- 1Behavioural Ecology Research Group, Department of Biology, Anglia Ruskin University, Cambridge, United Kingdom
- 2Biological Anthropology, Department of Archaeology, University of Cambridge, Cambridge, United Kingdom
- 3Department of Anthropology, Stony Brook University, Stony Brook, NY, United States
Understanding the nature of the relationship between vocal complexity and brain architecture across non-human primates may help elucidate some of the key elements underlying the evolution of human speech. Here, we report a positive correlation between vocal repertoire size and the relative size of cortical association areas (governing voluntary control over behavioural output) in non-human primates. We further demonstrate that a hominid grade shift in the relative volume of cortical association areas coincides with a similar grade shift in the hypoglossal nucleus (which is associated with the cranial nerve that innervates the muscles of the tongue). Our results support a qualitative continuity in the neural correlates of vocal repertoire, but a quantitative discontinuity in the extent to which the neural system supporting speech is innervated by cortical association areas in great apes and humans.
Introduction
Relative to humans, non-human primates (hereinafter “primates”) produce a very limited range of vocalisations. However, vocal repertoire varies widely among primate species, ranging from just two call types in Calabar angwantibos (Arctocebus calabarensis) to at least 38 call types in bonobos (Pan paniscus) (McComb and Semple, 2005). Understanding the evolutionary basis for such variation in vocal repertoire among our closest relatives may provide important insight into how a communication system as complex as human speech evolved.
The basic layout of the larynx and vocal tract is highly conservative and homologous in both form and function among virtually all terrestrial mammals, including humans (Fitch, 2000, 2006, 2010; Fitch and Zuberbuhler, 2013; Fitch et al., 2016). This strongly suggests that differences in vocal repertoire among primate species do not result from differences in vocal tract morphology (Fitch et al., 2016; Boë et al., 2017). Indeed, the macaque vocal tract has recently been shown to be “speech ready,” i.e., capable of producing an adequate range of speech sounds to support spoken language (Fitch et al., 2016). However, despite many attempts, no non-human primate has ever been trained to produce speech sounds.
One key factor that has been suggested to influence vocal repertoire is neural control (Fitch, 2010). This hypothesis posits that the reason primates are incapable of producing speech is because they lack adequate brain mechanisms to control and coordinate vocal production. The possibility has long been recognised; after examining the comparative data on vocal anatomy, Darwin concluded that “the development of the brain has no doubt been far more important” (Darwin, 1871). Nevertheless, this hypothesis has received surprisingly little attention, and, to date, there have been no comparative studies relating vocal repertoire to neuro-anatomy in primates.
Here, we test the hypothesis that vocal repertoire size in primates is associated with neural substrates of increased voluntary control over behavioural output. Two neural features are crucial in this regard: (1) the cortical association areas that govern voluntary control over behavioural output (Fuster, 1997; Miller, 2000); and (2) the brainstem nuclei that are involved in the neural control of the orofacial muscles (Sherwood et al., 2005). If voluntary control is an important element in vocal complexity and, by extension, the evolution of speech, we might expect these two neural features to be associated with each other and with vocal repertoire size. We also test for associations between vocal repertoire size and measures of both overall brain size and its main gross anatomical substructure, the neocortex.
Materials and Methods
Vocal Repertoire
We obtained data on primate vocal repertoire (the number of acoustically different calls that a species gives) from the literature. Following (McComb and Semple, 2005), we applied a series of rules when collecting data on vocal repertoire in order to make comparisons across species as systematic as possible. We only considered studies that reported the whole adult repertoire (excluding calls given exclusively by infants and juveniles) and which distinguished the calls on the basis of their acoustic structure. This led to the exclusion of studies that did not report the complete repertoire, or that only distinguished calls by the context in which they were given. To make all data consistent with that reported in (McComb and Semple, 2005), we did not consider lip smacking, teeth chattering/grinding or vomiting as part of the repertoire, and only included vocalisations that consisted of multiple units if any of these units had not previously been included as a distinct call in the repertoire. After applying these rules to each study, the vocal repertoire that we included in our analysis often differed from that reported in the original study. Humans were not included in these analyses, owing to the difficulty in quantifying vocal repertoire. Raw data are reported in Supplementary Table S1.
Brain Region Volumes
We obtained brain region volumes (i.e., volumes of gross-anatomical structures and of areas within those structures) from published studies (Stephan et al., 1981; Sherwood et al., 2005; Smaers et al., 2010, 2011, 2013), all of which report data for the same specimens housed at the Vogt Institute for Brain Research (Zilles et al., 2011). We analysed three cortical association areas – the prefrontal cortex, the frontal motor cortex, and the temporal-parietal cortex; three brain stem nuclei – the trigeminal, facial, and hypoglossal nuclei; as well as brain volume and neocortical volume. All raw data are provided in Supplementary Table S1.
