, A. (2007). Cholinergic innervation of the pancreas in the sheep. Acta Biol. Hungar. 58, 151–161. doi: 10.1556/ABiol.58.2007.2.2
Arciszewski, M. B., Mozel, S., and Sienkiewicz, W. (2015). Pituitary adenylate cyclase-activating peptide-27 (PACAP-27) is co-stored with galanin, substance P and corticotropin releasing factor (CRF) in intrapancreatic ganglia of the sheep. Pol. J. Vet. Sci. 18, 343–350. doi: 10.1515/pjvs-2015-0044
Balbo, S. L., Ribeiro, R. A., Mendes, M. C., Lubaczeuski, C., Maller, A. C., Carneiro, E. M., et al. (2016). Vagotomy diminishes obesity in cafeteria rats by decreasing cholinergic potentiation of insulin release. J. Physiol. Biochem. 72, 625–633. doi: 10.1007/s13105-016-0501-9
Cantor, P., and Rehfeld, J. F. (1984). The molecular nature of cholecystokinin in the feline pancreas and related nervous structures. Regul. Pept. 8, 199–208. doi: 10.1016/0167-0115(84)90061-2
Chandra, R., and Liddle, R. A. (2009). Neural and hormonal regulation of pancreatic secretion. Curr. Opin. Gastroenterol. 25, 441–446. doi: 10.1097/MOG.0b013e32832e9c41
Chandra, R., and Liddle, R. A. (2013). Modulation of pancreatic exocrine and endocrine secretion. Curr. Opin. Gastroenterol. 29, 517–522. doi: 10.1097/MOG.0b013e3283639326
Chandra, R., and Liddle, R. A. (2014). Recent advances in the regulation of pancreatic secretion. Curr. Opin. Gastroenterol. 30, 490–494. doi: 10.1097/MOG.0000000000000099
Chandrasekharan, B., and Srinivasan, S. (2007). Diabetes and the enteric nervous system. Neurogastroenterol. Motil. 19, 951–960. doi: 10.1111/j.1365-2982.2007.01023.x
Chaudhury, A. (2014). Similarity in transcytosis of nNOSalpha in enteric nerve terminals and beta cells of pancreatic islet. Front. Med. 1:20. doi: 10.3389/fmed.2014.00020
Chiba, Y., Kawai, K., Okuda, Y., Munekata, E., and Yamashita, K. (1985). Effects of substance P and substance P-(6-11) on hormone release from isolated perfused pancreas- their opposite actions on rat and canine islets. Endocrinology 117, 1996–2000. doi: 10.1210/endo-117-5-1996
Da Silva Xavier, G. (2018). The cells of the islets of langerhans. J. Clin. Med. 7:54. doi: 10.3390/jcm7030054
De Giorgio, R., Sternini, C., Anderson, K., Brecha, N., and Go, V. (1992a). Tissue distribution and innervation pattern of peptide immunoreactivities in the rat pancreas. Peptides 13, 91–98. doi: 10.1016/0196-9781(92)90145-S
De Giorgio, R., Sternini, C., Brecha, N. C., Widdison, A. L., Karanjia, N. D., Reber, H. A., et al. (1992b). Patterns of innervation of vasoactive intestinal polypeptide, neuropeptide y, and gastrin-releasing peptide immunoreactive nerves in the feline pancreas. Pancreas 7, 376–384. doi: 10.1097/00006676-199205000-00016
De Giorgio, R., Sternini, C., Widdison, A., Alvarez, C., Brecha, N., Reber, H., et al. (1993). Differential effects of experimentally induced chronic pancreatitis on neuropeptide immunoreactivities in the feline pancreas. Pancreas 8, 700–710. doi: 10.1097/00006676-199311000-00006
Di Cairano, E. S., Moretti, S., Marciani, P., Sacchi, V. F., Castagna, M., Davalli, A., et al. (2016). Neurotransmitters and neuropeptides: new players in the control of islet of langerhans’ cell mass and function. J. Cell. Physiol. 231, 756–767. doi: 10.1002/jcp.25176
Dolensek, J., Rupnik, M. S., and Stozer, A. (2015). Structural similarities and differences between the human and the mouse pancreas. Islets 7:e1024405. doi: 10.1080/19382014.2015.1024405
Dunning, B., Ahren, B., Böttcher, G., Sundler, F., and Taborsky, G. J. (1987). The presence and actions of NPY in the canine endocrine pancreas. Regul. Pept. 18, 253–265. doi: 10.1016/0167-0115(87)90183-2
Fendler, B., Zhang, M., Satin, L., and Bertram, R. (2009). Synchronization of pancreatic islet oscillations by intrapancreatic ganglia: a modeling study. Biophys. J. 97, 722–729. doi: 10.1016/j.bpj.2009.05.016
Furuzawa, Y., Ohmori, Y., and Watanabe, T. (1996). Immunohistochemical studies of neural elements in pancreatic islets of the cat. J. Vet. Med. Sci. 58, 641–646. doi: 10.1292/jvms.58.641
Gautam, D., Han, S. J., Hamdan, F. F., Jeon, J., Li, B., Li, J. H., et al. (2006). A critical role for beta cell M3 muscarinic acetylcholine receptors in regulating insulin release and blood glucose homeostasis in vivo. Cell Metab. 3, 449–461. doi: 10.1016/j.cmet.2006.04.009
Giannulis, I., Mondini, E., Cinti, F., Frontini, A., Murano, I., Barazzoni, R., et al. (2014). Increased density of inhibitory noradrenergic parenchymal nerve fibers in hypertrophic islets of Langerhans of obese mice. Nutr. Metab. Cardiovasc. Dis. 24, 384–392. doi: 10.1016/j.numecd.2013.09.006
Gilon, P., and Henquin, J. C. (2001). Mechanisms and physiological significance of the cholinergic control of pancreatic beta-cell function. Endocr. Rev. 22, 565–604.