Measures of Relative Size of Brain Regions
We only considered brain region volumes after controlling for overall size by using control variables that are functionally and neuroanatomically relevant (Dechmann and Safi, 2009; Passingham and Smaers, 2014). We controlled brain regions for size by obtaining residuals from a regression analysis. For the relative volume of the brain size, we used body size as the independent variable. For the relative volume of the neocortex, we used the rest of brain size as the independent variable. For the relative volume of brain stem nuclei, we used the volume of the rest of the brainstem as the independent variable. For the relative volume of cortical association areas, we used cortical primary sensory areas as the independent variable. Raw data are reported in Supplementary Table S1. We used different independent variables for these different neural regions considering the nature of their different functional and anatomical modularity and interconnectivity. It is crucial that the choice of appropriate comparative regions is made based on the functional and neuroanatomical realities of neural information processing. It has long been established that information processing in the brain occurs in a hierarchical manner. Stimuli are initially mapped onto primary sensory areas, and information subsequently projects to higher order association areas (Passingham et al., 2016). Throughout this process, perception, and interpretation of stimuli becomes increasingly complex (Desimone and Schein, 1987; Miller and Cohen, 2001). When aiming to assess the neural substrates of complex cognitive processing, an approach that adequately accounts for the functional and neuroanatomical underpinnings of neural information processing is to compare regions at the top of the hierarchy of information processing (heteromodal association areas) to those at the beginning of the hierarchy (primary sensory areas) (Passingham and Smaers, 2014). This approach effectively compares the amount of complex information processing relative to the amount of sensory input and is different to the more traditional procedure of comparing the size of a brain region to the size of the rest of the brain. This more traditional procedure is undesirable because it underestimates changes in neural systems (Passingham and Smaers, 2014) and erroneously assumes that neural information processing is isolated in particular regions, hereby ignoring the well-established hierarchical nature of neural information processing. Although it is advised to only consider relative volumes based on neurobiologically meaningful comparisons, we also checked results when calculating relative volumes compared to “rest of brain” in order facilitate comparisons with previous studies. These analyses provided similar results.
Group Size
One factor that is thought to act as a selection pressure driving increases in vocal repertoire is sociality. The “social complexity hypothesis” for communication posits that animals with complex social systems require more complex communicative systems to regulate interactions and relations among group members (Freeberg et al., 2012). Indeed, there is now evidence in a diverse range of animal taxa, in different communicative modalities, that complexity in social groups is related to, and/or can drive complexity in signalling systems (Freeberg, 2006; delBarco-Trillo et al., 2012; Krams et al., 2012; Pollard and Blumstein, 2012; White et al., 2012). For example, McComb and Semple (2005) found a positive association between vocal repertoire and both group size and grooming rate (a measure of the strength of social bonding between individuals in a group) among non-human primates. Therefore, there is a need to control for the confounding effect of social group size on vocal repertoire when analysing the relationship between brain architecture and vocal repertoire.
In most primates, individuals form clearly defined social groups, which are relatively stable over time. However, some species form temporary parties or subgroups during foraging (e.g., Macaca fascicularis, Pan troglodytes). Therefore, we have distinguished between three types of social groups: (1) the foraging group (ForGroup), being the animals that forage together; (2) the population group (PopGroup), being the animals that share a common range or at least come together frequently, usually sleep together, and among which foraging units have highly overlapping ranges [data from Clutton-Brock and Harvey (1977) and Nunn and van Schaik (2002) in both cases]; (3) for the sake of comparison, we also analysed the group size data originally reported in McComb and Semple (2005) (MSgroup), which represent a midpoint of a range given in Rowe (1996). This may underestimate group size when solitary, perhaps dispersing individuals, are included as the lower group size in the primary reference. We use PopGroup as our principle measure of group size in the main text, as this is the most biologically meaningful measure, and report equivalent results for ForGroup and MSgroup in Supplementary Table S2. Raw data are reported in Supplementary Table S1.
Statistical Methods
Comparative data points are not expected to be independent because of their shared phylogenetic history. To account for this data non-independence we use phylogenetic generalised least-squares procedures to analyse our data. We used phylogenetic analysis of covariance (pANCOVA) (Smaers and Rohlf, 2016; Smaers and Mongle, 2018) to assess the occurrence of grade shifts among primate clades in the relative volumes of brain regions and phylogenetic generalised least squares regression analysis [pGLS; Rohlf et al. (2001)] with a likelihood-fitted lambda parameter (Pagel, 1999) to test for correlations between vocal repertoire size and the relative size of brain regions. We calculated confidence intervals following Smaers and Rohlf (2016). The phylogeny (Supplementary Figure S1) was taken from the 10k Trees Project (Arnold et al., 2010). All data were log transformed prior to analysis.
Before deriving allometric residuals, we followed standard statistical procedures to test for significant differences in intercepts and slopes before interpreting allometry (Sokal and Rohlf, 2012). When subgroups indicate either a difference in slope, or a difference in intercept they should be considered as part of different allometry (Rao and Toutenburg, 1999). Therefore, if a multi-grade allometry was found to be a significantly better fit to the data than a single-grade allometry (Smaers and Rohlf, 2016), residuals were derived from the ancestral grade. For completeness, we also ran analyses using a single allometry. These analyses provided similar results.
Results
Our results indicate a significant positive association between vocal repertoire and the relative size of all three cortical association areas under investigation (Figure 1A: F = 11.23, P = 0.003, df = 2,10; Figure 1B: F = 15.05, P < 0.001, df = 2,10; Figure 1C: F = 12.72, P = 0.002, df = 2,10). Further analyses reveal no positive associations between vocal repertoire and the relative volume of the brain or neocortex, nor of any of the brain stem nuclei (Supplementary Figure S2). Using ForGroup, or MSGroup, instead of PopGroup as a control for vocal repertoire yielded equivalent results (Supplementary Table S2). Either considering or not considering the grade shift when calculating the relative size of brain region also produced equivalent results. Using absolute brain region volumes, rather than relative volumes, led to non-significant results (Supplementary Figure S3).
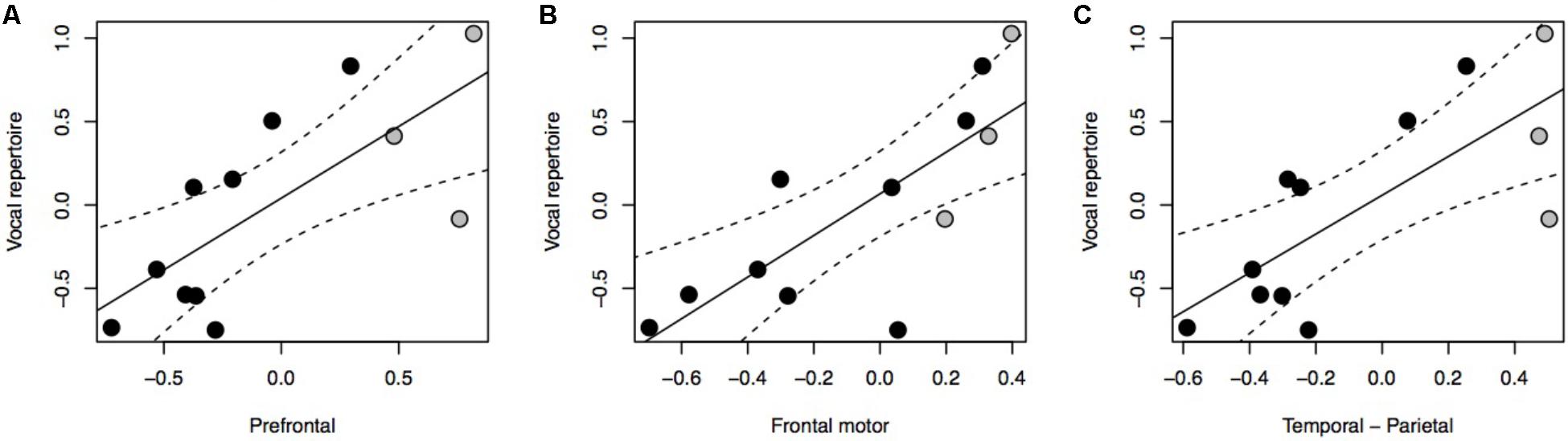
FIGURE 1. pGLS regressions of the residual volume of brain regions (A, prefrontal cortex; B, frontal motor cortex; C, temporal-parietal cortex) versus residual vocal repertoire. We represent 95% confidence intervals as dashed lines. Monkeys are represented in black, non-human apes in gray.
The relationship between brain stem nuclei and cortical association areas was not significant when considering all species in the sample (Supplementary Figure S4). This primate-general trend, however, masks a significant relationship with prefrontal cortex and a marked positive trend with other cortical association areas in apes (Figure 2A: F = 81.85, P = 0.012, df = 2,2; Figure 2B: F = 14.5, P = 0.065, df = 2,2; Figure 2C: F = 17.39, P = 0.054, df = 2,2). This hominoid trend is confirmed by a significant difference in the hominid versus non-hominid allometry for both the cortical association areas (no difference in slope, but a difference in intercept; Smaers et al., 2017) and the relative size of the hypoglossal nucleus (difference in slope: F = 9.274, P = 0.004) (Table 1).
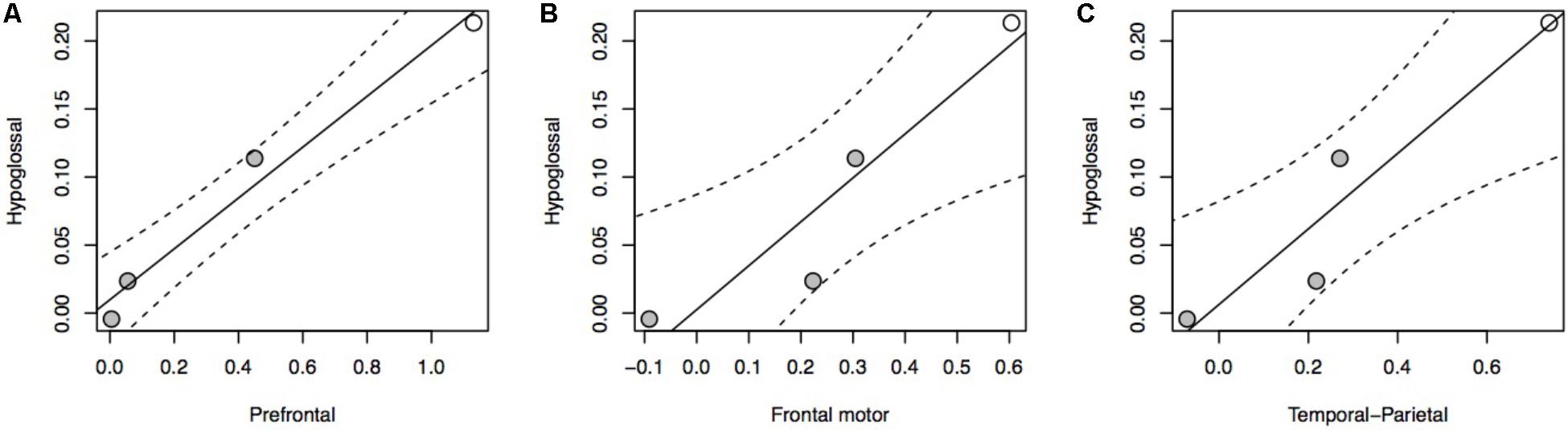
FIGURE 2. pGLS regressions of the residual volume of brain regions (A, prefrontal cortex; B, frontal motor cortex; C, temporal-parietal cortex) versus the residual volume of the hypoglossal nucleus in apes. We represent 95% confidence intervals as dashed lines. Non-human apes are represented in gray, humans in white.