Gylfe, E., and Tengholm, A. (2014). Neurotransmitter control of islet hormone pulsatility. Diabetes Obes. Metab. 16(Suppl. 1), 102–110. doi: 10.1111/dom.12345
Hannibal, J., and Fahrenkrug, J. (2000). Pituitary adenylate cyclase-activating polypeptide in intrinsic and extrinsic nerves of the rat pancreas. Cell Tissue Res. 299, 59–70. doi: 10.1007/s004410050006
Havel, P. J., Dunning, B. E., Vercherec, C. B., Baskin, D. G., Dorisio, T. O., and Taborsky, G. J. Jr. (1997). Evidence that vasoactive intestinal polypeptide is a parasympathetic neurotransmitter in the endocrine pancreas in dogs. Regul. Pept. 71, 163–170. doi: 10.1016/S0167-0115(97)01014-8
Havel, P. J., Gerald, J., and Taborsky, J. R. (1989). The contribution of the autonomic nervous system to changes of glucagon and insulin secretion during hypoglycemic stress. Endocr. Soc. 10, 332–350. doi: 10.1210/edrv-10-3-332
Hermansen, K. (1980). Effects of substance p and other peptides on the release of somatostatin, insulin, and glucagon in vitro. Endocrinology 107, 256–261. doi: 10.1210/endo-107-1-256
Hiramatsu, K., Ohshima, K., and Neurosci, L. (1994). Colocalization of NADPH-diaphorase with neuropeptides in the intrapancreatic neurons of the chicken. Neurosci. Lett. 182, 37–40. doi: 10.1016/0304-3940(94)90199-6
Karlsson, S., and Ahrén, B. (1993). Muscarinic receptor subtypes in carbachol-stimulated insulin and glucagon secretion in the mouse. J. Auton. Pharmacol. 13, 439–446. doi: 10.1111/j.1474-8673.1993.tb00291.x
Karlsson, S., and Ahrén, B. (1998). Insulin and glucagon secretion by ganglionic nicotinic activation in adrenalectomized mice. Eur. J. Pharmacol. 342, 291–295. doi: 10.1016/S0014-2999(97)01508-2
Karlsson, S., Sundler, F., and Ahrén, B. (1998). Insulin secretion by gastrin-releasing peptide in mice-ganglionic versus direct islet effect. Am. Physiol. Soc. 274, 124–129. doi: 10.1152/ajpendo.1998.274.1.E124
King, B. F., Love, J., and Szurszewski, J. (1989). Intracellular recordings from pancreatic ganglia of the cat. J. Physiol. 419, 379–403. doi: 10.1113/jphysiol.1989.sp017877
Kirchgessner, A. L., and Gershon, M. D. (1990). Innervation of the pancreas by neurons in the gut. J. Neurosci. 10, 1626–1642. doi: 10.1523/JNEUROSCI.10-05-01626.1990
Kirchgessner, A. L., and Gershon, M. D. (1995). Presynaptic inhibition by serotonin of nerve-mediated secretion of pancreatic amylase. Am. Physiol. Soc. 268, 339–345. doi: 10.1152/ajpgi.1995.268.2.G339
Kirchgessner, A. L., and Liu, M. T. (2001). Pituitary adenylate cyclase activating peptide (PACAP) in the enteropancreatic innervation. Anatom. Rec. 262, 91–100. doi: 10.1002/1097-0185(20010101)262:1<91::AID-AR1014>3.0.CO;2-2
Kirchgessner, A. L., and Pintar, J. E. (1991). Guinea pig pancreatic ganglia- projections, transmitter content, and the type-specific localization of monoamine oxidase. J. Comp. Neurol. 305, 613–631. doi: 10.1002/cne.903050407
Krivova, Y., Proshchina, A., Barabanov, V., Leonova, O., and Saveliev, S. (2016). Structure of neuro-endocrine and neuro-epithelial interactions in human foetal pancreas. Tissue Cell 48, 567–576. doi: 10.1016/j.tice.2016.10.005
Lartigue, G. D. (2016). Role of the vagus nerve in the development and treatment of diet-induced obesity. J. Physiol. 594, 5791–5815. doi: 10.1113/JP271538
Liu, H. P., Tay, S. S. W., Leong, S. K., and Schemann, M. (1998). Colocalization of ChAT, DbetaH and NADPH-d in the pancreatic neurons of the newborn guinea pig. Cell Tissue Res. 294, 227–231. doi: 10.1007/s004410051172