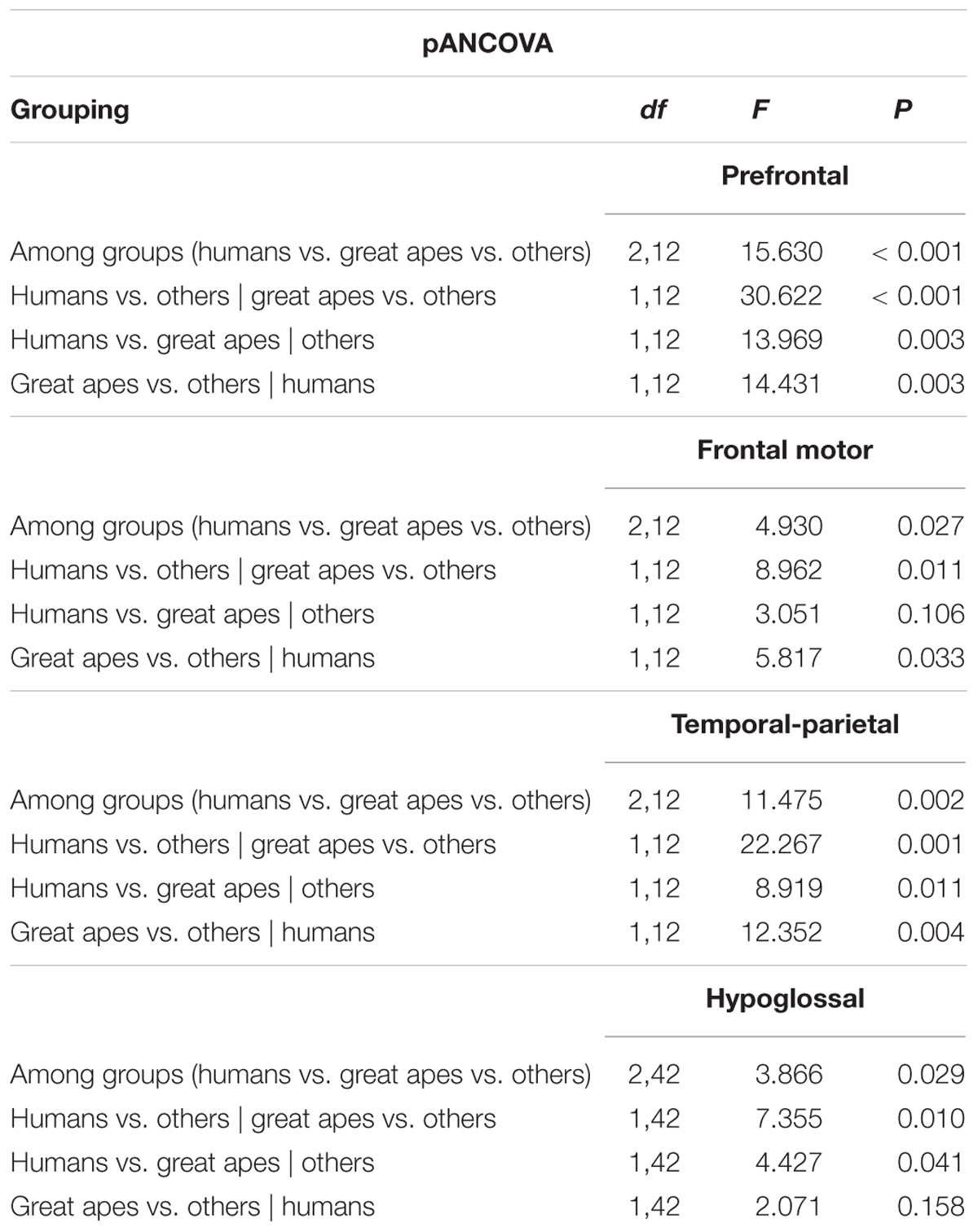
TABLE 1. Results from a phylogenetic ancova procedure on the relative size of cortical association areas and relative hypoglossal nucleus size, examining evidence for grade shifts among different primate clades.
Discussion
The brain is central to the adaptive profile of any animal, as it underlies the capacity to modify behaviour. Therefore, natural selection shaping behavioural capacities is likely to be reflected in changes to the systems mediating those capacities. Indeed, there is strong evidence for specific brain adaptations for different locomotor strategies (de Winter and Oxnard, 2001), activity timing, diet, and habitat (Barton et al., 1995; Barton, 1996), spatial learning and memory (Jacobs et al., 1990), visual specialisation (Barton, 1998, 2004), sex differences in behaviour (Jacobs, 1996; Lindenfors et al., 2007), and variation in group size (Dunbar, 1992). Among birds, there is a significant correlation between the relative volume of song control nuclei and the number of song types typically found in the repertoire (Devoogd et al., 1993), however, to date there has been no similar research among mammals.
Several studies have highlighted changes in brain size as a key factor in species adaptation. For example, bigger relative brain size has been related to higher cognitive ability among primates (Deaner et al., 2007) and increased survival in mammals, birds, and reptiles (Sol et al., 2005, 2008; Amiel et al., 2011). Both relative brain size and relative neocortical volume have also been cited as important elements in the evolution of human speech, partly because of their positive correlation with group size (Aiello and Dunbar, 1993; Dunbar, 2010). Moreover, measures of overall brain size have recently been suggested to be associated to aspects of complex behaviour such as self-control (MacLean et al., 2014) and manipulation complexity (Heldstab et al., 2016). The use of overall brain size as a relevant measure to explain variation in behavioural complexity, however, remains contentious because it does not accurately represent critical neurobiological features such as modularity and interconnectivity (Chittka and Niven, 2009).
Here, we found no evidence that brain size or neocortex size (neither relative nor overall) were positively correlated with vocal repertoire size. However, when considering functionally specific, cytoarchitectonically defined areas of gross-anatomical structures, strong correlations were revealed. We focus on cortical association areas and demonstrate significant positive correlations with vocal repertoire among primates. Cortical association areas are areas within the neocortex that underlie the higher cognitive processing capacities often considered to be the foundation for the complex forms of behaviour observed in primates (Miller, 2000). The prefrontal cortex, for example, specifies the spatial and object goals of action, which can include a series of goals in the context of planning or performing sequences of action (Mushiake et al., 2006; Shima et al., 2007; Yamagata et al., 2012). Prefrontal-parietal function has been argued to aid in reducing errors during foraging choices and to provide a context for generating foraging goals (Genovesio et al., 2014). Overall, the nature of the processing capacities that underlie the cortical association areas can be understood as an increased voluntary control over behavioural output governed by an increased foresight, insight, and hindsight in the potential relevance of environmental cues (Fuster, 1997; Miller and Cohen, 2001; Passingham et al., 2016). Our findings that the relative sizes of cortical association areas are strongly correlated with vocal repertoire size suggest that vocal complexity coevolved with increases in such higher cognitive processing capacities. However, more research is necessary to understand the exact causal factors driving this relationship.
Particularly intriguing is the positive association between the hypoglossal nucleus and the cortical association areas in hominoids, most strongly observed with the prefrontal cortex. These results align with previous work reporting direct corticoefferent projections to the orofacial motor neurons in humans (Iwatsubo et al., 1990; Jürgens and Alipour, 2002; Simonyan and Jürgens, 2003), but an absence of such direct projections in macaques (Jürgens and Alipour, 2002). Direct neocortical projections to motor neurons in the human brain stem have traditionally been interpreted as indicative of increased cognitive control over the muscles that are innervated by those motor neurons (Kuypers, 1958; Pearce et al., 2003; Rödel et al., 2003). Our results confirm these earlier findings and suggest that the increased corticoefferent projections in hominoids and humans may stem primarily from cortical association areas (and the prefrontal cortex in particular). The positive trend in hominoids between cortical association areas and the hypoglossal nucleus suggests an increased cognitive control over the tongue in hominoids, with humans lying at the high end of this correlation.
One key difference that has been highlighted between human speech and primate vocalisations is that the former is learned while the latter is innate or reflexive (Fitch, 2000). In light of our results, this difference may be understood as an expansion of the voluntary control over vocalisations in hominoids and humans. Given that both the cortical association areas (Deacon, 1990) and the hypoglossal nucleus are significantly expanded in great apes and humans, it may be argued that the cortical association areas exerted more influence over the innervation of the tongue. Larger neural areas are known to exert more influence over overall brain function by means of increased connexional invasion (Deacon, 1990). This process allows hypertrophied areas to invade targets they did not innervate ancestrally, and/or to increase target innervation relative to the ancestral condition. Such new connexions may displace old connexions causing the hypertrophied areas to exert more influence over brain functioning.
Overall, our results support the idea of a qualitative continuity in the association between vocal repertoire and higher cognitive processing capacities by demonstrating a significant comparative correlation between cortical association areas and vocal repertoire size across non-human primates. Our results also suggest a quantitative discontinuity of prefrontal inputs supporting the transition from more reflexive vocalisations in non-human primates toward more learned complex vocalisations underlying human speech. We suggest that this quantitative discontinuity is based on increased innervation of cortical association areas to subcortical areas involved in speech production in great apes and humans (e.g., the hypoglossal nucleus). An interesting next step would be to evaluate whether such continuities/discontinuities are also found across other mammalian clades, with a particular focus on species capable of vocal learning. Recent research on the evolution of the cerebellum demonstrates that species capable of vocal learning exhibit significantly larger lateral cerebella than those that are not, suggestive of an evolutionary association between changes in cerebellar processing and cognition (Smaers et al., 2018).
Future work should continue attempting to match behavioural and brain data for a wide variety of species in a continued endeavour to investigate the evolutionary/comparative basis of behaviour. For example, cortical association areas may also be relevant in explaining other aspects of complex behaviour (e.g., deception, tool use, or self-control). Underlying this endeavour lies a continued effort to collect comparative neuroanatomical data. Data on the nucleus ambiguous (which is associated with the vagus nerve that innervates the larynx) would, for example, allow for a more direct test on the neural substrates of vocal production and the evolution of human speech (Fitch, 2011).
Author Contributions
JD and JS participated in the design of the study, collected data, carried out analyses, and wrote the paper.
Funding
JD was supported by the Isaac Newton Trust, the Cambridge Humanities Research Grant Scheme, and the Royal Society (Grant No. 180340). JS was supported by the Wenner Gren Foundation (Grant No. 9209).
Conflict of Interest Statement
The authors declare that the research was conducted in the absence of any commercial or financial relationships that could be construed as a potential conflict of interest.
Acknowledgments
We thank T. Fitch, S. Semple, D. Bowling, and the two reviewers for very helpful comments on a previous version of the manuscript.