Liu, M. T., and Kirchgessner, A. L. (1997). Guinea pig pancreatic neurons: morphology, neurochemistry, electrical properties, and response to 5-HT. Am. J. Physiol. 273, 1273–1289. doi: 10.1152/ajpgi.1997.273.6.G1273
Llewellyn-Smith, I. J. (2009). Anatomy of synaptic circuits controlling the activity of sympathetic preganglionic neurons. J. Chem. Neuroanat. 38, 231–239. doi: 10.1016/j.jchemneu.2009.06.001
Love, J. A. (2000). Electrical properties and synaptic potentials of rabbit pancreatic neurons. Auton. Neurosci. 84, 68–77. doi: 10.1016/S1566-0702(00)00187-9
Love, J. A., and Szebeni, K. (1999a). Histochemistry and electrophysiology of cultured adult rabbit pancreatic neurons. Pancreas 18, 65–74.
Love, J. A., and Szebeni, K. (1999b). Morphology and histochemistry of the rabbit pancreatic innervation. Pancreas 18, 53–64. doi: 10.1097/00006676-199901000-00008
Love, J. A., Yi, E., and Smith, T. G. (2007). Autonomic pathways regulating pancreatic exocrine secretion. Auton. Neurosci. 133, 19–34. doi: 10.1016/j.autneu.2006.10.001
Lundberg, M., Lindqvist, A., Wierup, N., Krogvold, L., Dahl-Jorgensen, K., and Skog, O. (2017). The density of parasympathetic axons is reduced in the exocrine pancreas of individuals recently diagnosed with type 1 diabetes. PLoS One 12:e0179911. doi: 10.1371/journal.pone.0179911
Ma, J., and Vella, A. (2018). What has bariatric surgery taught us about the role of the upper gastrointestinal tract in the regulation of postprandial glucose metabolism? Front. Endocrinol. 9:324. doi: 10.3389/fendo.2018.00324
Ma, R. C., and Szurszewski, J. H. (1996a). Cholecystokinin depolarizes neurons of cat pancreatic ganglion. Peptides 17, 775–783.
Ma, R. C., and Szurszewski, J. H. (1996b). 5-Hydroxytryptamine depolarizes neurons of cat pancreatic ganglia. J. Auton. Nerv. Syst. 57, 78–86.
Meyers, E. E., Kronemberger, A., Lira, V., Rahmouni, K., and Stauss, H. M. (2016). Contrasting effects of afferent and efferent vagal nerve stimulation on insulin secretion and blood glucose regulation. Physiol. Rep. 4:e12718. doi: 10.14814/phy2.12718
Miranda, R. A., Torrezan, R., de Oliveira, J. C., Barella, L. F., da Silva Franco, C. C., Lisboa, P. C., et al. (2016). HPA axis and vagus nervous function are involved in impaired insulin secretion of MSG-obese rats. J. Endocrinol. 230, 27–38. doi: 10.1530/JOE-15-0467
Morgan, D., Kulkarni, R., Hurley, J., Wang, Z. L., Wang, R. M., Ghatei, M. A., et al. (1998). Inhibition of glucose stimulated insulin secretion by neuropeptide Y is mediated via the Y1 receptor and inhibition of adenylyl cyclase in RIN 5AH rat insulinoma cells. Diabetologia 41, 1482–1491. doi: 10.1007/s001250051095
Mundinger, T. O., Cooper, E., Coleman, M. P., and Taborsky, G. J. Jr. (2015). Short-term diabetic hyperglycemia suppresses celiac ganglia neurotransmission, thereby impairing sympathetically mediated glucagon responses. Am. J. Physiol. Endocrinol. Metab. 309, E246–E255. doi: 10.1152/ajpendo.00140.2015
Mundinger, T. O., Mei, Q., Foulis, A. K., Fligner, C. L., Hull, R. L., and Taborsky, G. J. Jr. (2016). Human type 1 diabetes is characterizedby an early,marked, sustained,and islet-selective loss of sympathetic nerves. Diabetes 65, 2322–2330. doi: 10.2337/db16-0284/-/DC1
Myojin, T., Kitamura, N., Hondo, E., Baltazar, E., Pearson, G., and Yamada, J. (2000). Immunohistochemical localization of neuropeptides in bovine pancreas. Anat. Histol. Embryol. 29, 167–172. doi: 10.1046/j.1439-0264.2000.00257.x
Niebergall-Roth, E., and Singer, M. V. (2001). Central and peripheral neural control of pancreatic exocrine secretion. J. Physiol. Pharmacol. 52, 523–538.