Supplementary Material
The Supplementary Material for this article can be found online at: https://www.frontiersin.org/articles/10.3389/fnins.2018.00534/full#supplementary-material
References
Aiello, L., and Dunbar, R. (1993). Neocortex size, group size, and the evolution of language. Curr. Anthropol. 34, 184–193. doi: 10.1086/204160
Amiel, J. J., Tingley, R., and Shine, R. (2011). Smart moves: effects of relative Brain size on Establishment success of invasive amphibians and reptiles. PLOS One 6:e18277. doi: 10.1371/journal.pone.0018277
Arnold, C., Matthews, L. J., and Nunn, C. L. (2010). The 10kTrees website: a new online resource for primate phylogeny. Evol. Anthropol. 19, 114–118. doi: 10.1002/evan.20251
Barton, R. A. (1996). Neocortex size and behavioural ecology in primates. Proc. R. Soc. B Biol. Sci. 263, 173–177. doi: 10.1098/rspb.1996.0028
Barton, R. A. (1998). Visual specialization and brain evolution in primates. Proc. R. Soc. Lond. B 265, 1933–1937. doi: 10.1098/rspb.1998.0523
Barton, R. A. (2004). Binocularity and brain evolution in primates. Proc. Natl. Acad. Sci. U.S.A. 101, 10113–10115. doi: 10.1073/pnas.0401955101
Barton, R. A., Purvis, A., and Harvey, P. (1995). Evolutionary radiation of visual and olfactory brain systems in priamtes, bats and insectivores. Philos. Trans. R. Soc. Lond. B Biol. Sci. 348, 381–392. doi: 10.1098/rstb.1995.0076
Boë, L. J., Berthommier, F., Legou, T., Captier, G., Kemp, C., Sawallis, T. R., et al. (2017). Evidence of a vocalic proto-system in the baboon (Papio papio) suggests pre-hominin speech precursors. PLOS One 12:e0169321. doi: 10.1371/journal.pone.0169321
Chittka, L., and Niven, J. (2009). Are bigger brains better? Review. Curr. Biol. 19, R995–R1008. doi: 10.1016/j.cub.2009.08.023
Clutton-Brock, T. H., and Harvey, P. H. (1977). Primate ecology and social organization. J. Zool. 183, 1–39. doi: 10.1111/j.1469-7998.1977.tb04171.x
Darwin, C. (1871). The descent of man and selection in relation to sex. J. Anat. Physiol. 5(Pt 2), 363–372.
de Winter, W., and Oxnard, C. E. (2001). Evolutionary radiations and convergences in the structural organization of mammalian brains. Nature 409, 710–714. doi: 10.1038/35055547
Deacon, T. W. (1990). Rethinking mammalian brain evolution. Am. Zool. 30, 629–705. doi: 10.1093/icb/30.3.629
Deaner, R. O., Isler, K., Burkart, J., and van Schaik, C. (2007). Overall brain size, and not encephalization quotient, best predicts cognitive ability across non-human primates. Brain Behav. Evol. 70, 115–124. doi: 10.1159/000102973
Dechmann, D. K. N., and Safi, K. (2009). Comparative studies of brain evolution: a critical insight from the Chiroptera. Biol. Rev. 84, 161–172. doi: 10.1111/j.1469-185X.2008.00067.x
delBarco-Trillo, J., Sacha, C. R., Dubay, G. R., and Drea, C. M. (2012). Eulemur, me lemur: the evolution of scent-signal complexity in a primate clade. Philos. Trans. R. Soc. Lond. B Biol. Sci. 367, 1909–1922. doi: 10.1098/rstb.2011.0225
Desimone, R., and Schein, S. J. (1987). Visual properties of neurons in area V4 of the macaque: sensitivity to stimulus form. J. Neurophysiol. 57, 835–868. doi: 10.1152/jn.1987.57.3.835
Devoogd, T. J., Krebs, J. R., Healy, S. D., and Purvis, A. (1993). Relations between song repertoire size and the volume of brain nuclei related to song: comparative evolutionary analyses amongst oscine birds. Proc. Biol. Sci. 254, 75–82. doi: 10.1098/rspb.1993.0129
Dunbar, R. I. M. (1992). Neocortex size as a constraint size in primates on group ecologically. J. Hum. Evol. 20, 469–493. doi: 10.1016/0047-2484(92)90081-J
Dunbar, R. I. M. (2010). Coevolution of neocortical size, group size and language in humans. Behav. Brain Sci. 16, 681–694. doi: 10.1017/S0140525X00032325
Fitch, T., and Zuberbuhler, K. (2013). “Primate precursors to human language: beyond discontinuity,” in The Evolution of Emotional Communication, eds E. Zimmerman and E. Altenmüller (Oxford: Oxford University Press).
Fitch, W. (2006). “Production of vocalizations in mammals,” in Encyclopedia of Language and Linguistics, ed. K. Brown (Oxford: Elsevier).
Fitch, W. T. (2000). The evolution of speech: a comparative review. Trends Cogn. Sci. 6613, 258–267. doi: 10.1016/S1364-6613(00)01494-7
Fitch, W. T. (2010). The Evolution of Language. Cambridge: Cambridge University Press. doi: 10.1017/CBO9780511817779
Fitch, W. T. (2011). The evolution of syntax: an exaptationist perspective. Front. Evol. Neurosci. 3:9. doi: 10.3389/fnevo.2011.00009
Fitch, W. T., de Boer, B., Mathur, N., and Ghazanfar, A. A. (2016). Monkey vocal tracts are speech-ready. Sci. Adv. 2:e1600723. doi: 10.1126/sciadv.1600723
Freeberg, T. M. (2006). Social complexity can drive vocal complexity. Psychol. Sci. 17, 557–561. doi: 10.1111/j.1467-9280.2006.01743.x
Freeberg, T. M., Dunbar, R. I. M., and Ord, T. J. (2012). Social complexity as a proximate and ultimate factor in communicative complexity. Philos. Trans. R. Soc. Lond. B Biol. Sci. 367, 1785–1801. doi: 10.1098/rstb.2011.0213
Genovesio, A., Wise, S. P., and Passingham, R. E. (2014). Prefrontal-parietal function: from foraging to foresight. Trends Cogn. Sci. 18, 72–81. doi: 10.1016/j.tics.2013.11.007
Heldstab, S. A., Kosonen, Z. K., Koski, S. E., Burkart, J. M., van Schaik, C. P., and Isler, K. (2016). Manipulation complexity in primates coevolved with brain size and terrestriality. Sci. Rep. 6:24528. doi: 10.1038/srep24528
Iwatsubo, T., Kuzuhara, S., Kanemitsu, A., Shimada, H., and Toyokura, Y. (1990). Corticofugal projections to the motor nuclei of the brainstem and spinal cord in humans. Neurology 40, 309–312. doi: 10.1212/WNL.40.2.309
Jacobs, L. F. (1996). Sexual selection and the brain. Trends Ecol. Evol. 11, 82–86. doi: 10.1016/0169-5347(96)81048-2
Jacobs, L. F., Gaulin, S. J., Sherry, D. F., and Hoffman, G. E. (1990). Evolution of spatial cognition: sex-specific patterns of spatial behavior predict hippocampal size. Proc. Natl. Acad. Sci. U.S.A. 87, 6349–6352. doi: 10.1073/pnas.87.16.6349
Jürgens, U., and Alipour, M. (2002). A comparative study on the cortico-hypoglossal connections in primates, using biotin dextranamine. Neurosci. Lett. 328, 245–248. doi: 10.1016/S0304-3940(02)00525-6
Krams, I., Krama, T., Freeberg, T. M., Kullberg, C., and Lucas, J. R. (2012). Linking social complexity and vocal complexity: a parid perspective. Philos. Trans. R. Soc. Lond. B Biol. Sci. 367, 1879–1891. doi: 10.1098/rstb.2011.0222
Kuypers, H. (1958). Corticobulbar connections to the pons and lower brainstem in man: an anatomical study. Brain 81, 364–388. doi: 10.1093/brain/81.3.364
Lindenfors, P., Nunn, C. L., and Barton, R. A. (2007). Primate brain architecture and selection in relation to sex. BMC Biol. 5:20. doi: 10.1186/1741-7007-5-20
MacLean, E. L., Hare, B., Nunn, C. L., Addessi, E., Amici, F., Anderson, R. C., et al. (2014). The evolution of self-control. Proc. Natl. Acad. Sci. U.S.A. 111, E2140–E2148. doi: 10.1073/pnas.1323533111
McComb, K., and Semple, S. (2005). Coevolution of vocal communication and sociality in primates. Biol. Lett. 1, 381–385. doi: 10.1098/rsbl.2005.0366
Miller, E. K. (2000). The prefrontal cortex and cognitive control. Nat. Rev. Neurosci. 1, 59–65. doi: 10.1038/35036228
Miller, E. K., and Cohen, J. D. (2001). An integrative theory of prefrontal cortex function. Annu. Rev. Neurosci. 24, 167–202. doi: 10.1146/annurev.neuro.24.1.167
Mushiake, H., Saito, N., Sakamoto, K., Itoyama, Y., and Tanji, J. (2006). Activity in the lateral prefrontal cortex reflects multiple steps of future events in action plans. Neuron 50, 631–641. doi: 10.1016/j.neuron.2006.03.045
Nunn, C. L., and van Schaik, C. P. (2002). “A comparative approach to reconstructing the socioecology of extinct primates,” in Reconstructing Behavior in the Primate Fossil Record, eds J. M. Plavcan, R. F. Kay, W. L. Jungers, and C. P. van Schaik (New York, NY: Kluwer Academic), 159–215. doi: 10.1007/978-1-4615-1343-8_5
Pagel, M. (1999). Inferring the historical patterns of biological evolution. Nature 401, 877–884. doi: 10.1038/44766
Passingham, R. E., and Smaers, J. B. (2014). Is the prefrontal cortex especially enlarged in the human brain allometric relations and remapping factors. Brain Behav. Evol. 84, 156–166. doi: 10.1159/000365183
Passingham, R. E., Smaers, J. B., and Sherwood, C. C. (2016). “Evolutionary specializations of the human prefrontal cortex,” in Evolution of Nervous Systems, ed. J. Kaas (Cambridge, MA: Academic Press), 207–226.
Pearce, S. L., Miles, T. S., Thompson, P. D., and Nordstrom, M. A. (2003). Responses of single motor units in human masseter to transcranial magnetic stimulation of either hemisphere. J. Physiol. 549, 583–596. doi: 10.1113/jphysiol.2002.035352
Pollard, K. A., and Blumstein, D. T. (2012). Evolving communicative complexity: insights from rodents and beyond. Philos. Trans. R. Soc. Lond. B Biol. Sci. 367, 1869–1878. doi: 10.1098/rstb.2011.0221
Rao, C. R., and Toutenburg, H. (1999). Linear Models?: Least Squares and Alternatives. Berlin: Springer.