Parker, E. M., Izzarelli, D. G., Nowak, H. P., Mahle, C. D., Iben, L. G., Wang, J. C., et al. (1995). Cloning and characterization of the rat GALR1 galanin receptor from Rin14B insulinoma cells. Brain Res. 34, 179–189. doi: 10.1016/0169-328X(95)00159-P
Pettersson, M., Ahrén, B., Lundquist, I., Böttcher, G., and Sundler, F. (1987). Neuropeptide Y: intrapancreatic neuronal localization and effects on insulin secretion in the mouse. Cell Tissue Res. 248, 43–48. doi: 10.1007/BF01239960
Proshchina, A. E., Krivova, Y. S., Barabanov, V. M., and Saveliev, S. V. (2014). Ontogeny of neuro-insular complexes and islets innervation in the human pancreas. Front. Endocrinol. 5:57. doi: 10.3389/fendo.2014.00057
Renehan, W. E., Zhang, X., Beierwaltes, W. H., and Fogel, R. (1995). Neurons in the dorsal motor nucleus of the vagus may integrate vagal and spinal information from the GI tract. Am. Physiol. Soc. 268, 780–790. doi: 10.1152/ajpgi.1995.268.5.G780
Rodriguez-Diaz, R., and Caicedo, A. (2013). Novel approaches to studying the role of innervation in the biology of pancreatic islets. Endocrinol. Metab. Clin. North Am. 42, 39–56. doi: 10.1016/j.ecl.2012.11.001
Rodriguez-Diaz, R., and Caicedo, A. (2014). Neural control of the endocrine pancreas. Best Pract. Res. Clin. Endocrinol. Metab. 28, 745–756. doi: 10.1016/j.beem.2014.05.002
Sha, L., Love, J. A., Ma, R., and Szurszewski, J. H. (1997). Cholinergic transmission in pancreatic ganglia of the cat. Pancreas 14, 83–93. doi: 10.1097/00006676-199701000-00013
Sha, L., Miller, S., and Szurszewski, J. H. (2001a). Electrophysiological effects of GABA on cat pancreatic neurons. Am. J. Physiol. Gastrointest. Liver Physiol. 280, 324–331.
Sha, L., Westerlund, J., Szurszewski, J. H., and Bergsten, P. (2001b). Amplitude modulation of pulsatile insulin secretion by intrapancreatic ganglion neurons. Diabetes 50, 51–55.