Rödel, R. M. W., Laskawi, R., and Markus, H. (2003). Tongue representation in the lateral cortical motor region of the human brain as assessed by transcranial magnetic stimulation. Ann. Otol. Rhinol. Laryngol. 112, 71–76. doi: 10.1177/000348940311200114
Rohlf, F. J., Methods, C., Methods, C., The, F. O. R., The, F. O. R., Of, A., et al. (2001). Comparative methods for the analysis of continuous variables: geometric interpretations. Evolution 55, 2143–2160. doi: 10.1111/j.0014-3820.2001.tb00731.x
Sherwood, C. C., Hof, P. R., Holloway, R. L., Semendeferi, K., Gannon, P. J., Frahm, H. D., et al. (2005). Evolution of the brainstem orofacial motor system in primates: a comparative study of trigeminal, facial, and hypoglossal nuclei. J. Hum. Evol. 48, 45–84. doi: 10.1016/j.jhevol.2004.10.003
Shima, K., Isoda, M., Mushiake, H., and Tanji, J. (2007). Categorization of behavioural sequences in the prefrontal cortex. Nature 445, 315–318. doi: 10.1038/nature05470
Simonyan, K., and Jürgens, U. (2003). Efferent subcortical projections of the laryngeal motorcortex in the rhesus monkey. Brain Res. 974, 43–59. doi: 10.1016/S0006-8993(03)02548-4
Smaers, J. B., Gómez-Robles, A., Parks, A. N., and Sherwood, C. C. (2017). Exceptional evolutionary expansion of prefrontal cortex in great apes and Humans. Curr. Biol. 27, 714–720. doi: 10.1016/j.cub.2017.01.020
Smaers, J. B., and Mongle, C. S. (2018). Evomap: R Package for the Evolutionary Mapping of Continuous Traits. San Francisco, CA: Github.
Smaers, J. B., and Rohlf, F. J. (2016). Testing species’ deviation from allometric predictions using the phylogenetic regression. Evolution 70, 1145–1149. doi: 10.1111/evo.12910
Smaers, J. B., Schleicher, A., Zilles, K., and Vinicius, L. (2010). Frontal white matter volume is associated with brain enlargement and higher structural connectivity in anthropoid primates. PLOS One 5:e9123. doi: 10.1371/journal.pone.0009123
Smaers, J. B., Steele, J., Case, C. R., and Amunts, K. (2013). Laterality and the evolution of the prefronto-cerebellar system in anthropoids. Ann. N. Y. Acad. Sci. 1288, 59–69. doi: 10.1111/nyas.12047
Smaers, J. B., Steele, J., Case, C. R., Cowper, A., Amunts, K., and Zilles, K. (2011). Primate prefrontal cortex evolution: human brains are the extreme of a lateralized ape trend. Brain. Behav. Evol. 77, 67–78. doi: 10.1159/000323671
Smaers, J. B., Turner, A. H., Gómez-Robles, A., and Sherwood, C. C. (2018). A cerebellar substrate for cognition evolved multiple times independently in mammals. eLife 7:e35696. doi: 10.7554/eLife.35696
Sokal, R. R., and Rohlf, F. J. (2012). Biometry: The Principles and Practice of Statistics in Biological Research. New York, NY: W.H. Freeman.
Sol, D., Bacher, S., Reader, S. M., and Lefebvre, L. (2008). Brain size predicts the success of mammal species introduced into novel environments. Am. Nat. 172(Suppl.), S63–S71. doi: 10.1086/588304
Sol, D., Duncan, R. P., Blackburn, T. M., Cassey, P., and Lefebvre, L. (2005). Big brains, enhanced cognition, and response of birds to novel environments. Proc. Natl. Acad. Sci. U.S.A. 102, 5460–5465. doi: 10.1073/pnas.0408145102
Stephan, H., Heiko, F., and Baron, G. (1981). New and revised data on volumes of brain structures in insectivores and primates. Folia Primatol. 35, 1–29. doi: 10.1159/000155963
White, D. J., Gersick, A. S., and Snyder-Mackler, N. (2012). Social networks and the development of social skills in cowbirds. Philos. Trans. R. Soc. Lond. B Biol. Sci. 367, 1892–1900. doi: 10.1098/rstb.2011.0223
Yamagata, T., Nakayama, Y., Tanji, J., and Hoshi, E. (2012). Distinct information representation and processing for goal-directed behavior in the dorsolateral and ventrolateral prefrontal cortex and the dorsal premotor cortex. J. Neurosci. 32, 12934–12949. doi: 10.1523/JNEUROSCI.2398-12.2012
Keywords: prefrontal cortex, cortical association areas, evolution of speech, vocal complexity, brain evolution, primate evolution, language, primates
Citation: Dunn JC and Smaers JB (2018) Neural Correlates of Vocal Repertoire in Primates. Front. Neurosci. 12:534. doi: 10.3389/fnins.2018.00534
Received: 27 April 2018; Accepted: 16 July 2018;
Published: 09 August 2018.
Edited by:
Robin Clark, University of Pennsylvania, United StatesReviewed by:
Andrea Ravignani, Max-Planck-Institut für Psycholinguistik, NetherlandsMarco Gamba, Università degli Studi di Torino, Italy
Copyright © 2018 Dunn and Smaers. This is an open-access article distributed under the terms of the Creative Commons Attribution License (CC BY). The use, distribution or reproduction in other forums is permitted, provided the original author(s) and the copyright owner(s) are credited and that the original publication in this journal is cited, in accordance with accepted academic practice. No use, distribution or reproduction is permitted which does not comply with these terms.
*Correspondence: Jacob C. Dunn, amFjb2IuZHVubkBhbmdsaWEuYWMudWs=; amNkNTRAY2FtLmFjLnVr