Sha, L., Miller, S. M., and Szurszewski, J. H. (1995). Nitric oxide is a neuromodulator in cat pancreatic ganglia_ histochemical and electrophysiological study. Neurosci. Lett. 192, 77–80. doi: 10.1016/0304-3940(95)11614-3
Sha, L., Ou, L., Miller, S. M., Ma, R., and Szurszewski, J. H. (1996). Cat pancreatic neurons morphology, electrophysiological properties, and responses to 5-HT. Pancreas 13, 111–124. doi: 10.1097/00006676-199608000-00001
Sha, L., and Szurszewski, J. H. (1999). Leptin modulates fast synaptic transmission in dog pancreatic ganglia. Neurosci. Lett. 263, 93–96. doi: 10.1016/S0304-3940(99)00122-6
Sheikh, S. P., Holst, J. J., Skak-Nielsen, T., Knigge, U., Warberg, J., Theodorsson-Norheim, E., et al. (1988). Release of NPY in pig pancreas: dual parasympathetic and sympathetic regulation. Am. J. Physiol. 255(1 Pt 1), G46–G54. doi: 10.1152/ajpgi.1988.255.1.G46
Shen, Q., Wang, Y., Zhang, N., Gao, D., Liu, Y., and Sha, L. (2016). Substance P expresses in intrapancreatic ganglia of the rats. Neuropeptides 59, 33–38. doi: 10.1016/j.npep.2016.06.004
Silvestre, R. A., Salas, M., Rodriguez-Gallardo, J., Garcia-Hermida, O., Fontela, T., and Marco, J. (1996). Effect of (8 - 32) salmon calcitonin, an amylin antagonist, on insulin, glucagon and somatostatin release study in the perfused pancreas of the rat.pdf. Br. J. Pharmacol. 117, 347–350. doi: 10.1111/j.1476-5381.1996.tb15197.x
Taborsky, G. J. Jr. (2011). Islets have a lot of nerve! or do they? Cell Metab. 14, 5–6. doi: 10.1016/j.cmet.2011.06.004
Tang, S. C., Baeyens, L., Shen, C. N., Peng, S. J., Chien, H. J., Scheel, D. W., et al. (2018a). Human pancreatic neuro-insular network in health and fatty infiltration. Diabetologia 61, 168–181. doi: 10.1007/s00125-017-4409-x
Tang, S. C., Shen, C. N., Lin, P. Y., Peng, S. J., Chien, H. J., Chou, Y. H., et al. (2018b). Pancreatic neuro-insular network in young mice revealed by 3D panoramic histology. Diabetologia 61, 158–167. doi: 10.1007/s00125-017-4408-y
Teff, K. L. (2008). Visceral nerves_ vagal and sympathetic innervation. J. Parenteral. Enteral. Nutr. 32, 569–571. doi: 10.1177/0148607108321705
Thorens, B. (2014). Neural regulation of pancreatic islet cell mass and function. Diabetes Obes. Metab. 16(Suppl. 1), 87–95. doi: 10.1111/dom.12346
Van der Zee, E. A., Buwalda, B., Strubbe, J. H., Strosberg, A. D., and Luiten, P. G. M. (1992). Immunocytochemical localization of muscarinic acetylcholine receptors in the rat endocrine pancreas. Cell Tissue Res. 269, 99–106. doi: 10.1007/BF00384730
Wang, J., Zheng, H., and Berthoud, H. R. (1999). Functional vagal input to chemically identified neurons in pancreatic ganglia as revealed by Fos expression. Am. Physiol. Soc. 277, 958–964. doi: 10.1152/ajpendo.1999.277.5.E958
Yi, E., and Love, J. A. (2005a). Alpha-adrenergic modulation of synaptic transmission in rabbit pancreatic ganglia. Auton. Neurosci 122, 45–57. doi: 10.1016/j.autneu.2005.07.008
Yi, E., and Love, J. A. (2005b). Short-term synaptic plasticity in rabbit pancreatic ganglia. Auton. Neurosci. 119, 36–47. doi: 10.1016/j.autneu.2005.03.001
Yi, E., Smith, T. G., Baker, R. C., and Love, J. A. (2004). Catecholamines and 5-hydroxytryptamine in tissues of the rabbit exocrine pancreas. Pancreas 29, 218–224. doi: 10.1097/00006676-200410000-00007
Yi, E., Smith, T. G., and Love, J. A. (2005). Noradrenergic innervation of rabbit pancreatic ganglia. Auton. Neurosci. 117, 87–96. doi: 10.1016/j.autneu.2004.11.004
Keywords: intrapancreatic ganglia, extrapancreatic nerves, pancreas, islet, insulin
Citation: Li W, Yu G, Liu Y and Sha L (2019) Intrapancreatic Ganglia and Neural Regulation of Pancreatic Endocrine Secretion. Front. Neurosci. 13:21. doi: 10.3389/fnins.2019.00021
Received: 12 July 2018; Accepted: 10 January 2019;
Published: 20 February 2019.
Edited by:
Lee E. Eiden, National Institutes of Health (NIH), United StatesReviewed by:
Youssef Anouar, Institut National de la Santé et de la Recherche Médicale (INSERM), FranceQingchun Tong, The University of Texas Health Science Center at Houston, United States
Copyright © 2019 Li, Yu, Liu and Sha. This is an open-access article distributed under the terms of the Creative Commons Attribution License (CC BY). The use, distribution or reproduction in other forums is permitted, provided the original author(s) and the copyright owner(s) are credited and that the original publication in this journal is cited, in accordance with accepted academic practice. No use, distribution or reproduction is permitted which does not comply with these terms.
*Correspondence: Lei Sha, bHNoYUBjbXUuZWR1LmNu